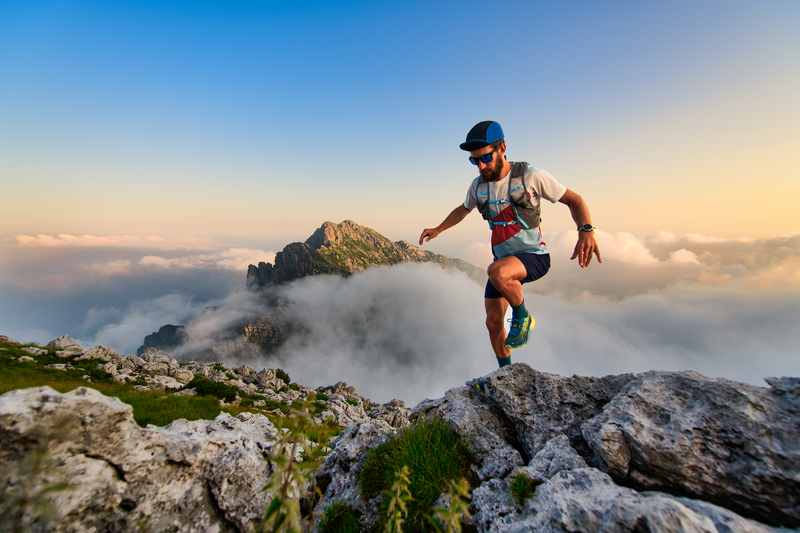
94% of researchers rate our articles as excellent or good
Learn more about the work of our research integrity team to safeguard the quality of each article we publish.
Find out more
ORIGINAL RESEARCH article
Front. Sustain. Food Syst. , 25 November 2022
Sec. Crop Biology and Sustainability
Volume 6 - 2022 | https://doi.org/10.3389/fsufs.2022.1017398
This article is part of the Research Topic Biotechnology for Agricultural Sustainability View all 7 articles
This study was carried out with the aim of determining the effects of jasmonic acid (JA) elicitation on the bioactive pigments' biosynthesis and the antioxidant activities in green callus of Azadirachta indica of two different ages (4- and 8-week-old). Plant tissue culture technique was employed to induce the formation of green callus from leaf explants of A. indica on Murashige and Skoog (MS) medium supplemented with 0.6 mg/L thidiazuron (CM) and three different concentrations of JA (2, 4, and 6 mg/L). The methanolic extracts from the green callus were used for determination of total chlorophyll content (TCh), total carotenoid content (TC), total anthocyanin content (TAC), total phenolic content (TPC), and total flavonoid content (TFC) through colorimetric and HPLC analyses. The highest amount of yield was obtained from CM and 2 mg/L JA (2JA) extracts for 4- and 8-week-old samples, respectively. Phytochemical screening revealed the presence of alkaloids, flavonoids, phenols, tannins, and terpenoids in all 4- and 8-week-old samples elicited with 2, 4 and 6 mg/L JA. The highest value for TAC, TCh, TC, TPC, and TFC of 4- and 8-week-old samples were from callus cultured on media supplemented with 6 mg/L JA (6JA) and 4 mg/L JA (4JA), respectively. The lowest IC50 values were found to be 8.29 ± 0.10 mg/mL (6JA) for 4-week-old and 7.73 ± 0.03 mg/mL (4JA) for 8-week-old samples. The highest Ferric Reducing Antioxidant Power (FRAP) values obtained in this study were 90.60 ± 1.55 g/g (6JA), and 74.59 ± 3.91 g/g (4JA), respectively, for 4- and 8-week-old samples. Moreover, Pearson's correlation analysis revealed a significant correlation between TAC, TCh, TC, TPC, and TFC with ABTS and FRAP assays. In addition, PCA analysis revealed that 83.5% of the information (variances) contained in the data were retained by the first two principal components. Overall, these findings suggested that JA supplementation into the culture media significantly increase the chlorophyll, carotenoid, anthocyanin, phenolic and flavonoid contents and JA concentrations at 6 mg/L JA and 4 mg/L JA yielded the highest pigments content in 4- and 8-weeks-old callus, respectively.
Fundamental life processes in plants are supported by the production of primary metabolites. These primary metabolites include carbohydrates, lipids, and amino acids. In addition, plants are also able to produce secondary metabolites which have been proven to have significant roles in their interaction with environmental stresses and pathogen attacks (Yang et al., 2018). In the industry, plant secondary metabolites are used in the production of drugs since they possess various pharmacological properties and mostly have been identified to be safe for medicinal purposes (Seca and Pinto, 2018). Neem (Azadirachta indica A. Juss), a member of the mahogany family, Meliaceae, used in this study is well-known for its antioxidants and pharmaceutical values (Alzohairy, 2016; Saleh Al-Hashemi and Hossain, 2016). Several studies have shown that secondary metabolites isolated from neem plants have the potential to be an anti-bacterial (Sarmiento et al., 2011; Quelemes et al., 2015), anti-cancer (Paul et al., 2011; Arumugam et al., 2014), as well as anti-diabetic agents (Dholi et al., 2011; Patil et al., 2013).
The usage of plant secondary metabolites in drug manufacturing despite current dependence on synthetic chemical drugs elevates the importance of plants in preventing and treating diseases. However, it is difficult to ensure a continuous supply of secondary metabolites from plant sources because the growth of plants in nature is highly influenced by exogenous factors such as environmental and climate changes (Gahukar, 2014). Moreover, most of the compounds produced through secondary metabolism of plants are often present in a very small amount, therefore, insufficient for testing a wide range of biological activities (Guerriero et al., 2018). Therefore, biotechnology approach is taken by introducing plant tissue culture as an alternative to help in producing and extracting valuable secondary metabolites with more reliable and simpler techniques, compared to extraction from complex whole plants (Karuppusamy, 2009; Hussain et al., 2012). Plant tissue culture technique has been widely employed in studies involving A. indica. The feasibility of this technique has contributed to the studies on the production of an important active constituent of A. indica, i.e., azadirachtin, conducted in vitro (Wewetzer, 1998; Srivastava and Chaturvedi, 2011; Ashokhan et al., 2020). Besides, better and greater production of plant secondary metabolites in vitro can be achieved by the addition of elicitors in the plant growth media (Ramakrishna and Ravishankar, 2011).
The classification of plant secondary metabolites can be made based on their chemical structures which divided them into three main groups, namely terpenoids, alkaloids, and phenolics (Hussein and El-Anssary, 2018). Terpenoids are the largest class of secondary metabolites which comprise of 40,000 different compounds that have therapeutic effects on different kind of diseases (Misawa, 2011). Classification of terpenoids is basically made depending on the number and structural organization of the five carbon isoprene units involved in their synthesis which includes C5 hemiterpenoids, C10 monoterpenoids, C15 sesquiterpenoids, C20 diterpenoids, C25 sesterterpenoids, C30 triterpenoids, C40 tetraterpenoids, and C>40 polyterpenoids (Abdallah and Quax, 2017). The next largest class of plant secondary metabolites, alkaloids, consist of ~12,000 low-molecular weight compounds (Ziegler and Facchini, 2008). The presence of a basic nitrogen atom at any position in the molecule becomes the basis in categorizing these compounds by excluding nitrogen in an amide or peptide bond (Ncube and Van Staden, 2015). Ecologically, alkaloids act as anti-feedants and toxins to pests by interfering with their nervous system in which they interact directly with molecular targets in the system (Kennedy and Wightman, 2011). The third largest class of secondary metabolites encompasses of phenolic compounds which is biosynthesized from precursors provided by glycolytic and pentose phosphate pathways to the shikimate pathway (Caretto et al., 2015).
In higher plants, phenolics compounds are believed to be involved in defense reaction against different types of stresses (Ahuja et al., 2010; Atkinson and Urwin, 2012; Suzuki et al., 2014; Nakabayashi and Saito, 2015). Structurally, phenolics have at least one aromatic ring with one or more hydroxyl groups which may further be esterified, methylated, etherified or glycosylated (Fresco et al., 2006). In addition to that, the grouping of phenolic compounds are also influenced by several other factors such as the nature and complexity of the basic carbonaceous skeleton, the degree of skeletal modification and the link between the base unit and other molecules, including primary and secondary metabolites (Ewané et al., 2012). These metabolites are diverse and some of them accumulate specifically depending on taxonomically related species but usually have similar roles in plant's interaction with the environment and immune mechanism against microbial pathogens for plant defense (Delgoda and Murray, 2017). Besides, numerous researches have been done to study the potential of these metabolites to be used as raw materials or active ingredients particularly in foods, cosmetics and medicine, due to their astounding biological activities (Clerici and Carvalho-Silva, 2011; Grof, 2018; Korkina et al., 2018; Seca and Pinto, 2018).
Studies have shown that compared to combined plant growth regulators (PGRs), media supplemented with thidiazuron (TDZ) was more effective in producing a high mass callus, while JA, among other phytohormones, plays a significant role in regulating secondary metabolites production in plant response to abiotic stresses through the crosstalk of JA with other phytohormones (Ashokhan et al., 2020; Wang et al., 2021). Therefore, this study utilizes plant tissue culture technique with TDZ as the PGR to induce the formation of callus from A. indica leaf explants in vitro and employs jasmonic acid (JA) in the growth medium as an abiotic elicitor to see its effect in increasing the production of secondary metabolites in the resulting green callus. These secondary metabolites are expected to accumulate, and their production is enhanced in response to stress. The objectives of the current study were to evaluate the effect of JA elicitation on the total amount of plant secondary metabolites (anthocyanins, chlorophylls, carotenoids, phenolics, and flavonoids), as well as the antioxidant potential in the elicited and non-elicited green callus of A. indica of two different ages (4- and 8-week-old).
A healthy A. indica plant was obtained from a nursery in Shah Alam, Selangor, Malaysia and used as the mother plant for subsequent tissue culture activities. Young and expanded leaves were excised and used as the explants to induce formation of callus from this species. The leaf explants were cultured on two types of media; CM and MSO. CM media consisted of 4.4 g of MS (Murashige and Skoog, 1962), 30 g/L of sucrose and 2 g/L of gelrite and supplemented with 0.6 mg/L TDZ, while MSO was similar to CM media but devoid of any plant growth regulators. The pH of both media (CM and MSO) were adjusted to 5.8 using 0.1 M NaOH or 0.1 M HCl (Ashokhan et al., 2018). The callus cultures were maintained in the culture room at 25 ± 2°C with a 16 h photoperiod and 1000 lux of light intensity. After 8 weeks of incubation, successfully developed green calli were subcultured onto JA-stress media (JSM), composed of CM added with different concentrations (0, 2, 4, and 6 mg/L) of jasmonic acid (Sigma chemical company St. Louis, Mo 63178 USA). The cultures were maintained in the culture room for another 4 weeks as well as 8 weeks before being harvested for subsequent analysis. Fresh weight of green callus developed on JSM media was recorded and the growth index (GI) of each sample for each treatment was calculated to determine the growth efficiency of the elicited and non-elicited A. indica green callus (Bhatia, 2015). The growth index (GI) was calculated using the formula described below (Sahraroo et al., 2014):
Green calli were harvested from CM and JSM media and subjected to freeze drying process using Labconco freeze dryer (Labconco Corporation, MO 64132 United States) at −50°C. About 2 g of freeze-dried green callus was ground on a chilled mortar and pestle together with trace amounts of MgCO3 and sand to enhance the pigment extraction process. Homogenized samples were then soaked in 60 mL of absolute methanol and incubated in the dark for 48 h at 4°C. After 48 h, the homogenates were filtered using a filter paper before being centrifuged at 8,000 rpm for 10 min, at 4°C (Universal 32 R centrifuge Hettich Zentrifugen, D-78532 Germany). The clear colored supernatants were transferred into new tubes. A portion of these supernatants were used instantly for subsequent pigment analysis to measure the total chlorophyll, carotenoid, and anthocyanin contents. Meanwhile, the remaining portion of the supernatants were concentrated under reduced pressure at 60°C using rotary evaporator (Rotavapor R-3 BÜCHI Labortechnik, 9230 Switzerland) to remove excess solvent. The resultant dried extracts were weighed and re-dissolved in absolute methanol to a concentration of 20 mg/mL and used for subsequent total phenolics, total flavonoids, phytochemical and antioxidants analysis (Ashokhan et al., 2018).
Standard methods were used to screen the presence of different types of bioactive pigments such as alkaloids, flavonoids, phenols, tannins, and terpenoids (Solihah et al., 2012).
The pH differential method was used to determine the amount of monomeric anthocyanin pigment (cyanidin-3-glucoside) of A. indica green callus (Giusti and Wrolstad, 2001). The pH of each extract was adjusted to pH 1 and pH 4.5 and the absorbance was taken in triplicate at 510 and 700 nm using a Multiskan Go plate reader (Thermo Scientific, Waltham, MA, USA). The total anthocyanin content was calculated and expressed in terms of mg/g DW. The following formula was used to calculate the concentrations of monomeric anthocyanin pigments in green callus of A. indica:
Multiskan Go plate reader (Thermo Scientific, Waltham, MA, USA) was used for the UV-VIS photometric determination of total chlorophyll contents (Ca and Cb) as well as total carotenoid content (C(x+c)). The absorbance for every wavelength involved was taken in triplicate. The chlorophyll and carotenoid contents were calculated using the formula as follows (Lichtenthaler and Buschmann, 2001):
Quantification of phenolic content was done using the method described by Yusof et al. (2018), but with several changes. About 20 μL of 20 mg/mL sample extract was mixed with 150 μL of diluted Folin-Ciocalteu reagent (FCR) and incubated for 10 min at room temperature. Prior to the mixture incubation, FCR was diluted 10-fold with deionized water. Then, 150 μL of 2% Na2CO3 was added to the mixture and incubated for another 45 min in the dark. Blank was prepared using 70% methanol and the absorbance was taken at 765 nm in triplicate using Multiskan Go plate reader (Thermo Scientific, Waltham, MA, USA). A standard curve was prepared using gallic as the standard with six different concentrations (0.01, 0.02, 0.03, 0.04, 0.05, 0.06 mg/mL). The TPC of the samples was expressed as mg of gallic acid equivalents/g dry weight (g GAE/g DW) of callus.
The total flavonoid content (TFC) in A. indica green callus was evaluated based on aluminium chloride colorimetric method (Yusof et al., 2018) with minor modifications. A total of 30 μL of 20 mg/mL sample extract was mixed with 90 μL of 70% methanol, 6 μL of 10% aluminium chloride hexahydrate, 6 μL of 1 M sodium acetate, and 168 μL of distilled water. The mixture was incubated for 40 min and the absorbance was taken at 415 nm in triplicate using Multiskan Go plate reader (Thermo Scientific, Waltham, MA, USA). A quercetin standard curve of concentrations ranging from 0.15 to 0.40 mg/mL was made to calculate the TFC of the samples and the results were expressed as quercetin equivalents in g per gram of dry weight (g QE/g DW) of callus.
A HPLC-based quantification of individual carotenoids was done according to the method described by Othman (2009). For every sample, 1 g of freeze-dried green callus was mixed with 1 g of calcium carbonate and then rehydrated in 1 mL of distilled water. The tissue was soaked in 5 mL of acetone:methanol at ratio 7:3 overnight at room temperature. The next day, the mixture was vortexed and centrifuged at 13,500 g for 2 min (Universal 32 R centrifuge Hettich Zentrifugen, D-78532 Germany). The supernatant was collected and transferred into foil-covered 50 mL graduated polypropylene centrifuge tubes. This procedure was repeated after adding in 5 mL of acetone:methanol of ratio 7:3 without addition of calcium carbonate until the sample became colorless. To remove any residual fine particulates, centrifugation of combined supernatants was repeated at 13,500 g for 5 min. Extraction of the carotenoids was done by adding 1:1 ratio of diethyl ether:distilled water to the sample mixture. After that, the solution was allowed to separate and the upper layer containing carotenoids was collected. By using diethyl ether alone, this procedure was repeated, and the combined upper phase was then dried using a vacuum concentrator (Thermo Scientific, Waltham, MA, USA) to remove adhering solvents. The vials were then immediately capped, sealed with parafilm, and stored at −80°C until subsequent analysis.
Quantification of individual carotenoids content was performed using Agilent 1200 series HPLC system (Agilent Technologies, USA) equipped with a diode array detector, as described by Othman (2009). Separation was attained using ZORBAX SB-C18 end capped column (5 μm, 4.6 × 250 mm) (Waters, Milford, MA, USA). The eluents used for mobile phase were (A) 9:1 v/v acetonitrile:water, and (B) ethyl acetate. The gradient program used was 0–40% solvent B (0–20 min), 40–60% Solvent B (20–25 min), 60–100% Solvent B (25–25.1 min), 100% Solvent B (25.1–35 min), and 100–0% Solvent B (35–35.1 min) and the flow rate was 1.0 mL/min. Prior to next sample injection, the column was allowed to re-equilibrate in 100% Solvent A for 10 min. The temperature of the column and injection volume were 20°C and 10 μL, respectively. Carotenoid peaks were detected between wavelengths ranging from 350 to 550 nm. The extracts were screened for eight types of carotenoid, namely α-carotene, β-carotene, lycopene, lutein, neoxanthin, violaxanthin, zeaxanthin, and β-cryptoxanthin. A standard curve was prepared for each carotenoid being quantified and the standards were diluted to five concentrations (0.02, 0.04, 0.06, 0.08, and 0.10 μg/μL). The concentration of the individual carotenoids was calculated as microgram per g dry weight (μg/g DW).
The extraction of flavonoids for HPLC analysis was done based on the method described by Seo et al. (2016) with minor modifications. The freeze-dried A. indica green callus of each treatment (2 g) was extracted with 20 mL of 80% ethanol at room temperature. After 3 days, the sample was filtrated. Ethanol was evaporated and freeze-dried to concentrate the extracts and then stored at −80°C. Crude extracts were redissolved in ethanol and filtered using a 0.2 μM membrane.
Quantification of individual flavonoids content was performed on Agilent Technologies 1100 series model HPLC system equipped with a Model ELSD 2000 detector (Waters, Milford, MA, USA) by following the method described by Seo et al. (2016). The column used was Agilent ZORBAX SB-C18 (5 μm, 4.6 × 250 mm) with mobile phase consisting of 100% acetonitrile. Sample was injected at a flow rate of 0.8 mL/min. The extracts were screened for six types flavonoids, namely rutin, kaempherol, kaempherol 3-glucoside, flavone, myricetin, and quercetin. Individual flavonoids concentration was calculated as microgram per g dry weight (μg/g DW).
Decolorization assay was used to determine the scavenging activity of ABTS free radical (Rajurkar and Hande, 2011). Initially, the solution of ABTS radical cation (ABTS.+) was prepared by mixing 10 mL of 2.45 mM potassium persulfate and 10 mL of 7 mM ABTS solution. Then, the mixture was incubated for 12 h in the dark at room temperature. After that, double distilled water (ddH20) was used to dilute the mixture until the absorbance produced is 0.70 ± 0.2 at 734 nm. Diluted ABTS.+ solution of 200 μL in volume was mixed with 20 μL of sample extract at six different concentrations and incubated for 30 min at room temperature. The absorbance was taken in triplicates at 734 nm. A non-linear regression (third-degree polynomial) was used to plot a graph of ABTS free radical scavenging activity percentage against concentration (Samad et al., 2016). Ascorbic acid was used as the positive control. The ABTS radical scavenging activity of the samples was calculated using the formula as follows:
FRAP assay of A. indica green callus extract was done following the standard protocol with slight changes (Benzie and Strain, 1999). FRAP reagent was prepared fresh during the assay by mixing 300 mM of acetate buffer (pH 3.6), 10 mM 2,4,6-tri(2-pyridyl)-s-triazine (TPTZ) solution and 20 mM iron (III) chloride hexahydrate (FeCl3.6H2O) at the ratio of 10:1:1, respectively. Then, FRAP reagent was incubated at 37°C in the water bath for 30 min. Ten milliliter of each sample extract was mixed with 300 mL of FRAP reagent and vortexed gently. To prepare the blank, sample extract was substituted with methanol. The mixture was incubated for another 30 min at room temperature. The absorbance was taken in triplicate at a wavelength of 593 nm. Ferrous sulfate of known concentration was used to construct a standard curve, and thus, the antioxidant activity will be shown as ferrous equivalent Fe (II) in gram per gram of dry weight of callus.
The experiments were conducted in a completely randomized block design with 15 replicates of three cultures for each treatment. Results obtained were presented as mean values ± standard error (SE) of triplicate measurements. The significant difference between the means was determined using one-way analysis of variance (ANOVA) and Duncan's multiple range test (DMRT) at p < 0.05, using IBM SPSS Statistics (version 24). Meanwhile, the principal component analysis (PCA) and correlations among antioxidant potential exhibited by the green callus extracts and bioactive pigments present in the callus were determined by Pearson's correlation analysis using R (version 4.2.0).
Callus induction on CM media which contained 0.6 mg/L of TDZ started after 7 days of incubation and leaf explants were observed to further develop into green compact calli. The growth and morphology of the callus were observed for 8 weeks (Figure 1). In contrast, the development of callus on MSO media was slower and there was no complete green callus formation noted from the leaf explants after 8 weeks, therefore, it could not be used for further analysis (Figure 1).
Figure 1. Callus induction and development observed on (A) MS medium supplemented with 0.6 mg/L of TDZ (CM media), and (B) MS medium without PGR (MSO) after 8 weeks.
The effects of sample ages and addition of different concentrations of JA into CM media were evaluated to better understand their influence on callus performance. Callus growth was monitored by measuring the callus fresh weight and growth index (GI), as shown in Table 1. The treatment with 2 mg/L JA was the most effective in enhancing callus regeneration capacity after 4 weeks of culture, with callus fresh weight and GI of 1.90 ± 0.18 g and 0.65 ± 0.02, respectively. Meanwhile, CM media produced the highest callus fresh weight (2.30 ± 0.35 g) and GI (0.74 ± 0.02) after 8 weeks of culture.
Table 1. Effect of TDZ and JA concentrations on callus fresh weight, growth index and yield of extracts.
A portion of the methanolic extracts were concentrated under reduced pressure. The yield of extracts gained from the concentration process were presented in Table 1. Concentrated extracts were then dissolved in methanol to a final concentration of 20 mg/mL and used for total phenolic, flavonoid, antioxidant, and phytochemical analysis. The methanolic extracts of A. indica green callus were also subjected to qualitative screening of phytochemical constituents by following the standard protocols described by Solihah et al. (2012). Results from the screening revealed the presence of alkaloids, flavonoids, phenols, tannins, and terpenoids in the methanolic extracts of A. indica green callus (Table 2).
Table 2. Qualitative screening of phytochemical constituents in green callus of A. indica methanolic extract.
The TAC, TPC, and TFC of the 4- and 8-week-old green callus were determined. TAC was expressed as mg per g dry weight of sample, while TPC was expressed as g Gallic Acid (GAE) per g dry weight of the sample, and TFC was expressed as g Quercetin (QE) per g dry weight of the sample. An increase in anthocyanins, phenolics, and flavonoids accumulation was noticed after elicitation with JA (for all concentrations) but the maximum accumulation was observed at two different time intervals; 4 and 8 weeks. Data analysis revealed that A. indica green callus cultured on CM added with 6 mg/L JA (6JA) and 4 mg/L JA (4JA) resulted in the highest amounts of TAC, TPC, and TFC after 4 and 8 weeks of culture. In general, the TAC, TPC, and TFC of methanolic extracts of 4-week-old A. indica green callus increased significantly with the increase in JA concentration (Figure 2). Green callus extract of 4-week-old 6JA sample showed the highest TAC, TPC, and TFC with compounds content of 0.31 ± 0.00 mg/g DW, 3.96 ± 0.02 g GAE/g DW, and 0.55 ± 0.03 g QE/g DW, respectively, followed by 4JA, 2JA, and CM (Figure 2). Nevertheless, the TAC, TPC, and TFC of the extracts of 8-week-old samples were found to decrease in CM media supplemented with high concentration of JA (6JA) (Figure 2). Interestingly, green callus extract from 8-week-old 4JA samples showed significantly highest values for TAC, TPC, and TFC which were 0.32 ± 0.01 mg/g DW, 3.40 ± 0.05 g GAE/g DW, and 0.52 ± 0.01 g QE/g DW, respectively, followed by 6JA, 2JA, and CM (Figure 2).
Figure 2. Effect of different concentration of jasmonic acid on (A) total anthocyanin, (B) phenolic, and (C) flavonoid contents in 4 and 8-week-old A. indica green callus. Data expressed as mean values ± standard error (SE) of three replicates. Treatment bars denoted with different letters (subject to samples' age) are significantly different at p ≤ 0.05 according to Duncan's multiple range test (DMRT), while * denotes significant difference between samples' age (at p < 0.05) according to Student's t-test. CM, callus induction media composed of 0.6 mg/L TDZ; TDZ, thidiazuron; JA, jasmonic acid.
The effect of sample ages on the TAC, TPC, and TFC of methanolic extracts of A. indica callus, with and without JA elicitation were also evaluated and compared. Generally, comparison between 4- and 8-week-old samples revealed that 4-week-old samples of CM, 2JA, 4JA, and 6JA had significantly greater amount of TAC, TPC, and TFC in comparison to 8-week-old samples of the same treatments, except for TAC and TFC of 4JA. The TAC and TFC of 8-week-old 4JA sample were higher by 0.07 mg/g DW and 0.09 g QE/g DW, respectively.
The total amount of photosynthetic pigments such as chlorophylls and carotenoids were measured and expressed as mg per g dry weight (mg/g DW) of callus. Based on Figures 3A,B, chlorophyll a and b contents in 4-week-old A. indica green callus increased as the concentration of JA increased. 6JA showed the highest amount of total chlorophylls (1.00 ± 0.03 mg/g DW) and carotenoids (0.13 ± 0.01 mg/g DW) contents for 4-week-old samples when compared to control (CM). For 8-week-old samples, 4JA green callus extract contained the highest amount of total carotenoids (0.10 ± 0.01 mg/g DW) compared to the control, followed by 6JA (0.09 ± 0.00 mg/g DW), 2JA (0.08 ± 0.00 mg/g DW), and CM (0.07 ± 0.00 mg/g DW) (Figure 3D). Generally, the amount of chlorophyll a is higher in all extracts compared to chlorophyll b for both 4- and 8-week-old samples.
Figure 3. Effect of different concentration of jasmonic acid on (A) chlorophyll a, (B) chlorophyll b, (C) total chlorophyll, (D) total carotenoid, (E) ratio of chlorophyll a to chlorophyll b, and (F) ratio of total chlorophyll to total carotenoid in 4 and 8-week-old A. indica green callus. Data expressed as mean values ± standard error (SE) of three replicates. Treatment bars denoted with different letters (subject to samples' age) are significantly different at p ≤ 0.05 according to Duncan's multiple range test (DMRT), while * denotes significant difference between samples' age (at p < 0.05) according to Student's t-test. CM, callus induction media composed of 0.6 mg/L TDZ; TDZ, thidiazuron; JA, jasmonic acid.
Figures 3E,F show the ratios of pigments content in methanolic extracts of A. indica. The weight ratio of chlorophyll a to chlorophyll b (Ca/Cb ratio) for 4 and 8-week-old extracts ranged between 1.52–1.66 and 1.32–1.58, respectively (Figure 3E). Other than that, the weight ratio of total chlorophylls to carotenoids (Ca + Cb/C(x+c) ratio) was determined to demonstrate the sample's greenness. Generally, the greenness of samples was about the same, as can be observed based on Ca + Cb/C(x+c) ratios obtained in this study. The ratios of 4-week-old samples ranged from 7.19 to 7.68, while the Ca + Cb/C(x+c) ratios for the 8-week-old samples ranged from 6.80 to 8.19 (Figure 3F).
In addition, the effects of sample ages on chlorophylls and carotenoid content of A. indica extracts were also compared. As shown in Figure 3, 4-week-old sample of all treatments exhibited significantly higher amount of chlorophyll a, chlorophyll b, total chlorophylls, as well as carotenoid contents compared to the extracts from 8-week-old samples. Longer incubation time was found to significantly affect the amount of total chlorophylls and carotenoids of the extracts. On the other hand, it was also observed that the total chlorophyll to total carotenoid ratio of 4-week-old samples decreased with increasing JA concentration, in contrast to 8-week-old samples (Figure 3F).
Previous data analysis revealed that 6JA and 4JA samples resulted in the highest amount of total carotenoid content in 4- and 8-week-old green callus, respectively. Thus, the samples were subjected to a HPLC analysis to determine and compare the presence of individual carotenoids in the samples compared to the control (CM). The extracts were screened for the presence of eight common carotenoids such as α-carotene, β-carotene, lycopene, lutein, neoxanthin, violaxanthin, zeaxanthin, and β-cryptoxanthin. Data analysis revealed the presence of four types of carotenoid in the samples, including β-carotene, lutein, neoxanthin, and violaxanthin. For 4-week-old callus extracts, CM was found to contain neoxanthin (0.93 ± 0.02 μg/g DW), violaxanthin (9.02 ± 0.61 μg/g DW), lutein (263.24 ± 4.96 μg/g DW), and β-carotene (2.59 ± 0.04 μg/g DW), however 6JA callus was found to contain only lutein (431.81 ± 1.60 μg/g DW) (Table 3). On the other hand, 8-week-old CM callus also showed the presence of only lutein (478.97 ± 4.59 μg/g DW), meanwhile 4JA callus extracts showed the absence of all eight carotenoids (Table 3).
Table 3. Distribution and amount of individual carotenoids present in 4 and 8-week-old green callus of A. indica.
Previous data analysis revealed that 6JA and 4JA samples resulted in the highest amount of TFC in 4- and 8-week-old green callus, respectively. Thus, the samples were subjected to a HPLC analysis to determine and compare the presence of individual flavonoids in the samples compared to the control (CM). Flavonoids detected include rutin, kaempherol, flavone, and myricetin (Table 4). According to the results, green callus extract of 4-week-old CM sample exhibited the highest amount of rutin (218.08 ± 18.08 μg/g DW) and flavone (5.30 ± 0.00 μg/g DW). On the other hand, 4-week-old 6JA extract showed significantly higher amount of kaempherol (14.45 ± 0.43 μg/g DW) and myricetin (45.32 ± 0.01 μg/g DW). For 8-week-old sample extracts, the amount of rutin (224.73 ± 1.94 μg/g DW) and kaempherol (4.20 ± 0.12 μg/g DW) were significantly higher in 4JA compared to CM. Interestingly, the amount of flavone and myricetin for 8-week-old CM and 4JA green callus extracts are similar, despite the fact that 4JA extract was elicited with JA (Table 4).
Table 4. Distribution and amount of individual flavonoids present in 4-week-old green callus of A. indica.
Antioxidant activities of all extracts against ABTS [2, 2-azino-bis (3-ethylbenzotiazoline-6-sulfonic acid)] radical and reducing ability of A. indica methanolic extracts by FRAP (ferric reducing antioxidant power) assay were depicted in Figure 4. The ABTS free radical scavenging activity of A. indica methanolic extracts were represented as IC50 (mg/mL) of each extract. The IC50 values defined the concentration of antioxidant needed to reduce the initial ABTS concentration by 50%. Meanwhile, FRAP reducing potential was expressed as g per g FeSO4 equivalent of each extract. Data analysis for 4-week-old samples revealed that the highest ABTS radical scavenging activity and FRAP reducing potential were shown by 6JA extract, with IC50 of 8.29 ± 0.10 mg/ml and FRAP value of 90.60 ± 1.55 g/g of sample, respectively (Figure 4). The ABTS IC50 values for 4-week-old sample extracts were found to decrease with increasing JA concentration. The same trend was observed for FRAP values of 4-week-old sample extracts. FRAP value of 2JA extract was slightly higher when compared to FRAP value of 4JA extract. However, all extracts of 4-week-old JA-treated samples showed higher FRAP values compared to control. On the other hand, extract of 8-week-old 4JA sample showed the highest ABTS radical scavenging potential and FRAP reducing activity, with ABTS IC50 of 7.73 ± 0.03 mg/ml and FRAP value of 74.59 ± 3.91 g/g of dried callus, respectively (Figure 4). Overall, elicitation with JA was found to yield higher antioxidant activity in the samples, as shown by the lower ABTS IC50 and higher FRAP values in JA-treated callus samples, compared to callus produced on CM media (control).
Figure 4. Effect of different concentration of jasmonic acid on (A) ABTS radical scavenging activity, and (B) FRAP reducing potential in 4 and 8-week-old A. indica green callus. Data expressed as mean values ± standard error (SE) of three replicates. Treatment bars denoted with different letters (subject to samples' age) are significantly different at p ≤ 0.05 according to Duncan's multiple range test (DMRT), while * denotes significant difference between samples' age (at p < 0.05) according to Student's t-test. CM, callus induction media composed of 0.6 mg/L TDZ; TDZ, thidiazuron; JA, jasmonic acid.
The effect of sample age on antioxidant potential of the extracts was also evaluated and compared (Figure 4). The scavenging activities of the 4-week-old sample extracts against ABTS radicals were found to be significantly higher than that shown by 8-week-old samples, except for 4JA. However, data analysis revealed that although the IC50 value of 4-week-old 4JA extract was higher than that of 8-week-old sample, the differences were not significant (p > 0.05). Therefore, it could be deduced that in general, extracts of 4-week-old samples had better ABTS radical scavenging activities than extracts of 8-week-old samples. Similarly, all 4-week-old samples also showed greater FRAP values compared to 8-week-old samples. Results revealed that shorter culture incubation period (4 weeks) resulted in production of callus with better FRAP (Fe3+ to Fe2+) reducing activities.
To comprehend the relationship between the JA concentration, samples age, antioxidant properties and bioactive pigments of green callus extracts of A. indica, a correlation analysis was conducted for both 4- and 8-week-old samples. Negative and significantly strong correlations were found between ABTS IC50 with TAC (r = −0.794), TPC (r = −0.696), and TFC (r = −0.766) of the extracts (Table 5). This show that the ABTS IC50 values would decrease with increasing TAC, TPC, and TFC amounts, indicating that ABTS radical scavenging activities would increase parallel to the increase in anthocyanin, phenolic and flavonoid contents in the samples. Positive and significantly strong correlations were also observed between FRAP values and the bioactive pigments (TAC, TPC, TFC, TC and TCh), with r values of 0.684, 0.834, 0.663, 0.764, and 0.718, respectively. These indicated that the ferric reducing potential of the extracts would increase with increasing pigments content (Table 5). JA concentration was also observed to possess significantly strong positive correlations with TAC (r = 0.819), TPC (r = 0.758), TFC (r = 0.857), and FRAP (r = 0.598), as well as significantly strong negative correlation with ABTS (r = −0.658). On the other hand, significantly strong negative correlations were observed between samples age with TPC (r = −0.554), TCh (r = −931), ChA (r = −0.927), ChB (r = −0.913), TC (r = −0.895), Ca/Cb ratio (r = −0.521), and FRAP (r = −0.601), implying that the contents of these bioactive pigments and ferric reducing potential would be significantly reduced with callus age (Table 5). These correlations can also be clearly visualized via the corrplot provided in Figure 5A.
Figure 5. Statistical evaluations between the parameters, (A) Corrplot describing the correlation coefficients between JA concentration, samples age, bioactive pigments and antioxidant properties, (B) Scree plot depicting the percentage of explained variances, (C) PCA-biplot summarizing the distribution of the tested extracts (from different storage period) on the factorial plan and representation of biological activities on the correlation circle, and (D) Heat map showing the relationship between JA concentrations with bioactive pigments and biological activities.
The data obtained in this study was also subjected to a PCA analysis, which revealed that 83.5% of the information (variances) contained in the data were retained by the first two principal components (as can be observed in the scree plot in Figure 5B). In addition, the findings obtained from the correlation analysis were also corroborated by the PCA findings, as observed from the angles between vectors in the PCA-biplot and heat map (Figures 5C,D).
No sign of callus formation was observed from the leaf explants when cultured on MSO, even after 8 weeks of incubation, due to the absence of TDZ as a growth regulator. Initiation of green callus formation from the leaf explants of A. indica can only be observed when the explants were cultured on CM media, which contained TDZ. Similar observations were recorded in a previous study where callus was successfully induced from different blueberry plant genotypes by utilizing TDZ as the only growth regulator in the basal media (BM) (Ghosh et al., 2017). Leaf explants of all genotypes started to develop into calli after 4 weeks of incubation on BM supplemented with different concentrations of TDZ (0.1, 0.5, and 1.0 mg/dm3) and fully formed calli after 12 weeks of culture. It was also reported that cultures cultivated on BM without TDZ did not form any callus and therefore, were excluded from data analysis (Ghosh et al., 2017).
In the present study, successfully developed calli on CM after 8 weeks of cultivation were subcultured on JSM. It appeared that the combination of TDZ with JA did not exert any significant effect on callus fresh weight and GI. Also, the application of JA as a stress factor resulted in no negative effects on the growth of A. indica green callus, thus it is suitable to be used as an elicitor to boost the production of bioactive pigments in the callus. In addition, the formation of green callus from A. indica leaf explants by using TDZ (0.2–0.6 mg/L) as growth regulator was also supported by findings of a previous study done by Ashokhan et al. (2018). The green callus was shown to have greater amount of bioactive pigments and antioxidant potential activities compared to brown and cream callus developed from media supplemented with 2,4-Dichlorophenoxyacetic acid. However, TDZ at higher concentrations (2.3–4.5 mg/L) was reported to induce brown callus in A. indica after 2 weeks of cultivation. The brown callus further developed into greenish globular embryos after a period of 2–3 weeks (Akula et al., 2003).
Besides, TDZ alone was apparently very efficient in stimulating biomass accumulation in A. indica callus cultures, which is in agreement with several reports on other medicinal plant species. Zhang et al. (2005) reported that callus formation of Scutellaria baicelensis, a Chinese medicinal plant, was well-induced by exogenous application of TDZ. Besides, higher concentrations of TDZ (1.0, 2.0, and 5.0 mg/L) generated multiple shoots from the induced calli but only after being subcultured on hormone free medium, whereas TDZ, at lower concentration (0.3 mg/L) produced shoots directly from calli on the induction medium. There was also a report on TDZ effects on stem, petiole, and leaf explants from 4-week-old Scutellaria bornmuelleri seedlings during callus formation induction. MS and half-strength MS media employed in the study were supplemented with 0.5, 1.0, 1.5, 2.0, 2.5, 3.0, and 4.0 mg/L of TDZ. Results revealed that, 4 days were needed for the stem and petiole explants to initiate callus formation but no callogenesis was observed from leaf explants. Moreover, the type of explant and medium used were shown to have different influences on the callus induction frequency. However, all stems were found to form callus within 2 weeks of culture, regardless of the medium used (Gharari et al., 2019).
In the present study, the total anthocyanin, phenolic, and flavonoid contents in A. indica green callus produced on CM, 2JA, 4JA, and 6JA media were determined. Compared to the control (CM media), the total anthocyanin, phenolic, and flavonoid contents were higher in callus grown on media elicited with JA. This finding is in agreement with a previous study, where low total anthocyanin, phenolic, and flavonoid contents were determined in A. indica green callus extract produced on MS media with TDZ but without the presence of any elicitor (Ashokhan et al., 2018). Besides, when compared to the control, the significant increase in the amount of bioactive pigments content when JA was added suggests that JA is a good stress-induced elicitor to enhance the biosynthesis of bioactive pigments in green callus of A. indica. Also, results obtained from the present study showed that the total anthocyanin, phenolic, and flavonoid increased significantly with the increase of JA concentrations for 4-week-old samples. However, the amount of TAC, TPC, and TFC of 8-week-old samples increased in 2JA and 4JA extracts only and then decreased in 6JA extract. Previous cell culture studies reported that, elicitation of bioactive pigments in plants was influenced by different parameters, for example, the elicitor specificity, concentration, and time of exposure (Vasconsuelo and Boland, 2007). Another study reported that even though plants respond to JA by altering their defense mechanisms as well as secondary metabolites production, the induced levels are commonly not stable after a long time (Kim et al., 2006). Therefore, treating sample with higher concentration of elicitor does not necessarily increase the amount of accumulated secondary metabolites. It was shown that treating plants with different JA concentration can be done as a mean to select for an optimum concentration of JA that could induce the maximum amount of bioactive compounds (Kim et al., 2007). Jasmonates such as JA and its methyl ester, methyl jasmonate (MeJA) were reported to increase the accumulation of phenolics in Mentha × piperita. However, the observed changes were different depending on elicitor's concentrations and the time of exposure (Krzyzanowska et al., 2011). Other than that, the increase in secondary metabolites under the influence of JA in the present study can be explained by studies conducted by Verma et al. (2016) and Zhu (2016). These studies reported that plant growth, development, and environmental responses are controlled by plant hormonal signaling pathways which require complex hormonal crosstalk such as between JA and other hormones (Kazan and Manners, 2008; Santner and Estelle, 2009; Wasternack and Strnad, 2016). A previous study had reported that under abiotic and biotic conditions, the interaction between JA and other hormone, particularly indole-3-acetic-acid (IAA) in plants, controlled the physiological processes including the production of secondary metabolites, coiling of tendril and cell elongation (Saniewski et al., 2002). Based on the results, the total flavonoid content in each treatment was found to be the highest compared to total anthocyanins and phenolics. Therefore, HPLC analysis was done to determine the individual flavonoids present in JA-treated samples that showed the highest TFC amounts, compared to control sample (CM). Flavonoid analysis performed by HPLC system detected four flavonoid peaks which were rutin, kaempherol, flavone, and myricetin. All compounds were scanned using three different wavelengths ranging from 250 to 350 nm to increase the efficiency of peak separation, hence avoiding the peak from overlapping (Seo et al., 2016). The major flavonoid constituent in A. indica green callus was found to be rutin. According to the study done by Vergallo et al. (2019), the qualitative-quantitative composition of flavonoids depends on the extraction solvent used which means different organic solvents may have different efficiency in extracting a particular group of flavonoids. In the present study, acetonitrile was shown to have better efficiency in extracting rutin, hence it was found to be the highest compound screened, followed by myricetin, kaempherol, and flavone. Meanwhile in another study, the ethanolic extracts obtained from leaves and bark of A. indica were shown to contain several phenolic and flavonoid compounds. Major compounds found in the extracts were quercetin (14.09 ± 0.01 μg/mL), kaempherol (8.15 ± 0.03 μg/mL), and coumarin (7.48 ± 0.01 μg/mL) while other compounds detected included gallic acid (2.65 ± 0.01 μg/mL), catechin (1.79 ± 0.03 μg/mL), chlorogenic acid (1.81 ± 0.01 μg/mL), rutin (3.72 ± 0.02 μg/mL), quercitrin (7.46 ± 0.01 μg/mL), and luteolin (3.74 ± 0.01 μg/mL) (Cristo et al., 2016). In another study, two flavonoids extracted from A. indica leaves, quercetin and kaempherol, were successfully characterized and isolated by HPLC and Preparative-HPLC (P-HPLC), respectively, and were shown to have potential antimicrobial properties as they exhibited valuable anti-MRSA activity (Ullah et al., 2018).
Morever, the supplementation of JA at different concentrations also influenced the regulation of photosynthetic pigments (chlorophylls and carotenoids) production in green callus of A. indica. Similar to TAC, TPC, and TFC analysis, the highest amount of chlorophylls and carotenoids were exhibited by the extracts of 4-week-old 6JA and 8-week-old 4JA samples, respectively. Thus, at this juncture, it could be deduced that 6 and 4 mg/L were the optimum concentrations for JA to elicit bioactive pigments production in the present study, depending on the duration of culture incubation (or callus age). Results showed that the amount of photosynthetic pigments of 8-week-old samples was significantly lower compared to 4-week-old samples. Most of the studies done on the effects of JA on plants showed that JA exerted inhibitory effect on the growth and photosynthetic pigments content of the plant samples grown in vitro and in vivo (Ueda et al., 1981; Kovač and Ravnikar, 1994, 1998; Golovatskaya and Karnachuk, 2008). A study had demonstrated that JA and MeJA strongly inhibited the accumulation of chlorophylls as time increased and the inhibitory effect of MeJA was greater than JA (Ananiev et al., 2004). Another previous study had reported that the negative effect of JA on plant pigments could be explained by the degradation of chloroplast proteins and pigment–protein complexes in chloroplasts (Kumari and Sudhakar, 2003). These observations suggested that JA concentration may increase or decrease the amount of photosynthetic pigments of plant samples treated and most of the time JA exhibited an inhibitory effect that reduce the photosynthetic pigment amount as time increases.
Besides, both 4- and 8-week-old controls (CM) were observed to have lower photosynthetic pigments amount than other respective treatments. Therefore, JA is a potent elicitor in inducing the biosynthesis of photosynthetic pigments in plants though the induced levels was commonly unstable for a long time (Kim et al., 2007). Elicitation of Lactuca sativa L. with JA in one study was proven to significantly increase the amount of carotenoids in the plant compared to the control. Interestingly, CM extract from 8-week-old callus had lower amount of total chlorophylls and carotenoids than 4-week-old callus, although both of the samples were not subjected to JA-stress elicitation. This implied that callus subjected to a longer culture period experienced greater deterioration of total chlorophylls and carotenoids, due to limited access to macronutrients. Chlorophylls and carotenoids are photosynthetic apparatus involved in photosynthesis, which is the key process in plant metabolism. Deprivation of macronutrients hinders plant metabolism and affects plant growth and development (Thuynsma et al., 2016; Samborska et al., 2018; Sitko et al., 2019).
The samples were also screened for the presence of eight major carotenoids using HPLC. In this part of the study, the extract from 4-week-old CM sample was used for optimization and was found to show good separation of compounds, thus the same protocol was used for all other samples. In contrast to the results gathered for 4-week-old CM samples, the extract of 4-week-old 6JA samples were found to contain only lutein. Meanwhile for 8-week-old samples, only lutein was detected in CM extract, and none was detected in the extract of 4JA sample. This is the first time where changes in lutein production during treatment with JA was observed. Nevertheless, there may be other unknown carotenoids present in the samples, but they could not be identified due to the presence of unresolved peaks in the HPLC chromatograms. According to Dworkin (2011), compounds do not split up well chromatographically due to the occurrence of chromatographic co-elution which happens when compounds involved have retention times that differ by less than the resolution of the method. Besides, degradation of column used and mass or volume overload on the column may also lead to peak distortion (Ahmad, 2017). Another possible cause of peak distortion is incompatibility of diluent and mobile phase where the elution strength of mobile phase used is significantly weaker than the diluent or there is other possible immiscibility issues (Wahab et al., 2017).
The ratio of chlorophyll a to chlorophyll b (Ca/Cb) and total chlorophylls to total carotenoids Ca+Cb/C(x+c) were measured in 4- and 8-week-old samples. Based on the results obtained, 8-week-old green callus samples showed lower Ca/Cb ratio as compared to 4-week-old green callus samples. In all sample extracts, chlorophyll a content is greater than chlorophyll b and Ca/Cb ratio is also higher compared to previous findings by Ashokhan et al. (2018). This is because when a plant is under stress, both chlorophyll a and b decreased, while the Ca/Cb ratio tend to increase due to greater reduction in chlorophyll b compared to chlorophyll a. In any situation, plants have more chlorophyll a than b because the maintenance of more chlorophyll a than b is important for survival. Therefore, under the influence of a stress factor, chlorophyll b may be converted into chlorophyll a during the chlorophyll degradation process that lead to the increase in chlorophyll a contents (Fang et al., 1998; Eckardt, 2009; Ashraf and Harris, 2013). A previous study using two types of potato plants grown in vitro under the influence of JA also showed higher content of chlorophyll a than b. In JA-treated leaves of potato cv. Sante, the amount of chlorophyll a and b were 2.92 ± 0.16 μg/mg DW and 1.17 ± 0.05 μg/mg DW, respectively. Meanwhile, the amount of chlorophyll a and b were reported to be 6.25 ± 0.45 μg/mg DW and 2.39 ± 0.14 μg/mg DW, respectively, for JA-treated leaves of potato cv. Ulster (Kovač and Ravnikar, 1994). Also, fennel plants treated with 50 μM MeJA, showed higher amount of chlorophyll a (3.25 ± 0.41 mg/g FW) than chlorophyll b (1.10 ± 0.17 mg/g FW) (Parmoon et al., 2018).
Other than that, the ratio of chlorophylls a and b to total carotenoid, Ca+Cb/C(x+c) was also determined in the present study to demonstrate the greenness of the samples (Lichtenthaler and Buschmann, 2001). The Ca+Cb/C(x+c) ratio values obtained in this study was lower than the Ca+Cb/C(x+c) ratio reported in the study done by Ashokhan et al. (2018). It was reported that greater degradation of chlorophylls and carotenoid would occur due to stress, senescence, and damage of the plant photosynthetic apparatus, thus resulting in lower Ca+Cb/C(x+c) ratio values (Lichtenthaler and Buschmann, 2001). Other than that, the development of chromoplast also may decrease Ca+Cb/C(x+c) ratio, which can be observed through the change in the plant color (Giusti and Wrolstad, 2001).
The chemical reaction between radicals and antioxidant compounds are different depending on the mechanism of actions employed by the molecules involved. Hence, a number of assays have been developed to measure the free radicals scavenging activity of the available antioxidants appropriately (Marathe et al., 2011). Developed methods are made based on hydrogen atom transfer (HAT) and also electron transfer (ET). In HAT methods, the extent of the radical scavenging activity caused by hydrogen or electron donation is measured. Meanwhile, ET methods employed an antioxidant system during radical generation where when antioxidants reacts with the chromogenic reagent, and the absorbance will increase at a prespecified wavelength (Moharram and Youssef, 2014). ABTS and FRAP assays are one of available HAT and ET methods, respectively. Therefore, to increase the accuracy, both ABTS and FRAP assays were used in this study to measure the antioxidant capacity of the plant extracts.
A recent study had revealed the effect of two different concentrations of an elicitor which was analyzed on day 3, 7, and 15 post-elicitation in shoot cultures of Knautia sarajevensis. The highest antioxidant activity was exhibited by 50 and 100 mM elicitor-treated extract harvested on day 7 and 3, respectively, while the lowest antioxidant activity was exhibited by both 50 and 100 mM elicitor-treated extracts harvested on day 15 (Karalija et al., 2019). Another antioxidant potential study using lettuce and endive showed that, 1 μM of JA-treated samples had the highest antioxidant activity after being stored for 4 days while 100 μM of JA-treated samples had the highest antioxidant activity for lettuce and endive after being stored for 0 and 2 days, respectively (Złotek, 2017). In addition, cultures of A. indica elicited with JA exhibited greater antioxidant potential as compared to other parts of the plants such as the flowers and leaves (Sithisarn et al., 2005; Nahak and Sahu, 2011).
Higher IC50 value were shown by all 8-week-old sample extracts, indicating a lower antioxidant activity than 4-weeek-old sample extracts. Similarly, 8-week-old sample also had lower FRAP values compared to 4-week-old sample extracts. Therefore, it could be deduced that increased duration of elicitor exposure (JA) reduced the antioxidant activity of the extracts. Moreover, this observation is supported by a study done using MeJA, salicyclic acid and combination of both as elicitors, where the antioxidant activity of some cell suspension cultures of Thevetia peruviana were evaluated at 24, 48, 72, 96, and 120 h post-elicitation. The results showed that the antioxidant activity of samples treated with MeJA increased from 48 to 96 h post elicitation but decreased after 120 h post elicitation (Mendoza et al., 2018). One previous study showed that, antioxidant activity of MeJA-treated Lactuca sativa var. capitata increased when evaluated on pre-harvest day 1, 3, 5, and 7 but decreased on day 15 (Moreno-Escamilla et al., 2017).
In the present study, it was observed that both ABTS radical scavenging and FRAP reducing activity of the green callus extracts would increase with increased amounts of bioactive pigments. Previously, it was reported that bioactive phytochemicals present in the plants will influence its antioxidant activities (Hsu et al., 2006). Strong and significant correlation between anthocyanins and antioxidants activities from the results is supported by a previous study where, anthocyanins were found to be major active components that contributed to high antioxidant activity in Elatostema rugosum (Neill et al., 2002). Phenolics are known as good hydrogen-donating antioxidants because they possess hydroxyl groups in their structures (Payá et al., 1992; Heim et al., 2002). Moreover, previous studies using extract from neem leaves suggested that phenolic compounds are excellent free radical scavengers and reducing agents because there were strong correlations between phenolic content and antioxidant activities in the extract (Pandey et al., 2014; Dhakal et al., 2016). Meanwhile, flavonoids' antioxidant potential enable them to protect against oxidative diseases, activate or inhibit various enzymes from binding to specific receptors, and protect against cardiovascular diseases by reducing the oxidation of low-density lipoproteins (Aron and Kennedy, 2008). Chlorophylls and carotenoids that were present in the methanolic extracts of A. indica were also observed to be correlated to their ABTS and FRAP activities. Carotenoids such as lutein, lycopene, β-carotene, and zeaxanthin are well-known as free radical scavengers and strong antioxidants, particularly vital in human diets (Johnson, 2002). Meanwhile, chlorophylls were reported to be responsible for breaking the chain reaction in cellular oxidation caused by free radicals by acting as the hydrogen donor (Endo et al., 1985).
The present study showed that different callus ages (4 and 8 weeks) and concentrations of JA (2–6 mg/L) influenced the production of bioactive pigments and antioxidant activities in neem callus. At 4-week-old, 6 mg/L of JA was found to be the optimum concentration to trigger the highest production of anthocyanins, phenolics, flavonoids, chlorophylls, and carotenoids while 4 mg/L of JA was the optimum concentration for 8-week-old samples. Parallel to the production of bioactive pigments, 6 and 4 mg/L were shown to be the optimum JA concentrations that resulted in better antioxidant activities. Hence, the findings of the present study revealed that depending on JA concentration and callus age, exogenous application of JA into the culture medium increased the production of bioactive pigments which was responsible for the antioxidant activities in green callus of A. indica.
The original contributions presented in the study are included in the article/supplementary material, further inquiries can be directed to the corresponding author.
JSY and NAM conceived and designed the experiments. NSAF and RO performed the experiments. NSAF, MHAR, RO, and JSY analyzed the data. JSY, NAM, RO, and MHAR contributed reagents/materials/analysis tools. NSAF wrote the first draft of the article. JSY and MHAR revised the article. All authors contributed to the article and approved the submitted version.
The authors thank Universiti Malaya, Malaysia (Grant No. ST009-2021) and the Ministry of Higher Education, Malaysia (FRGS Grant No. FP055-2017A, with reference code: FRGS/1/2017/WAB01/UM/02/2 as well as FRGS Grant No. 01-01-20-2323FR, with reference code: FRGS/1/2020/STG01/UPM/02/2) and for the financial support.
The authors also thank Universiti Malaya (UM), Malaysia, for the experimental facilities, and Ms. Radziah from Nanocat, UM for her technical assistance in operating the HPLC.
The authors declare that the research was conducted in the absence of any commercial or financial relationships that could be construed as a potential conflict of interest.
All claims expressed in this article are solely those of the authors and do not necessarily represent those of their affiliated organizations, or those of the publisher, the editors and the reviewers. Any product that may be evaluated in this article, or claim that may be made by its manufacturer, is not guaranteed or endorsed by the publisher.
Abdallah, I. I., and Quax, W. J. (2017). A glimpse into the biosynthesis of terpenoids. NRLS Confer. Proc. 2017, 81–98. doi: 10.18502/kls.v3i5.981
Ahmad, I. A. H. (2017). Necessary analytical skills and knowledge for identifying, understanding, and performing HPLC troubleshooting. Chromatographia 80, 705–730. doi: 10.1007/s10337-016-3225-7
Ahuja, I., de Vos, R. C. H., Bones, A. M., and Hall, R. D. (2010). Plant molecular stress responses face climate change. Trends Plant Sci. 15, 664–674. doi: 10.1016/j.tplants.2010.08.002
Akula, C., Akula, A., and Drew, R. (2003). Somatic embryogenesis in clonal neem, Azadirachta indica A. Juss. and analysis for in vitro azadirachtin production. In Vitro Cell Dev. Biol. Plant 39:304–310 doi: 10.1079/IVP2003415
Alzohairy, M. A. (2016). Therapeutics role of Azadirachta indica (neem) and their active constituents in diseases prevention and treatment. Evid. Based Complement. Alternative Med. ECAM 2016, 7382506. doi: 10.1155/2016/7382506
Ananiev, E. D., Ananieva, K. T., and Todorov, I. (2004). Effect of methyl ester of jasmonic acid, abscisic acid and benzyladenine on chlorophyll synthesis in excised cotyledons of Cucurbita pepo (zucchini). Bulgarian J. Plant Physiol. 30, 51–63.
Aron, P. M., and Kennedy, J. A. (2008). Flavan-3-ols: Nature, occurrence and biological activity. Mol. Nutr. Food Res. 52, 79–104. doi: 10.1002/mnfr.200700137
Arumugam, A., Agullo, P., Boopalan, T., Nandy, S., Lopez, R., Gutierrez, C., et al. (2014). Neem leaf extract inhibits mammary carcinogenesis by altering cell proliferation, apoptosis, and angiogenesis. Cancer Biol. Ther. 15, 26–34. doi: 10.4161/cbt.26604
Ashokhan, S., Othman, R., Abd Rahim, M. H., Karsani, S. A., and Yaacob, J. S. (2020). Effect of plant growth regulators on coloured callus formation and accumulation of Azadirachtin, an essential biopesticide in Azadirachta indica. Plants 9, 352. doi: 10.3390/plants9030352
Ashokhan, S., Ramasamy, S., Karsani, S. A., Othman, R., and Yaacob, J. S. (2018). Analysis of bioactive pigments in coloured callus of Azadirachta indica for possible use as functional natural colourants. Pigment Resin Technol. 48, 9–19. doi: 10.1108/PRT-11-2017-0095
Ashraf, M., and Harris, P. J. C. (2013). Photosynthesis under stressful environments: an overview. Photosynthetica 51, 163–190. doi: 10.1007/s11099-013-0021-6
Atkinson, N. J., and Urwin, P. E. (2012). The interaction of plant biotic and abiotic stresses: from genes to the field. J. Exp. Botany 63, 3523–3543. doi: 10.1093/jxb/ers100
Benzie, I. F., and Strain, J. J. (1999). Ferric reducing/antioxidant power assay: direct measure of total antioxidant activity of biological fluids and modified version for simultaneous measurement of total antioxidant power and ascorbic acid concentration. Methods Enzymol. 299, 15–27. doi: 10.1016/S0076-6879(99)99005-5
Bhatia, S. (2015). “Chapter 2-Plant tissue culture,” in Modern Applications of Plant Biotechnology in Pharmaceutical Sciences, eds S. Bhatia, K. Sharma, R. Dahiya, and T. Bera (Academic Press), 31–107. Available online at: http://www.sciencedirect.com/science/article/pii/B9780128022214000029
Caretto, S., Linsalata, V., Colella, G., Mita, G., and Lattanzio, V. (2015). Carbon fluxes between primary metabolism and phenolic pathway in plant tissues under stress. Int. J. Mol. Sci. 16, 26378–26394. doi: 10.3390/ijms161125967
Clerici, M. T. P. S., and Carvalho-Silva, L. B. (2011). Nutritional bioactive compounds and technological aspects of minor fruits grown in Brazil. Food Res. Int. 44, 1658–1670. doi: 10.1016/j.foodres.2011.04.020
Cristo, J. S., Matias, E. F. F., Figueredo, F. G., Santos, J. F. S., Pereira, N. L. F., Junior, J. G. A. S., et al. (2016). HPLC profile and antibiotic-modifying activity of Azadirachta indica A. Juss, (Meliaceae). Indus. Crops Products 94, 903–908. doi: 10.1016/j.indcrop.2016.10.001
Delgoda, R., and Murray, J. E. (2017). “Chapter 7-Evolutionary perspectives on the role of plant secondary metabolites,” in Pharmacognosy, eds S. Badal and R. Delgoda (Academic Press), 93–100. Available online at: http://www.sciencedirect.com/science/article/pii/B978012802104000007X
Dhakal, S., Aryal, P., Aryal, S., Basyal, D., and Khadka, D. (2016). Phytochemical and antioxidant studies of methanol and chloroform extract from leaves of Azadirachta indica A. Juss. In Tropical region of Nepal. J. Pharm. Phytother. 8, 203–208. doi: 10.5897/JPP2016.0425
Dholi, S., Raparla, R., Mankala, S., and Nagappan, K. (2011). In vivo antidiabetic evaluation of neem leaf extract in alloxan induced rats. J. Appl. Pharm. Sci. 1, 100–105.
Dworkin, J. P. (2011). “Chromatographic co-elution,” in Encyclopedia of Astrobiology, eds M. Gargaud, R. Amils, J. C. Quintanilla, H. J. (Jim) Cleaves, W. M. Irvine, D. L. Pinti, et al. (Berlin: Springer), 302–302.
Eckardt, N. A. (2009). A new chlorophyll degradation pathway. Plant Cell 21, 700. doi: 10.1105/tpc.109.210313
Endo, Y., Usuki, R., and Kaneda, T. (1985). Antioxidant effects of chlorophyll and pheophytin on the autoxidation of oils in the dark. II. The mechanism of antioxidative action of chlorophyll. J. Am. Oil Chemists' Soc. 62, 1387–1390. doi: 10.1007/BF02545965
Ewané, C. A., Lepoivre, P., de Bellaire, L., de, L., and Lassois, L. (2012). Involvement of Phenolic Compounds in the Susceptibility of Bananas to Crown Rot. A Review. BASE. Available online at: https://popups.uliege.be:443/1780-4507/index.php?id=8996
Fang, Z., Bouwkamp, J. C., and Solomos, T. (1998). Chlorophyllase activities and chlorophyll degradation during leaf senescence in non-yellowing mutant and wild type of Phaseolus vulgaris L. J. Exp. Botany 49, 503–510. doi: 10.1093/jxb/49.320.503
Fresco, P., Borges, F., Diniz, C., and Marques, M. P. M. (2006). New insights on the anticancer properties of dietary polyphenols. Medicinal Res. Rev. 26, 747–766. doi: 10.1002/med.20060
Gahukar, R. T. (2014). Factors affecting content and bioefficacy of neem (Azadirachta indica A. Juss.) phytochemicals used in agricultural pest control: a review. Crop Protection 62, 93–99. doi: 10.1016/j.cropro.2014.04.014
Gharari, Z., Bagheri, K., Sharafi, A., and Danafar, H. (2019). Thidiazuron induced efficient in vitro organogenesis and regeneration of Scutellaria bornmuelleri: an important medicinal plant. In Vitro Cell. Dev. Biol. Plant 55, 133–138. doi: 10.1007/s11627-019-09965-7
Ghosh, A., Igamberdiev, A. U., and Debnath, S. C. (2017). Detection of DNA methylation pattern in thidiazuron-induced blueberry callus using methylation-sensitive amplification polymorphism. Biologia Plantarum. 61, 511–519. doi: 10.1007/s10535-016-0678-3
Giusti, M. M., and Wrolstad, R. E. (2001). “Characterization and measurement of anthocyanins by UV-visible spectroscopy,” in Current Protocols in Food Analytical Chemistry, ed R. E. Wrolstad (New York, NY: John Wiley & Sons, Inc), F1.2.1–F1.2.13.
Golovatskaya, I. F., and Karnachuk, R. A. (2008). Effect of jasmonic acid on morphogenesis and photosynthetic pigment level in Arabidopsis seedlings grown under green light. Russian J. Plant Physiol. 55, 220–224. doi: 10.1134/S1021443708020088
Grof, C. P. L. (2018). Cannabis, from plant to pill. Br. J. Clin. Pharmacol. 84, 2463–2467. doi: 10.1111/bcp.13618
Guerriero, G., Berni, R., Muñoz-Sanchez, J., Apone, F., Abdel-Salam, E., Qahtan, A., et al. (2018). Production of plant secondary metabolites: examples, tips and suggestions for biotechnologists. Genes 9, 309–331. doi: 10.3390/genes9060309
Heim, K. E., Tagliaferro, A. R., and Bobilya, D. J. (2002). Flavonoid antioxidants: chemistry, metabolism and structure-activity relationships. J. Nutr. Biochem. 13, 572–584. doi: 10.1016/S0955-2863(02)00208-5
Hsu, B., Coupar, I. M., and Ng, K. (2006). Antioxidant activity of hot water extract from the fruit of the Doum palm, Hyphaene thebaica. Food Chem. 98, 317–328. doi: 10.1016/j.foodchem.2005.05.077
Hussain, Md. S., Fareed, S., Ansari, S., Rahman, Md. A., Ahmad, I. Z., and Saeed, M. (2012). Current approaches toward production of secondary plant metabolites. J. Pharm. Bioallied Sci. 4, 10–20. doi: 10.4103/0975-7406.92725
Hussein, R. A., and El-Anssary, A. A. (2018). “Plants secondary metabolites: the key drivers of the pharmacological actions of medicinal plants,” in Herbal Medicine. Available online at: https://www.intechopen.com/books/herbal-medicine/plants-secondary-metabolites-the-key-drivers-of-the-pharmacological-actions-of-medicinal-plants
Johnson, E. J. (2002). The role of carotenoids in human health. Nutr. Clin. Care 5, 56–65. doi: 10.1046/j.1523-5408.2002.00004.x
Karalija, E., Zeljković, S. C., and Parić, A. (2019). Harvest time-related changes in biomass, phenolics and antioxidant potential in Knautia sarajevensis shoot cultures after elicitation with salicylic acid and yeast. In Vitro Cell. Dev. Biol. Plant 56, 177–183. doi: 10.1007/s11627-019-10028-0
Karuppusamy, S. (2009). A review on trends in production of secondary metabolites from higher plants by in vitro tissue, organ and cell cultures. J. Medicinal Plants Res. 3, 1222–1239.
Kazan, K., and Manners, J. M. (2008). Jasmonate signaling: toward an integrated view. Plant Physiol. 146, 1459–1468. doi: 10.1104/pp.107.115717
Kennedy, D. O., and Wightman, E. L. (2011). Herbal extracts and phytochemicals: plant secondary metabolites and the enhancement of human brain function. Adv. Nutr. 2, 32–50. doi: 10.3945/an.110.000117
Kim, H.-J., Chen, F., Wang, X., and Rajapakse, N. C. (2006). Effect of methyl jasmonate on secondary metabolites of sweet basil (Ocimum basilicum L.). J. Agric. Food Chem. 54, 2327–2332. doi: 10.1021/jf051979g
Kim, H.-J., Fonseca, J. M., Choi, J.-H., and Kubota, C. (2007). Effect of methyl jasmonate on phenolic compounds and carotenoids of romaine lettuce (Lactuca sativa L.). J. Agric. Food Chem. 55, 10366–10372. doi: 10.1021/jf071927m
Korkina, L., Kostyuk, V., Potapovich, A., Mayer, W., Talib, N., and De Luca, C. (2018). Secondary plant metabolites for sun protective cosmetics: from pre-selection to product formulation. Cosmetics 5, 32–52. doi: 10.3390/cosmetics5020032
Kovač, M., and Ravnikar, M. (1994). The effect of jasmonic acid on the photosynthetic pigments of potato plants grown in vitro. Plant Sci. 103, 11–17. doi: 10.1016/0168-9452(94)03974-7
Kovač, M., and Ravnikar, M. (1998). Sucrose and jasmonic acid interact in photosynthetic pigment metabolism and development of potato (Solanum tuberosum L. cv. Sante) grown in vitro. Plant Growth Regul. 24, 101–107. doi: 10.1023/A:1005965626250
Krzyzanowska, J., Czubacka, A., Pecio, L., Przybyz, M., Doroszewska, T., Stochmal, A., et al. (2011). The effects of jasmonic acid and methyl jasmonate on rosmarinic acid production in Mentha × piperita cell suspension cultures. Plant Cell Tissue Organ Culture 108, 73–81. doi: 10.1007/s11240-011-0014-8
Kumari, G. J., and Sudhakar, C. (2003). Effects of jasmonic acid on groundnut during early seedling growth. Biologia Plantarum 47, 453–456. doi: 10.1023/B:BIOP.0000023894.72554.b2
Lichtenthaler, H. K., and Buschmann, C. (2001). “Chlorophylls and carotenoids: measurement and characterization by UV-VIS spectroscopy,” in Current Protocols in Food Analytical Chemistry, ed R. E. Wrolstad (New York, NY: John Wiley and Sons), F4.3.1–F4.3.8.
Marathe, S. A., Rajalakshmi, V., Jamdar, S. N., and Sharma, A. (2011). Comparative study on antioxidant activity of different varieties of commonly consumed legumes in India. Food Chem. Toxicol. 49, 2005–2012. doi: 10.1016/j.fct.2011.04.039
Mendoza, D., Cuaspud, O., Arias, J. P., Ruiz, O., and Arias, M. (2018). Effect of salicylic acid and methyl jasmonate in the production of phenolic compounds in plant cell suspension cultures of Thevetia peruviana. Biotechnol. Rep. 19, e00273. doi: 10.1016/j.btre.2018.e00273
Misawa, N. (2011). Pathway engineering for functional isoprenoids. Curr. Opin. Biotechnol. 22, 627–633. doi: 10.1016/j.copbio.2011.01.002
Moharram, H. A., and Youssef, M. M. (2014). Methods for determining the antioxidant activity: A review. Alex. J. Fd. Sci. Technol. 11, 31–42. doi: 10.12816/0025348
Moreno-Escamilla, J. O., Alvarez-Parrilla, E., de la Rosa, L. A., Núñez-Gastélum, J. A., González-Aguilar, G. A., and Rodrigo-García, J. (2017). Effect of different elicitors and preharvest day application on the content of phytochemicals and antioxidant activity of butterhead lettuce (Lactuca sativa var. Capitata) produced under hydroponic conditions. J. Agric. Food Chem. 65, 5244–5254. doi: 10.1021/acs.jafc.7b01702
Murashige, T., and Skoog, F. (1962). A revised medium for rapid growth and bio assays with tobacco tissue cultures. Physiol. Plantarum 15, 473–497. doi: 10.1111/j.1399-3054.1962.tb08052.x
Nahak, G., and Sahu, R. K. (2011). Evaluation of antioxidant activity of flower and seed oil of Azadirachta indica A. juss. J. Appl. Natural Sci. 3, 78–81. doi: 10.31018/jans.v3i1.158
Nakabayashi, R., and Saito, K. (2015). Integrated metabolomics for abiotic stress responses in plants. Curr. Opin. Plant Biol. 24, 10–16. doi: 10.1016/j.pbi.2015.01.003
Ncube, B., and Van Staden, J. (2015). Tilting plant metabolism for improved metabolite biosynthesis and enhanced human benefit. Molecules 20, 12698–12731. doi: 10.3390/molecules200712698
Neill, S. O., Gould, K. S., Kilmartin, P. A., Mitchell, K. A., and Markham, K. R. (2002). Antioxidant activities of red versus green leaves in Elatostema rugosum. Plant Cell Environ. 25, 539–547. doi: 10.1046/j.1365-3040.2002.00837.x
Othman, R. (2009). Biochemistry and genetics of carotenoid composition in potato tubers (Thesis). Lincoln University, Canterbury, New Zealand.
Pandey, G., Verma, K., and Singh, M. (2014). Evaluation of phytochemical, antibacterial and free radical scavenging properties of Azadirachta indica (neem) leaves. Int. J. Pharm. Pharmaceutical Sci. 6, 444–447.
Parmoon, G., Ebadi, A., Jahanbakhsh, S., Hashemi, M., and Moosavi, S. A. (2018). Effect of exogenous application of several plant growth regulators on photosynthetic pigments of fennel plants. Notulae Scientia Biol. 10, 508–515. doi: 10.15835/nsb10410356
Patil, P., Patil, S., Mane, A., and Verma, S. (2013). Antidiabetic activity of alcoholic extract of Neem (Azadirachta indica) root bark. Natl. J. Physiol. Pharm. Pharmacol. 3, 142–146. doi: 10.5455/njppp.2013.3.134-138
Paul, R., Prasad, M., and Sah, N. K. (2011). Anticancer biology of Azadirachta indica L. (neem): A mini review. Cancer Biol. Ther. 12, 467–476. doi: 10.4161/cbt.12.6.16850
Payá, M., Halliwell, B., and Hoult, J. R. (1992). Interactions of a series of coumarins with reactive oxygen species. Scavenging of superoxide, hypochlorous acid and hydroxyl radicals. Biochem. Pharmacol. 44, 205–214. doi: 10.1016/0006-2952(92)90002-Z
Quelemes, P. V., Perfeito, M. L. G., Guimarães, M. A., dos Santos, R. C., Lima, D. F., Nascimento, C., et al. (2015). Effect of neem (Azadirachta indica A. Juss) leaf extract on resistant Staphylococcus aureus biofilm formation and Schistosoma mansoni worms. J. Ethnopharmacol. 175, 287–294. doi: 10.1016/j.jep.2015.09.026
Rajurkar, N. S., and Hande, S. M. (2011). Estimation of phytochemical content and antioxidant activity of some selected traditional Indian medicinal plants. Indian J. Pharm. Sci. 73, 146–151. doi: 10.4103/0250-474X.91574
Ramakrishna, A., and Ravishankar, G. A. (2011). Influence of abiotic stress signals on secondary metabolites in plants. Plant Signal. Behav. 6, 1720–1731. doi: 10.4161/psb.6.11.17613
Sahraroo, A., Babalar, M., Mirjalili, M. H., Fattahi Moghaddam, M. R., and Nejad Ebrahimi, S. (2014). In-vitro callus induction and rosmarinic acid quantification in callus culture of Satureja khuzistanica Jamzad (Lamiaceae). Iran. J. Pharm. Res. 13, 1447–1456.
Saleh Al-Hashemi, Z. S., and Hossain, M. A. (2016). Biological activities of different neem leaf crude extracts used locally in Ayurvedic medicine. Pacific Sci. Rev. A Natural Sci. Eng. 18, 128–131. doi: 10.1016/j.psra.2016.09.013
Samad, M.A., Hashim, S.H., Simarani, K., and Yaacob, J.S. (2016). Antibacterial properties and effects of fruit chilling and extract storage on antioxidant activity, total phenolic and anthocyanin content of four date palm (Phoenix dactylifera) cultivars. Molecules 21, 419. doi: 10.3390/molecules21040419
Samborska, I. A., Kalaji, H. M., Sieczko, L., Goltsev, V., Borucki, W., and Jajoo, A. (2018). Structural and functional disorder in the photosynthetic apparatus of radish plants under magnesium deficiency. Functional Plant Biol. FPB 45, 668–679. doi: 10.1071/FP17241
Saniewski, M., Ueda, J., and Miyamoto, K. (2002). Relationships between jasmonates and auxin in regulation of some physiological processes in higher plants. Acta Physiol. Plant 24, 211–220. doi: 10.1007/s11738-002-0013-9
Santner, A., and Estelle, M. (2009). Recent advances and emerging trends in plant hormone signalling. Nature. 459, 1071–1078. doi: 10.1038/nature08122
Sarmiento, W. C., Maramba, C. C., and Gonzales, M. L. M. (2011). An in-vitro study on the antibacterial effect of neem (Azadirachta indica) leaf extract on methicillin-sensitive and methicillin-resistant Staphylococcus aureus. Pediatric Infect. Dis. Soc. Philippines 12, 40–45.
Seca, A. M. L., and Pinto, D. C. G. A. (2018). Plant secondary metabolites as anticancer agents: successes in clinical trials and therapeutic application. Int. J. Mol. Sci. 19, 263. doi: 10.3390/ijms19010263
Seo, J.-H., Kim, J.-E., Shim, J.-H., Yoon, G., Bang, M.-A., Bae, C.-S., et al. (2016). HPLC analysis, optimization of extraction conditions and biological evaluation of Corylopsis coreana Uyeki Flos. Molecules 21, 94. doi: 10.3390/molecules21010094
Sithisarn, P., Supabphol, R., and Gritsanapan, W. (2005). Antioxidant activity of Siamese neem tree (VP1209). J. Ethnopharmacol. 99, 109–112. doi: 10.1016/j.jep.2005.02.008
Sitko, K., Gieroń, Z., Szopiński, M., Zieleznik-Rusinowska, P., Rusinowski, S., Pogrzeba, M., et al. (2019). Influence of short-term macronutrient deprivation in maize on photosynthetic characteristics, transpiration and pigment content. Sci. Rep. 9, 14181. doi: 10.1038/s41598-019-50579-1
Solihah, M. A., Wan Ishak, W. R., and Nurhanan, A. R. (2012). Phytochemicals screening and total phenolic content of Malaysian Zea mays hair extracts. Int. Food Res. J. 19, 1533–1538. Available online at: https://core.ac.uk/download/83541532.pdf
Srivastava, P., and Chaturvedi, R. (2011). Increased production of azadirachtin from an improved method of androgenic cultures of a medicinal tree Azadirachta indica A. Juss. Plant Signal. Behav. 6, 974–981. doi: 10.4161/psb.6.7.15503
Suzuki, N., Rivero, R. M., Shulaev, V., Blumwald, E., and Mittler, R. (2014). Abiotic and biotic stress combinations. New Phytologist 203, 32–43. doi: 10.1111/nph.12797
Thuynsma, R., Kleinert, A., Kossmann, J., Valentine, A. J., and Hills, P. N. (2016). The effects of limiting phosphate on photosynthesis and growth of Lotus japonicus. South Afr. J. Botany 104, 244–248. doi: 10.1016/j.sajb.2016.03.001
Ueda, J., Kato, J., Yamane, H., and Takahashi, N. (1981). Inhibitory effect of methyl jasmonate and its related compounds on kinetin-induced retardation of oat leaf senescence. Physiol. Plantarum 52, 305–309. doi: 10.1111/j.1399-3054.1981.tb08511.x
Ullah, S., Khan, R. U., Afridi, S. R., Ullah, A., Hussain, A., and Khaliq-ur-Rehman. (2018). Isolation of quercetin and kaempferol from Azadirachta indica leaves and its synergistic action against clinically isolated multi drug resistant strains MRSA. Res. Rev. J. Pharm. Anal. 7, 1–5.
Vasconsuelo, A., and Boland, R. (2007). Molecular aspects of the early stages of elicitation of secondary metabolites in plants. Plant Sci. 172, 861–875. doi: 10.1016/j.plantsci.2007.01.006
Vergallo, C., Panzarini, E., and Dini, L. (2019). High performance liquid chromatographic profiling of antioxidant and antidiabetic flavonoids purified from Azadirachta indica (neem) leaf ethanolic extract. Pure Appl. Chem. 91, 1631–1640. doi: 10.1515/pac-2018-1221
Verma, V., Ravindran, P., and Kumar, P. P. (2016). Plant hormone-mediated regulation of stress responses. BMC Plant Biol. 16, 86. doi: 10.1186/s12870-016-0771-y
Wahab, M., farooq Patel, D. C., and Armstrong, D. W. (2017). Peak shapes and their measurements: the need and the concept behind total peak shape analysis. Lc Gc North Am. 12, 846–853.
Wang, Y., Mostafa, S., Zeng, W., and Jin, B. (2021). Function and mechanism of jasmonic acid in plant responses to abiotic and biotic stresses. Int. J. Mol. Sci. 22, 8568. doi: 10.3390/ijms22168568
Wasternack, C., and Strnad, M. (2016). Jasmonate signaling in plant stress responses and development-active and inactive compounds. New Biotechnol. 33, 604–613. doi: 10.1016/j.nbt.2015.11.001
Wewetzer, A. (1998). Callus cultures of Azadirachta indica and their potential for the production of azadirachtin. Phytoparasitica 26, 47–52. doi: 10.1007/BF02981265
Yang, L., Wen, K.-S., Ruan, X., Zhao, Y.-X., Wei, F., and Wang, Q. (2018). Response of plant secondary metabolites to environmental factors. Molecules 23, 762–788. doi: 10.3390/molecules23040762
Yusof, Z., Ramasamy, S., Mahmood, N. Z., and Yaacob, J. S. (2018). Vermicompost supplementation improves the stability of bioactive anthocyanin and phenolic compounds in Clinacanthus nutans Lindau. Molecules 23, 1345. doi: 10.3390/molecules23061345
Zhang, C. G., Li, W., Mao, Y. F., Zhao, D. L., Dong, W., and Guo, G. Q. (2005). Endogenous hormonal levels in Scutellaria baicalensis calli induced by thidiazuron. Russian J. Plant Physiol. 52, 345–351. doi: 10.1007/s11183-005-0052-3
Zhu, J. K. (2016). Abiotic stress signaling and responses in plants. Cell 167, 313–324 doi: 10.1016/j.cell.2016.08.029
Ziegler, J., and Facchini, P. J. (2008). Alkaloid biosynthesis: metabolism and trafficking. Annu. Rev. Plant Biol. 59, 735–769. doi: 10.1146/annurev.arplant.59.032607.092730
Keywords: jasmonic acid, Azadirachta indica, plant tissue culture, callus age, bioactive pigments, antioxidant activities
Citation: Ahmad Fauzi NS, Abd Rahim MH, Abdul Majid N, Othman R and Yaacob JS (2022) Evaluation of the effect of jasmonic acid elicitation on composition of pigments and biological activities in green callus of neem (Azadirachta indica). Front. Sustain. Food Syst. 6:1017398. doi: 10.3389/fsufs.2022.1017398
Received: 12 August 2022; Accepted: 20 October 2022;
Published: 25 November 2022.
Edited by:
Praveen Guleria, DAV University, IndiaReviewed by:
S. G. Ghane, Shivaji University, IndiaCopyright © 2022 Ahmad Fauzi, Abd Rahim, Abdul Majid, Othman and Yaacob. This is an open-access article distributed under the terms of the Creative Commons Attribution License (CC BY). The use, distribution or reproduction in other forums is permitted, provided the original author(s) and the copyright owner(s) are credited and that the original publication in this journal is cited, in accordance with accepted academic practice. No use, distribution or reproduction is permitted which does not comply with these terms.
*Correspondence: Jamilah Syafawati Yaacob, jamilahsyafawati@um.edu.my
Disclaimer: All claims expressed in this article are solely those of the authors and do not necessarily represent those of their affiliated organizations, or those of the publisher, the editors and the reviewers. Any product that may be evaluated in this article or claim that may be made by its manufacturer is not guaranteed or endorsed by the publisher.
Research integrity at Frontiers
Learn more about the work of our research integrity team to safeguard the quality of each article we publish.