- 1Marine Science Laboratory, Department of Biological Sciences, Charles E. Schmidt College of Science, Florida Atlantic University, Boca Raton, FL, United States
- 2Molecular Microbiology & Genetics Laboratory, Guy Harvey Oceanographic Center, Halmos College of Arts and Sciences, Nova Southeastern University, Dania Beach, FL, United States
- 3Department of Biological Sciences, Charles E. Schmidt College of Science, Florida Atlantic University, Boca Raton, FL, United States
Microbial communities play a crucial role in the physiology of animal hosts; however, little is known about bacterial symbionts with the group cephalopods, specifically octopuses, and the function of these symbionts. The goal of this study was to determine if octopuses have a unique skin microbiome. The skin microbiome of two sympatric octopuses (Octopus vulgaris and Macrotritopus defilippi) was compared with the surrounding environment, sediment and seawater, to determine if octopus have a unique skin microbiome. High throughput sequencing of the bacterial 16S rRNA gene (V3-V4 region) amplicons was performed using an Illumina MiSeq. Sediment showed the greatest alpha diversity followed by octopus then seawater. Beta diversity revealed a difference in microbial composition between the octopus skin microbiome and sediment and seawater. While phylum Bacteroidetes appeared rare in environmental samples, it was most abundant for the octopus skin microbiome with the majority of the bacteria comprising the family Flavobacteriaceae. Proteobacteria, the largest group of bacteria, also constituted the octopus skin microbiome. Many of these groups occur on both octopus species; however, certain taxa differed in relative abundance between octopus species and may show species-specific host selection. Several bacteria that were identified for the octopus skin microbiome have been isolated from other marine animal hosts, identified as biodegraders and/or produce pigments and squalene, or act as predators of other bacteria. These groups may play a role in defense against environmental stressors or pathogenic bacteria. This is the first study to characterize the skin microbiome in two wild sympatric octopuses. Due to the importance of bacterial symbionts, this can provide insight to the physiology, behavior, ecology, and ultimately the health of these important animals in marine environments as well as care in captive or laboratory settings.
1 Introduction
Microbial symbioses are abundant and diverse in marine organisms spanning from thermophilic deep sea polychaetes, corals, sponges, to sharks, and sea turtles (Campbell et al., 2001; Webster and Taylor, 2012; Blackall et al., 2015; Kuschke, 2022; Pratte et al., 2022). These microbes are involved in the immunology, behavior, evolution, development, anatomy and physiology of their host (Gilbert et al., 2012). Although common among marine invertebrates, symbiotic associations between coleiod cephalopods (squids, cuttlefishes, and octopuses) and bacteria have yet to be explored in great detail with the exception of a few species.
Symbioses involved in predator-prey dynamics have been studied in the bobtail squid (Euprymna scolopes) light organ which houses bioluminescent symbiotic bacterium, Vibrio fischeri, responsible for counter-illumination camouflage and the blue-ringed octopus species’ that host bacteria in their posterior salivary glands which produce a potent neurotoxin (tetrodotoxin, TTX) for defense and hunting. The TTX has also been found in the eggs of blue-ringed octopuses serving as egg protection (Williams, 2010; Williams et al., 2011; McFall-Ngai, 2014). To protect eggs from fungal and bacteria fouling, the Chilen octopus (Octopus mimus) contain certain environmental bacteria in their egg cases while squids (E. scolopes, Sepioteuthis lessoniana, and Doryteuthis (Loligo) pealei), secrete a unique group of bacteria from their accessory nidamental gland (ANG) into the egg jelly coat (Barbieri et al., 2001; Iehata et al., 2016; Kerwin et al., 2019; Tseng et al., 2023). Additionally, it was shown recently that environmental bacteria are also required for the development of the ANG in E. scolopes (McAnulty et al., 2023). The common cuttlefish (Sepia officinalis) microbiome of the digestive tract, gills, and skin consisted of bacterial communities different from those in the seawater environment and food source. Specifically, cuttlefish had a simple microbiome dominated by Vibrionaceae in the digestive tract (main colonization in the esophagus) and Piscirickettsiaceae in the gills that possibly aid in host digestion with their ability to produce chitinases, proteases, amylase, and lipase (Egerton et al., 2018; Lutz et al., 2019).
Although bacteria play a vital role in cephalopod lifestyles, there are also known pathogenic bacteria that are harmful to octopuses (Farto et al., 2003). Vibrionaceae also contain genera that have known pathogens to cephalopods (Ford et al., 1986; Hanlon, 1990; Hanlon R. T, 1990). A pathogenic bacterium (V. lentus) was identified and was capable of reproducing in skin lesions, colonizing internal organs, which lead to mortality in some healthy wild octopus. Different species of Vibrio, Photobacterium, Pseudomonas, Aeromonas, Cytophaga, Flavobacterium, and Klebsiella have been isolated from external skin lesions, muscle, or hemolymph of various diseased octopus species. Once a cephalopod is injured, its wound is invaded by opportunistic pathogens potentially leading to skin ulcers or, in advanced lesions, cause deep wounds in the arms, head, or mantle that exposes the muscle and can be fatal (Hanlon et al., 1984; Ford et al., 1986; Hanlon R. T, 1990; Malham and Runham, 1998).
The knowledge of immune mechanisms to protect cephalopods is limited, but the literature indicates they have an innate system (like other mollusks and invertebrates) and lack an adaptive response. This innate immune system’s cellular and humoral responses of the hemolymph, hemocytes, and various other organs are involved in recognition, activation, and elimination of pathogens. The innate immune system is effective at combating and protecting against potential pathogenic bacteria, but this does not include the ability for immunological memory of pathogens and other microbial associations (Lanz-Mendoza and Contreras-Garduño, 2022). Analyses confirm that bacteria, viruses, and protists are the most common pathogens affecting wild and reared cephalopods, all of which could lead to mortality (Gestal and Castellanos-Martínez, 2015). To stop pathogens from causing lesions leading to increased exposure of infection and possibly fatality, cephalopods have external barriers as the first line of defense. Characterizing the octopus skin microbiome would aid in identifying potential symbionts that may protect the octopus from pathogenic bacteria.
Cephalopod skin, arguably best known for its pigmented cells (chromatophores), reflector cells (iridophores and leucophores), and musculature that aid in rapid adaptive camouflage and different types of communication (Hanlon and Messenger, 2018), is complex and involved in many functions such as osmotic and ionic regulation, exchange of nutrients and oxygen, lubrication, and protection against mechanical damage, microbes, viruses, or proteolytic degradation (Packard, 1988; Roper CF, 1990; Accogli et al., 2017). Although studies on morphology and chemistry of the cephalopod epidermis have been reported over the last two decades, characterization and diversity of bacteria harboring the octopus’s skin and if certain bacteria are selected as symbionts is poorly known. To date, studies on the cephalopod skin microbiomes have been reported from cuttlefish (S. officinalis) cultured and raised in the laboratory and wild caught octopuses from South Korea (O. variabilis) and Spain (O. vulgaris) (Lee et al., 2017; Lutz et al., 2019; Rodríguez-Barreto et al., 2024). Like many aquatic animals without an external shell, the surface of cephalopod skin (epidermis) is covered with a mucus layer which acts as the first interface between the animal and the environment. These mucous-secreting cells not only exhibit properties important for lubrication (mantle) and adhesion (arm suckers), but their composition contains glycoconjugates that play an important role in protection from microbes or interactions with microbial symbionts (Schauer, 2009; Accogli et al., 2017; González-Costa et al., 2020).
Cephalopods play a significant role in biological and biomedical research, education and outreach at Zoos and Aquariums, fisheries, and coastal ecosystems. Identification of diseases and bacterial symbionts are essential for the health of this important animal group, especially under stressful environmental conditions. Here we present the first study to identify and characterize bacteria associated with sympatric, shallow water octopuses (O. vulgaris and Macrotritopus defilippi) and compare the octopus skin microbiome to their surrounding environment (seawater and sediment) to determine if octopuses have a unique skin microbiome.
2 Methods
2.1 Study site and animals
Data collection took place in south Florida’s intracoastal waterway known as Lake Worth Lagoon Blue Heron Bridge (BHB) Phil Foster Park (26.7843°N, -80.0427°W). The BHB study area was approximately 62,000 m2 with an average depth of 3 m and a heterogeneous benthic environment, consisting of sandy plains, rocks, rubble, and shells which partially contributes to the coexistence of two densely populated octopus species, Octopus vulgaris and Macrotritopus defilippi (Bennice et al., 2019). Due to the close proximity to the Atlantic Ocean via the Palm Beach Inlet, water visibility at BHB is heavily influenced by the tidal cycle. SCUBA dives for sample collection took place within 1-2 h of high tides to increase the likelihood of locating octopuses. Octopus species in the lagoon have also shown changes in seasonal abundance with spring-summer having highest densities. Thus, SCUBA dives were scheduled between May-June 2021.
Two octopus species were sampled from this coastal environment to determine if there were species-specific bacteria associations (Figure 1). Octopus vulgaris is a medium-to large-sized octopus that can weigh up to 5 kg and has an average mantle length (ML) of 250 mm. Mainly juvenile and subadults were observed at BHB ranging from a ML of 12 - 178 mm that inhabited rock, rubble, and shell substrates. This species is part of an O. vulgaris species complex spanning temperate, subtropical, and tropical waters worldwide and consisting of at least six other species. A recent report has suggested to reinstate the name O. americanus (Avendaño et al., 2020) for this western Atlantic O. vulgaris (Type I); however, we are still awaiting genetic and morphological confirmation for this species in south Florida and will retain the name O. vulgaris for this study. Macrotritopus defilipii is a small- to medium-sized octopus that inhabits sandy to muddy habitats in the Caribbean, Atlantic Ocean, and Mediterranean. A specimen collected from the Canary Islands had a ML of 41 mm and weighed 50 g; however, this species is known to have an adult ML of up to 90 mm (Hanlon, 1988; Guerra et al., 2010). Juvenile to adult stage were observed for this species at the site (ML 12 to 90 mm). These two species exhibit different foraging activity periods with O. vulgaris being nocturnal and M. defilippi being diurnal (Bennice et al., 2021). To assist with temporary octopus capture and sample collection, SCUBA dives were also scheduled during octopus peak activity times.
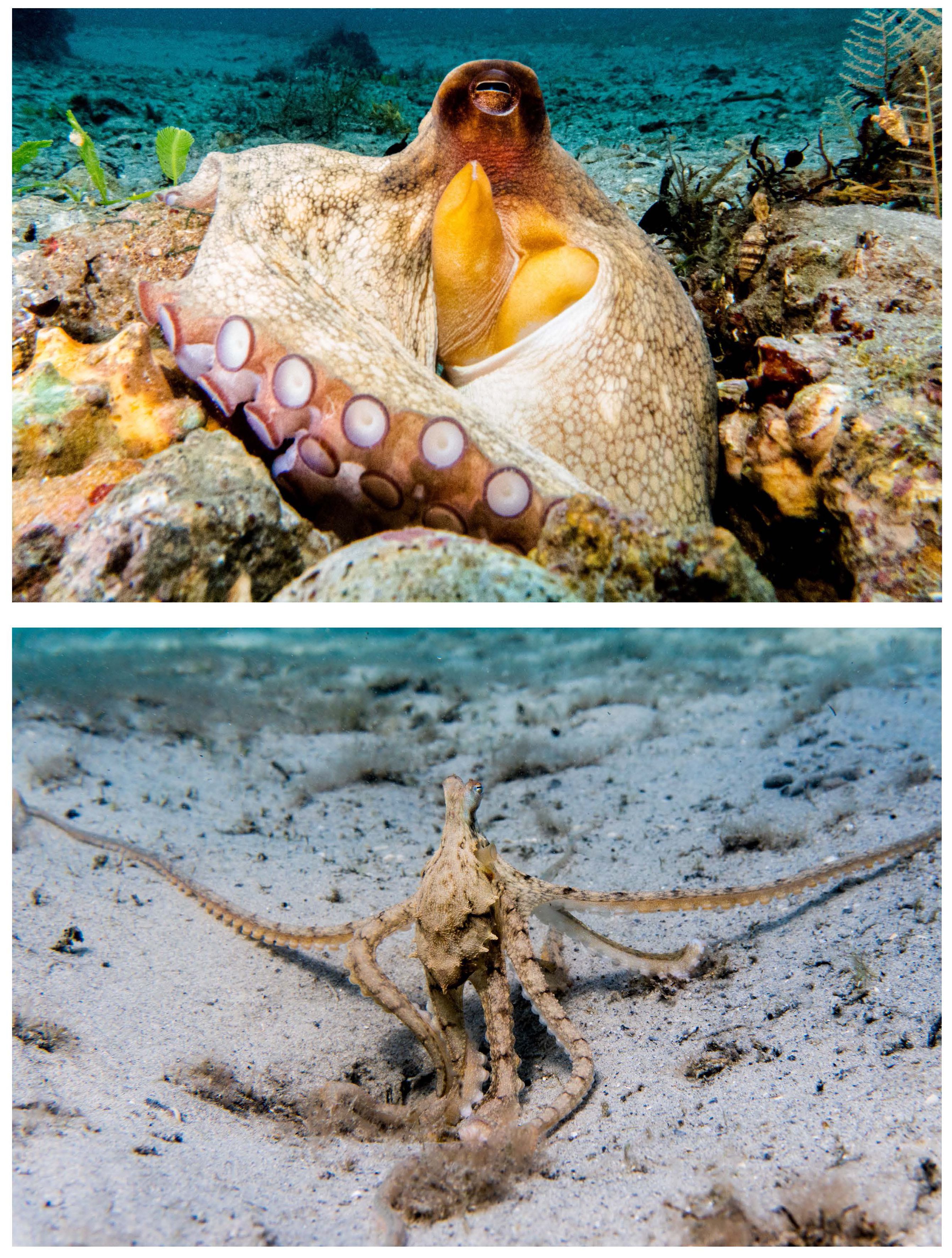
Figure 1. Two octopus species sampled for the octopus skin microbiome located in south Florida’s intracoastal waterway known as Lake Worth Lagoon Blue Heron Bridge Phil Foster Park (26.7843˚N, -80.0427˚W). Octopus vulgaris (top) known as a medium- to large-sized octopus inhabiting rock, rubble, or structured areas while active at night. Macrotritopus defilippi (bottom) a small- to medium- sized octopus inhabiting sandy plains and active during the day. Photo Credit: Chelsea O. Bennice.
2.2 Sample collection
SCUBA divers swam north-south paths until an octopus was located. Once an octopus was located, the lead scientific diver (COB) would do a visual health assessment. Signs of infection or disease frequently described include skin ulcers or lesions, deep wounds in arms, head, or mantle. Only healthy octopuses with no visual signs of infection were temporarily collected and sampled for this study under the Florida Fish and Wildlife Conservation Commission # SAL-20-2247 and Florida Atlantic University IACUC Protocol # A20-30. To obtain an accurate representation of the octopus skin microbiome and minimize animal stress and handling time, novel field collection methods were created.
After an octopus was deemed healthy by the visual health assessment, lead diver (COB) collected the octopus in a perforated bin then slowly ascended to the dive float which also served as a floating lab equipped with sampling containers and a holding arena for the octopus that allowed fresh seawater flow to the octopus until sampled. (Figure 2). Divers below marked the location where the octopus was collected and held the dive float/floating lab in place with tethered line. Next, COB removed a Puritan 6” Sterile Standard Foam-tipped swab (SKU#: 25-1506 1PF BT) from its dry transport tube to rub the mantle of the octopus. Method for swabbing the octopus’s mantle was adapted from Hollenbeck et al., 2017. The perforated bin holding the octopus was lifted out of the seawater and the octopus was swabbed for a total of 30 swipes up or down on the mantle’s dorsal surface. Two sterile swabs were used per octopus for a sample replicate. After swabbing was complete, the swab was placed back in its transport tube, stored on the floating lab, and the octopus was returned to its marked location. Sediment was then collected from each octopus location in a sterile falcon tube. Before the end of each dive, an ambient seawater sample (1 L) was collected from the mid-lower depth (2-3 m) of the water column. We avoided sampling the same octopus more than once by not approaching the same octopus den (i.e., where the octopus was collected from or returned to if out foraging) and surveying different areas of the study site. Samples were transported on ice back to Florida Atlantic University. Water was filtered using 0.45 μm filter paper (0.5 L of water per filter paper) and each placed into a 1.5 mL centrifuge tube. All samples (octopus swabs, sediment, and seawater) were then stored in a -80°C freezer until time of DNA extraction.
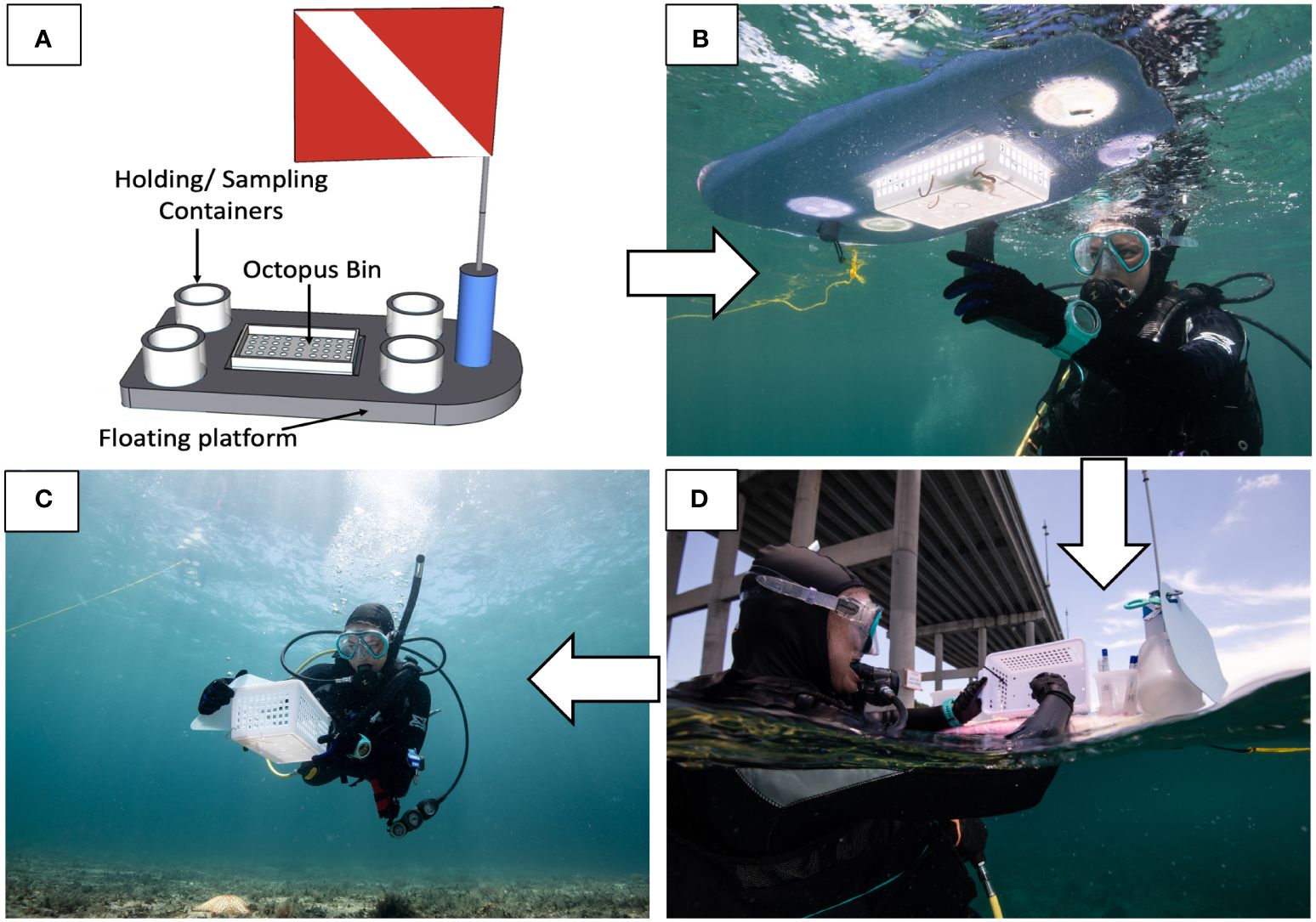
Figure 2. Novel field methodology. (A) Floating lab was designed to collect and temporarily hold octopus in a perforated bin. Once an octopus was in the bin (B), the bin was lifted to drain seawater and sterile swabs were used for sampling the skin microbiome (C). After the octopus’s mantle was swabbed, it was returned to its den or where it was found foraging (D). Sediment and seawater samples were taken at the site of octopus collection. Photo credit (B–D): Jennifer Adler.
2.3 DNA extraction, sample preparation and sequencing, analyses
2.3.1 DNA extractions
DNA extractions were performed on octopus skin swabs, seawater, and sediment (0.25 g) samples using the Qiagen DNeasy® PowerSoil® Kit following the protocol from the manufacturer (DNeasy® PowerSoil® Kit Handbook 2017, Catalog no. 12888-100). Modifications included homogenizing coarse sediment for 45s at 2,500 RPM and use of 50 uL instead of 100 uL of sterile elution buffer followed by a 5 min incubation at room temperature to complete the release and concentrate the DNA. DNA concentration for each sample was determined using Qubit®. If low amounts or no DNA was detected in the sample (only observed for octopus swab samples), then a replicate sample was pooled with the first. Extracted DNA samples were concentrated to 30 uL, stored in TrisHCL, frozen, and sent to Zymo Research, Irvine CA for PCR and sequencing services.
2.3.2 Targeted library preparation and sequencing
The DNA samples were prepared for targeted sequencing (16S rRNA gene) with the Quick-16S™ NGS Library Prep Kit (Zymo Research, Irvine, CA). The primer set used was to target and amplify the V3-V4 region. These primers were custom designed to provide the best coverage of the 16S gene while maintaining high sensitivity. PCR was performed using real-time PCR thermocyclers to control cycles and limit PCR chimera formation. Final PCR products were quantified with qPCR fluorescence readings and pooled together based on equal molarity. The final pooled library was cleaned with the Select-a-Size DNA Clean & Concentrator™ (Zymo Research, Irvine, CA), then quantified with TapeStation®(Agilent Technologies, Santa Clara, CA) and Qubit® (Thermo Fisher Scientific, Waltham, WA). The ZymoBIOMICS® Microbial Community DNA Standard (Zymo Research, Irvine, CA) was used as a positive control for targeted library preparation. The positive control utilizes a mock microbial community of well-defined composition to ensure the data generated are representative of the analyzed microbial samples. The microbial composition of ZymoBIOMICS® Microbial Community DNA Standard measured in this project can be found in Supplementary Materials Figure 1. A negative control (i.e., a blank library preparation sample) was included to assess the level of bioburden carried by the wet-lab process. An Illumina® MiSeq™ with a v3 reagent kit (600 cycles) was used to sequence the final library and sequencing was performed with 10% PhiX spike-in.
2.3.3 Analyses
For bioinformatics, amplicon sequence variants (ASVs) were inferred from raw reads using the DADA2 pipeline and potential sequencing errors and chimeric sequences were removed with the DADA2 pipeline (Callahan B.J. et al., 2016). Taxonomic assignments of ASVs were completed using the UCLUST from Qiime v.1.9.1 with the Zymo Research Database (a 16S database that Zymo Research internally designed and curated as a reference). Alpha- and beta diversity visualizations at different taxononomic levels were also performed with Qiime v.1.9.1 (Caporaso et al., 2010). Alpha diversity indices (Observed species, Chao1, Simpson’s E, and Shannon) were calculated from rarefied samples. Rarefaction curves were also visualized to determine if sequencing depth was sufficient. The Kruskal-Wallis test was used to determine significant differences between groups when groups did not meet parametric test assumptions (i.e., normality) followed by a Dunn’s multiple comparison test to determine which groups were statistically different for alpha diversity. To determine significant differences between octopus species, a Wilcoxon test was performed. Beta diversity was examined at the genus level through a 3-dimensional principal coordinate analysis (PCoA) plot using the matrix of pair-wise distance between samples calculated by the Bray-Curtis dissimilarity metric. To evaluate statistical significant differences between the groups, an Analysis of Similarities (ANOSIM) was performed.
3 Results
3.1 Microbial diversity and community composition of the octopus skin microbiome
DNA was extracted from twenty-five samples (9 octopuses, 9 sediment, 7 seawater) and sequenced to identify and characterize the microbial community comprising the octopus’s skin and associated environment. The number of ASVs was highest for sediment (median = 418 observed species, ± 187) followed by the octopus skin microbiome (median = 151 observed species, ± 285) then seawater (median = 123 observed species, ± 41) (Figure 3). Rarefaction curves demonstrated that sequencing depth was likely sufficient in discovering ASVs (Supplementary Materials Figure 2). Significant differences in all indices of alpha diversity were observed between groups (Kruskal-Wallis, p < 0.05) with the differences in alpha diversity between sediment and seawater (Dunn’s multiple comparison test: Observed species, p = 0.005; Chao1, p = 0.005; Simpson’s E, p = 0.001; Shannon, p = 0.004). Significant differences between octopus and sediment were reported for Simpson’s E metric of alpha diversity (Dunn’s multiple comparison test: p = 0.019). There were distinct differences in microbial community composition at the genus level between the three groups (ANOSIM: R = 0.76, p = 0.001). Clustering of the octopus samples were more variable than the seawater and sediment samples (Figure 4).
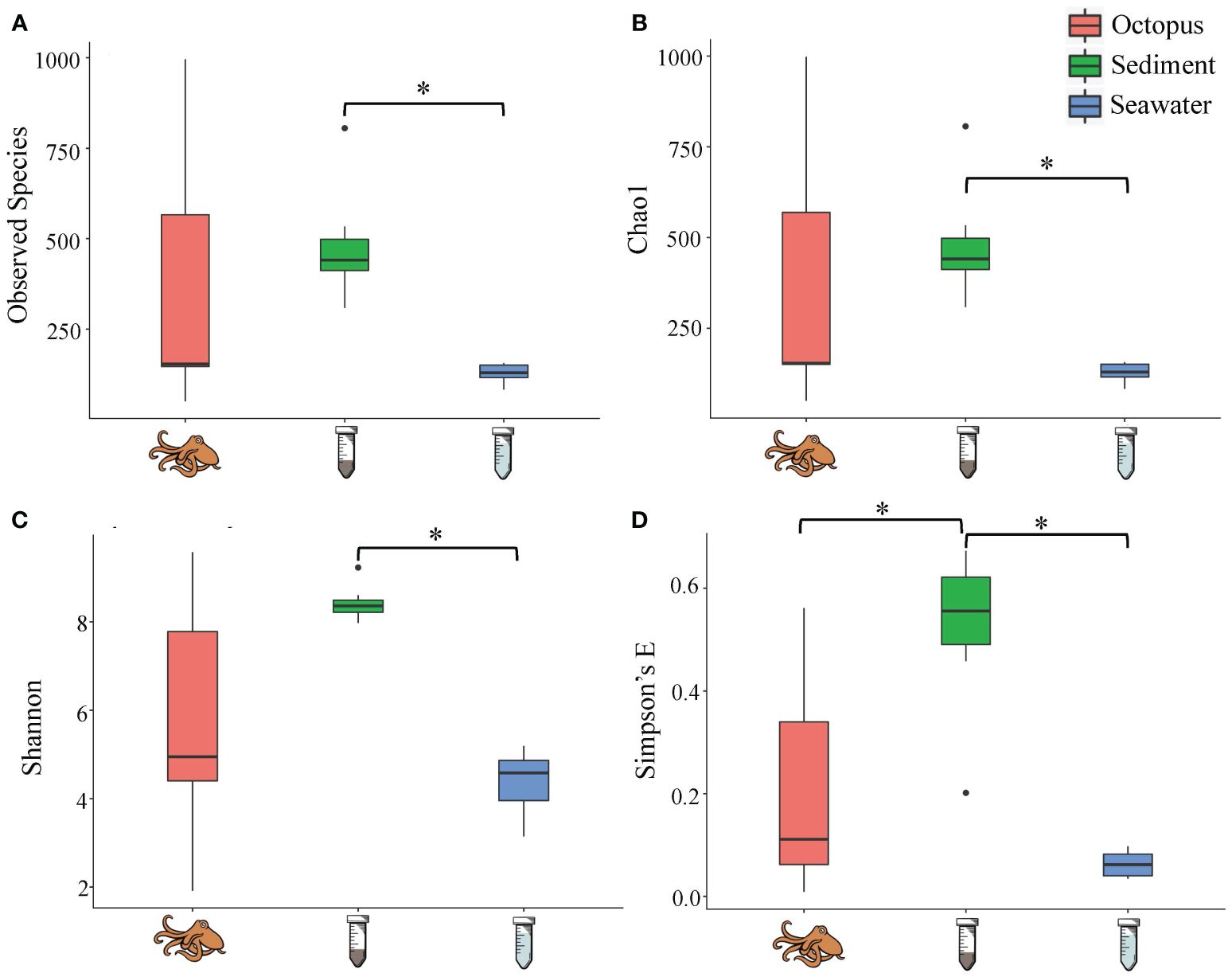
Figure 3. Alpha diversity (A) Observed species, (B) Chao1, (C) Shannon, (D) Simpson’s E) for octopus skin (n = 9, pink), sediment (n = 9, green), and seawater (n = 7, blue) within the Lake Worth Lagoon, FL. Alpha diversity was significantly different between groups (Kruskal-Wallis, p < 0.05; *Dunn’s multiple comparison test, p < 0.05). Boxes represent the interquartile range (IQR) between the first and third quartiles (25th and 75th percentiles, respectively) with the solid horizontal line indicating the median. Whiskers represent the lowest and highest values within 1.5 times the IQR from the first and third quartiles, respectively.
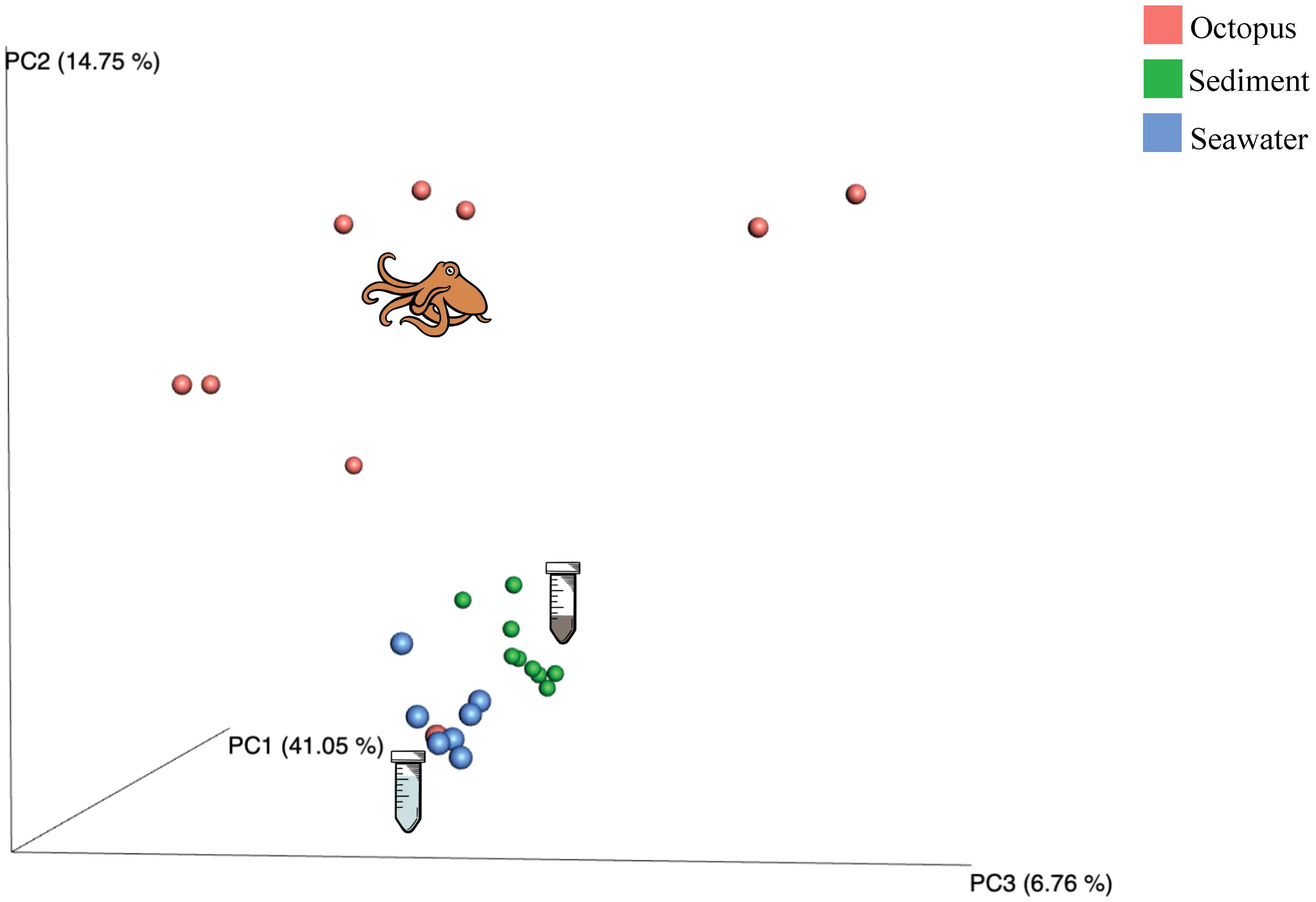
Figure 4. Beta diversity plot (PCoA, Bray-Curtis dissimilarity) for microbial composition at the genus level for octopus (n = 9, pink), sediment (n = 9, green), and seawater (n = 7, blue) samples. There were significant differences across groups with the octopus skin microbiome being statistically different from environmental samples sediment and seawater (ANOSIM: R = 0.76, p = 0.001).
Overall, 33 bacterial phyla were represented with the three most abundant phyla across sample type including Proteobacteria (53.2%), Bacteroidetes (16.7%) and Cyanobacteria (6.8%). The microbial community composition differed between groups (seawater, sediment, and octopus) with the top three phyla for seawater including Proteobacteria (84.3%), Cyanobacteria (9.0%), and Bacteroidetes (4.5%). Proteobacteria was also the top phylum for sediment (49.0%) followed by Actinobacteria (10.5%) and Bacteroidetes (7.9%). However, the phylum contributing the greatest to the microbial composition for octopus was Bacteroidetes (35.0%) followed by Proteobacteria (33.3%) and other that are unclassified (11.9%). (Figure 5A). Additional phyla that comprised the octopus skin microbiome >1% included: Cyanobacteria (5.4%), Actinobacteria (4.2%), Verrucomicrobia (2.9%), Firmicutes (1.2%), and Cloacimonetes (1.2%).
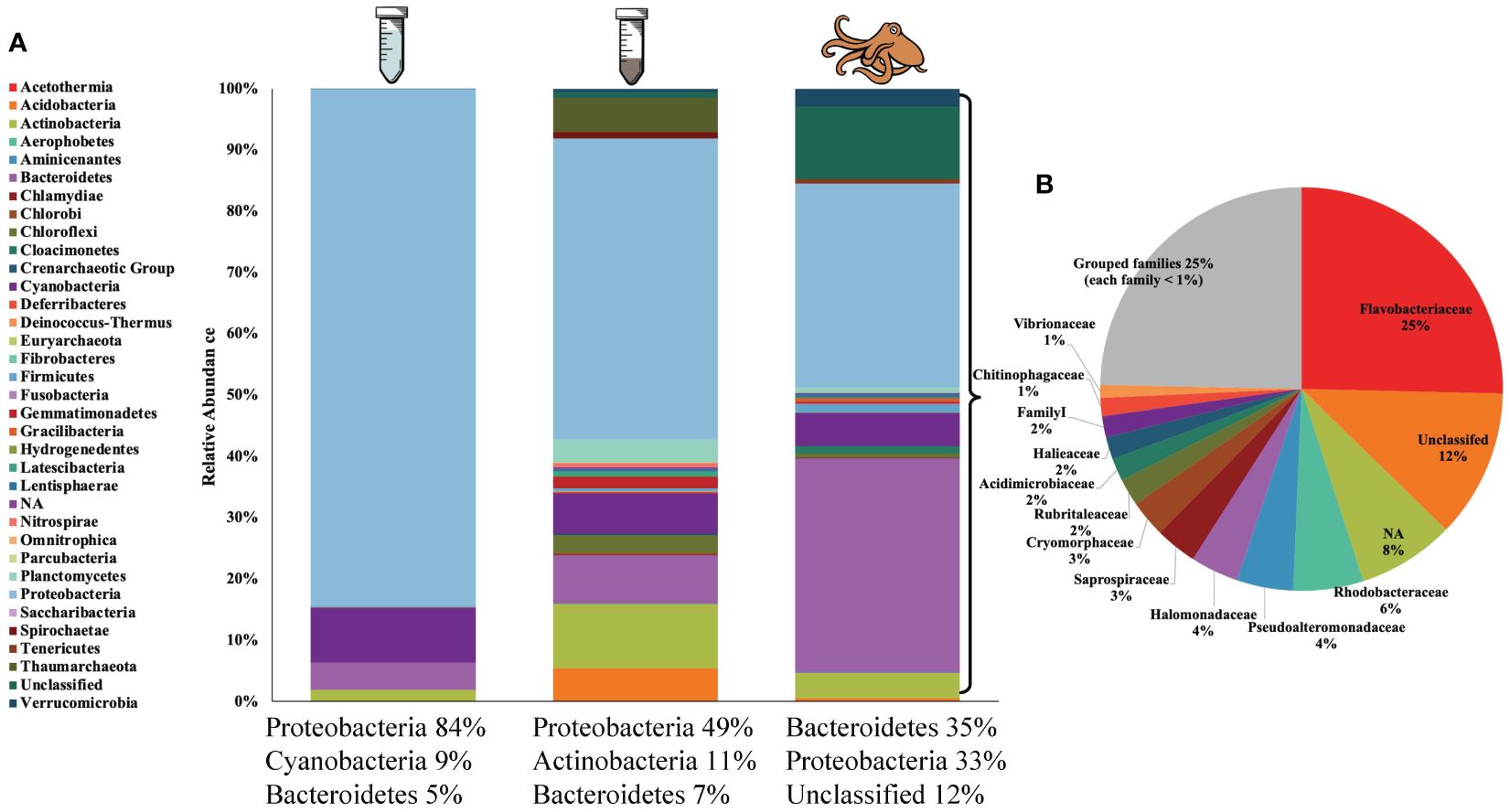
Figure 5. (A) Average relative abundances of microbial communities at the phylum level for seawater (n = 7, left), sediment (n = 9, middle) and octopus (n = 9, right) with top three most abundant phyla listed for each group. (B) Average relative abundance of microbial composition at the family level for octopus. Certain bacteria groups remained “unclassified” or could not be identified “NA”. “Grouped families” consisted of families with a relative abundance < 1%.
At the family level, there were 12 families that comprised the octopus skin microbiome > 1% with the most relative abundant families including: Flavobacteriaceae (25.4%), Rhodobacteraceae (5.6%), and Pseudoalteromonadaceae (4.5%) (Figure 5B). All 12 families were present on at least 7 out of 9 octopuses (all octopuses for Flavobacteriaceae and Rhodobacteraceae) with the exception of 2 families (Chitinophagaceae and Halomonadaceae) present on 5 out of 9 octopuses. There were 10 identified genera that comprised the octopus skin microbiome > 1% with the most relative abundant genera including: Tenacibaculum (13.7%), Pseudoalteromonas (4.5%) and Polaribacter (3.8%). The remaining 7 genera included: Psychroserpens, Cobetia, Rubritalea, Illumatobacter, Prochlorococcus, Crocinitomix and Saprospira. The most abundant genera were present on the majority of sampled octopuses with 8 out of 9 octopuses for Tenacibaculum, Pseudoalteromonas, and Polaribacter; and 7 out of 9 octopuses for Rubritalea and Crocinitomix. The remaining 5 genera (Illumatobacter, Prochlorococcus, Saprospira, Cobetia, Psychroserpens) ranged in presence from 3 to 6 out of 9 octopuses.
3.2 Microbial diversity and community composition between octopus species
For alpha diversity, Macrotritopus defilippi had a greater number of observed species and greater species richness; however, there was greater variation within M. defilippi samples and O. vulgaris exhibited greater species evenness (Figure 6). There were no significant differences in alpha diversity between M. defilippi and O. vulgaris for the four indices (Wilcoxon test: Observed species, p > 0.999; Chao1, p > 0.999; Simpson’s E, p = 0.375; Shannon, p = 0.875). Microbial community composition at the genus level was not different between the skin microbiome of these two species either (ANOSIM: R = 0.1938; p = 0.101; Figure 7).
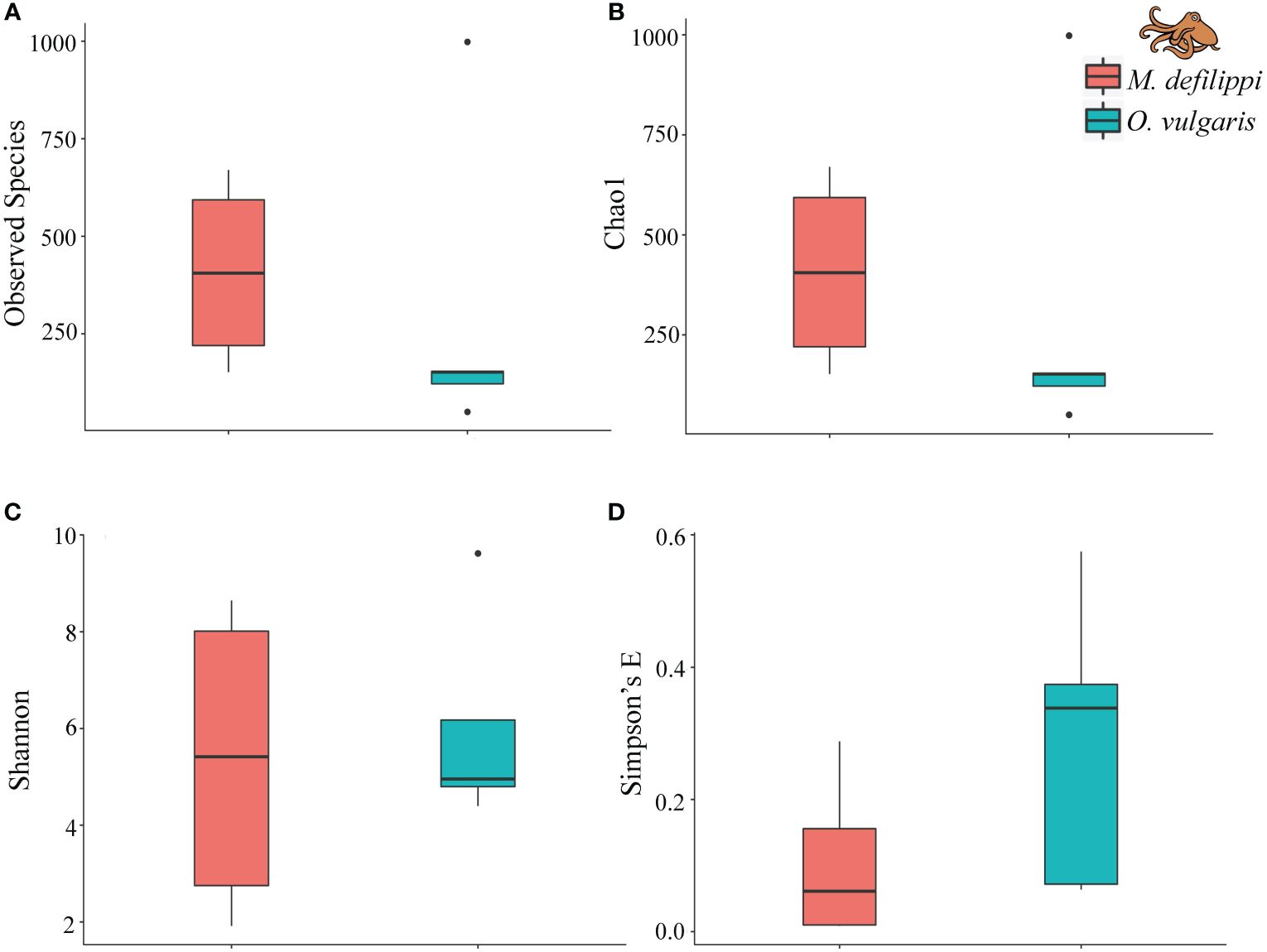
Figure 6. Alpha diversity (A) Observed species, (B) Chao1, (C) Shannon, (D) Simpson’s E) for Macrotritopus defilippi (n = 4, pink) and Octopus vulgaris (n = 5, blue) within the Lake Worth Lagoon, FL. Alpha diversity was not significantly different between octopus species (Wilcoxon test, p > 0.05). Boxes represent the interquartile range (IQR) between the first and third quartiles (25th and 75th percentiles, respectively) with the solid horizontal line indicating the median. Whiskers represent the lowest and highest values within 1.5 times the IQR from the first and third quartiles, respectively.
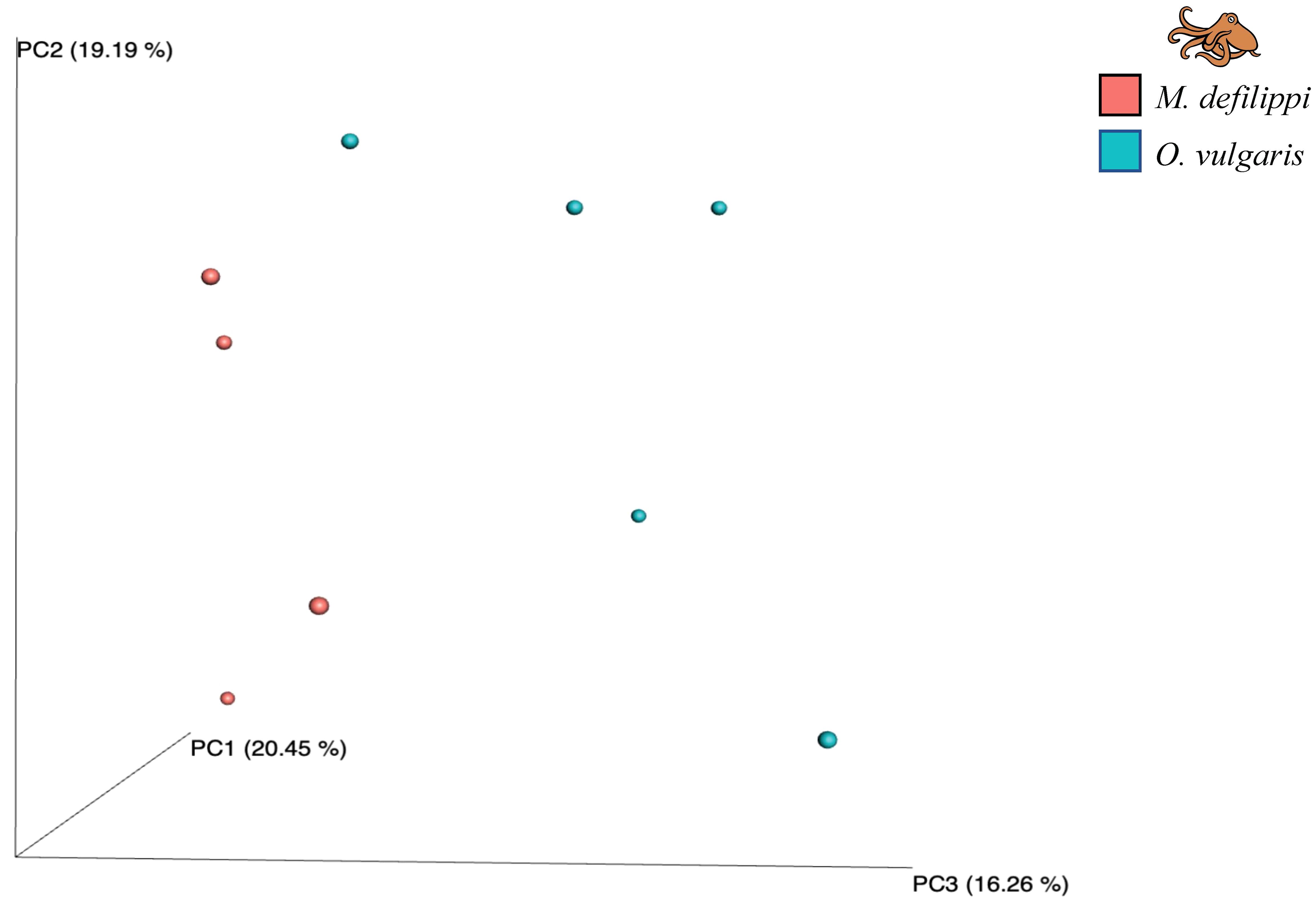
Figure 7. Beta diversity plot (PCoA, Bray-Curtis dissimilarity) for microbial composition at the genus level for Macrotritopus defilippi (n = 4, pink) and Octopus vulgaris (n = 5, blue) There were no significant differences between octopus species for microbial composition (ANOSIM: R = 0.1938, p = 0.101).
Although there were no statistical significant differences for microbial composition between octopuses, there were incidences of certain taxa being present/absent more often or in relatively larger abundances for one species versus the other (Figure 8; Supplementary Materials Table 1). Phylum Tenericutes was present on 4 out of 5 O. vulgaris and was not reported on M. defilippi. The average relative abundance for this phylum on the skin for O. vulgaris was 1.0% (3 of the 4 octopus had <1.0% and 1 octopus had 4.2%). Although phylum Verrucomicrobia was present on all octopuses, the average relative abundance was greater for O. vulgaris versus M. defilippi (4.8%, 0.5%; respectively). At the family level, Rubritaleaceae was a component of the octopus microbiome; however, there was a greater average relative abundance for O. vulgaris (3.9%) and was present on all individuals whereas it was only present on 2 of the 4 M. defilippi individuals with a lower average relative abundance (<0.1%). Other families that demonstrated greater average relative abundance on O. vulgaris versus M. defilippi included Pseudoalteromonadaceae (7.4%, 1.0%), Cryomorphaceae (4.2%, 1.5%), Acidimicrobiaceae (3.2%, 0.1%), Halieaceae (3.0%, 0.2%), FamilyI (2.4%, 0.8%), and Chitnophagaceae (2.3%, 0.3%). M. defilippi was mainly responsible for contributing to the “Unclassified” group (M.defilippi 24.4%, O. vulgaris 1.9%) and Vibrionaceae (M.defilippi 1.6%, O. vulgaris 0.6%). Other families that were not previously reported for the general octopus skin microbiome, but showed an average relative abundance > 1% for a specific species included Chromatiaceae and Flammeovirgaceae for O. vulgaris and Salinisphaeraceae and Alteromonadaceae for M. defilippi. The latter families mentioned for each species were present in higher abundance on only one or two individuals for each species.
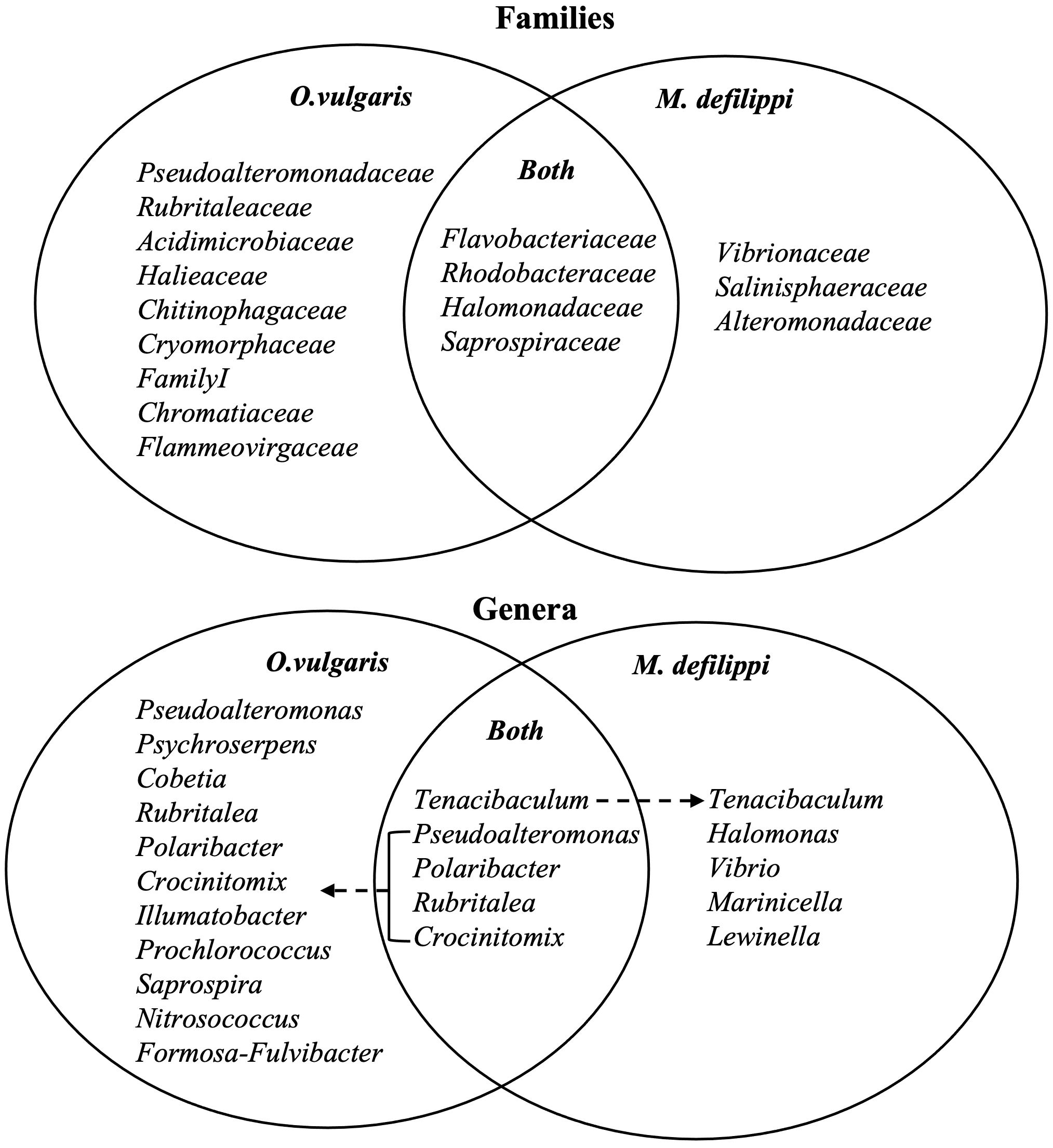
Figure 8. Microbial composition for Octopus vulgaris (n = 5) and Macrotritoups defilippi (n = 4) at the family (top) and genus (bottom) levels to summarize potential overlap and species-specific microbial symbionts for two sympatric octopuses. At the genus level, certain bacteria were present on the majority of octopuses (“Both”); however, these taxa had a higher average relative abundance on one octopus species compared to the other indicated by dashed arrow.
The majority of genera were present on both octopus species; however, average relative abundance differed between species (Figure 8; Supplementary Materials Table 1); and was usually driven by a large relative abundance on one or two individuals. Rubritalea was the exception being found on all O. vulgaris individuals and only 2 out of 4 M. defilippi individuals. Additional genera of > 1% average relative abundance were discovered when examining species-specific skin microbiomes. Genera Nitrosococcus and Formosa-Fulvibacter comprised O. vulgaris skin microbiome and Halomonas, Vibrio, Marinicella, and Lewinella comprised M. defilippi skin microbiome (Figure 8). Again, most of these trends were driven by one or two individuals with a larger composition of the bacterium. However, Vibrio and Marinicella were present on all M. defilippi individuals and Lewinella was present on 3 out of 4 M. defilippi individuals.
4 Discussion
Bacteria are one of the most common pathogens affecting wild and reared cephalopods (Gestal and Castellanos-Martínez, 2015) with cellular and humoral immune reactions interacting to protect against infection if the first lines of defense are breached – i.e., the skin and mucus. There are relatively few studies that have explored cephalopod microbial symbioses and a limited number that have examined their skin microbiome, especially for octopuses. It is likely that a unique set of bacteria would be associated with the external barriers, since microbiota play an important role in immune response and metabolic regulation within these hosts (Malham and Runham, 1998; Kau et al., 2011; Tremaroli and Bäckhed, 2012; Kang et al., 2022). We identified and characterized the skin microbiome of two wild sympatric octopus species, along with environmental samples, to determine if octopus have a unique skin microbial composition and discuss the potential role of these symbiotic bacteria.
This diversity of bacteria for the octopus’s skin was significantly different from its habitat (sediment) and surrounding seawater. Bacteroidetes and Proteobacteria were the two phyla that represented the majority (68.3%) of the octopus skin microbiome with Bacteroidetes being the predominant phylum. This phylum was rare in sediment and seawater samples suggesting that octopus skin may select for microbes that are generally in low abundance in the environment. This was the case for amphibian skin of multiple species (Walke et al., 2014).
This study’s findings were compared with the only two other studies to date that examined the skin microbiome of wild octopuses. Our results are consistent with a different species, O. variabilis, from South Korea (Lee et al., 2017). The predominant phylum for both studies was Bacteroidetes. Both studies also showed microbial composition (but less abundant) to include phyla Actinobacteria, Verrucomicrobia, and Firmicutes. However, there was a seasonal effect for O. variabilis skin microbial diversity and loads; octopus that were sampled in the month of August showed a microbiome predominantly consisting of Bacteroidetes whereas octopus sampled in November showed a microbiome predominately consisting of Proteobacteria. During August, phylum Verrucomicrobia was also present in O. variabilis microbiome as well as the greatest bacterial diversity and loads, which were influenced by environmental factors such as water temperature and location (Lee et al., 2017). All samples from our study were collected during the months of May and June from a single intracoastal location.
Although Bacteroidetes occurred on the skin microbiome for O. vulgaris in Spain, it was not the predominant phylum (Rodríguez-Barreto et al., 2024) as seen in our study. Female octopus in the study were dominated by Firmicutes and male octopus were dominated by Proteobacteria. Our study also reported both these phyla; however, in much lower abundance for Firmicutes and the sex of the animal was not recorded at the time of temporary collection. We observed some individuals of the same species with higher relative abundance of certain bacteria and this may be associated with a sex-specific bacterial microbiome (Rodríguez-Barreto et al., 2024). Both of these previous studies did not include analyses for comparisons against environmental samples; thus, our study is the first to determine a unique set of bacteria associated with wild octopuses that significantly differed from their environment. Future work for the octopus skin microbiome for these species and others should include comparisons for multiple locations, seasons, and sex.
One predominant family comprised the octopus skin microbiome from the phylum Bacteroidetes, Flavobacteriaceae. Family Flavobacteriaceae, the largest family from Bacteroidetes, has not only been known to be associated with seawater and sediment, but some members are associated with animals or plants. Most abundant genera from this family for the octopus skin microbiome included Tenacibaculum, Polaribacter, and Psychroserpens. Tenacibaculum mesophilum has often been found associated with marine hosts such as fish, sponges, and algae (Suzuki et al., 2001; Piñeiro-Vidal et al., 2008; Miyake et al., 2019). Other species from this genus (T. todarodis sp. nov. and T. aiptasiae sp. nov) have been isolated from the squid Todarodes pacificus from the East Sea off of Korea and a sea anemone Aiptasia pulchella (Wang et al., 2008; Shin et al., 2018). Many of these species are known opportunistic pathogens for fish species including T. maraitimum. This pathogen causes tenacibaculosis, an ulcerative disease (Avendaño-Herrera et al., 2006). Both Tenacibaulum potential symbionts (T. mesophilum, T. aiptasiae) and pathogens (T. maraitimum) were present for the octopus skin microbiome. Polaribacter spp. range in association from Antarctica sea ice, seawater, marine algae, marine sediment, and marine invertebrates (Han et al., 2017; Jeong et al., 2022) and are important for organic material degradation (Kirchman, 2008). Species that were identified on octopuses included P. dokdonensis and P. butkevichii (present on one octopus). From genus Psychroserpens, species that were identified included P. mesophilus and P. damuponensis.
Additional families from the predominant phylum Bacteroidetes observed for the octopus skin microbiome included Cryomorphaceae, Saprospiraceae, and Chitinophagaceae. From a general consensus of phylogenetic surveys, environmental samples of the marine-type Cryomorphaceae illustrated that this class is less numerous than other families belonging to phylum Bacteroidetes, especially family Flavobacteriaceae, thus members of this taxa could potentially be econiche specialized (Bowman, 2014). Although ecological data for this is scarce, this family was represented for the octopus microbiome with one genus, Crocinitomix, observed on the majority of octopuses. Family Saprospiraceae, consists of several genera including Saprospira that was reported on the octopus skin microbiome. Family members are likely important in the breakdown of complex organic compounds in the environment with the addition of helical gliding strains associated with predation of other bacteria and algae (McIlroy and Nielsen, 2014). To date, Saprospira grandis is the only species in the genus Saprospira that is known to predate on bacteria, such as Vibrio spp, through a process called ixotrophy by capturing bacteria in sticky surface materials (Lewin, 1997; Reichenbach, 2006; Heng et al., 2023).
Although the octopus skin microbiome was dominated by bacteria belonging to the phylum Bacteroidetes, families from phylum Proteobacteria were identified to have the next largest relative abundance: Rhodobacteraceae and Pseudoalteromonadaceae. Family Rhodobacteraceae is reported to be among the most widely distributed groups in marine habitats, specifically highly abundant within a coastal lagoon (Pohlner et al., 2019) and was previously reported on the skin of male O. vulgaris (Rodríguez-Barreto et al., 2024). Pseudoalteromonadaceae (genus Pseudoaltermonas) seems to be associated with eukaryotic hosts and has been isolated from various animals including mussels, pufferfish, tunicates, sponges, and now octopuses (Simidu et al., 1990; Ivanova et al., 1996; Holmström et al., 1998; Ivanova et al., 1998). It has been demonstrated that many Pseudoaltermonas species produce antibacterial products which aid in the colonization of surfaces such as their hosts (Holmström and Kjelleberg, 1999). Additional families from Proteobacteria that comprised the skin microbiome include Halomonadacease (genus Cobetia), Halieaceae, and Vibrionaceae. These three families have been documented in marine sediment and invertebrates (Halomondacease, genus Cobetia), coastal waters (Halieaceae) or are ubiquitous in marine, estuarine, and freshwater environments (Vibrionaceae) (Romanenko et al., 2013; Li et al., 2023). Family Vibrionaceae is a diverse group of bacteria known to include many facultative symbionts and pathogenic strains (Takemura et al., 2014).
Other phyla with their respective families and genera were present for the octopus skin microbiome in lower abundance. These groups included Cyanobacteria (Family I, genus Prochlorococcus), Actinobacteria (family Acidimicrobiaceae, genus Illumatobacter), Verrucombicrobia (family Rubritaleaceae, genus Rubritalea). These are diverse groups found in aquatic and terrestrial environments (Cyanobacteria), marine sediment (Actinobacteria, genus Illumatobacter) and have been found in associations with different hosts (Rubritaleaceae, genus Rubritalea) (Matsumoto et al., 2013; Mehdizadeh Allaf and Peerhossaini, 2022). Multiple species from the genus Rubritaela have been isolated from marine hosts including marine sponges (R. marina, R. spongiae, squalenifaciens), sea hare (R. tangerina), and a marine chordate (R. halochordaticola) (Yoon et al., 2007, 2011). Many members of this genus are characteristic of producing carotenoid pigments and squalene. Carotenoids are known to function as antioxidants (Johler et al., 2010) and play a role in defense against harmful conditions or environmental stressors such as changes in light, temperature, salinity, and pH (Ram et al., 2020). Squalene is a natural lipid belonging to the triterpene group or an isoprenoid compound that is found in plants, animals, protists, and bacteria. Along with carotenoids as a natural antioxidant, squalene can have antifungal, antibacterial, and antivirulence activities (Sri Charan Bindu et al., 2015).
These bacteria groups were present on the majority of octopuses (ranging 7 out of 9 octopuses or all 9 octopuses); however, variation in microbial composition may be further explained when comparing between octopus species’ skin microbiomes. Octopus species had differences in microbial composition at multiple taxonomic levels with certain taxa either absent or in lower relative abundance compared to the other species. Phylum, Tenericutes class Mollicutes was present on O. vulgaris and not reported on M. defilippi. This phylum was also previously reported as one of the predominant phyla for the study with O. variabilis (Lee et al., 2017). Class Mollicutes is widespread in nature and ranges from commensal symbionts to pathogens of humans, animals, and plants (Özbek et al., 2003; Elmnasri et al., 2018; Pimentel et al., 2021). Additionally, both bacterial families and genera may be species-specific for these co-occuring octopus species. Previous studies including amphibians, sea urchins, elasmobranchs, and teleost fishes demonstrated that host species shape their associated skin microbiota (Walke et al., 2014; Storo et al., 2021; Fronton et al., 2023; Rodríguez-Barreras et al., 2023; Lyons et al., 2024). The ecology of each species was reported to influence the differences in their microbiomes. For this study, specific genera were found on the majority of M. defilipii in a larger relative abundance than O. vulgaris which could be related to the species’ ecology. For example, genus Lewinella has multiple species that have been isolated from marine sediment (Lee, 2007). M. defilippi is exclusively a sand-dwelling species creating deep burrows in the sediment for its home, whereas O. vulgaris is a species typically found in structured areas with rock, rubble, fauna and flora. A previous study on Atlantic and green halibut populations reported the species-specific microbiomes were also found to vary according to sex, size, temperature, and geographical localization (Fronton et al., 2023). The goal of our study was to determine if octopus had a unique skin microbiota through a noninvasive sampling approach using sterile swabs. Given the high abundance of microbiota in the marine environment, skin swabs may have limitations capturing all microbial species on octopus skin which can include wrinkles, folds, and ridges. Future research with a larger sample size of each species with additional replicates, and factors including habitat, sex, size, and temperature should be conducted to further examine species-specific skin microbiomes. The previous report on O. vulgaris in Spain was on adult animals whereas our study collected mainly juveniles or subadults, which had a different microbiome (Rodríguez-Barreto et al., 2024). This could be related to geographical location. Future studies may also reveal ontogenetic shifts in octopus skin microbiota.
In summary, we characterized the skin microbiome of two shallow water benthic octopus species with their associated environment (both aquatic and benthic). This was the first study to identify an unique skin microbiome with HTS for sympatric wild octopuses and the first to characterize the skin microbiome for M. defilippi. Several bacteria groups and taxa comprising the octopus skin microbiome are known biodegraders, produce pigments, predate on other bacteria and/or have been isolated from other marine hosts. These bacteria may play an important role in defense against environmental stressors or inhibit fungal or bacterial growth of pathogens, which also constituted the octopus skin microbiome (i.e., V. harveyi and V. fortis) (Sun et al., 2023). Potential identification of species-specific microbiota of shallow water benthic species illustrates the importance of future research opportunities for additional octopus species inhabiting diverse habitats and their microbiota including bacteria and protists. Moreover, species confirmation and functional predictions of these bacterial symbionts are needed from cultured representatives.
Understanding the importance of microbial symbionts can provide insight to the physiology, behavior, and ultimately the health of these important animals in coastal and shallow marine environments, especially when agents of disease are predicted in the coming years due to ocean warming (Paillard et al., 2004; Reen et al., 2006; Lee et al., 2017). These likely positive and negative symbiotic interactions between bacteria and octopuses are not only important to marine ecosystems, but also for the care of octopuses in captive settings and to the marine bioprospecting industry.
Data availability statement
The data presented in the study are deposited in the National Center for Biotechnology Information Sequence Read Archive (NCBI SRA) repository, accession number PRJNA1136972.
Ethics statement
The animal study was approved by Florida Fish and Wildlife Special Activity License # SAL-20-2247 and Florida Atlantic University Institutional Animal Care and Use Committee (IACUC) Protocol # A20-30. The study was conducted in accordance with the local legislation and institutional requirements. Written informed consent was obtained from the individual(s) for the publication of any potentially identifiable images or data included in this article.
Author contributions
COB: Conceptualization, Data curation, Funding acquisition, Investigation, Methodology, Visualization, Writing – original draft, Writing – review & editing. LEK: Formal analysis, Methodology, Software, Writing – review & editing. WRB: Supervision, Writing – review & editing. JVL: Conceptualization, Resources, Supervision, Writing – review & editing.
Funding
The author(s) declare financial support was received for the research, authorship, and/or publication of this article. We are grateful for funding from the Broward Shell Club and the generous donors to the FAU Springboard Campaign.
Acknowledgments
We thank Florida Atlantic University’s scientific divers for accompanying COB on research dives throughout this study; specifically, Emily Serrano and Lizzie McNamee. Thank you to FAU’s diving and boating safety program for providing SCUBA tanks and compressed air. We thank Eric Fortman for assisting in the initial stages of field methodology and laboratory protocols.
Conflict of interest
The authors declare that the research was conducted in the absence of any commercial or financial relationships that could be construed as a potential conflict of interest.
Publisher’s note
All claims expressed in this article are solely those of the authors and do not necessarily represent those of their affiliated organizations, or those of the publisher, the editors and the reviewers. Any product that may be evaluated in this article, or claim that may be made by its manufacturer, is not guaranteed or endorsed by the publisher.
Supplementary material
The Supplementary Material for this article can be found online at: https://www.frontiersin.org/articles/10.3389/fmars.2024.1448199/full#supplementary-material
References
Accogli G., Scillitani G., Mentino D., Desantis S. (2017). Characterization of the skin mucus in the common octopus Octopus vulgaris (Cuvier) reared paralarvae. Eur. J. Histochem 61, 2815. doi: 10.4081/ejh.2017.2815
Avendaño O., Roura Á., Cedillo-Robles C. E., González Á.F., Rodríguez-Canul R., Velázquez-Abunader I., et al. (2020). Octopus americanus: a cryptic species of the O. vulgaris species complex redescribed from the Caribbean. Aquat. Ecol. 54, 909–925. doi: 10.1007/s10452-020-09778-6
Avendaño-Herrera R., Toranzo A. E., Magariños B. (2006). Tenacibaculosis infection in marine fish caused by Tenacibaculum maritimum: a review. Dis. Aquat Organ 71, 255–266. doi: 10.3354/dao071255
Barbieri E., Paster B. J., Hughes D., Zurek L., Moser D. P., Teske A., et al. (2001). Phylogenetic characterization of epibiotic bacteria in the accessory nidamental gland and egg capsules of the squid Loligo pealei (Cephalopoda: Loliginidae). Environ. Microbiol. 3, 151–167. doi: 10.1046/j.1462-2920.2001.00172.x
Bennice C. O., Brooks W. R., Hanlon R. T. (2021). Behavioral dynamics provide insight into resource exploitation and habitat coexistence of two octopus species in a shallow Florida lagoon. J. Exp. Mar. Biol. Ecol. 542-543, 151592. doi: 10.1016/j.jembe.2021.151592
Bennice C. O., Rayburn A. P., Brooks W. R., Hanlon R. T. (2019). Fine-scale habitat partitioning facilitates sympatry between two octopus species in a shallow Florida lagoon. Mar. Ecol. Prog. Ser. 609, 151–161. doi: 10.3354/meps12845
Blackall L. L., Wilson B., Van Oppen M. J. H. (2015). Coral-the world’s most diverse symbiotic ecosystem. Mol. Ecol. 24, 5330–5347. doi: 10.1111/mec.13400
Bowman J. P. (2014). “The family cryomorphaceae,” in The prokaryotes: other major lineages of bacteria and the archaea. Eds. Rosenberg E., Delong E. F., Lory S., Stackebrandt E. & Thompson F. (Springer Berlin Heidelberg, Berlin, Heidelberg).
Callahan B.J. M. P. J., Rosen M. J., Han A. W., Johnson A. J., Holmes S. P. (2016). DADA2: High resolution sample inference from Illumina amplicon data. Nat. Methods 13, 581–583. doi: 10.1038/nmeth.3869
Campbell B. J., Jeanthon C., Kostka J. E., Luther G. W., Cary S. C. (2001). Growth and Phylogenetic Properties of Novel Bacteria Belonging to the Epsilon Subdivision of the Proteobacteria Enriched fromAlvinella pompejana and Deep-Sea Hydrothermal Vents. Appl. Environ. Microbiol. 67, 4566–4572. doi: 10.1128/AEM.67.10.4566-4572.2001
Caporaso J. G., Kuczynski J., Stombaugh J., Bittinger K., Bushman F. D., Costello E. K., et al. (2010). QIIME allows analysis of high-throughput community sequencing data. Nat. Methods 7, 335–336. doi: 10.1038/nmeth.f.303
Egerton S., Culloty S., Whooley J., Stanton C., Ross R. P. (2018). The gut microbiota of marine fish. Front. Microbiol. 9. doi: 10.3389/fmicb.2018.00873
Elmnasri K., Hamdi C., Ettoumi B., Crotti E., Guesmi A., Najjari A., et al. (2018). Highly divergent Mollicutes symbionts coexist in the scorpion Androctonus australis. J. Basic Microbiol. 58, 827–835. doi: 10.1002/jobm.201800144
Farto R., Armada S. P., Montes M., Guisande J. A., Pérez M. J., Nieto T. P. (2003). Vibrio lentus associated with diseased wild octopus (Octopus vulgaris). J. Invertebr Pathol. 83, 149–156. doi: 10.1016/S0022-2011(03)00067-3
Ford L. A., Alexander S. K., Cooper K. M., Hanlon R. T. (1986). Bacterial populations of normal and ulcerated mantle tissue of the squid, Lolliguncula brevis. J. Invertebrate Pathol. 48, 13–26. doi: 10.1016/0022-2011(86)90138-2
Fronton F., Ferchiou S., Caza F., Villemur R., Robert D., St-Pierre Y. (2023). Insights into the circulating microbiome of Atlantic and Greenland halibut populations: the role of species-specific and environmental factors. Sci. Rep. 13, 5971. doi: 10.1038/s41598-023-32690-6
Gestal C., Castellanos-Martínez S. (2015). Understanding the cephalopod immune system based on functional and molecular evidence. Fish Shellfish Immunol. 46, 120–130. doi: 10.1016/j.fsi.2015.05.005
Gilbert S. F., Sapp J., Tauber A. I. (2012). A symbiotic view of life: we have never been individuals. Q Rev. Biol. 87, 325–341. doi: 10.1086/668166
González-Costa A., Fernández-Gago R., Carid S., Molist P. (2020). Mucus characterisation in the Octopus vulgaris skin throughout its life cycle. Anatomia Histologia Embryologia 49, 502–510. doi: 10.1111/ahe.12554
Guerra A., Allcock L., Pereira J. (2010). Cephalopod life history, ecology and fisheries: An introduction. Fisheries Res. 106, 117–124. doi: 10.1016/j.fishres.2010.09.002
Han J.-R., Wang K., Zhang J., Chen G.-J., Du Z.-J. (2017). Polaribacter tangerinus sp. nov., isolated from sediment in a sea cucumber culture pond. Int. J. Systematic Evolutionary Microbiol. 67, 4736–4741. doi: 10.1099/ijsem.0.002369
Hanlon R. T. (1988). Behavioral and body patterning characters useful in taxonomy and field identification of cephalopods. Malacologia 29, 247–264.
Hanlon Rt F.J. (1990). “1. Diseases of Mollusca: Cephalopoda. 1.1.Diseases caused by microorganisms,” in Diseases of marine animals. Ed. K. O. (Biologische Anstalt Helgoland, Hamburg, Germany).
Hanlon R. T., Forsythe J. W., Cooper K. M., Dinuzzo A. R., Folse D.S., Kelly M. T. (1984). Fatal penetrating skin ulcers in laboratory-reared octopuses. J. Invertebrate Pathol. 44, 67–83. doi: 10.1016/0022-2011(84)90047-8
Hanlon R. T., Messenger J. B. (2018). Cephalopod behaviour (Cambridge: Cambridge University Press). doi: 10.1017/9780511843600
Hanlon R. T A. F. J.W. (1990). 1. Diseases of Mollusca: Cephalopoda. 1.3.Structural abnormalities and neoplasia (Hamburg, Germany: Biologische Anstalt Helgoland).
Heng W. L., Lau N.-S., Furusawa G. (2023). Complete genome sequence of marine filamentous bacterium, Saprospira grandis strain WHT. Microbiol. Resource Announcements 12, e00441–e00423. doi: 10.1128/MRA.00441-23
Hollenbeck N., Scheel D., Gravley M. C., Sage G. K., Toussaint R., Talbot S. L. (2017). Use of swabs for sampling epithelial cells for molecular genetics analyses in enteroctopus. Am. Malacological Bull. 35, 145–157, 13. doi: 10.4003/006.035.0207
Holmström C., James S., Neilan B. A., White D. C., Kjelleberg S. (1998). Pseudoalteromonas tunicata sp. nov., a bacterium that produces antifouling agents. Int. J. Syst. Bacteriol 48 Pt 4, 1205–1212. doi: 10.1099/00207713-48-4-1205
Holmström C., Kjelleberg S. (1999). Marine Pseudoalteromonas species are associated with higher organisms and produce biologically active extracellular agents. FEMS Microbiol. Ecol. 30, 285–293. doi: 10.1016/S0168-6496(99)00063-X
Iehata S., Valenzuela F., Riquelme C. (2016). Evaluation of relationship between Chilean octopus (Octopus mimus Gould 1852) egg health condition and the egg bacterial community. Aquaculture Res. 47, 649–659. doi: 10.1111/are.12525
Ivanova E. P., Kiprianova E. A., Mikhailov V. V., Levanova G. F., Garagulya A. D., Gorshkova N. M., et al. (1998). Phenotypic diversity of Pseudoalteromonas citrea from different marine habitats and emendation of the description. Int. J. Systematic Evolutionary Microbiol. 48, 247–256. doi: 10.1099/00207713-48-1-247
Ivanova E. P., Kiprianova E. A., Mikhailov V. V., Levanova G. F., Garagulya A. D., Gorshkova N. M., et al. (1996). Characterization and identification of marine alteromonas nigrifaciens strains and emendation of the description. Int. J. Systematic Evolutionary Microbiol. 46, 223–228. doi: 10.1099/00207713-46-1-223
Jeong S.-W., Han J. E., Lee J.-Y., Yoo J.-H., Kim D.-Y., Jeong I. C., et al. (2022). Description of Polaribacter batillariae sp. nov., Polaribacter cellanae sp. nov., and Polaribacter pectinis sp. nov., novel bacteria isolated from the gut of three types of South Korean shellfish. J. Microbiol. 60, 576–584. doi: 10.1007/s12275-022-1604-3
Johler S., Stephan R., Hartmann I., Kuehner K. A., Lehner A. (2010). Genes Involved in Yellow Pigmentation of Cronobacter sakazakii ES5 and Influence of Pigmentation on Persistence and Growth under Environmental Stress. Appl. Environ. Microbiol. 76, 1053–1061. doi: 10.1128/AEM.01420-09
Kang W., Kim P. S., Tak E. J., Sung H., Shin N. R., Hyun D. W., et al. (2022). Host phylogeny, habitat, and diet are main drivers of the cephalopod and mollusk gut microbiome. Anim. Microbiome 4. doi: 10.1186/s42523-022-00184-x
Kau A. L., Ahern P. P., Griffin N. W., Goodman A. L., Gordon J. I. (2011). Human nutrition, the gut microbiome and the immune system. Nature 474, 327–336. doi: 10.1038/nature10213
Kerwin A. H., Gromek S. M., Suria A. M., Samples R. M., Deoss D. J., O’donnell K., et al. (2019). Shielding the next generation: symbiotic bacteria from a reproductive organ protect bobtail squid eggs from fungal fouling. mBio 10. doi: 10.1128/mBio.02376-19
Kirchman D. L. (2008). New light on an important microbe in the ocean. Proc. Natl. Acad. Sci. 105, 8487–8488. doi: 10.1073/pnas.0804196105
Kuschke S. G. (2022). What lives on and in the sea turtle? A literature review of sea turtle bacterial microbiota. Anim. Microbiome 4, 52. doi: 10.1186/s42523-022-00202-y
Lanz-Mendoza H., Contreras-Garduño J. (2022). Innate immune memory in invertebrates: Concept and potential mechanisms. Dev. Comp. Immunol. 127, 104285. doi: 10.1016/j.dci.2021.104285
Lee S. D. (2007). Lewinella agarilytica sp. nov., a novel marine bacterium of the phylum Bacteroidetes, isolated from beach sediment. Int. J. Systematic Evolutionary Microbiol. 57, 2814–2818. doi: 10.1099/ijs.0.65254-0
Lee J. J., Kim T. Y., Choi S. H., Kim B. S. (2017). Analysis of the bacterial microbiome in the small octopus, Octopus variabilis, from South Korea to detect the potential risk of foodborne illness and to improve product management. Food Res. Int. 102, 51–60. doi: 10.1016/j.foodres.2017.09.084
Lewin R. A. (1997). Saprospira grandis: A flexibacterium that can catch bacterial prey by “Ixotrophy. Microb. Ecol. 34, 232–236. doi: 10.1007/s002489900052
Li S.-H., Kang I., Cho J.-C. (2023). Metabolic versatility of the family halieaceae revealed by the genomics of novel cultured isolates. Microbiol. Spectr. 11, e03879–e03822. doi: 10.1128/spectrum.03879-22
Lutz H. L., Ramírez-Puebla S. T., Abbo L., Durand A., Schlundt C., Gottel N. R., et al. (2019). A simple microbiome in the european common cuttlefish, sepia officinalis. mSystems 4. doi: 10.1128/mSystems.00177-19
Lyons K., Bedore C. N., Carlisle A. B., Moniz L., Odom T. L., Ahmed R., et al. (2024). Network analysis reveals species-specific organization of microbial communities in four co-occurring elasmobranch species along the Georgia coast. Fishes 9, 34. doi: 10.3390/fishes9010034
Malham S. K., Runham N. W. (1998). A brief review of the immunobiology of Eledone cirrhosa. South Afr. J. Mar. Sci. 20, 385–391. doi: 10.2989/025776198784126304
Matsumoto A., Kasai H., Matsuo Y., Shizuri Y., Ichikawa N., Fujita N., et al. (2013). Ilumatobacternonamiense sp. nov. and Ilumatobactercoccineum sp. nov., isolated from seashore sand. Int. J. Systematic Evolutionary Microbiol. 63, 3404–3408. doi: 10.1099/ijs.0.047316-0
McAnulty S. J., Kerwin A. H., Koch E., Nuttall B., Suria A. M., Collins A. J., et al. (2023). Failure to launch”: development of a reproductive organ linked to symbiotic bacteria. mBio 14, e02131–e02122. doi: 10.1128/mbio.02131-22
McFall-Ngai M. (2014). Divining the essence of symbiosis: insights from the squid-vibrio model. PloS Biol. 12, e1001783. doi: 10.1371/journal.pbio.1001783
McIlroy S. J., Nielsen P. H. (2014). “The family saprospiraceae,” in The prokaryotes: other major lineages of bacteria and the archaea. Eds. Rosenberg E., Delong E. F., Lory S., Stackebrandt E., Thompson F (Springer Berlin Heidelberg, Berlin, Heidelberg).
Mehdizadeh Allaf M., Peerhossaini H. (2022). Cyanobacteria: model microorganisms and beyond. Microorganisms 10, 696. doi: 10.3390/microorganisms10040696
Miyake S., Soh M., Ding Y., Seedorf H. (2019). Complete genome sequence of sponge-associated tenacibaculum mesophilum DSM 13764T. Microbiol. Resource Announcements 8. doi: 10.1128/MRA.00517-19
Özbek E., Miller S. A., Meulia T., Hogenhout S. A. (2003). Infection and replication sites of Spiroplasma kunkelii (Class: Mollicutes) in midgut and Malpighian tubules of the leafhopper Dalbulus maidis. J. Invertebrate Pathol. 82, 167–175. doi: 10.1016/S0022-2011(03)00031-4
Packard A., Trueman E. R., Clarke M. R. (1988). The skin of cephalopods (Coleoids): general and special adaptations. Form and Function. E. R. Trueman and M. R. Clarke. San Diego, Academic Press. 11: 37-67. doi: 10.1016/B978-0-12-751411-6.50010-2
Paillard C., Le Roux F., Borrego J. J. (2004). Bacterial disease in marine bivalves, a review of recent studies: Trends and evolution. Aquat. Living Resour. 17, 477–498. doi: 10.1051/alr:2004054
Pimentel Z. T., Dufault-Thompson K., Russo K. T., Scro A. K., Smolowitz R. M., Gomez-Chiarri M., et al. (2021). Microbiome analysis reveals diversity and function of mollicutes associated with the eastern oyster, crassostrea virginica. mSphere 6. doi: 10.1128/mSphere.00227-21
Piñeiro-Vidal M., Riaza A., Santos Y. (2008). Tenacibaculum discolor sp. nov. and Tenacibaculum gallaicum sp. nov., isolated from sole (Solea Senegalensis) and turbot (Psetta maxima) culture systems. Int. J. Syst. Evol. Microbiol. 58, 21–25. doi: 10.1099/ijs.0.65397-0
Pohlner M., Dlugosch L., Wemheuer B., Mills H., Engelen B., Reese B. K. (2019). The majority of active rhodobacteraceae in marine sediments belong to uncultured genera: A molecular approach to link their distribution to environmental conditions. Front. Microbiol. 10, 659. doi: 10.3389/fmicb.2019.00659
Pratte Z. A., Perry C., Dove A. D. M., Hoopes L. A., Ritchie K. B., Hueter R. E., et al. (2022). Microbiome structure in large pelagic sharks with distinct feeding ecologies. Anim. Microbiome 4, 17. doi: 10.1186/s42523-022-00168-x
Ram S., Mitra M., Shah F., Tirkey S. R., Mishra S. (2020). Bacteria as an alternate biofactory for carotenoid production: A review of its applications, opportunities and challenges. J. Funct. Foods 67, 103867. doi: 10.1016/j.jff.2020.103867
Reen F. J., Almagro-Moreno S., Ussery D., Boyd E. F. (2006). The genomic code: inferring Vibrionaceae niche specialization. Nat. Rev. Microbiol. 4, 697–704. doi: 10.1038/nrmicro1476
Reichenbach H. (2006). “The genus saprospira,” in The prokaryotes: volume 7: proteobacteria: delta, epsilon subclass. Eds. Dworkin M., Falkow S., Rosenberg E., Schleifer K.-H., Stackebrandt E. (Springer New York, New York, NY).
Rodríguez-Barreras R., Dominicci-Maura A., Tosado-Rodríguez E. L., Godoy-Vitorino F. (2023). The epibiotic microbiota of wild caribbean sea urchin spines is species specific. Microorganisms 11, 391. doi: 10.3390/microorganisms11020391
Rodríguez-Barreto D., Sanz-González J. C., Martín M. V., Arrieta J. M., Almansa E. (2024). Sex-specific bacterial microbiome variation in octopus vulgaris skin. Front. Microbiol. 14. doi: 10.3389/fmicb.2023.1233661
Romanenko L. A., Tanaka N., Svetashev V. I., Falsen E. (2013). Description of Cobetia amphilecti sp. nov., Cobetia litoralis sp. nov. and Cobetia pacifica sp. nov., classification of Halomonas halodurans as a later heterotypic synonym of Cobetia marina and emended descriptions of the genus Cobetia and Cobetia marina. Int. J. Systematic Evolutionary Microbiol. 63, 288–297. doi: 10.1099/ijs.0.036863-0
Roper CF L. C. (1990). Comparative morphology and function of dermal structures in oceanic squids (Cephalopoda) (Washington, D.C: Smithsonian Institution Press).
Schauer R. (2009). Sialic acids as regulators of molecular and cellular interactions. Curr. Opin. Struct. Biol. 19, 507–514. doi: 10.1016/j.sbi.2009.06.003
Shin S. K., Kim E., Yi H. (2018). Tenacibaculum todarodis sp. nov., isolated from a squid. Int. J. Syst. Evol. Microbiol. 68, 1479–1483. doi: 10.1099/ijsem.0.002692
Simidu U., Kita-Tsukamoto K., Yasumoto T., Yotsu M. (1990). Taxonomy of four marine bacterial strains that produce tetrodotoxin. Int. J. Systematic Evolutionary Microbiol. 40, 331–336. doi: 10.1099/00207713-40-4-331
Sri Charan Bindu B., Mishra D. P., Narayan B. (2015). Inhibition of virulence of Staphylococcus aureus – a food borne pathogen – by squalene, a functional lipid. J. Funct. Foods 18, 224–234. doi: 10.1016/j.jff.2015.07.008
Storo R., Easson C., Shivji M., Lopez J. V. (2021). Microbiome analyses demonstrate specific communities within five shark species. Front. Microbiol. 12. doi: 10.3389/fmicb.2021.605285
Sun X., Li Y., Yang Q., Zhang H., Xu N., Tang Z., et al. (2023). Identification of quorum sensing-regulated Vibrio fortis as potential pathogenic bacteria for coral bleaching and the effects on the microbial shift. Front. Microbiol. 14. doi: 10.3389/fmicb.2023.1116737
Suzuki M., Nakagawa Y., Harayama S., Yamamoto S. (2001). Phylogenetic analysis and taxonomic study of marine Cytophaga-like bacteria: proposal for Tenacibaculum gen. nov. with Tenacibaculum maritimum comb. nov. and Tenacibaculum ovolyticum comb. nov., and description of Tenacibaculum mesophilum sp. nov. and Tenacibaculum amylolyticum sp. nov. Int. J. Syst. Evol. Microbiol. 51, 1639–1652. doi: 10.1099/00207713-51-5-1639
Takemura A. F., Chien D. M., Polz M. F. (2014). Associations and dynamics of Vibrionaceae in the environment, from the genus to the population level. Front. Microbiol. 5, 38. doi: 10.3389/fmicb.2014.00038
Tremaroli V., Bäckhed F. (2012). Functional interactions between the gut microbiota and host metabolism. Nature 489, 242–249. doi: 10.1038/nature11552
Tseng P.-W., Li H.-W., Chen C., Tseng Y.-C., Chang C.-F., Wu G.-C. (2023). Transcriptomic profile of symbiotic accessory nidamental gland during female maturation in bigfin reef squid. Front. Mar. Sci. 9. doi: 10.3389/fmars.2022.1026742
Walke J. B., Becker M. H., Loftus S. C., House L. L., Cormier G., Jensen R. V., et al. (2014). Amphibian skin may select for rare environmental microbes. Isme J. 8, 2207–2217. doi: 10.1038/ismej.2014.77
Wang J. T., Chou Y. J., Chou J. H., Chen C. A., Chen W. M. (2008). Tenacibaculum aiptasiae sp. nov., isolated from a sea anemone Aiptasia pulchella. Int. J. Syst. Evol. Microbiol. 58, 761–766. doi: 10.1099/ijs.0.65437-0
Webster N. S., Taylor M. W. (2012). Marine sponges and their microbial symbionts: love and other relationships. Environ. Microbiol. 14, 335–346. doi: 10.1111/j.1462-2920.2011.02460.x
Williams B. L. (2010). Behavioral and chemical ecology of marine organisms with respect to tetrodotoxin. Mar. Drugs 8, 381–398. doi: 10.3390/md8030381
Williams B. L., Hanifin C. T., Brodie E. D. Jr, Caldwell R. L. (2011). Ontogeny of tetrodotoxin levels in blue-ringed octopuses: maternal investment and apparent independent production in offspring of Hapalochlaena lunulata. J. Chem. Ecol. 37, 10–17. doi: 10.1007/s10886-010-9901-4
Yoon J., Matsuda S., Adachi K., Kasai H., Yokota A. (2011). Rubritalea halochordaticola sp. nov., a carotenoid-producing verrucomicrobial species isolated from a marine chordate. Int. J. Systematic Evolutionary Microbiol. 61, 1515–1520. doi: 10.1099/ijs.0.025031-0
Yoon J., Matsuo Y., Matsuda S., Adachi K., Kasai H., Yokota A. (2007). Rubritalea spongiae sp. nov. and Rubritalea tangerina sp. nov., two carotenoid- and squalene-producing marine bacteria of the family Verrucomicrobiaceae within the phylum ‘Verrucomicrobia’, isolated from marine animals. Int. J. Systematic Evolutionary Microbiol. 57, 2337–2343. doi: 10.1099/ijs.0.65243-0
Keywords: cephalopods, Octopus vulgaris, Macrotritopus defilippi, noninvasive sampling method, microbial community, 16S rRNA, Bacteroidetes, Flavobacteriaceae
Citation: Bennice CO, Krausfeldt LE, Brooks WR and Lopez JV (2024) Unique skin microbiome: insights to understanding bacterial symbionts in octopuses. Front. Mar. Sci. 11:1448199. doi: 10.3389/fmars.2024.1448199
Received: 12 June 2024; Accepted: 19 July 2024;
Published: 09 August 2024.
Edited by:
Lucia Zifcakova, Okinawa Institute of Science and Technology Graduate University, JapanReviewed by:
Anna Okunola, Stellenbosch University, South AfricaLarry J. Dishaw, University of South Florida St. Petersburg, United States
Copyright © 2024 Bennice, Krausfeldt, Brooks and Lopez. This is an open-access article distributed under the terms of the Creative Commons Attribution License (CC BY). The use, distribution or reproduction in other forums is permitted, provided the original author(s) and the copyright owner(s) are credited and that the original publication in this journal is cited, in accordance with accepted academic practice. No use, distribution or reproduction is permitted which does not comply with these terms.
*Correspondence: Chelsea O. Bennice, cbennice@fau.edu