- Marine Environment Center, Korea Marine Equipment Research Institute, Gyeongsangnam-do, Busan, Republic of Korea
The International Maritime Organization has adopted a revision to the 2023 International Maritime Organization biofouling guidelines and has been discussing the development of guidelines for evaluating the efficacy of in-water cleaning systems. In hull cleaning, which involves aquatic invasive species removal, capture is considered very important in preventing the release of by-products. However, capture efficacy is difficult to evaluate due to the absence of unified evaluation guidelines or standardized methods. Here, we describe a new test method for evaluating the capture efficacy of in-water cleaning systems, using artificial barnacles that simulate the physical characteristics of actual barnacles. The test was designed to evaluate the impact of in-water cleaning on biocide release and biofouling organisms in the marine environment. We used a test plate with artificial barnacles attached to evaluate the capture efficacy with respect to the fouling rating. This test overcomes limitations in the evaluation of the capture efficacy of in-water cleaning systems and provides a reference for the development of more comprehensive tests and in-water cleaning regulation in the future.
1 Introduction
Ships, representing the largest proportion of the transport involved in international trade, are related to the global spread of aquatic invasive species (AIS). AIS, which refers to species introduced away from their native habitats, alter their new ecosystems, resulting in ecological damage or economic loss. AIS are typically introduced via ballast water and biofouling. The International Maritime Organization (IMO) adopted the Ballast Water Management Convention in 2004 to intensively manage AIS in ballast water. In the world’s major ports, such as in the Philippines, Australia, New Zealand, and San Francisco (USA), however, biofouling causes worse ecosystem disturbance than ballast water (Sylvester et al., 2011; Chan et al., 2015). In New Zealand, 20–30% of AIS originates from ballast water and 55–60% is introduced via biofouling (Cranfield et al., 1998). In Australia, 50–55% of AIS is attributed to biofouling, with 78% attributed to biofouling in the Philip Bay survey (Hewitt et al., 2004). In addition to threatening biodiversity, AIS may have potentially serious social, environmental, and economic impacts. Hull-surface fouling increases friction resistance by increasing surface roughness, and severe fouling increases fuel consumption and greenhouse gas emissions by slowing the ship (Champ and Seligman, 1996; Schultz, 2007; Buhaug et al., 2009). The IMO’s Guidelines for the Control and Management of Ships’ Biofouling to Minimize the Transfer of Invasive Aquatic Species (IMO, 2011) recommend the management of biofouling through the periodic education of personnel on biofouling management. However, international regulations on biofouling remain at the recommendation level. The risk of AIS transfer will persist until regulations are enforced by international conventions. Some countries, including Australia, New Zealand, and the United States, have proactively prepared their own guidelines and reinforced domestic regulations. At Marine Environmental Protection Committee meetings, the IMO has also actively discussed the implementation of biofouling guidelines and has initiated the GloFouling partnership project to strengthen the biofouling management capabilities of developing countries.
Several prevention and removal approaches have been used to manage biofouling on ships. Antifouling paints that release one or more biocides through the paint surface have been the primary method for preventing the attachment of sea life to the hull for more than a century (Chambers et al., 2006; Gu et al., 2020). Coatings applied during dry docking, which typically occurs every 3 to 5 years for ships, are the foundation of biofouling management by the shipping industry. However, coatings cannot effectively protect certain ‘niche areas’ of the ship hull, such as intakes, propellers, rudders, and stern tubes (Davidson et al., 2009; Tamburri et al., 2020).
In dry docking, biofouling is directly and physically removed when the ship is out of the water. This prevents the release of biofouling organisms and antifouling agent components into the marine environment. Although dry docking is the most effective method of minimizing the biosecurity risks of shipping, it has limited value as a regular hull management method because it affects the ship’s operating schedule and is expensive and time-consuming. Several alternative methods and technologies for removing biofouling have recently been reported, including divers or remotely operated vehicles to physically remove biofouling. However, unlike the regulated waste streams generated from dry docking, the wastewater from in-water hull maintenance is currently unregulated (Forbes, 1996). Additionally, such methods may cause environmental hazards, such as increased biosecurity risks caused by the release of AIS and the discharge of antifouling biocides, organic substances, pigments, microplastics, or other contaminants that could adversely impact the environment. In this study, these contaminants were collectively referred to as debris. The marine pollution caused by the unregulated discharge of antifouling biocides during in-water cleaning is a serious problem. Severe biofouling can be removed through fragmentation, and its efficacy depends on the brush type and operating conditions of the cleaning unit. Therefore, the effective capture of these fragments and the safe treatment of the captured debris are required.
Recent studies have shown that capturing debris during in-water cleaning is essential (Scianni and Georgiades, 2019; Tamburri et al., 2020). Capture is defined as the process of containment, collection, and removal of biofouling material and waste substances detached from submerged surfaces during in-water cleaning or in dry dock (IMO, 2023a). The revised 2023 IMO biofouling guidelines state that reactive cleaning with capture is recommended for hulls that exceed fouling rating 2 and that proactive cleaning without capture should be conducted when the biofouling rating is less than 2 and performed in an area acceptable to the port authority (Table 1). Proactive cleaning is defined as the periodic removal of microfouling on ships’ hulls to prevent or minimize the attachment of macrofouling. Reactive cleaning means a corrective action during which biofouling is removed from a ship’s hull and niche areas either in water with capture or in dry dock. Two approaches to evaluating debris capture efficacy have been considered: 1) define an acceptable capture efficiency level, and 2) quantify the differences in water quality between the cleaning unit and the background water. Defining an acceptable level of capture efficiency as an evaluation criterion is difficult due to the difficulty in quantifying the total amount of biofouling and the debris generated during in-water cleaning. However, water quality comparison based on the Alliance for Coastal Technologies/Maritime Environmental Resource Center (ACT/MERC) and Baltic and International Maritime Council (BIMCO) guidelines (BIMCO, 2023; IMO, 2023b) make it possible to quantify capture efficacy by measuring changes in water quality factors (total suspended solids [TSS], particle size and distribution, particulate and dissolved organic carbon, total and dissolved biocide[s], and micro and nanoplastics). The Belgian ports of Antwerp, Zeebrugge, and Ghent have proposed a method for evaluating the suction performance of indoor in-water cleaning systems using non-toxic dyes for preliminary evaluation before field testing (IMO, 2021). These methods, however, involve many uncertainties in sampling and analysis depending on the background water environment and operating parameters. Frame rates, lighting requirements, and visibility levels that could allow for particle image velocimetry of debris and particulates in the area around the cleaning apparatus should all be considered (Morrisey et al., 2015; Tamburri et al., 2020).
Hull biofouling can be caused by macroalgae, hydrozoans, bryozoans, barnacles, polychaete tubeworms, mollusks, and ascidians (Cao et al., 2011; Flemming and Wuertz, 2019). Among these, barnacles, widespread across the global ocean and highly resistant to environmental change, have been used as a major invertebrate model group in biopollution-related research (Swain et al., 1992; Rainbow and Blackmore, 2001). Because they attach themselves to surfaces by secreting proteinaceous cement, barnacles have been used in many studies of biocide and artificial adhesive development. Recently, artificial barnacles were used to examine the impact of biofouling on the hydrodynamic characteristics of ships. A towing tank experiment was conducted using a plate covered with artificial barnacles (5–10 mm in diameter) to simulate the effect of barnacle biofouling on the ship’s resistance and power according to the barnacle growth stage. The methodology of adhesion tests has also been investigated through experiments with artificial (wooden) barnacles of various sizes, and the results of these experiments have been extrapolated to actual barnacles (Demirel et al., 2017; Kirkiz and Cavas, 2023). Artificial barnacles offer many benefits over actual barnacles, making it easier to achieve spatiotemporal diversity and standardized samples compared with using actual barnacles.
In this study, we developed a method for evaluating the capture efficiency of biofouling cleaning operations using an artificial barnacle model under controlled fouling conditions. As hull cleaning debris may contain high concentrations of both biocides and biofouling organisms (Thomas et al., 2002; Tamburri et al., 2020), we tested the efficacy of the capture of biocides and biofouling organisms separately. We assessed the release of biocides by measuring the biocide concentration generated during the in-water cleaning of a surface treated with antifouling coating and comparing it with the water quality standards set by domestic (South Korean) regulations. This method enables the identification of released biocides that can harm marine organisms. We also assessed the capture of the biofouling organism debris by calculating the dry weight of captured artificial barnacles relative to that of the attached barnacles before cleaning. This method quantified the percentage capture efficacy of the in-water cleaning system. The testing method presented here is independent of the pollution levels caused by operating conditions and the ship’s fouling rating, thus enabling the application of the method for the standardized evaluation of capture efficacy. In this study, the capture efficacy test was performed at a fouling rating of 2, which is the minimum standard requiring capture. This method provides a reference for the evaluation and regulation of capture efficacy for in-water cleaning operations.
2 Materials and methods
To evaluate the efficacy of biocide and biofouling debris capture in the cleaning and capture-and-treat system, we used artificial barnacles to simulate the biofouling organisms. Actual barnacles are protected by heavily calcified outer shell plates and attach themselves firmly to their substrate using a proteinaceous adhesive. To create artificial barnacles that mimic these characteristics of the actual organisms, we conducted compressive and adhesion strength tests.
2.1 Artificial barnacles
Artificial barnacles, which simulated biofouling organisms, were constructed from acrylic material using three-dimensional prints of actual barnacle scans. Depending on the species and habitat, barnacles can grow to 3.7–62.9 mm (with an average of 20 mm) (Doell et al., 2017). Based on ASTM D5618-20, a standard test for measuring barnacle adhesion strength, we selected a base diameter of 20 mm and a height of 10 mm (Figure 1).
2.2 Compressive and adhesion strength tests for artificial barnacles
To ensure that the artificial barnacles had a shell strength similar to that of actual barnacles, we conducted compressive and adhesion strength tests using a 100 kN universal testing machine (UTM; INSTRON 8800, Norwood, MA, USA). The final compressive strength was the average of the compressive values obtained from five artificial barnacles treated at 100 or 150°C for 24 or 48 h (to achieve a compressive strength comparable with that of actual barnacles). The compressive strength test was conducted following the ASTM D695 standard, and the sample was compressed at a deformation rate of 2 mm/min. The compressive strength (MPa) was calculated by dividing the maximum load by the maximum diameter of the artificial barnacle upon fracture.
The adhesion strength test was conducted on artificial barnacles to which various adhesives were uniformly applied following the ASTM D5618-20 standard. Five adhesives commonly used for plastics—acrylic, epoxy, cyanoacrylate, silicone, and polyurethane (A–E, respectively)—were selected. The test panel used for the adhesion was a steel plate coated with epoxy primer and a copper-based antifouling coat. The artificial barnacles were located at least 20 mm away from the edge of the test plate and were attached so there was no interference between the plate and the barnacles. The adhesion strength test was repeated three times for each test panel. After allowing the adhesives on the artificial barnacles to dry at room temperature for over a day, the test plate was immersed in natural seawater for 20 days. The adhesion strength was tested on more than five artificial barnacles for each adhesive. After fixing the test plate (with the artificial barnacles attached) in the UTM, the adhesion strength was tested at 4.5 N/s until the sample was separated from the surface. The adhesion strength (MPa) was calculated by dividing the measured removal strength (N) of the barnacle by its base area (mm2), as follows (Equation 1):
2.3 Test plate
The test plate—a steel plate sheeting (4 m × 1.5 m, with a thickness of 8 mm)—was designed according to the size and load of the cleaning unit. The plate surface was sandblasted with 50 μm alumina particles. The plate used to test biocide release during cleaning was coated with anticorrosive (Jotacote Universal N10, Jotun, Sandefjord, Norway) and a copper antifouling paint (SeaQuantum Pro U paint, Jotun, Sandefjord, Norway), and marked as AC-AF plate. To exclude the influence of the antifouling coating, the test plate used to test the capture of biofouling organisms was coated only with an anticorrosive coating product and marked as AC plate.
The test plate was constructed considering the acceleration and deceleration section of the cleaning unit, and the test area within the test plate was set to be at least 1.5 times the width of the cleaning unit. Artificial barnacles were attached according to the fouling rating using the adhesives selected in the adhesion strength test (Figure 2). According to New Zealand’s guidelines on the conduct of in-water biofouling surveys for domestic vessels (MPI, 2020), organism attachment (and hence biofouling) can be scattered or localized. Artificial barnacles were attached to 15% of the test area based on a fouling rating of 2, the minimum rating that requires capture by in-water cleaning systems in the revised IMO biofouling guidelines (IMO, 2023a). The tests were conducted by assuming the scattered distribution as the worst condition.
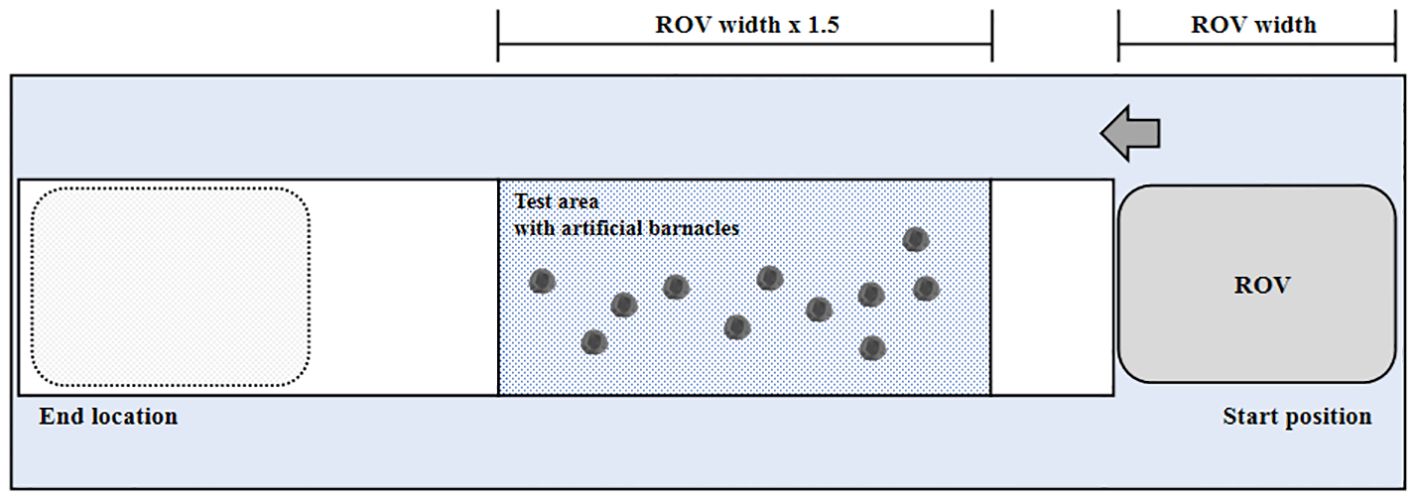
Figure 2. Schematic configuration of the test specimen for the ex situ capture efficacy test. ROV, remotely operated vehicle.
2.4 In-water cleaning system
The in-water cleaning system used in our tests was a proprietary cleaning unit developed by TAS GLOBAL (Gangseo-gu, Busan, South Korea) and is not yet available. This device removes biopollution and captures the cleaning debris in a transport hose and brush specific to the type of biofouling. In our tests, the cleaning unit was equipped with rotating brushes made of urethane and NC nylon to remove the artificial barnacles from the test plate and a watertight skirt to capture the cleaning debris. The capture equipment comprised a transport pump (100 ton/h capacity) and a 50 m transport hose to direct the captured debris to a treatment system on land.
2.5 Analysis of the change in biocide concentration
The biocide concentration change test was conducted at the testing tank facility of the Korea Marine Equipment Research Institute. The size of the test tank was 24 m (L) × 8 m (B) × 8 m (H). The tank was equipped with a 400 m3/h water intake, a drain pump, and a crane for installing the test plate. The AC-AF plate was installed according to the specifications, and the artificial barnacles were then attached to simulate a fouling rating of 2. To examine the quantification of the biocides, sampling was performed at three locations: near the test plate before and after testing (A1 and A2, respectively) and at the cleaning unit during cleaning (A3) (Figure 3). To obtain the sample near the cleaning unit, the rotation direction of the brushes was first determined, and the most likely debris discharge direction considering the design and operation of the equipment was determined; a separate sampling hose was then fixed to this discharge location, and the waste generated during the test period was continuously collected in the capture tank. Samples of approximately 20 L were collected for the analysis of biocides. Replicate samples were obtained at the three sampling locations (A1, A2, and A3) during cleaning, and water quality parameters were measured.
2.6 Capture efficacy test
An AC test plate was used in the capture efficacy test. A stainless steel filter (pore size, 50 μm) was installed in the capture tank to collect the captured artificial barnacles. The filtrate was dried at room temperature for more than 24 h, and the dry weight was measured. The capture efficiency (as a percentage) was calculated as the dry weight of the captured barnacles relative to that of the removed barnacles. The weight of the removed barnacles was calculated by subtracting the weight of the remaining barnacles on the test plate after cleaning from that of the attached barnacles before cleaning.
2.7 Analytical method
Before and after the cleaning test, the water quality and biocides were analyzed at the three sampling locations in the test tank (A1, A2, and A3). The water temperature, salinity, pH, and turbidity before and after cleaning were measured using a multi-parameter water quality instrument (DS5: Hydrolab, Loveland, CO, USA). To measure the TSS content in natural seawater, 250-ml samples were filtered through a preweighed glass fiber filter (GF/F filter, Whatman) and dried for at least 1 h at 105°C. The particle size distribution of the captured artificial barnacle debris was analyzed according to ISO 13320 using a laser diffraction particle size analyzer (Mastersizer-3000, Malvern Instruments, Great Malvern, UK). The concentrations of copper and zinc, the main biocide components, were analyzed using an inductively coupled plasma atomic emission spectrometer (Agilent 5110, Agilent Technologies, Santa Clara, CA, USA).
3 Results and discussion
3.1 Compressive and adhesion strength tests
Shell strength is a key factor in creating artificial barnacles. To achieve compressive strengths comparable with those of actual barnacles, the artificial barnacles used here were heat-treated. Compressive strength decreased as heat treatment time increased (Figure 4). The compressive strength of artificial barnacles decreased significantly with increasing heat treatment time due to the change in the barnacle’s acrylic structure. The compressive strength was 35.5 ± 1.7 MPa in the unheated control and 8.8 ± 0.8 and 3.8 ± 0.1 MPa after heating at 150°C for 24 and 48 h, respectively.
The compressive strength of barnacles varies significantly among species. Based on the relationship between barnacle volume and compressive strength, the estimated compressive strength of barnacles with a base diameter of 20 mm is 0.5–4.4 MPa (Gubbay, 2009). The compressive strength of the artificial barnacles subjected to heat treatment at 150°C for 48 h (3.8 ± 0.1 MPa) was similar to this estimate. By contrast, the compressive strength of the artificial barnacles treated at 100°C for 24 and 48 h (37.3 ± 3.2 and 35.0 ± 4.3 MPa, respectively) did not differ significantly from that of the control (35.5 ± 1.7 MPa), i.e., it was significantly different from that of natural barnacles. Heating at 100°C, therefore, did not induce structural deformation, even though this temperature was close to the heat deflection temperature of acrylic (95°C).
After the compressive strength test, the fracture pattern was visually determined (Figure 5). The removal of biofouling (such as barnacles and bivalves) using a scraper or cleaning unit sometimes breaks or fragments the shell. During the compressive strength testing, the artificial barnacles treated at 150°C were crushed and broken into small pieces. This fracture pattern closely resembles that observed in real barnacles removed by the cleaning unit.
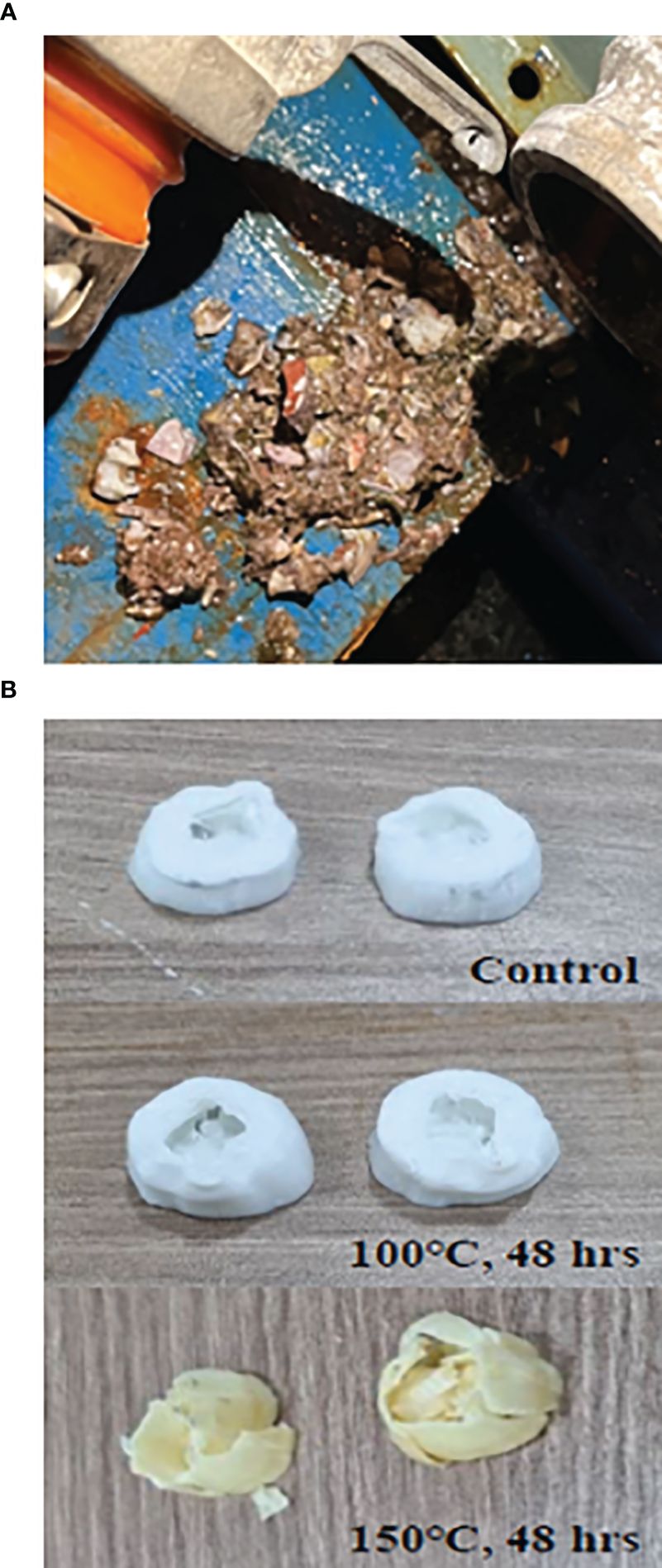
Figure 5. Shell fragmentation. (A) Actual barnacles. (B) Artificial barnacles during compressive strength testing.
Adhesion tests were conducted on five types of adhesives widely used for plastic bonding. The adhesive should exhibit high adhesive strength on the surface of the steel plate and at the same time have durability suitable for the marine environment. An adhesive that falls within the natural range was selected. The adhesion strength of the proteinaceous cement secreted by barnacles ranges from 0.1 to over 1 MPa (Yule and Walker, 1987; Watermann et al., 1997). There is an exponential relationship between barnacle diameter and the force required to remove the barnacles, and their adhesion strength depends on the type of substrate to which they are attached: 0.06 MPa for a silicon fouling release surface, 0.6 MPa for a copper base antifouling surface, 1.2 MPa for an epoxy surface, 1.83 MPa for a cathodically protected bronze surface, and 0.7–1.2 MPa for rough and rigid substrates (Swain et al., 1992; Swain and Schultz, 1996; Li et al., 2020; Swain et al., 2022). Based on these values, we set the barnacle adhesion strength at 0.8–1.2 MPa and conducted the adhesion strength test. Polyurethane adhesives (E) exhibited the highest adhesion strength of 1.08 ± 0.32 MPa, followed by silicone adhesives (D) with an adhesion strength of 1.01 ± 0.20 MPa. Acrylic, epoxy, and cyanoacrylate adhesives (A–C) exhibited adhesion strengths of 0.64 ± 0.17, 0.27 ± 0.04, and 0.54 ± 0.11 MPa, respectively, as determined with Equation 1 (Table 2). Polyurethane and silicone were thus the strongest adhesives in this study. Given the presence of chloride ions, these two materials are also the most suitable adhesives for seawater conditions. Polyurethane exhibits strong adhesion in air, humid environments, and water. Solvent-based or water-soluble adhesives can reach an adhesion strength of more than 1 MPa within a short curing time (Lu et al., 2024). Aqueous conditions, characterized by high humidity and seawater, are typically unfavorable for most adhesives, as they may affect their adhesion and durability. When designing a test of the efficacy of biofouling debris capture, it is, therefore, important to select the appropriate adhesive for the specific test design.
3.2 Biocide concentration before and after cleaning
In-water cleaning was performed using the AC-AF plate with attached artificial barnacles. The target biocides were copper and zinc, the most commonly used in commercial shipping (Dafforn et al., 2011; Wallström et al., 2011). No dissolved or particulate zinc was observed during the test period. No dissolved copper was detected; however, 0.030 mg/L of particulate copper was detected at A2, suggesting that copper can be released into the water during in-water cleaning (Table 3). In many cases, however, dissolved and particulate biocide can be released into the water during in-water cleaning (Tamburri et al., 2020; Soon et al., 2021). Therefore, regulations that specify the acceptable levels of debris release are required for in-water cleaning in the marine environment.
South Korea still lacks a clear emissions standard for biocides released during in-water cleaning. The country’s marine environmental standards are based on the Marine Environment Management Act; biopollution and biocides generated during biofouling removal may thus correspond to the pollutants specified in Article 22 of this act. According to this act and notices from the Ministry of Oceans and Fisheries, marine environmental standards are divided into those related to seawater quality, submarine sediments, and marine water conditions. These standards specify a maximum copper level of 0.003 mg/L (short-term standard) for marine ecosystem protection and 0.020 mg/L for human health protection. For zinc, these levels are 0.034 mg/L (short-term standard) for marine ecosystem protection and 0.100 mg/L for human health protection. The particulate copper concentrations before and after our test were below the method detection limit (0.020 mg/L), the same as the maximum allowable short-term copper level. For this reason, it was difficult to check whether water quality standards were met. However, the average concentration of particulate copper during cleaning (0.030 mg/L) exceeded the short-term limits for marine ecosystem protection and human health protection. This indicates that copper is not adequately captured, releasing unsafe environmental levels. Therefore, capture efficacy must be improved to reduce copper concentrations to an acceptable level.
3.3 Capture efficacy test
The discharge of biofouling is an important source of biological pollution in marine ecosystems. It is critical, therefore, to properly capture, treat, and discharge debris removed from the hull. In the aquatic environment, it is difficult to quantify the capture of the biofouling removed from the hull due to the difficulty in conducting tests controlling for variables such as water flow around the ship and marine conditions. Therefore, this study used a test plate with attached artificial barnacles to examine capture efficacy and environmental impacts in a controlled environment. The capture efficacy test was conducted using the test plate coated only with an anticorrosive (AC plate).
In this experiment, natural seawater was obtained from the coast near the test tank. During the test period, the water temperature in the tank was 15–16°C, salinity was 30–31 psu, and pH was 7.7–7.8. The average TSS in the seawater before the test was 68.33 ± 4.86 mg/L, increasing to 79.17 ± 2.31 mg/L after the test. The elevated TSS before the test may have obscured the apparent impact of uncaptured cleaning debris. This high concentration may have arisen from seasonal factors, such as river discharge and typhoon events. Therefore, consideration should be given to using filtered or artificial seawater in tests to reduce unacceptable variability.
The weight of the artificial barnacles attached to the test plate before the capture efficacy test was 907.02 g, and the 653.59 g of artificial barnacle debris was collected by the filter during the test. After the test, the artificial barnacles were completely removed from most of the test plate, although in some areas, the base plate of the shells remained, apparently affecting capture efficiency (Figure 6). The weight of the barnacles remaining on the test plate was measured and found to be 85.98 g, confirming that 167.45 g was released without being captured. The weight of the removed barnacles, considering the amount attached to the test panel before the test and the amount remaining after the test, was 821.04 g, and the capture efficiency according to Equation 2 was 79.6%. The weight of 167.45 g (approximately 18.5% of the amount attached to the test plate) of uncaptured artificial barnacles may represent complete or fragmented forms, and the release of uncaptured intact viable organisms may have detrimental effects on marine waters (Hopkins and Forrest, 2008; Woods et al., 2012). For this reason, we confirmed the fragmented particle size and distribution of artificial barnacle fragments generated during in-water cleaning. The artificial barnacles were broken into small fragments by the brushes, although some retained their original shape. This incomplete removal is consistent with the partial removal occurring during actual in-water cleaning, when the barnacles’ base plate remains on the hull surface, potentially providing a substrate for further biofouling (Oliveira and Granhag, 2016). The captured particle size ranged from 76 μm to a maximum of 18 mm, with particles >3 mm representing 25.76%. Of the captured particles <3 mm in size, approximately 80% were 500–2,000 μm and 47.5% were 500–1,000 μm (Table 4). In this study, the filter pore size was 50 μm; therefore, microparticles <50 μm were not collected.
Studies have shown that capture methods for biological debris should be used as much as possible, and cleaning technologies should aim to capture debris of at least 50 μm in size (Morrisey et al., 2013; McClay et al., 2015). However, the current processed-water discharge standards for in-water cleaning systems, the BIMCO guidelines (BIMCO, 2023), propose removing >95% of particulate matter >10 μm in diameter, and the Transport Canada (2021) guidelines propose removing particles >15 μm in diameter. Accordingly, the proportion of particles >10 μm in diameter is expected to be an important basic variable for evaluating the performance of water treatments, and a pore size of no more than 10 μm should be used when quantifying barnacle capture efficacy.
4 Conclusions
To prevent the release of harmful cleaning debris into the marine environment, the in-water cleaning system must fully capture these biofouling organisms and biocides, which must be treated in an environmentally safe manner before discharge. The 2023 IMO biofouling guidelines recommend reactive cleaning with capture for ships with major biofouling (fouling rating ≥2). Proactive cleaning without capture should be conducted on the biofouling rating of less than 2 and performed in area accepted by the port authority. The BIMCO and ACT/MERC guidelines for verifying the efficacy of in-water cleaning systems suggest that the capture efficacy should be measured by comparing the water quality of the cleaning site with that of the background waters. The capture efficacy of in-water cleaning systems can vary depending on several factors (e.g., ship type, coating condition, the extent of biofouling, and water characteristics such as water transparency and ocean currents). This study provides a new method for quantifying the efficacy of capturing in-water cleaning debris by identifying the minimum requirements for capture before field testing. This method tests capture efficacy using artificial barnacles to simulate major biofouling and measures the impact of biofouling and biocides, the main pollutants generated during in-water cleaning.
Currently, the levels of biocides released during in-water cleaning are regulated by the port where the cleaning operation is performed. We therefore examined whether a specific in-water cleaning system met the relevant regulatory requirements of the local port. Although there is an ongoing discussion in South Korea regarding the acceptable thresholds of biopollution and biocide levels following biofouling cleaning, no standards have been established. However, biofouling cleaning debris can be treated as pollutants or waste under the Marine Environment Management Act. The marine environment standards for biocides, such as copper and zinc, and human health protection standards specified in related notices likely apply to biofouling debris. In our tests, the particulate copper level during in-water cleaning (0.030 mg/L) exceeded the standard for marine ecosystem protection (0.003 mg/L), indicating that in-water cleaning can harm the marine environment. The environmental risk of these released biocides can be evaluated using environmental concentration prediction models based on the predicted environmental concentration and predicted no-effect concentration. The predicted environmental concentrations must be calculated based on the specific characteristics of individual ports and water quality data. The impact of biocides in the water can be evaluated by considering environmental risk assessment results.
The efficacy of cleaning debris capture varies depending on the type of biofouling, fouling rating, coverage, marine environmental conditions, type of ship, and season. These factors are important for evaluating biofouling cleaning technologies in the field, using actual ship hulls and biofouling accumulations. The capture efficacy evaluation method developed in this study simulates major hull biofouling based on the fouling rating to overcome the limited reproducibility of field tests due to site-specific environmental conditions. This testing method can be used to quantify and evaluate the capture efficiency of in-water cleaning systems for various biofouling conditions. To determine capture efficacy with greater accuracy, it is necessary to examine the design and operation of the in-water removal equipment, and samples should be collected by installing a sampling hose or pump at the location with the highest possible emissions of biocides during cleaning.
Various sizes of debris fragments are removed during in-water cleaning. According to the BIMCO standards and guidance document published by the ACT/MERC, capture efficacy must also be verified for 10-μm fragments, because debris capture targets fragments as small as 10 μm. In the Netherlands, official permits are required for all activities involving discharge into the water, including in-water cleaning. To remove suspended metal particulates and propagules of invasive species, particles of 0.5 to 1 μm in size must be filtered for both hull and propeller cleaning (IMO, 2023c). This suggests that the relevant in-water cleaning regulations will likely need to be revised and strengthened in the future.
Before field testing, land-based testing of in-water cleaning systems in a controlled environment is necessary to determine whether they are safe for the marine environment. Evaluation of capture efficacy must be repeatable and based on a standardized method. This study aimed to measure the biocide concentration released during in-water cleaning and compare it with water quality standards set by domestic (South Korean) regulations. Environmental risk assessments of local marine waters will also be required to predict the impact of biocides on the environment. Furthermore, the study aimed to verify the capture efficiency of biofouling organism debris using artificial barnacles. This method enables quantification of the percentage capture efficiency of in-water cleaning systems. In the future, this method should be validated using continuous sampling and data evaluation during testing.
Data availability statement
The datasets presented in this study can be found in online repositories. The names of the repository/repositories and accession number(s) can be found in the article/Supplementary Material.
Ethics statement
The manuscript presents research on animals that do not require ethical approval for their study.
Author contributions
YC: Conceptualization, Methodology, Supervision, Validation, Writing – original draft, Writing – review & editing. S-JK: Formal analysis, Investigation, Methodology, Validation, Writing – review & editing. M-SK: Data curation, Investigation, Methodology, Writing – review & editing. JY: Writing – review & editing, Funding acquisition. JC: Writing – review & editing, Funding acquisition.
Funding
The author(s) declare financial support was received for the research, authorship, and/or publication of this article. This research was supported by the Korea Institute of Marine Science & Technology Promotion (KIMST) funded by the Ministry of Oceans and Fisheries, Korea (20210500).
Conflict of interest
The authors declare that the research was conducted in the absence of any commercial or financial relationships that could be construed as a potential conflict of interest.
Publisher’s note
All claims expressed in this article are solely those of the authors and do not necessarily represent those of their affiliated organizations, or those of the publisher, the editors and the reviewers. Any product that may be evaluated in this article, or claim that may be made by its manufacturer, is not guaranteed or endorsed by the publisher.
Supplementary material
The Supplementary Material for this article can be found online at: https://www.frontiersin.org/articles/10.3389/fmars.2024.1404472/full#supplementary-material
References
BIMCO (2023). Procedures for testing and certification of in-water cleaning companies. Available online at: https://www.bimco.org/about-us-and-our-members/publications/procedure-for-independent-testing-and-certification-of-in-water-cleaning-companies (Accessed November 15, 2023).
Buhaug Ø., Corbett J. J., Endresen Ø., Eyring V., Faber J., Hanayama S. (2009). Second IMO GHG Study (London: International Maritime Organization), 220.
Cao S., Wang J., Chen H., Chen D. (2011). Progress of marine biofouling and antifouling technologies. Chin. Sci. Bull. 56, 598–612. doi: 10.1007/s11434-010-4158-4
Chambers L. D., Stokes K. R., Walsh F. C., Wood R. J. K. (2006). Modern approaches to marine antifouling coatings. Surf. Coatings Technol. 201, 3642–3652. doi: 10.1016/j.surfcoat.2006.08.129
Champ M. A., Seligman P. E. (1996). Organotin: Environmental fate and effects (London: Chapman and Hall). doi: 10.1007/978-94-009-1507-7
Chan F. T., MacIsaac H. J., Bailey S. A. (2015). Relative importance of vessel hull fouling and ballast water as transport vectors of nonindigenous species to the Canadian Arctic. Can. J. Fish. Aquat. Sci. 72, 1–13. doi: 10.1139/cjfas-2014-0473
Cranfield H. J., Gordon D. P., Willan R. C., Marshall B. A., Battershill C. N., Franis M. P., et al. (1998). Adventive marine species in New Zealand (Wellington: National Institute of Water and Atmospheric Research). NIWA Technical Report.
Dafforn K. A., Lewis J. A., Johnston E. L. (2011). Antifouling strategies: history and regulation, ecological impacts and mitigation. Mar. pollut. Bull. 62, 453–465. doi: 10.1016/j.marpolbul.2011.01.012
Davidson I. C., Brown C. W., Sytsma M. D., Ruiz G. M. (2009). The role of containerships as transfer mechanisms of marine biofouling species. Biofouling 25, 645–655. doi: 10.1080/08927010903046268
Demirel Y. K., Uzun D., Zhang Y., Fang H. C., Day A. H., Turan O. (2017). Effect of barnacle fouling on ship resistance and powering. Biofouling 33, 819–834. doi: 10.1080/08927014.2017.1373279
Doell S. A., Connolly R. M., Limpus C. J., Pearson R. M. (2017). Using growth rates to estimate age of the sea turtle barnacle Chelonibia testudinaria. Mar. Biol. 164, 222. doi: 10.1007/s00227-017-3251-5
Flemming H. C., Wuertz S. (2019). Bacteria and archaea on earth and their abundance in biofilms. Nat. Rev. Microbiol. 17, 247–260. doi: 10.1038/s41579-019-0158-9
Forbes D. J. (1996). Characteristics and treatment of wastewater generated during underwater hull cleaning operations of U.S. Navy ships. California: College Park. University of Maryland.
Gu Y., Yu L., Mou J., Wu D., Xu M., Zhou P., et al. (2020). Research strategies to develop environmentally friendly marine antifouling coatings. Mar. Drugs 18, 1–22. doi: 10.3390/md18070371
Gubbay S. (2009). Compressive and adhesive strengths of a variety of British barnacles. J. Mar. Biol. Assoc. 63, 541–555. doi: 10.1017/S0025315400070867
Hewitt C. L., Campbell M. L., Thresher R. E., Martin R. B., Boyd S., Cohen B. F., et al. (2004). Introduced and cryptogenic species in Port Philip Bay, Victoria, Australia. Mar. Biol. 144, 183–202. doi: 10.1007/s00227-003-1173-x
Hopkins G. A., Forrest B. M. (2008). Management options for vessel hull fouling: an overview of risks posed by in-water cleaning. ICES J. Mar. Sci. 65, 811–815. doi: 10.1093/icesjms/fsn026
IMO (2011). Guidelines for the Control and Management of Ships’ Biofouling to Minimize the Transfer of Invasive Aquatic Species (London: International Maritime Organization).
IMO (2021). Review of the 2011 guidelines for the control and management of ships’ biofouling to minimize the transfer of invasive aquatic species Resolution MEPC Vol. 290 (London: International Maritime Organization), 6. PPR 9/INF.
IMO (2023a). Guidelines for the Control and Management of Ships’ Biofouling to Minimize the Transfer of Invasive Aquatic Species (London: International Maritime Organization).
IMO (2023b). Review of the 2011 guidelines for the control and management of ships’ biofouling to minimize the transfer of invasive aquatic species Resolution MEPC Vol. 207 (London: International Maritime Organization). PPR 10/5.
IMO (2023c). Information about the best practice of in-water cleaning with capture in the Kingdom of the Netherlands (London: International Maritime Organization), 35. MEPC 81/INF.
Kirkiz I., Cavas L. (2023). First barnacle (Amphibalanus amphitrite) adhesion strength data on the self-polishing coatings off the Aegean sea. ACS Omega. 8, 33675–33683. doi: 10.1021/acsomega.3c03948
Li C., Wang K., Jia P., Wang L., Wang X., Yun F. (2020). Analysis of removing barnacles attached on rough substrate with cleaning robot. J. Mar. Sci. Eng. 8, 569. doi: 10.3390/jmse8080569
Lu Y., Aleksander C., Boxin Z. (2024). Solvent-free urethane-based prepolymer as a versatile underwater adhesive material. Chem. Eng. J. 481, 148487. doi: 10.1016/j.cej.2023.148487
McClay T., Zabin C., Davidson I., Young R., Elam D. (2015). Vessel biofouling prevention and management options report. Available online at: https://apps.dtic.mil/dtic/tr/fulltext/u2/a626612.pdf (Accessed January 2021).
Morrisey D., Gadd J., Page M., Lewis J., Bell A., Georgiades E. (2013). In-water cleaning of vessels: biosecurity and chemical contamination risks (Wellington: Ministry for Primary Industries). New Zealand Ministry for Primary Industries Technical Paper No. 2013/11.
Morrisey D., Inglis G., Tait L., Woods C., Lewis J., Georgiades E. (2015). Procedures for evaluating in-water systems to remove or treat vessel biofouling. Available online at: http://www.mpi.govt.nz/document-vault/10811 (Accessed February 11, 2018).
MPI (2020). Conduct of in-water biofouling surveys for domestic vessels (Wellington: Ministry for Primary Industries).
Oliveira D., Granhag L. (2016). Matching forces applied in underwater hull cleaning with adhesion strength of marine organisms. J. Mar. Sci. Eng. 4, 66–78. doi: 10.3390/jmse4040066
Rainbow P. S., Blackmore G. (2001). Barnacles as biomonitors of trace metal availabilities in Hong Kong coastal waters: changes in space and time. Mar. Environ. Res. 51, 441–463. doi: 10.1016/S0141-1136(00)00254-3
Schultz M. P. (2007). Effects of coating roughness and biofouling on ship resistance and powering. Biofouling. 23, 331–341. doi: 10.1080/08927010701461974
Scianni C., Georgiades E. (2019). Vessel in-water cleaning or treatment: identification of environmental risks and science needs for evidence-based decision making. Front. Mar. Sci. 6. doi: 10.3389/fmars.2019.00467
Soon Z. Y., Jung J. H., Loh A., Yoon C., Shin D., Kim M. (2021). Seawater contamination associated with in-water cleaning of ship hulls and the potential risk to the marine environment. Mar. pollut. Bull. 171, 112694. doi: 10.1016/j.marpolbul.2021.112694
Swain G., Erdogan C., Foy L., Gardner H., Harper M., Hearin J., et al. (2022). Proactive in-water ship hull grooming as a method to reduce the environmental footprint of ships. Front. Mar. Sci. 8. doi: 10.3389/fmars.2021.808549
Swain G., Griffith J. R., Bultman J. D., Vincent H. L. (1992). The use of barnacle adhesion measurements for the field evaluation of non-toxic foul release surface. Biofouling 6, 105–114. doi: 10.1080/08927019209386216
Swain G., Schultz M. P. (1996). The testing and evaluation of non-toxic antifouling coatings. Biofouling 10, 187–197. doi: 10.1080/08927019609386279
Sylvester F., Kalaci B., Leung B., Lacoursiere-Roussel C., Murray C., Choi F. M., et al. (2011). Hull fouling as an invasion vector: Can simple models explain a complex problem? J. Appl. Ecol. 48, 415–423. doi: 10.1111/j.1365-2664.2011.01957.x
Tamburri M. N., Davidson I. C., First M. R., Scianni C., Newcomer K., Inglis G. J. (2020). In-water cleaning and capture to remove ship biofouling: an initial evaluation of efficacy and environmental safety. Front. Mar. Sci. 7. doi: 10.3389/fmars.2020.00437
Thomas K. V., Mchugh M., Waldock M. (2002). Antifouling paint booster biocides in UK coastal waters: inputs, occurrence and environmental fate. Sci. Total Environ. 293, 117–127. doi: 10.1016/S0048-9697(01)01153-6
Transport Canada (2021). Draft Voluntary Guidance for Relevant Authorities on In-water Cleaning of Vessels for Consultation. Available online at: https://tc.Canada.ca/en/corporate-services/consultations/consultation-draft-voluntary-guidance-relevant-authorities-water-cleaning-vessels (Accessed September 2021).
Wallström E., Jespersen H. T., Schaumburg K. (2011). A new concept for anti-fouling paint for yachts. Prog. Org. Coat. 72, 109–114. doi: 10.1016/j.porgcoat.2011.03.001
Watermann B., Berger H.-D., Sönnichsen H., Willemsen P. (1997). Performance and effectiveness of non-stick coatings in seawater. Biofouling 11, 101–118. doi: 10.1080/08927019709378323
Woods C. M., Floerl O., Jones L. (2012). Biosecurity risks associated with in-water and shore-based marine vessel hull cleaning operations. Mar. pollut. Bull. 64, 1392–1401. doi: 10.1016/j.marpolbul.2012.04.019
Keywords: biofouling, in-water cleaning, capture efficacy test, artificial barnacle, capture efficiency
Citation: Cho Y, Kim S-J, Kim M-S, Yang J and Choi J (2024) Development of capture efficacy test method for in-water cleaning system using artificial barnacles. Front. Mar. Sci. 11:1404472. doi: 10.3389/fmars.2024.1404472
Received: 21 March 2024; Accepted: 16 July 2024;
Published: 09 August 2024.
Edited by:
James Scott Maki, Marquette University, United StatesReviewed by:
Emily Ralston, Florida Institute of Technology, United StatesEric Holm, Naval Surface Warfare Center Carderock Division, United States
Copyright © 2024 Cho, Kim, Kim, Yang and Choi. This is an open-access article distributed under the terms of the Creative Commons Attribution License (CC BY). The use, distribution or reproduction in other forums is permitted, provided the original author(s) and the copyright owner(s) are credited and that the original publication in this journal is cited, in accordance with accepted academic practice. No use, distribution or reproduction is permitted which does not comply with these terms.
*Correspondence: Yukyeong Cho, eWtjaG9Aa29tZXJpLnJlLmty