- 1Department of Plant, Food, and Environmental Sciences, Faculty of Agriculture, Dalhousie University, Truro, NS, Canada
- 2Department of Plant Protection, Phytopathology Unit, École Nationale d'Agriculture de Meknès, Rte. HajKaddour, Meknes, Morocco
The ovule is a plant structure that upon fertilization, transforms into a seed. Successful fertilization is required for optimum crop productivity and is strongly affected by environmental conditions including temperature and precipitation. Climate change refers to sustained changes in global or regional climate patterns over an extended period, typically decades to millions of years. These shifts can result from natural processes like volcanic eruptions and solar radiation fluctuations, but in recent times, human activities—especially the burning of fossil fuels, deforestation, and industrial emissions—have accelerated the pace and scale of climate change. Human-induced climate change impacts the agricultural sector mainly through global warming and altering weather patterns, both of which create conditions that challenge agricultural production and food security. With food demand projected to sharply increase by 2050, urgent action is needed to prevent the worst impacts of climate change on food security and allow time for agricultural production systems to adapt and become more resilient. Gaining insights into the female reproductive part of the flower and seed development under extreme environmental conditions is important to oversee plant evolution, agricultural productivity, and food security in the face of climate change. This review summarizes the current knowledge on plant reproductive development and the effects of temperature and water stress, soil salinity, elevated carbon dioxide, and ozone pollution on the female reproductive structure and development across grain legumes, cereal, oilseed, and horticultural crops. It identifies gaps in existing studies for potential future research and suggests suitable mitigation strategies for sustaining crop productivity in a changing climate.
1 Introduction
Climate change has exacerbated the adverse effects of environmental stress on crop production worldwide. Extreme weather events like heat waves, extreme droughts, and floods are becoming more frequent and more intense across the globe. For example, July 2023 was the hottest month on record since 1880 (National Aeronautics and Space Administration, 2023). Extreme weather can result in poor yields, or worse, crop failures. The average yield loss of cereal crops due to drought and heat stress was estimated at 9%–10% in recent decades, and the impact was greatest in developing nations compared to developed countries (Lesk et al., 2016). Together, these impacts pressure domestic and global food systems and increase the likelihood of supply chain disruptions and competition for increasingly limited resources (https://www.ccacoalition.org).
Grain (or seed) number and grain weight are two key yield components in grain crops. A grain is developed through the union of two sperm cells delivered by a pollen grain and two female gametes (the egg and central cells) in the ovule in a process known as double fertilization (Davies et al., 2018). The world’s leading crops - cereals, oilseeds, and pulses, are produced through sexual reproduction, which involves the fusion of male and female gametes to form seeds. These seeds are the grains or edible parts of the crops that ultimately contribute to the world’s food supply. Successful pollination and fertilization (seed set) play a key role in crop productivity and depend greatly on the environmental conditions in which these processes take place.
Reproduction is the most sensitive developmental stage to hot and cold temperatures, drought, combined heat and drought, and salinity stresses (Zinn et al., 2010; Suzuki et al., 2014). Adverse impacts of high-temperature stress on the morphological and quantitative parameters of pollen grains have been reported in many crops, such as maize (Zea mays) (Wang et al., 2019, 2021b), wheat (Triticum aestivum) (Khan et al., 2022), barley (Hordeum vulgare) (Sakata et al., 2010), rice (Oryza sativa) (Endo et al., 2009), field pea (Pisum sativum) (Lahlali et al., 2014; Jiang et al., 2015, 2019a,b), common bean (Phaseolus vulgaris) (Porch and Jahn, 2001; Prasad et al., 2002), peanut (Arachis hypogaea) (Prasad et al., 1999), cotton (Gossypium hirsutum) (Song et al., 2015), flax (Linum usitatissimum) (Cross et al., 2003), tomato (Solanum lycopersicum) (Giorno et al., 2013), and pepper (Capsicum annum) (Erickson and Markhart, 2002). Drought, like heat stress, can adversely impact male and female organs and contribute to yield reduction in crops (Ishimaru et al., 2022). The effects of drought stress during the reproductive stage are manifested in the form of degenerated meiocytes, disorientated microspores, expanded anther wall layers with a vacuolized tapetum, early anther dehiscence, and non-viable pollen (Giorno et al., 2013; De Storme and Geelen, 2014).
Depending on its concentration, the duration of exposure, the plant’s sensitivity, and the plant’s developmental stage, tropospheric ozone (O3) can be a source of stress and can have damaging effects on reproductive development in flowering plants (Pasqualini et al., 2011; Duque et al., 2021b). Some of the proposed mechanisms by which ozone might affect reproductive performance include (i) reduction in photosynthetic efficiency through damaging chloroplasts and reducing the plant’s overall energy production (ii) inhibition of assimilate translocation, and (iii) reduction in pollen viability (Pasqualini et al., 2011), pollen germination, and pollen tube growth and increase in abscission of reproductive sites (Fiscus et al., 2005; Leisner and Ainsworth, 2012). Ozone has been shown to alter the timing of the flowering onset (Rämö et al., 2007; Hayes et al., 2012; Duque et al., 2021a) and to decrease the number of flowers (Hayes et al., 2012; Duque et al., 2021a), which may alter reproductive success. According to Stabler (2016), exposure of broad bean (Vicia faba) plants to ozone reduced the amount of pollen produced and decreased its protein content.
Since the start of the industrial revolution, burning fossil fuels like coal and oil has increased the concentration of atmospheric carbon dioxide (CO2). In flowering plants, elevated levels of CO₂ can have both positive and negative effects on reproductive development, depending on the plant species (C3 or C4), environment, and interaction with other factors such as temperature and nutrient availability. In theory, elevated levels of CO2 increase photosynthesis if the other essential factors such as sunlight, water, temperature, or nutrients are available in abundance (Boretti and Florentine, 2019). This can lead to a slower increase in the atmospheric concentration of CO2, compared to what would be expected solely based on global CO2 emissions (Le Quéré et al., 2009). Additionally, higher atmospheric CO2 levels have been shown to reduce the water plants lose through transpiration, allowing plants to survive in drier conditions (Swann et al., 2016). These two processes can improve the uptake of CO2 by the biosphere, thus increasing the land carbon stock—a phenomenon known as the CO2 fertilization effect (Chen et al., 2022). Across 79 crop and undomesticated (wild) species, CO2 enrichment (500–800 μL l-1) resulted in substantial increases in number of flowers (+19%), fruits (+18%), and seeds (+16%) as well as greater individual seed mass (+4%) and total seed mass (+25%) than control treatments (Jablonski et al., 2002). Similarly, elevated CO2 significantly increased the number of flowers for the tropical legume forage species Stylosanthes capitata Vogel (Alzate-Marin et al., 2021). Even though the weight of evidence favors the idea that the male reproductive organ is more sensitive to abiotic stress than its female counterpart in several crops such as maize (Wang et al., 2019), sorghum (Sorghum bicolor) (Djanaguiraman et al., 2018b), barley (Callens et al., 2023), and field pea (Jiang et al., 2019a), evidence for species-specificity of reproductive organs’ sensitivity to abiotic stress is accumulating (Hedhly, 2011; Wang et al., 2021a,b). This review focuses on the status of the pistil’s morphological, physiological, and molecular changes subjected to abiotic stress, and bridging the gap between basic research and the applied field of agronomy.
2 Plant reproductive development
2.1 Male gametophyte development
Male gametophyte (also referred to as microgametophyte or pollen grain) development in anthers occurs over two distinct sequential phases, microsporogenesis and microgametogenesis (Huang et al., 2021). During microsporogenesis, diploid microspore mother cells (also called pollen mother cells or microsporocytes) undergo meiotic division to produce tetrads of haploid microspores. Unicellular microspores are released from tetrads and undergo microgametogenesis (Pandey et al., 2022). This process involves asymmetric mitosis (mitosis I) that generates two unequal cells, the larger vegetative and the smaller generative cells. The generative cell goes through symmetric mitosis (mitosis II) to produce two sperm cells (Figure 1A). Approximately 70% of male gametophytes of flowering plants have a sexually immature bicellular state when they are dispersed as pollen. The remainder have tricellular pollen containing fully formed sperm at anthesis (Williams et al., 2014). In species with bicellular pollen grains, pollen mitosis II occurs in the growing pollen tube within the pistil (Berger and Twell, 2011; Dresselhaus and Franklin-Tong, 2013), such as in field pea (Jiang et al., 2019b).
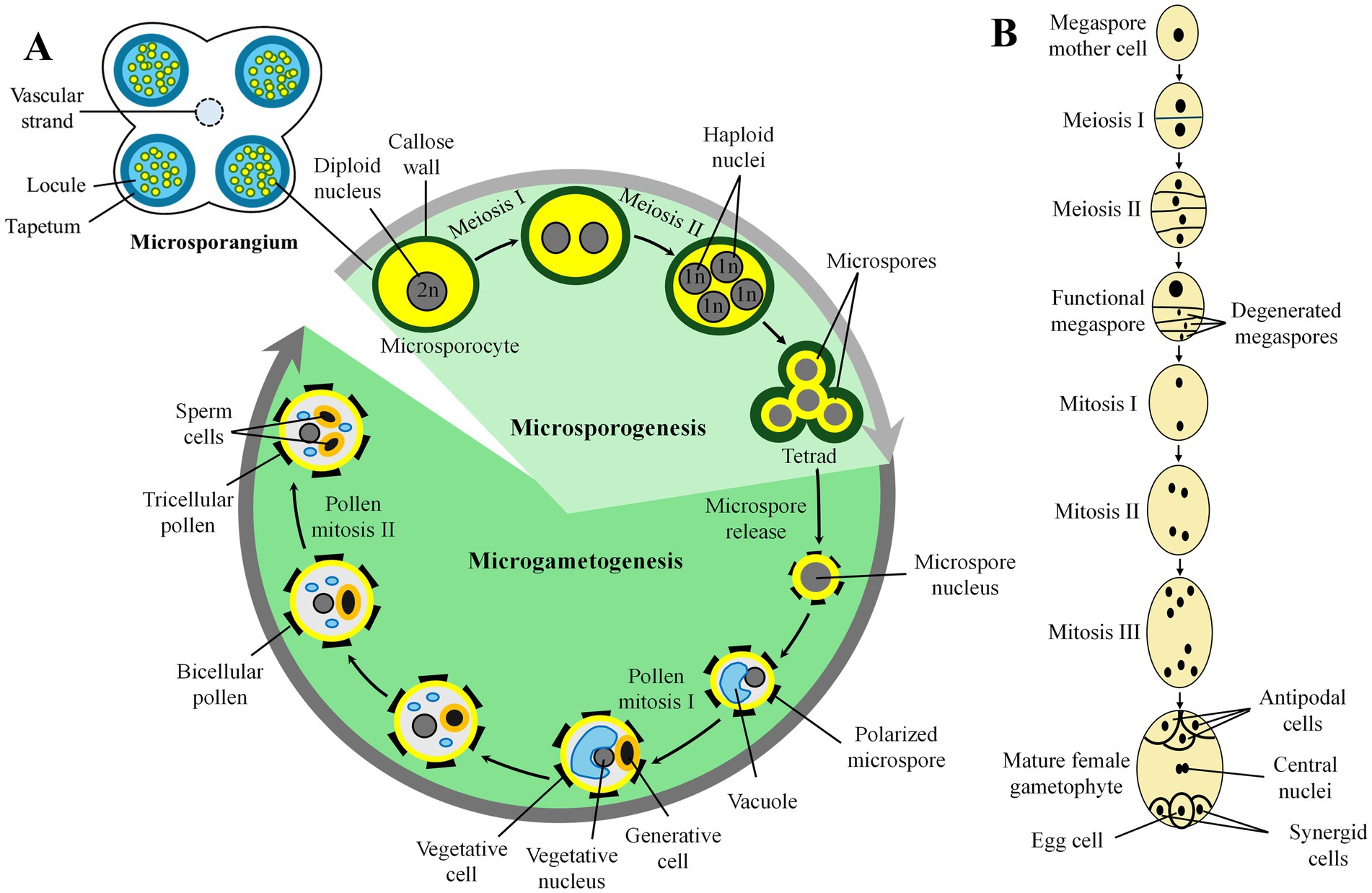
Figure 1. Schematic of (A) male gametophyte (pollen grain) development and (B) female gametophyte (embryo sac) in flowering plants.
2.2 Female gametophyte development
The gynoecium is the female reproductive portion and the innermost whorl of the flower in angiosperms (or flowering plants) and predominantly consists of one pistil (Cucinotta et al., 2020). The pistil has three main parts: the ovary at the base where ovules are housed, the style through which the pollen tubes grow, and the stigma on which the pollen grains germinate. The female gametophyte (also called embryo sac or megagametophyte) develops within the ovule over two phases, megasporogenesis and megagametogenesis. More than 15 different patterns of female gametophyte development have been characterized based on the variations in cytokinesis during meiosis, the number and pattern of mitotic divisions, and the pattern of cellularization (Yadegari and Drews, 2004). The Polygonum-type female gametophyte is the most typical type of embryo sac in many economically important families including Gramineae, Leguminosae, Solanaceae, Brassicaceae, and Malvaceae (Willemse and Van Went, 1984; Haig, 1990; Huang et al., 1998; Drews and Koltunow, 2011). It possesses seven cells with four cell types: one egg cell and two synergid cells at the micropylar pole, one big central cell with two polar nuclei in the middle, and three antipodal cells at the chalazal pole. Chalaza is the basal part of the body of the ovule from which the inner and outer integuments develop, and these finally envelop and protect the embryonic sac (Figure 1B). It is present opposite the micropylar end of an ovule (Davies et al., 2018).
During megasporogenesis, the diploid megaspore mother cell (megasporocyte) undergoes meiosis resulting in the formation of four megaspore nuclei (Drews and Koltunow, 2011). There are three main types of megasporogenesis in angiosperm, namely monosporic, bisporic, and tetrasporic (Haig, 2020). These three types differ mainly in whether cell plate formation occurs after these divisions, thus determining the number of meiotic products that affect the development of the mature female gametophyte (Yadegari and Drews, 2004). In the monosporic type of megasporogenesis, meiosis is followed by cytokinesis, leading to four megaspore cells. Subsequently, three megaspores, generally the micropylar-most megaspores, undergo cell death (Drews and Koltunow, 2011). In the bisporic type of megasporogenesis, meiosis I is followed by cytokinesis, but not meiosis II, which leads to two-nucleate megaspores, one of which degenerates. In the tetrasporic pattern, cytokinesis does not occur at all after meiosis, resulting in a single cell with four haploid nuclei. Thus, these three patterns give rise to a single functional megaspore that contains one (monosporic), two (bisporic), or four (tetrasporic) haploid megaspore nuclei (Haig, 2020). The monosporic pattern is the most common form and is represented within the Polygonum pattern (Willemse and Van Went, 1984; Haig, 1990; Yadegari and Drews, 2004). The monosporic, Polygonum type of female gametophyte is typically a seven-celled structure at maturity (Yadegari and Drews, 2004). However, this structure may be modified by cell death or cell proliferation events in various species (Grossniklaus and Schneitz, 1998; Yadegari and Drews, 2004; Friedman, 2006; Drews and Koltunow, 2011; Endress, 2011a,b).
2.3 Pollination and sexual reproduction in flowering plants
Efficient and successful pollination and fertilization are key processes for crop productivity (Suzuki, 2009; Bleckmann et al., 2014). Pollination is initiated after a pollen grain is transferred from the anther to a receptive stigma via various agents, such as pollinators, wind, or direct contact. Most pollen grains are metabolically quiescent and highly desiccated, ranging from 15 % to 35% water content when released from the anthers (Heslop-Harrison, 1979; Buitink et al., 2000). Stigma plays an important role in pollination and is composed of stigmatic papillae, which are divided into wet and dry types depending on the presence or absence of secretions at the stigma surface (Heslop-Harrison and Shivanna, 1977; Heslop-Harrison, 1981). Wet stigmas are covered with surface cells that often lyse to release a viscous surface secretion containing proteins, lipids, polysaccharides, and pigments (Edlund et al., 2004). Pistils in Leguminosae, Solanaceae, and Orchidaceae have wet stigmas (Zheng et al., 2018). Dry stigmas lack copious surface secretion (Edlund et al., 2004) and are further divided into unicellular and multicellular types (Katano and Suzuki, 2021). Stigmas in Gramineae, Brassicaceae, and Asteraceae are of the dry type (Elleman et al., 1992; Zheng et al., 2018).
Upon pollen adhesion, water, nutrients, and other small molecules are transported rapidly into the pollen grain from the stigma exudate (in the wet stigma type), leading to pollen hydration (Richards, 1997; Edlund et al., 2004). Following pollen hydration, the pollen tube germinates and penetrates the stigma cell wall and then passes through the nutrient-rich extracellular matrix of the transmitting tract toward the female gametophyte (Johnson and Preuss, 2002; Rejón et al., 2016). The pollen tube eventually enters the embryo sac in the ovule from the micropyle by growing between the two halves of the filiform apparatus of the synergid cells. Just after passing the filiform apparatus, wall deposition at the tip of the pollen tube appears disordered, resulting in pollen tube discharge. One of the two sperm cells fuses with the egg cell to develop into the embryo, and the other sperm cell fuses with the polar nuclei to develop into the endosperm during double fertilization (Faure, 2001; Higashiyama, 2010; Drews and Koltunow, 2011).
The ovule is the structure that gives rise to and contains the female reproductive cells. It consists of three parts: the integument, forming the outer layer; the nucellus (or remnant of the megasporangium), a diploid nutritive tissue; and the female gametophyte in the center of the ovule. Embryo development in a typical dicot includes the proembryo stage, globular stage, cotyledon stage, and mature seed. In contrast, embryo development in a typical monocot includes proembryo stage, globular stage, scutellar stage, coleoptilar stage, and mature seed stage (Davies et al., 2018). In most cases, antipodals at the chalazal end degrade soon before or soon after fertilization (Panoli et al., 2015). In other cases (such as many monocots, particularly grasses), the antipodals can continue to divide and develop 40–100 antipodal cells, for example in maize (Zea mays) (Diboll, 1968). For the latter, antipodals may provide nutrition for endosperm and embryo development (Davies et al., 2018).
3 Effects of abiotic stress on female reproductive organ
The pistilar sporophytic tissues provide an optimal environment to support several key events during plant reproductive development, including gametophyte development, pollen germination, pollen tube growth, and fertilization. To fulfill these roles, the pistil acquires adequate levels of enzymes (e.g., invertase and antioxidant enzymes), phytohormones, soluble carbohydrates, free amino acids, adenosine triphosphate (ATP), and lipids (Herrero and Hormaza, 1996; Jain et al., 2007; Deb et al., 2018; Liu et al., 2022; Shi et al., 2022). However, the pistil is prone to irreversible morphological and physiochemical changes due to changes or disruptions in climate variables (heat and drought stress, flooding, ozone pollution, and elevated CO2), which results in up to 100% yield loss depending on crop species, genotype, and stress conditions (Table 1). These disruptions collectively contribute to reduced fertilization success and can limit seed production and fruit set through different mechanisms such as impaired pollen tube growth and ovule number. For example, heat stress modifies source-sink relations, reduces the kernel-filling stage, and produces unfilled or aborted seeds in several globally important crops (Kaushal et al., 2016). Exposure to high temperatures during seed filling can accelerate the senescence of seeds, decrease seed set and seed weight, and lead to yield loss because plants tend to divert energy and resources such as photosynthates to deal with heat stress (Bueckert and Clarke, 2013). Schematic of some major impacts of changes or disruptions in climate variables (heat and drought stress, flooding, ozone pollution, and elevated CO2) on female reproductive organs in major food sources selected in this review is shown in Figure 2.
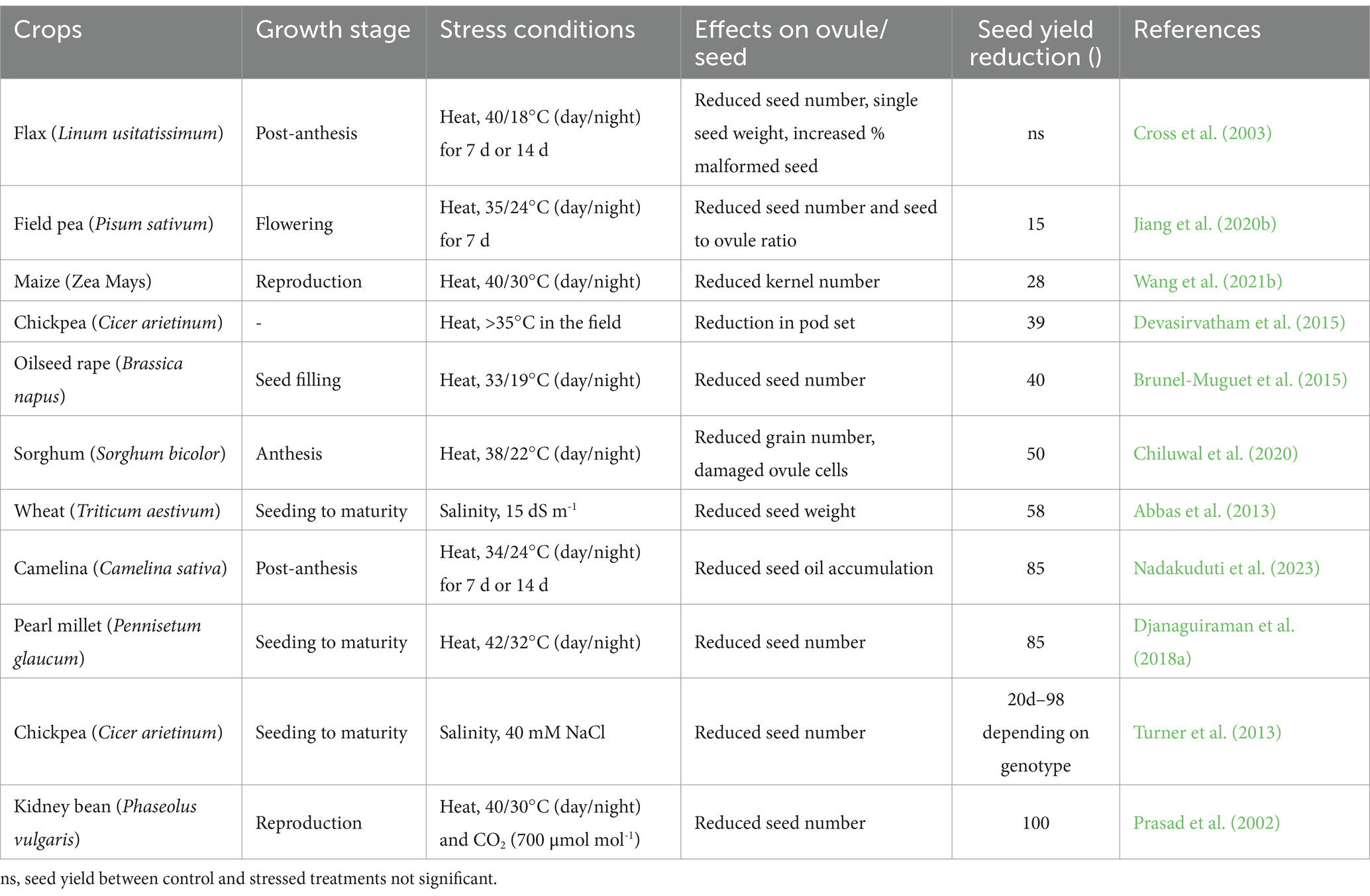
Table 1. Effects of abiotic stress on ovule/seed development and seed yield reduction in different field crops.
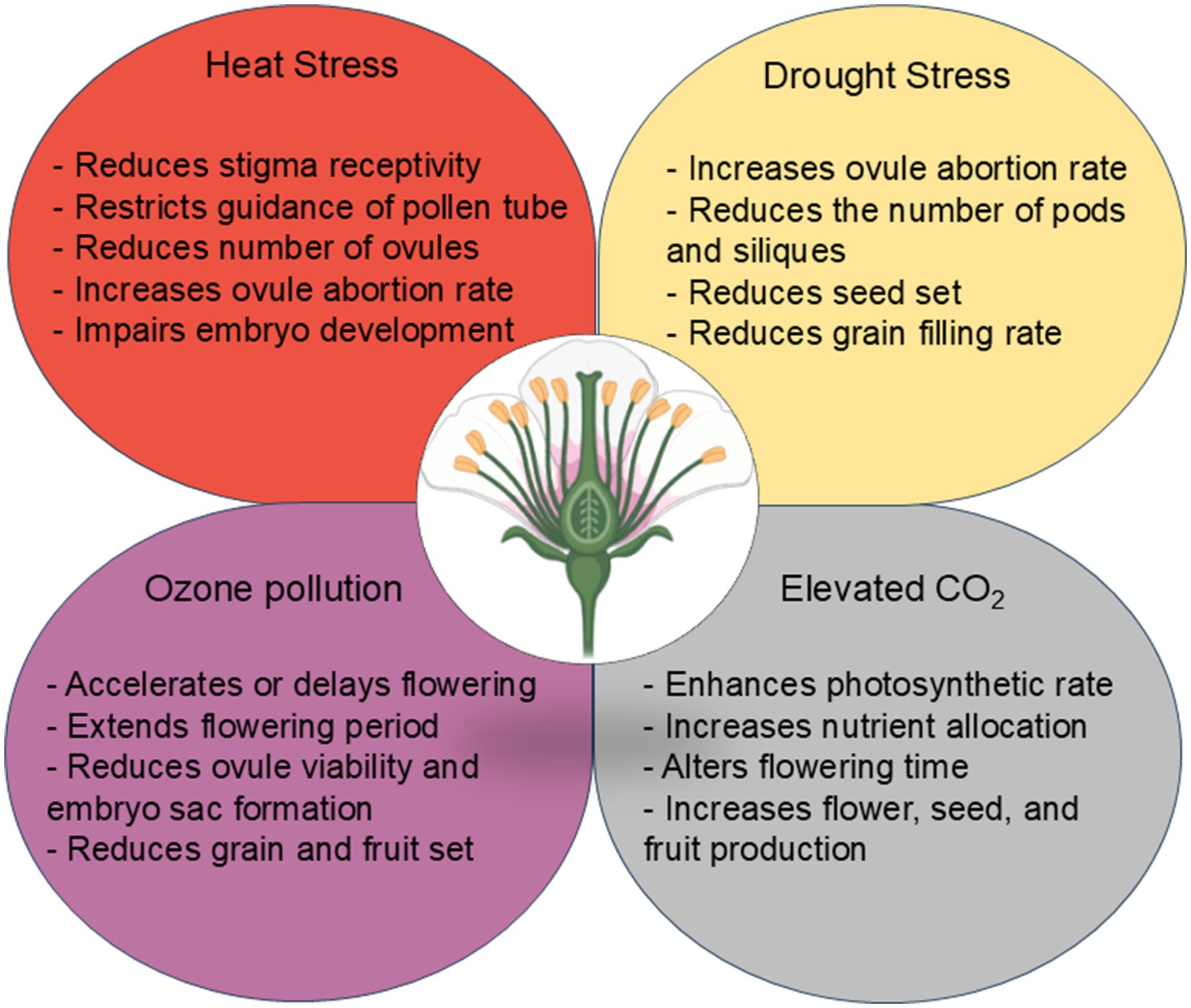
Figure 2. Schematic of some major impacts of climate change on female reproductive organs in crop species.
3.1 Effect of abiotic stress on the female reproductive organ in grain legumes
Heat-induced morphological changes in the pistil were reported in several crops. Exposure of a heat-sensitive chickpea (Cicer arietinum) genotype, ‘ICC 4567’, to high temperatures (39.4/27.2°C day/night temperatures) against a control treatment (27/16°C) for 7 days during pre-anthesis shortened the style length and led to ovule and ovary abnormalities (Devasirvatham et al., 2013). Heat stress accelerated seed abortion in all ovule positions within pods in field pea (Jiang et al., 2020). In half of the cultivars tested, ovules at the pod’s medial and stylar-end positions were more likely to develop into seeds compared to basal ovules. Pea cultivars with small seed size such as ‘40–10’ and ‘Naparnyk’ were able to retain the most ovules and seeds per pod compared to large seed size cultivars, and large-seeded cultivars like ‘MFR043’ aborted seeds when exposed to heat in growth chambers (Jiang et al., 2020). Under field conditions, the field pea cultivars ‘CDC Meadow’, ‘CDC Sage’, and ‘Carnival’ (identified as having a low and medium proportion of ovules developing into seeds per pod) which were subjected to heat stress during flowering phases displayed higher levels of ovule abortion and a significant decrease in ovaries, ovules, and embryo sac size than cultivars ‘40–10’, ‘Naparnyk’, and ‘MFR043’ (identified as having a high proportion of ovules developing into seeds per pod) (Osorio et al., 2023). Heat stress (>32/20°C) accelerated pod abortion as well as shortened the duration of seed set, leading to yield loss in lentil (Bhandari et al., 2016). In addition, heat stress was reported to decrease ovule number and ovule viability, and result in fertilization failure in common bean (Monterroso and Wien, 1990; Ormrod et al., 1967). Cold stress may also cause morphological changes in female reproductive cells and organs. Exposure of sensitive chickpea genotypes to low temperatures (15/0°C) significantly reduced ovary and style size and delayed floral development (Srinivasan et al., 1999).
Seed-filling processes are adversely affected by heat and drought stress in all crop species, including grain legumes, resulting in reduced seed yields and quality. For example, drought stress significantly reduced seed weight per plant, average seed weight, average seed size, and number of pods in chickpeas (Nayyar et al., 2006). Drought stress during pod-filling resulted in significant reductions in seed minerals (i.e., Fe, Zn, P, and N) and seed protein content in common bean (Ghanbari et al., 2013a, 2013b). Similarly, drought stress at seed filling stage led to significant reductions in starch, protein, amino acids, and minerals (Ca, P, and Fe) in chickpea seeds (Nayyar et al., 2006).
Salinity stress is another major abiotic factor that has adverse effects on reproductive processes through ionic imbalance, osmotic stress, and oxidative stress, leading to a reduction in nutrient uptake, cell division and expansion, and photosynthesis and photoassimilates (Khataar et al., 2018; Kumari et al., 2022; Atta et al., 2023). In sensitive chickpea genotypes, reproductive development related traits, such as flower number, ovule fertilization, pod development and retention, seed number, and seed size are highly sensitive to salt stress (Flowers et al., 2010). According to Khan et al. (2017), saline stress reduced photosynthesis and decreased tissue sugars by 22%–47%; both vegetative and reproductive development were severely impaired. Turner et al. (2013) reported that salt stress increased pod abortion in sensitive chickpea genotypes, but pollen viability, in vitro pollen germination, and in vivo pollen growth was not affected.
3.2 Effect of abiotic stress on the female reproductive organ in cereals
In maize, ovule development, ear growth, and ovary/kernel size and number were highly sensitive to heat and drought stress (Danilevskaya et al., 2019; Wang et al., 2019). Exposure of maize plants to low water potentials (Ψw) for 6 days during flowering [from silk emergence (day -5 from pollination to day 1 after pollination)] led to leaf Ψw of -1.25 MPa in water-stressed plants in comparison to leaf Ψw of 0.45 MPa in control plants at pollination, resulting in embryo abortion and a substantial reduction in kernel number, with abortion being linked to restricted carbon flow to the young ovaries due to impaired photosynthesis (Zinselmeier et al., 1999). Heat stress has negatively impacted ovule development in wheat, leading to yield loss (Saini and Aspinall, 1982; Saini et al., 1983). One-third of heat-stressed ovaries before anthesis in wheat exhibited an abnormal nucellus or embryo sac (Saini et al., 1983). Some of these abnormal ovules contained small embryo sacs without complete cellular organization or lacking any recognizable cells; the development of the nucellus degenerates in some of the ovules (Saini et al., 1983). In the wheat cultivar ‘Chinese spring’, short episodes (2 d or 5 days) of heat stress (35/25°C) during reproductive development (first at 8–6 days before anthesis and second at 2–0 days before anthesis) resulted in desiccated stigmas and styles with no pollen grains and flaccid and dried ovaries (Prasad and Djanaguiraman, 2014). Likewise, exposure of pearl millet (Pennisetum glaucum) to heat stress (39/29°C) against a non-stressed treatment (30/20°C) from gametogenesis through flowering in both controlled and field environments led to deformed and desiccated styles and ovaries (Djanaguiraman et al., 2018a). Six days of heat stress (38°C and 40°C) during megasporogenesis of a heat-tolerant rice genotype, ‘N22’, and a heat-sensitive genotype, ‘IR64’, resulted in degeneration of all four megaspores instead of just three nuclei, formation of mature embryo sacs without the female germ unit, improper positioning of nuclei, and shrunken embryo sacs in the sensitive IR64 as compared to the control (30°C) treatment (Shi et al., 2022).
Cell wall invertases are important metabolic enzymes that hydrolyze sucrose into glucose and fructose and play an important role in apoplasmic unloading of sucrose and hexoses to the elongating pollen tubes and developing seeds and hence crop yield (Shen et al., 2019). The invertase activity in female reproductive organs was significantly decreased under heat stress, thus limiting the supply of sucrose and hexoses to rice spikletes (Jiang et al., 2020) and tomato fruits (Liu et al., 2016). Severe drought stress significantly reduced the invertase activity in the placental region and the ovary dry mass, leading to poor grain set and grain yield in maize (Andersen et al., 2002). Drought stress caused inhibition of invertase enzymes, downregulation of genes encoding sucrose processing enzymes, and expression of senescence-related genes in the ovary of maize, leading to ovary abortion (McLaughlin and Boyer, 2004).
Metabolite profiling of pistils during the gametogenesis stage of a heat-sensitive rice genotype, IR64, and a heat-tolerant rice genotype, N22, grown under control (30°C) and two high-temperature treatments (38°C and 40°C) for 6 days, revealed significant reductions in starch content, TCA cycle (citrate cycle) related metabolites (i.e., citric acid, fumaric acid, and succinic acid), and amino acid metabolites (i.e., 3-cyanoalanine, proline, aspartic acid, lysine, valine, and isoleucine) in the developing pistils of IR64 than N22 (Shi et al., 2022). According to Zinselmeier et al. (1995), water deficit conditions, particularly during flowering and early embryo growth, contributed to arrested embryo growth, a decreased sucrose flux, and an altered carbohydrate metabolism in the ovaries of maize.
Long-term drought stress from growth stage V7 (the beginning of the rapid growth phase and stem elongation) through R1 (silking) under field conditions caused significant transcriptional changes in ears but only minor changes in the tassel of the maize ‘B73’ inbred line (Danilevskaya et al., 2019). Many key genes involved in DNA replication, cell cycle, cell division, floral development (e.g., MADS-box and non-MADs genes), and embryo sac development (i.e., IG1; indeterminate gametophyte) were suppressed under drought stress, which ultimately led to the formation of defective ovaries and a failure to produce kernels (Danilevskaya et al., 2019). In rice, the pectinase gene family overexpressed in the male and female floral organs by high-temperature treatments and was suggested to be the direct cause of rice floral organ abortion during heat stress (Wu et al., 2015). Previous research showed that ribosome inactivating protein (RIP2) and phospholipase D (PLD1) genes were transcriptionally activated under stress conditions due to a lack of carbon sources, leading to early senescence and ovule abortion in maize (Boyer and McLaughlin, 2007). A summary of the adverse effects of heat on drought stress on the female reproductive phase, crop productivity, and grain quality in grain legumes and cereal crops is included in Figure 3.
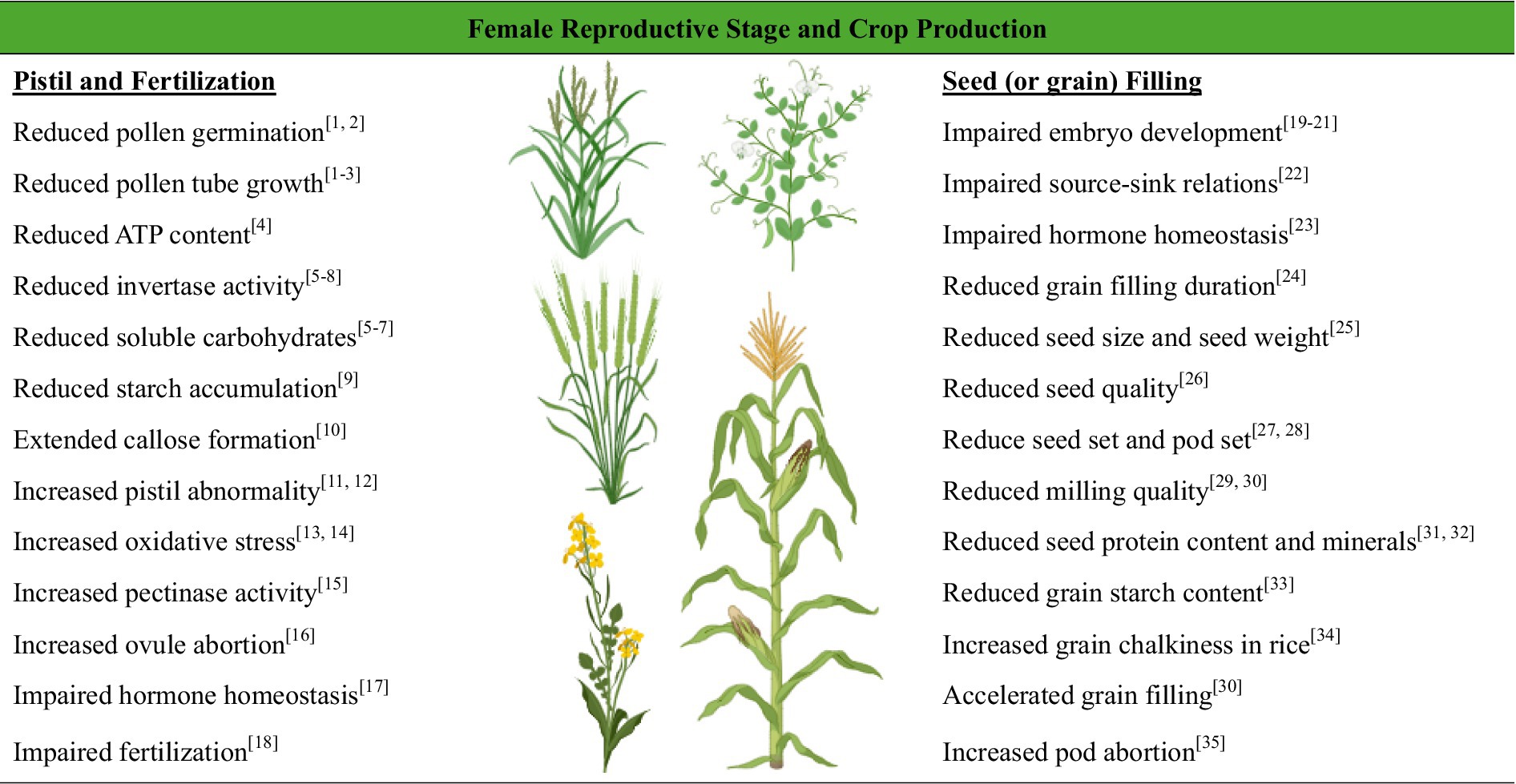
Figure 3. Adverse effects of heat and drought stress on female reproductive organs and seed (or grain) filling in crop plants. Chickpea-[1] (Kumar et al., 2013); pearl millet-[2] (Gupta et al., 2015); rice-[3] (Zhang et al., 2018a); cotton-[4] (Snider et al., 2009); tomato-[5] (Liu et al., 2016); cotton-[6] (Loka and Oosterhuis, 2016); rice-[7] (Jiang et al., 2020a); maize-[8] (Andersen et al., 2002); rice-[9] (Shi et al., 2022); pea-[10] (Jiang et al., 2019b); rice-[11] (Takeoka et al., 1991); chickpea [12] (Devasirvatham et al., 2013); pearl millet-[13] (Djanaguiraman et al., 2018a); rice-[14] (Jiang et al., 2020a); rice-[15] (Wu et al., 2015); Arabidopsis-[16] (Whittle et al., 2009); pea-[17] (Savada et al., 2017); rice-[18] (Li et al., 2015); rice-[19] (Begcy et al., 2018); spring wheat [20] (Prasad et al., 2008); common bean [21] (Vargas et al., 2021); common bean [22] (Soltani et al., 2019); pea-[23] (Ozga et al., 2009); wheat-[24] (Wardlaw, 2002); lentil-[25] (Kumar et al., 2016); soybean-[26] (Teixeira et al., 2016); common bean-[27] (Gross and Kigel, 1994); soybean-[28] (Liu et al., 2003, 2004); wheat-[29] (Hernández-Espinosa et al., 2018); [30] (Mahdavi et al., 2022); common bean- [31] (Ghanbari et al., 2013a); [32] (Ghanbari et al., 2013b); rice-[33] (Yamakawa et al., 2007); rice-[34] (Nakata et al., 2017); cool-season pulses-[35] (Bhandari et al., 2016) Created in BioRender.
Salinity stress significantly reduced the growth and productivity of cereal crops (Munns et al., 2006). In maize, salinity stress considerably reduced grain yield of maize by decreasing the number of grains and grain weight during the reproductive growth stage (Schubert et al., 2009). This was attributed to reduced photosynthesis and photoassimilates (Lohaus et al., 2000; Hiyane et al., 2010) and impaired translocation of assimilates from source to developing grains (sink) under salt stress. In wheat, salinity stress accelerates all phenological phases (Grieve et al., 1994), decreases the number of spikelets number per spike (Frank et al., 1987), and reduces grain yield (Abbas et al., 2013; Sorour et al., 2019), and grain quality traits (El Sabagh et al., 2021).
3.3 Effect of abiotic stress on female reproductive organ in oilseed crops
Under high-temperature conditions (32/26°C) most stigmas protruded significantly out of the closed flower buds in rapeseed (Brassica napus) (Polowick and Sawhney, 1988). Heat stress (32/26°C) caused abnormal embryo sac development in rapeseed – the ovule growth was not uniform along the length of the ovaries of both pollinated and unpollinated flowers (Polowick and Sawhney, 1988). Heat stress in rapeseed was associated with asynchronous embryo development, a high rate of defective embryos, accelerated embryo development, altered seed quality, induced pre-harvest sprouting, and decreased abscisic acid (ABA) levels in mature seeds (Brunel-Muguet et al., 2015; Mácová et al., 2022). Heat stress decreased seed oil content by 52% in canola, but the protein content increased under heat, drought, and a combination of heat and drought (Elferjani and Soolanayakanahally, 2018). Heat stress reduced seed oil content but increased seed sugar content in rapeseed, suggesting that the conversion of carbohydrates into triacylglycerols was affected under heat stress (Huang et al., 2019). The authors also found that reduced seed production was caused by the impairment of photosynthesis and downregulation of BnWRI1 and its downstream genes. Fewer fertile pods and seeds per pod were produced by canola florets under heat stress (Hu et al., 2024). Further, heat stress during seed development was reported to induce changes in the biosynthesis from polyunsaturated fatty acid synthesis to more monounsaturated fatty acid accumulation in camelina (Camelina sativa), leading to membrane stability to protect cell function and embryo development (Nadakuduti et al., 2023).
3.4 Effect of abiotic stress on the female reproductive organ in other horticulture crops
In apricots (Prunus armeniaca), mild heat stress (an average of 3°C higher than the control) during the last week of flower development shortened the style length and led to abnormal ovary development (Rodrigo and Herrero, 2002). Heat stress reduced ovule longevity in plum (Prunus mume) (Cerovic et al., 2000). Fertilized ovules may stop swelling rapidly in tomatoes due to high temperatures (Kinet and Peet, 1997). Heat stress also decreased ovule number and viability of tomato plants (Din et al., 2015). Similarly, a reduction of seed set under heat stress was associated with a decreased amount of carbohydrates and growth regulators in plant sink tissues in tomato (Kinet and Peet, 1997).
3.5 Callose deposition as a biomarker in response to abiotic stress in female reproductive organs
Callose is a polysaccharide β-1,3-glucan synthesized by a family of callose synthases and deposited in specialized cell walls or cell wall-associated structures such as cell plates, outer walls of pollen grains, pollen tubes, and plasmodesmata in a wide variety of higher plants (Nishikawa et al., 2005). Plant cell walls contain only small amounts of callose, ranging from 0.2%–0.5% in Arabidopsis and food crops to about 5% in energy crops under normal growth conditions (Wang et al., 2022). However, heavy callose deposition in cell walls may occur due to abiotic stress, wounding, and pathogen infection (Chen and Kim, 2009; Luna et al., 2011). Callose in the cell wall, plasmodesmata, and sieve pores contribute to the internal stabilities of cells during water stress and heat (Wang et al., 2022). In angiosperms with monosporic or bisporic embryo sac development, callose is formed in the megaspore mother cell walls (Rodkiewicz, 1970). Callose also accumulates in the walls of megaspore tetrads, the four haploid cells produced by the meiotic division of megaspore mother cells. Under optimal growth conditions, the callose begins to degrade when the functional megaspore undergoes its first mitosis and throughout the process of embryo sac development (Shi et al., 2016; Zhou et al., 2016; Brzezicka and Kozieradzka-Kiszkurno, 2024). However, callose accumulation can be extended around the synergid and at the micropylar in response to stress which can lead to ovule abortion and embryo senescence likely by hindering signals required for the pollen tube to enter the embryo sac and reducing sugar transport into ovules (Rodrigo and Herrero, 1998; Sun et al., 2004). A higher amount of callose deposition were observed in ovules of field pea under heat stress (Jiang et al., 2019b) and in sweet cherry (Prunus avium) (Zhang et al., 2018). A similar observation was reported in salt-stressed Arabidopsis plants (Sun et al., 2004). Callose has been used as a biomarker for embryo senescence such as in Arabidopsis (Sun et al., 2004) and field pea (Jiang et al., 2019b).
3.6 Effect of heat stress on reactive oxygen species (ROS) in female flower organs
Plants convert 1%–2% of consumed oxygen into reactive ROS, such as hydrogen peroxide (H2O2), singlet oxygen (1O2), superoxide radicals (O2•-), and hydroxyl radicals (•OH) as a byproduct of aerobic metabolism in several cellular compartments such as the chloroplast, mitochondria, and peroxisomes (Sachdev et al., 2021). ROS are important signaling molecules that are involved in reproductive processes such as megagametogenesis, pollen-pistil interaction, and degeneration of synergids (Zinta et al., 2016). ROS levels surge beyond physiological thresholds in response to environmental stressors which can cause irreversible damage to DNA, lipids, and proteins, leading to cell death (Dat et al., 2000; Zafar et al., 2022; Hong et al., 2024). To counteract these deleterious effects, plants have evolved a regulatory network comprising enzymatic [e.g., superoxide dismutase (SOD), peroxidase (POD), and catalase (CAT)] and non-enzymatic (e.g., ascorbic acid, glutathione, flavonoids, carotenoids, proline, and ubiquinone) antioxidant systems tends to keep the magnitude of ROS within plant cells to a non-damaging level (Zafar et al., 2023). However, antioxidants may fail to scavenge excess ROS. For example, under high-temperature stress, prolonged accumulation of ROS and low antioxidant activity in the pistils was suggested to be responsible for low fertilization rate and/or high rate of early embryo abortion in heat-sensitive genotypes of sorghum (Djanaguiraman et al., 2018b) and pearl millet (Djanaguiraman et al., 2018a). In contrast, the heat-tolerant rice genotype N22 maintained ROS homeostasis and antioxidant enzyme activities in its female gametophytes better than IR64, especially during post-stress recovery (Shi et al., 2022).
3.7 Effect of heat stress on phytohormones in female reproductive organs
Plant phytohormones such as abscisic acid (ABA), auxins, cytokinins, and ethylene play important roles in pollen-pistil interactions, pollen tube growth, and ovary development (Wu et al., 2008; Deb et al., 2018) and are altered to regulate the physiological and biochemical processes in response to environmental stresses (Zhang et al., 2023). Under high-temperature (34/18°C) conditions, pistils in a heat-sensitive rapeseed cultivar., ‘Westar’, had significantly lower levels of ABA than those developed under optimal (21/14°) conditions, suggesting that they are less protected against temperature stress (Mácová et al., 2022). Auxin plays an essential role in embryonic patterning at the early stage of seed development (Verma et al., 2021). Auxin levels were significantly decreased in heat-susceptible rice pistils due to high-temperature treatment (40°C for 2 h), and exogenous application of NAA (1-naphthaleneacetic acid) alleviated the restriction on pollen tube growth (Zhang C. et al., 2018). Ethylene may play a role in reproductive processes and seed development. It may also promote, inhibit or induce senescence depending upon the optimal or sub-optimal ethylene levels (Iqbal et al., 2017). The ethylene biosynthesis gene, 1-aminocyclopropane-1-carboxylic acid (ACC) oxidase (ACO) was expressed in early developing stigma, style, and ovary in tobacco (Nicotiana tabacum) (De Martinis and Mariani, 1999). In tomato, LeACO1,2,3, and 4 and LeACS1A transcripts were detected in pistils (Llop-Tous et al., 2000). In China rose (Hibiscus rosa-sinensis), ACC synthase (ACS) and ACO were found to be specifically expressed in developing style-stigma and ovary tissues (Trivellini et al., 2011). Ethylene seems to be involved in the senescence of unpollinated pistils in peas (Savada et al., 2017), tomato (Shinozaki et al., 2018), and Arabidopsis (Carbonell-Bejerano et al., 2011). In pea, PsACS and PsACO were differentially expressed in pre-pollinated ovaries under heat stress (33°C–35°C 6 h/day for 4 days) that promoted ovary senescence (Savada et al., 2017). The authors also suggested that the ethylene-dependent pollen germination and pollen tube growth on stigma and style were inhibited under heat (Savada et al., 2017).
4 Stress adaptation mechanisms during sexual reproduction
Plants can escape from environmental stress or resist it by avoidance or tolerance (Bueckert and Clarke, 2013). Management and breeding approaches, such as shifting planting date and genetic manipulation of crop phenology, have been used to facilitate plants to escape from heat stress (Sadras et al., 2015). Stress avoidance is the ability of plants to avoid stress conditions through some physiological mechanisms (Bueckert and Clarke, 2013). Stress tolerance is the ability of plants to survive under stress conditions and usually produce lower economic yield compared to optimal conditions (Hasanuzzaman et al., 2013). The terms, stress tolerance and stress resistance, are also used in the literature interchangeably to mean a trait with stress robustness compared to heat susceptibility. For example, dryland cereals (e.g., wheat, sorghum, and pearl millet) usually flower early morning or late evening during the cool hours of the day to avoid higher temperatures (Jagadish, 2020). In sorghum, 100% flowering was completed within 1 h before dawn, which helped sorghum plants escape dryer and hotter conditions during the day (Chiluwal et al., 2020).
Under stress conditions, several mechanisms are employed to maintain homeostasis and normal cellular functions. The strategy termed “differential transpiration” was recently reported by Sinha et al. (2023) – under combined heat and drought conditions, soybean plants prioritized their transpiration in flowers by opening flower stomata on sepals to have a cooling effect and kept their leaf stomata closed. The authors proposed that manipulating flower stomatal parameters (such as size and density) could be used as a strategy to enhance crop resilience to climate change (Sinha et al., 2023).
Heat shock proteins (HSPs) are a unique family of proteins produced in plant cells in response to environmental stresses (Singh et al., 2024). HSPs play a crucial role in conferring plant tolerance to heat and drought stress by maintaining the function of proteins and integrity of various biomembranes (Zhang et al., 2015; Liu et al., 2019). The application of genetic manipulation approach to enhance heat and drought tolerance in crops species has mainly focused on HSP encoding genes. For example, constitutive expression of Arabidopsis AtHsp101 (Katiyar-Agarwal et al., 2003) and OsHSP18.6 (Wang et al., 2015) increased thermotolerance in transgenic rice plants. Overexpression of small HSP17.7 enhanced rice tolerance to heat and UV-B exposure (Murakami et al., 2004). Pre-foliage exogenous application of ABA before high-temperature treatment of rice genotypes resulted in enhanced expression levels of HSP genes, increased levels of starch and soluble sugars, and proteins involved in sugar transport and conversion, and improved activity of antioxidant enzymes and ATP content in spikelets (Rezaul et al., 2019). Similarly, an auxin application of 1 μM resulted in a greater grain number and grain yield in wheat when plants were exposed to heat stress (34°C–35°C for 6 h for 6 days) (Abeysingha et al., 2021).
Cell wall invertases are involved in carbohydrate partitioning, hormone signaling, and stress responses and play fundamental roles in plant reproductive developments, including ovule development and seed/fruit set (Roitsch and González, 2004; Liu et al., 2022). In Arabidopsis, cell wall invertase functions as a positive regulator of ovule initiation through sugar signaling (Liao et al., 2020). In tomato crop, cell wall invertase promotes fruit set under heat stress by suppressing ROS-independent cell death (Liu et al., 2016). According to Li et al. (2012), tomato cultivars with elevated cell wall invertase activity in reproductive organs were found to be more tolerant to heat stress than the cultivars with lower cell wall invertase activity. Similarly, the improved heat tolerance was observed in genetically manipulated transgenic tomato in which the cell wall invertase activity was elevated both in ovaries and fruits by silencing the cell wall invertase inhibitor (Liu et al., 2016). In addition, the cell wall invertases-elevated transgenic tomato plants exhibited higher transcript levels of HSP90 and HSP100 in ovaries HspII17.6 in fruits and suppressed programmed cell death in fruits and alleviated fruit-set failure by promoting higher transcript levels of auxin biosynthesis gene ToFZY6 (Liu et al., 2016).
Recent studies have identified peptide/receptor signaling pathways that contribute to stress adaptation in female organs during sexual reproduction. In Arabidopsis, CLE45 (CLAVATA3/EMBRYO SURROUNDING REGION-RELATED45), a post-translationally modified peptide, was preferentially expressed in the stigma at normal temperature. Upon heat stress, the expression of CLE45 was expanded to the transmitting tract cells where it paired with RLK receptors SKM1 (STERILITY-REGULATING KINASE MEMBER1) and SKM2 of the male gametophyte to sustain pollen tube growth, leading to adequate fertilization and seed production (Endo et al., 2013). Endoplasmic reticulum-localized DnaJ (ERECTA) family receptor kinases play an important role in heat tolerance; when they are absent, high temperatures aggravate the negative effects on ovule development in Arabidopsis (Leng et al., 2022). A recent study showed that Class II TEOSINTE BRANCHED 1/CYCLOIDEA/ PCF (TCP) transcription factors protect ovule identity in Arabidopsis under high-temperature stress (Lan et al., 2023). Small RNAs play significant roles in ovule development (Petrella et al., 2021). Overexpression of OsRab7 gene increased the number of filled grains per hill by 35%, the seed filling rate by 21%, and the total grain weight by 27% in rice (El-Esawi and Alayafi, 2019).
5 Discussion
Ovule development is a critical step in plant reproduction, and maintaining optimal conditions during this process is essential for successful seed formation and overall plant productivity. Although a lot of previous work focused on the impact of abiotic stressors on the male gametophyte, several studies from recent years delved into the impact of these stress factors on the development of the female gametophyte (Djanaguiraman et al., 2018a; Jiang et al., 2019b; Wang et al., 2021a). Understanding how ovule and seed development respond to environmental stress is essential for developing effective strategies to enhance crop resilience to climate change. Further research in this field is not only crucial for advancing our knowledge but also for translating this understanding into practical solutions, such as abiotic stress resistance breeding and developing beneficial agronomy practices to mitigate the risk of climate change on crop production. In recent years, several research groups contributed to abiotic stress-resistant breeding (Rao et al., 2016; Gilliham et al., 2017; Derbyshire et al., 2022), such as the efforts in breeding for stress-resilient cultivars of pea (Jiang et al., 2017; Huang et al., 2023), wheat (Mondal et al., 2021; Tanin et al., 2022), and maize (Farooqi et al., 2022). Diversified cropping systems showed their effectiveness in improving crop resilience to climate change (Li et al., 2019). The concept of “green strategies” was proposed by González Guzmán et al. (2022), and they refer to environmentally friendly practices such as the use of beneficial soil microorganisms, chemical priming, and improved water management that aim to enhance plant tolerance to stress factors.
Soil microbes such as rhizobia, arbuscular mycorrhizal fungi, and plant growth-promoting rhizobacteria have shown the ability to improve plant growth and optimize reproductive development and seed set under adverse environmental conditions by employing several mechanisms, such as enhancing water use efficiency and nutrient acquisition through the production of phytohormones hormones, improving photosynthetic rate, balancing ionic equilibrium, producing antioxidants, and improving soil health and fertility (Sagar et al., 2021; Fahde et al., 2023). Salicylic acid is a phytohormone that is involved in plant flowering, pollen germination, pollen tube elongation, and post-fertilization ovary development (Luo et al., 2022). It is evidenced that foliar sprays or soil applications of priming agents like salicylic acid can alleviate yield losses due to heat stress (Zhang et al., 2017; Berková et al., 2023). Mulching is another green strategy that can contribute to the stability of reproductive processes during periods of low rainfall by reducing soil evaporation, conserving moisture, controlling soil temperature, reducing weed growth, and improving microbial activities (Iqbal et al., 2020).
Overall, these strategies optimize nutrient availability, reduce stress, and promote overall plant health, ensuring that ovule development in flowering plants is sustained and successful under changing environmental conditions, leading to improved plant development and reproduction.
Author contributions
ME: Writing – original draft, Writing – review & editing. DM: Writing – review & editing. RL: Writing – review & editing. YJ: Writing – review & editing, Conceptualization, Project administration, Writing – original draft.
Funding
The author(s) declare that no financial support was received for the research, authorship, and/or publication of this article.
Conflict of interest
The authors declare that the research was conducted in the absence of any commercial or financial relationships that could be construed as a potential conflict of interest.
The author(s) declared that they were an editorial board member of Frontiers, at the time of submission. This had no impact on the peer review process and the final decision.
Publisher’s note
All claims expressed in this article are solely those of the authors and do not necessarily represent those of their affiliated organizations, or those of the publisher, the editors and the reviewers. Any product that may be evaluated in this article, or claim that may be made by its manufacturer, is not guaranteed or endorsed by the publisher.
References
Abbas, G., Saqib, M., Rafique, Q., Rahman, A. U., Akhtar, J., Haq, M. A. U., et al. (2013). Effect of salinity on grain yield and grain quality of wheat (Triticum aestivum L.). Pak. J. Bot. 50, 185–189.
Abeysingha, D. N., Ozga, J. A., Strydhorst, S., Doyle, P., Iqbal, M., Yang, R., et al. (2021). The effect of auxins on amelioration of heat stress-induced wheat (Triticum aestivum L.) grain loss. J. Agron. Crop Sci. 207, 970–983. doi: 10.1111/jac.12555
Alzate-Marin, A. L., Rivas, P. M. S., Galaschi-Teixeira, J. S., Bonifácio-Anacleto, F., Silva, C. C., Schuster, I., et al. (2021). Warming and elevated CO2 induces changes in the reproductive dynamics of a tropical plant species. Sci. Total Environ. 768. doi: 10.1016/j.scitotenv.2020.144899
Andersen, M., Asch, F., Wu, Y., Jensen, C., Næsted, H., Mogensen, V., et al. (2002). Soluble invertase expression is an early target of drought stress during the critical, abortion-sensitive phase of young ovary development in maize. Plant Physiol. 130, 591–604. doi: 10.1104/pp.005637
Atta, K., Mondal, S., Gorai, S., Singh, A. P., Kumari, A., Ghosh, T., et al. (2023). Impacts of salinity stress on crop plants: improving salt tolerance through genetic and molecular dissection. Front. Plant Sci. 14:1736. doi: 10.3389/fpls.2023.1241736
Begcy, K., Sandhu, J., and Walia, H. (2018). Transient heat stress during early seed development primes germination and seedling establishment in rice. Front. Plant Sci. 871:1768. doi: 10.3389/fpls.2018.01768
Berger, F., and Twell, D. (2011). Germline specification and function in plants. Annu. Rev. Plant Biol. 62, 461–484. doi: 10.1146/annurev-arplant-042110-103824
Berková, V., Berka, M., Kameniarová, M., Kopecká, R., Kuzmenko, M., Shejbalová, Š., et al. (2023). Salicylic acid treatment and its effect on seed yield and seed molecular composition of Pisum sativum under abiotic stress. Int. J. Mol. Sci. 24:5454. doi: 10.3390/ijms24065454
Bhandari, K., Siddique, K. H. M., Turner, N. C., Kaur, J., Singh, S., Agrawal, S. K., et al. (2016). Heat stress at reproductive stage disrupts leaf carbohydrate metabolism, impairs reproductive function, and severely reduces seed yield in lentil. J. Crop Improv. 30, 118–151. doi: 10.1080/15427528.2015.1134744
Bleckmann, A., Alter, S., and Dresselhaus, T. (2014). The beginning of a seed: regulatory mechanisms of double fertilization. Front. Plant Sci. 5:452. doi: 10.3389/fpls.2014.00452
Boretti, A., and Florentine, S. (2019). Atmospheric CO2 concentration and other limiting factors in the growth of C3 and C4 plants. Plan. Theory 8:92. doi: 10.3390/plants8040092
Boyer, J. S., and McLaughlin, J. E. (2007). Functional reversion to identify controlling genes in multigenic responses: analysis of floral abortion. J. Exp. Bot., 267–277. doi: 10.1093/jxb/erl177
Brunel-Muguet, S., d’Hooghe, P., Bataillé, M.-P., Larré, C., Kim, T.-H., Trouverie, J., et al. (2015). Heat stress during seed filling interferes with sulfur restriction on grain composition and seed germination in oilseed rape (Brassica napus L.). Front. Plant Sci. 6:213. doi: 10.3389/fpls.2015.00213
Brzezicka, E., and Kozieradzka-Kiszkurno, M. (2024). Callose deposition analysis with special emphasis on plasmodesmata ultrastructure during megasporogenesis in sedum (Crassulaceae). Protoplasma 261, 31–41. doi: 10.1007/s00709-023-01879-x
Bueckert, R. A., and Clarke, J. M. (2013). Review: annual crop adaptation to abiotic stress on the Canadian prairies: six case studies. Can. J. Plant Sci. 93, 375–385. doi: 10.4141/cjps2012-184
Buitink, J., Leprince, O., Hemminga, M. A., and Hoekstra, F. A. (2000). The effects of moisture and temperature on the ageing kinetics of pollen: interpretation based on cytoplasmic mobility. Plant Cell Environ. 23, 967–974. doi: 10.1046/j.1365-3040.2000.00601.x
Callens, C., Fernandez-Goméz, J., Tucker, M. R., Zhang, D., and Wilson, Z. A. (2023). Heat stress responses vary during floret development in European spring barley cultivars. Front. Plant Sci. 13:18730. doi: 10.3389/fpls.2022.918730
Carbonell-Bejerano, P., Urbez, C., Granell, A., Carbonell, J., and Perez-Amador, M. A. (2011). Ethylene is involved in pistil fate by modulating the onset of ovule senescence and the GA-mediated fruit set in Arabidopsis. BMC Plant Biol. 11:84. doi: 10.1186/1471-2229-11-84
Cerovic, R., Ruzic, D., and Micic, N. (2000). Viability of plum ovules at different temperatures. Physiology, 53–58. doi: 10.1111/J.1744-7348.2000.TB00056.X
Chen, X.-Y., and Kim, J.-Y. (2009). Callose synthesis in higher plants. Plant Signal. Behav. 4, 489–492. doi: 10.4161/psb.4.6.8359
Chen, C., Riley, W. J., Prentice, I. C., and Keenan, T. F. (2022). CO2 fertilization of terrestrial photosynthesis inferred from site to global scales.
Chiluwal, A., Bheemanahalli, R., Kanaganahalli, V., Boyle, D., Perumal, R., Pokharel, M., et al. (2020). Deterioration of ovary plays a key role in heat stress-induced spikelet sterility in sorghum. Plant Cell Environ. 43, 448–462. doi: 10.1111/pce.13673
Cross, R. H., Mckay, S. A. B., Mhughen, A. G., and Bonham-Smith, P. C. (2003). Heat-stress effects on reproduction and seed set in Linum usitatissimum L. (flax). Plant Cell Environ. 26, 1013–1020. doi: 10.1046/j.1365-3040.2003.01006.x
Cucinotta, M., Di Marzo, M., Guazzotti, A., de Folter, S., Kater, M. M., and Colombo, L. (2020). Gynoecium size and ovule number are interconnected traits that impact seed yield. J. Exp. Bot. 71, 2479–2489. doi: 10.1093/jxb/eraa050
Danilevskaya, O. N., Yu, G. X., Meng, X., Xu, J., Stephenson, E., Estrada, S., et al. (2019). Developmental and transcriptional responses of maize to drought stress under field conditions. Plant Direct 3:129. doi: 10.1002/pld3.129
Dat, J., Vandenabeele, S., Vranova, E., Van Montagu, M., Inzé, D., and Van Breusegem, F. (2000). Dual action of the active oxygen species during plant stress responses. Cell. Mol. Life Sci. 57, 779–795. doi: 10.1007/s000180050041
Davies, F., Geneve, R., and Wilson, S. (2018). “Seed development” in Plant propagation principles and practices. ed. F. Davies (London, UK: Pearson), 120–159.
De Martinis, D., and Mariani, C. (1999). Silencing gene expression of the ethylene-forming enzyme results in a reversible inhibition of ovule development in transgenic tobacco plants. Plant Cell 11, 1061–1071. doi: 10.1105/tpc.11.6.1061
De Storme, N., and Geelen, D. (2014). The impact of environmental stress on male reproductive development in plants: biological processes and molecular mechanisms. Plant Cell Environ. 37, 1–18. doi: 10.1111/pce.12142
Deb, J., Bland, H. M., and Østergaard, L. (2018). Developmental cartography: coordination via hormonal and genetic interactions during gynoecium formation. Curr. Opin. Plant Biol. 41, 54–60. doi: 10.1016/j.pbi.2017.09.004
Derbyshire, M. C., Batley, J., and Edwards, D. (2022). Use of multiple ‘omics techniques to accelerate the breeding of abiotic stress tolerant crops. Curr Plant Biol 32:100262. doi: 10.1016/j.cpb.2022.100262
Devasirvatham, V., Gaur, P. M., Mallikarjuna, N., Raju, T. N., Trethowan, R. M., and Tan, D. K. Y. (2013). Reproductive biology of chickpea response to heat stress in the field is associated with the performance in controlled environments. Field Crop Res. 142, 9–19. doi: 10.1016/j.fcr.2012.11.011
Devasirvatham, V., Gaur, P. M., Raju, T. N., Trethowan, R. M., and Tan, D. K. Y. (2015). Field response of chickpea (Cicer arietinum L.) to high temperature. Field Crop Res. 172, 59–71. doi: 10.1016/j.fcr.2014.11.017
Diboll, A. G. (1968). Fine structural development of the megagametophyte of Zea mays following fertilization. Am. J. Bot. 55, 797–806. doi: 10.2307/2440968
Din, J. U., Khan, S. U., Khan, A., Qayyum, A., Abbasi, K. S., and Jenks, M. A. (2015). Evaluation of potential morpho-physiological and biochemical indicators in selecting heat-tolerant tomato (Solanum lycopersicum Mill.) genotypes. Hortic. Environ. Biotechnol. 56, 769–776. doi: 10.1007/s13580-015-0098-x
Djanaguiraman, M., Perumal, R., Ciampitti, I. A., Gupta, S. K., and Prasad, P. V. V. (2018a). Quantifying pearl millet response to high temperature stress: thresholds, sensitive stages, genetic variability and relative sensitivity of pollen and pistil. Plant Cell Environ. 41, 993–1007. doi: 10.1111/pce.12931
Djanaguiraman, M., Perumal, R., Jagadish, S. V. K., Ciampitti, I. A., Welti, R., and Prasad, P. V. V. (2018b). Sensitivity of sorghum pollen and pistil to high-temperature stress. Plant Cell Environ. 41, 1065–1082. doi: 10.1111/pce.13089
Dresselhaus, T., and Franklin-Tong, N. (2013). Male–female crosstalk during pollen germination, tube growth and guidance, and double fertilization. Mol. Plant 6, 1018–1036. doi: 10.1093/mp/sst061
Drews, G. N., and Koltunow, A. M. G. (2011). The female gametophyte. The Arabidopsis book/American Society of Plant Biologists, No. 9.
Duque, L., Poelman, E. H., and Steffan-Dewenter, I. (2021a). Effects of ozone stress on flowering phenology, plant-pollinator interactions and plant reproductive success. Environ. Pollut. 272:5953. doi: 10.1016/j.envpol.2020.115953
Duque, L., Poelman, E. H., and Steffan-Dewenter, I. (2021b). Plant age at the time of ozone exposure affects flowering patterns, biotic interactions and reproduction of wild mustard. Sci. Rep. 11:2878. doi: 10.1038/s41598-021-02878-9
Edlund, A. F., Swanson, R., and Preuss, D. (2004). Pollen and stigma structure and function: the role of diversity in pollination. Plant Cell 16, S84–S97. doi: 10.1105/tpc.015800
El Sabagh, A., Islam, M. S., Skalicky, M., Ali Raza, M., Singh, K., Anwar Hossain, M., et al. (2021). Salinity stress in wheat (Triticum aestivum L.) in the changing climate: adaptation and management strategies. Front. Agron. 3:1932. doi: 10.3389/fagro.2021.661932
El-Esawi, M. A., and Alayafi, A. A. (2019). Overexpression of rice Rab7 gene improves drought and heat tolerance and increases grain yield in rice (Oryza sativa L.). Genes (Basel) 10:10056. doi: 10.3390/genes10010056
Elferjani, R., and Soolanayakanahally, R. (2018). Canola responses to drought, heat, and combined stress: shared and specific effects on carbon assimilation, seed yield, and oil composition. Front. Plant Sci. 9:1224. doi: 10.3389/fpls.2018.01224
Elleman, C. J., Eranklin-Tong, V., and Dickinson, H. G. (1992). Pollination in species with dry stigmas: the nature of the early stigmatic response and the pathway taken by pollen tubes. New Phytol. 121, 413–424. doi: 10.1111/j.1469-8137.1992.tb02941.x
Endo, S., Shinohara, H., Matsubayashi, Y., and Fukuda, H. (2013). A novel pollen-pistil interaction conferring high-temperature tolerance during reproduction via CLE45 signaling. Curr. Biol. 23, 1670–1676. doi: 10.1016/j.cub.2013.06.060
Endo, M., Tsuchiya, T., Hamada, K., Kawamura, S., Yano, K., Ohshima, M., et al. (2009). High temperatures cause male sterility in rice plants with transcriptional alterations during pollen development. Plant Cell Physiol. 50, 1911–1922. doi: 10.1093/pcp/pcp135
Endress, P. K. (2011a). Angiosperm ovules: diversity, development, evolution. Ann. Bot. 107, 1465–1489. doi: 10.1093/aob/mcr120
Endress, P. K. (2011b). Evolutionary diversification of the flowers in angiosperms. Am. J. Bot. 98, 370–396. doi: 10.3732/ajb.1000299
Erickson, A. N., and Markhart, A. H. (2002). Flower developmental stage and organ sensitivity of bell pepper (Capsicum annuum L.) to elevated temperature. Plant Cell Environ. 25, 123–130. doi: 10.1046/j.0016-8025.2001.00807.x
Fahde, S., Boughribil, S., Sijilmassi, B., and Amri, A. (2023). Rhizobia: a promising source of plant growth-promoting molecules and their non-legume interactions: examining applications and mechanisms. Agriculture 13:1279. doi: 10.3390/agriculture13071279
Farooqi, M. Q. U., Nawaz, G., Wani, S. H., Choudhary, J. R., Rana, M., Sah, R. P., et al. (2022). Recent developments in multi-omics and breeding strategies for abiotic stress tolerance in maize (Zea mays L.). Front. Plant Sci. 13:965878. doi: 10.3389/fpls.2022.965878
Faure, J. E. (2001). Double fertilization in flowering plants: discovery, study methods and mechanisms. C. R. Acad. Sci. III 324, 551–558. doi: 10.1016/S0764-4469(01)01325-7
Fiscus, E. L., Booker, F. L., and Burkey, K. O. (2005). Crop responses to ozone: uptake, modes of action, carbon assimilation and partitioning. Plant Cell Environ. 28, 997–1011. doi: 10.1111/j.1365-3040.2005.01349.x
Flowers, T. J., Gaur, P. M., Gowda, C. L. L., Krishnamurthy, L., Samineni, S., Siddique, K. H. M., et al. (2010). Salt sensitivity in chickpea. Plant Cell Environ. 33, 490–509. doi: 10.1111/j.1365-3040.2009.02051.x
Frank, A. B., Bauer, A., and Black, A. L. (1987). Effects of air temperature and water stress on apex development in spring wheat. Crop Sci. 27, 113–116. doi: 10.2135/cropsci1987.0011183X002700010028x
Friedman, W. E. (2006). Embryological evidence for developmental lability during early angiosperm evolution. Nature 441, 337–340. doi: 10.1038/nature04690
Ghanbari, A. A., Mousavi, S. H., Gorji, A. M., and Rao, I. (2013a). Effect of water stress on leaves and seeds of bean (Phaseolus vulgaris L.). Field Crops Res. 20, 81–93. doi: 10.1016/0378-4290(89)90054-3
Ghanbari, A. A., Shakiba, M. R., Toorchi, M., and Choukan, R. (2013b). Nitrogen changes in the leaves and accumulation of some minerals in the seeds of red, white and Chitti beans (Phaseolus vulgaris) under water deficit conditions. Aust. J. Crop Sci. 7, 706–712.
Gilliham, M., Able, J. A., and Roy, S. J. (2017). Translating knowledge about abiotic stress tolerance to breeding programmes. Plant J. 90, 898–917. doi: 10.1111/tpj.13456
Giorno, F., Wolters-Arts, M., Mariani, C., and Rieu, I. (2013). Ensuring reproduction at high temperatures: the heat stress response during anther and pollen development. Plan. Theory 2, 489–506. doi: 10.3390/plants2030489
González Guzmán, M., Cellini, F., Fotopoulos, V., Balestrini, R., and Arbona, V. (2022). New approaches to improve crop tolerance to biotic and abiotic stresses. Physiol. Plant. 174:13547. doi: 10.1111/ppl.13547
Grieve, C. M., Francois, L. E., and Maas, E. V. (1994). Salinity affects the timing of phasic development in spring wheat. Crop Sci. 34, 1544–1549. doi: 10.2135/cropsci1994.0011183X003400060024x
Gross, Y., and Kigel, J. (1994). Differential sensitivity to high temperature of stages in the reproductive development of common bean (Phaseolus vulgaris L.). Field Crop Res. 36, 201–212. doi: 10.1016/0378-4290(94)90112-0
Grossniklaus, U., and Schneitz, K. (1998). “The molecular and genetic basis of ovule and megagametophyte development” in Seminars in cell and developmental biology. ed. U. Grossniklaus (Amsterdam, Netherlands: Elsevier), 227–238.
Gupta, S. K., Rai, K. N., Singh, P., Ameta, V. L., Gupta, S. K., Jayalekha, A. K., et al. (2015). Seed set variability under high temperatures during flowering period in pearl millet (Pennisetum glaucum L. (R.) Br.). Field Crop Res. 171, 41–53. doi: 10.1016/j.fcr.2014.11.005
Haig, D. (2020). Poles apart: monosporic, bisporic, and tetrasporic embryo sacs revisited. Front. Ecol. Evol. 8:516640. doi: 10.3389/fevo.2020.516640
Hasanuzzaman, M., Nahar, K., Alam, M. M., Roychowdhury, R., and Fujita, M. (2013). Physiological, biochemical, and molecular mechanisms of heat stress tolerance in plants. Int. J. Mol. Sci. 14, 9643–9684. doi: 10.3390/ijms14059643
Hayes, F., Williamson, J., and Mills, G. (2012). Ozone pollution affects flower numbers and timing in a simulated BAP priority calcareous grassland community. Environ. Pollut. 163, 40–47. doi: 10.1016/j.envpol.2011.12.032
Hedhly, A. (2011). Sensitivity of flowering plant gametophytes to temperature fluctuations. Environ. Exp. Bot. 74, 9–16. doi: 10.1016/j.envexpbot.2011.03.016
Hernández-Espinosa, N., Mondal, S., Autrique, E., Gonzalez-Santoyo, H., Crossa, J., Huerta-Espino, J., et al. (2018). Milling, processing and end-use quality traits of CIMMYT spring bread wheat germplasm under drought and heat stress. Field Crop Res. 215, 104–112. doi: 10.1016/j.fcr.2017.10.003
Herrero, M., and Hormaza, J. I. (1996). Pistil strategies controlling pollen tube growth. Sex. Plant Reprod. 9, 343–347. doi: 10.1007/BF02441953
Heslop-Harrison, J. (1979). An interpretation of the hydrodynamics of pollen. Am. J. Bot. 66, 737–743. doi: 10.1002/j.1537-2197.1979.tb06277.x
Heslop-Harrison, Y. (1981). Stigma characteristics and angiosperm taxonomy. Nord. J. Bot. 1, 401–420. doi: 10.1111/j.1756-1051.1981.tb00707.x
Heslop-Harrison, Y., and Shivanna, K. (1977). The receptive surface of the angiosperm stigma. Ann. Bot. 41, 1233–1258. doi: 10.1093/oxfordjournals.aob.a085414
Higashiyama, T. (2010). Peptide signaling in pollen–pistil interactions. Plant Cell Physiol. 51, 177–189. doi: 10.1093/pcp/pcq008
Hiyane, R., Hiyane, S., Tang, A. C., and Boyer, J. S. (2010). Sucrose feeding reverses shade-induced kernel losses in maize. Ann. Bot. 106, 395–403. doi: 10.1093/aob/mcq132
Hong, Y., Boiti, A., Vallone, D., and Foulkes, N. S. (2024). Reactive oxygen species signaling and oxidative stress: transcriptional regulation and evolution. Antioxidants 13:312. doi: 10.3390/antiox13030312
Hu, X., Chen, S., Siddique, K. H. M., and Cowling, W. A. (2024). Transient heat stress during gametophyte development in Brassica napus reduces subsequent floret fecundity. Plant Stress 13:522. doi: 10.1016/j.stress.2024.100522
Huang, J., Dong, J., and Qu, L.-J. (2021). From birth to function: male gametophyte development in flowering plants. Curr. Opin. Plant Biol. 63:102118. doi: 10.1016/j.pbi.2021.102118
Huang, S., Gali, K. K., Arganosa, G. C., Tar᾿an, B., Bueckert, R. A., and Warkentin, T. D. (2023). Breeding indicators for high-yielding field pea under normal and heat stress environments. Can. J. Plant Sci. 103, 259–269. doi: 10.1139/cjps-2022-0158
Huang, R., Liu, Z., Xing, M., Yang, Y., Wu, X., Liu, H., et al. (2019). Heat stress suppresses Brassica napus seed oil accumulation by inhibition of photosynthesis and BnWRI1 pathway. Plant Cell Physiol. 60, 1457–1470. doi: 10.1093/pcp/pcz052
Huang, B.-Q., Ye, X.-L., Yeung, E. C., and Zee, S. Y. (1998). Embryology of Cymbidium sinense: the microtubule organization of early embryos. Ann. Bot. 81, 741–750. doi: 10.1006/anbo.1998.0628
Iqbal, N., Khan, N. A., Ferrante, A., Trivellini, A., Francini, A., and Khan, M. I. R. (2017). Ethylene role in plant growth, development and senescence: interaction with other phytohormones. Front. Plant Sci. 8:475. doi: 10.3389/fpls.2017.00475
Iqbal, R., Raza, M. A. S., Valipour, M., Saleem, M. F., Zaheer, M. S., Ahmad, S., et al. (2020). Potential agricultural and environmental benefits of mulches—a review. Bull. Natl. Res. Cent. 44, 1–16. doi: 10.1186/s42269-020-00290-3
Ishimaru, T., Sasaki, K., Lumanglas, P. D., Leo, U., Cabral, C., Ye, C., et al. (2022). Effect of drought stress on flowering characteristics in rice (Oryza sativa L.): A study using genotypes contrasting in drought tolerance and flower opening time. Plant Prod. Sci. 25, 359–370. doi: 10.1080/1343943X.2022.2085589
Jablonski, L. M., Wang, X., and Curtis, P. S. (2002). Plant reproduction under elevated CO2 conditions: A meta-analysis of reports on 79 crop and wild species. New Phytol. 156, 9–26. doi: 10.1046/j.1469-8137.2002.00494.x
Jagadish, S. V. K. (2020). Heat stress during flowering in cereals – effects and adaptation strategies. New Phytol. 226, 1567–1572. doi: 10.1111/nph.16429
Jain, M., Prasad, P. V. V., Boote, K. J., Hartwell, A. L., and Chourey, P. S. (2007). Effects of season-long high temperature growth conditions on sugar-to-starch metabolism in developing microspores of grain sorghum (Sorghum bicolor L. Moench). Planta 227, 67–79. doi: 10.1007/s00425-007-0595-y
Jiang, Y., Davis, A. R., Vujanovic, V., and Bueckert, R. A. (2019a). Reproductive development response to high daytime temperature in field pea. J. Agron. Crop Sci. 205:12328. doi: 10.1111/jac.12328
Jiang, Y., Diapari, M., Bueckert, R. A., Taran, B., and Warkentin, T. D. (2017). Population structure and association mapping of traits related to reproductive development in field pea. Euphytica 213:2006. doi: 10.1007/s10681-017-2006-1
Jiang, Y., Lahlali, R., Karunakaran, C., Kumar, S., Davis, A. R., and Bueckert, R. A. (2015). Seed set, pollen morphology and pollen surface composition response to heat stress in field pea. Plant Cell Environ. 38, 2387–2397. doi: 10.1111/pce.12589
Jiang, Y., Lahlali, R., Karunakaran, C., Warkentin, T. D., Davis, A. R., and Bueckert, R. A. (2019b). Pollen, ovules, and pollination in pea: success, failure, and resilience in heat. Plant Cell Environ. 42:13427. doi: 10.1111/pce.13427
Jiang, Y., Lindsay, D. L., Davis, A. R., Wang, Z., MacLean, D. E., Warkentin, T. D., et al. (2020). Impact of heat stress on pod-based yield components in field pea (Pisum sativum L.). J. Agron. Crop Sci. 206:12365. doi: 10.1111/jac.12365
Jiang, N., Yu, P., Fu, W., Li, G., Feng, B., Chen, T., et al. (2020). “Acid invertase confers heat tolerance in rice plants by maintaining energy homoeostasis of spikelets” in Plant cell and environment. ed. N. Jiang (Hoboken, NJ: Blackwell Publishing Ltd), 1273–1287.
Johnson, M. A., and Preuss, D. (2002). Plotting a course: multiple signals guide pollen tubes to their targets. Dev. Cell 2, 273–281. doi: 10.1016/S1534-5807(02)00130-2
Katano, K., and Suzuki, N. (2021). What are the key mechanisms that alter the morphology of stigmatic papillae in Arabidopsis thaliana? Plant Signal. Behav. 16:1980999. doi: 10.1080/15592324.2021.1980999
Katiyar-Agarwal, S., Agarwal, M., and Grover, A. (2003). Heat-tolerant basmati rice engineered by over-expression of hsp101. Plant Mol. Biol. 51, 677–686. doi: 10.1023/A:1022561926676
Kaushal, N., Bhandari, K., Siddique, K. H. M., Nayyar, H., and Tejada Moral, M. (2016). Food crops face rising temperatures: an overview of responses, adaptive mechanisms, and approaches to improve heat tolerance. Cogent. Food Agric. 2:1134380. doi: 10.1080/23311932.2015.1134380
Khan, H. A., Siddique, K. H. M., and Colmer, T. D. (2017). Vegetative and reproductive growth of salt-stressed chickpea are carbon-limited: sucrose infusion at the reproductive stage improves salt tolerance. J. Exp. Bot. 68, 2001–2011. doi: 10.1093/jxb/erw177
Khan, I., Wu, J., and Sajjad, M. (2022). Pollen viability-based heat susceptibility index (HSIpv): A useful selection criterion for heat-tolerant genotypes in wheat. Front. Plant Sci. 13:569. doi: 10.3389/fpls.2022.1064569
Khataar, M., Mohhamadi, M. H., and Shabani, F. (2018). Soil salinity and matric potential interaction on water use, water use efficiency and yield response factor of bean and wheat. Sci. Rep. 8:20968. doi: 10.1038/s41598-018-20968-z
Kinet, J. M., and Peet, M. M. (1997). “Tomato” in The physiology of vegetable crops. ed. H. C. Wien (Wallingford, UK: Centre for Agricultural and Bioscience International), 207–258.
Kumar, J., Gupta, S., Gupta, P., Dubey, S., Tomar, R. S. S., and Kumar, S. (2016). Breeding strategies to improve lentil for diverse agro-ecological environments. Indian J. Genet. Plant Breed. 76, 530–549. doi: 10.5958/0975-6906.2016.00071.7
Kumar, S., Thakur, P., Kaushal, N., Malik, J. A., Gaur, P., and Nayyar, H. (2013). Effect of varying high temperatures during reproductive growth on reproductive function, oxidative stress and seed yield in chickpea genotypes differing in heat sensitivity. Arch. Agron. Soil Sci. 59, 823–843. doi: 10.1080/03650340.2012.683424
Kumari, V. V., Banerjee, P., Verma, V. C., Sukumaran, S., Chandran, M. A. S., Gopinath, K. A., et al. (2022). Plant nutrition: an effective way to alleviate abiotic stress in agricultural crops. Int. J. Mol. Sci. 23:8519. doi: 10.3390/ijms23158519
Lahlali, R., Jiang, Y., Kumar, S., Karunakaran, C., Liu, X., Borondics, F., et al. (2014). ATR-FTIR spectroscopy reveals involvement of lipids and proteins of intact pea pollen grains to heat stress tolerance. Front. Plant Sci. 5:747. doi: 10.3389/fpls.2014.00747
Lan, J., Wang, N., Wang, Y., Jiang, Y., Yu, H., Cao, X., et al. (2023). Arabidopsis TCP4 transcription factor inhibits high temperature-induced homeotic conversion of ovules. Nat. Commun. 14:416. doi: 10.1038/s41467-023-41416-1
Le Quéré, C., Raupach, M. R., Canadell, J. G., Marland, G., Bopp, L., Ciais, P., et al. (2009). Trends in the sources and sinks of carbon dioxide. Nat. Geosci. 2, 831–836. doi: 10.1038/ngeo689
Leisner, C. P., and Ainsworth, E. A. (2012). Quantifying the effects of ozone on plant reproductive growth and development. Glob. Chang. Biol. 18, 606–616. doi: 10.1111/j.1365-2486.2011.02535.x
Leng, Y. J., Yao, Y. S., Yang, K. Z., Wu, P. X., Xia, Y. X., Zuo, C. R., et al. (2022). Arabidopsis ERdj3B coordinates with ERECTA-family receptor kinases to regulate ovule development and the heat stress response. Plant Cell 34, 3665–3684. doi: 10.1093/plcell/koac226
Lesk, C., Rowhani, P., and Ramankutty, N. (2016). Influence of extreme weather disasters on global crop production. Nature 529, 84–87. doi: 10.1038/nature16467
Li, J., Huang, L., Zhang, J., Coulter, J. A., Li, L., and Gan, Y. (2019). Diversifying crop rotation improves system robustness. Agron. Sustain. Dev. 39:584. doi: 10.1007/s13593-019-0584-0
Li, X., Lawas, L. M. F., Malo, R., Glaubitz, U., Erban, A., Mauleon, R., et al. (2015). Metabolic and transcriptomic signatures of rice floral organs reveal sugar starvation as a factor in reproductive failure under heat and drought stress. Plant Cell Environ. 38, 2171–2192. doi: 10.1111/pce.12545
Li, Z., Palmer, W. M., Martin, A. P., Wang, R., Rainsford, F., Jin, Y., et al. (2012). High invertase activity in tomato reproductive organs correlates with enhanced sucrose import into, and heat tolerance of, young fruit. J. Exp. Bot. 63, 1155–1166. doi: 10.1093/jxb/err329
Liao, S., Wang, L., Li, J., and Ruan, Y.-L. (2020). Cell wall invertase is essential for ovule development through sugar signaling rather than provision of carbon nutrients. Plant Physiol. 183, 1126–1144. doi: 10.1104/pp.20.00400
Liu, F., Andersen, M. N., and Jensen, C. R. (2003). Loss of pod set caused by drought stress is associated with water status and ABA content of reproductive structures in soybean. Funct. Plant Biol. 30, 271–280. doi: 10.1071/FP02185
Liu, C., Hu, S., Liu, S., Shi, W., Xie, D., Chen, Q., et al. (2022). Functional characterization of a cell wall invertase inhibitor StInvInh1 revealed its involvement in potato microtuber size in vitro. Front. Plant Sci. 13:1015815. doi: 10.3389/fpls.2022.1015815
Liu, F., Jensen, C. R., and Andersen, M. N. (2004). Pod set related to photosynthetic rate and endogenous ABA in soybeans subjected to different water regimes and exogenous ABA and BA at early reproductive stages. Ann. Bot. 94, 405–411. doi: 10.1093/aob/mch157
Liu, Y., Li, J., Zhu, Y., Jones, A., Rose, R. J., and Song, Y. (2019). Heat stress in legume seed setting: effects, causes, and future prospects. Front. Plant Sci. 10:938. doi: 10.3389/fpls.2019.00938
Liu, J., Lim, S. L., Zhong, J. Y., and Lim, B. L. (2022). Bioenergetics of pollen tube growth in Arabidopsis thaliana revealed by ratiometric genetically encoded biosensors. Nat. Commun. :13. doi: 10.1038/s41467-022-35486-w
Liu, Y. H., Offler, C. E., and Ruan, Y. L. (2016). Cell wall invertase promotes fruit set under heat stress by suppressing ROS-independent cell death. Plant Physiol. 172, 163–180. doi: 10.1104/pp.16.00959
Llop-Tous, I., Barry, C. S., and Grierson, D. (2000). Regulation of ethylene biosynthesis in response to pollination in tomato flowers. Plant Physiol. 123, 971–978. doi: 10.1104/pp.123.3.971
Lohaus, G., Hussmann, M., Pennewiss, K., Schneider, H., Zhu, J.-J., and Sattelmacher, B. (2000). Solute balance of a maize (Zea mays L.) source leaf as affected by salt treatment with special emphasis on phloem retranslocation and ion leaching 51, 1721–1732. doi: 10.1093/jexbot/51.351.1721
Loka, D. A., and Oosterhuis, D. M. (2016). Effect of high night temperatures during anthesis on cotton (Gossypium hirsutum L.) pistil and leaf physiology and biochemistry. Aust. J. Crop. Sci. 10, 741–748. doi: 10.21475/ajcs.2016.10.05.p7498
Luna, E., Pastor, V., Robert, J., Flors, V., Mauch-Mani, B., and Ton, J. (2011). Callose deposition: a multifaceted plant defense response. Mol. Plant-Microbe Interact. 24, 183–193. doi: 10.1094/MPMI-07-10-0149
Luo, Y., Liu, M., Cao, J., Cao, F., and Zhang, L. (2022). The role of salicylic acid in plant flower development. Forestry Research 2:14. doi: 10.48130/FR-2022-0014
Mácová, K., Prabhullachandran, U., Štefková, M., Spyroglou, I., Pěnčík, A., Endlová, L., et al. (2022). Long-term high-temperature stress impacts on embryo and seed development in Brassica napus. Front. Plant Sci. 13:292. doi: 10.3389/fpls.2022.844292
Mahdavi, S., Arzani, A., Maibody, S. A. M. M., and Kadivar, M. (2022). Grain and flour quality of wheat genotypes grown under heat stress. Saudi J. Biol. Sci. 29:103417. doi: 10.1016/j.sjbs.2022.103417
McLaughlin, J. E., and Boyer, J. S. (2004). Sugar-responsive gene expression, invertase activity, and senescence in aborting maize ovaries at low water potentials. Ann. Bot. 94, 675–689. doi: 10.1093/aob/mch193
Mondal, S., Sallam, A., Sehgal, D., Sukumaran, S., Farhad, M., Navaneetha Krishnan, J., et al. (2021). “Advances in breeding for abiotic stress tolerance in wheat” in Genomic designing for abiotic stress resistant cereal crops. ed. S. Mondal (Berlin: Springer Nature), 71–103.
Monterroso, V. A., and Wien, H. C. (1990). Flower and pod abcission due to heat stress in beans. jashs 115, 631–634. doi: 10.21273/JASHS.115.4.631
Munns, R., James, R. A., and Läuchli, A. (2006). Approaches to increasing the salt tolerance of wheat and other cereals. J. Exp. Bot. 1, 1025–1043. doi: 10.1093/jxb/erj100
Murakami, T., Matsuba, S., Funatsuki, H., Kawaguchi, K., Saruyama, H., Tanida, M., et al. (2004). Over-expression of a small heat shock protein, sHSP17. 7, confers both heat tolerance and UV-B resistance to rice plants. Mol. Breed. 13, 165–175. doi: 10.1023/B:MOLB.0000018764.30795.c1
Nadakuduti, S. S., Laforest, L. C., Tachev, M., Decker, A. N., Egesa, A. O., Shirazi, A. S., et al. (2023). Heat stress during seed development leads to impaired physiological function and plasticity in seed oil accumulation in Camelina sativa. Front. Plant Sci. 14:1284573. doi: 10.3389/fpls.2023.1284573
Nakata, M., Fukamatsu, Y., Miyashita, T., Hakata, M., Kimura, R., Nakata, Y., et al. (2017). High temperature-induced expression of rice α-amylases in developing endosperm produces chalky grains. Front. Plant Sci. 8:2089. doi: 10.3389/fpls.2017.02089
National Aeronautics and Space Administration . (2023). NASA Clocks July 2023 as Hottest Month on Record Ever Since 1880. Available at: https://www.nasa.gov/news-release/nasa-clocks-july-2023-as-hottest-month-on-record-ever-since-1880/#:~:text=Overall%2C%20July%202023%20was%200.43,July%20between%201951%20and201980.
Nayyar, H., Kaur, S., Singh, S., and Upadhyaya, H. D. (2006). Differential sensitivity of Desi (small-seeded) and Kabuli (large-seeded) chickpea genotypes to water stress during seed filling: effects on accumulation of seed reserves and yield. J. Sci. Food Agric. 86, 2076–2082. doi: 10.1002/jsfa.2574
Nishikawa, S., Zinkl, G. M., Swanson, R. J., Maruyama, D., and Preuss, D. (2005). Callose (beta-1,3 glucan) is essential for Arabidopsis pollen wall patterning, but not tube growth. BMC Plant Biol. 5:22. doi: 10.1186/1471-2229-5-22
Ormrod, D. P., Woolley, C. J., Eaton, G. W., and Stobbe, E. H. (1967). Effect of temperature on embryo sac development in Phaseolus vulgaris L. Can. J. Bot. 45, 948–950. doi: 10.1139/b67-097
Osorio, E., Davis, A. R., Warkentin, T. D., and Bueckert, R. A. (2023). Ovule abortion and seed set of field pea (Pisum sativum L.) grown under high temperature. Can. J. Plant Sci. 103, 270–284. doi: 10.1139/cjps-2022-0156
Ozga, J. A., Reinecke, D. M., Ayele, B. T., Ngo, P., Nadeau, C., and Wickramarathna, A. D. (2009). Developmental and hormonal regulation of gibberellin biosynthesis and catabolism in pea fruit. Plant Physiol. 150, 448–462. doi: 10.1104/pp.108.132027
Pandey, S., Moradi, A. B., Dovzhenko, O., Touraev, A., Palme, K., and Welsch, R. (2022). Molecular control of sporophyte-gametophyte ontogeny and transition in plants. Front. Plant Sci. 12:789789. doi: 10.3389/fpls.2021.789789
Panoli, A., Martin, M. V., Alandete-Saez, M., Simon, M., Neff, C., Swarup, R., et al. (2015). Auxin import and local auxin biosynthesis are required for mitotic divisions, cell expansion and cell specification during female gametophyte development in Arabidopsis thaliana. PLoS One 10:6164. doi: 10.1371/journal.pone.0126164
Pasqualini, S., Tedeschini, E., Frenguelli, G., Wopfner, N., Ferreira, F., D’Amato, G., et al. (2011). Ozone affects pollen viability and NAD(P)H oxidase release from Ambrosia artemisiifolia pollen. Environ. Pollut. 159, 2823–2830. doi: 10.1016/j.envpol.2011.05.003
Petrella, R., Cucinotta, M., Mendes, M. A., Underwood, C. J., and Colombo, L. (2021). The emerging role of small RNAs in ovule development, a kind of magic. Plant Reprod. 34, 335–351. doi: 10.1007/s00497-021-00421-4
Polowick, P. L., and Sawhney, V. K. (1988). High temperature induced male and female sterility in canola (Brassica napus L). Ann. Bot. 62, 83–86.
Porch, T. G., and Jahn, M. (2001). Effects of high-temperature stress on microsporogenesis in heat-sensitive and heat-tolerant genotypes of Phaseolus vulgaris. Plant Cell Environ. 24, 723–731. doi: 10.1046/j.1365-3040.2001.00716.x
Prasad, P. V. V., Boote, K. J., Allen, L. H. A. Jr., and Thomas, J. M. G. (2002). Effects of elevated temperature and carbon dioxide on seed-set and yield of kidney bean (Phaseolus vulgaris L.). Glob. Chang. Biol. 8, 710–721. doi: 10.1046/j.1365-2486.2002.00508.x
Prasad, P. V. V., and Craufurd, P. Q.Summerfield (1999). Fruit number in relation to pollen production and viability in groundnut exposed to short episodes of heat stress. Ann. Bot. 84, 381–386. doi: 10.1006/anbo.1999.0926
Prasad, P. V. V., and Djanaguiraman, M. (2014). Response of floret fertility and individual grain weight of wheat to high temperature stress: sensitive stages and thresholds for temperature and duration. Funct. Plant Biol. 41, 1261–1269. doi: 10.1071/FP14061
Prasad, P. V. V., Pisipati, S. R., Ristic, Z., Bukovnik, U., and Fritz, A. K. (2008). Impact of nighttime temperature on physiology and growth of spring wheat. Crop Sci. 48, 2372–2380. https://doi.org/10.2135/cropsci2007.12.0717
Rämö, K., Kanerva, T., Ojanperä, K., and Manninen, S. (2007). Growth onset, senescence, and reproductive development of meadow species in mesocosms exposed to elevated O3 and CO2. Environ. Pollut. 145, 850–860. doi: 10.1016/j.envpol.2006.03.054
Rao, G. J. N., Reddy, J. N., Variar, M., and Mahender, A. (2016). “Molecular breeding to improve plant resistance to abiotic stresses” in Advances in plant breeding strategies: agronomic, abiotic and biotic stress traits. eds. J. M. Al-Khayri, S. M. Jain, and D. V. Johnson (Berlin: Springer), 283–326.
Rejón, J. D., Delalande, F., Schaeffer-Reiss, C., de Alché, J. D., Rodríguez-García, M. I., Van Dorsselaer, A., et al. (2016). The pollen coat proteome: At the cutting edge of plant reproduction. Proteomes 4:5. doi: 10.3390/proteomes4010005
Rezaul, I. M., Baohua, F., Tingting, C., Weimeng, F., Caixia, Z., Longxing, T., et al. (2019). Abscisic acid prevents pollen abortion under high-temperature stress by mediating sugar metabolism in rice spikelets. Physiol. Plant. 165, 644–663. doi: 10.1111/ppl.12759
Rodkiewicz, B. (1970). Callose in cell walls during megasporogenesis in angiosperms. Berlin: Springer-Verlag.
Rodrigo, J., and Herrero, M. (1998). Influence of intraovular reserves on ovule fate in apricot (Prunus armeniaca L.). Sex. Plant Reprod. 11, 86–93. doi: 10.1007/s004970050124
Rodrigo, J., and Herrero, M. (2002). Effects of pre-blossom temperatures on flower development and fruit set in apricot. Sci. Hortic. 92, 125–135. doi: 10.1016/S0304-4238(01)00289-8
Roitsch, T., and González, M.-C. (2004). Function and regulation of plant invertases: sweet sensations. Trends Plant Sci. 9, 606–613. doi: 10.1016/j.tplants.2004.10.009
Sachdev, S., Ansari, S. A., Ansari, M. I., Fujita, M., and Hasanuzzaman, M. (2021). Abiotic stress and reactive oxygen species: generation, signaling, and defense mechanisms. Antioxidants 10, 1–37. doi: 10.3390/antiox10020277
Sadras, V. O., Vadez, V., Purushothaman, R., Lake, L., and Marrou, H. (2015). Unscrambling confounded effects of sowing date trials to screen for crop adaptation to high temperature. Field Crop Res. 177, 1–8. doi: 10.1016/j.fcr.2015.02.024
Sagar, A., Rathore, P., Ramteke, P. W., Ramakrishna, W., Reddy, M. S., and Pecoraro, L. (2021). Plant growth promoting rhizobacteria, arbuscular mycorrhizal fungi and their synergistic interactions to counteract the negative effects of saline soil on agriculture: key macromolecules and mechanisms. Microorganisms 9:1491. doi: 10.3390/microorganisms9071491
Saini, H. S., and Aspinall, D. (1982). Abnormal sporogenesis in wheat (Triticum aestivum L.) induced by short periods of high temperature. Ann. Bot. 49, 835–846. doi: 10.1093/oxfordjournals.aob.a086310
Saini, H. S., Sedgleyc, M., and Aspinalla, D. (1983). Effect of heat stress during floral development on pollen tube growth and ovary anatomy in wheat (Triticum aestivum L.). Aust. J. Physiol. 10, 137–144.
Sakata, T., Oshino, T., Miura, S., Tomabechi, M., Tsunaga, Y., Higashitani, N., et al. (2010). Auxins reverse plant male sterility caused by high temperatures. Proc. Natl. Acad. Sci. U. S. A. 107, 8569–8574. doi: 10.1073/pnas.1000869107
Savada, R. P., Ozga, J. A., Jayasinghege, C. P. A., Waduthanthri, K. D., and Reinecke, D. M. (2017). Heat stress differentially modifies ethylene biosynthesis and signaling in pea floral and fruit tissues. Plant Mol. Biol. 95, 313–331. doi: 10.1007/s11103-017-0653-1
Schubert, S., Neubert, A., Schierholt, A., Sümer, A., and Zörb, C. (2009). Development of salt-resistant maize hybrids: the combination of physiological strategies using conventional breeding methods. Plant Sci. 177, 196–202. doi: 10.1016/j.plantsci.2009.05.011
Shen, S., Ma, S., Liu, Y., Liao, S., Li, J., Wu, L., et al. (2019). Cell wall invertase and sugar transporters are differentially activated in tomato styles and ovaries during pollination and fertilization. Front. Plant Sci. :10:506. doi: 10.3389/fpls.2019.00506
Shi, X., Han, X., and Lu, T. (2016). Callose synthesis during reproductive development in monocotyledonous and dicotyledonous plants. Plant Signal. Behav. 11:e1062196. doi: 10.1080/15592324.2015.1062196
Shi, W., Yang, J., Kumar, R., Zhang, X., Impa, S. M., Xiao, G., et al. (2022). Heat stress during gametogenesis irreversibly damages female reproductive organ in rice. Rice 15:578. doi: 10.1186/s12284-022-00578-0
Shinozaki, Y., Ezura, H., and Ariizumi, T. (2018). The role of ethylene in the regulation of ovary senescence and fruit set in tomato (Solanum lycopersicum). Plant Signal. Behav. 13:e1146844. doi: 10.1080/15592324.2016.1146844
Singh, S., Viswanath, A., Chakraborty, A., Narayanan, N., Malipatil, R., Jacob, J., et al. (2024). Identification of key genes and molecular pathways regulating heat stress tolerance in pearl millet to sustain productivity in challenging ecologies. Front. Plant Sci. 15:1443681. doi: 10.3389/fpls.2024.1443681
Sinha, R., Shostak, B., Induri, S. P., Sen, S., Zandalinas, S. I., Joshi, T., et al. (2023). Differential transpiration between pods and leaves during stress combination in soybean. Plant Physiol. 192, 753–766. doi: 10.1093/plphys/kiad114
Snider, J. L., Oosterhuis, D. M., Skulman, B. W., and Kawakami, E. M. (2009). Heat stress-induced limitations to reproductive success in Gossypium hirsutum. Physiol. Plant. 137, 125–138. doi: 10.1111/j.1399-3054.2009.01266.x
Soltani, A., Weraduwage, S. M., Sharkey, T. D., and Lowry, D. B. (2019). Elevated temperatures cause loss of seed set in common bean (Phaseolus vulgaris L.) potentially through the disruption of source-sink relationships. BMC genomics 20 20, 312. doi: 10.1186/s12864-019-5669-2
Song, G., Wang, M., Zeng, B., Zhang, J., Jiang, C., Hu, Q., et al. (2015). Anther response to high-temperature stress during development and pollen thermotolerance heterosis as revealed by pollen tube growth and in vitro pollen vigor analysis in upland cotton. Planta 241, 1271–1285. doi: 10.1007/s00425-015-2259-7
Sorour, S. G., Aiad, M. A., Ahmed, A. A., Henash, M. I. A., Metwaly, E. M., Alharby, H., et al. (2019). Yield of wheat is increased through improving the chemical properties, nutrient availability and water productivity of salt affected soils in the north delta of Egypt. Appl. Ecol. Environ. Res., 17, 8291–8306. doi: 10.15666/aeer/1704_82918306
Srinivasan, A., Saxena, N. P., and Johansen, C. (1999). Cold tolerance during early reproductive growth of chickpea (Cicer arietinum L.): genetic variation in gamete development and function. Field Crop Res. 60, 209–222. doi: 10.1016/S0378-4290(98)00126-9
Stabler, D. (2016). Impacts of ozone pollution on nectar and pollen quality and their significance for pollinators. United Kingdom: Newcastle University.
Sun, K., Hunt, K., and Hauser, B. A. (2004). Ovule abortion in Arabidopsis triggered by stress. Plant Physiol. 135, 2358–2367. doi: 10.1104/pp.104.043091
Suzuki, G. (2009). Recent progress in plant reproduction research: the story of the male gametophyte through to successful fertilization. Plant Cell Physiol 50, 1857–1864. doi: 10.1093/pcp/pcp142
Suzuki, N., Rivero, R. M., Shulaev, V., Blumwald, E., and Mittler, R. (2014). Abiotic and biotic stress combinations. New Phytol. 203, 32–43. doi: 10.1111/nph.12797
Swann, A. L. S., Hoffman, F. M., Koven, C. D., and Randerson, J. T. (2016). Plant responses to increasing CO2 reduce estimates of climate impacts on drought severity. Proc. Natl. Acad. Sci. U. S. A. 113, 10019–10024. doi: 10.1073/pnas.1604581113
Takeoka, Y., Hirop, K., Kitano, H., and Wada, T. (1991). Sexual plant pistil hyperplasia in rice spikelets as affected by heat stress. Berlin: Springer.
Tanin, M. J., Saini, D. K., Sandhu, K. S., Pal, N., Gudi, S., Chaudhary, J., et al. (2022). Consensus genomic regions associated with multiple abiotic stress tolerance in wheat and implications for wheat breeding. Sci. Rep. 12:13680. doi: 10.1038/s41598-022-18149-0
Teixeira, R. N., Ligterink, W., de França-Neto, J. B., Hilhorst, H. W. M., and da Silva, E. A. A. (2016). Gene expression profiling of the green seed problem in soybean. BMC Plant Biol. 16, 1–15. doi: 10.1186/s12870-016-0729-0
Trivellini, A., Ferrante, A., Vernieri, P., and Serra, G. (2011). Effects of abscisic acid on ethylene biosynthesis and perception in Hibiscus rosa-sinensis L. flower development. J. Exp. Bot. 62, 5437–5452. doi: 10.1093/jxb/err218
Turner, N. C., Colmer, T. D., Quealy, J., Pushpavalli, R., Krishnamurthy, L., Kaur, J., et al. (2013). Salinity tolerance and ion accumulation in chickpea (Cicer arietinum L.) subjected to salt stress. Plant Soil 365, 347–361. doi: 10.1007/s11104-012-1387-0
Vargas, Y., Mayor-Duran, V. M., Buendia, H. F., Ruiz-Guzman, H., and Raatz, B. (2021). Physiological and genetic characterization of heat stress effects in a common bean RIL population. PLoS One 16:e0249859. doi: 10.1371/journal.pone.0249859
Verma, S., Attuluri, V. P. S., and Robert, H. S. (2021). An essential function for auxin in embryo development. Cold Spring Harb. Perspect. Biol. 13:a039966. doi: 10.1101/cshperspect.a039966
Wang, B., Andargie, M., and Fang, R. (2022). The function and biosynthesis of callose in high plants. Heliyon 8:248. doi: 10.1016/j.heliyon.2022.e09248
Wang, Y., Impa, S. M., Sunkar, R., and Jagadish, S. V. K. (2021a). The neglected other half - role of the pistil in plant heat stress responses. Plant Cell Environ. 44, 2200–2210. doi: 10.1111/pce.14067
Wang, Y., Sheng, D., Zhang, P., Dong, X., Yan, Y., Hou, X., et al. (2021b). High temperature sensitivity of kernel formation in different short periods around silking in maize. Environ. Exp. Bot. 183:104343. doi: 10.1016/j.envexpbot.2020.104343
Wang, Y., Tao, H., Tian, B., Sheng, D., Xu, C., Zhou, H., et al. (2019). Flowering dynamics, pollen, and pistil contribution to grain yield in response to high temperature during maize flowering. Environ. Exp. Bot. 158, 80–88. doi: 10.1016/j.envexpbot.2018.11.007
Wang, A., Yu, X., Mao, Y., Liu, Y., Liu, G., Liu, Y., et al. (2015). Overexpression of a small heat-shock-protein gene enhances tolerance to abiotic stresses in rice. Plant Breed. 134, 384–393. doi: 10.1111/pbr.12289
Wardlaw, I. F. (2002). Interaction between drought and chronic high temperature during kernel filling in wheat in a controlled environment. Ann. Bot. 90, 469–476. doi: 10.1093/aob/mcf219
Whittle, C. a., Otto, S. P., Johnston, M. O., and Krochko, J. E. (2009). Adaptive epigenetic memory of ancestral temperature regime in Arabidopsis thaliana this paper is one of a selection of papers published in a special issue from the National Research Council of Canada – plant biotechnology institute. Botany 87, 650–657. doi: 10.1139/B09-030
Willemse, M. T. M., and Van Went, J. L. (1984). “The female gametophyte” in Embryology of angiosperms. ed. M. T. M. Willemse (Berlin: Springer), 159–196.
Williams, J. H., Taylor, M. L., and O’Meara, B. C. (2014). Repeated evolution of tricellular (and bicellular) pollen. Am. J. Bot. 101, 559–571. doi: 10.3732/ajb.1300423
Wu, J., Qin, Y., and Zhao, J. (2008). Pollen tube growth is affected by exogenous hormones and correlated with hormone changes in styles in Torenia fournieri L. Plant Growth Regul. 55, 137–148. doi: 10.1007/s10725-008-9268-5
Wu, L., Taohua, Z., Gui, W., Xu, L., Li, J., and Ding, Y. (2015). Five pectinase gene expressions highly responding to heat stress in rice floral organs revealed by RNA-seq analysis. Biochem. Biophys. Res. Commun. 463, 407–413. doi: 10.1016/j.bbrc.2015.05.085
Yadegari, R., and Drews, G. N. (2004). Female gametophyte development. Plant Cell 16, S133–S141. doi: 10.1105/tpc.018192
Yamakawa, H., Hirose, T., Kuroda, M., and Yamaguchi, T. (2007). Comprehensive expression profiling of rice grain filling-related genes under high temperature using DNA microarray. Plant Physiol. 144, 258–277. doi: 10.1104/pp.107.098665
Zafar, M. M., Chattha, W. S., Khan, A. I., Zafar, S., Subhan, M., Saleem, H., et al. (2023). Drought and heat stress on cotton genotypes suggested agro-physiological and biochemical features for climate resilience. Front. Plant Sci. 14:1265700. doi: 10.3389/fpls.2023.1265700
Zafar, M. M., Shakeel, A., Haroon, M., Manan, A., Sahar, A., Shoukat, A., et al. (2022). Effects of salinity stress on some growth, physiological, and biochemical parameters in cotton (Gossypium hirsutum L.) germplasm. J. Nat. Fibers 19, 8854–8886. doi: 10.1080/15440478.2021.1975596
Zhang, C. X., Feng, B. H., Chen, T. T., Zhang, X. F., Tao, L. X., and Fu, G. F. (2017). Sugars, antioxidant enzymes and IAA mediate salicylic acid to prevent rice spikelet degeneration caused by heat stress. Plant Growth Regul. 83, 313–323. doi: 10.1007/s10725-017-0296-x
Zhang, L., Ferguson, L., and Whiting, M. D. (2018). Temperature effects on pistil viability and fruit set in sweet cherry. Sci. Hortic. 241, 8–17. doi: 10.1016/j.scienta.2018.06.039
Zhang, C., Li, G., Chen, T., Feng, B., Fu, W., Yan, J., et al. (2018). Heat stress induces spikelet sterility in rice at anthesis through inhibition of pollen tube elongation interfering with auxin homeostasis in pollinated pistils. Rice 11, 1–12.
Zhang, Y., Xu, J., Li, R., Ge, Y., Li, Y., and Li, R. (2023). Plants’ response to abiotic stress: mechanisms and strategies. Int. J. Mol. Sci. 24:10915. doi: 10.3390/ijms241310915
Zhang, L., Zhao, H.-K., Dong, Q.-L., Zhang, Y.-Y., Wang, Y.-M., Li, H.-Y., et al. (2015). Genome-wide analysis and expression profiling under heat and drought treatments of HSP70 gene family in soybean (Glycine max L.). Front Plant Sci 6:773. doi: 10.3389/fpls.2015.00773
Zheng, Y. Y., Lin, X. J., Liang, H. M., Wang, F. F., and Chen, L. Y. (2018). The long journey of pollen tube in the pistil. Int. J. Mol. Sci. 19:529. doi: 10.3390/ijms19113529
Zhou, H. C., Jin, L., Li, J., and Wang, X. J. (2016). Altered callose deposition during embryo sac formation of multi-pistil mutant (mp1) in Medicago sativa. Genet. Mol. Res. 15, 10–4238. doi: 10.4238/gmr.15027968
Zinn, K. E., Tunc-Ozdemir, M., and Harper, J. F. (2010). Temperature stress and plant sexual reproduction: uncovering the weakest links. J. Exp. Bot. 61, 1959–1968. doi: 10.1093/jxb/erq053
Zinselmeier, C., Jeong, B. R., and Boyer, J. S. (1999). Starch and the control of kernel number in maize at low water potentials. Available at: https://academic.oup.com/plphys/article/121/1/25/6085199.
Zinselmeier, C., Lauer, M. J., and Boyer, J. S. (1995). Reversing drought-induced losses in grain yield: sucrose maintains embryo growth in maize. Crop Sci. 35, 1390–1400. doi: 10.2135/cropsci1995.0011183X003500050022x
Keywords: abiotic stress, climate change, ovule development, seed development, seed production
Citation: Erfatpour M, MacLean D, Lahlali R and Jiang Y (2024) Ovule and seed development of crop plants in response to climate change. Front. Sustain. Food Syst. 8:1495610. doi: 10.3389/fsufs.2024.1495610
Edited by:
Raju Datla, Global Institute for Food Security, CanadaReviewed by:
Nasreen Bano, University of Pennsylvania, United StatesWaqas Shafqat Chattha, University of Agriculture, Faisalabad, Pakistan
Copyright © 2024 Erfatpour, MacLean, Lahlali and Jiang. This is an open-access article distributed under the terms of the Creative Commons Attribution License (CC BY). The use, distribution or reproduction in other forums is permitted, provided the original author(s) and the copyright owner(s) are credited and that the original publication in this journal is cited, in accordance with accepted academic practice. No use, distribution or reproduction is permitted which does not comply with these terms.
*Correspondence: Yunfei Jiang, eXVuZmVpLmppYW5nQGRhbC5jYQ==
†Present address: Mohammad Erfatpour, Department of Plant Agriculture, University of Guelph, Guelph, ON, Canada