- 1Tecnologico de Monterrey, Escuela de Ingenieria y Ciencias, Zapopan, Mexico
- 2Tecnologico de Monterrey, Centro de Biotecnologia-FEMSA, Escuela de Ingenieria y Ciencias, Monterrey, Mexico
- 3SYMPLANTA GmbH & Co. KG, Darmstadt, Germany
Harsh environmental conditions in drylands force plants and their associated microbial communities to adapt to abiotic stresses. In semi-arid environments, climatic conditions and poor agricultural management have a strong impact on plant yield and thus, enhancing soil fertility by means of beneficial microorganisms such as plant growth-promoting rhizobacteria (PGPR) has been proposed as part of sustainable agricultural management. As drylands will increase due to climate change, studying microbial community dynamics of crops under such conditions is crucial as it might favor rhizobacteria adapted to drought. While the microbiome of many native dryland crops has been characterized, the microbial community composition from non-native crops under semi-arid environmental conditions is understudied. Thus, the aim of this study was to characterize the bacterial community associated with the roots of three crops with different growth cycles, cultivated in the same semi-arid environment, to understand their microbial community composition during the season with the highest temperature in northeast Mexico. We performed high throughput sequencing of the V3-V4 region of the 16S rRNA gene from root samples of Pequin pepper, soybean and orange trees. Classified taxa were evaluated according to crop, sampling time and climatological parameters. Our findings revealed that changes in temporal dynamics of microbial communities correlate with environmental temperature. Moreover, the microbial community of pepper was more diverse and differed from that of soybean and orange. Regarding PGPR, 47.6% of the genera were shared among crops with a high relative abundance of Bacillus, but we also detected crop-specific microbial associations where Serratia was specific to orange trees and Rhodobacter to pepper. When analyzing PGPR in correlation to climatological parameters, Bacillus was found to thrive under lower precipitation rates, higher temperatures and higher evaporation rates in pepper and orange. In contrast, some PGPR commonly used in commercial biofertilizers such as Rhizobium and Azospirillum were affected by high temperatures. This study provides a better understanding of the rhizobacterial assemblies of economically relevant crops grown under a semi-arid environment.
Introduction
Drylands represent around 41% of the total Earth's land surface, being mostly composed by semi-arid regions (Huang et al., 2016) which are characterized by adverse environmental conditions that urge plants and other organisms to adapt in order to alleviate abiotic stress (Soussi et al., 2016). In drylands, plants are prone to experience high temperature, drought, high salinity and osmotic stress. Surviving these climatic conditions requires plants to adapt and acclimate, overall impacting crop yield (Vimal et al., 2017) and consequently, affecting the global production of cereals, oil crops, fruits and vegetables, and experiencing hundreds of billion-dollar losses (Dwivedi et al., 2018). In this environment, plant's growth is limited due to low nutrient availability caused by decreased primary productivity, high nutrient immobilization and loss by soil erosion (Cui et al., 2019).
Additionally, interactions of climate change and human activities have a strong impact on semi-arid locations. In recent years the intensive practices of modern agriculture have brought awareness of their environmental impact, for instance in greenhouse gas emissions and nutrient leaching. Besides, climate change has contributed to an expansion of the drylands all over the world with semi-arid regions having the largest growth (Huang et al., 2016). More than ever, finding sustainable alternatives to fertilize and protect plants against phytopathogens while increasing food production is extremely important (Ferreira et al., 2019). Therefore, practices that aim at enhancing soil fertility and at the same time maintain crop yield by supporting diverse microbiota from the rhizosphere have been proposed (Hartman et al., 2018).
The rhizosphere, which is rich in nutrients from root exudates, represents a stable and protected interface with the adjacent soil. Its microbiota is composed of bacteria and other microorganisms that collectively regulate ecosystem processes and play key roles in nutrient cycling, i.e., by decomposing organic matter as well as transforming and fixing soil nutrients (Hartman et al., 2018; Singh et al., 2019). Understanding rhizosphere communities is not trivial, as they are affected by interactions between soil types, plant species and their growth stages (Smalla et al., 2001; Xu et al., 2009; Philippot et al., 2013; Yuan et al., 2015; Qiao et al., 2017). In addition, an increasing amount of research has shown that in drylands the composition of bacterial assemblies is favored by drought adaptation to alleviate plant stress (Soussi et al., 2016). The root microbiome in these conditions is especially sensitive to disturbances. This aspect has been considered key to make drylands particularly prone to desertification caused by poor management and climatic events (Taketani et al., 2017).
Beneficial microorganisms like plant growth promoting rhizobacteria (PGPR) can be used as biofertilizer by working as nutrient solubilizers, but also strengtheners, biostimulants, and biopesticides (Mahnert et al., 2018; Wang et al., 2020). Bacteria classified as PGPR present at least one trait that will result in an increase of plant growth, e.g., nitrogen fixation, enhancing resistance to abiotic or biotic stress, or phytohormone production (Hayat et al., 2012; Gopalakrishnan et al., 2015; Lyngwi et al., 2016; Nehra et al., 2016; Zaefarian and Rezvani, 2016). PGPR have been identified in cereals (rice, wheat, barley, and maize) and important agricultural crops (soybean, potato, pepper, sugar cane, coffee, tea, and grapevine) (Numan et al., 2018). Moreover, some PGPR genera such as Pseudomonas, Rhizobium, Azospirillum, Azotobacter, Burkholderia, Bacillus, and Serratia, are present in large-scale commercial biofertilizers (Ramakrishna et al., 2019).
Insights on the impact of soil moisture on wheat rhizosphere microbiome in a semi-arid ecosystem have identified significant differences in specific taxa classified as PGPR (Mavrodi et al., 2018). However, current studies that evaluate plant root microbiome on drylands are mainly limited to native crops (Coleman-Derr et al., 2016; Fonseca-García et al., 2016; Dastogeer et al., 2018; Khan et al., 2020) and do not focus on introduced species that may require growth enhancers for their production. Hence, to produce biofertilizers specific for crops cultivated in drylands it is necessary to conduct studies of microbiota dynamics in field conditions for each crop.
The aim of this study is to characterize the rhizosphere bacterial community of three crops with different cycles growing under the same field conditions on a semi-arid ecosystem. A perennial crop of Pequin pepper (Capsicum annuum var. glabriusculum) and annual crops of soybean (Glycine max L.) and orange trees (Citrus sinensis) were analyzed to identify crop specific rhizobacteria and the effects of climatic conditions on microbial communities. Identified taxa were evaluated according to sampling time and crop type. The PGPR community was analyzed in correlation with climatological parameters characteristic of drylands. To our knowledge, this is the first study that evaluates the microbiome dynamics from non-native plants with different growth cycles in a semi-arid environment.
Materials and Methods
Site Selection, Sampling, and DNA Extraction
Pequin pepper, soybean and orange trees were sampled from the Tecnologico de Monterrey experimental field site in Hualahuises, Nuevo Leon, Mexico (24°52.50' N, 99°37.26' W). This site has been described by the Mexican Environmental and Natural Resources Secretary as a semi-arid location based on Penman's aridity index. The sampling was done in May, July and September 2017. The sites planted with soybean and pepper were in close proximity (10 m apart), whereas the orange trees and young peppers were planted ~50 m from these crops (Figure 1). Two composite soil samples were taken each at 0–30 cm depth and were analyzed by the ISO certificated company Fertilab (Guanajuato, Mexico). The soils are of a medium clay-loam texture with pH 8.1–8.5 and only slight differences were found among the sites regarding soil characteristics (Supplementary Table 1). Lateral roots were collected at a depth of 10 cm from the ground and at least 10 cm of root-length. For Pequin pepper, plants with different ages, young (2 months old) and mature (1 year old), were sampled; for the orange trees, peripheral roots under the tree canopy were taken from approximately a radial length of 2 m, details from each sampling time are resumed in Table 1. The collected roots were kept in Whirl-Pak bags at 4°C for transportation. Roots were cleaned using a sieve, washed with tap water and 96% ethanol, clean roots were cut in 1 cm long pieces and stored at −20°C.
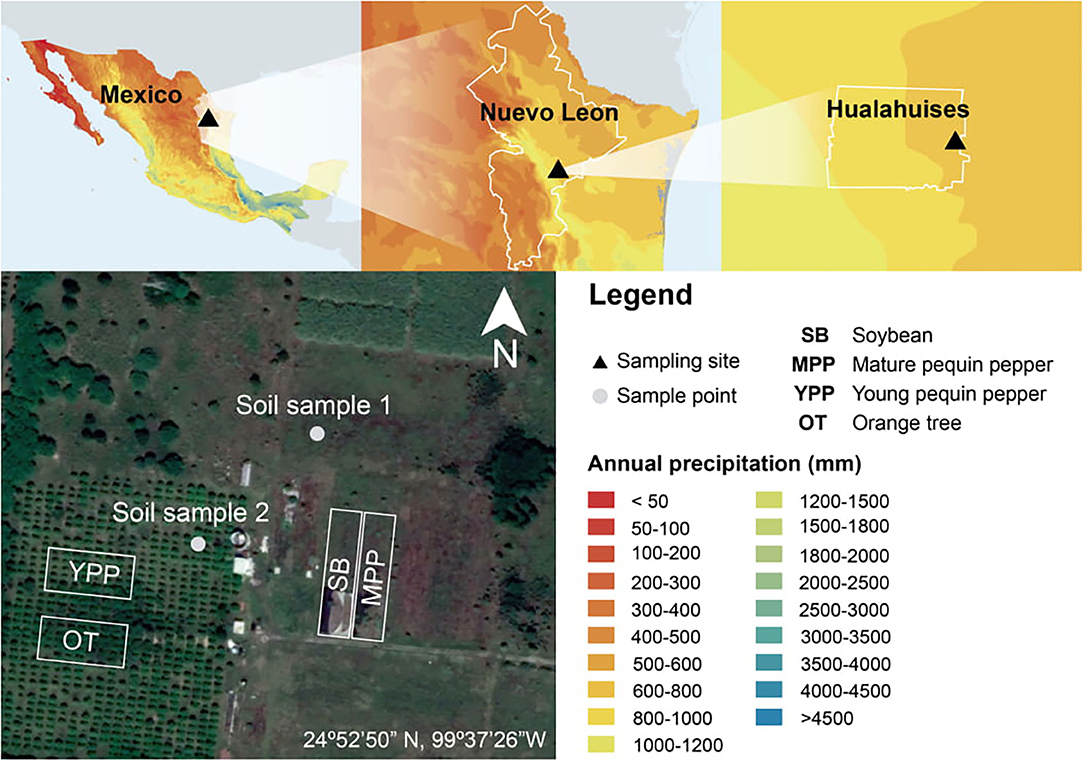
Figure 1. Map of Tecnologico de Monterrey experimental field site in Hualahuises, Nuevo Leon, Mexico (24°52.50' N, 99°37.26' W). The sampling site was cataloged as semi-arid since it had < 500 mm of annual precipitation (CONAGUA Gobierno de México, 2020).
In total, 33 samples (four biological replicates) were selected for DNA extraction performed by the FastDNA Spin Kit for Soil, according to the standard protocol, with the lysing matrix type A, but with one extra ¼ inch ceramic bead to assure complete rupture of the roots (Senés-Guerrero et al., 2014). DNA was stored at −20°C until sequencing library preparation.
PCR, Library Preparation, and Sequencing
Amplification of the variable regions V3-V4 (~550 bp, including indexes and adapters) from the 16S rRNA gene was performed using the primers Bakt_341F (5′-CCTACGGGNGGCWGCAG-3′) and Bakt_805R (5′-GACTACHVGGGTATCTAATCC-3′), following the Illumina protocol for 16S Metagenomic Sequencing Library Preparation. Each PCR was performed in triplicate per sample and visualized in a 2% agarose gel. Nextera v2 indexes (Illumina, San Diego, CA, USA) were attached to pooled PCR products according to the afore mentioned protocol. Amplicon clean-up was done using AMPure XP beads and indexed PCR products were observed in a 2% agarose gel.
The Qubit® dsDNA HS Assay Kit (Thermo Fisher Scientific, Waltham, MA, USA) was used for library quantification in a Qubit 2.0 Fluorometer (Thermo Fisher Scientific, Waltham, MA). Samples were pooled at a concentration of 6.3 pM and loaded together with 30% Phix control into an Illumina® MiSeq sequencer using the MiSeq Reagent Kit v3 (Illumina, San Diego, CA, USA) for paired-end sequencing (2 × 300 bp) carried out at the sequencing facilities of Tecnologico de Monterrey, Campus Monterrey.
Bioinformatic and Statistical Analyses
Raw reads were used as input for QIIME2 version 2019.4 (Bolyen et al., 2019) and a standard pipeline for 16S rDNA amplicon bioinformatics analysis was followed. Briefly, forward and reverse reads were joined and filtered according to Q score prior to denoising with Deblur, obtaining a table with samples grouped in Amplicon Sequence Variants (ASVs). To assign taxonomy, a classifier was constructed using 99% similarity and the GreenGenes database version 13.4. The classifier was trained using the primers and the length of the samples. Taxa bar plots were generated to assign the corresponding taxonomy to the ASV table. Files of phyla and genera were downloaded from view.qiime2.org in CSV format to continue further analysis.
Results were analyzed using RStudio (version 1.1.463; R Core Team, 2019). Rarefaction curves were constructed per sample. Diversity and richness indexes were computed with the vegan package (Oksanen et al., 2019) rarefied to the median read abundance, the samples that had less reads than the median were kept rarefied to the maximum. To analyze temporal dynamics, data was normalized with the DESeq2 package and used as input for perMANOVA, principal coordinate analysis (PCoA) and non-metric multidimensional scaling (NMDS) representing the distribution with 95% interval ellipses and eliminating the genera that were present in less than 10% of the samples. Statistical non-parametric analyses were done using the vegan package and plotted with the package ggplot2 (Wickham, 2009). Unclassified genera were denoted by “Un” and the previous taxonomic level to which they were classified. For relative read abundance, taxa bar plots and presence-absence plots were generated where pepper samples were grouped in young and mature plants. Genera with relative read abundance <1% were classified as “Others.” Genera known to promote plant growth were selected as PGPR genera. Additionally, a CIRCOS plot of the PGPR genera was constructed using the package circlize (Gu et al., 2014), relating the presence of PGPR genera to each crop.
To understand the effects of climatic conditions, Mexico's Water National Commission datasets were used to construct boxplots comparing rainfall, evaporation rate, maximal and minimal temperature of the year from which sampling was done (2017) to historic data from 1998 to 2018 (CONAGUA Gobierno de México, 2020). The climatic variables were measured at a climatological station located at 2 km from the experimental field. These climatological parameters were used to analyze their correlation with PGPR genera using Pearson's correlation with the corrplot package (Wei and Simko, 2017). Historic climatic data was also analyzed from 1998 to 2018 to classify the sampling site as semi-arid.
Results
Rhizobacteria Community Composition of Pequin Pepper, Soybean, and Orange Trees
As a first approach to describe temporal dynamics of rhizobacteria of Pequin pepper, soybean and orange trees, we characterized their root endophytic bacterial community at three sampling times in a single field site under semi-arid environmental conditions. Taxonomic classification was assigned at two distinct levels, phylum and genus (Figure 2). Regarding the most abundant phyla, crop microbial composition was highly similar among samples (Figure 2A), where the most abundant phyla were Proteobacteria ranging from 41.1 to 43.6% relative read abundance and Bacteroidetes from 37.2 to 40.7%. Among the tested crops, orange was the only one that possessed Acidobacteria and Chloroflexi in an abundance higher than 1%.
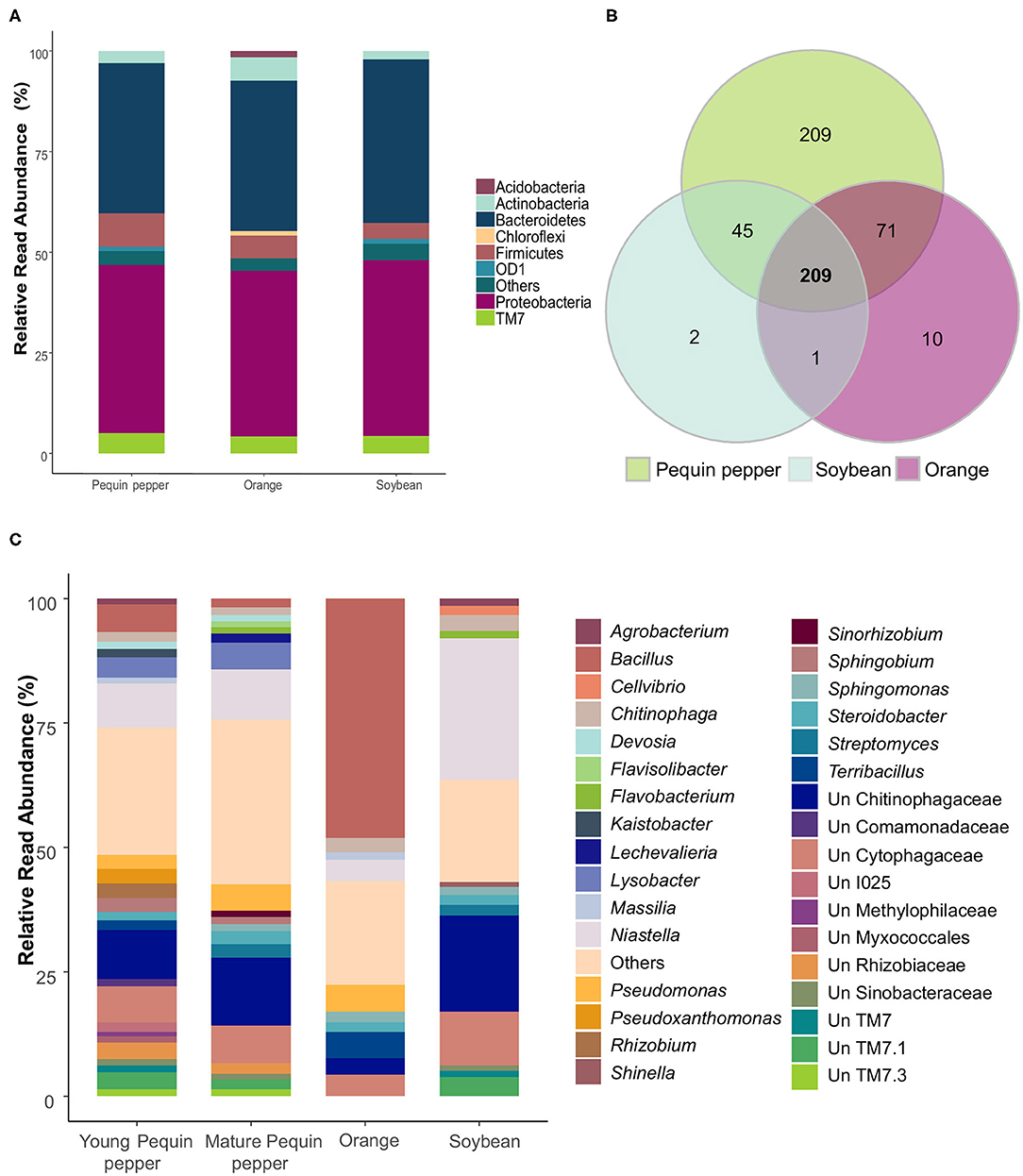
Figure 2. Bacterial community composition of Pequin pepper, soybean and orange tree. (A) Relative read abundance of phyla for each crop. (B) Venn diagram of genera shared among crops. (C) Relative read abundance of genera present in Pequin pepper (young and mature shown separately), orange and soybean. “Un” stands for unclassified to the genus level. Genera represented by < 1% were grouped as “Others”.
At the genus level, the Venn diagram showed a total of 547 genera. Five hundred thirty-four genera were identified in Pequin pepper, 291 in orange tree and 257 in soybean roots (Figure 2B). Differences in shared genera among crops revealed that the Pequin pepper had 209 specific genera, absent in the other crops, while soybean and orange had only 2 and 10 specific genera, respectively. The three crops shared 209 genera among them. Young and mature Pequin pepper samples were grouped as they did not significantly differ in microbial community composition (Supplementary Figure 2, Supplementary Table 2). They shared 45.2% of the total amount of genera that represented 55.8 and 57.1% of the relative abundance of all genera, for young and mature plants, respectively. Soybean samples had a lower abundance of unassigned genera of 17.6% compared to pepper that ranged from 43.9 to 44.5% and soybean with 46.1% (Figure 2C). In addition, orange trees and soybean possessed fewer genera with a predominance of Bacillus (48.1%) in orange and Niastella (28.3%) in soybean.
Additionally, to compare crop bacterial assemblies, as well as the two ages of pepper plants, diversity and richness at the genus level was calculated using the Shannon diversity index and Chao1 richness index. Pequin pepper showed a higher diversity and richness of the microbial root endosphere community compared to soybean but not to orange (P < 0.05) (Figure 3A); there were no significant differences between young and mature Pequin pepper (Figure 3B).
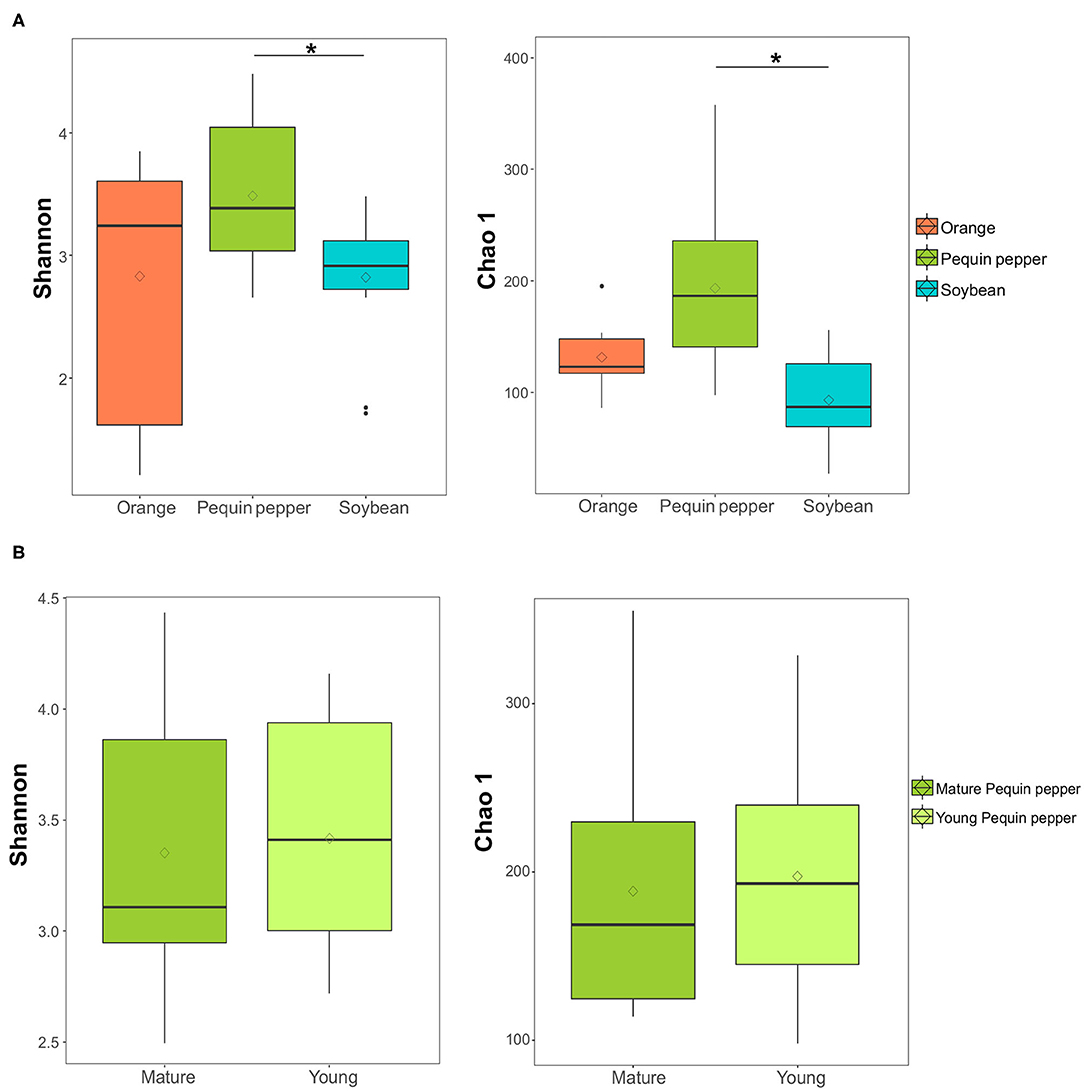
Figure 3. Differences in diversity and richness among crops. (A) Boxplots of Shannon diversity index and Chao1 richness index of crops, and (B) of mature and young Pequin pepper. Statistically significant differences (Kruskal-Wallis test, P-value < 0.05) are represented by *. Diamonds represent the average and thick black lines the median.
Crop Specificity and Temporal Dynamics of Rhizobacteria
Microbial community differences among crops, sampling times and the interaction between these parameters were shown to be statistically significant when analyzing results at the genus level (Table 2). Furthermore, these dissimilarities in microbial communities were visualized by performing NMDS and PCoAs analyses of crop and sampling time (Figure 4) and sampling time for each crop (Figure 5). Both methods yielded similar results, where rhizobacteria community composition of Pequin pepper was significantly different from that of orange and soybean (Figure 4A).
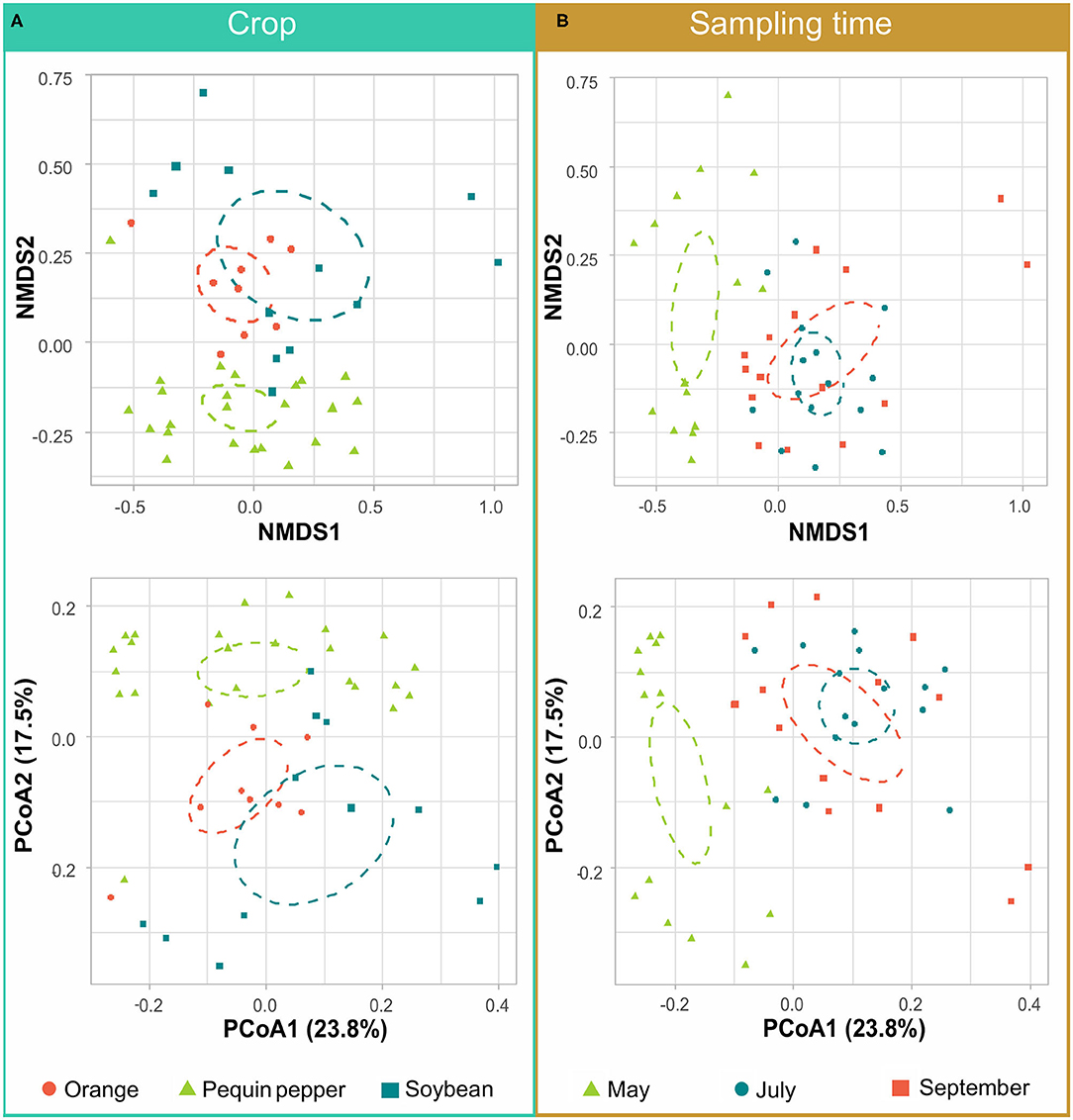
Figure 4. Non-metric multidimensional scaling (NMDS) and principal coordinate analysis (PCoA) plots show differences in community composition among crops and sampling times. Samples are grouped by crop (A) and by sampling time (B). Distribution of distinct groups is represented by 95% confidence interval ellipses. Variation explained by the data in PCoA plots is presented in percentage.
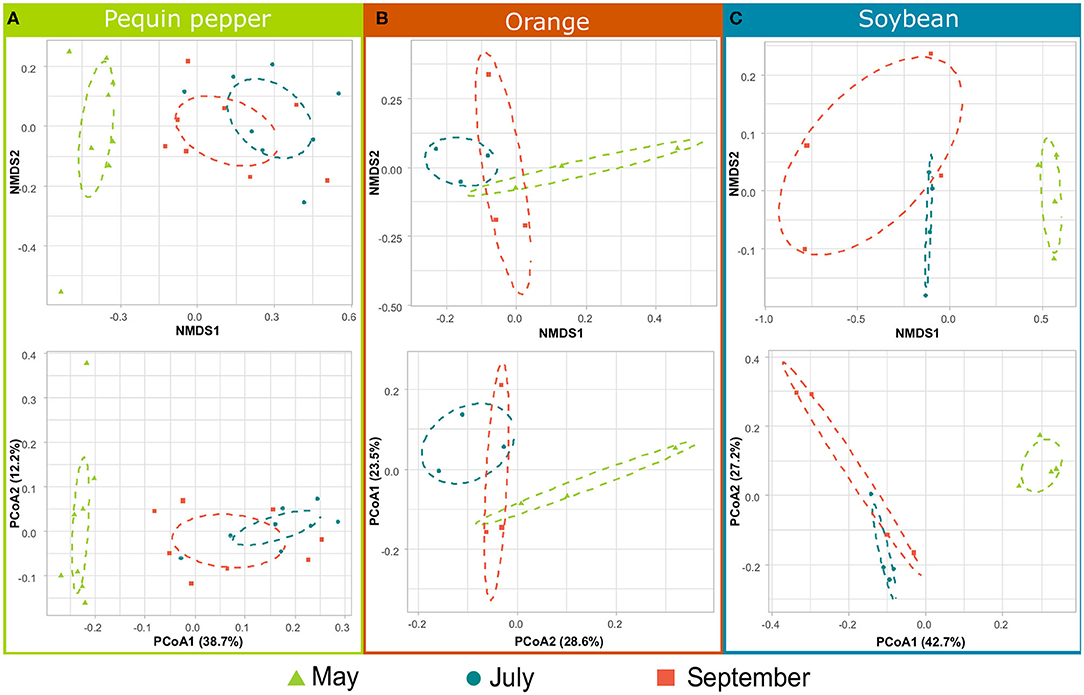
Figure 5. Non-metric multidimensional scaling (NMDS) and principal coordinate analysis (PCoA) plots show differences in community composition among sampling times for (A) Pequin pepper, (B) orange tree, and (C) soybean. Distribution of distinct groups is represented by 95% confidence interval ellipses. Variation explained by the data in PCoA plots is presented in percentage.
Regarding temporal dynamics, May samples were significantly different from July and September (Figure 4B). In the same way, differences between sampling times of individual crops resulted in May microbial communities being more dissimilar for Pequin pepper and soybean (Figures 5A,C). However, in the case of the orange trees all sampling times were similar (Figure 5B). Concerning climatological parameters during sampling, May exhibited in average lower minimum temperature (20.0 ± 3.2°C) compared to July and September (Supplementary Figure 3). July exhibited the highest average maximum evaporation rate (5.9 ± 2.0 mm; daily average) and lowest rainfall (46.1 mm; accumulated for the month), whereas September had the highest average rainfall (180.9 mm; accumulated for the month) and a maximum temperature (35.2 ± 3.1°C) very similar to May (34.4 ± 3.1°C) and July (36.7 ± 2.9°C).
Plant Growth Promoting Rhizobacteria Community by Crop and Sampling Time
Among the 547 identified genera in this study, 21 (3.84%) represented genera known to play roles as PGPR. Of these, 11 PGPR genera were found in all crops. Bacillus, Pseudomonas, and Agrobacterium were highly abundant in all crops, with variations in abundance depending on sampling time (Figure 6). Soybean exhibited the most stable PGPR community, where microbial component proportions slightly changed with time (Figure 6D). Contrary, Pequin pepper and orange tree showed strong changes in abundance of various genera such as Rhizobium, Bacillus, Pseudomonas and Sinorhizobium (Figures 6A–C). There are similarities among crops such as the presence of Agrobacterium and Bacillus in all samples at all sampling times. However, in contrast to global genera distribution, PGPR genera showed differences between young and mature Pequin peppers through sampling times (Figures 6A,B). For instance, the most abundant genus in May is Rhizobium (66.5%) in young Pequin pepper, whereas in mature Pequin pepper is Sinorhizobium (70.8%). Bacillus was found in larger quantity in July for all samples of Pequin pepper and orange, in the latter representing up to 99.1% of the PGPR community (Figure 6C). This tendency, however, is not observed in soybean samples, where Bacillus has the highest relative abundance in May with 8.21%. For soybean, there was not a high dominance of a single genus at any sampling point, where Agrobacterium, Flavobacterium, and Sinorhizobium presented high abundances (Figure 6D). The number of samples in which each PGPR genus was identified revealed that a few genera were only observed in specific crops, e.g., Serratia in orange, Azospirillum in young Pequin pepper and Rhodobacter in young and mature Pequin pepper (Figure 7A). Consequently, we found few PGPR genera specific to one crop and sampling time, such as Serratia, Azospirillum and Alicyclobacillus (Figure 7B).
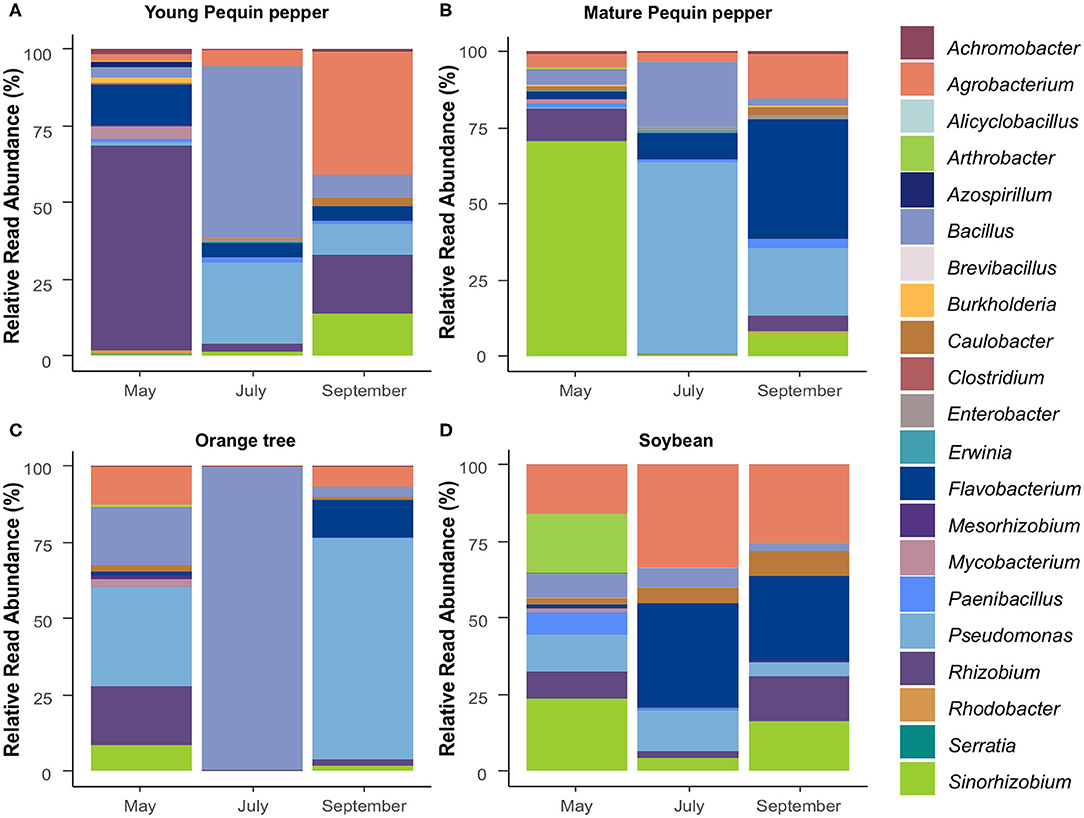
Figure 6. Genera composition of growth promoting rhizobacteria (PGPR) for young (A) and mature (B) Pequin pepper, orange tree (C), and soybean (D).
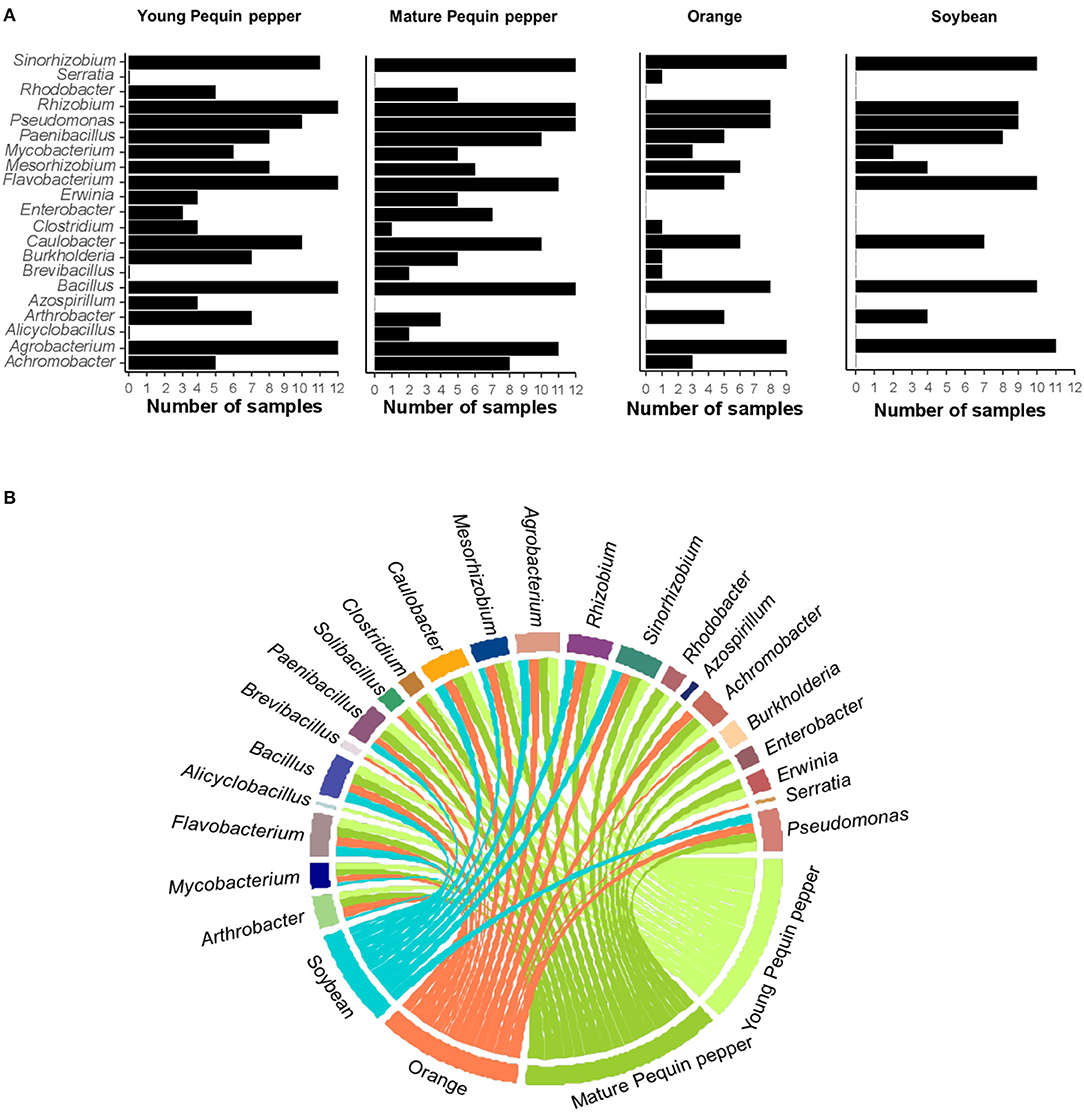
Figure 7. Presence of plant growth promoting rhizobacteria (PGPR) genera in crops during experimental period (four samples per sampling time, except for orange with three samples). (A) Bar plot of presence/absence of PGPR genera per cumulative number of samples. (B) Circus plot of PGPR genera where thickness of line represents the number of samples each genus was present in each crop.
Since PGPR genera showed differential distributions among sampling times, their correlation with climatological parameters characteristic of drylands (i.e., rainfall, evaporation rate and temperature) was tested using Pearson's correlation (Figure 8). Overall, young Pequin pepper had the highest amount of PGPR genera influenced by climatological parameters, Mycobacterium, Arthrobacter, Mesorhizobium, Rhizobium, Azospirillum, and Burkholderia were significantly affected by an increase in temperature. This trend was similar in mature Pequin pepper for Mycobacterium, Rhizobium and, specific to this crop, in Sinorhizobium. Also, in soybean Arthrobacter presence was affected by high temperatures. For orange trees, Bacillus was significantly correlated to all climatological parameters, affected by high rainfall and increasing its abundance as evaporation rate and temperature increased.
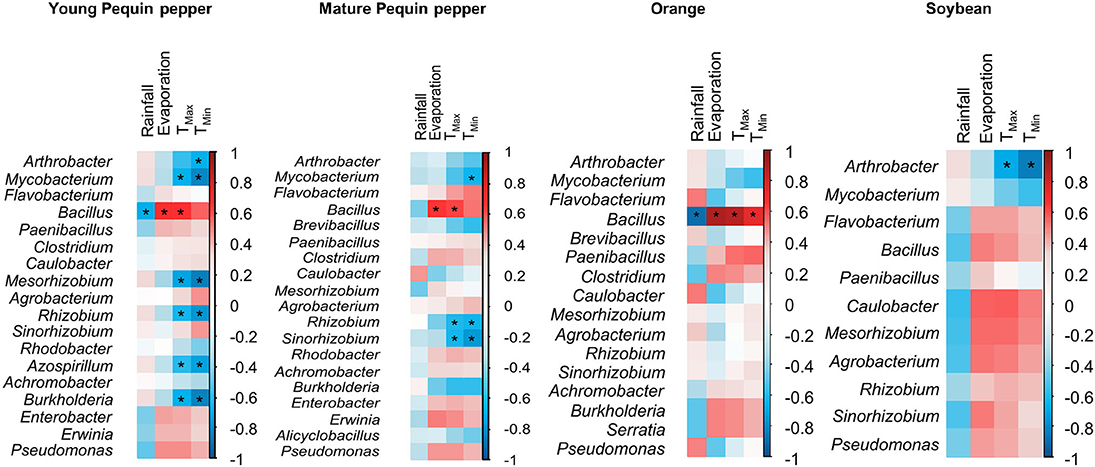
Figure 8. Plant growth promoting rhizobacteria (PGPR) correlated with climatological parameters. Correlation matrix was constructed using Pearson lineal correlation method. “*”: significant correlation (P ≤ 0.05). Red and blue color represents positive and negative correlation, respectively. +1 means a perfect positive correlation and −1 means a perfect negative correlation. Rainfall, Evaporation, Tmax and Tmin are the climatic variables measured at the climatological station located at 2 km from the experimental field during 2017.
Discussion
For semi-arid ecosystems, it has been shown that bacterial assemblies of adapted plants have a reduced diversity and are strongly influenced by climatological parameters (Taketani et al., 2017). In places with soil aridity and drought, rhizosphere microbiota is driven by plant drought stress alleviation and/or adaptation to environmental conditions (Cherni et al., 2019; see Jansson and Hofmockel, 2019 for a review). For example, Acidobacteria was shown to decrease in abundance as aridity increases, while Chloroflexi and Proteobacteria showed the opposite behavior (Maestre et al., 2015). Here, we also observed this pattern except for Chloroflexi, which we only found in an abundance of around 1% in orange tree roots and which is known for multiple adaptation mechanisms to harsh environments (Lacap et al., 2011). Moreover, Proteobacteria and Firmicutes were reported as the most abundant phyla under drought stress for orange trees (Cherni et al., 2019) but we observed a high abundance of Bacteroidetes, instead of Firmicutes, and Proteobacteria in all three crops. Contrary to the climatic conditions in our study, it was reported that both Firmicutes and Bacteroidetes increased in abundance under heavy rain and low temperatures (Št'ovíček et al., 2017). Also, a higher abundance in Bacteroidetes has been shown to be the result of rich organic root exudates composed of sugar, amino and organic acids (Sánchez-Cañizares et al., 2017).
For pepper plants (Capsicum annuum L.) cultivated in arid ecosystems, it was found that the root endosphere is colonized by a restricted community of bacteria compared to the rhizosphere and adjacent soil (Marasco et al., 2012), but no information is available on whether pepper plant-age affects the microbial communities. Interestingly, no statistical difference was found when analyzing young and mature Pequin pepper root bacteria at the genus level, which is in contrast to some other plants (e.g., rice) where endophytic microbiota communities are characteristic of younger plants when exposed to drought stress (Edwards et al., 2018). In soybean, the rhizosphere community has been reported as a subset of the bulk soil bacterial assembly with some microbial groups being associated with the rhizosphere, such as bacteria belonging to the phyla Chloroflexi, Proteobacteria, Acidobacteria, and Bacteroidetes. These associations are related to plant needs of nutrient uptake and growth enhancement, impacting the diversity of the root microbiome (Mendes et al., 2014).
The dynamics of bacterial assemblies can be related to climatic stressors and plant development (Cao et al., 2019). Bacterial communities in drylands are affected significantly by soil aridity index (evaporation rate/rainfall), total water holding capacity, total organic carbon, total nitrogen and pH (Wang et al., 2017). Here, results indicate that significant differences are due to crop specificity and climatic conditions, most pronounced in May. Regarding climatic conditions, even though July had the harshest environmental circumstances due to the lowest average rainfall and highest temperature and evaporation rate, we hypothesize that significant differences in May root microbiome could have been the result of low or nearly lacking rainfall in the previous 4 months (Supplementary Figure 3). In contrast to July, higher rainfall and a lower evaporation rate occur in September. In leguminous trees, a higher diversity was observed during rainy season compared to the dry season, even though the taxa abundance distribution remained consistent (Taketani et al., 2017). Indeed, microbial response to rain is shown to be dependent not only on its intensity but also on ambient temperature (Št'ovíček et al., 2017). This may indicate that changes in microbial communities may be observed after September and October, the only 2 months where rainfall increases.
With respect to crop specificity, pepper is reported to be more sensitive to water stress, requiring a specific bacteria consortium to reduce water deprivation such as high abundances of Bacillus spp. and Klebsiella spp., known to improve drought stress (Marasco et al., 2012). For orange trees, Bacillus was the most abundant genus at this time, whereas Bacillus has generally been reported as a dominant genus of the endophytic communities in Citrus (Wu and Srivastava, 2012). In the case of soybean, sampling times corresponded to plant developmental stages (i.e., vegetative, flowering and fruiting, and senescent). It has been previously recognized that for soybean the variation in rhizosphere bacterial communities is more influenced by plant growth than by environmental factors (Sugiyama, 2019), which may be reflected by the young soybean microbial composition differing from that of mature or senescent plants, in this study. In comparison, the orange tree root microbiome remained more static, which is in accordance to previous reports indicating that tree-microbiome interactions differ from annual plants, since trees need decades to grow and strongly depend on the nutrients from the soil (Colin et al., 2017).
Adaptation to the harsh environment of drylands leads to changes in root bacterial assemblies, increasing the presence of PGPR (Dimkpa et al., 2009). When analyzing the correlation of climatological parameters and PGPR genera, Bacillus was found to increase its relative abundance under high temperature and evaporation rates as well as under low rainfall. In this matter, it has been previously reported that Bacillus species enhance resistance to drought stress in various plants (Dimkpa et al., 2009) by inducing systemic resistance and creating a primed physiological state that may cause increased tolerance against abiotic stress (Chakraborty et al., 2006). PGPR strains development for field application is often hampered by the difficulty of bacteria survival under harsh environmental conditions, including temperature (Kaushal and Wani, 2016). Indeed, Arthrobacter, Azospirillum, Burkholderia, Rhizobium, Mesorhizobium, and Sinorhizobium were shown to be affected by high temperature (Huang et al., 2017). Burkholderia spp. have been reported to reduce evapotranspiration under drought stress and improve survival of maize (Huang et al., 2017). Azospirillum, Rhizobium, Mesorhizobium, and Sinorhizobium species have nitrogen fixing activity, form symbiosis with plants and promote their growth (Antoun and Kloepper, 2001; Zarea, 2017). While Azotobacter spp. also can fix nitrogen in a non-symbiotic way, other plant growth promoting traits like phytohormone production and phosphorous solubilization are responsible of promoting plant growth (Antoun and Kloepper, 2001; El_Komy et al., 2020). Arthrobacter spp. have caused mild biotic stress in pepper plants while increasing plant biomass and induced osmotic stress relief in a controlled environment (Sziderics et al., 2007). PGPR genera susceptibility to high temperature is problematic since drylands are extremely prone to present heat stress. Since young Pequin pepper plants showed more PGPR genera affected by high temperatures, our results indicate that they presented PGPR more susceptible to climatic conditions than mature plants. Consistently, it has been reported that the root microbiota community presents high dynamics during the vegetative plant growth phase and stabilizes in composition during the remaining plant life cycle (Edwards et al., 2018); once well-established, the root associated bacteria assembly is structurally more robust (Dombrowski et al., 2017).
In conclusion, pepper root bacterial community dynamics in drylands may be explained by environmental stresses such as long periods of drought, short seasons with rainfall and high temperature and evaporation rates. While these environmental conditions can possibly shape bacterial population in pepper (perennial), for soybean, which is an annual plant, growth stages may be more significant than climatic conditions. For orange trees, the microbial assemblies were more robust. Overall, the presence of Bacillus was correlated to a decrease in rainfall and increase of temperature and evaporation. Our results revealed significant preferential crop-microbe associations, as well as different profiles of PGPR genera affected by rainfall, evaporation rate and temperature. Additionally, several drought stress resistance mediating bacteria may be susceptible to high temperature. Together, these findings suggest that dynamics of root bacterial assemblies are influenced by crop type and semi-arid climatic conditions. Moreover, they provide a better understanding of the suitability of commonly used PGPR genera to promote plant growth on drylands. Further efforts are needed to evaluate potential PGPR candidates for biofertilizers under these conditions.
Data Availability Statement
The datasets presented in this study can be found in online repositories. The names of the repository/repositories and accession number(s) can be found at: https://www.ncbi.nlm.nih.gov/bioproject/PRJNA657454, PRJNA657454.
Author Contributions
AMD-G performed bioinformatic and statistical analyses. AS and CS-G conceived the research project. AP, JIF-R, and CS-G collected field samples, constructed the library for Illumina sequencing, and sequenced the samples. MSG-H performed statistical analyses. All authors wrote and revised the manuscript.
Funding
This study was funded by the Mexican-German bilateral scientific and technological cooperation project, FONCICYT-CONACYT-National Council of Science and Technology of Mexico (grant no. 267782) and BMBF Germany (grant no. 01DN17031). CS-G postdoctoral fellowship no. 253929 was provided by CONACYT and Tecnologico de Monterrey Emerging Technologies Research Chair (GIEE EICIM01).
Conflict of Interest
The authors declare that the research was conducted in the absence of any commercial or financial relationships that could be construed as a potential conflict of interest.
Acknowledgments
We are grateful to Cristina Chuck and Juan Valiente for giving us access to the plants in the field site and to Diego Diaz-Vazquez for his collaboration with geographic localization and data research.
Supplementary Material
The Supplementary Material for this article can be found online at: https://www.frontiersin.org/articles/10.3389/fsufs.2020.602283/full#supplementary-material
References
Antoun, H., and Kloepper, J. (2001). “Plant growth promoting rhizobacteria (PGPR),” in Encyclopedia of Genetics eds Brenner, S., and Miller, J. F., (San Diego, CA: Academic Press), 1477–1480. doi: 10.1006/rwgn.2001.1636
Bolyen, E., Rideout, J. R., Dillon, M. R., Bokulich, N. A., Abnet, C. C., Al-Ghalith, G. A., et al. (2019). Reproducible, interactive, scalable and extensible microbiome data science using QIIME 2. Nat. Biotechnol. 37, 852–857. doi: 10.1038/s41587-019-0209-9
Cao, M., Jia, T., Mi, J., Jing, J., and Chai, B. (2019). Relative roles of niche and neutral processes on turnover of plant, fungal and bacterial communities in arid and semi-arid areas at the regional scale. Basic Appl. Ecol. 40, 43–54. doi: 10.1016/j.baae.2019.08.005
Chakraborty, U., Chakraborty, B., and Basnet, M. (2006). Plant growth promotion and induction of resistance in Camellia sinensis by Bacillus megaterium. J. Basic Microbiol. 46, 186–195. doi: 10.1002/jobm.200510050
Cherni, M., Ferjani, R., Mapelli, F., Boudabous, A., Borin, S., and Ouzari, H.-I. (2019). Soil parameters drive the diversity of citrus sinensis rhizosphere microbiota which exhibits a potential in plant drought stress alleviation. Appl. Soil Ecol. 135, 182–193. doi: 10.1016/j.apsoil.2018.12.006
Coleman-Derr, D., Desgarennes, D., Fonseca-Garcia, C., Gross, S., Clingenpeel, S., Woyke, T., et al. (2016). Plant compartment and biogeography affect microbiome composition in cultivated and native agave species. New Phytol. 209, 798–811. doi: 10.1111/nph.13697
Colin, Y., Nicolitch, O., Van Nostrand, J. D., Zhou, J. Z., Turpault, M.-P., and Uroz, S. (2017). Taxonomic and functional shifts in the beech rhizosphere microbiome across a natural soil toposequence. Sci. Rep. 7:9604. doi: 10.1038/s41598-017-07639-1
CONAGUA Gobierno de México (2020). Información Estadística Climatológica. Resource Document. CONAGUA. Available online at : https://smn.conagua.gob.mx/es/climatologia/informacion-climatologica/informacion-estadistica-climatologica (accessed July 19, 2020).
Cui, Y., Fang, L., Guo, X., Han, F., Ju, W., Ye, L., et al. (2019). Natural grassland as the optimal pattern of vegetation restoration in arid and semi-arid regions: evidence from nutrient limitation of soil microbes. Sci. Total Environ. 648, 388–397. doi: 10.1016/j.scitotenv.2018.08.173
Dastogeer, K. M. G., Li, H., Sivasithamparam, K., Jones, M. G. K., and Wylie, S. J. (2018). Host specificity of endophytic mycobiota of wild nicotiana plants from arid regions of northern Australia. Microb. Ecol. 75, 74–87. doi: 10.1007/s00248-017-1020-0
Dimkpa, C., Weinand, T., and Asch, F. (2009). Plant-rhizobacteria interactions alleviate abiotic stress conditions: plant-rhizobacteria interactions. Plant Cell. Environ. 32, 1682–1694. doi: 10.1111/j.1365-3040.2009.02028.x
Dombrowski, N., Schlaeppi, K., Agler, M. T., Hacquard, S., Kemen, E., Garrido-Oter, R., et al. (2017). Root microbiota dynamics of perennial Arabis alpina are dependent on soil residence time but independent of flowering time. ISME J. 11, 43–55. doi: 10.1038/ismej.2016.109
Dwivedi, S. L., Siddique, K. H. M., Farooq, M., Thornton, P. K., and Ortiz, R. (2018). Using biotechnology-led approaches to uplift cereal and food legume yields in dryland environments. Front. Plant Sci. 9:1249. doi: 10.3389/fpls.2018.01249
Edwards, J. A., Santos-Medellín, C. M., Liechty, Z. S., Nguyen, B., Lurie, E., Eason, S., et al. (2018). Compositional shifts in root-associated bacterial and archaeal microbiota track the plant life cycle in field-grown rice. PLoS Biol. 16:e2003862. doi: 10.1371/journal.pbio.2003862
El_Komy, M. H., Hassouna, M. G., Abou-Taleb, E. M., Al-Sarar, A. S., and Abobakr, Y. (2020). A mixture of azotobacter, azospirillum, and klebsiella strains improves root-rot disease complex management and promotes growth in sunflowers in calcareous soil. Eur. J. Plant Pathol. 156, 713–726. doi: 10.1007/s10658-019-01921-w
Ferreira, C. M. H., Soares, H. M. V. M., and Soares, E. V. (2019). Promising bacterial genera for agricultural practices: an insight on plant growth-promoting properties and microbial safety aspects. Sci. Total Environ. 682, 779–799. doi: 10.1016/j.scitotenv.2019.04.225
Fonseca-García, C., Coleman-Derr, D., Garrido, E., Visel, A., Tringe, S. G., and Partida-Martínez, L. P. (2016). The cacti microbiome: interplay between habitat-filtering and host-specificity. Front. Microbiol. 7:150. doi: 10.3389/fmicb.2016.00150
Gopalakrishnan, S., Sathya, A., Vijayabharathi, R., Varshney, R. K., Gowda, C. L. L., and Krishnamurthy, L. (2015). Plant growth promoting rhizobia: challenges and opportunities. 3 Biotech 5, 355–377. doi: 10.1007/s13205-014-0241-x
Gu, Z., Gu, L., Eils, R., Schlesner, M., and Brors, B. (2014). Circlize implements and enhances circular visualization in R. Bioinformatics 30, 2811–2812. doi: 10.1093/bioinformatics/btu393
Hartman, K., van der Heijden, M. G. A., Wittwer, R. A., Banerjee, S., Walser, J.-C., and Schlaeppi, K. (2018). Cropping practices manipulate abundance patterns of root and soil microbiome members paving the way to smart farming. Microbiome 6:14. doi: 10.1186/s40168-018-0456-x
Hayat, R., Ahmed, I., and Sheirdil, R. (2012). “An overview of plant growth promoting rhizobacteria (PGPR) for sustainable agriculture,” in Crop Production for Agricultural Improvement, eds Ashraf, M., Öztürk, M., Ahmad, M., and Aksoy, A., (Dordrecht: Springer), 557–579. doi: 10.1007/978-94-007-4116-4_22
Huang, J., Ji, M., Xie, Y., Wang, S., He, Y., and Ran, J. (2016). Global semi-arid climate change over last 60 years. Clim. Dyn. 46, 1131–1150. doi: 10.1007/s00382-015-2636-8
Huang, X.-F., Zhou, D., Lapsansky, E. R., Reardon, K. F., Guo, J., Andales, M. J., et al. (2017). Mitsuaria sp. and Burkholderia sp. from arabidopsis rhizosphere enhance drought tolerance in arabidopsis thaliana and maize (Zea mays L.). Plant Soil 419, 523–539. doi: 10.1007/s11104-017-3360-4
Jansson, J. K., and Hofmockel, K. S. (2019). Soil microbiomes and climate change. Nat. Rev. Microbiol. 18, 35–46. doi: 10.1038/s41579-019-0265-7
Kaushal, M., and Wani, S. P. (2016). Plant-growth-promoting rhizobacteria: drought stress alleviators to ameliorate crop production in drylands. Ann. Microbiol. 66, 35–42. doi: 10.1007/s13213-015-1112-3
Khan, A. L., Asaf, S. M., Abed, R. M., Chai, Y. N., Al-Rawahi, A., Mohanta, T. K., et al. (2020). Rhizosphere microbiome of arid land medicinal plants and extra cellular enzymes contribute to their abundance. Microorganisms 8:213. doi: 10.3390/microorganisms8020213
Lacap, D. C., Warren-Rhodes, K. A., McKay, C. P., and Pointing, S. B. (2011). Cyanobacteria and chloroflexi-dominated hypolithic colonization of quartz at the hyper-arid core of the atacama desert, chile. Extremophiles 15, 31–38. doi: 10.1007/s00792-010-0334-3
Lyngwi, N. A., Nongkhlaw, M., Kalita, D., and Joshi, S. R. (2016). Bioprospecting of plant growth promoting bacilli and related genera prevalent in soils of pristine sacred groves: biochemical and molecular approach. PLoS ONE 11:e0152951. doi: 10.1371/journal.pone.0152951
Maestre, F. T., Delgado-Baquerizo, M., Jeffries, T. C., Eldridge, D. J., Ochoa, V., Gozalo, B., et al. (2015). Increasing aridity reduces soil microbial diversity and abundance in global drylands. Proc. Natl. Acad. Sci. U.S.A. 112, 15684–15689. doi: 10.1073/pnas.1516684112
Mahnert, A., Haratani, M., Schmuck, M., and Berg, G. (2018). Enriching beneficial microbial diversity of indoor plants and their surrounding built environment with biostimulants. Front. Microbiol. 9:2985. doi: 10.3389/fmicb.2018.02985
Marasco, R., Rolli, E., Ettoumi, B., Vigani, G., Mapelli, F., Borin, S., et al. (2012). A drought resistance-promoting microbiome is selected by root system under desert farming. PLoS ONE 7:e48479. doi: 10.1371/journal.pone.0048479
Mavrodi, D. V., Mavrodi, O. V., Elbourne, L. D. H., Tetu, S., Bonsall, R. F., Parejko, J., et al. (2018). Long-term irrigation affects the dynamics and activity of the wheat rhizosphere microbiome. Front. Plant Sci. 9:345. doi: 10.3389/fpls.2018.00345
Mendes, L. W., Kuramae, E. E., Navarrete, A. A., van Veen, J. A., and Tsai, S. M. (2014). Taxonomical and functional microbial community selection in soybean rhizosphere. ISME J. 8, 1577–1587. doi: 10.1038/ismej.2014.17
Nehra, V., Saharan, B. S., and Choudhary, M. (2016). Evaluation of brevibacillus brevis as a potential plant growth promoting rhizobacteria for cotton (Gossypium hirsutum) crop. Springerplus 5:948. doi: 10.1186/s40064-016-2584-8
Numan, M., Bashir, S., Khan, Y., Mumtaz, R., Shinwari, Z. K., Khan, A. L., et al. (2018). Plant growth promoting bacteria as an alternative strategy for salt tolerance in plants: a review. Microbiol. Res. 209, 21–32. doi: 10.1016/j.micres.2018.02.003
Oksanen, J., Blanchet, F. G., Friendly, M., Kindt, R., Legendre, P., McGlinn, D., et al. (2019). Vegan: Community Ecology Package. Available online at: https://CRAN.R-project.org/package=vegan (accessed May 8, 2020).
Philippot, L., Raaijmakers, J. M., Lemanceau, P., and van der Putten, W. H. (2013). Going back to the roots: the microbial ecology of the rhizosphere. Nat. Rev. Microbiol. 11, 789–799. doi: 10.1038/nrmicro3109
Qiao, Q., Wang, F., Zhang, J., Chen, Y., Zhang, C., Liu, G., et al. (2017). The variation in the rhizosphere microbiome of cotton with soil type, genotype and developmental stage. Sci. Rep. 7:3940. doi: 10.1038/s41598-017-04213-7
R Core Team (2019). R: A Language and Environment for Statistical Computing. R Foundation for Statistical Computing: Vienna, Austria. Available online at: https://www.R-project.org/.
Ramakrishna, W., Yadav, R., and Li, K. (2019). Plant growth promoting bacteria in agriculture: Two sides of a coin. Appl. Soil Ecol. 138, 10–18. doi: 10.1016/j.apsoil.2019.02.019
Sánchez-Cañizares, C., Jorrín, B., Poole, P. S., and Tkacz, A. (2017). Understanding the holobiont: the interdependence of plants and their microbiome. Curr. Opin. Microbiol. 38, 188–196. doi: 10.1016/j.mib.2017.07.001
Senés-Guerrero, C., Torres-Cortés, G., Pfeiffer, S., Rojas, M., and Schüßler, A. (2014). Potato-associated arbuscular mycorrhizal fungal communities in the peruvian andes. Mycorrhiza 24, 405–417. doi: 10.1007/s00572-013-0549-0
Singh, D., Raina, T. K., Kumar, A., Singh, J., and Prasad, R. (2019). Plant microbiome: a reservoir of novel genes and metabolites. Plant Gene. 18:100177. doi: 10.1016/j.plgene.2019.100177
Smalla, K., Wieland, G., Buchner, A., Zock, A., Parzy, J., Kaiser, S., et al. (2001). Bulk and rhizosphere soil bacterial communities studied by denaturing gradient gel electrophoresis: plant-dependent enrichment and seasonal shifts revealed. Appl. Environ. Microbiol. 67, 4742–4751. doi: 10.1128/AEM.67.10.4742-4751.2001
Soussi, A., Ferjani, R., Marasco, R., Guesmi, A., Cherif, H., Rolli, E., et al. (2016). Plant-associated microbiomes in arid lands: diversity, ecology and biotechnological potential. Plant Soil. 405, 357–370. doi: 10.1007/s11104-015-2650-y
Št'ovíček, A., Azatyan, A., Soares, M. I. M., and Gillor, O. (2017). The impact of hydration and temperature on bacterial diversity in arid soil mesocosms. Front. Microbiol. 8:1078. doi: 10.3389/fmicb.2017.01078
Sugiyama, A. (2019). The soybean rhizosphere: metabolites, microbes, and beyond—a review. J. Adv. Res. 19, 67–73. doi: 10.1016/j.jare.2019.03.005
Sziderics, A. H., Rasche, F., Trognitz, F., Sessitsch, A., and Wilhelm, E. (2007). Bacterial endophytes contribute to abiotic stress adaptation in pepper plants (Capsicum annuum L.). Can. J. Microbiol. 53, 1195–1202. doi: 10.1139/W07-082
Taketani, R. G., Lançoni, M. D., Kavamura, V. N., Durrer, A., Andreote, F. D., and Melo, I. S. (2017). Dry season constrains bacterial phylogenetic diversity in a semi-arid rhizosphere system. Microb. Ecol. 73, 153–161. doi: 10.1007/s00248-016-0835-4
Vimal, S. R., Singh, J. S., Arora, N. K., and Singh, S. (2017). Soil-plant-microbe interactions in stressed agriculture management: a review. Pedosphere 27, 177–192. doi: 10.1016/S1002-0160(17)60309-6
Wang, J., Li, R., Zhang, H., Wei, G., and Li, Z. (2020). Beneficial bacteria activate nutrients and promote wheat growth under conditions of reduced fertilizer application. BMC Microbiol. 20, 1–12. doi: 10.1186/s12866-020-1708-z
Wang, J., Zhang, T., Li, L., Li, J., Feng, Y., and Lu, Q. (2017). The patterns and drivers of bacterial and fungal β-diversity in a typical dryland ecosystem of northwest China. Front. Microbiol. 8:2126. doi: 10.3389/fmicb.2017.02126
Wei, T., and Simko, V. (2017). R Package “Corrplot”: Visualization of a Correlation Matrix (Version 0.84). Available online at: https://github.com/taiyun/corrplot (accessed May 8, 2020).
Wu, Q.-S., and Srivastava, A. K. (2012). “Rhizosphere microbial communities: isolation, characterization, and value addition for substrate development,” in Advances in Citrus Nutrition, ed. Srivastava, A. K., (Dordrecht: Springer), 169–194. doi: 10.1007/978-94-007-4171-3_13
Xu, Y., Wang, G., Jin, J., Liu, J., Zhang, Q., and Liu, X. (2009). Bacterial communities in soybean rhizosphere in response to soil type, soybean genotype, and their growth stage. Soil Biol. Biochem. 41, 919–925. doi: 10.1016/j.soilbio.2008.10.027
Yuan, J., Chaparro, J. M., Manter, D. K., Zhang, R., Vivanco, J. M., and Shen, Q. (2015). Roots from distinct plant developmental stages are capable of rapidly selecting their own microbiome without the influence of environmental and soil edaphic factors. Soil Biol. Biochem. 89, 206–209. doi: 10.1016/j.soilbio.2015.07.009
Zaefarian, F., and Rezvani, M. (2016). “5 - Soybean (glycine max [L.] Merr.) production under organic and traditional farming,” in Environmental Stresses in Soybean Production, ed. Miransari, M., (San Diego: Academic Press), 103–129. doi: 10.1016/B978-0-12-801535-3.00005-X
Keywords: dryland, plant growth promoting rhizobacteria (PGPR), Mexico, sustainable agriculture, biofertilizers, 16S rRNA-based metagenomic analysis
Citation: Diaz-Garza AM, Fierro-Rivera JI, Pacheco A, Schüßler A, Gradilla-Hernández MS and Senés-Guerrero C (2020) Temporal Dynamics of Rhizobacteria Found in Pequin Pepper, Soybean, and Orange Trees Growing in a Semi-arid Ecosystem. Front. Sustain. Food Syst. 4:602283. doi: 10.3389/fsufs.2020.602283
Received: 02 September 2020; Accepted: 15 October 2020;
Published: 19 November 2020.
Edited by:
Everlon Cid Rigobelo, São Paulo State University, BrazilReviewed by:
Lamine Baba-Moussa, University of Abomey-Calavi, BeninNaeem Khan, University of Florida, United States
Anelise Beneduzi, Livestock and Irrigation, Brazil
Copyright © 2020 Diaz-Garza, Fierro-Rivera, Pacheco, Schüßler, Gradilla-Hernández and Senés-Guerrero. This is an open-access article distributed under the terms of the Creative Commons Attribution License (CC BY). The use, distribution or reproduction in other forums is permitted, provided the original author(s) and the copyright owner(s) are credited and that the original publication in this journal is cited, in accordance with accepted academic practice. No use, distribution or reproduction is permitted which does not comply with these terms.
*Correspondence: Carolina Senés-Guerrero, Y2Fyb2xpbmEuc2VuZXNAdGVjLm14
†ORCID: Adriana Pacheco orcid.org/0000-0002-9512-7674
Arthur Schüßler orcid.org/0000-0002-6459-0519
Misael Sebastián Gradilla-Hernández orcid.org/0000-0002-8236-4400
Carolina Senés-Guerrero orcid.org/0000-0002-3089-6501