- 1Department of Environmental Studies and Science, University of Winnipeg, Winnipeg MB, Canada
- 2Norwegian Polar Institute, Fram Centre, Tromsø, Norway
- 3Arctic Aquatic Research Division, Freshwater Institute, Fisheries and Oceans Canada, Winnipeg, MB, Canada
- 4Centre for Earth Observation Science, Department of Environment and Geography, The University of Manitoba, Winnipeg, MB, Canada
The rapid rate of climate change in the Arctic is causing broad-scale changes to the physical environment and biological communities. Characterizing the foraging ecology and habitat use of Arctic marine top predators of high ecological and cultural significance is essential to our understanding of their vulnerability to ecosystem change, especially when there is potential for dietary resource competition in spatially co-occurring species. We therefore undertook this study to explore dietary resource competition between seasonally sympatric ringed seals (Pusa hispida) and harp seals (Pagophilus groenlandicus) in Mittimatalik (previously known as Pond Inlet), Nunavut, Canadian Arctic. We used bulk stable isotopes (δ13C and δ15N), fatty acid-specific carbon isotopes (δ13C), fatty acid profiles, and diatom lipid biomarkers in a multi-tracer framework to track carbon and trophic pathways in liver and muscle of both seal species. Altogether, the dietary tracers showed little overlap in the diet between ringed seals and harp seals when they co-occurred in the open-water season in northwest Baffin Bay. Though a high dependence on sea ice-derived carbon sources for both species was found, dietary tracers suggested that ringed seals relied to a far greater extent on sympagic carbon sources (~80% vs. 50% for harp seals) and a greater extent of benthic/coastal prey species compared to the more pelagic feeding harp seals. Ringed seal samples were available year-round, and analyses revealed a clear seasonal pattern of foraging on sympagic and benthic prey during ice-covered months of the year and shifting to greater reliance on pelagic prey during the summer open-water season. Overall, ringed seals may be more vulnerable to changes in sea-ice dynamics in the rapidly changing Arctic than harp seals, though it remains difficult to predict future dietary plasticity and competition between co-occurring top predators in an increasingly ice-free Arctic with a changing prey community.
Introduction
Anthropogenic climate change is responsible for the approximately 1°C rise in mean global surface temperature over the past century, though the Arctic has experienced a temperature increase over twice the global rate (Overland et al., 2014; IPCC, 2021). One of the most emblematic consequences of climate warming is occurring in the cryosphere, where dramatic changes in the seasonal timing, composition, extent, and thickness of sea ice have been documented over the past decades (Serreze et al., 2007; Serreze and Stroeve, 2015; Stroeve and Notz, 2018; Post et al., 2019). The projected result of ongoing declines in sea ice is ultimately a seasonally ice-free Arctic before the end of this century (Overland and Wang, 2013). The observed and anticipated loss of Arctic sea ice is having widespread ecological consequences across the ecosystem. Ice-associated seals rely on the physical structure of sea ice for critical components of their life history cycle, including reproduction, moulting, and hunting (Laidre et al., 2008; Post et al., 2013). Timing and extent of summer sea-ice melt has important implications for the total amount and relative composition of sympagic (sea ice-derived) and pelagic primary production in the Arctic, which subsequently impact the flow of carbon and energy through all trophic levels (Post et al., 2013; Post et al., 2019; Tedesco et al., 2019; Lannuzel et al., 2020). Arctic marine biological communities are changing in structure and distribution as warmer temperatures also allow for northward expansion of temperate species (Wassmann et al., 2011; Post et al., 2013; Fossheim et al., 2015; Kortsch et al., 2015). Increasingly ice-free summers in the Arctic also facilitates the northward expansion of human activities such as tourism, shipping, fisheries, and natural resource extraction (Pizzolato et al., 2016). Altogether, a perfect storm of multiple environmental stressors for endemic species in the Arctic will be amplified over the coming century.
Given the changes occurring across the Arctic, a major unknown is how endemic species will respond to new environmental conditions and potentially increased competition with species migrating northward and/or seasonally expanding their time in the Arctic during longer ice-free summer periods. Competition between species arises when they co-occur in a region and overlap in their use of resources (e.g., prey), which may already be or become in limited supply. If resources are not partitioned (i.e. niche partitioning), competitive exclusion may lead to more adverse consequences for one of the species (Hardin, 1960). In many Arctic regions, ringed seals (Pusa hispida) and harp seals (Pagophilus groenlandicus) are at least seasonally sympatric and thought to target similar invertebrate and fish prey species (Laidre et al., 2008; Ogloff et al., 2019; Kunisch et al., 2021). The Northwest Atlantic harp seal population overwinters off the coast of eastern Canada and migrates northward after pupping and moulting to spend the summer months in Arctic Canada and Greenland (Stenson and Sjare, 1997; Stenson et al., 2020). Ringed seals, in contrast, are considered a year-round resident ice-obligate species in the Arctic (Reeves, 1998). Thus, in regions like Baffin Bay, Nunavut, Canada, the potential for competition between ringed and harp seals in the summer open-water period underlies the importance of quantifying the foraging ecology of these species. Such information also provides useful baseline information upon which to gauge the impacts of future environmental and ecosystem changes.
Characterizing the foraging ecology of marine top predators can be carried out using direct observations or stomach content analysis, though these methods are not always feasible (e.g., invasive sampling), may overrepresent certain prey taxa (e.g. hard-shelled prey), and can only provide a very short temporal representation (e.g. daily) of diet (Horswill et al., 2018). Biochemical dietary tracers are thus often used to infer animal diet from tissue samples (e.g., Ramos and González-Solís, 2012). Researchers can make use of the metabolic activity or protein turnover rates of different tissues to distinguish dietary signals at different temporal resolution. Liver and plasma have relatively fast protein turnover and thus reflect more recent diet, compared to slower turnover tissue like muscle or adipose (Ramos and González-Solís, 2012; Vander Zanden et al., 2015). Most common approaches include the analysis of bulk stable isotopes of carbon (δ13C) and nitrogen (δ15N) to infer habitat use/carbon sources and trophic position, respectively, as well as fatty acid (FA) signatures to infer trophic interactions (i.e. diet) (Hobson et al., 1995; Dalsgaard et al., 2003; Budge et al., 2006; Newsome et al., 2010). δ13C can be used to distinguish benthic, coastal, and/or sympagic carbon sources (enriched in 13C) from offshore and pelagic sources (depleted in 13C), while δ15N is used to infer trophic position as higher trophic organisms are enriched in 15N compared to their prey. Fatty acids known to derive from particular prey sources or communities relatively unchanged during trophic interactions, termed marker FAs, are used to infer information about relative (qualitative) or absolute (quantitative) diet composition. Increasingly, these traditional tracers are being complemented by additional dietary tracers that provide more specific information about carbon source origin and energy flow in marine ecosystems, such as compound-specific stable isotopes and highly branched isoprenoid (HBI) diatom lipids (Budge et al., 2008b; Brown et al., 2017; Brown et al., 2018; Belt, 2018; Kunisch et al., 2021). Unlike bulk stable isotopes, which provide an integrative estimate of all organic compounds in a sample, compound-specific stable isotope analysis offers greater specificity about sources if select compounds, like FAs, differ between sources, i.e. sympagic vs. pelagic algae (Budge et al., 2008b; Twining et al., 2020). Similarly, because some HBIs are produced selectively by sea-ice diatoms (mono and diene; sympagic HBIs) and others by pelagic phytoplankton (triene; pelagic HBI) (Brown et al., 2014a; Brown et al., 2014b; Belt, 2018), this source specificity allows for a detailed analysis of carbon sourcing in seasonal Arctic marine ecosystems. Given the advantages and limitations of each dietary tracer (Leu et al., 2020), the more comprehensive approach of varying state-of-the-art techniques is to combine multiple tracers to derive a more complete picture of species foraging ecology.
In this study, we apply a multi-dietary tracer approach, including the analyses of bulk and FA-specific stable isotopes and FAs in muscle and liver tissue, as well as HBIs in liver tissue, to investigate the foraging ecology of ringed seals and harp seals sympatric to the northwestern Baffin Bay region in Arctic Canada. Our specific objectives were to (1) compare the diet and trophic pathways between harp seals and ringed seals to describe potential food competition, (2) quantify the contribution of sympagic and pelagic primary production sources in both seal species, and (3) characterize seasonal patterns in diet and trophic pathways in ringed seals. Increased understanding of the foraging ecology of these important ecological and cultural Arctic marine species is invaluable for evaluating and predicting potential population responses to the multiple changes expected in rapidly changing Arctic ecosystems.
Material and method
Biological sampling
Ringed seal and harp seal muscle and liver samples were collected in Eclipse Sound, Nunavut from 2015 to 2017 by Inuit hunters from Mittimatalik (previously known as Pond Inlet), Nunavut (72° 42′ 0″ N, 77° 57′ 40″ W), as part of their subsistence harvests (Table 1). Tissue samples were stored at -20°C before processing. Samples were shipped to the Freshwater Institute (Winnipeg, MB) to be processed and analyzed at the Biotracers Laboratory. Ringed seal and harp seal liver and muscle were used for analysis based on the premise of a stable isotope half-life representing approximately 1-2 month and 3-6 months, respectively, based on average body masses of ringed seals and harp seals (Vander Zanden et al., 2015). Seal sex was recorded by hunters. Seal ages were estimates by Matson’s Laboratory LLC (Missoula, MT, USA) by counting annual growth layer groups in cementum of the lower right canine (Stewart et al., 2006). Standard length (straight line) was measured as the distance from the tip of the nose to the end of the tail.
Bulk stable isotope analysis
Samples of muscle and liver tissue were freeze-dried (−50°C, 0.2 mbar, 24 h), homogenized with mortar and pestle. Due to the presence of lipids in Arctic marine mammal liver and muscle (Yurkowski et al., 2015) which can bias ecological interpretations, lipids were extracted with 2:1 chloroform:methanol solvent using a modified version of the Bligh and Dyer, (1959) method. For δ13C and δ15N analysis, subsamples of 400-600 µg of sample were weighed into tin capsules. These samples were then analyzed with a continuous flow isotope ratio mass spectrometer (Delta V Plus; Thermo Scientific) interfaced with an elemental analyzer (ECS 4010; Costech Instruments) and connected via a Conflo IV interface (Thermo Scientific). Isotopic ratios are presented in delta (δ) notation: δ = [(Rsample/Rstandard) − 1] × 1000, as ‰ deviation from the primary (calibration) standards atmospheric nitrogen (δ15N) and Vienna Pee Dee Belemnite (VPDB, δ13C). The certified reference materials USGS40 (δ15N = −4.52‰, δ13C = −26.39‰) and USGS41a (δ15N = 47.55‰, δ13C = 36.55‰), provided by the International Atomic Energy Agency (IAEA), were used to verify accuracy and precision of the isotopic measurements (USGS40: SD< 0.1‰, n = 50; USGS41a: SD< 0.15‰, n = 50).
Fatty acid analysis
Prior to lipid extraction for producing fatty acid methyl esters (FAMEs), muscle and liver tissue were freeze-dried (−50°C, 0.2 mbar, 24 h) and homogenized with mortar and pestle. Total lipids were extracted with chloroform/methanol (2:1, v/v) containing 0.01% butylated hydroxytoluene and cleaned with 0.7% sodium chloride solution. Extracted lipids were converted into FAMEs by transesterification in 3% concentrated sulfuric acid in methanol at 50°C overnight. FAMEs were separated on an Agilent Technologies 7890A gas chromatograph with a DB-23 capillary column (30 m, 0.25 mm i.d., 0.15 µm film thickness) and a flame ionization detector operating at 350°C. The oven program was as follows: 60 °C for 0.66 min; 22.82 °C/min to 165 °C with a 1.97 min hold; 4.56 °C/min to 174 °C; and 7.61 °C/min to 200 °C with a 6 min hold. Samples were injected split-less at 260°C. Hydrogen was used as a carrier gas. Individual FAMEs were identified via FAME standard mixtures (Supelco 37 component FAME mix, Nu-Check GLC 455 and 463). The proportions of individual FAs were reported as percent of total FA content.
When relevant prey items are known and collected in tandem with predator samples and calibration coefficients are known, quantitative FA analysis (QFASA) can be used to estimate individual prey consumption patterns (Iverson et al., 2004). In lieu of that, qualitative methods exist to infer composition of primary production and dietary sources using well characterized marker FAs (Budge et al., 2006). Here we use the qualitative method to infer prey sources for ringed seals and grey seals from their FA profiles and well characterized prey FA markers. We use the following FA markers as dietary indicators based on (Dalsgaard et al., 2003) and Kelly and Scheibling (2012), and summarized by Pedro et al. (2020). Diatoms produce higher amounts of C16 FAs (16:4n-1, 16:1n-7, C16-PUFAs [polyunsaturated FAs]) and 20:5n-3 in comparison to dinoflagellates, which biosynthesize C18-FAs (18:1n-9, 18:4n-3, C18-PUFAs) and C22-PUFAs (e.g., 22:6n-3) in higher quantities. While both species can be found in pelagic food webs, generally ice-algae are composed primarily of diatoms (sympagic) and open water communities are dominated mostly by dinoflagellates (pelagic) (Dalsgaard et al., 2003). Long-chained C20 FAs (20:1n-9, 20:1n-11) and C22 (22:1n-9, 22:1n-11) are good indicators of a diet high in Calanus copepods, or Calanus feeding fish (pelagic). Benthic and coastal foraging markers included those for macroalgae (20:4n-6), vascular plants (18:3n-3, 18:2n-6), and bacteria (18:1n-7).
Fatty acid-specific stable isotope analysis
For compound-specific stable isotope measurements, δ13C signatures of the diatom-associated FAs 16:1n-7 and 20:5n-3, the dinoflagellate-associated FA 22:6n-3 and the benthic macroalgae marker 20:4n-6 were determined directly from the FA extracts using a Thermo GC-c-IRMS system, equipped with a Trace GC Ultra gas chromatograph, connected to the mass spectrometer via the Conflo IV interface. FAMEs were injected in split-less mode at 260°C and separated on a DB-FFAP column (30 m, 0.25 mm I.D., 0.25 µm film thickness), using a temperature program from 60°C to 240°C. For calibration of the δ13C values, the certified standard FAMEs 14:0 (δ13C = –29.8%) and 20:0 (δ13C = –30.68%), supplied by Indiana University (Department of Earth and Atmospheric Science), were used. We did not correct isotope values for any possible effect of adding a methyl group during transesterification.
Highly branched isoprenoid diatom lipid analysis
To study the seals’ recent trophic dependency on sympagic carbon (~1-2 month) (Brown et al., 2014a; Vander Zanden et al., 2015) the metabolically highly active, and high protein turnover, liver tissue was chosen for HBI analysis. Samples of ~1g of liver tissue were freeze-dried (−50°C, 0.2 mbar, 24 h), homogenized with mortar and pestle and saponified with 20% potassium hydroxide in water/methanol 1:9 (v/v) at 80°C for 1h (Brown et al., 2018). HBIs were fractionated using column chromatography with silica gel and subsequently analyzed by gas chromatography-mass spectrometry (Agilent Technologies 7890B; 5977 MSD) as previous described (Brown et al., 2018), but with a precolumn backflush and shorter gas chromatography program. In brief, the gas chromatography oven program ran from 40 to 300°C at 5°C/min with a 10 minute hold at the end. Each run was 12.6 minutes, which started at 55°C with a 0.5 minute hold, then increased to 200°C at 30°C/min, to 225°C at 5°C/min, and finally to 250°C at 30°C/min with a 1 minute hold. We used a GC column (DB-5, 30m x.25mm x 0.25um) and a 4m x 0.25mm uncoated precolumn in front of the analytical column, connected using Agilent’s purged ultimate union. The HBIs IP25 (specific mass to charge ratio [m/z] 350.30), II (m/z 348.3) and III (m/z 346.3) were quantified via their mass spectral intensities in selective ion monitoring (SIM) mode to obtain relative abundances.
HBI-fingerprint, or “H-Print”, has been proposed as an indicator of the relative composition of sympagic and pelagic carbon sources in sea ice environments where:
Pelagic HBIs included the triene compound, while sympagic HBIs included the mono (IP25) and diene compounds. H-print values for individual samples were then converted to estimates of the percentage of sympagic carbon using the following equation from Brown et al. (Brown et al., 2018):
Statistical analysis
All data analyses were conducted in Software R version 4.1.3 (R core Team, 2022). Age was compared between species using the non-parametric Mann-Whitney test because data did not meet assumptions of normality. The effect of age on bulk stable isotope values was assessed using simple linear regression. Bulk stable isotopes and FA-specific carbon isotope values were compared across species and tissue using a two-way analysis of variance (ANOVA), followed by Tukey’s post-hoc test. Results were generally considered significant with p-values below 0.05. The sub-annual temporal pattern of dietary tracers was assessed using generalized additive models (GAM), with month of the year, tissue, and standard animal length included as predictor variables. Only month was included as a non-linear term and was assessed by tissue type. GAMs were run using the R package mgcv (Wood, 2011).
For seasonal comparisons of all dietary tracers, we used the distinction set out in Young and Ferguson (Young and Ferguson, 2013) for ringed seals in the Arctic: ice cover (Jan-May), fasting (June-July), and open water (Aug-Dec). Because of sample size restrictions, we combined ice cover and fasting together to one group to test for seasonal differences between the ice-covered and open water periods. Comparison of bulk stable isotopes between seasons was carried out using isotopic niche size and overlap through the R package NicheROVER (Swanson et al., 2015). This method uses a probabilistic method to calculate a multidimensional niche region in multivariate space in a Bayesian framework. A 40% probability niche region, which represents a robust estimate of the average niche of the population (Jackson et al., 2011), for each season was calculated using δ13C and δ15N values of individuals, using uninformative priors at 1000 iterations. The two-dimensional area of the calculated probability niche was determined for each model iteration and displayed as boxplots. The percentage overlap in niche mean area between seasons was then calculated. To assess whether niche size varied by season, we calculated the probability that the posterior distribution of niche area was smaller/larger between seasons.
The visualization of FA profiles between various grouping variables (species, tissue, and season) was carried out using principal component analysis (PCA). Changes in FA profiles between selected grouping variables were tested for statistical significance using permutation ANOVA (permanova) in the R package in vegan (Oksanen et al., 2020). To identify which FAs were most influential in distinguishing profiles between select grouping variables we used the global R-values and a similarity percentages routine (SIMPER) analysis in the R package vegan (Oksanen et al., 2020).
Results
Bulk stable isotopes
Collected ringed seals were on average nine years younger than harp seals (Table 1; W=951, p=0.004). Age was not significantly related to δ13C (F1,80 = 0.96, p=0.33) or δ15N (F1,14 = 0.37, p=0.55) for ringed seals, or δ15N (F1,14 = 0.14, p=0.71) in harp seals, but was positively correlated with δ13C (F1,80 = 04.67, p=0.049) for harp seals. A two-way analysis of variance revealed that species did not differ in their δ13C values (F1,95 = 0.87, p=0.35), though muscle tissue was 0.2 (0.07-0.4) ‰ lower than liver tissue (F1,95 = 9.26, p=0.003) (Table 2). Statistically significant differences were found for δ15N between species (F1,95 = 15.25, p=0.0001) and tissue (F1,95 = 13.11, p=0.0005); ringed seals values were 1.0 (0.5-1.5) ‰ higher than harp seals and liver was 0.7 (0.3-1.1) ‰ higher than muscle δ15N (Table 2). Because harp seals were only sampled in September, analysis of variance was repeated comparing only samples collected in that month. Here, δ13C values did not differ between tissues (F1,19 = 0.11, p=0.74), but were 0.5 (0.2-0.7) ‰ higher in harp seals compared to ringed seals (F1,19 = 15.17, p=0.0009). For δ15N, harp seal liver tissue was 1.4 (0.7-2.1) ‰ higher than muscle (no difference in ringed seals), and ringed seal muscle was 1.3 (0.5-2.1) ‰ higher than harp seal muscle (no difference in liver).
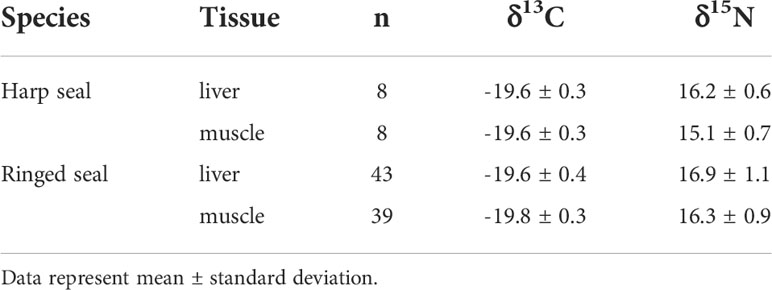
Table 2 Biological and bulk stable isotope data for ringed and harp seals collected in Mittimatalik, Nunavut.
Although we were unable to assess seasonality in harp seals, δ13C varied over the year in both tissues of ringed seal, with the temporal pattern being much stronger in liver than muscle (Figure 1A). δ13C in liver peaked in July and reached its lowest point in September and October (Figure 1A). δ15N trends were only significant in liver tissue and followed a similar pattern to δ13C, with a seasonal low occurring in September and October (Figure 1B). Further evidence of seasonal shifts in isotope values were apparent in biplots and differences were greater in the liver than muscle (Figure 1C). In liver tissue, samples taken during the ice-covered season were characterized by higher δ15N (W=254, p=0.008) and δ13C (t40.92=2.66, p=0.011) values (Figure 1C). The median isotopic niche size was greater in the ice cover (0.86‰2) compared to open water (0.47‰2), with an overall probability that the niche size was larger during the ice-covered than the open water period of 96.9%. In addition, the niche area overlap was only 26.0% (Figure 1C). In muscle tissue, there were no statistically significant difference in δ13C (t32.57 =1.44, p=0.16) or δ15N (W=148, p=0.68) between seasons (Figure 1C). Muscle tissue niche areas had greater seasonal overlap (44.0%) compared to liver, and median niche size was also greater in ice cover (0.79‰2) compared to the open-water (0.39‰2). There was a probability of 98.9% that ice cover niche size was larger than that in open-water (Figure 1C).
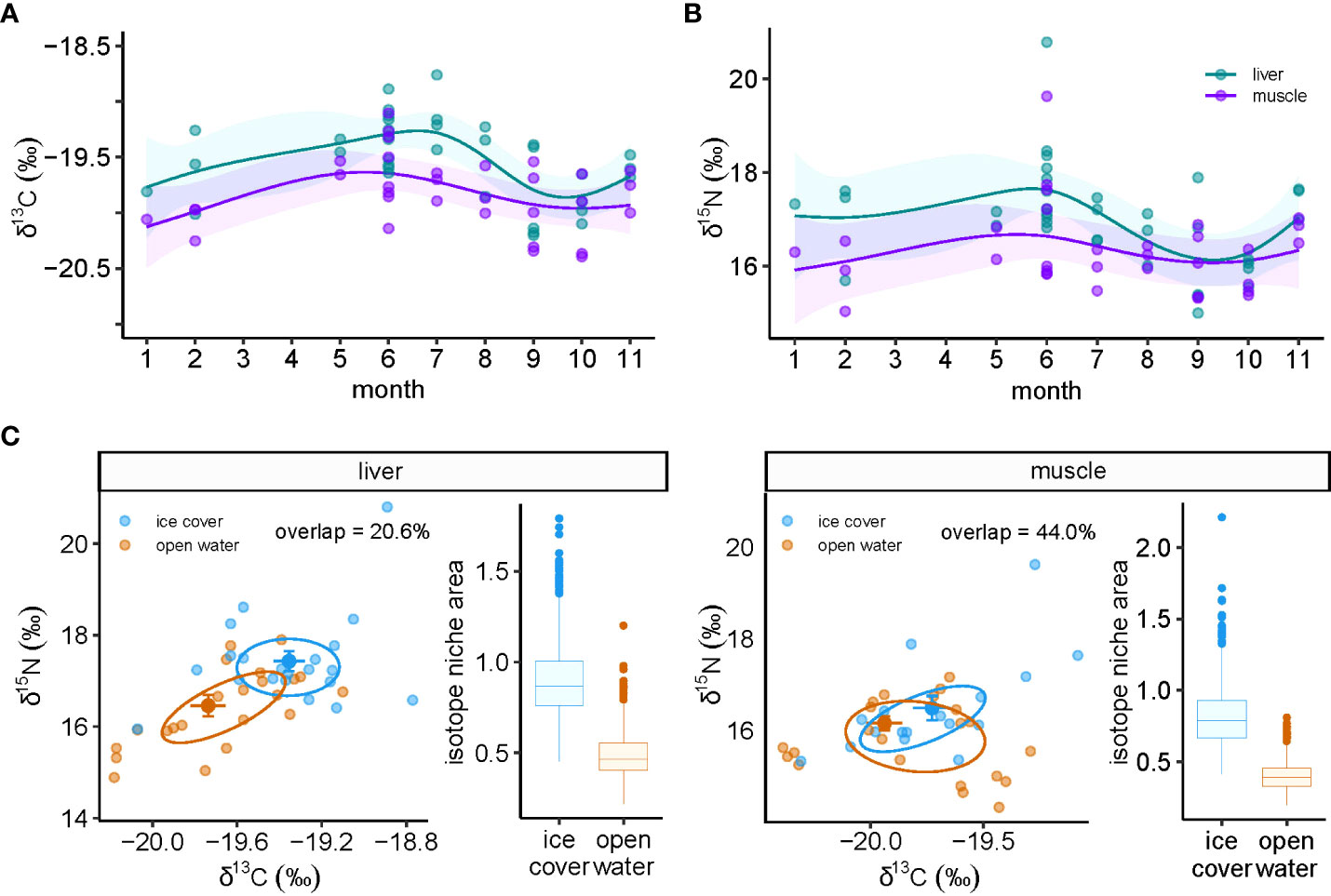
Figure 1 Temporal dynamics of bulk stable isotopes of carbon (δ13C) and nitrogen (δ15N) in ringed seal muscle and liver tissue collected in Mittimatalik, Nunavut. (A) Sub-annual δ13C trends. (B) Sub-annual δ15N trends. Dots represent individual samples and lines show the predicted curves for each tissue and the corresponding 95% confidence intervals from the GAM, adjusted for animal standard length. (C) Isotope biplots and niche area boxplots for liver and muscle tissue where color code represents seasons of sample collection. Isotopic niche ellipses were calculated on core niche size of 40%. Percentage of niche overlap between seasons is also provided.
Fatty acid composition
The major FAs in ringed and harp seal tissue were similar, with 16:0, 16:1n-7, 18:0, 18:1n-9, 18:1n-7, 20:1n-9, 20:4n-6, 20:5n3, and 22:1n-11 generally dominating the profiles (Table 3). The FA composition varied between species and tissues, though tissue differences were far greater than species differences (Figure 2A). Age was not significantly correlated with either of the first two principal components (p>0.10 for both species). The FA profile between liver and muscle tissue were significantly different (F1,94 = 114.87, R2 = 0.55, p<0.001), with greater relative proportions of 18:0, 18:1n-7, 20:4n-6 and 20:5n-3 in liver, and greater proportions of 14:0, 16:0, 16:1n-7, 18:1n-9, 20:1n-9, and 22:1n-11 in muscle vs. liver tissue (Figure 2A). These were the most influential FAs in the SIMPER analysis and accounted for 82.4% of the tissue dissimilarity. Overall, saturated and polyunsaturated FAs were higher in liver, while monounsaturated FAs were higher in muscle (Table 3).
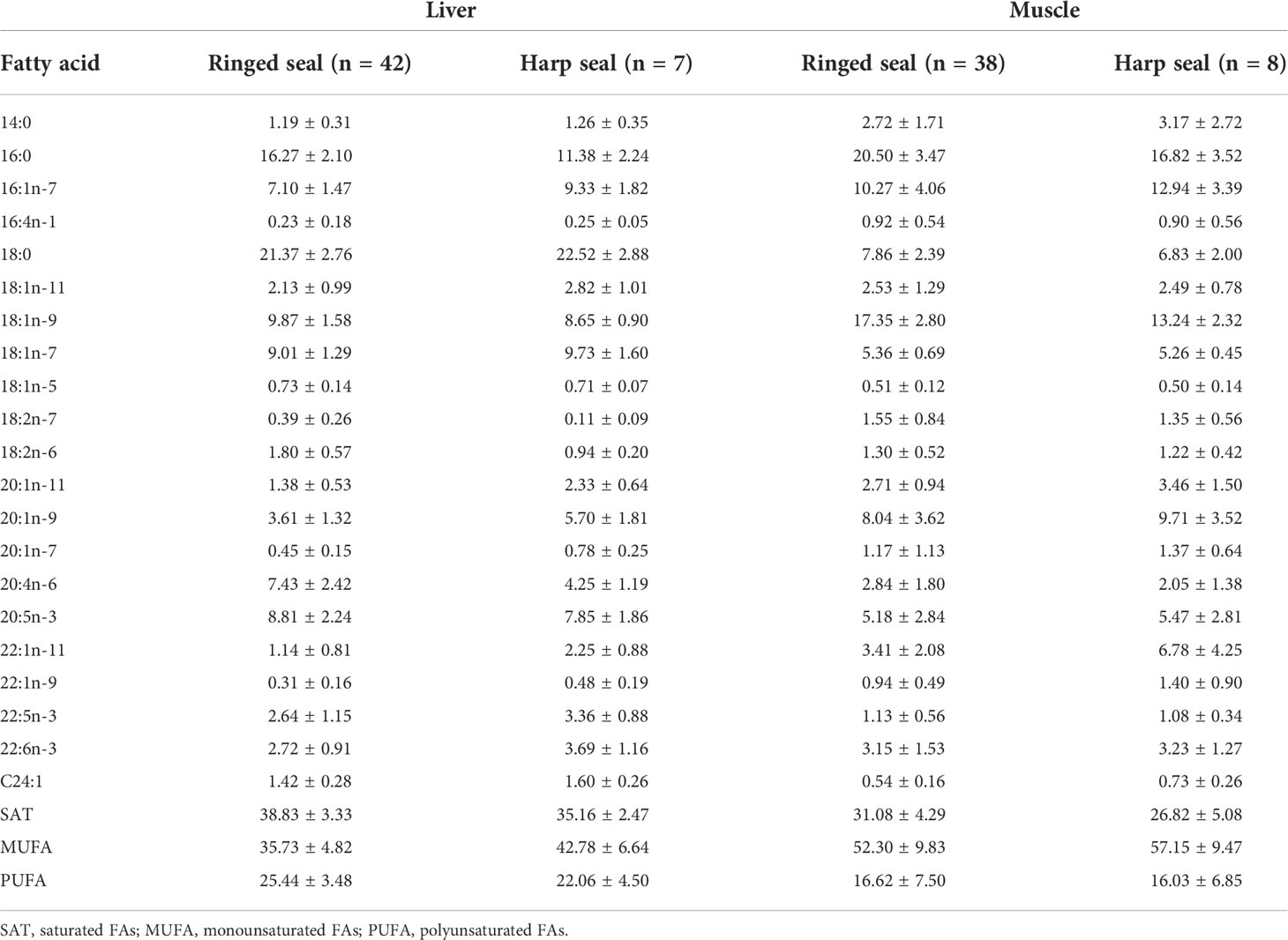
Table 3 Relative proportions (mean ± 1 SD) of fatty acids (FAs) with a mean contribution ≥0.5% to the fatty acid content in ringed seal muscle and liver tissue collected in Mittimatalik, Nunavut.
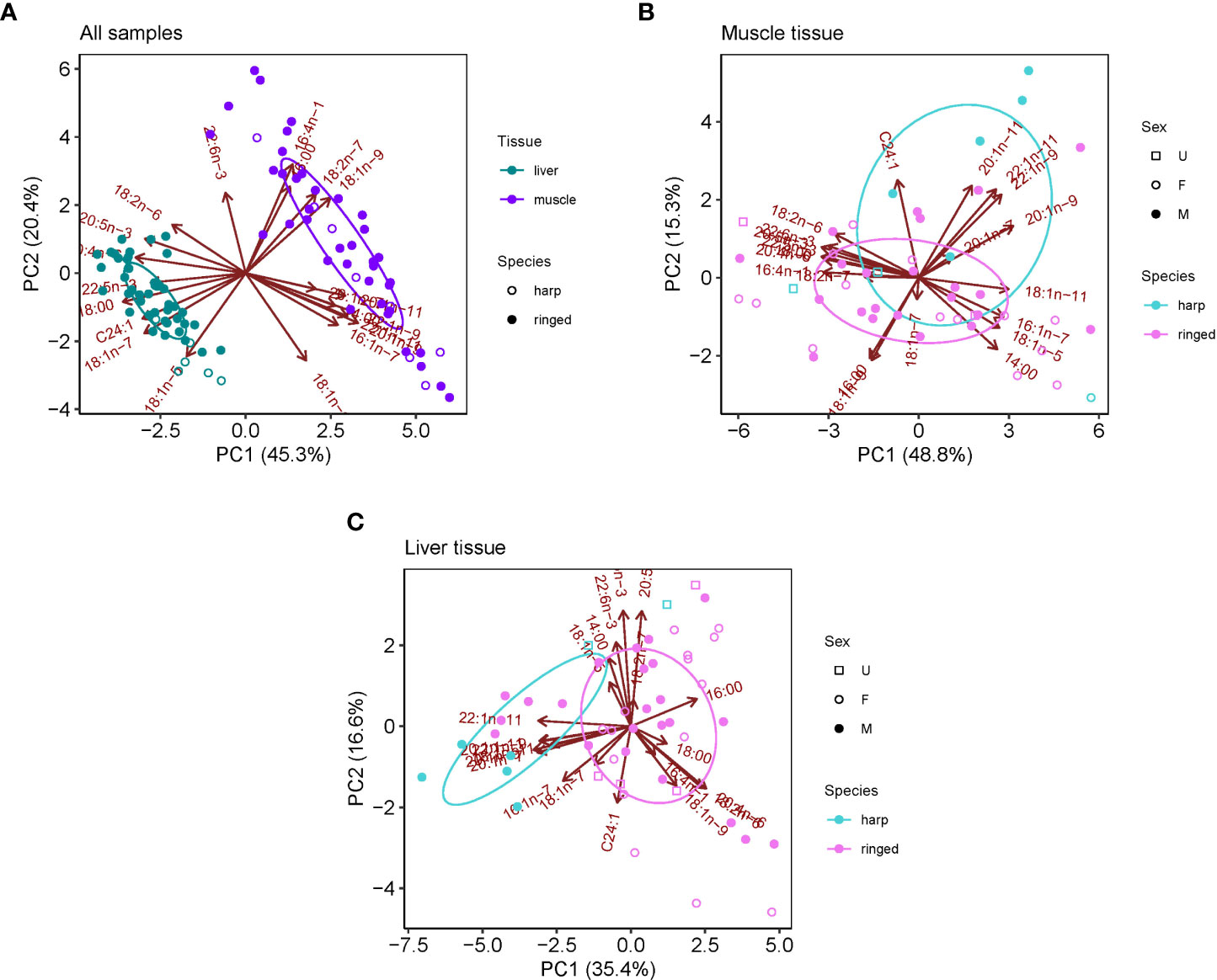
Figure 2 Principle component analyses (PCA) for fatty acid composition in ringed and harp seal tissues collected in Mittimatalik, Nunavut. (A) Liver and muscle tissue for both seal species. (B) Muscle tissue only for both species. (C) Liver tissue only for both species. Ellipses represent core fatty acid niches of 40%.
Differences in the FA profile between species was significantly greater in liver (F1,48 = 9.078, R2 = 0.16, p<0.001) than in muscle tissue (F1,45 = 3.592, R2 = 0.07, p=0.020) (Figures 2B, C). In muscle, the most influential FAs (81.1% of dissimilarity) included Calanus markers 22:1n-11, 20:1n-9, and 20:1n-11 enriched in harp seals, and dinoflagellate markers 18:1n-9, diatom markers 16:1n-7 and 20:5n-3, benthic macroalgae marker 20:4n-6, and saturated FAs 18:0 and 14:0 relatively enriched in ringed seals. In liver, harp seals had greater proportion of Calanus markers 20:1n-9, 20:1n-11 and 22:1n-11, and diatom marker 16:1n-7, and ringed seals greater proportions of dinoflagellate markers 18:1n-9 and 22:6n-3, benthic macroalgae marker 20:4n-6, diatom marker 20:5n-3, and vascular plant marker 18:2n-6. Regardless of tissue, sex was not found to be an important driver of FA profiles in the PCAs (Figure 2).
There was a distinct seasonal pattern in the FA profile of ringed seals, though it was only evident in liver tissue (Figure 3). In muscle tissue, no statistical difference was found in the FA profile between samples collected during ice-covered and open-water seasons (F1,31 = 0.697, R2 = 0.03, p=0.51; Figure 3A). In liver tissue, FA profiles differed significantly (F1,34 = 2.465, R2 = 0.07, p=0.034), with samples taken during summer open-water months having greater proportions of 18:0, diatom marker 20:5n-3, dinoflagellate markers 22:5n-3, and 22:6n-3, and greater proportion during ice-covered months of diatom marker 16:1n-7, benthic macroalgae marker 20:4n-6, dinoflagellate marker 18:1n-9, bacteria marker 18:1n-7 and Calanus marker 20:1n-9 (Figure 3B).
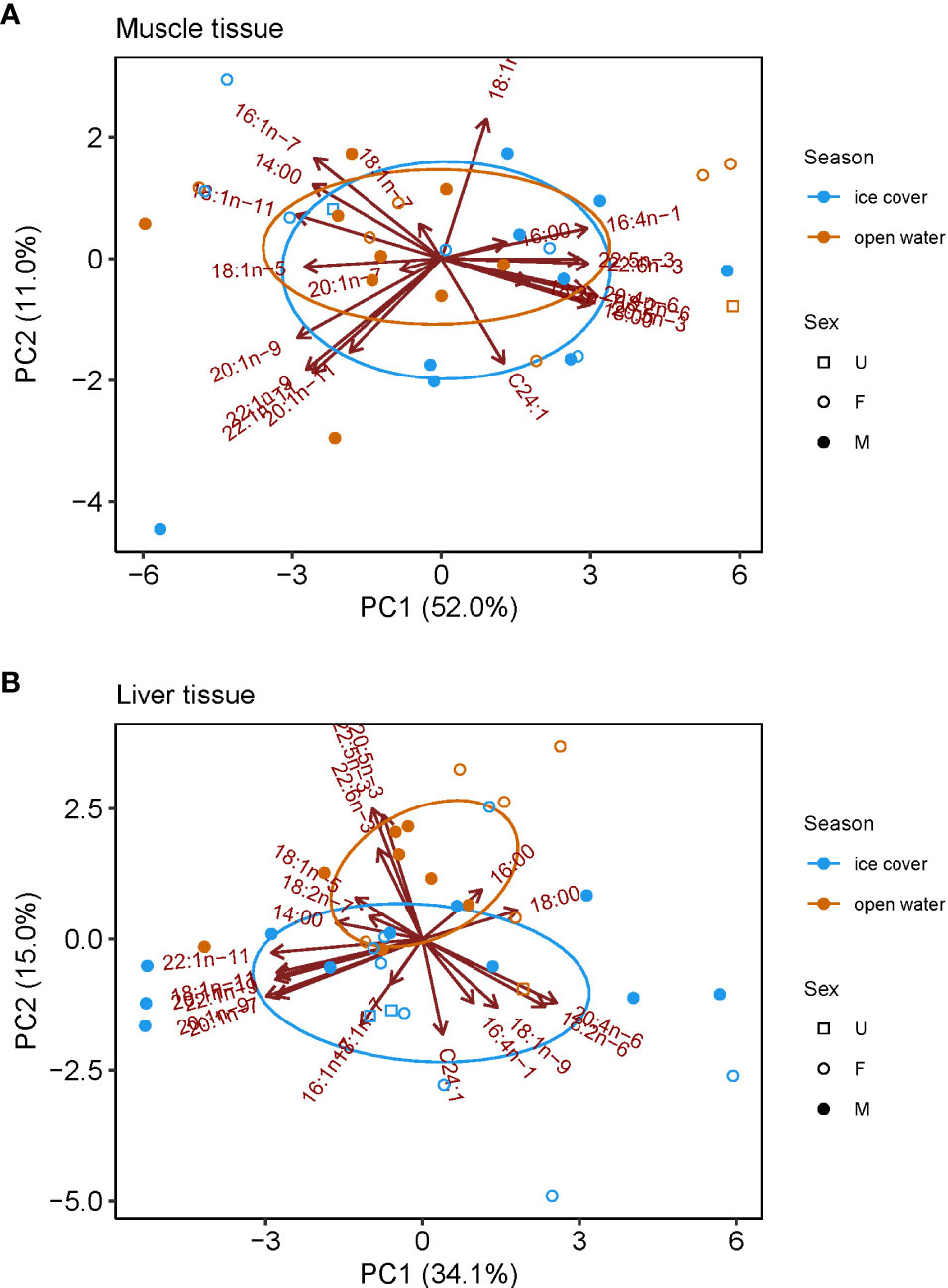
Figure 3 Principle component analyses (PCA) for fatty acid composition in ringed seal tissues collected in Mittimatalik, Nunavut. (A) Muscle tissue only. (B) Liver tissue only. Ellipses represent core fatty acid niches of 40%.
Fatty acid-specific stable isotopes
A two-way analysis of variance for δ13C of the diatom-associated 16:1n-7 revealed that both species and tissues had an effect on isotope values: harp seals had 0.47 (-0.03-0.98) ‰ higher values than ringed seal (F1,47 = 3.57, p=0.065), and muscle had 0.69 (0.32-1.06) ‰ higher values than liver tissue (F1,47 = 13.92, p=0.0005) (Figure 4A). For δ13C of the diatom-associated 20:5n-3, harp seals had 0.81 (0.39-1.22) ‰ higher values than ringed seal (F1,47 = 15.56, p=0.0003), and muscle had 0.49 (0.18-0.79) ‰ higher values than liver tissue (F1,47 = 10.57, p=0.002) (Figure 4B). For δ13C of the dinoflagellate-associated 22:6n-3, no statistical differences were found between tissues (F1,44 = 0.09, p=0.77), though harp seal had 0.88 (0.42-1.35) ‰ higher values than ringed seal (F1,47 = 15.56, p=0.0003) (Figure 4C). For δ13C of the benthic macroalgae-associated 20:4n-6, species did not differ significantly (F1,43 = 1.13, p=0.29), and tissue differences were marginally significant (F1,44 = 3.22, p=0.08) (Figure 4D). No significant correlations were found between any isotope values of FA markers and age in ringed and harp seals (p>0.10 in all cases).
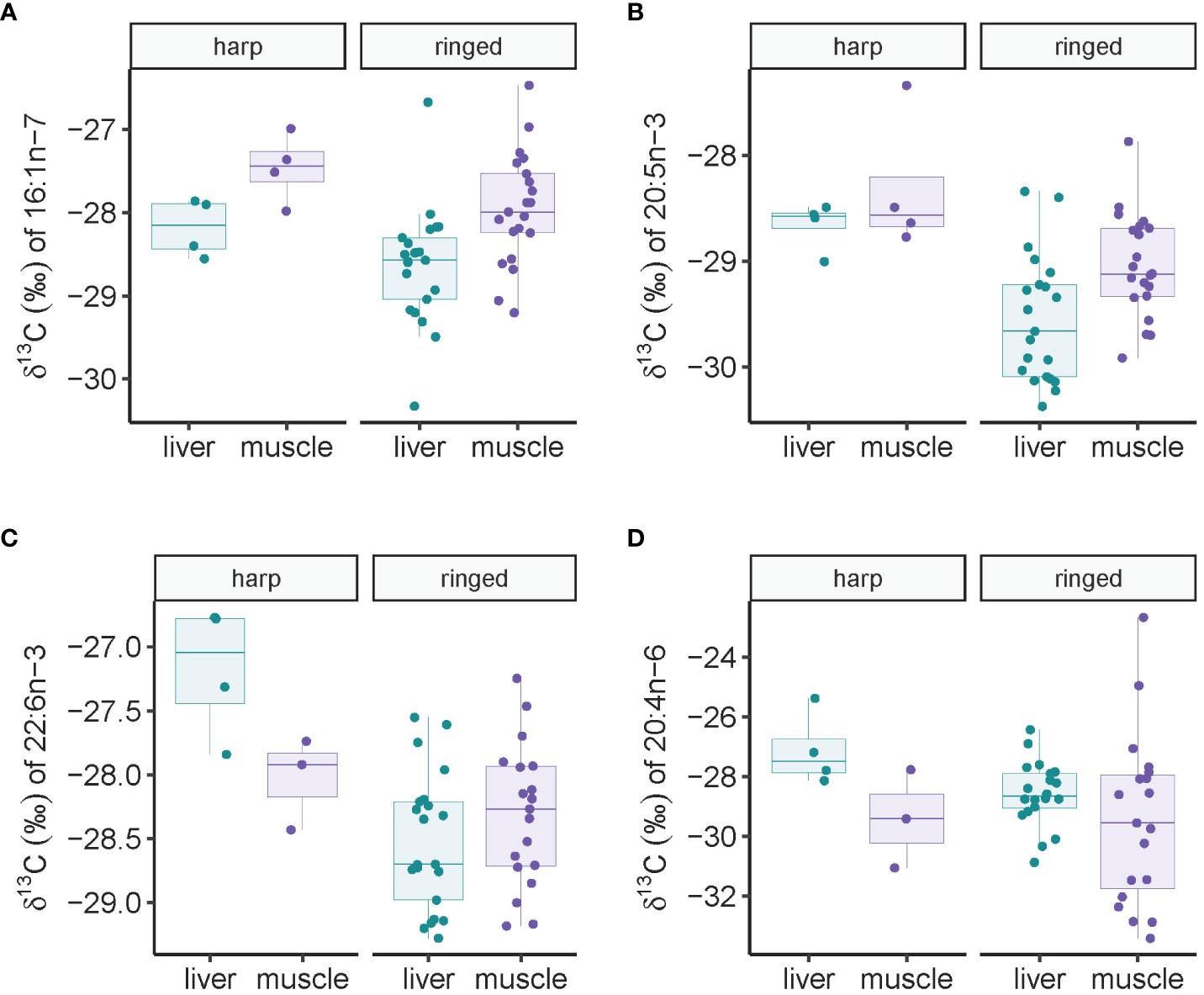
Figure 4 Fatty acid-specific stable carbon isotope values (A–D) in ringed and harp seal liver and muscle tissue collected in Mittimatalik, Nunavut.
Much like bulk stable isotopes of carbon, FA-specific isotopes of carbon varied throughout the year and trends were more prominent in liver compared to muscle for ringed seals (Figures 5A–D). The exception was for δ13C of 20:4n-6 which did not show any sub-annual temporal pattern (Figure 5D). For all other FA-specific isotopes, values peaked between month 5 and 7, and reached lowest values near the end of the year in months 9 to 11 (Figures 5A–C). Comparing ice cover and open-water seasons, differences were greater again in liver tissue compared to muscle for all FA-specific carbon isotopes, though statistically significant differences existed only for δ13C of 20:5n-3 in liver (t19.0 = 4.34, p=0.0004), and marginally so for δ13C of 22:6n-3 in liver (t19.0 = 1.70, p=0.10) and δ13C of 16:1n-7 in muscle (t16.2 = 1.87, p=0.08). All markers had greater values in ice-covered months compared to open water (Figure 5E).
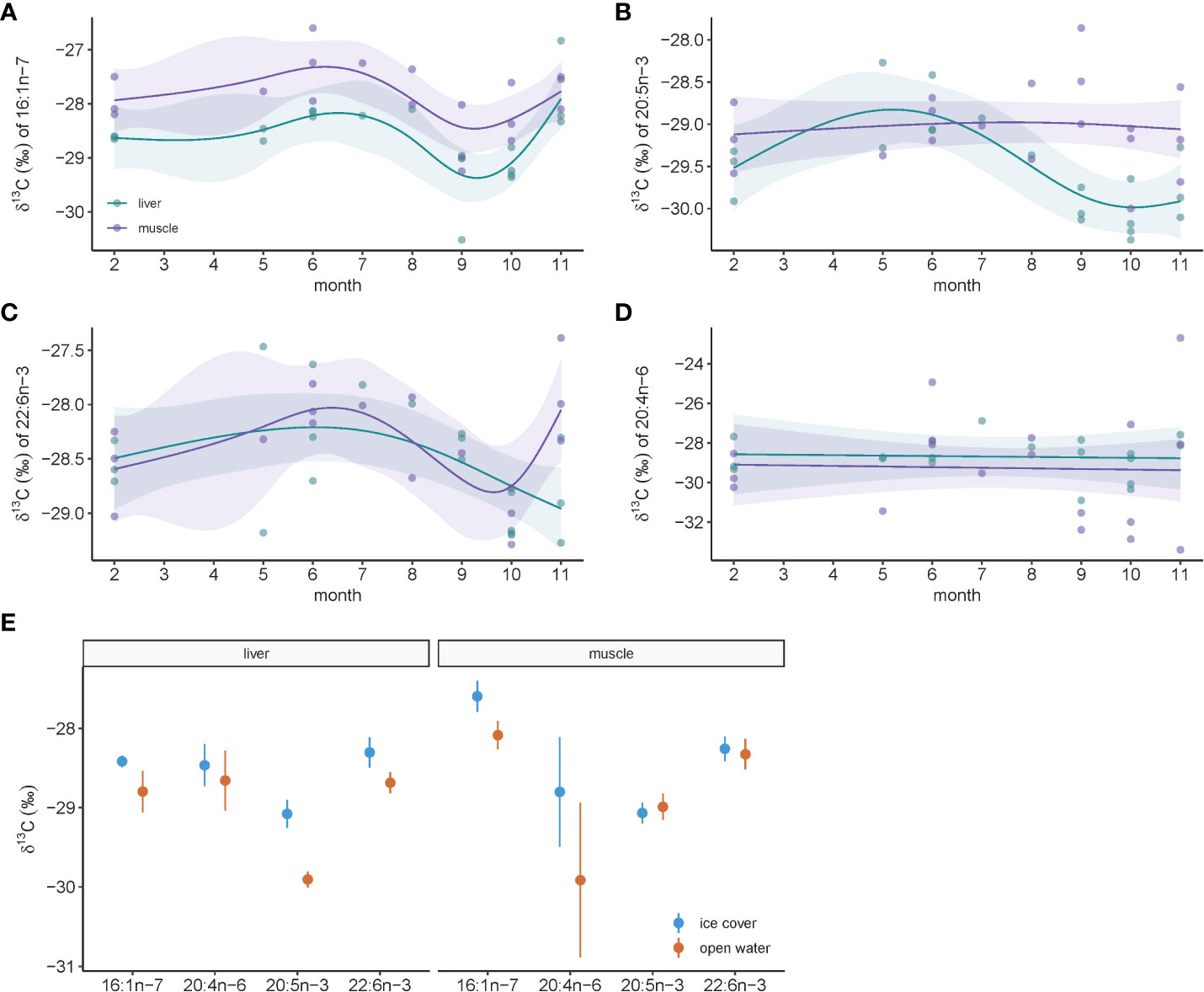
Figure 5 Temporal dynamics of fatty acid-specific stable carbon isotopes in ringed seal muscle and liver tissue collected in Mittimatalik, Nunavut. (A–D) Sub-annual trends for each fatty acid. Dots represent individual samples and lines show the predicted curves for each tissue and the corresponding 95% confidence intervals from the GAM, adjusted for animal standard length. (E) Seasonal pattern for each fatty acid where color code represents seasons of sample collection. Dots represent mean and lines the standard error.
Highly branched isoprenoids
All three HBIs were detected in all samples of harp and ringed seal liver tissues. Ringed seals had higher proportions of the sea ice-derived HBIs monoene (IP25; W=36, p=0.0001) and diene (W=37, p=0.0001), whereas the pelagic HBI triene was higher in harp seals (W=318, p<0.0001) and was the dominant marker in that species (Figure 6A). Sea ice-derived carbon, estimated using H-print values, was 1.6 times higher in ringed seals than harp seals (W=26, p<0.0001) (Figure 6B). Sea ice-derived carbon estimates were not correlated with age in either seal species (p>0.10 in both species). The sub-annual temporal pattern for sea ice-derived carbon percentage in ringed seal liver tissue showed lowest levels in September, with little variation in other months of the year (Figure 6C). Comparing ice cover and open-water seasons in ringed seal liver, the contribution of sea ice-derived carbon was almost 20% greater in ice-covered months (t26.2 = 2.12, p=0.044) (Figure 6D).
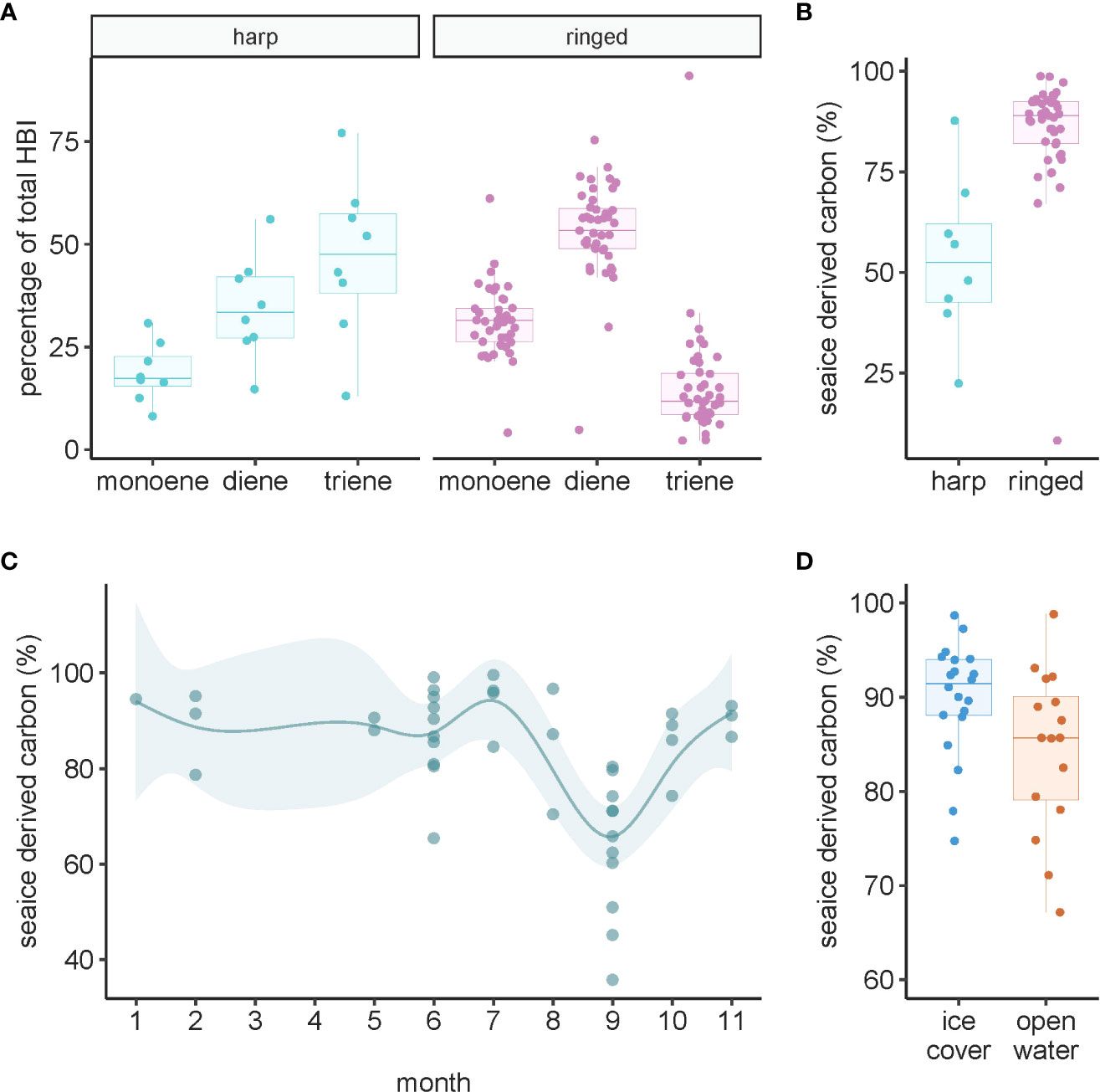
Figure 6 Highly branched isoprenoids (HBIs) in ringed and harp seal liver tissue collected in Mittimatalik, Nunavut. (A) HBI composition. Composition is calculated as the percentage total of all monoene, diene, and triene compounds. Monoene and diene are sea-ice proxies and triene is a pelagic proxy. (B) The percentage of sea-ice derived carbon (see Materials and Methods for calculation). (C) Sub-annual temporal trend of sea ice-derived carbon estimates. Dots represent individual samples, and the line shows the predicted curve and corresponding 95% confidence intervals from the GAM, adjusted for animal standard length. (D) Seasonal pattern of estimated sea ice-derived carbon where color code represents seasons of sample collection.
Discussion
Characterization of the foraging ecology and habitat use of top predators is essential to our understanding of the functioning of Arctic marine ecosystems, particularly given the long-term environmental changes in the physical components and biological communities across the Arctic (Thiemann et al., 2008; Post et al., 2013; Post et al., 2019). Potential impacts of global change may be exacerbated by competition between seasonally sympatric species that depend upon similar resources as described by the competitive exclusion principle (Hardin, 1960). Using a comprehensive combination of dietary tracers we found relatively little dietary resource competition between seasonally sympatric and sea ice dependent ringed seals and harp seals in Mittimatalik, Nunavut, an area with little previous information on the diet of these species.
Species differences
Ample studies have demonstrated that biogeochemical tracers, such as stable isotopes, FAs, and HBIs, in predators are largely determined by their diet, and therefore that observed variability in tracer profiles can be indicative of differences in foraging ecology and habitat use (Hobson et al., 1995; Iverson et al., 2002; Iverson et al., 2004; Rubenstein and Hobson, 2004; Budge et al., 2008a; Budge et al., 2008b; Newsome et al., 2010; Ramos and González-Solís, 2012; Brown et al., 2014a; Brown et al., 2018). Using a combination of dietary tracers, we found a multitude of differences in the dietary profiles of sympatric Arctic ringed seals and harp seals sampled in Mittimatalik, Nunavut. The most distinctive difference between the two species was observed in their utilization of carbon sources based on the distribution of HBI diatom lipids in liver tissue, where ringed seals were found to rely to a much greater extent on sea ice-derived carbon than harp seals (~80 vs. 50%). Fatty acid signatures generally supported HBI findings with greater relative proportion of benthic and/or coastal prey markers in ringed seals that would likely be higher in sea-ice derived carbon (see details below for seasonal changes; Fortier et al., 2002; Renaud et al., 2007), and greater relative importance of pelagic prey markers (Calanus spp. food web) in harp seals (Dalsgaard et al., 2003; Brown et al., 2015). Fatty acid profiles, HBIs, and stable isotope signatures in benthic organisms in Mittimatalik show a strong connectivity to sea-ice derived carbon (Kohlbach et al., 2019). The relatively higher bulk δ15N values in ringed seals are also consistent with some benthic feeding (Iken et al., 2005) or higher trophic position.
At first glance, however, bulk and FA-specific carbon stable isotope profiles were inconsistent with other tracers, suggesting presumably greater pelagic foraging in ringed seals compared to harp seal. In a similar study in southern Baffin Bay using bulk stable isotopes, the authors also reported more pelagic feeding in ringed seals compared to harp seals and suggest that harp seals may acquire a more benthic isotope signal from feeding on larger fish at deeper water depths (Ogloff et al., 2019). This hypothesis is not entirely consistent with our findings based on δ15N, FAs, and HBIs. A possible explanation for this discrepancy is the seasonal migratory behaviour of harp seals (Stenson et al., 2020) compared to the more resident behaviour of ringed seals who inhabit Arctic waters throughout the year (Laidre et al., 2008). Harp seals of the Northwest Atlantic overwinter in the Gulf of St. Lawrence or off northern Newfoundland and migrate northward between May and August depending on environmental conditions (Stenson and Sjare, 1997). North Atlantic isoscapes demonstrate a strong increasing latitudinal gradient in both carbon and nitrogen isotope trends (Graham et al., 2010), thus harp seals foraging in the southern extent of their range would acquire more 13C-depleted resources, which could be at least partially retained until September when the animals were sampled given the likely turnover rate of isotopes in muscle (~ 4-6 months) and liver (~1-2 months) (Vander Zanden et al., 2015). Turnover of FAs and HBIs are also likely in the order of weeks to months in liver of marine mammals (Budge et al., 2006; Brown et al., 2014a), suggesting that these tracers in harp seals may also have been impacted by regional migration to some degree depending on when seals arrived in northwestern Baffin Bay. We therefore posit that the isotope signatures and potentially other dietary tracers reflect a temporal and migratory difference between species rather than local foraging differences between sympatric species in the Mittimatalik area. These results highlight the benefit of combining multiple lines of evidence from complementary dietary tracers to unravel questions of species foraging ecology.
Other than the carbon isotope inconsistencies described above, our results are consistent with previous studies of distribution, habitat use, and diet of ringed seals and harp seals, in that harp seals have a higher contribution of pelagic prey than ringed seals. While both seals are ice-associated species, ringed seals are highly reliant on sea ice throughout the year, generally considered to not undertake long-distance movements as adults, and prefer relatively shallow coastal areas (Reeves, 1998; Laidre et al., 2008). Ringed seals tend to forage under the ice and dietary analyses have shown a diverse diet of fish and invertebrates, though polar cod (Arctogadus glacialis) is commonly consumed as well as Arctic cod (Boreogadus saida), capelin (Mallotus villosus), smelt (Osmerus sp.) and herring (Clupea sp.) as well as many invertebrates during the open-water season (Reeves, 1998; Thiemann et al., 2007; Laidre et al., 2008; Young and Ferguson, 2013; Yurkowski et al., 2016; Ogloff et al., 2019). Harp seals are a pelagic, migratory species that rely on drifting pack-ice for parts of the year and spend a considerable amount of time in ice-free Arctic waters (Laidre et al., 2008; Stenson et al., 2020). Harp seals are opportunistic feeders known to feed on over 70 species of fish and invertebrates, which varies by age, season, and region (Lawson and Stenson, 1997; Tucker et al., 2009; Ogloff et al., 2019). The most important species taken in the subarctic is capelin, while polar cod is the most important in the Arctic as well as pelagic euphausiids (Thysanoessa spp.) and amphipods (Parathemisto spp.) (Tucker et al., 2009; Stenson et al., 2020). Given the older age of harp seals than ringed seals in our dataset, it is possible that part of the dietary signal differences between species relates to ontogenic dietary changes, where older animals are known to forage more heavily on larger fish (Ogloff et al., 2019). Taken together with our results, it is probable that ringed seals and harp seals rely on similar prey species in Mittimatalik, but that the prey themselves cover a diversity of habitat types across seasons, causing diverging dietary tracer signals in the seals that forage in these different habitats.
Previous studies have estimated the contribution of sympagic carbon sources in various Arctic marine predators, including several ice-associated seal species (Budge et al., 2008b; Brown et al., 2014a; Wang et al., 2016; Kunisch et al., 2021), bowhead whales (Budge et al., 2008b), beluga (Brown et al., 2017), and polar bears (Brown et al., 2018) (Table 3). While the tracer methods (HBIs vs. FA-specific stable isotopes), region, seasons, and years vary between studies, all studies estimate a relatively high contribution of sympagic carbon to Arctic marine top predators (up to 100%), though with high individual variability within populations (Table 4). Our estimates for ringed seals are on the upper end of those found in other ringed seal populations in the Arctic, likely in part due to the more northern location of our sampling as well as high contribution of samples from winter months in our study, expected to have higher sea ice-derived carbon compared to other locations that relied on summer samples (Brown et al., 2014a). Differences in regional diet, ages, and sexes between ringed seal populations across the Arctic may also have contributed to observed differences in sympagic carbon contributions since these factors are known to modulate foraging ecology (Thiemann et al., 2007; Laidre et al., 2008), though the main prey items are likely to be similar. Ringed seals in the Chukchi Sea were reported to have the lowest sympagic carbon signal of all populations and authors hypothesized that these animals may have been foraging in a different isoscape (Wang et al., 2016), similar to what we propose for harp seals in our study. Bowhead whales have the lowest reliance on sympagic carbon of all Arctic marine mammals and polar bears and ringed seals the highest (Table 3). Overall, these results highlight and confirm previous dietary and life history knowledge for Arctic marine mammals and how they relate to source apportionment of sympagic and pelagic carbon in marine food webs.
Seasonal differences in ringed seals
We observed a clear and consistent seasonal pattern in all dietary tracers used in this study that indicated a shift in ringed seal foraging on sympagic and benthic prey during ice-covered months of the year to greater reliance on pelagic prey during the summer open-water season. Alternatively, prey items may be similar across seasons, and seasonally fluctuate in their source of carbon. As in previous studies, we note that the seasonal signatures are far more apparent in liver than muscle, reflecting the more rapid metabolic rate and thus turnover of dietary markers in liver enabling to investigate more recent diet compositions (Newsome et al., 2010; Young and Ferguson, 2013; Ogloff et al., 2019). Our pelagic markers suggested relative decreases in bulk and FA-specific δ13C, decreased trophic level, increased relative importance of pelagic dinoflagellate FA markers, and decreased sympagic carbon percentage from winter to summer. As a good indicator of habitat use and carbon source, δ13C can be used to distinguish benthic, coastal, and/or or sea-ice (enriched in 13C) or pelagic (depleted in 13C) carbon sources f (Graham et al., 2010; Newsome et al., 2010; Hoh et al., 2012; Kohlbach et al., 2019). The observed shift from high δ13C values during winter to lower values during summer therefore indicates a change in ringed seal diet from a sea ice and benthic food web to a more pelagic food web as the season progressed. The higher δ15N values in winter compared to summer also support an increased benthic habitat use and diet of ringed seals during ice-covered months as benthic food webs tend to be enriched in 15N relative to pelagic ones (Iken et al., 2005). The higher δ15N values could also indicate a greater contribution of higher trophic prey, i.e. greater intake of fish and less invertebrates (Ogloff et al., 2019). We also found that the overall isotopic niche size was larger in ice-covered vs. ice-free months and that there was little niche overlap between seasons, further supporting the notion of seasonal foraging differences with broader diversity of prey consumed in winter. Although we cannot determine the exact diet of ringed seals throughout the year, the enrichment in dinoflagellate FA markers during summer ice-free months suggests foraging within the pelagic water column (Dalsgaard et al., 2003; Kohlbach et al., 2016). Benthic food webs typically contain diverse primary producer communities and greater proportion of benthic/coastal FA markers, including those for macroalgae, vascular plants, and bacteria (Pedro et al., 2020), in ringed seals during ice-covered months suggested increased foraging along the benthos in winter. Finally, HBI markers are increasingly being used to help decipher the relative contribution of sympagic carbon sources in Arctic marine food webs based on source-specificity of HBIs in sympagic and pelagic primary producers (Brown et al., 2014a; Brown et al., 2014b; Belt, 2018). The observed increase in sympagic HBI markers during ice-covered months in ringed seals indicated greater foraging in benthic food webs under ice (Brown et al., 2015), similar to what has been shown in ringed seals from Cumberland Sound (Brown et al., 2014a). It is important to note however, that even during summer we found a relatively high sympagic carbon signal using HBIs, suggesting that ice algae represent an important component of carbon and energy transfer in ringed seals all year round in the Northwest Baffin Bay region, as has been shown in Southwest Baffin Bay (Brown et al., 2014a). The important contribution of sea ice carbon to ringed seals likely occurs through benthic foraging since ice algae are efficiently exported to the benthos and provide an important food supply (Fortier et al., 2002; Renaud et al., 2007).
Seasonal shifts in the dietary signal of ringed seals corresponding to changes in presence of sea ice in winter and summer are commonly reported across the Arctic (Thiemann et al., 2007; Young and Ferguson, 2013; Brown et al., 2014a; Ogloff et al., 2019). While relying on small sample sizes, our analysis of multiple dietary tracers on a monthly time-scale indicated a distinct dietary transition from benthic/sympagic to pelagic feeding in September. The dietary transition found here for the Northwest Baffin Bay region occurred later than similar transitions reported for ringed seals in southwest Baffin Bay (April-May) (Brown et al., 2014a) and Hudson Bay (June) (Young and Ferguson, 2013). The later pelagic dietary transition in our study could be associated with the more northern location of Eclipse Sound (~72 degrees) compared to Cumberland Sound (~62 degrees). The bloom of ice algae in Eclipse Sound (Kohlbach et al., 2019) and in the Canadian High Arctic (e.g. Resolute bay, 75°N; (Pogson et al., 2011; Galindo et al., 2014) typically occurs during the month of May/June although predictability of ice algal phenology is increasingly difficult to predict as the Arctic continues to warm rapidly. In their pan-Arctic study, Tedesco et al. modeled sea-ice algae blooms across latitudinal gradients in the Arctic and report that sympagic primary production typically begins in May and June for latitudes representative of southern and northern Baffin Bay, respectively (Tedesco et al., 2019). These estimates represent historical averages (1961-2005) and are not region-specific, thus may not align with the years and locations of ringed seal samples, but nonetheless support a later sympagic algae bloom around our study area which may explain the later pelagic dietary transition relative to more southern populations. The importance of sea-ice dynamics for sympagic carbon flows to top predators has also been demonstrated in populations of ringed seals and beluga sampled over many years in Cumberland Sound (Brown et al., 2014a; Brown et al., 2017). These studies have shown that sympagic carbon signals are sensitive to reductions of annual sea-ice extent, suggesting climate warming is likely to cause increased pelagic foraging in Arctic marine mammals.
Conclusion
In summary, while ringed seals and harp seals overlap spatially in the Canadian high-Arctic during the open-water season, we found little evidence for dietary resource competition between the two species in Mittimatalik, Nunavut. It is likely that these seal species share many common prey items, but that prey cover diverse habitats and thus transfer different dietary tracer signals to ringed and harp seals that forage in coastal or pelagic regions, respectively. Using a multi-dietary tracer approach, we show that bulk stable isotopes were not as good at differentiating carbon sources and dietary pathways between the two seal species compared to higher resolution and more specific markers such as FAs, FA-specific carbon isotopes, and HBIs. We showed that both seals were supported by sympagic carbon sources, though the dependence was far greater for the resident ringed seal. Dietary tracer profiles may have been biased in harp seals as they could have retained part of their pre-migration signal, thus not truly reflecting a direct comparison of diet with seasonally sympagic ringed seals that do not migrate from more southern regions. Further studies are needed to investigate the impact of dietary tracer turnover rates in tissue of seasonally migratory top predators. Sea-ice extent and thickness will continue to decline across the Arctic and result in broad scale ecosystem changes that will impact endemic species like ringed seals and harp seals. It appears that these species may rely on slightly different carbon and trophic pathways for now, but with increasing open-water season length and northward expansion of temperate prey fish it remains to be seen how ringed seals and harp seals will respond and potentially intensify dietary resource competition. The high dependence on sympagic carbon throughout the year for ringed seals puts them at significant risk of further ecosystem change.
Data availability statement
The data presented in the study are deposited in the Mendeley Data repository, accession number 10.17632/w2pbnbtmyp.1.
Ethics statement
Ethical review and approval was not required for the animal study because Samples were collected as part of Inuit subsistence harvests and then provided by Inuit beneficiaries to DFO as part of a community-based monitoring program.
Author contributions
All authors contributed to the study planning, design, and interpretation of results. J-PD led the data analysis and writing. DY, CC and SF coordinated sample collection. SF, DK, CC, LL, CM, and BR coordinated and/or performed laboratory analyses. All authors revised and reviewed the manuscript.
Funding
This project was funded by Fisheries and Oceans Canada (DFO) Strategic Program for Ecosystem-Based Research and Advice (SPERA) to CM and SF and DFO’s Marine Productivity Laboratory funds to CM Funding was also contributed by Nunavut Wildlife Management Board and Natural Sciences and Engineering Research Council of Canada (NSERC) Discovery Grant to SF #1068936. DK received a Visiting Fellowship in Canadian Laboratory from the NSERC, supported by SPERA. DK is currently funded by the Research Council of Norway through the project The Nansen Legacy (RCN #276730).
Acknowledgments
The authors gratefully acknowledge the Mittimatalik Hunters and Trappers Organization in Pond Inlet and Inuitits hunters for the collection of seal samples. We thank the Biotracers Laboratory (Fisheries and Oceans Canada, Freshwater Institute) under the direction of Lisa Loseto and Bruno Rosenberg as well as Anke Reppchen (both Fisheries and Oceans Canada) for their help with laboratory analyses.
Conflict of interest
The authors declare that the research was conducted in the absence of any commercial or financial relationships that could be construed as a potential conflict of interest.
Publisher’s note
All claims expressed in this article are solely those of the authors and do not necessarily represent those of their affiliated organizations, or those of the publisher, the editors and the reviewers. Any product that may be evaluated in this article, or claim that may be made by its manufacturer, is not guaranteed or endorsed by the publisher.
References
Belt S. T. (2018). Source-specific biomarkers as proxies for Arctic and Antarctic sea ice. Org. Geochem. 125, 277–298. doi: 10.1016/j.orggeochem.2018.10.002
Bligh E. G., Dyer W. J. (1959). A rapid method of total lipid extraction and purification. Canadian J. Biochem. Physiol. 37, 911–917. doi: 10.1139/o59-099
Brown T. A., Alexander C., Yurkowski D. J., Ferguson S. H., Belt S. T. (2014a). Identifying variable sea ice carbon contributions to the Arctic ecosystem: A case study using highly branched isoprenoid lipid biomarkers in Cumberland sound ringed seals. Limnol. Oceanogr. 59, 1581–1589. doi: 10.4319/lo.2014.59.5.1581
Brown T. A., Belt S. T., Tatarek A., Mundy C. J. (2014b). Source identification of the Arctic sea ice proxy IP 25. Nat. Commun. 5, 1–7. doi: 10.1038/ncomms5197
Brown T. A., Chrystal E., Ferguson S. H., Yurkowski D. J., Watt C., Hussey N. E., et al. (2017). Coupled changes between the h-print biomarker and δ15N indicates a variable sea ice carbon contribution to the diet of Cumberland sound beluga whales. Limnol. Oceanogr. 62, 1606–1619. doi: 10.1002/lno.10520
Brown T. A., Galicia M. P., Thiemann G. W., Belt S. T., Yurkowski D. J., Dyck M. G. (2018). High contributions of sea ice derived carbon in polar bear (Ursus maritimus) tissue. PLoS One 13, 1–13. doi: 10.1371/journal.pone.0191631
Brown T. A., Hegseth E. N., Belt S. T. (2015). A biomarker-based investigation of the mid-winter ecosystem in rijpfjorden, Svalbard. Polar Biol. 38, 37–50. doi: 10.1007/s00300-013-1352-2
Budge S. M., Iverson S. J., Koopman H. N. (2006). Studying trophic ecology in marine ecosystems using fatty acids: A primer on analysis and interpretation. Mar. Mammal Sci. 22, 759–801. doi: 10.1111/j.1748-7692.2006.00079.x
Budge S. M., Springer a. M., Iverson S. J., Sheffield G., Rosa C. (2008a). Blubber fatty acid composition of bowhead whales, balaena mysticetus: Implications for diet assessment and ecosystem monitoring. J. Exp. Mar. Bio. Ecol. 359, 40–46. doi: 10.1016/j.jembe.2008.02.014
Budge S. M., Wooller M. J., Springer A. M., Iverson S. J., McRoy C. P., Divoky G. J. (2008b). Tracing carbon flow in an arctic marine food web using fatty acid-stable isotope analysis. Oecologia 157, 117–129. doi: 10.1007/s00442-008-1053-7
Dalsgaard J., St. John M., Kattner G., Müller-Navarra D., Hagen W. (2003). Fatty acid trophic markers in the pelagic marine environment. Adv. Mar. Biol. 46, 225–340. doi: 10.1016/S0065-2881(03)46005-7
Fortier M., Fortier L., Michel C., Legendre L. (2002). Climatic and biological forcing of the vertical flux of biogenic particles under seasonal Arctic sea ice. Mar. Ecol. Prog. Ser. 225, 1–16. doi: 10.3354/meps225001
Fossheim M., Primicerio R., Johannesen E., Ingvaldsen R. B., Aschan M. M., Dolgov A. V. (2015). Recent warming leads to a rapid borealization of fish communities in the Arctic. Nat. Clim. Change 5, 673–677. doi: 10.1038/nclimate2647
Galindo V., Levasseur M., Mundy C. J., Gosselin M., Tremblay J.-É., Scarratt M., et al. (2014). Biological and physical processes influencing sea ice, under-ice algae, and dimethylsulfoniopropionate during spring in the Canadian Arctic archipelago. J. Geophys. Res. Ocean. 119, 3746–3766. doi: 10.1002/2013JC009497
Graham B., Koch P., Newsome S., McMahon K., Aurioles D. (2010). Isoscapes. Eds. West J. B., Bowen G. J., Dawson T. E., Tu Dordrecht K. P. (Netherlands: Springer). doi: 10.1007/978-90-481-3354-3
Hardin G. (1960). The competitive exclusion principle. Science 131, 1292–1297. doi: 10.1126/science.131.3409.1292
Hobson K. A., Ambrose W. G., Renaud P. E. (1995). Sources of primary production, benthic-pelagic coupling, and trophic relationships within the northeast water polynya: Insights from δ13C and δ15N analysis. Mar. Ecol. Prog. Ser. 128, 1–10. doi: 10.3354/meps128001
Hoh E., Dodder N. G., Lehotay S. J., Pangallo K. C., Reddy C. M., Maruya K. A. (2012). Nontargeted comprehensive two-dimensional gas Chromatography/Time-of-Flight mass spectrometry method and software for inventorying persistent and bioaccumulative contaminants in marine environments. Environ. Sci. Technol. 46, 8001–8008. doi: 10.1021/es301139q
Horswill C., Jackson J. A., Medeiros R., Nowell R. W., Trathan P. N., O’Connell T. C. (2018). Minimising the limitations of using dietary analysis to assess foodweb changes by combining multiple techniques. Ecol. Indic. 94, 218–225. doi: 10.1016/j.ecolind.2018.06.035
Iken K., Bluhm B., Gradinger R. (2005). Food web structure in the high Arctic Canada basin: evidence from d13C and d15N analysis. Polar Biol. 28, 238–249. doi: 10.1007/s00300-004-0669-2
IPCC (2021). “Climate change,” in The physical science basis. contribution of working group I to the sixth assessment report of the intergovernmental panel on climate change. Eds. Masson-Delmotte V., Zhai P., Pirani A., Connors S. L., Péan C., Berger S., Caud N., Chen Y. (United Kingdom New York, NY, USA: Cambridge Univ. Press. Cambridge).
Iverson S. J., Field C., Don Bowen W., Blanchard W. (2004). Quantitative fatty acid signature analysis: a new method of estimating predator diets. Ecol. Monogr. 74, 211–235. doi: 10.1890/02-4105
Iverson S., Frost K., Lang S. (2002). Fat content and fatty acid composition of forage fish and invertebrates in prince William sound, Alaska: Factors contributing to among and within species variability. Mar. Ecol. Prog. Ser. 241, 161–181. doi: 10.3354/meps241161
Jackson A. L., Inger R., Parnell A. C., Bearhop S. (2011). Comparing isotopic niche widths among and within communities: SIBER - stable isotope Bayesian ellipses in r. J. Anim. Ecol. 80, 595–602. doi: 10.1111/j.1365-2656.2011.01806.x
Kelly J., Scheibling R. (2012). Fatty acids as dietary tracers in benthic food webs. Mar. Ecol. Prog. Ser. 446, 1–22. doi: 10.3354/meps09559
Kohlbach D., Ferguson S., Brown T., Michel C. (2019). Landfast sea ice-benthic coupling during spring and potential impacts of system changes on food web dynamics in eclipse sound, Canadian Arctic. Mar. Ecol. Prog. Ser. 627, 33–48. doi: 10.3354/meps13071
Kohlbach D., Graeve M., A. Lange B., David C., Peeken I., Flores H. (2016). The importance of ice algae-produced carbon in the central Arctic ocean ecosystem: Food web relationships revealed by lipid and stable isotope analyses. Limnol. Oceanogr. 61, 2027–2044. doi: 10.1002/lno.10351
Kortsch S., Primicerio R., Fossheim M., Dolgov A. V., Aschan M. (2015). Climate change alters the structure of arctic marine food webs due to poleward shifts of boreal generalists. Proc. R. Soc B Biol. Sci. 282, 20151546. doi: 10.1098/rspb.2015.1546
Kunisch E. H., Graeve M., Gradinger R., Haug T., Kovacs K. M., Lydersen C., et al. (2021). Ice-algal carbon supports harp and ringed seal diets in the European Arctic: Evidence from fatty acid and stable isotope markers. Mar. Ecol. Prog. Ser. 675, 181–197. doi: 10.3354/meps13834
Laidre K. L., Stirling I., Lowry L. F., Wiig O., Heide-Jørgensen M. P., Ferguson S. H. (2008). Quantifying the sensitivity of Arctic marine mammals to climate-induced habitat change. Ecol. Appl. 18, S97–125. doi: 10.1890/06-0546.1
Lannuzel D., Tedesco L., van Leeuwe M., Campbell K., Flores H., Delille B., et al. (2020). The future of Arctic sea-ice biogeochemistry and ice-associated ecosystems. Nat. Clim. Change 10, 983–992. doi: 10.1038/s41558-020-00940-4
Lawson J. W., Stenson G. B. (1997). Diet of northwest Atlantic harp seals (Phoca groenlandica) in offshore areas. Can. J. Zool. 75, 2095–2106. doi: 10.1139/z97-844
Leu E., Brown T. A., Graeve M., Wiktor J., Hoppe C. J. M., Chierici M., et al. (2020). Spatial and temporal variability of ice algal trophic markers–with recommendations about their application. J. Mar. Sci. Eng. 8, 676. doi: 10.3390/jmse8090676
Newsome S. D., Clementz M. T., Koch P. L. (2010). Using stable isotope biogeochemistry to study marine mammal ecology. Mar. Mammal Sci. 26, 509–572. doi: 10.1111/j.1748-7692.2009.00354.x
Ogloff W. R., Yurkowski D. J., Davoren G. K., Ferguson S. H. (2019). Diet and isotopic niche overlap elucidate competition potential between seasonally sympatric phocids in the Canadian Arctic. Mar. Biol. 166, 103. doi: 10.1007/s00227-019-3549-6
Oksanen J., Blanchet G. F., Friendly M., Kindt R., Legendre P., McGlinn D., et al. (2020). Vegan: Community ecology package. R Packag. 2, 5–7.
Overland J. E., Wang M. (2013). When will the summer Arctic be nearly sea ice free? Geophys. Res. Lett. 40, 2097–2101. doi: 10.1002/grl.50316
Overland J. E., Wang M., Walsh J. E., Stroeve J. C. (2014). Future Arctic climate changes: Adaptation and mitigation time scales. Earth’s Futur. 2, 68–74. doi: 10.1002/2013EF000162
Pedro S., Fisk A. T., Ferguson S. H., Hussey N. E., Kessel S. T., McKinney M. A. (2020). Broad feeding niches of capelin and sand lance may overlap those of polar cod and other native fish in the eastern Canadian Arctic. Polar Biol. 43, 1707–1724. doi: 10.1007/s00300-020-02738-8
Pizzolato L., Howell S. E. L., Dawson J., Laliberté F., Copland L. (2016). The influence of declining sea ice on shipping activity in the Canadian Arctic. Geophys. Res. Lett. 43, 12,146–12,154. doi: 10.1002/2016GL071489
Pogson L., Tremblay B., Lavoie D., Michel C., Vancoppenolle M. (2011). Development and validation of a one-dimensional snow-ice algae model against observations in resolute passage, Canadian Arctic archipelago. J. Geophys. Res. 116, C04010. doi: 10.1029/2010JC006119
Post E., Alley R. B., Christensen T. R., Macias-Fauria M., Forbes B. C., Gooseff M. N., et al. (2019). The polar regions in a 2°C warmer world. Sci. Adv. 5, 1–12. doi: 10.1126/sciadv.aaw9883
Post E., Bhatt U. S., Bitz C. M., Brodie J. F., Fulton T. L., Hebblewhite M., et al. (2013). Ecological consequences of Sea-ice decline. Science 341, 519–524. doi: 10.1126/science.1235225
Ramos R., González-Solís J. (2012). Trace me if you can: The use of intrinsic biogeochemical markers in marine top predators. Front. Ecol. Environ. 10, 258–266. doi: 10.1890/110140
R Core Team (2022). R: A language and environment for statistical computing. Vienna, Austria: R Foundation for Statistical Computing.
Reeves R. R. (1998). Distribution, abundance and biology of ringed seals (Phoca hispida): an overview. NAMMCO Sci. Publ. 1, 9. doi: 10.7557/3.2979
Renaud P. E., Riedel A., Michel C., Morata N., Gosselin M., Juul-Pedersen T., et al. (2007). Seasonal variation in benthic community oxygen demand: A response to an ice algal bloom in the Beaufort Sea, Canadian Arctic? J. Mar. Syst. 67, 1–12. doi: 10.1016/j.jmarsys.2006.07.006
Rubenstein D. R., Hobson K. A. (2004). From birds to butterflies: Animal movement patterns and stable isotopes. Trends Ecol. Evol. 19, 256–263. doi: 10.1016/j.tree.2004.03.017
Serreze M. C., Holland M. M., Stroeve J. (2007). Perspectives on the arctic’s shrinking sea-ice cover. Science 315, 1533–1536. doi: 10.1126/science.1139426
Serreze M. C., Stroeve J. (2015). Arctic Sea ice trends, variability and implications for seasonal ice forecasting. Philos. Trans. R. Soc A Math. Phys. Eng. Sci. 373, 20140159. doi: 10.1098/rsta.2014.0159
Stenson G. B., Haug T., Hammill M. O. (2020). Harp seals: Monitors of change in differing ecosystems. Front. Mar. Sci. 7. doi: 10.3389/fmars.2020.569258
Stenson G. B., Sjare B. (1997). Seasonal distribution of harp seals, pltoca groenlandica, in the Northwest Atlantic. Int. Counc. Explor. Sea, 1–23.
Stewart R. E. A., Campana S. E., Jones C. M., Stewart B. E. (2006). Bomb radiocarbon dating calibrates beluga ( delphinapterus leucas ) age estimates. Can. J. Zool. 84, 1840–1852. doi: 10.1139/z06-182
Stroeve J., Notz D. (2018). Changing state of Arctic sea ice across all seasons. Environ. Res. Lett. 13, 103001. doi: 10.1088/1748-9326/aade56
Swanson H. K., Lysy M., Power M., Stasko A. D., Johnson J. D., Reist J. (2015). A new probabilistic method for quantifying n-dimensional ecological niches and niche overlap. Ecology 96, 318–324. doi: 10.1890/14-0235.1
Tedesco L., Vichi M., Scoccimarro E. (2019). Sea-Ice algal phenology in a warmer Arctic. Sci. Adv. 5, eaav4830. doi: 10.1126/sciadv.aav4830
Thiemann G. W., Iverson S. J., Stirling I. (2007). Variability in the blubber fatty acid composition of ringed seals (Phoca hispida) across the Canadian Arctic. Mar. Mammal Sci. 23, 241–261. doi: 10.1111/j.1748-7692.2007.00101.x
Thiemann G. W., Iverson S. J., Stirling I. (2008). Variation in blubber fatty acid composition among marine mammals in the Canadian Arctic. Mar. Mammal Sci. 24, 91–111. doi: 10.1111/j.1748-7692.2007.00165.x
Tucker S., Bowen W. D., Iverson S. J., Blanchard W., Stenson G. B. (2009). Sources of variation in diets of harp and hooded seals estimated from quantitative fatty acid signature analysis (QFASA). Mar. Ecol. Prog. Ser. 384, 287–302. doi: 10.3354/meps08000
Twining C. W., Taipale S. J., Ruess L., Bec A., Martin-Creuzburg D., Kainz M. J. (2020). Stable isotopes of fatty acids: Current and future perspectives for advancing trophic ecology. Philos. Trans. R. Soc B Biol. Sci. 375, 20190641. doi: 10.1098/rstb.2019.0641
Vander Zanden M. J., Clayton M. K., Moody E. K., Solomon C. T., Weidel B. C. (2015). Stable isotope turnover and half-life in animal tissues: A literature synthesis. PloS One 10, e0116182. doi: 10.1371/journal.pone.0116182
Wang S. W., Springer A. M., Budge S. M., Horstmann L., Quakenbush L. T., Wooller M. J. (2016). Carbon sources and trophic relationships of ice seals during recent environmental shifts in the Bering sea. Ecol. Appl. 26, 830–845. doi: 10.1890/14.2421/suppinfo
Wassmann P., Duarte C. M., Agustí S., Sejr M. K. (2011). Footprints of climate change in the Arctic marine ecosystem. Glob. Change Biol. 17, 1235–1249. doi: 10.1111/j.1365-2486.2010.02311.x
Wood S. N. (2011). Fast stable restricted maximum likelihood and marginal likelihood estimation of semiparametric generalized linear models. J. R. Stat. Soc Ser. B (Statistical Methodol. 73, 3–36. doi: 10.1111/j.1467-9868.2010.00749.x
Young B. G., Ferguson S. H. (2013). Seasons of the ringed seal: Pelagic open-water hyperphagy, benthic feeding over winter and spring fasting during molt. Wildl. Res. 40, 52–60. doi: 10.1071/WR12168
Yurkowski D. J., Ferguson S. H., Semeniuk C. A. D., Brown T. M., Muir D. C. G., Fisk A. T. (2016). Spatial and temporal variation of an ice-adapted predator’s feeding ecology in a changing Arctic marine ecosystem. Oecologia 180, 631–644. doi: 10.1007/s00442-015-3384-5
Keywords: arctic, feeding ecology, HBI, sympagic carbon, stable isotope, fatty acid, dietary tracer, seals
Citation: Desforges J-P, Kohlbach D, Carlyle CG, Michel C, Loseto LL, Rosenberg B, Yurkowski DJ and Ferguson SH (2022) Multi-dietary tracer approach reveals little overlap in foraging ecology between seasonally sympatric ringed and harp seals in the high Arctic. Front. Mar. Sci. 9:969327. doi: 10.3389/fmars.2022.969327
Received: 14 June 2022; Accepted: 20 September 2022;
Published: 07 October 2022.
Edited by:
Oliver Nicholas Shipley, Beneath the Waves, Inc., United StatesReviewed by:
John Whiteman, Old Dominion University, United StatesBobby Nakamoto, University of New Brunswick Fredericton, Canada
Copyright © 2022 Desforges, Kohlbach, Carlyle, Michel, Loseto, Rosenberg, Yurkowski and Ferguson. This is an open-access article distributed under the terms of the Creative Commons Attribution License (CC BY). The use, distribution or reproduction in other forums is permitted, provided the original author(s) and the copyright owner(s) are credited and that the original publication in this journal is cited, in accordance with accepted academic practice. No use, distribution or reproduction is permitted which does not comply with these terms.
*Correspondence: Jean-Pierre Desforges, j.desforges@uwinnipeg.ca; Steven H. Ferguson, steve.ferguson@dfo-mpo.gc.ca