- 1Norwegian Polar Institute, Fram Centre, Tromsø, Norway
- 2School of Geography, Earth and Environmental Sciences, University of Plymouth, Plymouth, United Kingdom
- 3Alfred Wegener Institute, Helmholtz Centre for Polar and Marine Research, Bremerhaven, Germany
- 4Institute of Oceanology Polish Academy of Sciences, Sopot, Poland
- 5Plymouth Marine Laboratory, Plymouth, United Kingdom
We investigated diets of 24 Barents Sea zooplankton taxa to understand pelagic food-web processes during late summer, including the importance of sea ice algae-produced carbon. This was achieved by combining insights derived from multiple and complementary trophic marker approaches to construct individual aspects of feeding. Specifically, we determined proportions of algal-produced fatty acids (FAs) to reflect the reliance on diatom- versus dinoflagellate-derived carbon, highly branched isoprenoid (HBI) lipids that distinguish between ice-associated and pelagic carbon sources, and sterols to indicate the degree of carnivory. Copepods had the strongest diatom signal based on FAs, while a lack of sea ice algae-associated HBIs (IP25, IPSO25) suggested that they fed on pelagic rather than ice-associated diatoms. The amphipod Themisto libellula and the ctenophores Beroë cucumis and Mertensia ovum had a higher contribution of dinoflagellate-produced FAs. There was a high degree of carnivory in this food web, as indicated by the FA carnivory index 18:1(n−9)/18:1(n−7) (mean value < 1 only in the pteropod Clione limacina), the presence of copepod-associated FAs in most of the taxa, and the absence of algal-produced HBIs in small copepod taxa, such as Oithona similis and Pseudocalanus spp. The coherence between concentrations of HBIs and phytosterols within individuals suggested that phytosterols provide a good additional indication for algal ingestion. Sea ice algae-associated HBIs were detected in six zooplankton species (occurring in krill, amphipods, pteropods, and appendicularians), indicating an overall low to moderate contribution of ice-associated carbon from late-summer sea ice to pelagic consumption. The unexpected occurrence of ice-derived HBIs in pteropods and appendicularians, however, suggests an importance of sedimenting ice-derived material at least for filter feeders within the water column at this time of year.
Introduction
The Barents Sea epitomizes a valuable, high latitude system undergoing rapid climatic warming. This sea comprises a highly productive Arctic shelf sea and nursery ground for commercially important fish species (Hunt et al., 2013; Fuglestad et al., 2020; Kvamsdal et al., 2020). While its southern part is influenced by Atlantic waters supporting rich fish stocks, the northern part is governed by a colder Arctic climate. During winter, most of the Barents Sea north of 75°N is covered with sea ice, while the southern part remains ice-free (Vinje and Kvambekk, 1991). Seasonal and interannual variability in ice coverage is large, however, and depends directly on the inflow of Atlantic water as well as atmospheric and oceanic circulation patterns (Schlichtholz, 2011; Årthun et al., 2012; Lind et al., 2018). Increasing atmospheric temperatures and warming Atlantic waters in recent decades have resulted in a decline in sea-ice coverage and a decrease in sea-ice import, which is particularly pronounced in the northern Barents Sea (Long and Perrie, 2017; Onarheim and Årthun, 2017; Lind et al., 2018).
Such environmental changes have major impacts on primary producers (algae) and their interactions with consumers in the Barents Sea ecosystem (Wassmann et al., 2006; Stige et al., 2019; Dalpadado et al., 2020; Dong et al., 2020). Zooplankton are key components of marine ecosystems, transferring energy from algae to fish and ultimately to top predators (Wassmann et al., 2006; Hop and Gjøsæter, 2013; Planque et al., 2014). As such, they are well adapted to the prevailing environmental conditions and the seasonality of food supply, and therefore zooplankton can be sensitive to changes in their habitat, with cascading effects along the food chain (Blanchard, 2015).
As the Barents Sea has warmed, sea-ice retreat and the longer open water season have caused a sharp increase in estimated net pelagic primary production (e.g., Ellingsen et al., 2008; Arrigo and van Dijken, 2015), which can improve feeding conditions for zooplankton and fish in phytoplankton-based food chains. However, organisms with a strong reliance on sea ice can be negatively impacted (Stige et al., 2019; Dalpadado et al., 2020). Many Arctic species, across all trophic levels, are known to have a strong association with sea ice, for feeding, reproduction and refuge (Hop et al., 2000; Poltermann, 2001; Lone et al., 2019). Shrinkage and confinement of the sea-ice habitat to more northern regions will likely have negative consequences for these species. While algae associated with the sea ice may comprise less than 25% of the annual Barents Sea primary production (Hegseth, 1998; Wassmann et al., 2006), they can nevertheless be an important carbon source for the food web both early in the season (Conover et al., 1986; Søreide et al., 2010) and later during summer (Tamelander et al., 2006; Assmy et al., 2013; Kohlbach et al., 2016, 2017b).
In the Barents Sea, pronounced interannual variability in physical conditions is reflected in large fluctuations of zooplankton composition between seasons and years (Dalpadado et al., 2003; Gluchowska et al., 2017; Stige et al., 2019). Calanus copepods generally dominate the zooplankton biomass in the northern regions (Aarflot et al., 2018). While Calanus glacialis is more abundant in Arctic shelf waters, C. finmarchicus dominates in the Atlantic sector of the Barents Sea, and C. hyperboreus is overall less abundant than the former two species as it is predominantly found in waters deeper than the Barents Sea (Hirche, 1997). Moreover, small copepod taxa, such as Oithona spp., Pseudocalanus spp., Microcalanus spp. as well as krill (Thysanoessa spp.), amphipods (Themisto spp.) and pteropods (Clione limacina and Limacina helicina) can occur in high abundances (Falk-Petersen et al., 1999; Wassmann et al., 2006).
Gelatinous zooplankton have been less studied, though scientific interest in such species and their function in food-web processes has increased worldwide in recent years (Condon et al., 2012; Jaspers et al., 2015; Aubert et al., 2018). Gelatinous zooplankton, such as ctenophores, are not assumed to be a major prey item for fish, although recent studies suggest that increasing water temperatures might favor ctenophores, as they were found more frequently preyed on by cod (Gadus morhua) (Eriksen et al., 2018a). For these reasons, our study compared a wide suite of Arctic zooplankton species, assessing diets of the gelatinous component in a comparative context with the other species.
To study predator-prey relationships of marine organisms, different biochemical methods can be applied. Marine algae produce a number of biomolecules that are transferred along the food chain without significant changes, and can be employed as so-called trophic markers (Ruess and Müller-Navarra, 2019). Marker fatty acids (FAs) can be traced in the consumers across multiple trophic levels (Scott et al., 1999; Haug et al., 2017) and provide information on their carbon and food source preferences (Wang et al., 2015; Kohlbach et al., 2016). Furthermore, distinguishing between pelagic or ice-associated carbon can potentially be achieved by investigating the presence and distribution of certain highly branched isoprenoid (HBI) lipids biosynthesized by specific pelagic and ice-associated diatoms (Brown et al., 2017b, 2018; Schmidt et al., 2018). In contrast, sterols yield less specific dietary information, but can be used to distinguish, to some extent, phytoplankton-produced versus animal-produced carbon (Drazen et al., 2008; Ruess and Müller-Navarra, 2019). Each of these biochemical methods has strengths and weaknesses, but when applied in combination they yield different facets to feeding and are a powerful approach to study food webs (Leu et al., 2020).
Since the Barents Sea has rich fishery resources and is considered a ‘climate change hotspot,’ it has been the focus of a series of large-scale and long-term ecological surveys as a basis for risk assessment and the prediction of future scenarios (Johannesen et al., 2012; Eriksen et al., 2017; Prozorkevich and Sunnanå, 2017; Eriksen et al., 2018b; Arneberg et al., 2020). Elucidating the present-day variability in diets of the key zooplankton species is crucial to gain a better understanding of the impacts of climate change on the Barents Sea food-web. In this context, the Nansen Legacy Project (arvenetternansen.com) represents a Norwegian research effort to address the impact of climate change on biological, chemical and physical processes in the northern Barents Sea on a seasonal scale. As a contribution to this project, we explored the dietary carbon sources, including sea-ice algae, for Barents Sea zooplankton collected during late summer 2019 to infer food-web interactions at the base of the marine ecosystem during the late summer period. This research question was approached comprehensively by sampling at multiple stations along a south-north transect using a multi-trophic marker approach. The results are discussed in the context of a reducing Arctic sea-ice cover.
Materials and Methods
Sample Collection
Sampling was carried out as part of the Norwegian Nansen Legacy project during the seasonal cruise Q3 (05–27 August 2019) with RV Kronprins Haakon north of 76°N in the Barents Sea (Figure 1). During the sampling, areas represented by sampling stations P1 and P2 were ice-free, P4 was located close to the ice edge, and stations P5, P6, and P7 were ice-covered (Figure 1). Additional information about individual sampling stations can be found in Supplementary Table 1.
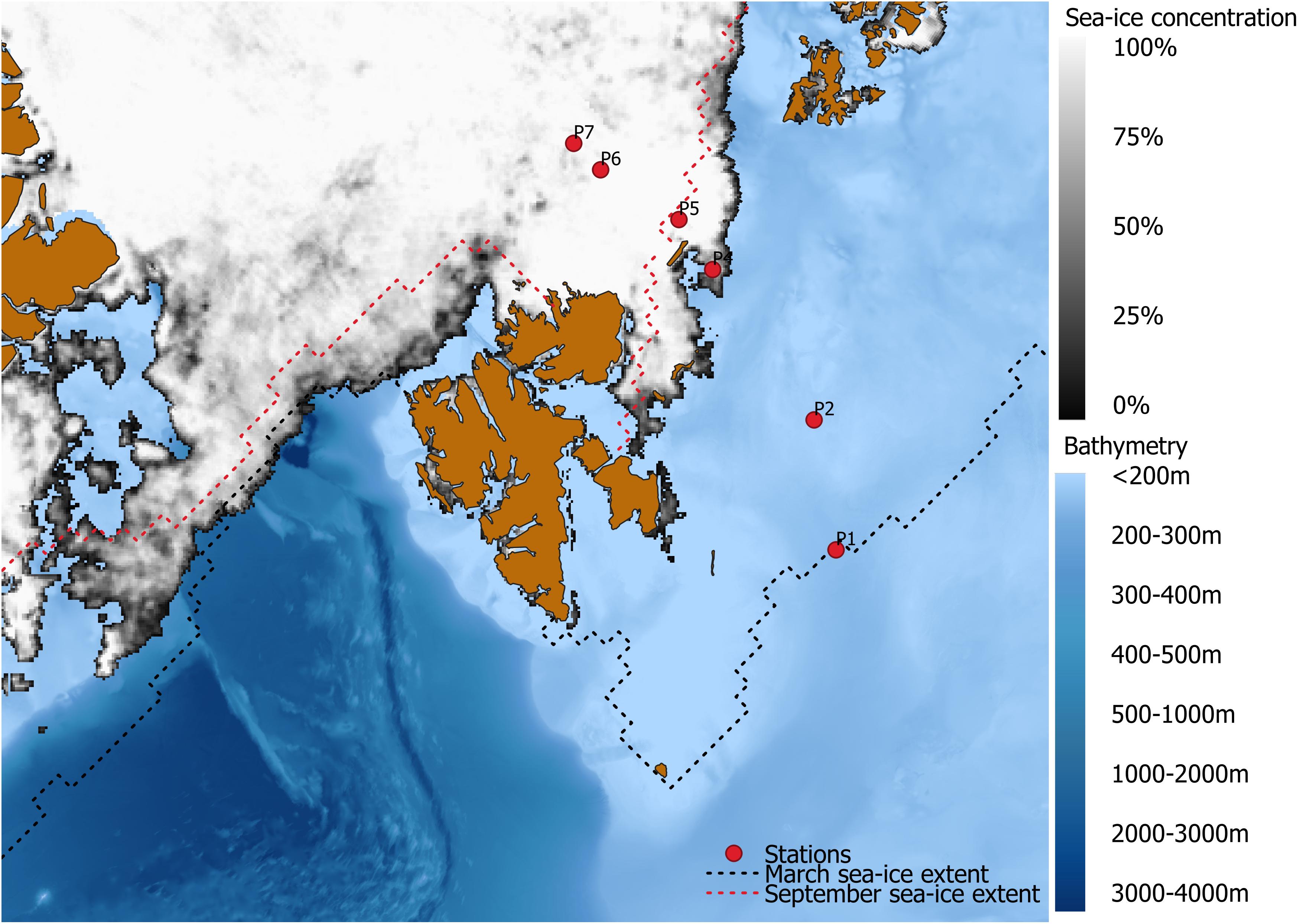
Figure 1. Map showing the Nansen Legacy transect across the Barents Sea in August 2019. Sea-ice concentration (SIC%) along the sampling transect is shown for 15 August 2019. SIC data was acquired from Bremen University (https://seaice.uni-bremen.de/sea-ice-concentration/amsre-amsr2/) (Spreen et al., 2008). Maximum sea-extent occurred in March (black stippled line), minimum sea-ice extent in September 2019 (red stippled line) [acquired from National Snow and Ice Data Center; Fetterer et al. (2017)].
Samples of 24 zooplankton taxa including copepods, krill, amphipods, pteropods and gelatinous species (Table 1) were collected at six stations. We used MIK nets (1,200 μm with 500 μm cod end) and WP3 net (1,000 μm) for large taxa; Macroplankton trawl for mostly Thysanoessa spp. (multiple mesh sizes along the net, tapering to 8 mm at its end); Bongo net (180 μm) and Multinet (180 μm) for copepods (all species); and WP2 net (90 μm) for small copepods (Supplementary Table 1). Samples were sorted into the lowest possible taxonomic level, and/or stage/size groups (where possible) onboard the ship and immediately frozen at −80°C in 2 mL cryovials. The three Calanus species were differentiated based on morphology and prosome lengths according to Kwasniewski et al. (2003). Small species/individuals were pooled by species (and by stage/size group, if applicable) in order to obtain sufficient sample material for analyses (Table 1).
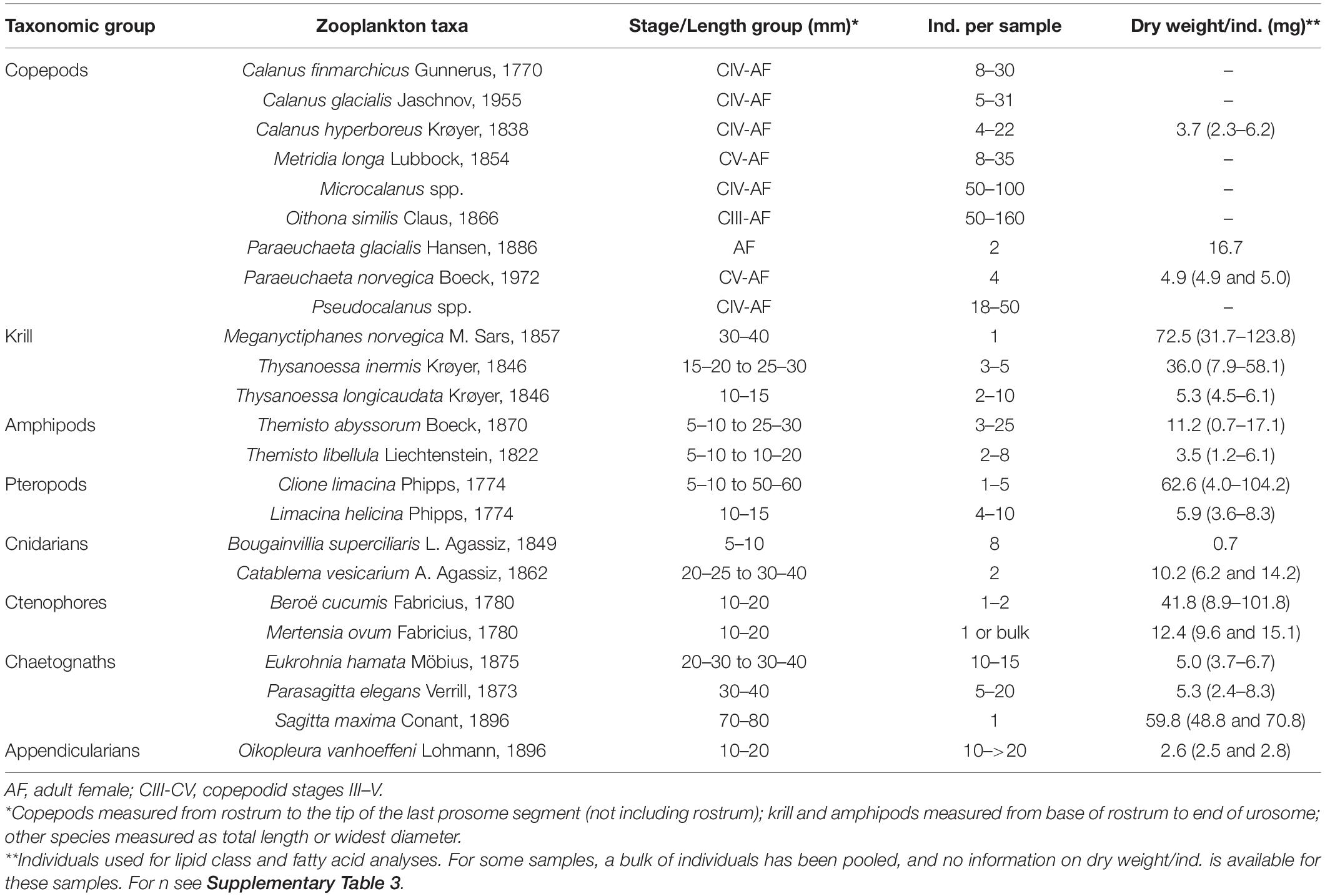
Table 1. Overview of zooplankton taxa collected during Nansen Legacy cruise Q3 in August 2019 in the Barents Sea.
Pelagic particulate organic matter (PPOM) was collected at the chlorophyll a (Chl a) maximum with Niskin bottles attached to a CTD rosette, which varied in depth from 14 to 73 m at the six investigated stations. Volumes from 1.2 to 2.5 L of seawater were filtered via a vacuum pump (−20 kPA) through pre-combusted (3 h, 550°C) 47 mm Whatman® GF/F filters. Ice-associated POM (IPOM) was collected at two stations by sampling ice cores using an ice corer of 9 cm interior diameter (Kovacs Enterprises, Inc., United States). The lowest 10 cm of the ice cores were melted in the dark at 4 °C without the addition of seawater, which was assumed to have a negligible effect on the bulk biochemical properties of IPOM (Roukaerts et al., 2019). Samples for taxonomic analysis were melted with the addition of filtered seawater to conserve the more delicate algae, such as flagellates, during the melting process (Garrison and Buck, 1986). For each sample, 600 mL of melted ice sample was filtered through pre-combusted GF/F filters. All filters were stored at −80°C until further processing.
Lipid Classes and Fatty Acids (FAs)
Lipid classes and FAs were analyzed at the Alfred Wegener Institute, Bremerhaven, Germany. Detailed information on analytical procedure and lab equipment can be found in Kohlbach et al. (2016). Samples were freeze-dried and zooplankton dry weights were determined gravimetrically (Table 1; no dry weights were available for C. finmarchicus, C. glacialis and small copepods). Briefly, an internal standard (C23:0) was added and total lipids were extracted using a modified procedure from Folch et al. (1957) with dichloromethane/methanol (2:1, v/v). Lipid class analysis was performed directly on the extracted lipids (Graeve and Janssen, 2009) via high performance liquid chromatography. Lipid classes were distinguished into neutral (i.e., storage) lipids and polar (i.e., membrane) lipids (Supplementary Table 2). Lipid class analysis was not performed on Metridia longa, Microcalanus spp., Oithona similis, and Pseudocalanus spp. due to lack of sufficient sample material. In these taxa, the relative proportions of wax esters were estimated from the relation of FAs to fatty alcohols.
The extracted lipids were converted into fatty acid methyl esters (FAMEs) and free fatty alcohols derived from wax esters by transesterification in methanol, containing 3% concentrated sulfuric acid. After a subsequent hexane extraction, the FAMEs and alcohols were separated by gas chromatography. PPOM samples were analyzed in triplicates per station (total n = 18) and IPOM samples in duplicates per station (total n = 4).
Fatty acids were expressed by the nomenclature A:B(n−X); where A represents the number of carbon atoms, B the amount of double bonds, and X is the position of the first double bond starting from the methyl end of the carbon chain. The proportions of individual FAs were expressed as mass percentage of the total FA content.
In this study, we focus on trophic marker FAs that can be used to trace food-source preferences through the food web. The FAs 16:1(n−7), 16:4(n−1), and 20:5(n−3) are mostly produced by diatoms (i.e., diatom-associated FAs), and the FAs 18:4(n−3) and 22:6(n−3) (i.e., dinoflagellate-associated FAs) are predominantly produced by dinoflagellates and the prymnesiophyte Phaeocystis (Falk-Petersen et al., 1998; Reuss and Poulsen, 2002; Dalsgaard et al., 2003). Trophic marker ratios of the FAs 16:1(n−7)/16:0, the sum of C16 FAs (produced in high amounts by diatoms) versus the sum of C18 FAs (produced in high amounts by dinoflagellates; hereafter referred to as ΣC16/ΣC18), and the FA ratio 20:5(n-3)/22:6(n-3) > 1 can indicate a dominance of diatom-produced versus dinoflagellate-produced carbon in an algal community or carbon pool of a consumer. The monounsaturated long-chained FAs 20:1 and 22:1, which are isomers synthesized de novo by copepods, typically indicate the importance of copepods, such as Calanus spp., as a food source for a consumer (Sargent and Falk-Petersen, 1988; Falk-Petersen et al., 1990). The relative proportion of polyunsaturated FAs (PUFAs) can be used as an indicator of food quality of an algal community or as a herbivore index for a consumer based on the assumption that algae are the main producers of PUFAs (Stevens et al., 2004). Additionally, high ratios of 18:1(n−9)/18:1(n−7) indicate an omnivorous/carnivorous/detritivorous rather than herbivorous feeding mode (Graeve et al., 1997; Falk-Petersen et al., 2000; Auel et al., 2002).
Highly Branched Isoprenoids (HBIs) and Sterols
Highly branched isoprenoids and sterols were analyzed at the University of Plymouth, United Kingdom. Detailed information about analytical procedure and lab equipment can be found in Brown and Belt (2012) and Schmidt et al. (2018).
Briefly, samples were freeze-dried and homogenized. Two standards, 9-octyl-8-heptadecene and 7-hexylnonadecane, were added for subsequent trophic marker quantification. Total lipids were extracted with chloroform/methanol (2:1, v/v). Thereafter, samples were saponified with 20% potassium hydroxide in water/methanol. Non-saponifiable lipids were extracted with hexane and purified by open column chromatography filled with SiO2 and HBIs were analyzed by gas chromatography-mass spectrometry (GC-MS). Quantification of HBIs was achieved by integrating individual ion responses in single-ion monitoring mode, and normalizing these to the corresponding peak area of the internal standard and an instrumental response factor obtained from purified standards (Belt et al., 2012). The GC-MS-derived masses of the HBIs were converted to concentrations in body fractions of the zooplankton using the lipid mass of the extracted sample. The two tri-unsaturated HBIs, hereafter referred to as HBIs III and IV (m/z 346.3), can indicate the consumption of pelagic algal material, including that from the marginal ice zone (MIZ) (Brown et al., 2014; Belt, 2018), while the mono- and di-unsaturated HBIs IP25 (m/z 350.3) and IPSO25 (m/z 348.3) are assumed to be produced exclusively by certain sea-ice diatoms, providing information on the origin of carbon sources in a consumer (Brown et al., 2018; Schmidt et al., 2018).
Sterols were eluted from the same silica column using hexane:methylacetate (4:1,v/v). Sterol fractions were derivatised using N,O-bis(trimethylsilyl)trifluoroacetamide (BSTFA) and analyzed by GC-MS. Individual sterols were identified by comparison of the mass spectra of their trimethylsilyl-ethers with published data (Belt et al., 2018). The principal identifiable sterols were the phytoplankton-produced sterols (hereafter referred to as phytosterols) brassicasterol (24-methylcholesta-5,22E-dien-3β–ol; m/z 470), sitosterol (24-ethylcholest-5-en-3β–ol; m/z 396), chalinasterol (24-methylcholesta-5,24(28)-dien-3β–ol; m/z 470) and campesterol (24-methylcholest-5-en-3β–ol; m/z 382) and the phyto-and-zooplankton-produced sterols (hereafter referred to as zoosterols) cholesterol (Cholest-5-en-3β–ol; m/z 458), desmosterol (Cholesta-5,24-dien-3β–ol; m/z 343) and dehydrocholesterol (Cholesta-5,22E-dien-3β–ol; m/z 327).
Statistical Analyses
Spatial variability in PPOM and Calanus spp. FAs was tested for statistical significance with 1-way ANOVAs followed by Tukey’s HSD post hoc tests. Results with p ≤ 0.05 were considered significant. All measures of statistical variation are reported as means ± 1 SD. FA data were transformed by applying an arcsine square root function to meet normality requirements for parametric statistics (Legendre and Legendre, 2012). Variability in FA datasets of PPOM and IPOM, as well as spatial variability in Calanus copepods, was visualized with correspondence analysis (CA), which is suitable for analyzing compositional data (Greenacre and Primicerio, 2014; Greenacre, 2017). Differences in FA composition between zooplankton taxa were visualized with a principal component analysis (PCA) and tested for statistical relevance with ANOSIM (dissimilarity measure: Bray–Curtis). Correlation between total zoosterols/total phytosterols and the FA ratio 18:1(n-9)/18:1(n-7) as well as HBI concentrations versus the total abundance of phytosterols and zoosterols was tested using Pearson’s correlation; data have been LOG-transformed to appropriately present species-specific distributions. To visually summarize the main results of this study, a PCA was applied to show zooplankton-specific differences in marker FA ratios, HBI concentrations and degree of carnivory. All statistical analyses were run in R v.3.4.3 (R Core Team, 2017) using the vegan (Oksanen et al., 2019) and ggplot2 packages (Wickham, 2016), the map was produced with QGIS version 3.16.1.
Results
FA Composition of Pelagic and Ice-Associated Particulate Organic Matter (PPOM and IPOM)
Our CA explained ∼84% of the variability in the FA dataset, showing that the FA composition in PPOM (P1-P7) differed from that in IPOM (P6_ice, P7_ice; Figure 2). While PPOM was associated with higher mean proportional contributions of the dinoflagellate-associated FAs 18:4(n−3) and 22:6(n−3), the mean relative proportion of the diatom-associated FA 16:1(n−7) was more than four times higher in IPOM than in PPOM. In IPOM, all three trophic marker FA ratios indicated a dominance of diatoms over dinoflagellates (Table 2). In PPOM, both diatom- and dinoflagellate-associated FA contributed largely to the FA composition, and contributions were more equally distributed compared to IPOM.
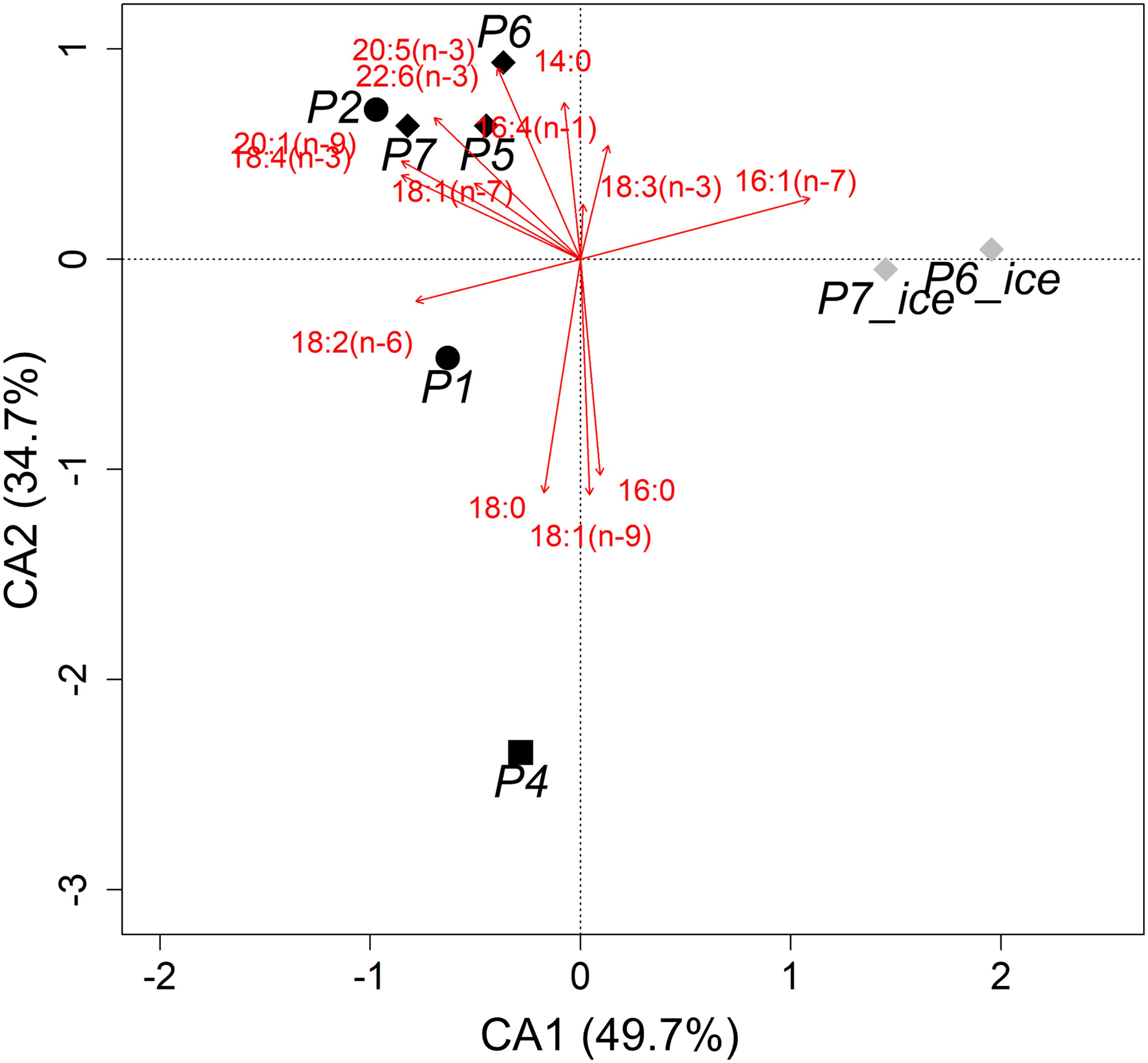
Figure 2. Contribution biplot of correspondence analysis (CA) of fatty acids (≥1% to the total FA content) in pelagic particulate organic matter (PPOM; black symbols) and ice-associated POM (IPOM; gray symbols) collected during Nansen Legacy cruise Q3 in August 2019 in the Barents Sea. Shapes represent sampling locations with different sea-ice concentrations (P1 and P2: ice-free; P4: close to ice edge; P5, P6, and P7: ice-covered).
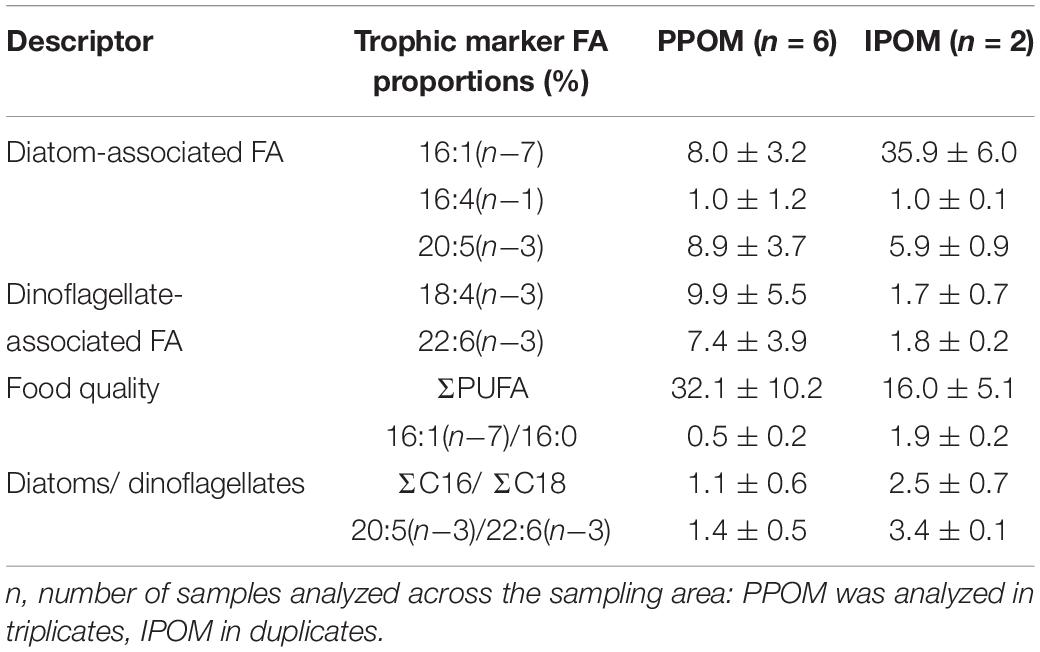
Table 2. Relative proportions of trophic marker fatty acids (FAs; mean ± SD%) and FA ratios (mean ± SD) in pelagic particulate organic matter (PPOM) and ice-associated POM (IPOM) collected during Nansen Legacy cruise Q3 in August 2019 in the Barents Sea.
There was large variability in PPOM FA proportions along the sampling transect. PPOM from samples collected at stations P1 and P4 were clearly separated from the other stations in the CA plot with significantly higher mean relative contributions of FA 18:1(n−9) (10 and 24%, respectively) compared to most of the other stations (3–5%, respectively, Figure 2; ANOVA, F5,12 = 55.1, p < 0.001, Tukey HSD p < 0.05). Additionally, PPOM from samples collected at station P4 had significantly higher relative proportions of 18:0 (mean 22%) compared to most other stations (mean 4–14%; F5,12 = 7.9, p = 0.002, Tukey HSD p < 0.05). In contrast, the relative proportions of the diatom-associated FAs 16:1(n−7) (F5,12 = 12.3, p < 0.001, Tukey HSD p < 0.05) and 20:5(n−3) (F5,12 = 21.4, p < 0.001, Tukey HSD p < 0.001), and the dinoflagellate-associated FAs 18:4(n−3) (F5,12 = 14.7, p < 0.001, Tukey HSD p < 0.05) and 22:6(n−3) (F5,12 = 9.9, p < 0.001, Tukey HSD p < 0.05) were lower at P4 compared to most of the other stations. At station P1, relative proportions of 16:1(n−7) in PPOM were also lower compared to stations P5 and P6, and relative proportions of 20:5(n−3) were lower compared to stations P5, P6, and P7.
Lipid Class and FA Composition of Barents Sea Zooplankton
In all copepod taxa as well as krill, amphipods and the pteropod Clione limacina, the cnidarians, the chaetognath Eukrohnia hamata, and the ctenophore Mertensia ovum, mean relative proportions of neutral (storage) lipids were higher than those of polar (membrane) lipids. In contrast, the pteropod Limacina helicina, the ctenophore Beroë cucumis, the chaetognaths Parasagitta elegans and Sagitta maxima and the appendicularian Oikopleura vanhoeffeni had the reverse. Levels of wax esters (neutral lipids) were generally high in all copepod species (mean > 90% in Paraeuchaeta spp.) and E. hamata (mean 64%). Triacylglycerols (TAGs) dominated the neutral lipid fraction in the amphipods, the krill Meganyctiphanes norvegica and Thysanoessa longicaudata, and C. limacina (up to 76%). Among the polar lipids, phosphatidylethanolamine (PE) and phosphatidylcholine (PC) were the most abundant lipid classes, with up to 23% of the total lipids in O. vanhoeffeni and up to 48% in Parasagitta/Sagitta spp., respectively. Lipid class compositions of individual taxa are reported in Supplementary Table 2.
In the PCA (explained ∼65% of the variance in the FA dataset), all taxonomic groups (ANOSIM, R = 0.328, p = 0.001) and most species (ANOSIM, R = 0.878, p < 0.001) could be clearly distinguished from each other based on their FA profiles (Figure 3). Except for the copepods Oithona similis and Pseudocalanus spp. and the cnidarian Catablema vesicarium, FA proportions were similar within a species/taxa.
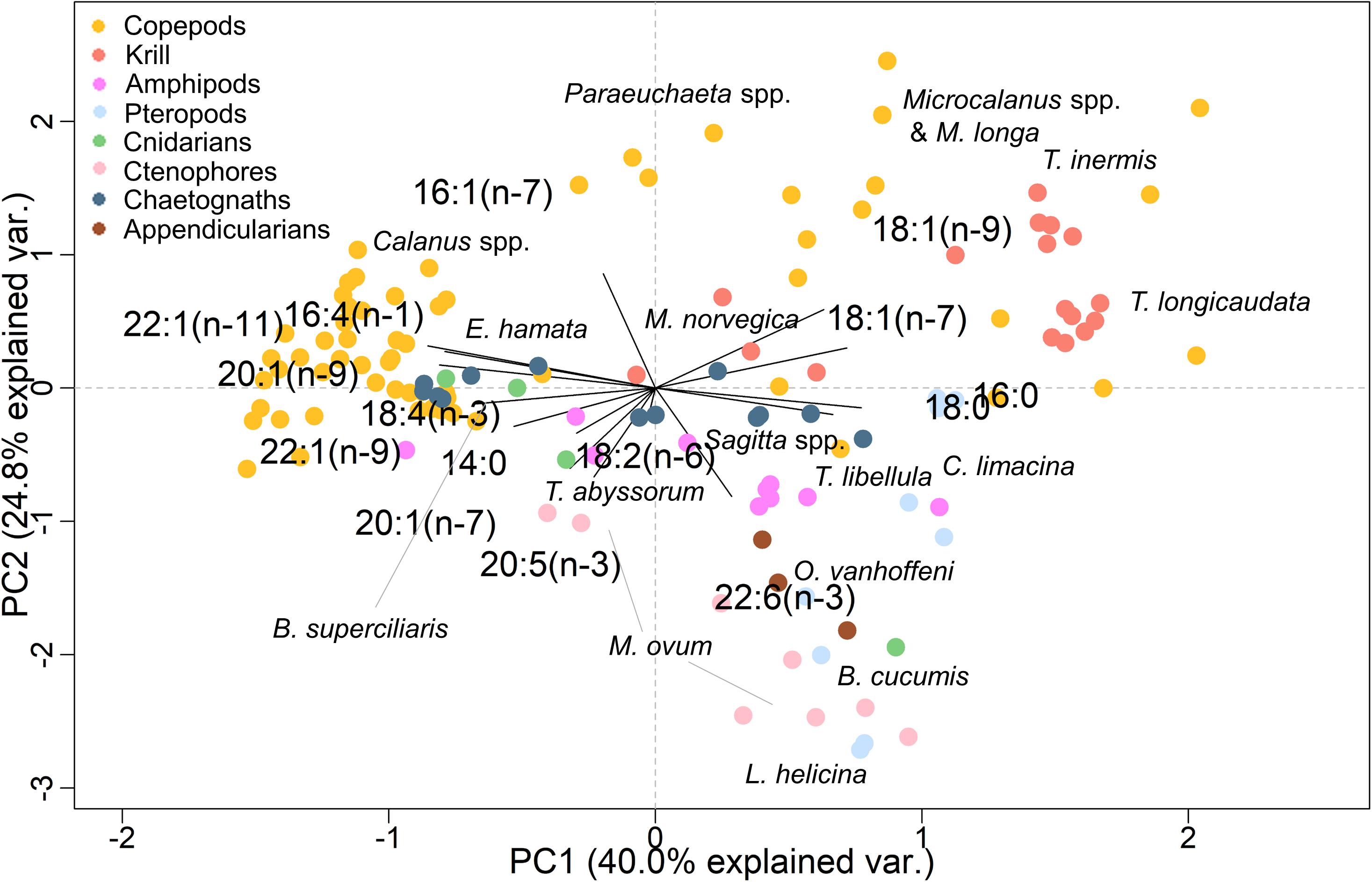
Figure 3. Principal component analysis (PCA) of fatty acids (FAs; ≥1% to the total FA content) in the zooplankton collected during Nansen Legacy cruise Q3 in August 2019 in the Barents Sea. Relative proportions of individual FAs are shown in Supplementary Table 3.
Among all taxa, the copepod Metridia longa had the highest mean relative proportion of the diatom-associated FA 16:1(n−7) (26%), and B. cucumis had the lowest mean relative level of this FA (2%). The diatom-associated FA 16:4(n−1) showed the maximum mean proportion in the copepod Calanus hyperboreus (3%), and the minimum in B. cucumis and C. limacina (both 0.1%). The proportions of the diatom-associated FA 20:5(n−3) were lower in the copepods Microcalanus spp. (7%) and Paraeuchaeta spp. (7%) compared to all other taxa (10–24%). Calanus hyperboreus had a substantially higher mean relative proportion of the dinoflagellate-associated FA 18:4(n−3) (11%) than all other taxa. Amphipods (11 and 19%), pteropods (15 and 25%) and gelatinous zooplankton (8–24%) had distinctly higher relative proportions of the dinoflagellate-associated FA 22:6(n−3) than most copepods (4–8%) and krill (4–7%). Only in the amphipod T. libellula and the ctenophores were all three diatom/dinoflagellate ratios ≤ 1. Clione limacina was the only species with a mean ratio of 18:1(n−9)/18:1(n−7) < 1 (Table 3). Fatty acid compositions of individual taxa are reported in Supplementary Table 3.
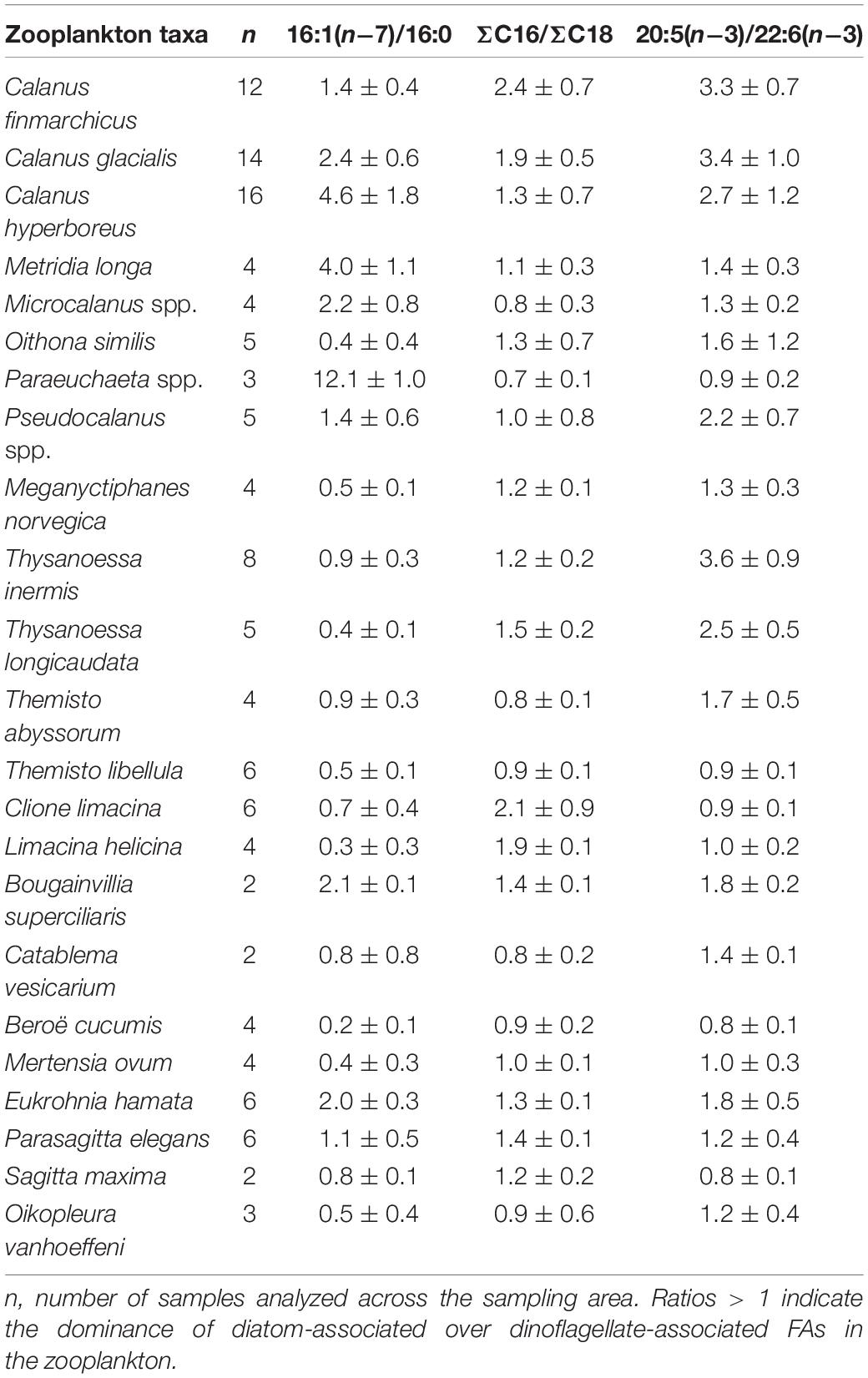
Table 3. Ratios of fatty acids (FAs; mean ± SD) in zooplankton collected during Nansen Legacy cruise Q3 in August 2019 in the Barents Sea.
In all three Calanus species, the FA composition varied along the sampling transect (Figures 4A–C). In all three species, individuals from stations with low proportions of 16:1(n−7), i.e., station P7 for C. finmarchicus (Figure 4A) and C. hyperboreus (Figure 4C) and station P1 for C. glacialis (Figure 4C), were associated with a higher degree of carnivory, i.e., higher ratios of 18:1(n−9)/18:1(n−7). Relative proportions of the diatom-associated FA 20:5(n−3) were high in all species from station P7. Overall, C. glacialis from station P1 had the lowest diatom signal, i.e., low relative proportions of the diatom-associated FAs 16:1(n−7), 16:4(n−1), and 20:5(n−3) [low ratios of 16:1(n−7)/16:0, ΣC16/ΣC18 and 20:5(n−3)/22:6(n−3)], but the strongest dinoflagellate signal, i.e., high proportions of the dinoflagellate-associated FAs 18:4(n−3) and 22:6(n−3), among the four sampled stations (Figure 4B). Additionally, in C. finmarchicus and C. hyperboreus, individuals from station P6 were different in their FA composition from that of the other stations (Figures 4A,C). They were associated with higher levels of the diatom-associated FAs 16:4(n−1) and also 20:5(n−3) compared to the other stations. Furthermore, C. hyperboreus from station P6 had a low dinoflagellate signal, i.e., low levels of 18:4(n−3) and 22:6(n−3). Statistical output is reported in Supplementary Table 4.
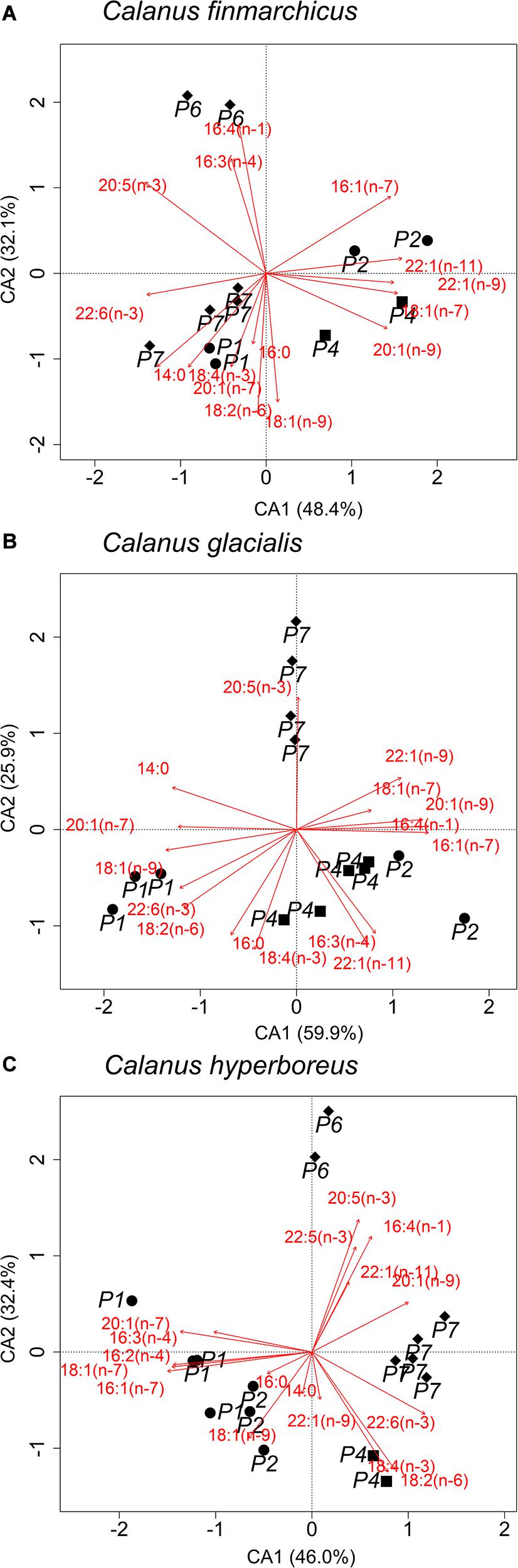
Figure 4. Contribution biplot of correspondence analysis (CA) of fatty acids (≥1% to the total FA content) representing spatial variability in (A) Calanus finmarchicus, (B) C. glacialis and (C) C. hyperboreus collected during Nansen Legacy cruise Q3 in August 2019 in the Barents Sea. Shapes represent sampling locations with different sea ice concentrations (P1 and P2: ice-free; P4: close to ice edge; P6 and P7: ice-covered).
HBIs and Sterols
None of the pelagic/MIZ and sea ice algae-associated HBIs were detected in PPOM. The pelagic/MIZ HBIs were found in all zooplankton taxa, except for the small copepod species Microcalanus spp., O. similis, Pseudocalanus spp., the pteropod C. limacina, the chaetognath S. maxima and the appendicularian O. vanhoeffeni. Maximum concentrations of both HBIs III and IV were found in the cnidarian C. vesicarium. The sea ice algae-associated HBI IP25 was detected in the amphipod Themisto abyssorum, the pteropod L. helicina and O. vanhoeffeni (Figure 5A). The other sea ice algae-associated HBI IPSO25 was found in the krill M. norvegica and Thysanoessa inermis, the amphipods T. abyssorum and T. libellula, C. limacina and L. helicina as well as O. vanhoeffeni. Limacina helicina had the highest concentrations of both sea ice algae-associated HBIs. In all taxa, species-specific variability in HBI concentrations was high. Mean ratios of sea ice algae-associated to pelagic/MIZ HBIs were >1 in T. abyssorum, the two pteropod species and O. vanhoeffeni. HBI concentrations of individual taxa/species are reported in Supplementary Table 5.
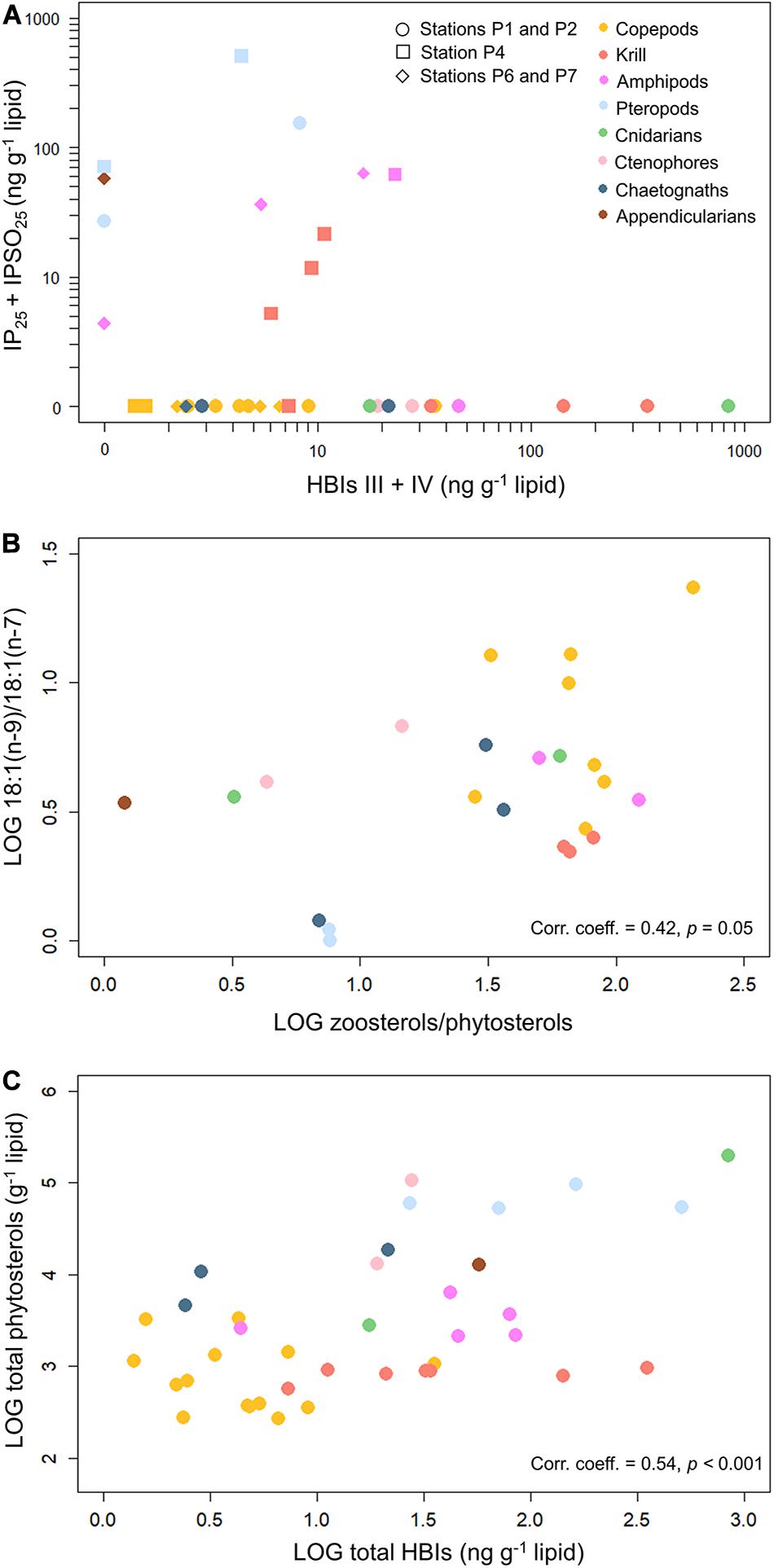
Figure 5. (A) Concentrations of the pelagic/MIZ HBIs III and IV versus the sea ice algae-associated HBIs IP25 and IPSO25 in the zooplankton collected during Nansen Legacy cruise Q3 in August 2019 in the Barents Sea. Considered are only samples that contained either pelagic/MIZ or sea ice algae-associated HBIs or both. Datapoints represent individual samples. All data can be found in Supplementary Table 5. (B) Pearson’s correlation of the mean ratio of zoosterols/phytosterols versus the mean carnivory index 18:1(n–9)/18:1(n–7) in the zooplankton. (C) Pearson’s correlation of total phytosterols versus total HBIs in the zooplankton. Considered are only samples that either contained pelagic/MIZ or sea ice algae-associated HBIs or both. Datapoints represent individual samples.
In most zooplankton, the most abundant phytosterols were brassicasterol (up to 90% of total phytosterols) and campesterol (up to 69% of total phytosterols). In most taxa, desmosterol had the highest relative contribution to the total zoosterol content (up to 90%), while in krill, cholesterol had the highest relative contributions (up to 93% of total zoosterols). Ratios of the four phytosterols versus cholesterol were generally the highest in O. vanhoeffeni and the pteropods and the lowest in P. norvegica and krill (Supplementary Figure 1).
The relationship of the FA carnivory ratio 18:1(n−9)/18:1(n−7) versus total zoosterols/total phytosterols was positively correlated (Figure 5B). For both ratios, P. norvegica had the highest values. The pteropods and O. vanhoeffeni showed lower values than most of the other taxa.
In the zooplankton, the total abundance of phytosterols had a strong positive correlation with the total HBI concentrations (Figure 5C), and this significant correlation was found for all four phytosterols. The correlation between phytosterols and total HBIs was stronger than the correlation between zoosterols and total HBIs (Supplementary Figure 2).
Carbon Sources of Barents Sea Zooplankton: Summary
Fatty acid ratios indicating the dominance of diatom-associated carbon versus dinoflagellate-associated carbon were the highest in the copepods Paraeuchaeta spp. and Calanus spp., and the lowest in the ctenophores B. cucumis and M. ovum as well as the amphipod T. libellula (Figure 6A).
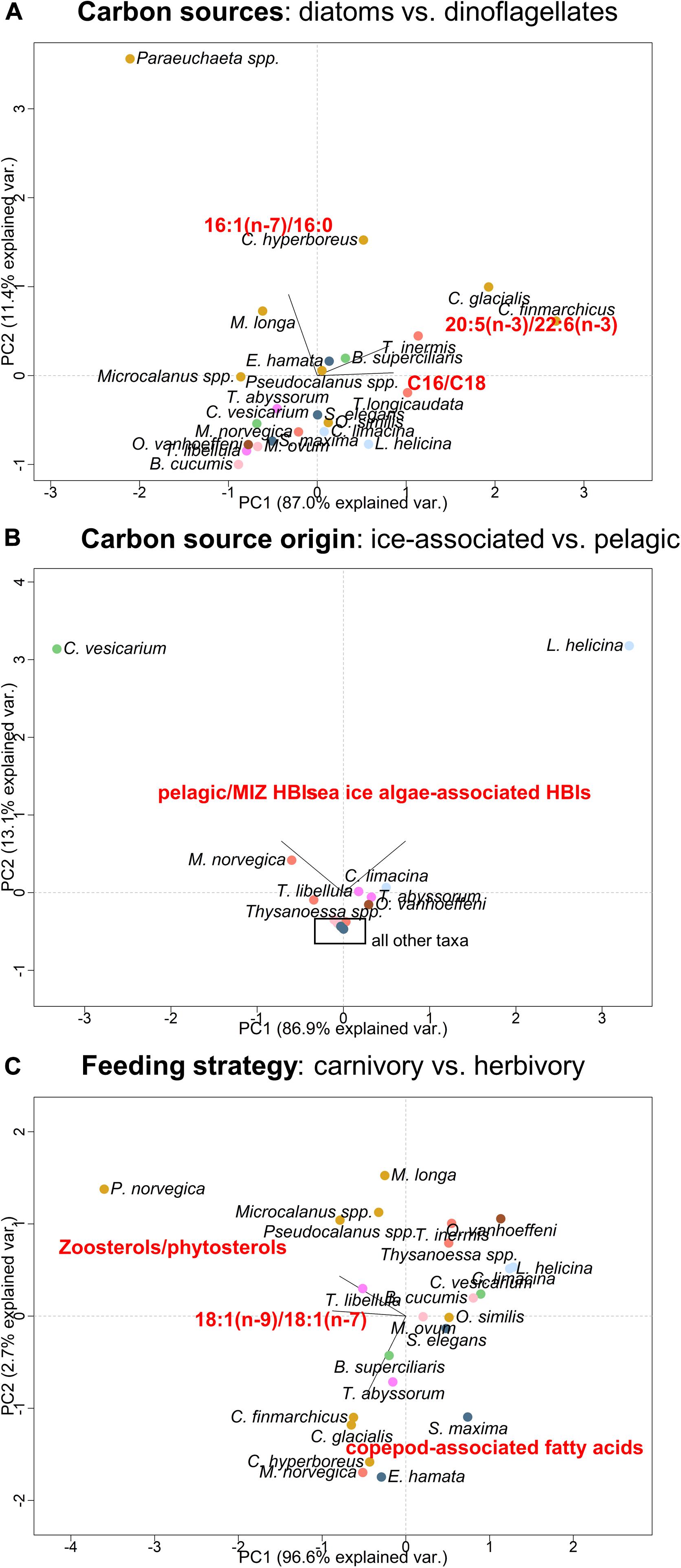
Figure 6. Summary of results representing principal component analysis (PCA) of (A) the carbon source composition based on diatom- versus dinoflagellate-associated fatty acids (FAs; trophic marker FA ratios are shown in Table 3), (B) the origin of these carbon sources based on concentrations of pelagic/MIZ highly branched isoprenoids (HBIs III and IV) and sea ice algae-associated HBIs (IP25, IPSO25; HBI concentrations are shown in Figure 5A and Supplementary Table 5) and (C) the degree of carnivory versus herbivory in the zooplankton taxa based on concentrations of zoosterols/phytosterols, and relative proportions of the carnivory FA 18:1(n–9) versus the algal-produced FA 18:1(n–7), and calanoid copepod-associated FAs 20:1 and 22:1 (FA proportions are shown in Supplementary Table 3).
Pelagic/MIZ HBIs were found in all taxa, except for small copepods, with highest concentrations in the cnidarian C. vesicarium. Sea ice algae-associated HBIs were found in the pteropod L. helicina in highest concentrations, and further in the pteropod C. limacina, the krill M. norvegica and T. inermis, the amphipods Themisto spp. and the appendicularian O. vanhoeffeni (Figure 6B).
Fatty acid- and sterol-based carnivory ratios were the highest in the copepod P. norvegica and other copepods and the lowest in O. vanhoeffeni. The highest proportions of copepod-associated FAs were found in M. norvegica, and the lowest proportions in the krill Thysanoessa spp., the pteropods and O. vanhoeffeni (Figure 6C).
Discussion
Composition of PPOM and IPOM
During our sampling period, pelagic and sea ice-associated algal communities, referred to as PPOM and IPOM, respectively, differed in their FA composition (Figure 2). The diatom-associated FA 16:1(n−7) has frequently been found to be strongly associated with Arctic sea-ice diatoms (Henderson et al., 1998; Budge et al., 2008; Kohlbach et al., 2016), suggesting a diatom-dominated sea-ice community in the current study, in agreement with microscopic analysis which revealed high abundances of typical sea ice-associated pennate diatoms, such as Nitzschia spp. However, diatom-produced marker FAs accounted for almost 20% of the PPOM FAs, suggesting that diatoms also played an important part in structuring the pelagic algal community (Ratkova and Wassmann, 2002). Furthermore, elevated levels of the FAs 18:4(n−3) and 22:6(n−3) in PPOM indicated the presence of flagellates/prymnesiophytes in the water column, typical for European Arctic protistan plankton during summer (Wassmann et al., 2005, 2006). Taxonomic analysis confirmed the importance of diatoms, including Thalassiosira and Fragilariopsis genera, and dinoflagellates, e.g., Gymnodinium spp. and taxa of the order Peridiniales, for the phytoplankton community composition. The prymnesiophyte Phaeocystis was found in high abundances at stations P4, P6, and P7.
Carbon Sources of Barents Sea Zooplankton During Late Summer
In this study, all of the diatom- and dinoflagellate-associated trophic marker FAs were found in all investigated zooplankton. Thus, all taxa are capable of utilizing carbon of different origin, directly obtained and/or, more likely based on the generally high carnivory indices in this study (presence of copepod-associated FAs, high 18:1(n−9)/18:1(n−7) ratios, presence of zoosterols), indirectly by feeding on other organisms. The overall high degree of carnivory among the zooplankton agrees with findings that dominant species prefer microzooplankton (if abundant) over phytoplankton (Campbell et al., 2009; Stoecker and Pierson, 2019). Additionally, it could reflect a high abundance of heterotrophic protists in the sampling region (Wassmann et al., 2005), and it is noteworthy that heterotrophic or mixotrophic dinoflagellates of the genus Gymnodinium were a key component of the phytoplankton community in this and other studies in the Arctic (Kubiszyn and Wiktor, 2016; Hardge et al., 2017).
Copepods, Krill and Amphipods
Overall, the diverse taxonomic group of copepods showed the strongest diatom signal in their FA pool among the different zooplankton groups (Table 3 and Figure 6A). Diatoms have been identified as major food items for Calanus spp. (Søreide et al., 2008; Cleary et al., 2017), and most of the other copepods have also been reported to utilize diatom-derived carbon (Atkinson, 1996; Cleary et al., 2016). Calanus hyperboreus was more likely to graze directly on autotrophic algae according to its lower degree of carnivory [lower 18:1(n−9)/18:1(n−7), higher levels of PUFAs] and its high proportions of the short-term FA 18:4(n−3) compared to all other copepods (Stevens et al., 2004), suggesting also the ingestion of fresh flagellate material and/or Phaeocystis (Hansen et al., 1994; Søreide et al., 2008).
Variability in FA composition in all three Calanus species along the transect (Figures 4A–C) showed high species-specific consistency, and likely reflects spatial and temporal variations in carbon and food sources, i.e., composition of algal communities and prey species. Variability in FA composition was also pronounced in PPOM (Figure 2) and suggested differences in community structure, which was likely the result of varying environmental factors, e.g., Atlantic versus Arctic water masses and water-mass characteristics (temperature and salinity), mixing processes, sea-ice concentrations and nutrient availability (Engelsen et al., 2002; Ardyna et al., 2011). In all three Calanus species, stations with higher 18:1(n−9)/18:1(n−7) ratios were also linked to a lower diatom signal: in C. glacialis at station P1 and in the other two Calanus species at station P7, where a high proportion of unidentified (possibly heterotrophic or mixotrophic) eukaryotic species contributed to the pelagic protist composition. This might mirror an increased availability of preferred heterotrophic prey at these sampling locations other than diatoms, resulting in a higher degree of carnivory in these species (Søreide et al., 2008).
In comparison to our study, samples from the central Arctic Ocean (CAO) in August/September (Kohlbach et al., 2016) had a considerably higher contribution of the diatom-associated FA 16:1(n−7) in both IPOM (mean over 50% versus mean 36% in this study) and Calanus copepods (e.g., mean 26% in C. glacialis versus mean 16% in this study). Based on FA-specific stable isotope mixing models, ice-associated carbon contributed up to 48% of the copepods’ carbon budget in the CAO (Kohlbach et al., 2016), and was similar to what was reported for the Bering Sea during summer ice-free conditions with up to 54% (Wang et al., 2015). However, the lack of sea ice algae-associated HBIs in all of the investigated copepod taxa indicates that carbon was mainly obtained from phytoplankton and pelagic prey during our study representative of the late summer season. In contrast to the Barents Sea, ice algal primary production in the CAO can constitute over half of the total primary production during August/September due to the extensive sea-ice coverage, somewhat counteracting the relatively poor pelagic primary production in the CAO (Fernández Méndez, 2014; Fernández-Méndez et al., 2015). Consequently, the generally high availability of sea-ice algae in a sea ice-dominated ecosystem such as the CAO likely promotes and necessitates an increased dependency on ice-associated carbon sources, which could rapidly change with further reduction in sea ice and longer open-water season. However, it needs to be noted that one of the dominant sea-ice diatoms during the above-mentioned studies from the CAO, Melosira arctica, is not known to produce HBIs and is therefore not captured by our biomarker approach.
HBIs produced by algae in open waters or the MIZ (HBIs III and IV) were found in all sampled taxa except for the small copepods Microcalanus spp., O. similis and Pseudocalanus spp. (Figure 5A). Along with their high ratios of 18:1(n−9)/18:1(n−7) and elevated levels of zoosterols, this suggests further that these small copepod species were feeding to a greater extent as omnivores, carnivores or detritivores (Norrbin et al., 1990; Lischka and Hagen, 2007). Oithona similis has been described as an ambush feeder (Svensen and Kiørboe, 2000), preferring mobile prey such as ciliates, and is only rarely found to feed on diatoms (Zamora-Terol et al., 2013), in line with the lower diatom FA signal compared to the other copepods in our study. Furthermore, phytoplankton assemblages containing smaller protist taxa might have hampered efficient grazing by copepods with a different prey-size preference, such as O. similis (10–40 μm; Zamora-Terol et al., 2013).
Despite the common co-occurrence of the two sea ice algae-associated HBIs IP25 and IPSO25 in Arctic sea-ice diatoms (Belt and Müller, 2013; Brown et al., 2014; Belt et al., 2016), only IPSO25 was detected, for example in the krill species, whereas IP25 was absent. This could have many reasons, such as lack or unavailability of source taxa, differing nutrient availability affecting metabolite production (Brown et al., 2020), or selective feeding of these zooplankton taxa. It could also point to different metabolic turnover rates or concentrations of the two HBIs in the consumer, retaining IPSO25 in the animal’s tissue while concentrations of IP25 were already below the detection limit as IPSO25 can be several times more abundant than IP25 in Arctic animals (Brown et al., 2015). Pelagic HBIs were found in substantially higher concentrations in M. norvegica in comparison to Thysanoessa spp., and its stronger pelagic signal was supported by the FA compositions.
Based on all three diatom/dinoflagellate ratios being <1, low levels of the diatom-associated FA 16:1(n−7), but high levels of the dinoflagellate-associated FA 22:6(n−3), the amphipod T. libellula and the ctenophores B. cucumis and M. ovum had the highest dinoflagellate, i.e., pelagic signal, in their FA pool (Figure 6A and Supplementary Table 3). In previous studies, the link between the predominantly carnivorous T. libellula and sea-ice primary production varied during summer in the Bering Sea (mean contribution of ice-associated carbon between 38 and 79%, depending on stable isotope model parameters; Wang et al., 2015) and the CAO (mean contribution of ice-associated carbon between 23 and 55%, depending on stable isotope parameters; Kohlbach et al., 2016). In this study, sea ice algae-associated and pelagic/MIZ HBIs were detected in both Themisto species, showing that they utilized carbon of different origin, likely by preying on Calanus spp., based on their high proportions of copepod-associated FAs and elevated wax ester levels (Scott et al., 1999; Auel et al., 2002) and/or possibly also on krill and pteropods (Marion et al., 2008) based on the presence of HBIs in these taxa. Differences in lipid class, FA composition and HBI concentrations between T. abyssorum and T. libellula, however, reflect differences in energy storage mode and their highly diverse feeding behavior (Auel et al., 2002; Havermans et al., 2019). HBI concentrations in T. abyssorum were only analyzed for samples from the ice-covered stations P6 and P7, so that a strictly pelagic feeding style of this species in the more southern Barents Sea cannot be excluded.
Pteropods and Gelatinous Zooplankton
Based on high amounts of phytosterols and a ratio of 18:1(n−9)/18:1(n−7) < 1, the pteropod C. limacina was characterized by a herbivorous rather than carnivorous feeding strategy, challenging the idea that C. limacina is exclusively feeding on L. helicina (Conover and Lalli, 1972; Böer et al., 2005), at least during the summer period when phytoplankton should be abundant. Correspondingly, the lipid class and FA composition were distinctly different between the two pteropods (e.g., more TAGs in C. limacina, more polar lipids in L. helicina), suggesting that L. helicina was not a major food item for C. limacina in our study. The significantly higher proportions of the PUFAs 20:5(n−3) and 22:6(n−3) in L. helicina were at least partly the result of the large proportion of polar lipids in L. helicina compared to C. limacina, since these long-chain FAs are mainly incorporated into cell membranes (Stübing et al., 2003). Furthermore, L. helicina had substantially higher proportions of free FAs (average 19%) than all other taxa (mean 0.2 to 10.7%), except for C. vesicarium, which could indicate gonad maturation during summer (Gannefors et al., 2005).
Interestingly, C. limacina is assumed to be a true pelagic species, with relatively low (trophic) interaction with the sea-ice system (mean contribution of ice-associated carbon between 14 and 30% during summer in the CAO, depending on stable isotope model parameters; Kohlbach et al., 2016). Yet, in this study, C. limacina alongside six other species out of 24, including L. limacina and the appendicularian O. vanhoeffeni, were the only species containing sea ice algae-associated HBIs. In both pteropod species, sea ice algae-associated HBIs were determined in individuals collected at station P2, where sea ice was absent during the sampling period. This might reflect the great mobility of these species, able to cover large distances with varying sea-ice coverage, or a low turnover rate of HBIs in these species if it is assumed that sea ice-associated material was ingested prior to sampling when sea ice was still present at this station.
In the ctenophores B. cucumis and M. ovum, the strong pelagic signal in their FA compositions as well as the absence of sea ice algae-associated HBIs likely reflects that they fed on pelagic prey, probably copepods, but also amphipods and krill, as reported previously (Swanberg and Båmstedt, 1991). The two cnidarian species were largely different in their lipid class and FA composition with an overall stronger diatom signal in Bougainvillia superciliaris compared to Catablema vesicarium. In accordance with previous studies, both species were likely feeding as omnivores/carnivores, and phytoplankton-derived carbon contributed largely to their diets (Hansson et al., 2005; Prudkovsky and Neretina, 2016). Among the chaetognaths, E. hamata had a stronger diatom signal compared to Parasagitta/Sagitta spp., likely as a result of its higher trophic dependency on Calanus spp. (Connelly et al., 2014) or other copepods, corresponding to its higher relative proportions of wax esters and somewhat higher proportions of copepod-associated FAs, while Parasagitta and Sagitta have been found to rather rely on smaller copepods (Pseudocalanus and Oithona) (Falkenhaug, 1991; Alvarez-Cadena, 1993). In Parasagitta elegans, the presence of the diatom-derived HBI III suggests that this species also utilized food sources other than small copepods.
The failure to detect IP25 and IPSO25 in PPOM suggests that zooplankton containing HBIs received these lipid components by feeding on sea ice-derived material. This does not necessarily mean that these species were actively grazing on sea-ice biota (where sea ice was present) but rather that the animals fed on ice algae that had sunk to deeper water or the seabed, possibly as marine snow (Olli et al., 2002; Tamelander et al., 2006; Tamelander et al., 2009; Schmidt et al., 2018). In ice-covered regions, the phytoplankton community is strongly influenced by water stratification due to ice melt, while more porous ice conditions during summer could have triggered the release and vertical export of ice algal material, thus contributing to the PPOM community composition (Hegseth, 1998; Tamelander et al., 2009). Limacina helicina is described as a flux-feeder with the ability to consume fast-sinking food particles (Stukel et al., 2019), such as ice algal aggregates dominated by pennate diatoms (Assmy et al., 2013), which have been shown to contain HBI-producing diatoms albeit in low abundances (Brown et al., 2017a), providing an explanation for the highest concentrations of sea ice algae-associated HBIs in L. helicina in this study. The absence of sea ice algae-associated HBIs does not exclude the possibility of the other taxa to feed on sea ice-derived carbon. The so far identified ice-associated HBIs are specific for certain sea-ice diatoms (Belt et al., 2007; Brown et al., 2014) and these diatom species have usually a relatively low contribution to the ice algal community (Volkman et al., 1994; Brown et al., 2014). Thus, the lack of these trophic markers could simply indicate that the organisms were feeding on ice-associated algae that did not produce these HBIs.
Suitability of Trophic Marker Methods for Tracing Food-Web Relationships
By combining dietary information derived from different biochemical approaches, we gained insights on (a) differences in energy storage modes between the zooplankton taxa based on lipid class composition (wax esters versus TAGs), (b) the taxonomic composition of carbon sources in zooplankton based on FA compositions (diatoms versus dinoflagellates), (c) the origin of these carbon sources based on HBI compositions (sea ice versus water column), and (d) tendencies regarding herbivory versus carnivory based on sterol compositions, FA carnivory indices and the presence of copepod-associated FAs (Figures 6A–C). To reveal food-web relationships, FAs have been traced from producer to consumer in numerous food-web studies in both the Arctic (Falk-Petersen et al., 1987; Graeve et al., 1994; Kohlbach et al., 2016) and the Antarctic (Schmidt et al., 2006; Kohlbach et al., 2018). Recent literature, however, suggests that marine invertebrates have the potential to de novo synthesize certain PUFAs (Kabeya et al., 2018), which is somewhat contradictory to the general assumption that these FAs are exclusively produced by marine algae and thus essentially obtained as dietary components in the marine environment. Kabeya et al. (2018) reported that numerous zooplankton possess the respective genes for PUFA biosynthesis, however, actual concentrations of zooplankton-produced PUFAs and the relation of zooplankton-produced versus algal-produced PUFAs in the FA composition of a consumer are unknown and are likely to vary greatly from species to species. Since the ability of herein investigated species to biosynthesize their own PUFAs is unclear at this point, we assume that their trophic marker PUFAs were primarily obtained from dietary sources.
Compared to lipid class and FA compositions, the use of HBI and sterol lipid data in Arctic food-web studies is relatively new (e.g., Brown et al., 2018; Schmidt et al., 2018; Kohlbach et al., 2019), and likely benefits from a multidimensional application as employed here. The recent literature on HBIs and their role in Arctic marine processes (e.g., Brown and Belt, 2012; Brown et al., 2015, 2017a) strongly suggests their potential, and crucially these markers are highly stable; if a polar bear or a paleo-sediment sample contains ice marker, we can be fairly sure that some of the organic carbon was indeed originally sourced from sea-ice algae. All the trophic marker approaches have their strengths and weaknesses, and our study highlights the value of combining them into a multi-biomarker approach, whereby independent approaches provide both alternative insights and complementary information (Schmidt et al., 2018).
One key methodological issue for HBIs concerns their specificity to particular diatom species. The number of HBIs found to be produced by Arctic algae is continuously growing (Belt, 2018; Belt et al., 2019; Brown et al., 2020), but while the presence of the ice markers IP25 and IPSO25 is believed to provide unequivocal sourcing from ice algae, their absence is not so straightforward to interpret (Belt, 2018). Also, species-specific turnover rates of HBIs in the consumers are still unknown. It might be assumed, however, that turnover times of these lipids lengthen with increasing trophic level (c.f. FAs), reaching up to several weeks in polar zooplankton (Boissonnot et al., 2016, 2019; Graeve et al., 2020). The failure to detect pelagic/MIZ HBIs in PPOM suggests that either HBI-producing algae were not present at the depth of the Chl a maximum, where the PPOM samples were collected, or that HBI concentrations were below the detection limit, both of which might be related to the seasonality of some HBI production. The presence of pelagic/MIZ HBIs in most of the zooplankton species could reflect the animals’ vertical mobility (e.g., Fortier et al., 2001) to encounter differing algal assemblages consisting of different algal taxa throughout the water column.
Even though phytosterols can potentially be metabolically changed by herbivorous consumers (Ruess and Müller-Navarra, 2019), they can support biomarker studies by informing about plant-derived food intake in comparison to a rather animal-based diet (Nichols et al., 1993). In this study, PPOM and zooplankton clustered according to their sterol composition, with the overall pattern being robust across taxa and sampling locations (Supplementary Figure 2). Moreover, we found an internal consistency and strong positive correlation between the animals’ HBI content and phytosterols (Figure 5C), further suggesting the suitability of phytosterols as an indicator for algal ingestion. The most abundant phytosterols found in Arctic sea ice are brassicasterol, chalinasterol, and sitosterol (Belt et al., 2013), and brassicasterol is also produced by pelagic algae (Müller et al., 2011). However, cholesterol can also be abundant in algal assemblages (Brown et al., 2011; Belt et al., 2018), offering an explanation for the presence of zoosterols in, e.g., C. limacina and O. vanhoeffeni that indicated the overall lowest degree of carnivory in this study. Sterols have been found to be predominantly produced by, but not restricted to diatoms (Volkman et al., 1998; Rampen et al., 2010). The importance of diatoms in both sea-ice and pelagic protist communities was apparent from microscopic analysis and their FA compositions, suggesting that algae from both the sea ice and the pelagic habitat could have contributed to the overall sterol content in the zooplankton.
Conclusion
Tracing FAs, stable isotopes and/or HBIs, previous studies have indicated the role of sea ice-derived organic matter for Arctic ice-associated invertebrates (Tamelander et al., 2006; Kohlbach et al., 2016), pelagic animals in Arctic (Budge et al., 2008; Tamelander et al., 2008; Wang et al., 2015) and Antarctic marine environments (Schmidt et al., 2018), and Arctic benthic consumers (Brown and Belt, 2012; Kohlbach et al., 2019) across multiple trophic levels during spring and summer. In the present study, we found sea ice algae-specific metabolites (i.e., HBIs IP25 and IPSO25) in filter-feeders of the mid-water column (pteropods, appendicularians) and in mobile omnivorous crustaceans (krill, pelagic amphipods), extending present knowledge about the role of ice algae as a food source for pelagic consumers in the Barents Sea during summer. Despite the ‘heavy’ sea-ice conditions in the Barents Sea during summer 2019 compared to previous years, the investigated pelagic food web showed a taxon-specific but relatively weak trophic relation to sea ice-derived carbon. During the time of the sampling, most of the sea-ice algae had already sloughed off and the remaining ice algae material seemed decayed. This corresponds to the lower PUFA levels in IPOM (average 16%) compared to PPOM (average 32%). Thus, not surprisingly, ice algae were providing a less abundant and attractive carbon source, in terms of lower food quality, than food of pelagic origin for the zooplankton grazers during the late summer melting season.
Until now, little information is available about direct consequences of declining sea ice on food-web interactions in the Arctic (e.g., Wassmann et al., 2011; Stige et al., 2019; Dalpadado et al., 2020). Overall, ice-associated species are expected to be more susceptible to sea-ice decline than pelagic ones (Dalpadado et al., 2020) and might be forced to a stronger association with the pelagic environment (Hop and Gjøsæter, 2013), with consequences for associated pelagic and benthic food webs and trophic interactions (Michalsen et al., 2013; Blanchard, 2015). With the change in primary production, i.e., the composition and availability of carbon sources, species with opportunistic feeding behavior, such as O. similis and Pseudocalanus spp. (Castellani et al., 2005; Lischka and Hagen, 2007; Cornwell et al., 2020) might further have a higher tolerance to more variable environmental conditions and an advantage over highly specialized (ice-associated) species.
Due to great uncertainties of consequences for individual species, it is crucial to continuously monitor and assess climate vulnerability on a species level during the transition to a warmer Arctic Ocean to reliably inform about changes in food-web structure and functioning (Eamer et al., 2013; Poloczanska, 2020). Ultimately, the understanding of present food-web processes through the seasons will be the requisite for meaningful projections of winners and losers in the future Barents Sea with less sea ice and changing sea-ice properties.
Data Availability Statement
The raw data supporting the conclusions of this article will be made available by the authors, without undue reservation.
Author Contributions
PA, HH, and DK were responsible for study design. AW conducted sampling. HH and PA provided sampling logistics. DK is the main author of this manuscript and did the fatty acid laboratory analyses and data evaluation. MW carried out lipid class analysis with support of MG. KS did the HBI and sterol analysis and data evaluation with the help of LS, SB, and AA. Taxonomic analysis of POM samples was carried out by AD and AT. MG and SB provided laboratory materials, methodological expertise, and laboratory space. The map was created by AKA-H. Data analyses and figure assemblage was done by DK with the help of the other authors. All authors contributed significantly to data interpretation and to the writing of the manuscript.
Funding
This work was funded by the Research Council of Norway through the project The Nansen Legacy (RCN # 276730). Contributions by KS, SB, and AA were funded by the UK’s Natural Environment Research Council MOSAiC-Thematic project SYM-PEL: “Quantifying the contribution of sympagic versus pelagic diatoms to Arctic food webs and biogeochemical fluxes: application of source-specific highly branched isoprenoid biomarkers” (NE/S002502/1).
Conflict of Interest
The authors declare that the research was conducted in the absence of any commercial or financial relationships that could be construed as a potential conflict of interest.
Acknowledgments
We thank the captain and the crew of RV Kronprins Haakon for their excellent support at sea during the Nansen Legacy seasonal cruise Q3. We also thank Kasia Dmoch for her help with sampling. We thank Sinah Müller, Valeria Adrian (AWI), and Angela Stippkugel (NTNU) for their help with the lipid laboratory analyses and Józef Maria Wiktor (IOPAN) for supervising the analysis of the algal taxonomy samples.
Supplementary Material
The Supplementary Material for this article can be found online at: https://www.frontiersin.org/articles/10.3389/fmars.2020.610248/full#supplementary-material
References
Aarflot, J. M., Skjoldal, H. R., Dalpadado, P., and Skern-Mauritzen, M. (2018). Contribution of Calanus species to the mesozooplankton biomass in the Barents Sea. ICES J. Mar. Sci. 75, 2342–2354. doi: 10.1093/icesjms/fsx221
Alvarez-Cadena, J. N. (1993). Feeding of the chaetognath Sagitta elegans Verrill. Estuar. Coast. Shelf Sci. 36, 195–206. doi: 10.1006/ecss.1993.1013
Ardyna, M., Gosselin, M., Michel, C., Poulin, M., and Tremblay, J. -É (2011). Environmental forcing of phytoplankton community structure and function in the Canadian High Arctic: contrasting oligotrophic and eutrophic regions. Mar. Ecol. Prog. Ser. 442, 37–57. doi: 10.3354/meps09378
Arneberg, P., van der Meeren, G. I., Frantzen, S., and Vee, I. (red.). (2020). “Status of the environment of the Barents Sea- Report from Monitoring group 2020”. Fisken og Havet 2020-13. Bergen: Institute of Marine Research.
Arrigo, K. R., and van Dijken, G. L. (2015). Continued increases in Arctic Ocean primary production. Prog. Oceanogr. 136, 60–70. doi: 10.1016/j.pocean.2015.05.002
Årthun, M., Eldevik, T., Smedsrud, L. H., Skagseth, Ø, and Ingvaldsen, R. B. (2012). Quantifying the influence of Atlantic heat on Barents Sea ice variability and retreat. J. Clim. 25, 4736–4743. doi: 10.1175/JCLI-D-11-00466.1
Assmy, P., Ehn, J. K., Fernández-Méndez, M., Hop, H., Katlein, C., Sundfjord, A., et al. (2013). Floating ice-algal aggregates below melting Arctic sea ice. PLoS One 8:e76599. doi: 10.1371/journal.pone.0076599
Atkinson, A. (1996). Subantarctic copepods in an oceanic, low chlorophyll environment: ciliate predation, food selectivity and impact on prey populations. Mar. Ecol. Prog. Ser. 130, 85–96. doi: 10.3354/meps130085
Aubert, A., Antajan, E., Lynam, C., Pitois, S., Pliru, A., Vaz, S., et al. (2018). No more reason for ignoring gelatinous zooplankton in ecosystem assessment and marine management: concrete cost-effective methodology during routine fishery trawl surveys. Mar. Policy 89, 100–108. doi: 10.1016/j.marpol.2017.12.010
Auel, H., Harjes, M., da Rocha, R., Stübing, D., and Hagen, W. (2002). Lipid biomarkers indicate different ecological niches and trophic relationships of the Arctic hyperiid amphipods Themisto abyssorum and T. libellula. Polar Biol. 25, 374–383. doi: 10.1007/s00300-001-0354-7
Belt, S. T. (2018). Source-specific biomarkers as proxies for Arctic and Antarctic sea ice. Org. Geochem. 125, 277–298. doi: 10.1016/j.orggeochem.2018.10.002
Belt, S. T., Brown, T. A., Ringrose, A. E., Cabedo-Sanz, P., Mundy, C. J., Gosselin, M., et al. (2013). Quantitative measurement of the sea ice diatom biomarker IP25 and sterols in Arctic sea ice and underlying sediments: Further considerations for palaeo sea ice reconstruction. Org. Geochem. 62, 33–45. doi: 10.1016/j.orggeochem.2013.07.002
Belt, S. T., Brown, T. A., Sanz, P. C., and Rodriguez, A. N. (2012). Structural confirmation of the sea ice biomarker IP25 found in Arctic marine sediments. Environ. Chem. Lett. 10, 189–192. doi: 10.1007/s10311-011-0344-0
Belt, S. T., Brown, T. A., Smik, L., Assmy, P., and Mundy, C. J. (2018). Sterol identification in floating Arctic sea ice algal aggregates and the Antarctic sea ice diatom Berkeleya adeliensis. Org. Geochem. 118, 1–3. doi: 10.1016/j.orggeochem.2018.01.008
Belt, S. T., Massé, G., Rowland, S. J., Poulin, M., Michel, C., and LeBlanc, B. (2007). A novel chemical fossil of palaeo sea ice: IP25. Org. Geochem. 38, 16–27. doi: 10.1016/j.orggeochem.2006.09.013
Belt, S. T., and Müller, J. (2013). The Arctic sea ice biomarker IP25: a review of current understanding, recommendations for future research and applications in palaeo sea ice reconstructions. Quat. Sci. Rev. 79, 9–25. doi: 10.1016/j.quascirev.2012.12.001
Belt, S. T., Smik, L., Brown, T. A., Kim, J.-H., Rowland, S. J., Allen, C. S., et al. (2016). Source identification and distribution reveals the potential of the geochemical Antarctic sea ice proxy IPSO25. Nat. Commun. 7:12655. doi: 10.1038/ncomms12655
Belt, S. T., Smik, L., Köseoǧlu, D., Knies, J., and Husum, K. (2019). A novel biomarker-based proxy for the spring phytoplankton bloom in Arctic and sub-arctic settings–HBI T25. Earth Planet. Sci. Lett. 523:115703. doi: 10.1016/j.epsl.2019.06.038
Blanchard, J. (2015). Climate change: a rewired food web. Nature 527, 173–174. doi: 10.1038/nature16311
Böer, M., Gannefors, C., Kattner, G., Graeve, M., Hop, H., and Falk-Petersen, S. (2005). The Arctic pteropod Clione limacina: seasonal lipid dynamics and life-strategy. Mar. Biol. 147, 707–717. doi: 10.1007/s00227-005-1607-8
Boissonnot, L., Niehoff, B., Ehrenfels, B., Søreide, J. E., Hagen, W., and Graeve, M. (2019). Lipid and fatty acid turnover of the pteropods Limacina helicina, L. retroversa and Clione limacina from Svalbard waters. Mar. Ecol. Prog. Ser. 609, 133–149. doi: 10.3354/meps12837
Boissonnot, L., Niehoff, B., Hagen, W., Søreide, J. E., and Graeve, M. (2016). Lipid turnover reflects life-cycle strategies of small-sized Arctic copepods. J. Plankton Res. 38, 1420–1432. doi: 10.1093/plankt/fbw076
Brown, T. A., Assmy, P., Hop, H., Wold, A., and Belt, S. T. (2017a). Transfer of ice algae carbon to ice-associated amphipods in the high-Arctic pack ice environment. J. Plankton Res. 39, 664–674. doi: 10.1093/plankt/fbx030
Brown, T. A., and Belt, S. T. (2012). Identification of the sea ice diatom biomarker IP25 in Arctic benthic macrofauna: direct evidence for a sea ice diatom diet in Arctic heterotrophs. Polar Biol. 35, 131–137. doi: 10.1007/s00300-011-1045-7
Brown, T. A., Belt, S. T., Philippe, B., Mundy, C. J., Massé, G., Poulin, M., et al. (2011). Temporal and vertical variations of lipid biomarkers during a bottom ice diatom bloom in the Canadian Beaufort Sea: further evidence for the use of the IP25 biomarker as a proxy for spring Arctic sea ice. Polar Biol. 34, 1857–1868. doi: 10.1007/s00300-010-0942-5
Brown, T. A., Belt, S. T., Tatarek, A., and Mundy, C. J. (2014). Source identification of the Arctic sea ice proxy IP25. Nat. Commun. 5:4197. doi: 10.1038/ncomms5197
Brown, T. A., Chrystal, E., Ferguson, S. H., Yurkowski, D. J., Watt, C., Hussey, N. E., et al. (2017b). Coupled changes between the H-Print biomarker and δ15N indicates a variable sea ice carbon contribution to the diet of Cumberland Sound beluga whales. Limnol. Oceanogr. 62, 1606–1619. doi: 10.1002/lno.10520
Brown, T. A., Galicia, M. P., Thiemann, G. W., Belt, S. T., Yurkowski, D. J., and Dyck, M. G. (2018). High contributions of sea ice derived carbon in polar bear (Ursus maritimus) tissue. PLoS One 13:e0191631. doi: 10.1371/journal.pone.0191631
Brown, T. A., Hegseth, E. N., and Belt, S. T. (2015). A biomarker-based investigation of the mid-winter ecosystem in Rijpfjorden, Svalbard. Polar Biol. 38, 37–50. doi: 10.1007/s00300-013-1352-2
Brown, T. A., Rad-Menéndez, C., Ray, J. L., Skaar, K. S., Thomas, N., Ruiz-Gonzalez, C., et al. (2020). Influence of nutrient availability on Arctic sea ice diatom HBI lipid synthesis. Org. Geochem. 141, 103977. doi: 10.1016/j.orggeochem.2020.103977
Budge, S. M., Wooller, M. J., Springer, A. M., Iverson, S. J., McRoy, C. P., and Divoky, G. J. (2008). Tracing carbon flow in an arctic marine food web using fatty acid-stable isotope analysis. Oecologia 157, 117–129. doi: 10.1007/s00442-008-1053-7
Campbell, R. G., Sherr, E. B., Ashjian, C. J., Plourde, S., Sherr, B. F., Hill, V., et al. (2009). Mesozooplankton prey preference and grazing impact in the western Arctic Ocean. Deep Sea Res. II Top. Stud. Oceanogr. 56, 1274–1289. doi: 10.1016/j.dsr2.2008.10.027
Castellani, C., Irigoien, X., Harris, R. P., and Lampitt, R. S. (2005). Feeding and egg production of Oithona similis in the North Atlantic. Mar. Ecol. Prog. Ser. 288, 173–182. doi: 10.3354/meps288173
Cleary, A. C., Durbin, E. G., Rynearson, T. A., and Bailey, J. (2016). Feeding by Pseudocalanus copepods in the Bering Sea: trophic linkages and a potential mechanism of niche partitioning. Deep Sea Res. II Top. Stud. Oceanogr. 134, 181–189. doi: 10.1016/j.dsr2.2015.04.001
Cleary, A. C., Søreide, J. E., Freese, D., Niehoff, B., and Gabrielsen, T. M. (2017). Feeding by Calanus glacialis in a high arctic fjord: potential seasonal importance of alternative prey. ICES J. Mar. Sci. 74, 1937–1946. doi: 10.1093/icesjms/fsx106
Condon, R. H., Graham, W. M., Duarte, C. M., Pitt, K. A., Lucas, C. H., Haddock, S. H. D., et al. (2012). Questioning the rise of gelatinous zooplankton in the world’s oceans. BioScience 62, 160–169. doi: 10.1525/bio.2012.62.2.9
Connelly, T. L., Deibel, D., and Parrish, C. C. (2014). Trophic interactions in the benthic boundary layer of the Beaufort Sea shelf, Arctic Ocean: combining bulk stable isotope and fatty acid signatures. Prog. Oceanogr. 120, 79–92. doi: 10.1016/j.pocean.2013.07.032
Conover, R. J., Herman, A. W., Prinsenberg, S. J., and Harris, L. R. (1986). Distribution of and feeding by the copepod Pseudocalanus under fast ice during the Arctic spring. Science 232, 1245–1247. doi: 10.1126/science.232.4755.1245
Conover, R. J., and Lalli, C. M. (1972). Feeding and growth in Clione limacina (Phipps), a pteropod mollusc. J. Exp. Mar. Biol. 9, 279–302. doi: 10.1016/0022-0981(72)90038-X
Cornwell, L. E., Fileman, E. S., Bruun, J. T., Hirst, A. G., Tarran, G. A., Findlay, H. S., et al. (2020). Resilience of the copepod Oithona similis to climatic variability: Egg Production, mortality, and vertical habitat partitioning. Front. Mar. Sci. 7:29. doi: 10.3389/fmars.2020.00029
Dalpadado, P., Arrigo, K. R., van Dijken, G. L., Skjoldal, H. R., Bagøien, E., Dolgov, A. V., et al. (2020). Climate effects on temporal and spatial dynamics of phytoplankton and zooplankton in the Barents Sea. Prog. Oceanogr. 185:102320. doi: 10.1016/j.pocean.2020.102320
Dalpadado, P., Ingvaldsen, R. B., and Hassel, A. (2003). Zooplankton biomass variation in relation to climatic conditions in the Barents Sea. Polar Biol. 26, 233–241. doi: 10.1007/s00300-002-0470-z
Dalsgaard, J., St John, M., Kattner, G., Müller-Navarra, D., and Hagen, W. (2003). Fatty acid trophic markers in the pelagic marine environment. Adv. Mar. Biol. 46, 227–237. doi: 10.1016/S0065-2881(03)46005-7
Dong, K., Kvile, K. Ø, Stenseth, N. C., and Stige, L. C. (2020). Associations among temperature, sea ice and phytoplankton bloom dynamics in the Barents Sea. Mar. Ecol. Prog. Ser. 635, 25–36. doi: 10.3354/meps13218
Drazen, J. C., Phleger, C. F., Guest, M. A., and Nichols, P. D. (2008). Lipid, sterols and fatty acid composition of abyssal holothurians and ophiuroids from the North-East Pacific Ocean: food web implications. Comp. Biochem. Physiol. B Biochem. Molec. Biol. 151, 79–87. doi: 10.1016/j.cbpb.2008.05.013
Eamer, J., Donaldson, G. M., Gaston, A. J., Kosobokova, K. N., Lárusson, K. F., Melnikov, I. A., et al. (2013). Life Linked to Ice. A guide to Sea-ice-Associated Biodiversity in this Time of Rapid Change. CAFF Assessment Series No. 10. Iceland: Conservation of Arctic Flora and Fauna.
Ellingsen, I. H., Dalpadado, P., Slagstad, D., and Loeng, H. (2008). Impact of climatic change on the biological production in the Barents Sea. Clim. Change 87, 155–175. doi: 10.1007/s10584-007-9369-6
Engelsen, O., Hegseth, E. N., Hop, H., Hansen, E., and Falk-Petersen, S. (2002). Spatial variability of chlorophyll-a in the Marginal Ice Zone of the Barents Sea, with relations to sea ice and oceanographic conditions. J. Mar. Syst. 35, 79–97. doi: 10.1016/S0924-7963(02)00077-5
Eriksen, E., Bogstad, B., Dolgov, A., and Beck, I. M. (2018a). Cod diet as an indicator of Ctenophora abundance dynamics in the Barents Sea. Mar. Ecol. Prog. Ser. 591, 87–100. doi: 10.3354/meps12199
Eriksen, E., Gjøsæter, H., Prozorkevich, D., Shamray, E., Dolgov, A., Skern-Mauritzen, M., et al. (2018b). From single species surveys towards monitoring of the Barents Sea ecosystem. Prog. Oceanogr. 166, 4–14. doi: 10.1016/j.pocean.2017.09.007
Eriksen, E., Skjoldal, H. R., Gjøsæter, H., and Primicerio, R. (2017). Spatial and temporal changes in the Barents Sea pelagic compartment during the recent warming. Prog. Oceanogr. 151, 206–226. doi: 10.1016/j.pocean.2016.12.009
Falkenhaug, T. (1991). Prey composition and feeding rate of Sagitta elegans var. arctica (Chaetognatha) in the Barents Sea in early summer. Polar Res. 10, 487–506. doi: 10.3402/polar.v10i2.6761
Falk-Petersen, S., Hagen, W., Kattner, G., Clarke, A., and Sargent, J. (2000). Lipids, trophic relationships, and biodiversity in Arctic and Antarctic krill. Can. J. Fish. Aquat. Sci. 57, 178–191. doi: 10.1139/f00-194
Falk-Petersen, S., Hopkins, C. C. E., and Sargent, J. R. (1990). “Trophic relationships in the pelagic, Arctic food web,” in Trophic Relationships in the Marine Environment, eds M. Barnes and R. N. Gibson (Aberdeen: Aberdeen University Press), 315–333.
Falk-Petersen, S., Pedersen, G., Kwasniewski, S., Hegseth, E. N., and Hop, H. (1999). Spatial distribution and life-cycle timing of zooplankton in the marginal ice zone of the Barents Sea during the summer melt season in 1995. J. Plankton Res. 21, 1249–1264. doi: 10.1093/plankt/21.7.1249
Falk-Petersen, S., Sargent, J. R., Henderson, J., Hegseth, E. N., Hop, H., and Okolodkov, Y. B. (1998). Lipids and fatty acids in ice algae and phytoplankton from the Marginal Ice Zone in the Barents Sea. Polar Biol. 20, 41–47. doi: 10.1007/s003000050274
Falk-Petersen, S., Sargent, J. R., and Tande, K. S. (1987). Lipid composition of zooplankton in relation to the sub-Arctic food web. Polar Biol. 8, 115–120. doi: 10.1007/BF00297065
Fernández Méndez, M. (2014). Primary Productivity in Arctic Sea Ice and Ocean. Ph.D. thesis, University of Bremen, Bremen.
Fernández-Méndez, M., Katlein, C., Rabe, B., Nicolaus, M., Peeken, I., Bakker, K., et al. (2015). Photosynthetic production in the central Arctic Ocean during the record sea-ice minimum in 2012. Biogeosciences 12, 3525–3549. doi: 10.5194/bg-12-3525-2015
Fetterer, F., Knowles, K., Meier, W. N., Savoie, M., and Windnagel, A. K. (2017). Updated Daily. Sea Ice Index, Version 3. [G02135]. Boulder, CO: NSIDC.
Folch, J., Lees, M., and Stanley, G. H. S. (1957). A simple method for the isolation and purification of total lipides from animal tissues. J. Biol. Chem. 226, 497–509.
Fortier, M., Fortier, L., Hattori, H., Saito, H., and Legendre, L. (2001). Visual predators and the diel vertical migration of copepods under Arctic sea ice during the midnight sun. J. Plankton Res. 23, 1263–1278. doi: 10.1093/plankt/23.11.1263
Fuglestad, J. L., Benestad, R., Ivanov, V., Jørgensen, L. L., Kovacs, K. M., Nilssen, F., et al. (2020). “Ecosystems of the Barents Sea Region,” in Governing Arctic Seas: Regional Lessons from the Bering Strait and Barents Sea, eds O. R. Young, P. A. Berkman, and A. N. Vylegzhanin (Berlin: Springer), 119–142. doi: 10.1007/978-3-030-25674-6_6
Gannefors, C., Böer, M., Kattner, G., Graeve, M., Eiane, K., Gulliksen, B., et al. (2005). The Arctic sea butterfly Limacina helicina: lipids and life strategy. Mar. Biol. 147, 169–177. doi: 10.1007/s00227-004-1544-y
Garrison, D. L., and Buck, K. R. (1986). Organism losses during ice melting: a serious bias in sea ice community studies. Polar Biol. 6, 237–239. doi: 10.1007/BF00443401
Gluchowska, M., Dalpadado, P., Beszczynska-Möller, A., Olszewska, A., Ingvaldsen, R. B., and Kwasniewski, S. (2017). Interannual zooplankton variability in the main pathways of the Atlantic water flow into the Arctic Ocean (Fram Strait and Barents Sea branches). ICES J. Mar. Sci. 74, 1921–1936. doi: 10.1093/icesjms/fsx033
Graeve, M., Boissonnot, L., Niehoff, B., Hagen, W., and Kattner, G. (2020). Assimilation and turnover rates of lipid compounds in dominant Antarctic copepods fed with 13C-enriched diatoms. Philos. Trans. R. Soc. B 375:20190647. doi: 10.1098/rstb.2019.0647
Graeve, M., and Janssen, D. (2009). Improved separation and quantification of neutral and polar lipid classes by HPLC–ELSD using a monolithic silica phase: application to exceptional marine lipids. J. Chromatogr. B 877, 1815–1819. doi: 10.1016/j.jchromb.2009.05.004
Graeve, M., Kattner, G., and Hagen, W. (1994). Diet-induced changes in the fatty acid composition of Arctic herbivorous copepods: experimental evidence of trophic markers. J. Exp. Mar. Biol. Ecol. 182, 97–110. doi: 10.1016/0022-0981(94)90213-5
Graeve, M., Kattner, G., and Piepenburg, D. (1997). Lipids in Arctic benthos: does the fatty acid and alcohol composition reflect feeding and trophic interactions? Polar Biol. 18, 53–61. doi: 10.1007/s003000050158
Greenacre, M., and Primicerio, R. (2014). Multivariate Analysis of Ecological Data. Bilbao: Fundacion BBVA.
Hansen, B., Verity, P., Falkenhaug, T., Tande, K. S., and Norrbin, F. (1994). On the trophic fate of Phaeocystis pouchetti (Harriot). V. Trophic relationships between Phaeocystis and zooplankton: an assessment of methods and size dependence. J. Plankton Res. 16, 487–511. doi: 10.1093/plankt/16.5.487
Hansson, L. J., Moeslund, O., Kiørboe, T., and Riisgård, H. U. (2005). Clearance rates of jellyfish and their potential predation impact on zooplankton and fish larvae in a neritic ecosystem (Limfjorden, Denmark). Mar. Ecol. Prog. Ser. 304, 117–131. doi: 10.3354/meps304117
Hardge, K., Peeken, I., Neuhaus, S., Lange, B. A., Stock, A., Stoeck, T., et al. (2017). The importance of sea ice for exchange of habitat-specific protist communities in the Central Arctic Ocean. J. Mar. Syst. 165, 124–138. doi: 10.1016/j.jmarsys.2016.10.004
Haug, T., Falk-Petersen, S., Greenacre, M., Hop, H., Lindstrøm, U., Meier, S., et al. (2017). Trophic level and fatty acids in harp seals compared with common minke whales in the Barents Sea. Mar. Biol. Res. 13, 919–932. doi: 10.1080/17451000.2017.1313988
Havermans, C., Auel, H., Hagen, W., Held, C., Ensor, N. S., and Tarling, G. A. (2019). Predatory zooplankton on the move: Themisto amphipods in high-latitude marine pelagic food webs. Adv. Mar. Biol. 82, 51–92. doi: 10.1016/bs.amb.2019.02.002
Hegseth, E. N. (1998). Primary production of the northern Barents Sea. Polar Res. 17, 113–123. doi: 10.1111/j.1751-8369.1998.tb00266.x
Henderson, R. J., Hegseth, E. N., and Park, M. T. (1998). Seasonal variation in lipid and fatty acid composition of ice algae from the Barents Sea. Polar Biol. 20, 48–55. doi: 10.1007/s003000050275
Hirche, H.-J. (1997). Life cycle of the copepod Calanus hyperboreus in the Greenland Sea. Mar. Biol. 128, 607–618. doi: 10.1007/s002270050127
Hop, H., and Gjøsæter, H. (2013). Polar cod (Boreogadus saida) and capelin (Mallotus villosus) as key species in marine food webs of the Arctic and the Barents Sea. Mar. Biol. Res. 9, 878–894. doi: 10.1080/17451000.2013.775458
Hop, H., Poltermann, M., Lønne, O. J., Falk-Petersen, S., Korsnes, R., and Budgell, W. P. (2000). Ice amphipod distribution relative to ice density and under-ice topography in the northern Barents Sea. Polar Biol. 23, 357–367. doi: 10.1007/s003000050456
Hunt, G. L. Jr., Blanchard, A. L., Boveng, P., Dalpadado, P., Drinkwater, K. F., Eisner, L., et al. (2013). The Barents and Chukchi Seas: comparison of two Arctic shelf ecosystems. J. Mar. Syst. 109, 43–68. doi: 10.1016/j.jmarsys.2012.08.003
Jaspers, C., Acuña, J. L., and Brodeur, R. D. (2015). Interactions of gelatinous zooplankton within marine food webs. J. Plankton Res. 37, 985–988. doi: 10.1093/plankt/fbv068
Johannesen, E., Ingvaldsen, R. B., Bogstad, B., Dalpadado, P., Eriksen, E., Gjøsæter, H., et al. (2012). Changes in Barents Sea ecosystem state, 1970–2009: climate fluctuations, human impact, and trophic interactions. ICES J. Mar. Sci. 69, 880–889. doi: 10.1093/icesjms/fss046
Kabeya, N., Fonseca, M. M., Ferrier, D. E. K., Navarro, J. C., Bay, L. K., Francis, D. S., et al. (2018). Genes for de novo biosynthesis of omega-3 polyunsaturated fatty acids are widespread in animals. Sci. Adv. 4:eaar6849. doi: 10.1126/sciadv.aar6849
Kohlbach, D., Ferguson, S. H., Brown, T. A., and Michel, C. (2019). Landfast sea ice-benthic coupling during spring and potential impacts of system changes on food web dynamics in Eclipse Sound, Canadian Arctic. Mar. Ecol. Prog. Ser. 627, 33–48. doi: 10.3354/meps13071
Kohlbach, D., Graeve, M., Lange, B. A., David, C., Peeken, I., and Flores, H. (2016). The importance of ice algae-produced carbon in the central Arctic Ocean ecosystem: Food web relationships revealed by lipid and stable isotope analyses. Limnol. Oceanogr. 61, 2027–2044. doi: 10.1002/lno.10351
Kohlbach, D., Graeve, M., Lange, B. A., David, C., Schaafsma, F. L., van Franeker, J. A., et al. (2018). Dependency of Antarctic zooplankton species on ice algae-produced carbon suggests a sea ice-driven pelagic ecosystem during winter. Glob. Change Biol. 24, 4667–4681. doi: 10.1111/gcb.14392
Kohlbach, D., Lange, B. A., Schaafsma, F. L., David, C., Vortkamp, M., Graeve, M., et al. (2017a). Ice algae-produced carbon is critical for overwintering of Antarctic krill Euphausia superba. Front. Mar. Sci. 4:310. doi: 10.3389/fmars.2017.00310
Kohlbach, D., Schaafsma, F. L., Graeve, M., Lebreton, B., Lange, B. A., David, C., et al. (2017b). Strong linkage of polar cod (Boreogadus saida) to sea ice algae-produced carbon: evidence from stomach content, fatty acid and stable isotope analyses. Prog. Oceanogr. 152, 62–74. doi: 10.1016/j.pocean.2017.02.003
Kubiszyn, A. M., and Wiktor, J. M. (2016). The Gymnodinium and Gyrodinium (Dinoflagellata: Gymnodiniaceae) of the West Spitsbergen waters (1999–2010): biodiversity and morphological description of unidentified species. Polar Biol. 39, 1739–1747. doi: 10.1007/s00300-015-1764-2
Kvamsdal, S. F., Sandal, L. K., and Poudel, D. (2020). Ecosystem wealth in the Barents Sea. Ecol. Econ. 171:106602. doi: 10.1016/j.ecolecon.2020.106602
Kwasniewski, S., Hop, H., Falk-Petersen, S., and Pedersen, G. (2003). Distribution of Calanus species in Kongsfjorden, a glacial fjord in Svalbard. J. Plankton Res. 25, 1–20. doi: 10.1093/plankt/25.1.1
Leu, E., Brown, T. A., Graeve, M., Wiktor, J. M., Hoppe, C. J., Chierici, M., et al. (2020). Spatial and temporal variability of ice algal trophic markers—with recommendations about their application. J. Mar. Sci. Eng. 8:676. doi: 10.3390/jmse8090676
Lind, S., Ingvaldsen, R. B., and Furevik, T. (2018). Arctic warming hotspot in the northern Barents Sea linked to declining sea-ice import. Nat. Clim. Change 8, 634–639. doi: 10.1038/s41558-018-0205-y
Lischka, S., and Hagen, W. (2007). Seasonal lipid dynamics of the copepods Pseudocalanus minutus (Calanoida) and Oithona similis (Cyclopoida) in the Arctic Kongsfjorden (Svalbard). Mar. Biol. 150, 443–454. doi: 10.1007/s00227-006-0359-4
Lone, K., Hamilton, C. D., Aars, J., Lydersen, C., and Kovacs, K. M. (2019). Summer habitat selection by ringed seals (Pusa hispida) in the drifting sea ice of the northern Barents Sea. Polar Res. 38:3483. doi: 10.33265/polar.v38.3483
Long, Z., and Perrie, W. (2017). Changes in ocean temperature in the Barents Sea in the twenty-first century. J. Clim. 30, 5901–5921. doi: 10.1175/JCLI-D-16-0415.1
Marion, A., Harvey, M., Chabot, D., and Brêthes, J.-C. (2008). Feeding ecology and predation impact of the recently established amphipod, Themisto libellula, in the St. Lawrence marine system, Canada. Mar. Ecol. Prog. Ser. 373, 53–70. doi: 10.3354/meps07716
Michalsen, K., Dalpadado, P., Eriksen, E., Gjøsæter, H., Ingvaldsen, R. B., Johannesen, E., et al. (2013). Marine living resources of the Barents Sea–Ecosystem understanding and monitoring in a climate change perspective. Mar. Biol. Res. 9, 932–947. doi: 10.1080/17451000.2013.775459
Müller, J., Wagner, A., Fahl, K., Stein, R., Prange, M., and Lohmann, G. (2011). Towards quantitative sea ice reconstructions in the northern North Atlantic: a combined biomarker and numerical modelling approach. Earth Planet. Sci. Lett. 306, 137–148. doi: 10.1016/j.epsl.2011.04.011
Nichols, D. S., Nichols, P. D., and Sullivan, C. W. (1993). Fatty acid, sterol and hydrocarbon composition of Antarctic sea ice diatom communities during the spring bloom in McMurdo Sound. Antarct. Sci. 5, 271–278. doi: 10.1017/S0954102093000367
Norrbin, M. F., Olsen, R.-E., and Tande, K. S. (1990). Seasonal variation in lipid class and fatty acid composition of two small copepods in Balsfjorden, northern Norway. Mar. Biol. 105, 205–211. doi: 10.1007/BF01344288
Oksanen, J., Blanchet, G. F., Friendly, M., Kindt, R., Legendre, P., McGlinn, D., et al. (2019). vegan: Community Ecology Package. R Package Version 2.5-4. Available online at: https://CRAN.R-project.org/package=vegan doi: 10.1007/bf01344288 (accessed August 10, 2020).
Olli, K., Riser, C. W., Wassmann, P., Ratkova, T., Arashkevich, E., and Pasternak, A. (2002). Seasonal variation in vertical flux of biogenic matter in the marginal ice zone and the central Barents Sea. J. Mar. Syst. 38, 189–204. doi: 10.1016/S0924-7963(02)00177-X
Onarheim, I. H., and Årthun, M. (2017). Toward an ice-free Barents Sea. Geophys. Res. Lett. 44, 8387–8395. doi: 10.1002/2017GL074304
Planque, B., Primicerio, R., Michalsen, K., Aschan, M., Certain, G., Dalpadado, P., et al. (2014). Who eats whom in the Barents Sea: a food web topology from plankton to whales. Ecology 95, 1430–1430. doi: 10.1890/13-1062.1
Poloczanska, E. (2020). The IPCC Special Report on Ocean and Cryosphere in a Changing Climate-a View from the Mountain Tops to the Deepest Depths. San Diego, CA: Earth and Space Science Open Archive. doi: 10.1002/essoar.10502454.1
Poltermann, M. (2001). Arctic sea ice as feeding ground for amphipods–food sources and strategies. Polar Biol. 24, 89–96. doi: 10.1007/s003000000177
Prozorkevich, D., and Sunnanå, K. (2017). Survey Report from the Joint Norwegian/Russian Ecosystem Survey in the Barents Sea and Adjacent Waters, August-October 2016. IMR/PINRO Joint Report Series, No. 2/2017. Bergen: Havforskningsinstituttet.
Prudkovsky, A. A., and Neretina, T. V. (2016). The life cycle of Catablema vesicarium (A. Agassiz, 1862)(Hydrozoa, Pandeidae). Polar Biol. 39, 533–542. doi: 10.1007/s00300-015-1805-x
R Core Team (2017). R: A Language and Environment for Statistical Computing. Vienna: R Foundation for Statistical Computing.
Rampen, S. W., Abbas, B. A., Schouten, S., and Sinninghe Damste, J. S. (2010). A comprehensive study of sterols in marine diatoms (Bacillariophyta): implications for their use as tracers for diatom productivity. Limnol. Oceanogr. 55, 91–105. doi: 10.4319/lo.2010.55.1.0091
Ratkova, T. N., and Wassmann, P. (2002). Seasonal variation and spatial distribution of phyto-and protozooplankton in the central Barents Sea. J. Mar. Syst. 38, 47–75. doi: 10.1016/S0924-7963(02)00169-0
Reuss, N., and Poulsen, L. (2002). Evaluation of fatty acids as biomarkers for a natural plankton community. A field study of a spring bloom and a post-bloom period off West Greenland. Mar. Biol. 141, 423–434. doi: 10.1007/s00227-002-0841-6
Roukaerts, A., Nomura, D., Deman, F., Hattori, H., Dehairs, F., and Fripiat, F. (2019). The effect of melting treatments on the assessment of biomass and nutrients in sea ice (Saroma-ko lagoon, Hokkaido, Japan). Polar Biol. 42, 347–356. doi: 10.1007/s00300-018-2426-y
Ruess, L., and Müller-Navarra, D. (2019). Essential biomolecules in food webs. Front. Ecol. Evol. 7:269. doi: 10.3389/fevo.2019.00269
Sargent, J. R., and Falk-Petersen, S. (1988). “The lipid biochemistry of calanoid copepods,” in Biology of Copepods, eds G. A. Boxshall and H. K. Schminke (Berlin: Springer), 101–114. doi: 10.1007/978-94-009-3103-9_9
Schlichtholz, P. (2011). Influence of oceanic heat variability on sea ice anomalies in the Nordic Seas. Geophys. Res. Lett. 38, L05705. doi: 10.1029/2010GL045894
Schmidt, K., Atkinson, A., Petzke, K.-J., Voss, M., and Pond, D. W. (2006). Protozoans as a food source for Antarctic krill, Euphausia superba: Complementary insights from stomach content, fatty acids, and stable isotopes. Limnol. Oceanogr. 51, 2409–2427. doi: 10.4319/lo.2006.51.5.2409
Schmidt, K., Brown, T. A., Belt, S. T., Ireland, L. C., Taylor, K. W. R., Thorpe, S. E., et al. (2018). Do pelagic grazers benefit from sea ice? Insights from the Antarctic sea ice proxy IPSO25. Biogeosciences 15, 1987–2006. doi: 10.5194/bg-15-1987-2018
Scott, C. L., Falk-Petersen, S., Sargent, J. R., Hop, H., Lønne, O. J., and Poltermann, M. (1999). Lipids and trophic interactions of ice fauna and pelagic zooplankton in the marginal ice zone of the Barents Sea. Polar Biol. 21, 65–70. doi: 10.1007/s003000050335
Søreide, J. E., Falk-Petersen, S., Hegseth, E. N., Hop, H., Carroll, M. L., Hobson, K. A., et al. (2008). Seasonal feeding strategies of Calanus in the high-Arctic Svalbard region. Deep Sea Res. II Top. Stud. Oceanogr. 55, 2225–2244. doi: 10.1016/j.dsr2.2008.05.024
Søreide, J. E., Leu, E., Berge, J., Graeve, M., and Falk-Petersen, S. (2010). Timing of blooms, algal food quality and Calanus glacialis reproduction and growth in a changing Arctic. Glob. Change Biol. 16, 3154–3163. doi: 10.1111/j.1365-2486.2010.02175.x
Spreen, G., Kaleschke, L., and Heygster, G. (2008). Sea ice remote sensing using AMSR-E 89-GHz channels. J. Geophys. Res. (Oceans) 113:C02S03. doi: 10.1029/2005JC003384
Stevens, C. J., Deibel, D., and Parrish, C. C. (2004). Species-specific differences in lipid composition and omnivory indices in Arctic copepods collected in deep water during autumn (North Water Polynya). Mar. Biol. 144, 905–915. doi: 10.1007/s00227-003-1259-5
Stige, L. C., Eriksen, E., Dalpadado, P., and Ono, K. (2019). Direct and indirect effects of sea ice cover on major zooplankton groups and planktivorous fishes in the Barents Sea. ICES J. Mar. Sci. 76(Suppl. 1), i24–i36. doi: 10.1093/icesjms/fsz063
Stoecker, D., and Pierson, J. (2019). Predation on protozoa: its importance to zooplankton revisited. J. Plankton Res. 41, 367–373. doi: 10.1093/plankt/fbz027
Stübing, D., Hagen, W., and Schmidt, K. (2003). On the use of lipid biomarkers in marine food web analyses: an experimental case study on the Antarctic krill, Euphausia superba. Limnol. Oceanogr. 48, 1685–1700. doi: 10.4319/lo.2003.48.4.1685
Stukel, M. R., Ohman, M. D., Kelly, T. B., and Biard, T. (2019). The roles of suspension-feeding and flux-feeding zooplankton as gatekeepers of particle flux into the mesopelagic ocean in the Northeast Pacific. Front. Mar. Sci. 6:397. doi: 10.3389/fmars.2019.00397
Svensen, C., and Kiørboe, T. (2000). Remote prey detection in Oithona similis: hydromechanical versus chemical cues. J. Plankton Res. 22, 1155–1166. doi: 10.1093/plankt/22.6.1155
Swanberg, N., and Båmstedt, U. (1991). Ctenophora in the Arctic: the abundance, distribution and predatory impact of the cydippid ctenophore Mertensia ovum (Fabricius) in the Barents Sea. Polar Res. 10, 507–524. doi: 10.1111/j.1751-8369.1991.tb00669.x
Tamelander, T., Reigstad, M., Hop, H., Carroll, M. L., and Wassmann, P. (2008). Pelagic and sympagic contribution of organic matter to zooplankton and vertical export in the Barents Sea marginal ice zone. Deep Sea Res. II Top. Stud. Oceanogr. 55, 2330–2339. doi: 10.1016/j.dsr2.2008.05.019
Tamelander, T., Reigstad, M., Hop, H., and Ratkova, T. N. (2009). Ice algal assemblages and vertical export of organic matter from sea ice in the Barents Sea and Nansen Basin (Arctic Ocean). Polar Biol. 32:1261. doi: 10.1007/s00300-009-0622-5
Tamelander, T., Renaud, P. E., Hop, H., Carroll, M. L., Ambrose, W. G. Jr., and Hobson, K. A. (2006). Trophic relationships and pelagic–benthic coupling during summer in the Barents Sea Marginal Ice Zone, revealed by stable carbon and nitrogen isotope measurements. Mar. Ecol. Prog. Ser. 310, 33–46. doi: 10.3354/meps310033
Vinje, T., and Kvambekk, ÅS. (1991). Barents Sea drift ice characteristics. Polar Res. 10, 59–68. doi: 10.1111/j.1751-8369.1991.tb00635.x
Volkman, J. K., Barrett, S. M., Blackburn, S. I., Mansour, M. P., Sikes, E. L., and Gelin, F. (1998). Microalgal biomarkers: a review of recent research developments. Org. Geochem. 29, 1163–1179. doi: 10.1016/S0146-6380(98)00062-X
Volkman, J. K., Barrett, S. M., and Dunstan, G. A. (1994). C25 and C30 highly branched isoprenoid alkenes in laboratory cultures of two marine diatoms. Org. Geochem. 21, 407–414. doi: 10.1016/0146-6380(94)90202-X
Wang, S. W., Budge, S. M., Iken, K., Gradinger, R. R., Springer, A. M., and Wooller, M. J. (2015). Importance of sympagic production to Bering Sea zooplankton as revealed from fatty acid-carbon stable isotope analyses. Mar. Ecol. Prog. Ser. 518, 31–50. doi: 10.3354/meps11076
Wassmann, P., Duarte, C. M., Agusti, S., and Sejr, M. K. (2011). Footprints of climate change in the Arctic marine ecosystem. Glob. Change Biol. 17, 1235–1249. doi: 10.1111/j.1365-2486.2010.02311.x
Wassmann, P., Ratkova, T., and Reigstad, M. (2005). The contribution of single and colonial cells of Phaeocystis pouchetii to spring and summer blooms in the north-eastern North Atlantic. Harmful Algae 4, 823–840. doi: 10.1016/j.hal.2004.12.009
Wassmann, P., Reigstad, M., Haug, T., Rudels, B., Carroll, M. L., Hop, H., et al. (2006). Food webs and carbon flux in the Barents Sea. Prog. Oceanogr. 71, 232–287. doi: 10.1016/j.pocean.2006.10.003
Wickham, H. (2016). ggplot2: Elegant Graphics for Data Analysis. Berlin: Springer. doi: 10.1007/978-0-387-98141-3
Wold, A., Darnis, G., Søreide, J. E., Leu, E., Philippe, B., Fortier, L., et al. (2011). Life strategy and diet of Calanus glacialis during the winter-spring transition in Amundsen Gulf, south-eastern Beaufort Sea. Polar Biol. 34, 1929–1946. doi: 10.1007/s00300-011-1062-6
Keywords: food web, Barents Sea, sea ice, carbon sources, trophic markers, fatty acids, highly branched isoprenoid (HBI) lipids, sterols
Citation: Kohlbach D, Hop H, Wold A, Schmidt K, Smik L, Belt ST, Keck Al-Habahbeh A, Woll M, Graeve M, Dąbrowska AM, Tatarek A, Atkinson A and Assmy P (2021) Multiple Trophic Markers Trace Dietary Carbon Sources in Barents Sea Zooplankton During Late Summer. Front. Mar. Sci. 7:610248. doi: 10.3389/fmars.2020.610248
Received: 25 September 2020; Accepted: 17 December 2020;
Published: 14 January 2021.
Edited by:
Angel Borja, Technology Center Expert in Marine and Food Innovation (AZTI), SpainReviewed by:
C. J. Mundy, University of Manitoba, CanadaJory Cabrol, Fisheries and Oceans Canada, Canada
Copyright © 2021 Kohlbach, Hop, Wold, Schmidt, Smik, Belt, Keck Al-Habahbeh, Woll, Graeve, Dąbrowska, Tatarek, Atkinson and Assmy. This is an open-access article distributed under the terms of the Creative Commons Attribution License (CC BY). The use, distribution or reproduction in other forums is permitted, provided the original author(s) and the copyright owner(s) are credited and that the original publication in this journal is cited, in accordance with accepted academic practice. No use, distribution or reproduction is permitted which does not comply with these terms.
*Correspondence: Doreen Kohlbach, ZG9yZWVuLmtvaGxiYWNoQG5wb2xhci5ubw==; ZG9yZWVuLmtvaGxiYWNoQGF3aS5kZQ==