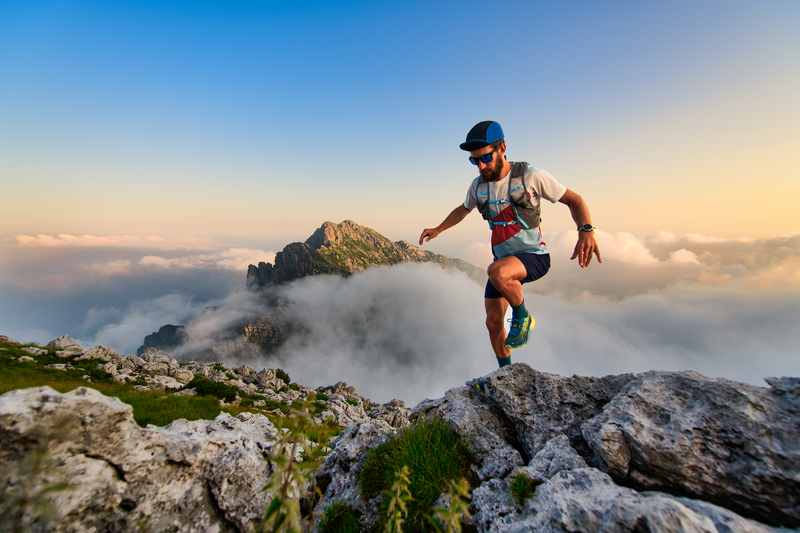
95% of researchers rate our articles as excellent or good
Learn more about the work of our research integrity team to safeguard the quality of each article we publish.
Find out more
ORIGINAL RESEARCH article
Front. Sustain. Food Syst. , 08 May 2024
Sec. Crop Biology and Sustainability
Volume 8 - 2024 | https://doi.org/10.3389/fsufs.2024.1407359
This article is part of the Research Topic Vertical Farming: New Trends, Products, and Production Approaches View all 10 articles
Compared with conventional crop cultivation in greenhouses or fields, plant factories with artificial light (PFAL) have advantages in the highly efficient use of space, energy, and resources available for cultivation. However, few studies on environmental controls for improving the space use efficacy (SUE) of PFAL in the production of edamame, a vegetable soybean, have been reported. Therefore, developing an environmental control method for high productivity with minimal space and energy requirements is of high priority. The aims of this study were to (1) identify the optimal photosynthetic photon flux density (PPFD) and light quality to enhance the SUE of edamame at the vegetative growth stage, and (2) examine the effects of PPFD, light quality, and their interaction on edamame plant growth at the vegetative stage. SUE is defined as the crop biomass produced per unit cubic volume of cultivation during the growth period. We examined three PPFD treatments (300, 500, and 700 μmol m−2 s−1) with three color temperature LED lamps (3,000, 5,000, and 6,500 K), for a total of nine treatments. The results demonstrated that, under the same light quality treatment, higher PPFDs resulted in larger fresh and dry weights of all organs, higher stem length, and lower specific leaf area. Under the same PPFD treatment, a high ratio of blue (400–499 nm) to red (600–699 nm) photon flux density increased the plant height but decreased the projected leaf area. The values of SUE at 700 μmol m−2 s−1 increased by 213, 163, and 92% with 3,000, 5,000, and 6,500 K, respectively compared with those at 300 μmol m−2 s−1. The values of SUE at 700 μmol m−2 s−1 increased by 34 and 23% in 5,000 and 6,500 K treatments, respectively compared with that in the 3,000 K treatment. In conclusion, a combination of 700 μmol m−2 s−1 PPFD and 5,000 K color temperature is the suitable condition to increase the SUE of edamame at the vegetative growth stage in a PFAL.
With the decrease in agricultural land resources, increase in unusual weather, and aggravated environmental pollution, a stable, safe, and sustainable food production system is necessary. To solve these problems, many studies have been conducted on closed-plant production systems have been proposed (Goto, 2012; Kozai, 2013; Ji et al., 2023). Recently, plant factories with artificial light (PFALs) and vertical farms—characterized by a multilayered cultivation system and utilization of artificial light—have been widely used to produce high-quality and fresh agricultural products throughout the year (Kozai and Niu, 2016; Benke and Tomkins, 2017; Sharath Kumar et al., 2020; Van Gerrewey et al., 2022). PFAL controls environmental factors such as light, temperature, humidity, CO2 enrichment, and air velocity for plant growth. It has water and CO2 savings for production compared with greenhouses and fields (Kozai, 2005; Graamans et al., 2018).
Soybeans are crucial crops containing high-quality proteins. Vegetable soybeans, also called edamame in Japanese, are harvested and consumed at the immature R6 developmental stage which occurs when the seeds or pods become larger but do not turn yellow (Ogles et al., 2016; Carneiro et al., 2020). Therefore, the difference between soybeans and edamame lies in the harvest or consumption time. Because edamame also contains high levels of protein, isoflavones, edible oil, and dietary fiber, it is very popular in the United States, Japan, China, and several other countries (Sánchez et al., 2005; Zeipina et al., 2017). In addition, edamame takes only 70–100 days from planting to harvest, shorter than that of soybeans (Konovsky et al., 1994; Moseley et al., 2021). In our preliminary study using a PFAL, edamame was harvested ~65 d after germination; therefore, it is more suitable for high-efficiency PFAL production. There have been some reports on PFAL cultivation of fruits and vegetables, such as strawberries and tomatoes (Yoshida et al., 2013; Ke et al., 2023; Park et al., 2023), wherein the time from germination to harvest is 100–150 d (Li et al., 2019). In comparison, edamame requires a shorter cultivation period, rendering it a marketable crop produced using PFAL. It is common for crops to be cultivated without agrichemicals in a PFAL; therefore, fresh and agrichemical-free edamame could be produced and made available to the market in the future (Wang, 2018).
In a PFAL, the energy consumption includes that of lamps and air conditioning systems for cooling, heating, and dehumidification (Graamans et al., 2018) and is a major production cost. Therefore, energy consumption should be reduced using lamps with higher electric efficiency and performance air conditioning systems. Reducing the space of the cultivation shelf of the multilayered cultivation system in a PFAL is also a good means to reduce the operational cost of the air-conditioning system because the volume of the targeted air to be controlled. Space use efficacy (SUE) is defined as the dry biomass produced per unit of cumulative volume of cultivation during a growth period and that combines both the integrated volume occupied by crops and dry biomass production. According to this definition, it is considered that SUE is improved by reducing the volume of cultivation space and/or increasing the dry biomass of crops. The volume of space required for crop production is determined by crop shape, with plant height being the most influential factor. For more efficient use of space, short plants are desirable to increase the number of plant layers in a multilayered cultivation system.
Photosynthetic photon flux density (PPFD) may influence SUE because it can affect plant morphology (Van Roekel and Purcell, 2014; Graham and Wheeler, 2017). Additionally, PPFD can affect dry biomass production, and decreased PPFD or shading conditions can significantly decrease soybean yield and total dry biomass accumulation (Kurosaki and Yumoto, 2003; Zhang et al., 2011; Wu et al., 2016). Moreover, light quality, as defined by color temperature in the present study, may also affect SUE. Developing a strategy to control the edamame light environment during the vegetative stage is important for determining its morphology and yield (Feng et al., 2018; Moseley et al., 2021). In addition, PPFD and light quality can affect the biomass accumulation and morphology of edamame, which are principal SUE determinants. Maximizing SUE is the primary strategy for achieving a high edamame yield in a PFAL. Biomass accumulation, such as that of non-structural carbohydrates, is essential for high translocation from leaves to seeds during the reproductive stage. This study had two objectives. The first was to assess the influence of PPFD and light quality on edamame SUE and to identify the optimal PPFD and light quality for improving SUE during the vegetative growth stage. The second was to identify the influence of PPFD and light quality, and their effect on edamame plant growth at the vegetative stage.
This experiment was conducted in a PFAL at the Matsudo Campus, Chiba University, Japan using the soybean cultivar, Glycine max L. Merrill. Enrei, which is available as both a vegetable and a conventional soybean. This cultivar accounted for 9% of the total soybean planting area in Japan in 2014 (Shimomura et al., 2015); consequently, ‘Enrei’ was chosen for the present study. Edamame seeds were sterilized in a 20% sodium hypochlorite (NaClO) solution for 10 min, washed with distilled water, and then germinated on wet filter paper in the dark for 4 d. After germination, the seedlings were put under white fluorescent lamps (FL, FHF32EXNH; Panasonic Corporation, Osaka, Japan) with 200 μmol m−2 s−1 PPFD at the top of the seedling canopy. Conditions were maintained at 1000 μmol mol−1 CO2 concentration, 25/20°C (day/night period) air temperature with 65–70% relative humidity, and 16/8 h (light/dark) photoperiod. All seedlings were cultivated using tap water for irrigation for 12 d after sowing (DAS). At 12 DAS—when the first true leaf had fully expanded—the seedlings were transplanted into the treatment area. Seedlings were cultivated using 1/2 OAT house A nutrients (OAT Agrio Co., Ltd., Tokyo, Japan). The height of the cultivation shelf and the area of cultivation area used in the study were 60 cm and 0.78 m2, respectively.
Different color temperatures of LED lamps with various spectral characteristics were used for the light quality treatments here (Tables 1, 2). White LED lamps (XLX450DELP LE9, XLX450NHNU LE9, and XLX460NEDT LE9; Panasonic Corporation, Osaka, Japan) with the color temperatures of 3,000 K (31.9 W), 5,000 K (26.3 W) and 6,500 K (43.1 W) were used as light sources, respectively. A spectroradiometer (USR-45DA, USHIO INC., Japan) was used to measure the spectral photon flux distribution of the lamps (spectral data are shown in Figure 1). The photon flux density (PFD) was recorded at three wavelengths: 400–499 nm (blue light), 500–599 nm (green light), and 600–699 nm (red light), the ratio of blue-to-red photon flux (B/R) and ratio of red-to-far-red photo flux (R/FR) ratio was calculated (Table 1 and Supplementary Figure S1). The ratios of B/R for the 3,000, 5,000, and 6,500 K lamps were 0.22, 0.70, and 1.04, respectively. There were three levels of PPFD: 300, 500, and 700 μmol m−2 s−1 with daily light integrals (DLIs) of 17.28, 28.80, and 40.32 mol m−2 d−1, respectively. In each PPFD level, there were three light qualities: 3,000, 5,000, and 6,500 K. The blue and red photon flux densities (BPFD and RPFD), green, and far-red wavelengths in different PPFDs and color temperature treatments are shown in Table 2. The power and energy consumptions in each light treatment are shown in Supplementary Table S1. Each treatment was performed in duplicate.
Table 2. Photon flux density (PFD) of blue, green, red, and far-red wavelengths in different photosynthetic photon flux density (PPFD) and light quality treatments.
Figure 1. Specific leaf area of edamame at 21 DAS in different PPFD and light quality treatments. Vertical bars indicate standard error (n = 6). Lowercase letters indicate significant differences between PPFD treatments but within the same light quality treatments, determined using Tukey’s HSD test at p < 0.05. Asterisks indicate significant differences at different light quality treatments but within the same PPFD treatments, determined using Tukey’s HSD test at p < 0.05.
The growth parameters were measured at 21 DAS with stem length, leaf area, and fresh and dry weights of the leaves, stems, branches, and roots of each plant measured using a ruler, leaf area meter (LI-3000C; LI-Cor Inc., Lincoln, NE, United States), and a digital balance, respectively. Plant organs were put in a convection oven (DV600 dry oven, Yamato Scientific Co., Ltd., Japan) and dried for 72 h at 80°C. The specific leaf area (SLA) of all leaves at 21 DAS was calculated by using the leaf area by the leaf dry weight. Daily canopy photographs from the top of the plants were used to calculate the projected leaf area (PLA) using a free imaging software (LIA 32 ver. 0.378).
Chlorophyll pigments were extracted from the first fully expanded leaf from the top of the seedlings at 21 DAS. Fresh leaves (0.08 g) were soaked in 2 mL 80% acetone for 24 h. The absorbance of the leaf extracts was measured at 663 and 645 nm using an ultraviolet–visible spectrophotometer (V-750; JASCO Co., Ltd., Japan), according to the method described by Porra et al. (1989).
A LI-6400 portable photosynthesis system (LI-COR Inc., Lincoln, NE, USA) with a leaf chamber containing a transparent cuvette was used to measure the net leaf photosynthetic rate (Pn). The first fully expanded leaf from the top of the edamame plant at 21 DAS for each of the nine treatments was used. For the measurements, the leaf was clamped in the chamber and environmental conditions of the leaf chamber were set as 25 ± 1°C leaf temperature, 1,000 μmol mol−1 CO2 concentration, 65–70% relative humidity, and 500 μmol s−1 air flow rate through the system. The leaves from each treatment were clamped, and Pn was measured after both Pn and stomatal conductance had stabilized.
SUE (g m−3) is defined as the ratio of the accumulated total dry biomass to the cumulative volume occupied by the plant at the growth stage as:
Where W (g) is the accumulated total dry biomass at the growth stage and V (m3) is the cumulative volume occupied by the plant at the growth stage. W (g) during the growth period is defined as:
Where Wn (g) and Wm (g) are the plant dry weights at day ‘m’ and day ‘n’. In the experiment, ‘m’ and ‘n’ are, respectively, 21 and 12. The V (m3) during the growth period is defined as:
Where PLA (t) (m2) is the plant projected leaf area on day t (n ≤ t ≤ m, integer) and h (t) (m) is the plant height on day t.
The mean values ± standard deviation of six individuals in each of the nine treatments are expressed in the results. SPSS for Windows (Version 24.0; SPSS Inc., Chicago, IL, United States) was used to conduct two-way analysis of variance (ANOVA) to determine if there was an interactive effect between ‘PPFD’ and ‘light quality’ variables on measurement and calculation parameters. An open-source statistical software program, JASP (Version 0.16.4; JASP Team, Amsterdam, The Netherlands), was used for the Test of Simple Effects to uncover the extent to which one factor exhibited different effectiveness at each level of a second factor. Subsequently, a post-hoc analysis was performed using Tukey’s HSD test method and p < 0.05 was considered as statistically significant (Goss-Sampson, 2020).
Biomass accumulation at 21 DAS is shown in Table 3. Biomass accumulation significantly increased with increasing PPFD under the same light quality treatments. In the 3,000, 5,000, and 6,500 K treatments, the W values of at 21 DAS increased by 175, 189, and 88%, respectively at 700 compared to 300 μmol m−2 s−1. Moreover, they increased by 119, 74, and 62%, respectively at 500 compared to 300 μmol m−2 s−1. At 300 μmol m−2 s−1, the W values increased with an increasing ratio of B/R, and at 500 μmol m−2 s−1, the W values decreased with an increasing ratio of B/R. When PPFD was 700 μmol m−2 s−1, the W values were significantly higher in the 3,000 and 5,000 K treatments than those in the 6,500 K treatment. In addition, the interaction between the PPFD and light quality affected W (Table 4).
Table 3. The cumulative volume occupied by the plant (V), dry biomass accumulation during the growth period (W), and space use efficacy (SUE) of edamame at 21 DAS under different PPFD and light quality treatments.
Table 4. Analysis of two-way variance for the effects of PPFD, light quality, and their interactions on root dry weigh (RDW), stem dry weight (SDW), leaf dry weight (LDW), branch dry weight (BDW), biomass accumulation during the growth period (W), plant height (PH), stem length (SL), stem diameter (SD), leaf area (LA), specific leaf area (SLA), project leaf area (PLA), chlorophyll a, b, and a + b concentrations, chlorophyll a/b ratio, and photosynthetic rate (Pn) of edamame at 21 DAS.
The SLA values were significantly higher at 300 μmol m−2 s−1 than those at 500 and 700 μmol m−2 s−1 (Figure 1). Additionally, the SLA was significantly higher in the 3,000 K treatment than that in the 5,000 K treatment when the PPFD was 500 and 700 μmol m−2 s−1. There was an interaction effect between PPFD and light quality on SLA (Table 4).
Figure 2 shows the plant height from 12 to 21 DAS in edamame under different PPFDs and light qualities, the interaction between which affected both plant height and stem length (Table 4). Plant height increased rapidly at 17 DAS (Figure 2). The plant height at 700 μmol m−2 s−1 in 3,000, 5,000, and 6,500 K at 21 DAS increased by 13.0, 26.4, and 11.6%, respectively, compared to that at 300 μmol m−2 s−1. In addition, plant height in the same PPFD treatments was higher in the 3,000 K treatment than that in the 5,000 and 6,500 K treatments. At all PPFD treatments, the plant height in the 6,500 K treatment at 21 DAS increased by 30.4, 29.6, and 28.8% at 300, 500, and 700 μmol m−2 s−1, respectively, compared to that in the 3,000 K treatment.
Figure 2. Plant height from 12 to 21 DAS in edamame regulated by different PPFDs and light qualities. Pictures (A-C) show the color temperatures of 3,000, 5,000, and 6,500 K, respectively. The B/R ratio of 3,000, 5,000, and 6,500 K lamps from Table 1 are 0.22, 0.74, and 1.22, respectively. Pictures (D-F) show the PPFDs were 300, 500, and 700 μmol m−2 s−1, respectively. Vertical bars indicate standard error (n = 6). Lowercase letters indicate significant differences at different treatments, determined using Tukey’s HSD test at p < 0.05.
Figure 3 shows the effects of BPFD and the ratio of BPFD/PPFD on stem length at 21 DAS. The stem length decreased with increasing BPFD. Interestingly, the stem length significantly decreased with increase in BPFD up to 107.5 μmol m−2 s−1 and then slightly declined when BPFD further increased up to 188.9 μmol m−2 s−1. Both the BPFD and BPFD/PPFD affected stem elongation. Far-red photon flux density (FRPFD) had a slightly stronger effect on stem elongation than BPFD did.
Figure 3. The effects of blue photon flux density (A) and ratio of blue photon flux density to photosynthetic photon flux density (B) on stem length in edamame at 21 DAS. The effects of far-red photon flux density (C) and the ratio of far-red photon flux density to photosynthetic photon flux density. (D) on SL in edamame at 21 DAS. Vertical bars indicate standard error (n = 6). BPFD means blue photon flux density (μmol m−2 s−1). PPFD means photosynthetic photon flux density (μmol m−2 s−1). FRPFD means far-red photon flux density (μmol m−2 s−1).
Under the same PPFD treatments, as BPFD/PPFD increased, PLA decreased (Figure 4). PLA was significantly higher in the 3,000 K treatment than those in the 5,000 and 6,500 K treatments when PPFD was 300 and 700 μmol m−2 s−1.
Figure 4. The projected leaf area from 12 to 21 DAS in edamame regulated by different PPFDs and light qualities. Pictures (A–C) show the PPFDs were 300, 500, and 700 μmol m−2 s−1, respectively. The B/R ratio of 3,000, 5,000, and 6,500 K lamps from Table 1 are 0.22, 0.74, and 1.22, respectively. Vertical bars indicate standard error (n = 5). Lowercase letters indicate significant differences at different light quality treatments but within the same PPFD treatments, determined using Tukey’s HSD test at p < 0.05.
The V values were highest at 300 μmol m−2 s−1 under the same light quality treatments at 21 DAS (Table 3), and significantly increased after 17 DAS (Figure 5). Under the same PPFD treatments, as the ratio of B/R increased, V decreased. When PPFD was 300 and 700 μmol m−2 s−1, the V values were significantly higher in the 3,000 K treatment than those in the 5,000 and 6,500 K treatments at 21 DAS.
Figure 5. The cumulative volume (V) occupied by the plant from 12 to 21 DAS in edamame regulated by different PPFDs and light qualities. Pictures (A–C) show the PPFDs were 300, 500, and 700 μmol m−2 s−1, respectively. The B/R ratio of 3,000, 5,000, and 6,500 K lamps from Table 1 are 0.22, 0.74, and 1.22, respectively. Vertical bars indicate standard error (n = 5). Lowercase letters indicate significant differences between different light quality treatments but within the same PPFD treatments, determined using Tukey’s HSD test at p < 0.05.
Chlorophyll a (Chl a) concentration decreased with increasing PPFD in the 5,000 K treatment (Figure 6). The Chl a concentration had the highest value at 700 μmol m−2 s−1 in both 3,000 and 6,500 K treatments. Chlorophyll b (Chl b) concentration decreased with increasing PPFD, and it was significantly higher at 300 μmol m−2 s−1 than that at 500 and 700 μmol m−2 s−1 in the 5,000 K treatment. The chlorophyll a + b concentrations (Chl a + b) were higher at 300 and 500 μmol m−2 s−1 compared to 700 μmol m−2 s−1 in the same light quality treatments. The interaction between PPFD and light quality affected the ratio of chlorophyll a/b (Chl a/b) (Table 4) which was significantly higher at 700 μmol m−2 s−1 than those at 300 and 500 μmol m−2 s−1.
Figure 6. The chlorophyll a (A), chlorophyll b (B), and chlorophyll a + b (C) concentrations, and chlorophyll a/b ratio (D) of edamame at 21 DAS in different PPFD and light quality treatments. Vertical bars indicate standard error (n = 6). Lowercase letters indicate significant differences between PPFD treatments but within the same light quality treatment, determined using Tukey’s HSD test at p < 0.05. Asterisks indicate significant differences between light quality treatments but within the same PPFD treatments, determined using Tukey’s HSD test at p < 0.05.
The interaction between PPFD and light quality affected Pn (Table 4) which increased with increasing PPFD under the same light quality (Figure 7). It was significantly higher at 500 and 700 μmol m−2 s−1 than at 300 μmol m−2 s−1. Moreover, Pn was significantly higher in the 3,000 K treatment than in the 5,000 and 6,500 K treatments when PPFD was 300 μmol m−2 s−1.
Figure 7. The photosynthetic rate (Pn) of edamame leaves at 21 DAS in different PPFD and light quality treatments. Vertical bars indicate standard error (n = 3). Lowercase letters indicate significant differences between PPFD treatments but within the same light quality treatment, determined using Tukey’s HSD test at p < 0.05. Asterisks indicate significant differences between light quality treatments but within the same PPFD treatments, determined using Tukey’s HSD test at p < 0.05.
SUE values increased with increasing PPFD under the same light quality treatments (Table 3). Under the 3,000 K treatment, SUE values were significantly higher at 500 and 700 μmol m−2 s−1 than those at 300 μmol m−2 s−1. In the 5,000 K treatment, all SUE values differed significantly among the three PPFD treatments. Under 3,000, 5,000, and 6,500 K treatments, the SUE values at 700 μmol m−2 s−1 increased by 213, 163, and 92%, respectively compared to those at 300 μmol m−2 s−1. Moreover, SUE at 500 μmol m−2 s−1 increased by 150, 83, and 44%, respectively compared to 300 μmol m−2 s−1. When PPFD was 300 μmol m−2 s−1, the SUE values increased when the ratio of B/R was increased, and they also increased by 100 and 60% in 6,500 and 5,000 K treatments, respectively compared to those in the 3,000 K treatment. When PPFD was 500 μmol m−2 s−1, SUE values increased by 16 and 17% in 6,500 and 5,000 K treatments, respectively compared to those in the 3,000 K treatment. When PPFD was 700 μmol m−2 s−1, SUE values in 6,500 and 5,000 K treatments increased by 23 and 34%, respectively compared to those in the 3,000 K treatment.
The value of SUE depends on the W and V (Eq. 1) and W was calculated using (Eq. 2). Under the same light quality treatments, high PPFD (500 and 700 μmol m−2 s−1) increased SUE by increasing the W and reducing the V (Table 3). As PPFD increased, the W increased and V decreased under the same light quality treatments. Moreover, PPFD affected the W more than the V. The W had a greater influence on SUE than V because there was a significant difference in W in the different PPFD treatments under the same light quality. However, there were no significant differences in V among the different PPFD treatments under the same light quality.
Dry biomass production depends on Pn and leaf area (Trouwborst et al., 2011; Malek et al., 2012). The results of our study indicated that the Pn (Figure 7) of the leaves increased with increasing PPFD under the same light quality treatments. In addition, the leaf area increased with increasing PPFD (Supplementary Figure S2). The chlorophyll concentration in leaves is an important element that affects their photosynthetic capacity (Dai et al., 2009; Shao et al., 2014). Gong et al. (2017) found that soybean leaves have higher chlorophyll concentrations under shaded conditions, which enables a more effective capture of light. Our results showed high PPFD led to low chlorophyll concentrations and high ratio of Chl a/b (Figure 6). The greater decrease in Chl b concentration compared to Chl a concentration was mainly responsible for decreasing the ratio of Chl a/b at 700 μmol m−2 s−1. Chl b tends to absorb solar radiation scattered in a manner complementary to that of Chl a. Therefore, Chl b enhances the ability of plants to absorb solar radiation (Voitsekhovskaja and Tyutereva, 2015). With increasing PPFD, the requirement for light-harvesting complexes to efficiently capture light decreases, leading to a decrease in the Chl b concentration at a higher PPFD (Pearcy and Seemann, 1990). Leaf thickness and the relative proportions of conductive, assimilatory, and mechanical tissues were determined using SLA (Reich et al., 1998). In particular, the increased total leaf thickness with increasing PPFD primarily results from an increase in the thickness of the palisade parenchyma, epidermal tissue, and spongy parenchyma (Evans, 1999). Such a structure is beneficial for leaves to intercept more light resources. This is one of the main reasons why Pn at 500 and 700 μmol m−2 s−1 was significantly higher than those at 300 μmol m−2 s−1 under the same light quality treatments (Figure 7).
The value of V depends on the PLA and plant height (Eq. 3). PPFD had a significant effect on V (Figure 5) because there was a significant difference in plant height (Figure 2) among the different PPFD treatments under the same light quality treatments. Some studies reported that the plant height increased with the increasing PPFD from 200 to 290 μmol m−2 s−1 in lettuce (Kang et al., 2013) and from 50 to 600 μmol m−2 s−1 in basil (Larsen et al., 2020). However, some studies verified that the plant height decreased with increasing PPFD from 200 to 500 μmol m−2 s−1 in tomato (Ke et al., 2023) and from 100 to 800 μmol m−2 s−1 in wheat (Li et al., 2021). In the case of soybean, the plant height decreased when the PPFD increased from 63 to 700 μmol m−2 s−1 (Feng et al., 2018; Yang et al., 2020) which was consistent with our results (Figure 2). Low PPFD induces a shade response in plants (Smith and Whitelam, 1997). Plants grown under shaded conditions allocate more biomass to stem growth than to leaf expansion (Franklin, 2008). Gibberellins (GAs) and auxins—two endogenous plant hormones—regulate the shade-induced differential growth and elongation of stems (Kurepin et al., 2007; Yamaguchi, 2008). Low PPFD increases GA levels in the internodes of soybeans (Yang et al., 2018) which may be the reason for increasing plant height of edamame in the present study. The results suggest 700 μmol m−2 s−1 is the best PPFD for edamame at the vegetative growth stage to improve SUE.
Under the same PPFD treatments, light quality increased SUE mainly by reducing V (Table 3) which is due to the difference in the B/R ratio between the different light quality treatments. Our results indicate that a high B/R ratio (0.70 and 1.04) led to a lower plant height (Figure 2) and PLA (Figure 4). The W increased with an increasing B/R ratio at 300 μmol m−2 s−1. However, the high B/R ratio decreased the W at 500 and 700 μmol m−2 s−1.
Blue light is an important single light source that alters plant morphology (Crosson and Moffat, 2001). Low blue light or low light intensity enhances gibberellin and auxin accumulation in plant stems, causing stem cells to elongate (Spalding and Cosgrove, 1989; Goyal et al., 2013). The elongation response of the stem to low blue light is consistent with the shade avoidance response to low PPFD, which causes low absolute blue light intensity (Cope and Bugbee, 2013; Hitz et al., 2019). Blue light reduces leaf area and stem elongation by inhibiting gibberellin synthesis (Lyu et al., 2021). Lyu et al. (2021) found that soybean stem length increased with increasing BPFD from 4 μmol m−2 s−1 to 106 μmol m−2 s−1. Here, we found that the shade avoidance response can be triggered by a low BPFD even under high PPFD (500 and 700 μmol m−2 s−1). Interestingly, stem length was more influenced by BPFD than by BPFD/PPFD, which is consistent with the findings of Cope and Bugbee (2013); however, the current study investigated a wider PPFD range. BPFD instead of BPFD/PPFD was found to be an important factor that determines the stem elongation response to blue light (Wheeler et al., 1991).
A low R/FR ratio can trigger a shade avoidance response. Hitz et al. (2019) found that the stem length of soybeans was higher under a R/FR ratio of 1.5 than of 5. Yang et al. (2020) found that the stem length of soybeans was higher under a R/FR ratio of 0.42 than of 1.33. However, in our study, far-red light had a weaker effect on stem elongation than blue light (Figure 3) because the R/FR ratio in our treatments was higher than that in studies by Hitz et al. (2019) and Yang et al. (2020). Under natural environmental conditions, the ratio of R/FR is ~1.2 in daylight, under a canopy with a leaf area index of 4 (Holmes and Smith, 1975). This ratio is lower than that observed in this study (Table 1); therefore, the R/FR ratio did not affect stem elongation. In addition, Lyu et al. (2021) showed that low blue PFD predominantly induced excessive soybean stem elongation compared with far-red light, consistent with the results here.
Our results reveal that light quality had no significant influence on leaf area under the same PPFD treatments, consistent with the results of Cope and Bugbee (2013). Although an increase in the B/R ratio from 0.22 to 1.04 increased the photosynthetic capacity of the leaves under the same PPFD treatments (Supplementary Figure S3), the increase was smaller at a PPFD of 700 μmol m−2 s−1. This is because the R/FR ratios were higher in the 5,000 and 6,500 K treatments than in the 3,000 K treatment. Far-red light can increase Pn by increasing the quantum yield of photosystems (Zhen and van Iersel, 2017; Zhen et al., 2019). PLA was significantly influenced by the color temperature (Figure 4 and Supplementary Figure S4); additionally, the PLA content decreased as the B/R ratio increased. The reduction of PLA at the 6,500 K treatment was the main reason for the decrease in W at a PPFD of 700 μmol m−2 s−1. The reduction of W eventually led to the decreased SUE in the 6,500 K treatment compared to the 5,000 K treatment at PPFDs of 500 and 700 μmol m−2 s−1.
These results suggest that high levels of BPFD (21.0 and 26.3%) increased the SUE by reducing plant height and PLA with the same PPFD. In addition, the SUE was higher in the 5,000 K treatment than in 3,000 and 6,500 K treatments at PPFDs of 500 and 700 μmol m−2 s−1. Furthermore, the energy consumptions of lamps in 5,000 K treatments were the lowest at the same PPFD (Supplementary Table S1). Therefore, the 5,000 K color temperature was the best light quality for edamame at the vegetative growth stage for improving SUE.
PPFD and light quality can also affect SUE through their interactive effects on the growth and morphology of edamame. Effects of the interactions between PPFD and light quality were found for W, plant height, stem length, SLA, Chl a/b, and Pn (Table 4). We found that the light quality affected W at different PPFD levels (Table 3). W increased with the increasing B/R ratio at the PPFD of 300 μmol m−2 s−1; however, W decreased with the increasing B/R ratio at the PPFDs of 500 and 700 μmol m−2 s−1. This indicated that W was affected by the light quality, and the results of this effect depended on the PPFD. In addition to the anticipated increase in stem length in response to a low PPFD, a decrease in the ratio of B/R increased the elongation response to PPFD. Stem length decreased in response to increasing PPFD, with an additive effect of a decreasing B/R ratio. This may explain why BPFD had a greater influence on stem length than BPFD/PPFD. Therefore, stem length responds to PPFD, light quality, and their interactions, which ultimately affect plant height.
PPFD and light quality affected SUE differently. PPFD had a significant effect on SUE by influencing W and plant height; however, light quality affected SUE by influencing the PLA and plant height. In addition, the interaction between PPFD and light quality indirectly affected SUE by affecting the W and height of edamame during the vegetative growth stage. White LEDs with full spectra were used in this study. From a physiological viewpoint, the influence of red, blue, and far-red light on the growth and SUE of edamame cannot be accurately explained. Moreover, light is an important resource in cultivation spaces, and its utilization efficiency affects SUE within a certain range. In addition, only the vegetative growth stage was considered in this study. Total dry weight increased significantly at 18 DAS (Supplementary Figure S5), and the plants were in a stage of rapid growth until 21 DAS. Therefore, the effects of PPFDs and light quality on the growth and SUE of edamame may differ between the reproductive and vegetative growth stages. Further research is necessary to determine the suitable light conditions to improve the SUE of edamame during the reproductive growth stage.
Our results show that increasing PPFD could significantly improve the SUE of edamame at the vegetative growth stage by increasing W and decreasing V. High PPFD improved W by increasing Pn and leaf area and decreased V by decreasing plant height or stem length. Moreover, a high color temperature improved SUE, mainly by reducing V. This was because the high B/R ratio decreased V by reducing plant height and PLA under LED lamps with a high color temperature. However, a high B/R ratio decreased W and further decreased SUE at the PPFDs of 500 and 700 μmol m−2 s−1. In conclusion, a combination of 700 μmol m−2 s−1 PPFD and 5,000 K color temperature is recommended for edamame cultivation to improve SUE at the vegetative growth stage in a PFAL. This knowledge suggests that the optimal combination between PPFD and light quality can improve efficiency for the sustainable production of pesticide-free and high-quality edamame in commercial PFALs and further can be utilized in space agriculture.
The original contributions presented in the study are included in the article/Supplementary material, further inquiries can be directed to the corresponding author.
QL: Conceptualization, Formal analysis, Investigation, Methodology, Validation, Visualization, Writing – original draft. XK: Writing – review & editing. HY: Writing – review & editing. SH: Writing – review & editing. EG: Conceptualization, Funding acquisition, Methodology, Writing – review & editing.
The author(s) declare that financial support was received for the research, authorship, and/or publication of this article. This research was funded by the Ministry of Agriculture, Forestry and Fisheries (MAFF) strategy project “Development of a highly resource-recycling food system that supports long-term stays on the moon, etc.” Grant Number JPJ01857 and the Program on Open Innovation Platform with Enterprises, Research Institute, and Academia, Japan Science and Technology Agency (JST-OPERA, JPMJOP1851).
The authors declare that the research was conducted in the absence of any commercial or financial relationships that could be construed as a potential conflict of interest.
All claims expressed in this article are solely those of the authors and do not necessarily represent those of their affiliated organizations, or those of the publisher, the editors and the reviewers. Any product that may be evaluated in this article, or claim that may be made by its manufacturer, is not guaranteed or endorsed by the publisher.
The Supplementary material for this article can be found online at: https://www.frontiersin.org/articles/10.3389/fsufs.2024.1407359/full#supplementary-material
Benke, K., and Tomkins, B. (2017). Future food-production systems: vertical farming and controlled-environment agriculture. Sustain. Sci. Pract. Policy 13, 13–26. doi: 10.1080/15487733.2017.1394054
Carneiro, R. C. V., Duncan, S. E., O'Keefe, S. F., Yin, Y., Neill, C. L., and Zhang, B. (2020). Sensory and consumer studies in plant breeding: a guidance for edamame development in the U.S. Front. Sustain. Food Syst. 4:124. doi: 10.3389/fsufs.2020.00124
Cope, K. R., and Bugbee, B. (2013). Spectral effects of three types of white light-emitting diodes on plant growth and development: absolute versus relative amounts of blue light. HortScience 48, 504–509. doi: 10.21273/HORTSCI.48.4.504
Crosson, S., and Moffat, K. (2001). Structure of a flavin-binding plant photoreceptor domain: insights into light-mediated signal transduction. Proc. Natl. Acad. Sci. USA 98, 2995–3000. doi: 10.1073/pnas.051520298
Dai, Y. J., Shen, Z. G., Liu, Y., Wang, L. L., Hannaway, D., and Lu, H. F. (2009). Effects of shade treatments on the photosynthetic capacity, chlorophyll fluorescence, and chlorophyll content of Tetrastigma hemsleyanum Diels et Gilg. Environ. Exp. Bot. 65, 177–182. doi: 10.1016/j.envexpbot.2008.12.008
Evans, J. R. (1999). Leaf anatomy enables more equal access to light and CO2 between chloroplasts. New Phytol. 143, 93–104. doi: 10.1046/j.1469-8137.1999.00440.x
Feng, L., Raza, M. A., Li, Z., Chen, Y., Khalid, M. H. B., Du, J., et al. (2018). The influence of light intensity and leaf movement on photosynthesis characteristics and carbon balance of soybean. Front. Plant Sci. 9:1952. doi: 10.3389/fpls.2018.01952
Franklin, K. A. (2008). Shade avoidance. New Phytol. 179, 930–944. doi: 10.1111/j.1469-8137.2008.02507.x
Gong, J., Liu, M., Xu, S., Jiang, Y., Pan, Y., Zhai, Z., et al. (2017). Effects of light deficiency on the accumulation of saikosaponins and the ecophysiological characteristics of wild Bupleurum chinense DC. in China. Ind. Crops Prod. 99, 179–188. doi: 10.1016/j.indcrop.2017.01.040
Goss-Sampson, M. A. (2020). Bayesian inference in JASP: a guide for students. Available at http://static.jasp-stats.org/Manuals/Bayesian_Guide_v0_12_2_1.pdf
Goto, E. (2012). Plant production in a closed plant factory with artificial lighting. Acta Hortic. 956, 37–49. doi: 10.17660/ActaHortic.2012.956.2
Goyal, A., Szarzynska, B., and Fankhauser, C. (2013). Phototropism: at the crossroads of light-signaling pathways. Trends Plant Sci. 18, 393–401. doi: 10.1016/j.tplants.2013.03.002
Graamans, L., Baeza, E., Van Den Dobbelsteen, A., Tsafaras, I., and Stanghellini, C. (2018). Plant factories versus greenhouses: comparison of resource use efficiency. Agric. Syst. 160, 31–43. doi: 10.1016/j.agsy.2017.11.003
Graham, T., and Wheeler, R. (2017). Mechanical stimulation modifies canopy architecture and improves volume utilization efficiency in bell pepper: implications for bioregenerative life-support and vertical farming. Open Agric. 2, 42–51. doi: 10.1515/opag-2017-0004
Hitz, T., Hartung, J., Graeff-Hönninger, S., and Munz, S. (2019). Morphological response of soybean (Glycine max (L.) Merr.) cultivars to light intensity and red to far-red ratio. Agronomy 9:428. doi: 10.3390/agronomy9080428
Holmes, M. G., and Smith, H. (1975). The function of phytochrome in plants growing in the natural environment. Nature 254, 512–514. doi: 10.1038/254512a0
Ji, Y., Kusuma, P., and Marcelis, L. F. (2023). Vertical farming. Curr. Biol. 33, R471–R473. doi: 10.1016/j.cub.2023.02.010
Kang, J. H., KrishnaKumar, S., Atulba, S. L. S., Jeong, B. R., and Hwang, S. J. (2013). Light intensity and photoperiod influence the growth and development of hydroponically grown leaf lettuce in a closed-type plant factory system. Hortic. Environ. Biotechnol. 54, 501–509. doi: 10.1007/s13580-013-0109-8
Ke, X., Yoshida, H., Hikosaka, S., and Goto, E. (2023). Photosynthetic photon flux density affects fruit biomass radiation-use efficiency of dwarf tomatoes under LED light at the reproductive growth stage. Front. Plant Sci. 14:1076423. doi: 10.3389/fpls.2023.1076423
Konovsky, J., Lumpkin, T. A., and McClary, D. (1994). “Edamame: the vegetable soybean,” In Understanding the Japanese food and agrimarket: a multifaceted opportunity. Ed. A. D. O’Rourke (Boca Raton, FL, USA: CRC Press), 173–181.
Kozai, T. (2005). “Closed systems for high quality transplants using minimum resources” in Plant culture engineering. eds. S. D. Gupta and Y. Ibaraki (Berlin: Springer), 275–312.
Kozai, T. (2013). Resource use efficiency of closed plant production system with artificial light: concept, estimation and application to plant factory. Proc. Jpn. Acad. 89, 447–461. doi: 10.2183/pjab.89.447
Kozai, T., and Niu, G. (2016). “Plant factory as a resource-efficient closed plant production system” in Plant factory: An indoor vertical farming system for efficient quality food production. eds. T. Kozai, G. Niu, and M. Takagaki (Cambridge, MA: Academic Press), 69–90.
Kurepin, L. V., Emery, R. J., Pharis, R. P., and Reid, D. M. (2007). The interaction of light quality and irradiance with gibberellins, cytokinins and auxin in regulating growth of Helianthus annuus hypocotyls. Plant Cell Environ. 30, 147–155. doi: 10.1111/j.1365-3040.2006.01612.x
Kurosaki, H., and Yumoto, S. (2003). Effects of low temperature and shading during flowering on the yield components in soybeans. Plant Prod. Sci. 6, 17–23. doi: 10.1626/pps.6.17
Larsen, D. H., Woltering, E. J., Nicole, C. C. S., and Marcelis, L. F. M. (2020). Response of basil growth and morphology to light intensity and spectrum in a vertical farm. Front. Plant Sci. 11:597906. doi: 10.3389/fpls.2020.597906
Li, Y., Yang, C., Zhang, Z., Zhao, S., and Gao, H. (2021). Photosynthetic acclimation strategies in response to intermittent exposure to high light intensity in wheat (Triticum aestivum L.). Environ. Exp. Bot. 181:104275. doi: 10.1016/j.envexpbot.2020.104275
Li, J., Zhang, N., Luo, J., Yu, Q., Ai, W., Zhang, L., et al. (2019). Growth and biomass yield of 25 crops in the 4-subject 180-day integrated experiment. Acta Astronaut. 162, 336–343. doi: 10.1016/j.actaastro.2019.06.028
Lyu, X., Cheng, Q., Qin, C., Li, Y., Xu, X., Ji, R., et al. (2021). GmCRY1s modulate gibberellin metabolism to regulate soybean shade avoidance in response to reduced blue light. Mol. Plant 14, 298–314. doi: 10.1016/j.molp.2020.11.016
Malek, M. A., Mondal, M. M. A., Ismail, M. R., Rafii, M. Y., and Berahim, Z. (2012). Physiology of seed yield in soybean: growth and dry matter production. Afr. J. Biotechnol. 11, 7643–7649. doi: 10.5897/AJB11.3879
Moseley, D., Silva, M. P., Mozzoni, L., Orazaly, M., Florez-Palacios, L., Acuña, A., et al. (2021). Effect of planting date and cultivar maturity in edamame quality and harvest window. Front. Plant Sci. 11:585856. doi: 10.3389/fpls.2020.585856
Ogles, C. Z., Guertal, E. A., and Weaver, D. B. (2016). Edamame cultivar evaluation in Central Alabama. Agron. J. 108, 2371–2378. doi: 10.2134/agronj2016.04.0218
Park, Y., Sethi, R., and Temnyk, S. (2023). Growth, flowering, and fruit production of strawberry ‘Albion’in response to photoperiod and photosynthetic photon flux density of sole-source lighting. Plan. Theory 12:731. doi: 10.3390/plants12040731
Pearcy, R. W., and Seemann, J. R. (1990). Photosynthetic induction state of leaves in a soybean canopy in relation to light regulation of ribulose-1-5-bisphosphate carboxylase and stomatal conductance. Plant Physiol. 94, 628–633. doi: 10.1104/pp.94.2.628
Porra, R. J., Thompson, W. A., and Kriedemann, P. E. (1989). Determination of accurate extinction coefficients and simultaneous equations for assaying chlorophylls a and b extracted with four different solvents: verification of the concentration of chlorophyll standards by atomic absorption spectroscopy. Biochim. Biophys. Acta 975, 384–394. doi: 10.1016/S0005-2728(89)80347-0
Reich, P., Ellsworth, D., and Walters, M. (1998). Leaf structure (specific leaf area) modulates photosynthesis-nitrogen relations: evidence from within and across species and functional groups. Funct. Ecol. 12, 948–958. doi: 10.1046/j.1365-2435.1998.00274.x
Sánchez, E., Kelley, K., and Butler, L. (2005). Edamame production as influenced by seedling emergence and plant population. HortTechnology 15, 672–676. doi: 10.21273/HORTTECH.15.3.0672
Shao, Q. S., Wang, H. Z., Guo, H. P., Zhou, A. C., Huang, Y. Q., Sun, Y. L., et al. (2014). Effects of shade treatments on photosynthetic characteristics, chloroplast ultrastructure, and physiology of Anoectochilus roxburghii. PLoS One 9:e85996. doi: 10.1371/journal.pone.0085996
Sharath Kumar, M., Heuvelink, E., and Marcelis, L. F. (2020). Vertical farming: moving from genetic to environmental modification. Trends Plant Sci. 25, 724–727. doi: 10.1016/j.tplants.2020.05.012
Shimomura, M., Kanamori, H., Komatsu, S., Namiki, N., Mukai, Y., Kurita, K., et al. (2015). The Glycine max cv. Enrei genome for improvement of Japanese soybean cultivars. Int. J. Genomics 2015:358127. doi: 10.1155/2015/358127
Smith, H., and Whitelam, G. (1997). The shade avoidance syndrome: multiple responses mediated by multiple phytochromes. Plant Cell Environ. 20, 840–844. doi: 10.1046/j.1365-3040.1997.d01-104.x
Spalding, E. P., and Cosgrove, D. J. (1989). Large plasma-membrane depolarization precedes rapid blue-light-induced growth inhibition in cucumber. Planta 178, 407–410. doi: 10.1007/BF00391869
Trouwborst, G., Hogewoning, S. W., Harbinson, J., and van Ieperen, W. (2011). The influence of light intensity and leaf age on the photosynthetic capacity of leaves within a tomato canopy. J. Hortic. Sci. Biotechnol. 86, 403–407. doi: 10.1080/14620316.2011.11512781
Van Gerrewey, T., Boon, N., and Geelen, D. (2022). Vertical farming: the only way is up? Agronomy 12, 1–15. doi: 10.3390/agronomy12010002
Van Roekel, R. J., and Purcell, L. C. (2014). Soybean biomass and nitrogen accumulation rates and radiation use efficiency in a maximum yield environment. Crop Sci. 54, 1189–1196. doi: 10.2135/cropsci2013.08.0546
Voitsekhovskaja, O. V., and Tyutereva, E. V. (2015). Chlorophyll b in angiosperms: functions in photosynthesis, signaling and ontogenetic regulation. J. Plant Physiol. 189, 51–64. doi: 10.1016/j.jplph.2015.09.013
Wang, K. C. (2018). Food safety and contract edamame: the geopolitics of the vegetable trade in East Asia. Geogr. Rev. 108, 274–295. doi: 10.1111/gere.12254
Wheeler, R. M., Mackowiak, C. L., and Sager, J. C. (1991). Soybean stem growth under high-pressure sodium with supplemental blue lighting. Agron. J. 83, 903–906. doi: 10.2134/agronj1991.00021962008300050024x
Wu, Y., Gong, W., Yang, F., Wang, X., Yong, T., and Yang, W. (2016). Responses to shade and subsequent recovery of soya bean in maize-soya bean relay strip intercropping. Plant Prod. Sci. 19, 206–214. doi: 10.1080/1343943X.2015.1128095
Yamaguchi, S. (2008). Gibberellin metabolism and its regulation. Ann. Rev. Plant Biol. 59, 225–251. doi: 10.1146/annurev.arplant.59.032607.092804
Yang, F., Fan, Y., Wu, X., Cheng, Y., Liu, Q., Feng, L., et al. (2018). Auxin-to-gibberellin ratio as a signal for light intensity and quality in regulating soybean growth and matter partitioning. Front. Plant Sci. 9:56. doi: 10.3389/fpls.2018.00056
Yang, F., Liu, Q., Cheng, Y., Feng, L., Wu, X., Fan, Y., et al. (2020). Low red/far-red ratio as a signal promotes carbon assimilation of soybean seedlings by increasing the photosynthetic capacity. BMC Plant Biol. 20:148. doi: 10.1186/s12870-020-02352-0
Yoshida, H., Hikosaka, S., Goto, E., Takasuna, H., and Kudo, T. (2013). Effects of continuous lighting and time of initiation of treatments on the flowering time and growth of everbearing strawberry nursery plants in a closed plant factory. J. Sci. High Technol. Agric. 25, 77–82. doi: 10.2525/shita.25.77
Zeipina, S., Alsina, I., and Lepse, L. (2017). Insight in edamame yield and quality parameters: a review. Res. Rural Dev. 2, 40–45. doi: 10.22616/rrd.23.2017.047
Zhang, J., Smith, D., Liu, W., Chen, X., and Yang, W. (2011). Effects of shade and drought stress on soybean hormones and yield of main-stem and branch. Afr. J. Biotechnol. 10, 14392–14398. doi: 10.5897/AJB11.2143
Zhen, S., Haidekker, M., and van Iersel, M. W. (2019). Far−red light enhances photochemical efficiency in a wavelength−dependent manner. Physiol. Plant. 167, 21–33. doi: 10.1111/ppl.12834
Keywords: biomass accumulation, color temperature, cumulative volume, white LED, plant factory with artificial light, plant height, vegetable soybean, vertical farming
Citation: Liu Q, Ke X, Yoshida H, Hikosaka S and Goto E (2024) Optimizing photosynthetic photon flux density and light quality for maximizing space use efficacy in edamame at the vegetative growth stage. Front. Sustain. Food Syst. 8:1407359. doi: 10.3389/fsufs.2024.1407359
Received: 26 March 2024; Accepted: 12 April 2024;
Published: 08 May 2024.
Edited by:
José Pinela, Instituto Politécnico de Bragança, PortugalReviewed by:
Toyoki Kozai, Japan Plant Factory Association, JapanCopyright © 2024 Liu, Ke, Yoshida, Hikosaka and Goto. This is an open-access article distributed under the terms of the Creative Commons Attribution License (CC BY). The use, distribution or reproduction in other forums is permitted, provided the original author(s) and the copyright owner(s) are credited and that the original publication in this journal is cited, in accordance with accepted academic practice. No use, distribution or reproduction is permitted which does not comply with these terms.
*Correspondence: Eiji Goto, Z290b0BmYWN1bHR5LmNoaWJhLXUuanA=
Disclaimer: All claims expressed in this article are solely those of the authors and do not necessarily represent those of their affiliated organizations, or those of the publisher, the editors and the reviewers. Any product that may be evaluated in this article or claim that may be made by its manufacturer is not guaranteed or endorsed by the publisher.
Research integrity at Frontiers
Learn more about the work of our research integrity team to safeguard the quality of each article we publish.