- 1Department of Biological and Chemical Engineering, Aarhus University, Aarhus, Denmark
- 2Teagasc, Johnstown Castle, Environment Research Centre, Wexford, Ireland
- 3Department of Chemical Sciences, School of Natural Sciences, University of Limerick, Limerick, Ireland
- 4College of Science and Engineering, Civil Engineering and Ryan Institute, National University of Ireland, Galway, Ireland
- 5UniLaSalle, Aghyle, Rouen, Rue du Tronquet, Mont-Saint-Aignan, France
- 6Soil Biology Group, Wageningen University and Research, Wageningen, Netherlands
- 7Department of Agroecology, Aarhus University, Tjele, Denmark
- 8Danish Technological Institute, Taastrup, Denmark
Worldwide dairy processing plants produce high volumes of dairy processing sludge (DPS), which can be converted into secondary derivatives such as struvite, biochar and ash (collectively termed STRUBIAS). All of these products have high fertilizer equivalent values (FEV), but future certification as phosphorus (P)-fertilizers in the European Union will mean they need to adhere to new technical regulations for fertilizing materials i.e., content limits pertaining to heavy metals (Cd, Cu, Hg, Ni, Pb, and Zn), synthetic organic compounds and pathogens. This systematic review presents the current state of knowledge about these bio-based fertilizers and identifies knowledge gaps. In addition, a review and calculation of greenhouse gas emissions from a range of concept dairy sludge management and production systems for STRUBIAS products [i.e., biochar from pyrolysis and hydrochar from hydrothermal carbonization (HTC)] is presented. Results from the initial review showed that DPS composition depends on product type and treatment processes at a given processing plant, which leads to varied nutrient, heavy metal and carbon contents. These products are all typically high in nutrients and carbon, but low in heavy metals. Further work needs to concentrate on examining their pathogenic microorganism and emerging contaminant contents, in addition to conducting an economic assessment of production and end-user costs related to chemical fertilizer equivalents. With respect to STRUBIAS products, contaminants not present in the raw DPS may need further treatment before being land applied in agriculture e.g., heated producing ashes, hydrochar, or biochar. An examination of these products from an environmental perspective shows that their water quality footprint could be minimized using application rates based on P incorporation of these products into nutrient management planning and application by incorporation into the soil. Results from the concept system showed that elimination of methane emissions was possible, along with a reduction in nitrous oxide. Less carbon (C) is transferred to agricultural fields where DPS is processed into biochar and hydrochar, but due to high recalcitrance, the C in this form is retained much longer in the soil, and therefore STRUBIAS products represent a more stable and long-term option to increase soil C stocks and sequestration.
Introduction
Mineral phosphorus (P) is a listed European Union (EU) critical raw material due to its importance in food production (European Commission, 2017; Espinoza et al., 2020). As agriculture is the largest consumer of mined P in Europe (1.1 million tons in 2015; Eurostat, 2020), security of supply may be challenging because the source of non-renewable rock phosphate is in geopolitically sensitive regions (Cordell et al., 2009). The dairy processing sector produces P-rich dairy processing sludge (DPS) which, when used directly or in derived secondary products such as STRUBIAS (STRUvite, BIOchar, AShes), may reduce the dependence on mined rock P (Figure 1). The European dairy processing industry processed about 144.6 million tons or 140.4 billion liters of domestic milk in 2020 (Table 1), about 46% more than the USA which is the second largest milk producing country in the world (Agriland, 2020). It is estimated that dairy food processing wastewater treatment can generate up to 20 kg (mean 17.45 kg m−3) DPS per m3 of milk processed (Ashekuzzaman et al., 2019b), which resulted in 2.45 million tons of DPS (wet weight) across the EU in 2020 (Table 1).
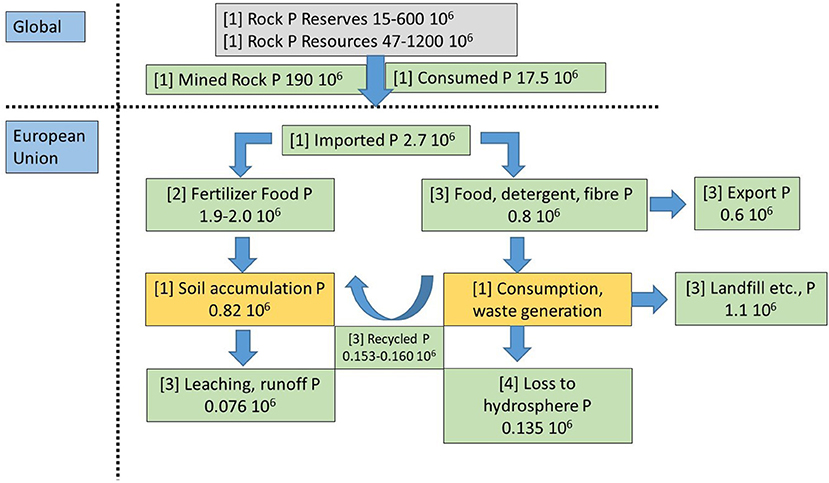
Figure 1. Global P resources and consumption of P and associated P usage streams in the EU in tons per year. Based on Ott and Rechberger (2012), Scholz and Wellmer (2013), and Schoumans et al. (2015).
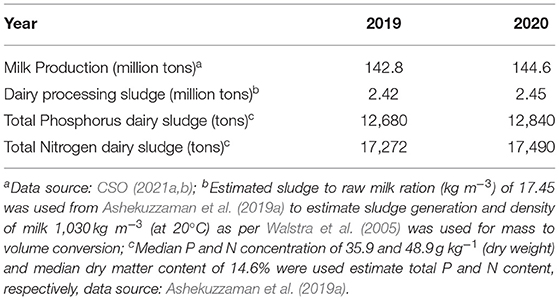
Table 1. EU domestic milk intake and associated estimated dairy processing sludge generation (wet weight) with total phosphorus and nitrogen quantity.
Phosphorus in DPS can be recycled to fields, creating a circular economy, and can be used as a replacement for mineral P fertilizer produced from mined rock phosphate (Mayer et al., 2016). Similar to other organic fertilizers, land application only occurs in growing seasons (Sommer and Knudsen, 2021), meaning that storage is required for extended periods, which increases the cost of management. Storage and land application of DPS is a source of the greenhouses gases (GHGs), methane (CH4) and nitrous oxide (N2O) (Smith et al., preparation)1, and when applied to soil may result in incidental losses of nutrients along surface or near surface pathways (Fenton et al., 2017; Shi et al., 2021a). As production becomes more centralized on a smaller number of larger farms in some EU member states (e.g., Denmark or France), the land bank opportunities for DPS application may become limited depending on the land use change and farmer willingness to accept DPS. Due to the nutrient content value and related transport costs, a land bank radius of about 10 km around a processing plant pertains. This may cause an oversupply to land areas near the processing plant and an increased risk of P losses to water (i.e., critical source areas). In addition to centralization of production, future application rates of fertilizer will be limited by P application, and not by nitrogen (N) rates. Presently, the limits imposed on N application rates have led to significant over-application of P (i.e., P:N ratio higher than the ratio needed by crops) and increased potential for eutrophication in regions with a high proportion of sludge and slurry production (Lu et al., 2012). As an example, in Brittany, France, the issue of P and eutrophication is of major importance due to the local high permeability bedrock (granite, shale and sandstone) and agricultural (high level of livestock density) context, which prevents localized organic fertilizer land application. This means there is not always a match between DPS and suitable land availability (e.g., P-deficient soils) in the local area near the dairy processing plant (Le Noë et al., 2018). Such conditions have created a market for the transport and application of DPS e.g., the total cost of spreading DPS (including transport, analyses, spreading and monitoring) is between 20 and 30 € per ton in France (Laperche, 2014). Any new regulations pertaining to the dairy industry will incentivise producers to reduce the volume and weight of DPS transported over long distances to end users. The post processing of DPS to produce secondary STRUBIAS products is a potential solution in this regard.
The main objective of this systematic review is to collate information that will help give DPS and their secondary products certification as P-fertilizers in accordance with technical proposals for new fertilizing materials under the Fertilizing Products Regulation (European Commission, 2003, 2019; Huygens et al., 2019). The purpose of this systematic review is to contribute to decision making about the most sustainable transformation of these “wastes” into high value bio-fertilizers used by both traditional and organic farmers.
Materials and Methods
Search Criteria
All search results were evaluated using the PRISMA statement (Page et al., 2021). In a systematic review, information was collated to answer the following two research questions: (1) how DPS and STRUBIAS production on in-situ and ex-situ treatment and processing units affect product characteristic, fertilizer replacement value (FEV) and P dynamics in soil, risks of GHG emissions and pathogen and heavy metal pollution? (2) how to combine this information to develop production systems necessary to certify DPS and STRUBIAS products as P-fertilizers in accordance with technical proposals for new fertilizing materials under the Fertilizing Products Regulation? A comprehensive systematic literature search of three online databases was performed, Scopus (www.scopus.com), PubMed (https://pubmed.ncbi.nlm.nih.gov/) and Web of Science (https://apps.webofknowledge.com). Searches were conducted in English on literature from 1983 to (1 July) 2021. All research articles related to DPS and STRUBIAS were identified. In addition, Google Scholar was used to find reports pertaining to some sections of this review. The search terms and keywords used to identify research studies were: dairy processing sludge/waste, fertilizer replacement value, phosphorus, circular economy, treatment, characterization, composition, heavy metals, greenhouse gas, methane, nitrous oxide, E. coli, and PAHs (Polycyclic Aromatic Hydrocarbons). Studies that did not contain an abstract in English were excluded from this study during the screening stage. As there were not enough published studies dealing with DPS and STRUBIAS, no meta-analysis was possible for this paper.
Screening of Search Results
Duplicates were removed manually and abstracts were screened by two screeners against the target research questions. Exclusion criteria, described below, were developed and selected and cross-checking was performed on these excluded articles. If disagreements between the two screeners occurred, a third screener adjudicated. Full-text review was independently conducted by three reviewers and reasons for exclusion were annotated and tracked (e.g., “data pertaining to a different waste other than DPS or STRUBIAS”). The primary reasons for excluding papers were: (i) articles completely un-related to search questions; (ii) general knowledge papers; and (iii) papers that did not follow the basic criteria of scientific research (e.g., experimental design with sufficient replication). Articles clearly meeting the inclusion criteria were obtained for full-text review unless unavailable. These included articles related to the search inquiry, providing that scientific laboratory experiments or field studies had a minimum number of replicas and a negative control. Articles were not considered further when their title and abstract clearly indicated that the study did not meet the inclusion criteria (see Supplementary Figure 1). The studies included in tables consist of those that were reviewed in detail.
Greenhouse Gas Emission
Some data needed for the holistic review were not available in the literature and needed to be developed outside of the systematic review within the present study. Greenhouse gas emission from DPS sludge and secondary STRUBIAS products was calculated using a whole systems approach. Herein, methods presented calculate CH4, N2O, and ammonia (NH3) emissions from sludge and secondary STRUBIAS products from production to field application. Ammonia emission is included in the calculation due to risk of N2O emission from NH3 deposition to land. In this analysis, various conceptual scenarios (from production to field application) are compared with the aim to find potential scenarios that can minimize or eliminate emissions.
Assumptions for management of sludge and STRUBIAS products for a Danish dairy production site are used in the scenarios as follows: each month 1/12 of 1 ton of annual produced standard sludge is transferred to a store and the temperature in the stored sludge is similar to the monthly average air temperature of Denmark. The tanks with stored sludge are emptied at the start of April and subsequently land applied to fields in April. In the scenarios where sludge is processed to biochar or hydrochar, the same monthly amount is treated and the products are land applied in April. The annual amount of DPS treated is 1 ton.
In the calculations a 100-year global warming potential (GWP100) for CH4 were set to 34 kg CO2eqv kg−1[CH4] and N2O to 298 kg CO2eqv kg−1[N2O] (Myhre et al., 2013).
Calculation of CH4 Emission
Methane emissions from stored liquid sludge is calculated with the CH4 emission model as used in the Integrated Farm Systems Model (IFSM) for manure management in beef and dairy production systems (Chianese et al., 2009). This has been used previously to assess the impact of GHG reduction strategies in agriculture (Rotz and Hafner, 2011; Dutreuil et al., 2014). Equation 1 is used to calculate CH4 emission from anaerobic stored livestock liquid manure and digestate from biogas plants if concentration volatile solids (VS) and air temperature is known (Baral et al., 2018):
where FCH4 is CH4 emission rate (g CH4 kg−1 VS day−1), A is the pre-exponential factor of 31.2 g CH4 kg VS−1 h−1 (Petersen et al., 2016), Ea the apparent activation energy set to 81 kJ mol−1 (Elsgaard et al., 2016) giving the temperature response of CH4 production. R is the gas constant (8.314 J mol−1 K−1) and T the temperature (K). The degradable fraction VSD is 56% of VS in the sludge (Smith et al., preparation)1. This equation and the parameters presented here is used to calculate CH4 emission from stored DPS.
Emission of NH3 and N2O
In the Danish GHG emission inventory (Nielsen et al., 2018), it is assumed that 0.5% of total N is emitted in form of N2O from stored liquid manure and sludge, and 1% of total-N is emitted from slurry and sludge applied to soil [based on the standard emission factors given by IPCC (2019)]. Another assumption is that N2O emissions from soil N are unaffected by any application of biochar or hydrochar applied to soil. When struvite is applied, it is assumed that 1% of the ammonium (NH+4) is emitted as N2O as is the case for mineral fertilizer N applied to soil (IPCC, 2019).
During storage of the sludge 34% of the proteins are transformed to total ammonium nitrogen (TAN = NH3 + NH+4) (Mottet et al., 2010) and may be emitted from the storage. Emission of NH3 from stores with a cover of PVC roof are set to 2.6% of TAN i.e., similar to emissions from covered stored liquid manure (Hansen et al., 2008) and of sludge injected into black soil to 2% of TAN (Olesen et al., 2020). The negative charge of biochar will contribute to a negligible NH3 emission from soils to which hydro-biochar is applied (Chu et al., 2019), biochar from pyrolysis do not contain TAN and the NH+4 in struvite is assumed not to volatilize due to the low pH (Sommer et al., 2004). At deposition on land or water, a fraction of NH3 will be transformed to N2O and be emitted to the atmosphere. This indirect N2O emission is estimated using Equation 2 (IPCC, 2019):
where FN2O is given in kg N2O, FNH3 is given in kg NH3-N emitted, 0.01 is a default factor given by the IPCC for calculation of the climate warming effect of emitted NH3, and 44/28 is to calculate from concentration given in 2*N g mol−1 to 2*N + O g mol−1 (IPCC, 2006).
Carbon Sequestration
During storage of untreated sludge, a fraction of C is lost in form of carbon dioxide (CO2) and CH4. This emission is calculated assuming that 2.6 kg VS is lost in the form of CO2 and CH4 for each kg of CH4 produced. The sludge is heated in hydrothermal carbonization due to the oxidation of C, and in this process about 30% of C is lost in the form of CO2, and about 70% of C in treated biomass is retained in the hydrochar (Kambo and Dutta, 2015). In the pyrolysis process, more CO2 is lost and between 25 and 35% (avg. 30%) of solids (40% C) are retained in the biochar (Kambo and Dutta, 2015).
In the calculations used herein, the C retention of field applied sludge-C was set to 25%, which is between 12% of manure C input in the longer term (avg. 18 years; Maillard and Angers, 2014) and 35% for a 20-year period by scaling results from a study of transformation of C in digestate applied to soil (Thomsen et al., 2013). The longer retention time of C in sludge than in animal slurry was due to sludge organic matter (OM) from a wastewater treatment plant that has been transformed to a more stable form of C. It is calculated that C concentration in VS is 517 g C kg VS−1, and this estimate is used to calculate C concentration in sludge, where OM is measured as VS.
It has been calculated that between 90 and 97% of the C in biochar from pyrolysis of a range of different biomasses will still remain in the soil after 100 years (Lehmann and Joseph, 2015; Wang et al., 2016). No study of the recalcitrance of biochar from pyrolysis of dairy sludge was found in the literature, and a conservative/cautious estimate is that 90% of the C in biochar from pyrolysis (PC) of dairy sludge is recalcitrant.
Hydrochar from hydrothermal carbonization (HTC) is produced at a lower temperature than when producing biochar by pyrolysis, and C component in the hydrochar has been oxidized less than pyrolysis products, i.e., less oxygen is added during the process. Our assumption is that C in hydrochar is less recalcitrant than biochar produced by pyrolysing dried biomass, and that only 50% C in hydrochar is recalcitrant. This assumption is supported by the study of Malghani et al. (2013), who stated that “although both HTC and PC chars were produced from the same feedstock, PC chars had markedly higher potential for carbon sequestration than HTC.”
Results and Discussion
Treatment Options at Dairy Processing Plants and Volumes and Composition of DPS
The dairy processing industry generates a large volume of waste, which is high in OM. Discharge licensing, which is site specific, aims to prevent a reduction in surface water oxygen (O2) concentrations and the onset of surface water eutrophication (Neal and Heathwaite, 2005). To achieve such discharge thresholds, a chain of treatment before discharge at dairy processing plants is needed (Figure 2). In fact, most DPS applied to land in the EU is first treated, composted or incinerated before being applied to land (Figure 2). Research now focusses on converting DPS into secondary STRUBIAS products (Figure 2).
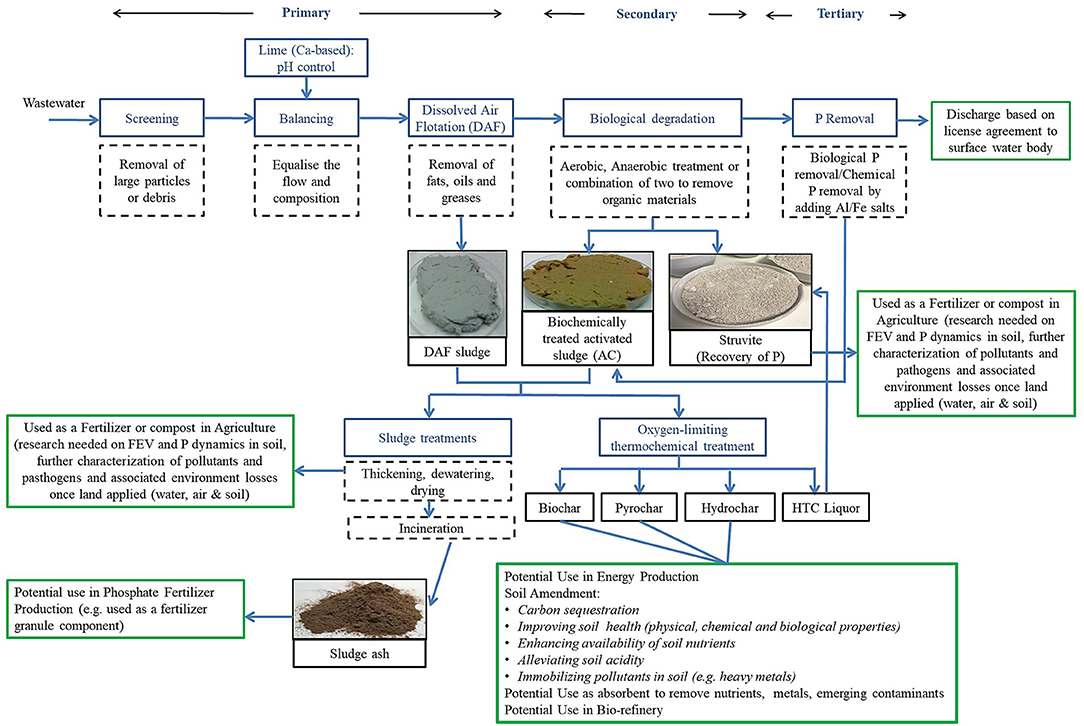
Figure 2. Dairy wastewater treatment flowcharts showing DPS and STRUBIAS products [modified from Shi et al. (2021a)].
Dairy Biological Wastewater Treatment
A schematic of typical dairy wastewater treatment processes and DPS generation is shown in Figure 2. Pre-treatment removes particulate matter and retains fats that could interfere with subsequent treatment. The pre-treatments and physio-chemical treatments most frequently used on dairy processing plants are buffer tanks, neutralization, sieving, flotation and degreasing (Droste and Gehr, 2018). Flocculation (coagulation) is the most simple and economical pre-treatment method, and reduces water turbidity by reducing particulate substances and fats, which could interfere with subsequent treatments (Carvalho et al., 2013). Pre-treatment by flocculation enhanced by the presence of lactic acid bacteria (Lactobacillus plantarum), which ferment the lactose and produce lactic acid. The acidity of this compound precipitates the milk proteins, and thus significantly reduces the chemical oxygen demand (COD). This reduction increases with addition of flocculants such as chitosan or carboxymethyl cellulose (CMC) to this solution. The COD removed varied by 65–78% for CMC and 49–82% for chitosan (Dyrset et al., 1999).
In the secondary treatment, sludge undergoes aerobic treatment that is cost efficient and controllable (Kolev Slavov, 2017). This treatment step can reduce more than 90% of COD (Carta-Escobar et al., 2004; Kushwaha et al., 2011; Carvalho et al., 2013). In aeration ponds, the aerobic pathway uses microorganisms contained in biological reactors, which in the presence of O2, degrade the OM suspended in the sludge.
The soluble organic components transformed and emitted mainly in the form of CO2 and NH3, while the insoluble pollution fraction, including the microorganisms, is recovered by separation and form “activated” sludge (Sustarsic, 2009). A large number of aerobic treatment types are being used e.g., rotating biological contractors (RBCs), sequencing batch reactors (SBRs), or membrane reactors (MBRs) (Goli et al., 2019). In particular, SBRs are effective at varying loading capacities (Kolev Slavov, 2017). In this step, a single tank used for filling, aeration, settlement and effluent withdrawal, and recycling of solids. The sludge produced during primary and secondary treatment may be treated anaerobically in biogas reactors. During this process, OM is transformed by microorganisms to CH4 and CO2. This process produces small volumes of sludge (<0.05 kg of dry matter (DM) per kg of COD eliminated) (Omil et al., 2003). The most common anaerobic reactors are up-flow anaerobic sludge blanket (UASB), completely stirred tank reactors (CSTRs) and membrane anaerobic reactor systems (MARSs). Finally, where needed, the N, P, micropollutants or pathogenic microorganisms in the wastewater can be further reduced using a finishing treatment e.g., for P content reduction alternation between aerobic/anaerobic conditions or P coagulation and precipitation by ferric chloride or aluminum sulfate can be deployed (Rivas et al., 2010). After these treatments, the particles settle in a clarification pond. The supernatant is discharged, while the precipitated sludge is dewatered after addition of polymers to promote flocculation and separation between water and suspended matter (dewatering). This is then thickened (thickening) during its passage through dewatering grids. This sludge is stored in tanks or treated further and then used as a bio-based fertilizer (Huygens et al., 2019).
Wastewater Volumes and Contents
The volume of wastewater produced at processing plants can be high and is product-dependent. For example, 1 liter of processed milk can produce up to 10 L of effluent (Lateef et al., 2013). In the cheese manufacturing industry, the whey is the main pollutant discharged to water and soil. This is mainly due to its high carbohydrate content (4–5%) of which lactose is the main constituent (Kolev Slavov, 2017). Consequently, DPS produced from cheese production, called Cheese Whey Wastewater (CWW), contains high concentrations of organic components contributing to a high COD and biological oxygen demand (BOD) (Ahmad et al., 2019). The COD of CWW is higher (0.79-77.3 g L−1) than COD of milk plant effluent (0.183–10 g L−1) (Carvalho et al., 2013) or milk-treated condensate wastewater (<0.001 g L−1), which is the water obtained during the concentration and evaporation processes of milk and its by-products (Bourbon and Huet, 2018). The CWW contains some milk or milk by-products, oils and greases, and cleaning water containing sterilizing agents, acid and alkaline detergent (Carvalho et al., 2013; Ahmad et al., 2019). As a result, the concentration of inorganic compound may be heterogeneous, with ranges observed for P from 8 to 510 mg L−1 and N from 14 to 1,462 mg L−1 (Demirel et al., 2005).
The amount and composition of DPS is determined by the composition of milk and use of additives, the dairy production line and cleaning and disinfectant products used for cleaning. In addition, wastewater treatment, varies between plants (Figure 2), and depends on the type of dairy processing involved (Rico Gutiérrez et al., 1991; Karadag et al., 2015; Ahmad et al., 2019; Shi et al., 2021a). Recently, physical and chemical characteristics of 63 DPS samples (9 dairy processing plants in Ireland) were reported (Ashekuzzaman et al., 2019a). The main DPS types included in the study were bio-chemically treated activated sludge leading to aluminum-precipitated sludge (Al-DPS) and iron-precipitated sludge (Fe-DPS) depending the dosing of alum or ferric salt to remove P, and to lime-stabilized calcium-precipitated sludge (Ca-DPS) generated after dissolved air floatation (DAF). In some processing plants, mixing of DPS generated from aerobic biological wastewater treatment and DAF processes occurs before land application or further disposal processing. A few of the examined plants have anaerobic digesters (AD) that produced AD sludge. That concentration of components varies between sludge categories and treatments and differences in composition was highest for N, P and K (Ashekuzzaman et al., 2019a). This difference is higher between activated and DAF sludge types than those in the combined sludge (sludge mixed from both activated and DAF process). The addition of chemicals such as Fe-, Al-, or Ca-based coagulants during the removal of P from the effluent may explain these results. In addition, the N concentration was lower in DAF sludge compared to AD sludge in contrast to the increased P concentration (Ashekuzzaman et al., 2019a). The addition of lime during the DAF process explains this result by an increase of the pH, which promotes the volatilization of N in the form of NH3. On the other hand, anaerobic degradation during the AD process increases the TAN concentration in the sludge due to organic N mineralization.
Tables 2, 3 illustrate the physicochemical characteristics of dairy processing effluents and DPS reported in the literature. These characteristics highlight the variability in dairy effluent compositions related to the type of bio-products processed (i.e., milk, cheese, yogurt, and butter) (Omil et al., 2003; Carvalho et al., 2013; Karadag et al., 2015), and the wastewater treatment processes used (Britz et al., 2006; Ashekuzzaman et al., 2019a).
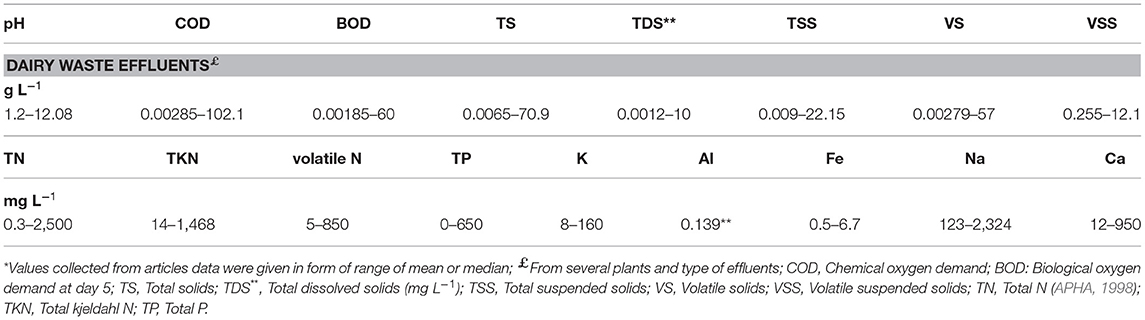
Table 2. Physicochemical characteristics of dairy waste effluents* (Danalewich et al., 1998; Omil et al., 2003; Demirel et al., 2005; Byrne, 2011; Carvalho et al., 2013; Karadag et al., 2015; Kolev Slavov, 2017; Verma and Singh, 2017; Goli et al., 2019; Ferreira et al., 2021; Sivaprakasam and Balaji, 2021).
In France, information and data pertaining to the agronomic benefits and risks of applying organic waste to agricultural soils was collated by Houot et al. (2014) and used to re-evaluate the EU Sewage Sludge Directive (86/278) (CEC, 1986). This work emphasized the need for sludge used in agriculture to have P recycling as a main priority, and that this use must not be a risk to the environment or to human health due to their contents of heavy metals, organic trace compounds, pathogenic microorganisms and pharmaceutical compounds. To avoid some of these concerns, the EU Council Directive 86/278/EEC set limits for the content of heavy metals (Cd, Cu, Hg, Ni, Pb, and Zn) (CEC, 1986), and individual European countries have set limits for synthetic organic compounds and pathogens (Hudcová et al., 2019).
In a comprehensive study across nine Irish dairy plants, the concentration of heavy metals (i.e., Cr, Cu, Ni, Pb, and Zn) was examined in all major DPS types with lowest concentrations found in DAF sludge and highest in AD sludge (Ashekuzzaman et al., 2019a). Overall, the heavy metal concentrations across all tested DPS samples were significantly lower than limits set by the EU for avoiding accumulation in agricultural soil to which sludge is applied (CEC, 2008) and the levels were below those of livestock manure (Sommer et al., 2013), and composts (Bernal et al., 2017). The results of the Irish study are in line with the current knowledge on heavy metals content of DPS (Table 4) and indicates that heavy metal concentrations will not be a limiting factor for legal and safe application rate of DPS to agricultural soils (Ashekuzzaman et al., 2019a; Shi et al., 2021b). The concentration varies between dairies and this is due to the diversity of the milk bio-products and the various possible steps in the treatment of the effluent. It is important to have knowledge pertaining to the heavy metal content of DPS and DPS-derived STRUBIAS products before land application, because farmers and society must be assured that the heavy metal content is lower (in soil and plants) than the limits given for use before making final decisions and rules of use of the waste as a fertilizer (Shi et al., 2021b).
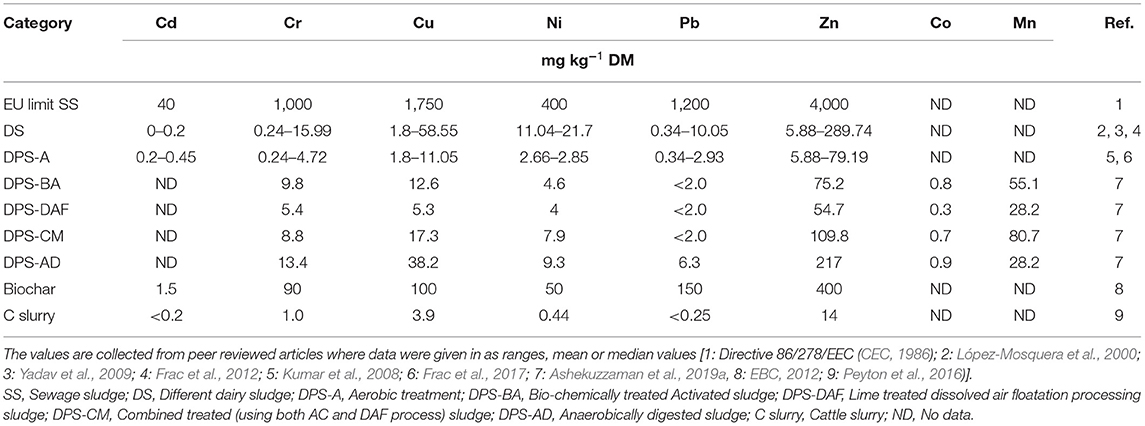
Table 4. Concentration (mg kg−1 dry weight) of heavy metals in DPS, comparison with European Union (EU) regulation upper limit values for sewage sludge (SS) and a range of organic fertilizers.
Organic Trace Compounds (OTCs) are chemical products (hydrocarbons and their derivatives, degradation products, solvents, etc.) present in organic waste or derived due to degradation of the organic compounds by the microorganisms in sewage treatment plants or in the soil. They often accumulate by biomagnification and bioaccumulation in biological organisms and cause irreversible damage to biological systems. They are directly or indirectly toxic to humans and animals (such as endocrine disruption and tumor initiation) (Barret et al., 2012). Table 5 presents the European Commission limit values for organic contaminants (CEC, 2009).
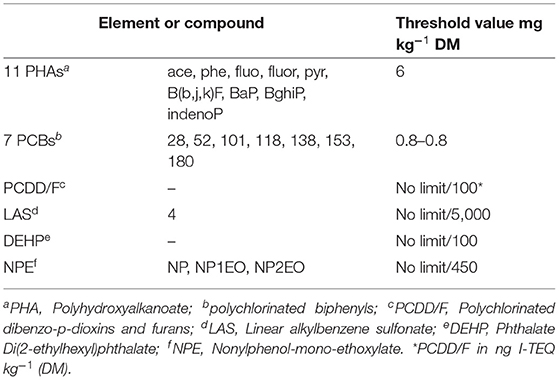
Table 5. Threshold values of organic contaminants in organic wastes that may be recycled to soil for crop production [option 2-3 of the 2009 European report (CEC, 2009)].
In Europe, there are proposals for limits on polycyclic aromatic hydrocarbons (PAHs) contents in municipal sewage sludge applied to land (CEC, 2010). Depending on the country-specific regulations, the type of OTCs and the threshold limits differ. Where organic waste is land applied, the following three PAHs i.e., fluoranthene 5, benzo(b)fluoranthene 2.5, benzo(a)pyrene 2 and 7 PolyChloroBiphenyls (PCBs) (PCB 28, 52, 101, 118, 138, 153, 180) are considered good indicators of compound resistant to biodegradation, and according to French regulation must be below concentration limits (Hudcová et al., 2019). In contrast, German authorities do not regulate PAHs, while the threshold for the sum of nine PAHs is more stringent in Denmark than for the sum of three PAHs in France. Companies that handle wastes must be aware that the concentration of 16 PAHs depends on the type of sludge (Boruszko, 2017). Research has shown that anaerobic fermentation and post-flotation may reduce the content of PAHs up to seven times its original concentration (e.g., 689 μg kg−1 of DM) (Boruszko, 2017), with most of the reduction of hydrocarbons taking place in the final phase of fermentation. The concentrations of PAHs in DPS are low and do not exceed the amount allowed by the European Commission (CEC, 2009; Table 5). Therefore, their use in agriculture will not be limited by PAHs (Pérez et al., 2001; Boruszko, 2017), but it is recommended that these are investigated in DPS-derived STRUBIAS products.
DPS contains living microorganisms originating from the treated wastewater. There are pathogens (viruses, bacteria, fungi and parasites) derived from animal manure (Sobsey et al., 2006), and if dairy cows ingest grass from fields where dairy manure has been applied, then there is a risk for transfer of pathogens in infectious levels to the cows and subsequently to milk and therefore to wastes. The European Commission (CEC, 2000) has in its third draft of the Working Document on Sludge, proposed the following thresholds for a range of bacteria and worms for sludge to be recycled to soil: (1) E. coli <5 × 105 colony forming units (CFU) per gram (wet weight) of conventional treated sludge; (2) for advanced sanitized sludge E. coli must be below 1 × 103 CFU g−1 wet colony of treated sludge; (3) Salmonella Senftenberg W775 in sludge spiked with this microorganism must be reduced 99.99%; (4) no content of Ascaris ova (5) a sample of 1 g DM of the treated sludge must not contain more than 3 × 103 spores of Clostridium perfringens, and (6) a sample of 50 g (wet weight) of the treated sludge must not contain Salmonella spp.
There are few studies reported about reduction of pathogens in raw DPS. Laboratory studies have quantified reductions of microbial infectivity (inactivation) in animal organic wastes under controlled temperature conditions and the samples have been stored aerobically or anaerobically (Sobsey et al., 2006). For example, the high initial level of pathogens (Enterobacteriacea, fecal coli forms and E. coli) in dairy slurry was higher than in sludge from two urban wastewater treatment plants where anaerobic digestion was followed by mechanical dehydration (one treatment also received a heat-dried process). The concentrations in treated dairy slurry were 5.1 × 107 CFU g−1 of DM of Enterobacteriacea; 4.4 × 107 CFU g−1 of DM of fecal coli forms, and 4 × 106 CFU g−1 of DM of E. coli and all are below the limits set by the regulation. Concerning the persistence of these pathogens in the soil, then after a 80-day trial across soil/sludge treatments, the populations of fecal coliforms and E. coli decreased considerably or were not detectable (Estrada et al., 2004). The study of Ravva et al. (2006) also found that the pathogenic strain E. coli O157:H7 introduced in water from on farm dairy waste lagoons, failed to establish and proliferate in dairy wastewater microcosms with or without circulating aerators. On the other hand, high concentrations of Listeria are found in manure and sewage sludge and have survived in topsoil between 12 and 182 days (Sobsey et al., 2006). If these are present in DPS, then additional sludge treatments such as anaerobic digestion, hygienization by adding lime, or composting will reduce the concentration of pathogens to allowable values.
Dairy processing wastes (even after treatment at source) may contain harmful substances, which need testing and quantification across all the DPS and STRUBIAS types. The substances in focus should be antimicrobial drugs, hormones, pesticides, emerging contaminants, pathogens, disinfectants, persistent organic pollutant residues, microplastics, and nanoparticles in DPS or DPS-derived STRUBIAS (Shi et al., 2021a).
DPS-Derived STRUBIAS Production
Another strategy that is being deployed to manage DPS is to further process these raw products into other more usable and stable forms. Struvite (magnesium ammonium phosphate hexahydrate, MgNH4PO4, 6H2O) is widely used in agriculture due to its N and P content, which is in a form that efficiently can be used by plants (Adam et al., 2009). Phosphorus can exist as particulate and dissolved species in both organic and inorganic forms. The inorganic P species is mainly in orthophosphate form, which is plant available and important for soil fertility but can be readily lost to the environment (Frossard et al., 1996). However, the chemical composition of struvite obtained from DPS is not always consistent with pure struvite equivalents (Hall et al., 2020). Metal impurities such as Al, Fe, Ca, and small amounts of heavy metals can precipitate along with the struvite and could pose problems later for crops and soil when land applied.
The term “char-based materials” is used here to replace “biochar” in the STRUBIAS acronym as they have different terms depending on the technology. Char-based materials, obtained from the thermochemical conversion of biomass in an oxygen-depleted atmosphere, are porous and carbonaceous, and are more stable and C-rich and less toxic than the feedstock (Kambo and Dutta, 2015; Atallah et al., 2020). The significance of thermochemical treatment lies in overcoming the structural inferiority of biomass, which enhances the chances of energy and resource recovery from waste (Kambo and Dutta, 2015). There are many functions of char-based materials including, but not limited to, energy production, agriculture, C sequestration, wastewater treatment, and bio-refinery (Kambo and Dutta, 2015). The utility of a specific char-based material for any particular application depends on its inherent properties. Feedstock, pre-treatment method, and temperature are all important (Amoah-Antwi et al., 2020). However, thermochemical treatments increase the risk of producing chars with other highly toxic compounds produced from high-temperature reactions such as PAHs, PCBs, dioxins, furans, and PCDD/Fs (Kambo and Dutta, 2015; Amoah-Antwi et al., 2020). Heavy metals present in the feedstock are most likely to remain and concentrate in the chars (Shackley et al., 2010).
Ashes are characterized as fly ash or bottom ash, or a combination formed through the incineration of biowastes by oxidation (Huygens et al., 2019). Ash normally contains valuable plant macronutrients such as K, P, S, Ca, and Mg (Haraldsen et al., 2011; Knapp and Insam, 2011; Brod et al., 2012). In addition, they contain large amounts of P (13.7–25.7% P2O5), which are comparable to commercial superphosphate (Xu et al., 2012). Obstacles to the use of ash as a fertilizer or soil amendment could be their heavy metal contents (Franz, 2008; Herzel et al., 2016).
DPS and DPS-Derived STRUBIAS as Fertilizers
DPS and DPS-derived STRUBIAS products are used or research is underway to ascertain their potential as bio-based fertilizers in agriculture (Shi et al., 2021a). DPS is typically stored off site until applied to land in spring, whereas STRUBIAS products can be processed and stored until needed. Many knowledge gaps still exist pertaining to their respective effects on P dynamics once spread onto soils and their FEV. It should be noted that FEV is used herein (Shi et al., 2021a), but can be often known in the literature as Mineral Fertilizer Equivalence (MFE; Delin, 2012) or Mineral Fertilizer Replacement Value (MFRV; Schröder et al., 2007).
Phosphorus Dynamics in Agricultural Soils
In cropped agricultural systems, P applied to soil with fertilizers can be utilized by crops, absorbed by soil minerals (González Jiménez et al., 2019), or lost along surface (runoff) or subsurface (leaching and loss along natural or artificial lateral transport or deeper recharge to groundwater) pathways to surface waters (Murnane et al., 2016). Soil P transformation passes through several interconnected pools. These are the soluble P pool, which is considered to be immediately available for plants; labile or weakly adsorbed P, insoluble P chemically bound with Ca ions in calcareous and alkaline soils or occluded by Fe and Al oxides in acidic soils, P strongly adsorbed by hydrous oxides of Fe and Al, and insoluble organic P within soil organic matter (Stevenson and Cole, 1999; Bennett and Carpenter, 2002). Figure 3 presents a simplified diagram reflecting P soil cycling and interactions between these pools. Briefly, the soil P cycle consists of the following processes: weathering and precipitation, mineralization and immobilization, adsorption and desorption, and P losses through surface or near surface runoff and subsurface leaching with eventual recharge to groundwater (the proportions of which are dependent on soil/subsoil/bedrock permeability and chemistry).
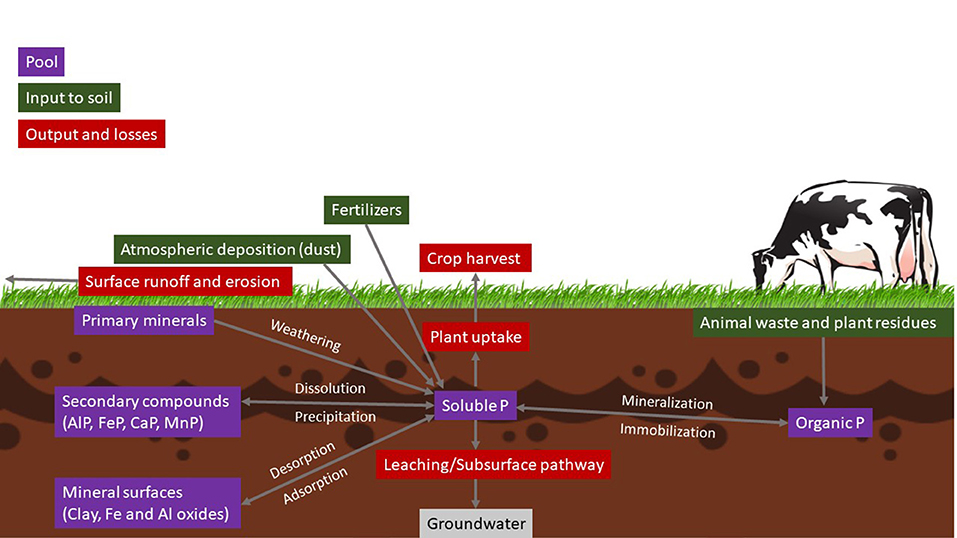
Figure 3. Soil phosphorus turnover in soil and pathways of P loss to waters on agricultural landscapes.
Mineralization and immobilization of P are part of the organic P cycle. Mineralization is a process of transformation of organic P to soluble H2PO-4 or HPO2-4. Mineralization of organic P slowly releases soluble P, which is crucial during the growing season as it provides a continuous supply of P to crops. Mineralization of organic P in soil occurs through breakdown of organic bonds, which is driven by the release of enzymes produced by plants and soil microflora. Organic P mineralization is driven by phosphatase enzyme activity in soil, which mainly occurs during the growing season when soil temperature ranges between 18 and 40°C (Prasad et al., 2016). Phosphatases synthesis are believed to be driven by P availability and enhances under P limiting conditions (Luo et al., 2017). However, in some cases application of mineral P (Paredes et al., 2011), and organic amendments of soil (Parham et al., 2002) can increase phosphatases activity. Some other factors which have an impact on phosphatases production are P availability and availability of other soil nutrients (Marklein and Houlton, 2012), soil moisture, pH, and availability of other soil nutrients, and energy supply (Acosta-Martínez and Waldrip, 2014; Prasad et al., 2016).
Precipitation and dissolution and desorption and absorption are part of inorganic P cycle. The direction of P transfer between inorganic P soil pools though precipitation and dissolution can be either reversible or irreversible, and can be impacted by a number of factors, including geochemical soil composition and soil pH. For instance, in acidic soils P precipitation occurs in the presence of Fe, Al, and Mg, and soluble P in such soil can be limited, while in alkaline soils precipitation primarily occurs through reactions involving Ca2+ compounds (Prasad et al., 2014). Adsorption, or fixation, binds soluble P compounds to soil particles, whereas desorption releases P which is bound with soil minerals to soil solution, thereby increasing the soluble P pool. Unlike precipitation, this process is reversible, and P does not involve permanent change in chemical and structural changes in P-containing compounds and soil minerals.
The consideration of the aforementioned P fluxes in agricultural soil and recycled DPS composition is essential for developing guidelines of alternative P fertilizers. Specifically products derived from chemically treated dairy effluents treated with lime, ferric sulfate or aluminum chloride may contain elements, which can limit P release into available P pool such as Ca, Fe, and Al (Ashekuzzaman et al., 2019a). An inherent soil pH range optimal for P fertilizers to remain in soluble pool is between 6 and 7.5. Decreasing soil pH can lead to soluble P fixation by Fe and Al oxides. While fixation can be a limiting factor for soil P availability for crops (Daly et al., 2015; Prasad et al., 2016), fixation of P by minerals present in the soil is also a limiting factor. To ensure sustainable use of the P source and avoid P losses into the environment, such best practice should be followed (Science Communication Unit University of the West of England Bristol, 2013; Arenas-Montaño et al., 2021).
Fertilizer Equivalent Value of DPS and DPS-Derived STRUBIAS
The FEV defined as the equivalent application rate of an inorganic fertilizer achieved by an organic waste to achieve the same crop yield or nutrient uptake (Brod et al., 2012). The efficiency of most bio-based organic fertilizers is lower than inorganic fertilizers because of their slow nutrient release rates (Chen, 2006). The FEV of an organic fertilizer can both provide a quantitative estimate of the amount of efficient nutrients in bio-based fertilizer and estimate of the actual value when compared with a chemical equivalent. This information, which is currently lacking, would give growers accurate information to help with nutrient management planning on farms.
Two methods used to assess the FEV of bio-based fertilizers such as DPS or DPS-derived STRUBIAS products, are pot or field-scale studies, which include different fertilizer rates, crops, and soils. The most common method is to compare yields or nutrient uptake results from DPS or DPS-derived STRUBIAS treatments with uptake from commercial mineral fertilizers as used with other organic fertilizers e.g., Lalor et al. (2011) examined the FEV of dairy cattle slurry. Typically, data fitted to linear, quadratic, or cubic polynomial regressions, creates a relationship equation. For example, Figure 4 illustrates a fitted polynomial function, describing crop yield or nutrient uptake corresponding to different mineral fertilizer application rates. This is the method used to determine the corresponding mineral fertilizer rate (×1) to any crop yield or nutrient uptake by a bio-based application. The mineral fertilizer rate, ×1, expressed as a percentage of total nutrient applied from that bio-based treatment and estimates the FEV. Alternatively, calculation of FEV by the apparent nutrient recovery method without the need of a response curve is used. There is, however, a difference between apparent N or P recovery (ANR or APR) and N-P FEV. The first is the N or P fraction taken up by the test crop of total applied nutrients and the second is the ratio of the apparent N and P recovery of bio-based fertilizer and that of mineral fertilizer at the same rate (Cavalli et al., 2016; Sigurnjak et al., 2019). They are determined as follows using Equations 3–6:
The most comprehensive grassland study on the FEV of DPS, conducted by Ashekuzzaman et al. (2021a,b), examined two main types of DPS. The first is aluminum or iron-precipitated activated sludge (Al- or Fe-DPS) and the second is a lime-stabilized calcium-precipitated sludge (Ca-DPS). At field scale, an assessment of N and P availability for crop yield and uptake in comparison to reference mineral fertilizers over one seasonal year was undertaken. Ashekuzzaman et al. (2021b) found N-FEV of 22–25, 54, and 8%, respectively, for Ca-DPS, Fe-DPS, and Al-DPS. They indicated that N-FEV varied between activated and lime treated DPS types, as affected by wastewater and sludge treatment processes and storage. The different treatments affect the proportion of mineral and organic N in the DPS, and thus the available N pool in amended soil. With regards to P availability, the results of Ashekuzzaman et al. (2021a) show that first-year cumulative P availability (over the four harvests) differs significantly between Al- and Ca-DPS where Al-DPS P-FEV was 109% compared to mineral P (applied at 40 kg P ha−1) and Ca-DPS P-FEV was only 31%. Their findings show that mineral P fertilizer was a better starter fertilizer that at application provided more readily available P for plant uptake than either Al-DPS or Ca-DPS, as they observed 50 and 16% P-FEV for the two DPS, respectively, in the first harvest. Although the Al concentration (1,122 mmol kg−1) in Al-DPS did not limit first-year P bioavailability, the initial nature of P fractions, and their biological and bio-chemical mineralisation processes, might be the reason of lower P availability for immediate uptake by plant. For Ca-DPS, high Ca content (Ca/P molar ratio 1.86) and alkaline pH in Ca-DPS was likely to be associated with formation of low soluble Ca-P compounds and low P availability. Future studies on the aspect of P composition and mineralisation process in DPS would help to realize and correlate P uptake efficiency.
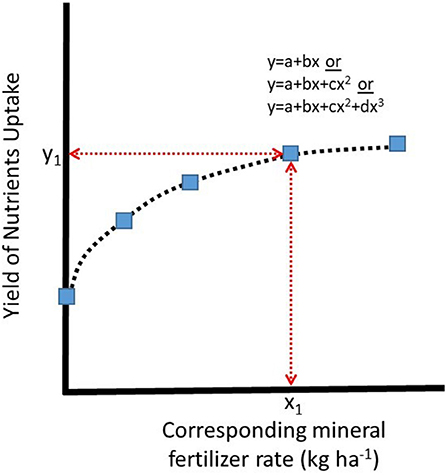
Figure 4. Illustration of a FEV idealized response curve, where “a” is the intercept (crop yield or nutrients uptake at 0 kg ha−1 of mineral fertilizer); “b, c, and d” are the linear, quadratic, and cubic coefficients, respectively.
Other literature pertaining to the FEV of DPS, and especially DPS-derived STRUBIAS products is still limited. Many factors affect the calculation of FEV such as the treatment processes used to produce a DPS or DPS STUBIAS type (crop type, fertilizer application rate, duration of experiment and scale of experiment (pot vs. field) (Brod et al., 2012; Cerný et al., 2012).
The dosing of Al or Fe salts used to capture P in sewage wastewater treatment plant affect P-FEV, because high concentrations (more than 2,800 mmol kg−1) of either or both may significantly reduce P bioavailability (Khiari et al., 2020). The P fertilizer effects of 14 different bio-based fertilizers had been tested in pot experiments with ryegrass (Delin, 2016). At the first cut, the P-FEV of Fe- and Al-precipitated sewage sludge were 37 and 33%, respectively (Delin, 2016). Falk Øgaard and Brod (2016) found that P-FEV of 11 sewage sludges treated with Al and/or Fe salts varied significantly between sludges, but was low for all sludges in a pot experiment with ryegrass. It was lowest at first cut, where it ranged from 2 to 24% (Falk Øgaard and Brod, 2016). Both studies indicated that sludge derived from a treatment with Fe had a higher P-FEV than when coagulation with Al salts occurred. This is due to a higher solubility of Fe phosphate compared to Al phosphate. The amount of Fe used is also important and sludge with a Fe:P ratio at 1:6 contains more plant available P than sludge with a higher ratio (e.g., Fe:P ratio of 9:8) (Kahiluoto et al., 2015). Calcium is another element that has an effect on the P availability. High dosing of Ca in the wastewater with a Ca:P ratio of 2:1 reduce the P-FEV due to formation of Ca-P compounds such as hydroxylapatite, which has a low solubility, an effect shown when using the sludge to produce compost and biochar (Nest et al., 2021). As mentioned above, solubility of the P crystals are affected by pH and liming increases the plant-available P in sludge produced from the wastewater treated by Al or Fe salts (Krogstad et al., 2005; Montgomery et al., 2005; Bøen and Haraldsen, 2013).
When processing DPS to a STRUBIAS product, the FEV will change. This is influenced by the untreated DPS physiochemical characteristics and the processing methods and parameters used. For example, the P-FEV in ash produced from incineration is low when wood is used (P-FEV, 8–16%), but gets higher using chicken manure (P-FEV = 13–39%) (Yusiharni et al., 2007), and highest when incineration of biogas residue is used (MFE = 76–99%; Kuligowski et al., 2010). The plant availability of P in thermochemical products such as ash and biochar depends on the temperature during combustion/pyrolysis, and is halved by increasing the incineration temperature from 400 to 700°C, which is due to hydroxyapatite formation (Thygesen et al., 2011). To increase the amount of P in STRUBIAS products, the use of flocculants or biological processes to increase P availability in raw DPS whilst avoiding high temperatures during the production of STRUBIAS could be implemented.
For N-FEV, the proportion of ammonium N (NH+4-N) to the total N content and the C/N ratio of the DPS or DPS-derived STRUBIAS products are the most important factors for the FEV (Sommer et al., 2013; Webb et al., 2013). Ammonium is immediately available for the crop and is often a growth-limiting factor (Brod et al., 2012; Gómez-Muñoz et al., 2017). When fertilizer rates increased, the N-FEV of meat and bone meal (MBM) and composted fish sludge (CFS) decreased from 76–65% to 67–53%, respectively (Brod et al., 2012; Gómez-Muñoz et al., 2017). This is consistent with crop response trials, where increasing amounts of N are applied (e.g., Brod et al., 2012, see Figure 4). In that example, two industrial composts (i.e., neutral and acid Dynea composts) had only N-FEV values ranging from 7 to 30%, as they contained low amounts of NH+4 and the N-mineralization rate was low. Acidification of the compost increased the ANR and N-FEV of Dynea composts compared to untreated compost (Brod et al., 2012). This is due to a reduced NH3 emission during composting, as is seen when acidifying stored pig and cattle slurry. In long-term studies adding human sewage sludge (i.e., biosolids) to silage maize, the FEV was 55% at low application rates and 64% at high application rates (Cerný et al., 2012). This result, compared with the finding by Brod et al. (2012), implied that in short-term fertilizer application, doubling the application rate might not have a higher ANR and FEV, but in the long-term application, an increase of the rate increase ANR and FEV. The reason can be that higher application rate in short-term studies leads to emission of easily available N (NH3 emission, denitrification) and that reduces FEV, while in long-term studies there still is this immediate loss but organic N increases in the soil and this will lead to higher amounts of N mineralised with time. Gómez-Muñoz et al. (2017) found in the long-term experiment that continuous application of agricultural and urban wastes improved soil quality, and long-term N availability correlates with the accumulation of N and C in soil. That study reported the ANR and FEV in the final year (2013) had generally increased compared to those in the first year of the study (2003), except for composted household waste and cattle deep litter. The effect of C:N ratio was documented for biochar produced by the pyrolysis of eucalyptus wood, as ANR values increased from 28–40% with increasing C:N ratios (2–4.9). Therefore, as new DPS-derived STRUBIAS products are emerging, there needs to be a test phase before their use in agriculture. This should involve short to long-term pot and field trials across crop and soil types to investigate P dynamics in soil and their N-P FEV values.
Potential Environmental Losses
During the storage and land application of DPS, there may be the risk of nutrient loss or emissions to waters (surface and subsurface) and/or the atmosphere, respectively.
Potential Losses From DPS/STRUBIAS to Waters
As with all fertilizers, there is an associated risk of pollutants loss to waters (surface and subsurface pathways) (Sørensen and Jensen, 2013) (Figure 3). DPS contains high levels of P and other constituents such as C, N, Na and Cl that can alter soil composition and runoff behavior (Liu and Haynes, 2010, 2011). The application timing and method of DPS are both important factors to control to minimize pollutant losses to waters. Two recent studies have examined nutrient losses from DPS in field soil experiments. The first micro-plot lab study applied several DPS types to a grassland soil in Ireland and investigated the potential losses on P and N in runoff using simulated overland flow after 48 h of DPS application (Ashekuzzaman et al., 2020). That study found that the soluble P loss was highest for Ca-DPS (5.7 mg L−1) followed by Al-DPS (0.8 mg L−1) and Fe-DPS (0.15 mg L−1). In addition, P losses from DPS, including Ca–P-rich DPS, are much lower when compared to cattle slurry (7.0 mg L−1). With regard to N, that study observed dominant N losses were NH4-N (nitrate (NO-3) losses were negligible) in the runoff pathway with concentrations ranging from 2.6 to 3.3 mg L−1. Such concentrations are significantly lower than equivalent studies that focused on dairy cattle slurry (17.4 mg L−1). The availability of N in organic wastes can be predicted from their C:N ratio (Delin et al., 2012), and DPS has a C:N ratio of ~6 (Ashekuzzaman et al., 2019a), which is comparable with human sewage sludge. According to Delin et al. (2012), this implies that around 50% of the N content is easily available, as also found for sewage sludge (Petersen et al., 2003). The second field study examined P accumulation in soil and potential losses in surface runoff and leaching (multi-depth) at seven sites in New Zealand (Lizarralde et al., 2021). Results showed that after the long-term application of DPS (based on N content), high amounts of P in the soil at least to 30-cm depths accumulated. The level of accumulation varied across soils and was due to the history of wastewater application, the capacity of the soils to sorb P and the land use and system management.
Organic fertilizers such as DPS delivered and applied on arable land (e.g., winter cereals) can be an effective component of any nutrient management plan. For practical reasons, DPS is often applied in autumn before sowing a winter cereal like winter wheat. However, under free draining soils (loamy sand and sandy loam soils with a yearly drainage surplus of 300–400 mm) and wet and cool North-European conditions, extra NO-3 leaching losses, equivalent to 20–30% of total N, can be expected after application of organic fertilizers with similar N availability to winter wheat in autumn (Sørensen and Rubæk, 2012). Under conditions with less surplus precipitation or application to crops with a large capacity for N uptake in autumn, less NO-3 leaching by application in autumn are expected. By waste application in spring, NO-3 leaching is significantly lower (Sørensen and Rubæk, 2012) and NO-3 leaching is often proportional to total N application (De Notaris et al., 2018; Pedersen et al., 2021) and thus nearly similar for organic N and mineral N. The total N applied with organic fertilizers is higher than with mineral fertilizers to obtain the same fertilizer value and thereby crop yield. This also implies that NO-3 leaching is higher by application of organic wastes in spring compared to mineral N fertilization, but higher leaching losses can be prevented by use of cover crops (Pedersen et al., 2021). Pedersen et al. (2021) found extra NO-3 leaching equivalent to 8% of the N input in the first year and 4% in the second year after application for both mineral and organic N applied to a loamy sand and a sandy loam soil in spring.
In contrast to N, soluble P is strongly bound to soil implying that very low leaching losses of P occur after application of organic fertilizers. However, if DPS is applied directly to soil and not incorporated or injected, there is risk of incidental P losses (0.3–7.6% of total input) by surface runoff (Ashekuzzaman et al., 2020) and by leaching through macropores in soil (Sørensen and Jensen, 2013). Such losses can both occur by transport in soluble form (e.g., dissolved reactive phosphorus) and in the form of particle and colloid-bound P. Christiansen et al. (2020) found large variation in water-extractable P (0.1–9 % of total P) in various sludge types and therefore the risk of soluble P loss is also variable. A reduced risk of P losses along surface runoff and subsurface macropore leaching pathways by incorporation of DPS into soil or by injection is possible (Sørensen and Jensen, 2013). When soils are loaded with excessive amounts of P over a longer period, the soil is saturated with P and P leaching to drains is significantly increased (Heckrath et al., 1995). By precipitation of P and N in struvite, nutrients become concentrated like in mineral fertilizers and can be stored and applied as for mineral equivalents. This means that its application can occur following best practice for precision farming i.e., right time, right place, right amount, right method, and right product.
After pyrolysis of sludge for biochar production, most of the organic N is lost. The availability and fate of this N is not well investigated e.g., Christiansen et al. (2020) found that a biochar derived from a mixture of human sewage sludge and straw contained 5% of total P in water-extractable form and most of the P content was soluble in a weak acid (citric acid). Therefore, a part of the P in sludge-based biochar solubilises in soil. Weak biochar binding on clay minerals and its low density can lead to environmental losses to waters. In addition, translocation of biochar due to hydrological connectivity is observed. For instance, Rumpel et al. (2006) showed that biochar accumulates at the bottom of slopes within the landscape and such losses are important to quantify as they can be delivered to surface water (Major et al., 2010). Therefore, biochar needs to be incorporated into soil to avoid loss of P in surface runoff either in soluble or in particulate form. This is also the case in grasslands, where a significant reduction in P losses may occur where injection rather than surface application of manure is practiced (Uusi-Kämppä and Heinonen-Tanski, 2008). This precision farming application method where available could be a DPS application method that minimizes incidental losses of pollutants in runoff during rainfall events.
Potential Losses From DPS/STRUBIAS to the Atmosphere
To date, there have not been many studies that have measured or calculated the accumulated emissions of GHG and NH3 from the production, storage or land application of these products (Figure 5). Therefore, DPS or STRUBIAS emissions of CH4, N2O, and NH3 from production until after field application are calculated using a combination of information about emission from the products or by using similar products as a proxy. Herein, such risks and mitigation for each management step i.e., from production, to processing of the sludge, to storage of the sludge and sludge products, and to application of DPS and secondary STRUBIAS products is considered.
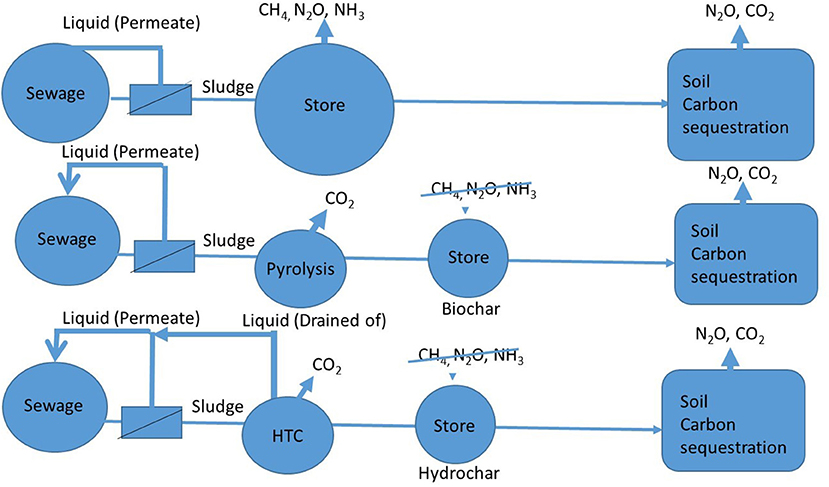
Figure 5. GHG emission from scenario of dairy sludge management no treatment of sludge, pyrolysis, and hydrothermal (HTC) treatment of sludge.
The DPS is usually stored anaerobically until application to soil. During this phase CH4 and NH3 may be emitted; little N2O is emitted during the storage phase as the waste tends to not have a surface crust where nitrification-denitrification may take place (Baral et al., 2018). However, application to soil emits N2O (Scott et al., 2000; Yoshida et al., 2015). A fraction of the C in sludge applied to soil will contribute to C storage. The emitted NH3 can contribute to N2O emission after deposition to land or water. Transforming sludge into biochar, hydrochar, or ash will cause an emission of CO2, but this treatment will eliminate CH4 emission and may affect N2O emission when applied to soil. The CO2 emitted during treatment of the sludge is part of the circulation of C between the atmosphere, plants, intake by dairy cows, and recycling of the waste and therefore considered climate warming neutral.
In recent field studies, biochar has not increased N2O emissions from “fertilized soil” when applied to fields (Clough and Condron, 2010; Taghizadeh-Toosi et al., 2011; Liao et al., 2020; Thers et al., 2020). There is no emission of N2O during storage of biochar, as it has been shown in compost studies that biochar reduces N2O production and emission (Shakoor et al., 2021). In several meta-analyses it has been shown that N2O emissions from soil decreases after the addition of biochar (Cayuela et al., 2014; Sri Shalini et al., 2020). Reasons for reduced N2O emission from soil treated with biochar could be improved soil aeration, increased soil pH, enhanced N immobilization, and possible toxic effect induced by biochar organic compounds (polycyclic aromatic hydrocarbons) on nitrifier and denitrifier communities (Taghizadeh-Toosi et al., 2011; Cayuela et al., 2014; Harter et al., 2014). In contrast, some studies show increased N2O emission from soil with biochar, which is attributed to an increased soil water content in the presence of biochar favoring denitrification, or the release of biochar embodied-N (Lorenz and Lal, 2014).
The N content of biochar or hydrochar is not high due to the transformation of N during initial feedstock thermolysis (Majumder et al., 2019). Although N content in biochar or hydrochar is low, the application of biochar materials into the soils can affect the soil N cycle. Biochar and hydrochar have been shown to adsorb NH+4 on biochar particles and reduce NH3 volatilization; however, the increased NH3 volatilization, observed from some soil treated with hydrochar, is possibly due to the reduced ability to absorb NH+4 associated with greater hydrophobicity of hydrochar (Clough and Condron, 2010; Taghizadeh-Toosi et al., 2012; Subedi et al., 2015).
Carbon in DPS and in DPS-derived STRUBIAS products added to soil will contribute to soil C storage, the sequestering potential or mean residence time (MRT) being related to the rate of transformation of the added carbon to CO2 (Tian et al., 2009). It has been shown that CO2 fluxes were suppressed when biochar was added to fertilized soils (Wang et al., 2016), which may be due to reduced enzymatic activity and the precipitation of CO2 onto the biochar surface (Case et al., 2014). Ethylene, which is frequently present in biochar, can sometimes inhibit the transformation of C in soil (Spokas et al., 2010). However, if there is labile C input in biochar or hydrochar, it can result in positive priming effects (He et al., 2017) and increased CO2 emissions, although part of the CO2 may have originated from carbonate formed during pyrolysis (Kuzyakov et al., 2009). Pyrolysis and gasification materials have been assessed by many (e.g., Lal, 2009; Beesley et al., 2011; Wu et al., 2017) to increase soil organic C content and to improve overall soil health.
The present literature review shows that STRUBIAS production potentially can reduce GHG emission from all sites of the sludge management chain. An analysis of the emission of GHG from sludge is stored until it was applied to soil or alternatively processed, chars stored and then applied to soil was carried out to provide insight in the potential total GHG reduction due to production of biochar and hydrochar (Figure 5). In calculations carried out using the model outlined in material and methods, the total GHG increase in the atmosphere due to sludge managed traditionally is 359 kg CO2eqv (Table 6). In contrast to sludge management, CH4 emission during storage of STRUBIAS products is avoided, and N2O emission from biochar is negligible and from hydrochar reduced to 1/3 of the emission from sludge, because 55% of the N in sludge is recycled to the wastewater plant. Due to the recalcitrant nature of C in STRUBIAS products, more C is sequestered when these are applied to fields. Within the boundary of the sludge managing system, the emission from standard sludge management is 359 kg CO2eqv (an increase in CO2 in the atmosphere). Producing hydrochar reduces GHG in the atmosphere corresponding to −30 kg CO2eqv and biochar production to −92 kg CO2eqv. Avoiding CH4 and N2O emission from the sludge are the most important factors to reduce the climate warming potential of dairy waste management.
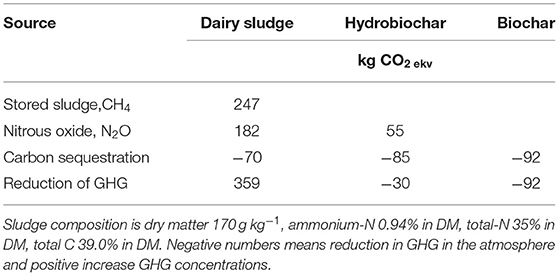
Table 6. Greenhouse gas emission from the management chain of dairy sludge management untreated and after HTC or pyrolysis—calculated in the present study.
Effects on Soil Microorganisms
Soil microorganisms play a pivotal role in nutrient cycling in agricultural ecosystems. Their activities enhance the availability of essential nutrients for crop growth and contributes to an improvement of soil properties such as OM content and water retention capacity. The application of organic residues like DPS modifies soil microbial communities significantly. These modifications are highly variable and depend strongly on the composition of the bio-based residues applied.
Microbial Analysis
The methods used to assess soil microbial processes divide into abundance, diversity and activities. Determination of soil microbial biomass in soil by chloroform fumigation is a common indicator applied after the application of different types of sludge (Charlton et al., 2016). Other techniques that can provide information on microbial biomass are phospholipid fatty acid assays (PLFAs) and the quantification of total DNA. These two techniques are also powerful methods to determine changes in the diversity of soil microorganisms. DNA-based techniques such as 16S and 18S gene quantification, metagenomics and metabarcoding, provide very insightful information on the community composition (Abdelfattah et al., 2018; Bünemann et al., 2018; Bastida et al., 2019). PLFAs allow distinguishing between bacteria and fungi, and further distinctions between bacterial groups such as Gram+ and Gram− bacteria (Frostegård and Bååth, 1996). Other commonly used methods to determine changes in microbial metabolic diversity are community-level physiological profiling (CLPP) assays such as Biolog Ecoplates (Liu et al., 2017). The latter technique provides profiles of potential degradation of different complex chemical C substrates, which assess the ability of soil microbial communities to degrade natural soil constituents (Siebielec et al., 2018). Lastly, enzymatic activities are the most common techniques to study soil microbial activities after the application of organic residues. The most common enzymes measured are phosphatases, β-glucosidases, dehydrogenases and ureases (Table 7). Enzymes are substrate-specific and can be associated with different nutrient cycles in the soil (Burns et al., 2013).
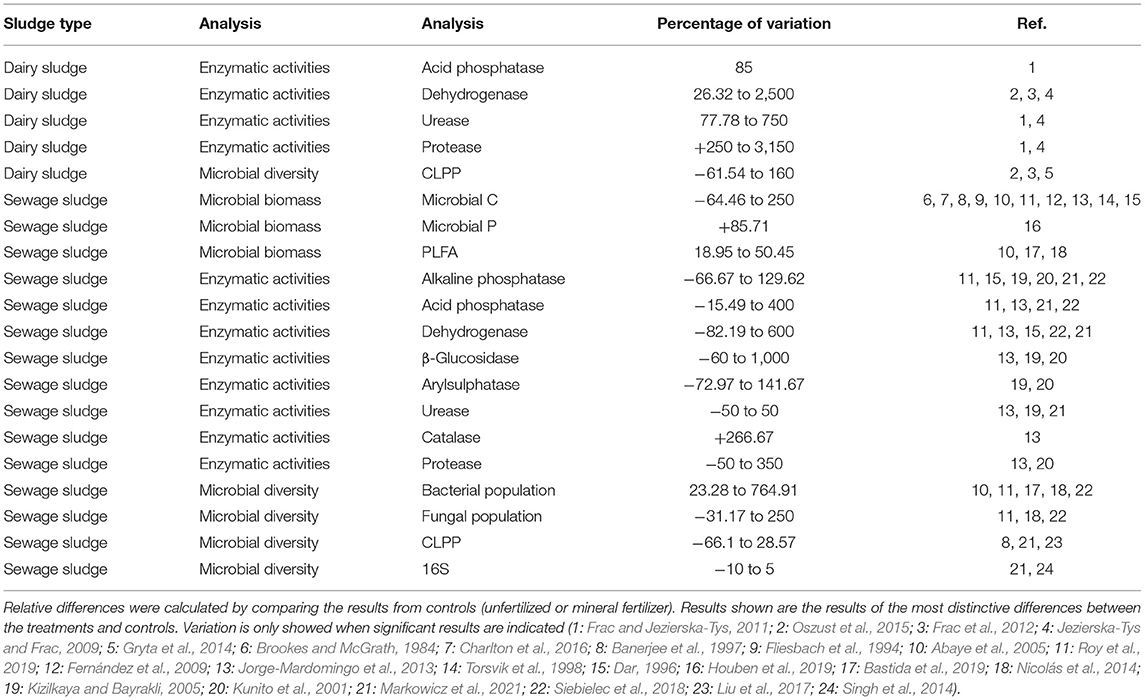
Table 7. Information on relative changes of microbial indicators after the application of sewage and dairy sludge to soil.
Effects of DPS Application on Soil Microbial Communities
Little information is available and from a small number of research teams on the direct effects of dairy sludge on soil biological properties, and the studies do not always come to the same conclusion (Table 7). Reported increases of microbiological indexes and improvements in soil nutrient cycling after the application of DPS appear in the literature. Soil enzymatic activities (Frac and Jezierska-Tys, 2011; Frac et al., 2012; Oszust et al., 2015) and soil microbial diversity as revealed by CLPPs analyses (Frac et al., 2012; Oszust et al., 2015) were reported to increase after the application of dairy sludge. Gryta et al. (2014) show that the effect of heavy metal contamination due to DPS application caused a significantly lower (−61.54 %) C degradation as shown by a Biolog Ecoplate assay and DPS is a source of heavy metal contamination in soils (López-Mosquera et al., 2000). Heavy metal concentrations below guideline values found in studies by Ashekuzzaman et al. (2020) and Shi et al. (2021a) point to the importance of regulated application rates (based on P) but also recognizing heavy metal application which varies DPS types. As wastewater treatment is improved, heavy metal concentrations should be reduced further e.g., biological P, removal strives to replace the need for metal coagulants.
Similar results were found when assessing other types of sludge such as sewage sludge, which has a high content of C and contributes to increased soil microbial biomass and activities (Torsvik et al., 1998; Abaye et al., 2005). Yet, similar to the results of Gryta et al. (2014) on dairy sludge, sewage sludge effects on soil microorganisms depend also on the amount of pollutants. Sewage sludge may contain large concentrations of pollutants such as heavy metals and pathogens that often can decrease soil microbial indicators (Torsvik et al., 1998; Charlton et al., 2016; Major et al., 2020; Table 7). Although concentrations of heavy metals and other pollutants may be low in DPS compared to sewage sludge, there is a need for long-term field trials, especially pertaining to bioaccumulation of heavy metals in soil (and crops) as this has the potential to damage soil microbial communities.
Gryta et al. (2014) examined DPS heavy metal content on soil biology. Their results showed that high concentrations of heavy metals led to a decrease in most soil biological descriptive variables, associated with damage of the cell membrane, mitochondria or DNA (Dar, 1996; Tchounwou et al., 2012). Torsvik et al. (1998) compared the application of contaminated sewage sludge with unpolluted equivalents to examine microbial diversity. Using a technique that uses “total number of genomes,” as equivalent to the E. coli genome, their results indicated that the application of polluted sludge had negative impacts on soil biodiversity, causing a reduction of up to 6.5 times less biodiversity. Previous experiments also compared polluted vs. unpolluted sewage sludge with similar conclusions (Brookes and McGrath, 1984; Fliesbach et al., 1994). Other studies using Biolog (CLPPs) assessments (Banerjee et al., 1997) and PLFA analyses have shown similar results (Singh et al., 2014; Table 7). Similarly, several studies show the depressed activities of soil microorganisms by reduced enzymatic activities after polluted sludge applications to soil (Dar, 1996; Kunito et al., 2001; Kizilkaya and Bayrakli, 2005; Speir et al., 2007; Fernández et al., 2009; Markowicz et al., 2021; Table 7).
Another risk derived from the application of DPS is the introduction of pathogens and organic pollutants. Research from sewage sludge shows the presence of human and animal pathogens such as E. coli, Listeria, Clostridium perfringens, Enterococcus, or Salmonella (Brochier et al., 2012). These pathogens may survive on plant tissues, in soils and in hydroponic systems to which sludge is applied (Brochier et al., 2012; Kyere et al., 2019). Native soil microbial communities are known to decrease the survival of potential pathogens (Xing et al., 2020), but specific strains of bacteria, such as E. coli O104:H4, may survive in soil for over a year after its inoculation (Knödler et al., 2016). Moreover, sewage and DPS introduce antibiotic resistant genes to soils, causing the development of antibiotic resistant bacteria with severe implications on human and environmental health (Rizzo et al., 2013; Dungan et al., 2018; Urra et al., 2019). Nevertheless, even if pathogens are present in DPS, their concentration is believed to be 10–15 times lower than in sewage sludge (Kwapinska et al., 2020). As a consequence, the risks of introducing pathogens and other organic pollutants after the application of DPS are smaller than other residues such as sewage sludge.
Study results with respect to the effect of sludge applications in agriculture and their effects on soil communities vary, but for dairy derived sludge most studies show increases in microbial functional diversity (CLPP) and activities (Table 7). Activities of dehydrogenase (Jezierska-Tys and Frac, 2009; Frac et al., 2012; Oszust et al., 2015), acid phosphatase (Frac and Jezierska-Tys, 2011), urease and protease (Frac et al., 2012; Oszust et al., 2015) have been reported to increase after the application of dairy sludge (Table 7). CLPP experiments have also revealed increased degradation of C substrates (Frac et al., 2012; Oszust et al., 2015).
When sewage sludge is not heavily polluted, similar trends can be observed (Table 7). Enzymatic activities have been shown to increase with the application of sewage sludge (Dar, 1996; Jorge-Mardomingo et al., 2013; Siebielec et al., 2018; Roy et al., 2019), soil microbial biomass (Charlton et al., 2016) and diversity indexes has been reported to improve based on Biolog assessments (Liu et al., 2017). DNA approaches have also revealed little effect on soil microbial diversity and bacterial antibiotic-resistance after the application of sludge (Rutgersson et al., 2020).
The application of unpolluted sludge to soils might be very beneficial in improving soil health and fertility. Sludge contains large concentrations of easily decomposable C, readily available N, P, and other essential nutrients for plant and microbial growth (Krogstad et al., 2005; Singh and Agrawal, 2008; Peltre et al., 2011). The recalcitrant components of sludge release at a slow rate by specific microbial groups such as P solubilising microorganisms (PSM) (Clarholm, 1985; Khan et al., 2009; Kuypers et al., 2018). The relative distribution of the different nutrient pools in sewage sludge is highly dependent on the production, treatments and origin of the sludge (Singh and Agrawal, 2008).
The high C content constitutes the most significant attribute of sludge that can affect the development and growth of soil microbial communities, because C is the most limiting element for bacterial and fungal growth in soils (Demoling et al., 2007; Hobbie and Hobbie, 2013). Boosting the C content in soil leads to a concatenated stimulus in the cycling of other nutrients such as N or P (Demoling et al., 2007). Field studies have also confirmed the positive effects of sludge application on both N and P cycles (Hallin et al., 2009; Frac and Jezierska-Tys, 2011; Houben et al., 2019). The application of large amounts of C is associated with a significant growth in soil microbial biomass (Charlton et al., 2016; Houben et al., 2019). Yet, this positive effect of sewage sludge on soil microbial communities has been reported to lead to significant nutrient immobilization by soil biota (Smith and Tibbett, 2004; Gómez-Muñoz et al., 2017). It is expected that sewage sludge with a high C:N ratio (>15) might lead to N immobilization by soil biota (Gómez-Muñoz et al., 2017). The same applies for the immobilization of P, application of organic materials with a high C:P ratio might lead to its immobilization (Zhang et al., 2018). Whenever sludge is applied as a bio-based fertilizer, these aspects should be considered. However, in the long term, the application of C-rich materials such as sewage sludge should improve soils from an agronomic and environmental point of view. Building up C content in soils would improve nutrient cycling, providing a slower release maintained over time and lower losses that might contaminate soils and water bodies (Gómez-Muñoz et al., 2017; Zhang et al., 2018).
Conclusion
This review collated information that will help give DPS and their secondary products certification as P-fertilizers in accordance with technical proposals for new fertilizing materials under forthcoming EU Fertilizing Product Regulations. It presents the current state of knowledge pertaining to dairy processing sludge and STRUBIAS bio-based fertilizers and identifies knowledge gaps and potential solutions to minimize environmental losses to soil, water and air.
STRUBIAS products have a high P concentration compared to that of sludge, and a well-defined fertilizer efficiency of the P applied to fields. To achieve high P fertilizer efficiency, dairy wastewater treatment must aim at producing sludge with soluble P-components and avoid Al coagulation and P insolubility. In STRUBIAS production, conditions producing less soluble P should be avoided, i.e., high temperatures. The benefits of STRUBIAS production is a reduction of transport cost of P due to a high P concentration. In the development of production units, it is important that STRUBIAS products can be applied with traditional mineral fertilizer application machinery. Heavy metal concentration of known products are below the limits set for the use of these as fertilizers, and the risk of disease spreading and negative effects on microbial activity in soil is low. Producing STRUBIAS products eliminates GHG emissions from management of the sludge from dairies. A goal of STRUBIAS production could be recycling of plant nutrients and C to organic farms, thereby providing a sustainable circular economy. More information is needed to carry out an economic analysis (e.g., a cost comparison across dairy sludge management, production, and management systems for STRUBIAS products and mineral fertilizer), which should include a value chain analysis of the whole system.
Data Availability Statement
The original contributions presented in the study are included in the article/Supplementary Material, further inquiries can be directed to the corresponding author/s.
Author Contributions
YH, OK, WS, ÁV-S, SA, NB-L, JL, KD, OF, MH, PS, SS, AT-T, and IT-G made substantial contributions to the conception of the work, the acquisition, analysis, interpretation of data for the work, and were involved in drafting the work. YH, OK, WS, ÁV-S, SA, NB-L, KD, OF, MH, PS, SS, AT-T, and IT-G revised it critically for important intellectual content. All authors contributed to the article and approved the submitted version.
Funding
This project (REFLOW) has received funding from the European Union's Horizon 2020 research and innovation programme under the Marie Skłodowska-Curie grant agreement no. 814258.
Conflict of Interest
The authors declare that the research was conducted in the absence of any commercial or financial relationships that could be construed as a potential conflict of interest.
Publisher's Note
All claims expressed in this article are solely those of the authors and do not necessarily represent those of their affiliated organizations, or those of the publisher, the editors and the reviewers. Any product that may be evaluated in this article, or claim that may be made by its manufacturer, is not guaranteed or endorsed by the publisher.
Supplementary Material
The Supplementary Material for this article can be found online at: https://www.frontiersin.org/articles/10.3389/fsufs.2021.763020/full#supplementary-material
Abbreviations
NH3, Ammonia; AD, Anaerobic digesters; BOD, Biological oxygen demand; C, Carbon; CO2, Carbon dioxide; CWW, Cheese whey wastewater; COD, Chemical oxygen demand; CFU, Colony forming units; CLPP, Community-level physiological profiling; CSTR, Completely stirred tank reactor; DPS, Dairy processing sludge; DAF, Dissolved air floatation; DM, Dry matter; EU, European union; FEV, Fertilizer equivalent value; GHG, Greenhouse gas; HTC, Hydrothermal carbonification; IFSM, Integrated farm systems model; MRT, Mean residence time; MARS, Membrane Anaerobic reactor system; MBR, Membrane reactor; CH4, Methane; MFE, Mineral fertilizer equivalence; MFRV, Mineral fertilizer replacement value; N, Nitrogen; N2O, Nitrous oxide; OM, Organic matter; OTCs, Organic trace compounds; O2, Oxygen; P, Phosphorus; PLFAs, Phospholipid fatty acid assays; PCBs, PolyChloroBiphenyls; PAHs, Polycyclic aromatic hydrocarbons; PSM, P solubilising microorganisms; PC, Pyrolysis; RBC, Rotating biological contractors; SBR, Sequencing batch reactor; STRUBIAS, struvite, biochar, ashes; TAN, Total ammonium nitrogen; UASB, Up-flow anaerobic sludge blanket; VS, Volatile solids.
Footnotes
1. ^Smith, A. M., Sommer, S. G., Pedersen, I. F., Taghizadeh-Toosi, A., and Petersen, S. O. (preparation). Greenhouse gas emission reduction by hydrothermal sludge carbonization of dairy sludge.
References
Abaye, D. A., Lawlor, K., Hirsch, P. R., and Brookes, P. C. (2005). Changes in the microbial community of an arable soil caused by long-term metal contamination. Eur. J. Soil Sci. 56, 93–102. doi: 10.1111/j.1365-2389.2004.00648.x
Abdelfattah, A., Malacrinò, A., Wisniewski, M., Cacciola, S. O., and Schena, L. (2018). Metabarcoding: a powerful tool to investigate microbial communities and shape future plant protection strategies. Biol. Control 120, 1–10. doi: 10.1016/j.biocontrol.2017.07.009
Acosta-Martínez, V., and Waldrip, H. M. (2014). “Soil enzyme activities as affected by manure types, application rates, and management practices,” in Applied Manure and Nutrient Chemistry for Sustainable Agriculture and Environment, eds Z. He, and H. Zhang (Dordrecht: Springer), 99–122. doi: 10.1007/978-94-017-8807-6_6
Adam, C., Peplinski, B., Michaelis, M., Kley, G., and Simon, F. G. (2009). Thermochemical treatment of sewage sludge ashes for phosphorus recovery. Waste Manage. 29, 1122–1128. doi: 10.1016/j.wasman.2008.09.011
Agriland (2020). How Did Milk Production in Ireland Compare to Other Countries in 2019? Available online at: https://www.agriland.ie/farming-news/how-did-milk-production-in-ireland-compare-to-other-countries-in-2019/ (accessed April 28, 2021).
Ahmad, T., Aadil, R. M., Ahmed, H., Rahman, U. U., Soares, B. C. V., and Souza, S. L. Q. (2019). Treatment and utilization of dairy industrial waste: a review. Trends Food Sci. Techn. 88, 361–372. doi: 10.1016/j.tifs.2019.04.003
Amoah-Antwi, C., Kwiatkowska-Malina, J., Thornton, S. F., Fenton, O., Malina, G., and Szara, E. (2020). Restoration of soil quality using biochar and brown coal waste: a review. Sci. Total Environ. 722:137852. doi: 10.1016/j.scitotenv.2020.137852
APHA (1998). Standard Methods for the Examination of Water and Wastewater, 20th edn. Washington, DC: American Public Health Association, American Water Works Asso-ciation, and Water Pollution Control Federation.
Arenas-Montaño, V., Fenton, O., Moore, B., and Healy, M. G. (2021). Evaluation of the fertiliser replacement value of phosphorus-saturated filter media. J. Clean. Produc. 291:125943. doi: 10.1016/j.jclepro.2021.125943
Arun, C., and Sivashanmugam, P. (2018). Enhanced production of biohydrogen from dairy waste activated sludge pre-treated using multi hydrolytic garbage enzyme complex and ultrasound-optimization. Energy Conver. Manage. 164, 277–287 doi: 10.1016/j.enconman.2018.02.095
Ashekuzzaman, S. M., Fenton, O., Meers, E., and Forrestal, P. J. (2021a). Differing phosphorus crop availability of aluminium and calcium precipitated dairy processing sludge potential recycled alternatives to mineral phosphorus fertiliser. Agronomy 11:427. doi: 10.3390/agronomy11030427
Ashekuzzaman, S. M., Forrestal, P., Richards, K., Daly, K., and Fenton, O. (2021b). Grassland phosphorus and nitrogen fertiliser replacement value of dairy processing dewatered sludge. Sustain. Prod. Consum. 25, 363–373. doi: 10.1016/j.spc.2020.11.017
Ashekuzzaman, S. M., Forrestal, P., Richards, K., and Fenton, O. (2019a). Dairy industry derived wastewater treatment sludge: generation, type and characterization of nutrients and metals for agricultural reuse. J. Clean. Prod. 230, 1266–1275. doi: 10.1016/j.jclepro.2019.05.025
Ashekuzzaman, S. M., Forrestal, P., Richards, K., and Fenton, O. (2020). Potential loss of nutrients, carbon and metals in simulated runoff associated with dairy processing sludge application. Inter. J. Environ. Sci. Techn. 17, 3955–3968. doi: 10.1007/s13762-020-02768-z
Ashekuzzaman, S. M., Kwapinska, M., Leahy, J. J., Richards, K., and Fenton, O. (2019b). Novel use of dairy processing sludge derived pyrogenic char (DPS-PC) to remove phosphorus in discharge effluents. Waste Biomass Valor. 11, 1453–1465. doi: 10.1007/s12649-019-00731-9
Atallah, E., Zeaiter, J., Ahmad, M. N., Kwapinska, M., Leahy, J. J., and Kwapinski, W. (2020). The effect of temperature, residence time, and water-sludge ratio on hydrothermal carbonization of DAF dairy sludge. J. Environ. Chem. Eng. 8:103599. doi: 10.1016/j.jece.2019.103599
Banerjee, M. R., Burton, D. L., and Depoe, S. (1997). Impact of sewage sludge application, on soil biological characteristics. Agric. Ecosyst. Environ. 66, 241–249. doi: 10.1016/S0167-8809(97)00129-1
Baral, K. R., Jégo, G., Amon, B., Bol, R., Chantigny, M. H., Olesen, J. E., et al. (2018). Greenhouse gas emissions during storage of manure and digestates: key role of methane for prediction and mitigation. Agric. Sys. 166, 26–35. doi: 10.1016/j.agsy.2018.07.009
Barret, M., Delgadillo-Mirquez, L., Trably, E., Delgenes, N., Braun, F., and Cea-Barcia, G. (2012). Anaerobic removal of trace organic contaminants in sewage sludge: 15 years of experience. Pedosphere 22, 508–517. doi: 10.1016/S1002-0160(12)60035-6
Bastida, F., Jehmlich, N., Martínez-Navarro, J., Bayona, V., García, C., and Moreno, J. L. (2019). The effects of struvite and sewage sludge on plant yield and the microbial community of a semiarid Mediterranean soil. Geoderma 337, 1051–1057. doi: 10.1016/j.geoderma.2018.10.046
Beesley, L., Moreno-Jiménez, E., Gomez-Eyles, J. L., Harris, E., Robinson, B., and Sizmur, T. (2011). A review of biochars' potential role in the remediation, revegetation and restoration of contaminated soils. Environ. Pollut. 159, 3269–3282. doi: 10.1016/j.envpol.2011.07.023
Bennett, E., and Carpenter, S. R. (2002). P Soup (the Global Phosphorus Cycle). Washington, DC: World Watch Magazine, 24–32.
Bernal, M. P., Sommer, S. G., Chadwick, D., Qing, C., Guoxue, L., and Michel, F. C. Jr. (2017). Current approaches and future trends in compost quality criteria for agronomic, environmental, and human health benefits. Advan. Agron. 144, 143–233. doi: 10.1016/bs.agron.2017.03.002
Bøen, A., and Haraldsen, T. K. (2013). Meat and bone meal and biosolids as slow-release phosphorus fertilizers. Agric. Food Sci. 22, 235–246. doi: 10.23986/afsci.7498
Boruszko, D. (2017). Research on the influence of anaerobic stabilization of various dairy sewage sludge on biodegradation of polycyclic aromatic hydrocarbons PAHs with the use of effective microorganisms. Environ. Res. 155 344–352. doi: 10.1016/j.envres.2017.02.019
Bourbon, B., and Huet, J. (2018). AQUAREL: Project aimed at improving water management in French dairy plants. J. Food Sci. Engng. 8, 137–142. doi: 10.17265/2159-5828/2018.03.005
Britz, T. J., van Schalkwyk, C., and Hung, Y.-T. (2006). “Treatment of dairy processing wastewaters,” in Waste Treat Food Process, eds, L. K. Wang, Y. Hung, H. H. Lo, and C. Yapijakis (Boca Raton, FL: CRC Press; Taylor & Francis Group), 33487–2742 doi: 10.1201/9781420037128.ch1
Brochier, V., Gourland, P., Kallassy, M., Poitrenaud, M., and Houot, S. (2012). Occurrence of pathogens in soils and plants in a long-term field study regularly amended with different composts and manure. Agric. Ecosyst. Environ. 160, 91–98. doi: 10.1016/j.agee.2011.05.021
Brod, E., Haraldsen, T. K., and Breland, T. A. (2012). Fertilization effects of organic waste resources and bottom wood ash: results from a pot experiment. Agric. Food Sci. 21, 332–347 doi: 10.23986/afsci.5159
Brookes, P. C., and McGrath, S. P. (1984). Effect of metal toxicity on the size of the soil microbial biomass. J. Soil Sci. 35, 341–346. doi: 10.1111/j.1365-2389.1984.tb00288.x
Bünemann, E. K., Bongiorno, G., Bai, Z., Creamer, R. E., De Deyn, G., de Goede, R., et al. (2018). Soil quality – a critical review. Soil Biol. Biochem. 120, 105–125. doi: 10.1016/j.soilbio.2018.01.030
Burns, R. G., DeForest, J. L., Marxsen, J., Sinsabaugh, R. L., Stromberger, M. E., Wallenstein, M. D., et al. (2013). Soil enzymes in a changing environment: current knowledge and future directions. Soil Biol. Biochem. 58, 216–234. doi: 10.1016/j.soilbio.2012.11.009
Byrne, R. J. (2011). “Utilities and effluent treatment | design and operation of dairy effluent treatment plants,” in Encyclopedia of Dairy Sciences, 2nd Edn, eds J. W. Fuquay, P. F. Fox, and P. L. H. McSweeney (London: Elsevier Science), 619–630. doi: 10.1016/B978-0-12-374407-4.00477-5
Carta-Escobar, F., Pereda-Marin, J., Alvarez-Mateos, P., Romero-Guzman, F., Duran-Barrantes, M. M., and Barriga-Mateos, F. (2004). Aerobic purification of dairy wastewater in continuous regime: part I: analysis of the biodegradation process in two reactor configurations. Biochem. Engng. J. 21, 183–191. doi: 10.1016/j.bej.2004.06.007
Carvalho, F., Prazeres, A. R., and Rivas, J. (2013). Cheese whey wastewater: characterization and treatment. Sci.Total Environ. 445, 385–396. doi: 10.1016/j.scitotenv.2012.12.038
Case, S. D., McNamara, N. P., Reay, D. S., and Whitaker, J. (2014). Can biochar reduce soil greenhouse gas emissions from a miscanthus bioenergy crop? GCB Bioen. 6, 76–89. doi: 10.1111/gcbb.12052
Cavalli, D., Cabassi, G., Borrelli, L., Geromel, G., Bechini, L., Degano, L., et al. (2016). Nitrogen fertilizer replacement value of undigested liquid cattle manure and digestates. Eur J. Agron. 73, 34–41 doi: 10.1016/j.eja.2015.10.007
Cayuela, M. L., Van Zwieten, L., Singh, B. P., Jeffery, S., Roig, A., and Sánchez-Monedero, M. A. (2014). Biochar's role in mitigating soil nitrous oxide emissions: a review and meta-analysis. Agric. Ecosys. Environ. 191, 5–16. doi: 10.1016/j.agee.2013.10.009
CEC (1986). European Commission. Council Directive 86/278/EEC of 12 June 1986 on the Protection of the Environment, and in Particular of the Soil, when Sewage Sludge is Used in Agriculture. Luxembourg: CEC.
CEC (2008). Compliance With Limits Required for Compost From Source Separated Bio-Waste Only. Commission Regulation (EC) No 889/2008. Available online at: eur-lex.europa.eu/legal-content/EN/TXT/PDF/?uriCELEX:32008R0889&fromEN (accessed March 18, 2019).
CEC (2009). Environmental, economic and social impacts of the use of sewage sludge on land. Consultation Report on Options and Impacts, Report by RPA, Milieu Ltd and WRc for the European Commission, DG Environment under Study Contract DG ENV.G.4/ETU/2008/0076r. Brussels: CEC.
CEC (2010). Commission Regulation of 27 September 2010, Amending Regulation (EC) No 2150/2002 of the European Parliament and of the Council on Waste Statistics Official. Brussels: Journal European Union (2010).
Cerný, J., Balík, J., Kulhánek, M., Vašák, F., Peklová, L., and Sedlár, O. (2012). The effect of mineral N fertiliser and sewage sludge on yield and nitrogen efficiency of silage maize. Plant Soil Environ. 58, 76–83. doi: 10.17221/538/2011-PSE
Charlton, A., Sakrabani, R., Tyrrel, S., Rivas Casado, M., McGrath, S. P., Crooks, B., et al. (2016). Long-term impact of sewage sludge application on soil microbial biomass: an evaluation using meta-analysis. Environ. Pollut. 219, 1021–1035. doi: 10.1016/j.envpol.2016.07.050
Chen, J. H. (2006). “The combined use of chemical and organic fertilizers and/or biofertilizer for crop growth and soil fertility,” in International Workshop on Sustained Management of the Soil-Rhizosphere System for Efficient Crop Production and Fertilizer Use (Bangkok: Land Development Department).
Chianese, D. S., Rotz, C. A., and Richard, T. L. (2009). Simulation of methane emissions from dairy farms to assess greenhouse gas reduction strategies. Trans. ASABE 52, 1313–1323. doi: 10.13031/2013.27781
Christiansen, N. H., Sørensen, P., Labouriau, R., Christensen, B. T., and Rubæk, G. H. (2020). Characterizing phosphorus availability in waste products by chemical extractions and plant uptake. J. Plant Nutr. Soil Sci. 183, 416–428. doi: 10.1002/jpln.202000015
Chu, L., Hennayake, H. M. K. D., and Sun, H. J. (2019). Biochar effectively reduces ammonia volatilization from nitrogen-applied soils in tea and bamboo plantations. Phyt. Inter. J. Exp. Bot. 88, 261–267. doi: 10.32604/phyton.2019.07791
Clarholm, M. (1985). Interactions of bacteria, protozoa and plants leading to mineralization of soil nitrogen. Soil Biol. Biochem. 17, 181–187. doi: 10.1016/0038-0717(85)90113-0
Clough, T. J., and Condron, L. M. (2010). Biochar and the nitrogen cycle: introduction. J. Environ. Qual. 39, 1218–1223. doi: 10.2134/jeq2010.0204
Cordell, D., Drangert, J.-O., and White, S. (2009). The story of phosphorus: Global food security and food for thought. Glob. Environ. Change 19, 292–305. doi: 10.1016/j.gloenvcha.2008.10.009
CSO (2021a). Milk statistics, February 2021–Table 2 EU Milk intake by Creameries and Pasteurisers. Dublin: Central Statistics Office. Available online at: https://www.cso.ie/en/releasesandpublications/er/ms/milkstatisticsfebruary2021/ (accessed April 13, 2021).
CSO (2021b). Milk Statistics, December 2020, Table 2 EU Milk Intake by Creameries and Pasteurisers. Dublin: Central Statistics Office. Available online at: https://www.cso.ie/en/releasesandpublications/er/ms/milkstatisticsdecember2020/ (accessed April 13, 2021).
Daly, K., Styles, D., Lalor, S., and Wall, D. (2015). Phosphorus sorption, supply capacity and availability in soils with contrasting parent material and chemical properties. Eur. J. Soil Sci. 66, 792–801. doi: 10.1111/ejss.12260
Danalewich, J. R., Papagiannis, T. G., Belyea, R. L., Tumbleson, M. E., and Raskin, L. (1998). Characterization of dairy waste streams current treatment practices, and potential for biological nutrient removal. Water Res. 32, 3555–3568. doi: 10.1016/S0043-1354(98)00160-2
Dar, G. H. (1996). Effects of cadmium and sewage-sludge on soil microbial biomass and enzyme activities. Bioresour. Technol. 56, 141–145. doi: 10.1016/0960-8524(95)00186-7
De Notaris, C., Rasmussen, J., Sørensen, P., and Olesen, J. E. (2018). Nitrogen leaching: a crop rotation perspective on the effect of N surplus, field management and use of catch crops. Agric. Ecosys. Environ. 255, 1–11. doi: 10.1016/j.agee.2017.12.009
Delin, S. (2012). Fertilizer value of nitrogen in hen and broiler manure after application to spring barley using different application timing. Soil Use Manage. 27, 415–426. doi: 10.1111/j.1475-2743.2011.00362.x
Delin, S. (2016). Fertilizer value of phosphorus in different residues. Soil Use Manage. 32, 17–26. doi: 10.1111/sum.12227
Delin, S., Stenberg, B., Nyberg, A., and Brohede, L. (2012). Potential methods for estimating nitrogen fertilizer value of organic residues. Soil Use Manage. 28, 283–291. doi: 10.1111/j.1475-2743.2012.00417.x
Demirel, B., Yenigun, O., and Onay, T. T. (2005). Anaerobic treatment of dairy wastewaters: a review. Proc. Biochem. 40, 2583–2595. doi: 10.1016/j.procbio.2004.12.015
Demoling, F., Figueroa, D., and Båth, E. (2007). Comparison of factors limiting bacterial growth in different soils. Soil Biol. Biochem. 39, 2485–2495. doi: 10.1016/j.soilbio.2007.05.002
Droste, R. L., and Gehr, R. L. (2018). Theory and Practice of Water and Wastewater Treatment. John Wiley & Sons.
Dungan, R. S., McKinney, C. W., and Leytem, A. B. (2018). Tracking antibiotic resistance genes in soil irrigated with dairy wastewater. Sci. Tot. Environ. 635, 1477–1483. doi: 10.1016/j.scitotenv.2018.04.020
Dutreuil, M., Wattiaux, M., Hardie, C. A., and Cabrera, V. E. (2014). Feeding strategies and manure management for cost-effective mitigation of greenhouse gas emissions from dairy farms in Wisconsin. J. Dairy Sci. 97, 5904–5917. doi: 10.3168/jds.2014-8082
Dyrset, N., Havrevoll, E. Ø., Ratnaweer, H., and Birkeland, S.-E. (1999). Feed supplement recovered from dairy wastewater by biological and chemical pretreatment. J. Chem. Tech. Biotech. 73, 175–182. doi: 10.1002/(SICI)1097-4660(1998110)73:3<175::AID-JCTB936>3.0.CO;2-0
EBC (2012). European Biochar Certificate - Guidelines for a Sustainable Production of Biochar.' Arbaz: European Biochar Foundation (EBC). Available online at: http://european-biochar.org (accessed April 11, 2021).
Elsgaard, L., Olsen, A. B., and Petersen, S. O. (2016). Temperature response of methane production in liquid manures and co-digestates. Sci. Tot. Environ. 539, 78–84. doi: 10.1016/j.scitotenv.2015.07.145
Espinoza, L. T., Loibl, A., Langkau, S., De Koning, A., Van Der Voet, E., and Michaux, S. (2020). “Report on the future use of critical raw materials,” in Accessed the 5th of March 2021 From SCRREEN-D2.3-Report-on-the-Future-Use-of-Critical-Raw-Materials. Available online at: https://www.readkong.com/page/report-on-the-future-use-of-critical-raw-materials-scrreen-4073726 (assessed March 20, 2021).
Estrada, I. B., Aller, A., Aller, F., Gomez, X., and Moran, A. (2004). The survival of Escherichia coli, faecal coliforms and enterobacteriaceae in general in soil treated with sludge from wastewater treatment plants. Biores. Tech. 93, 191–198 doi: 10.1016/j.biortech.2003.10.022
European Commission (2003). Revision of the Fertilizers Regulation (EC). Available online at: No 2003/2003http://ec.europa.eu/smart-regulation/roadmaps/docs/2012_grow_001_fertilizers_en.pdf (assessed July 16, 2021).
European Commission (2017). Communication From the Commission to the European Parliament, the Council, the European Economic and Social Committee and the Committee of the Regions on the 2017 List of Critical Raw Materials for the EU. 2017. Available online at: https://eur-lex.europa.eu/legal-content/EN/TXT/PDF/?uri=CELEX:52017DC0490 (accessed January 1, 2021).
European Commission (2019). Regulation of the European Parliament and of the Council Laying Down Rules on the Making Available on the Market of EU Fertilising Products and Amending Regulations (EC) No 1069/2009 and (EC) No 1107/2009 and Repealing Regulation (EC) No 2003/2003. Available online at: https://data.consilium.europa.eu/doc/document/PE-76-2018-INIT/en/pdf (accessed March 5, 2021).
Eurostat (2020). European Database of Key Statistics Eurostat 2020. Available online at: http://ec.europa.eu/eurostat. 2020 (accessed January 4, 2021).
Falk Øgaard, A., and Brod, E. (2016). Efficient phosphorus cycling in food production: predicting the phosphorus fertilization effect of sludge from chemical wastewater treatment. J. Agric. Food Chem. 64, 4821–4829. doi: 10.1021/acs.jafc.5b05974
Fenton, O., Mellander, P. E., Daly, K., Wall, D. P., Jahangir, M. M. R., and Jordan, P. (2017). Integrated assessment of agricultural nutrient pressures and legacies in karst landscapes. Agric. Ecosys. Environ. 239, 246–256. doi: 10.1016/j.agee.2017.01.014
Fernández, J. M., Plaza, C., García-Gil, J. C., and Polo, A. (2009). Biochemical properties and barley yield in a semiarid Mediterranean soil amended with two kinds of sewage sludge. Appl. Soil Ecol. 42, 18–24. doi: 10.1016/j.apsoil.2009.01.006
Ferreira, T. F., Santos, P. A., Paula, A. V., de Castro, H. F., and Andrade, G. S. (2021). Biogas generation by hybrid treatment of dairy wastewater with lipolytic whole cell preparations and anaerobic sludge. Biochem. Eng. J. 169:107965. doi: 10.1016/j.bej.2021.107965
Fliesbach, A., Martens, R., and Reber, H. (1994). Soil microbial biomass and microbial activity in soils treated with heavy metal contaminated sewage sludge. Soil Biol. Biochem. 26, 1201–1205. doi: 10.1016/0038-0717(94)90144-9
Frac, M., and Jezierska-Tys, S. (2011). Agricultural utilisation of dairy sewage sludge: its effect on enzymatic activity and microorganisms of the soil environment. African J. Microbiol. Res. 5:707. doi: 10.5897/AJMR10.707
Frac, M., Jezierska-Tys, S., Oszust, K., Gryta, A., and Pastor, M. (2017). Assessment of microbiological and biochemical properties of dairy sewage sludge. Int. J. Environ. Sci. Technol. 14, 679–688. doi: 10.1007/s13762-016-1179-9
Frac, M., Oszust, K., and Lipiec, J. (2012). Community level physiological profiles (CLPP), characterization and microbial activity of soil amended with dairy sewage sludge. Sensors 12, 3253–3268. doi: 10.3390/s120303253
Franz, M. (2008). Phosphate fertilizer from sewage sludge ash (SSA). Waste Manage. 28, 1809–1818. doi: 10.1016/j.wasman.2007.08.011
Frossard, E., Sinaj, S., Zhang, L. M., and Morel, J. L. (1996). The fate of sludge phosphorus in soil-plant systems. Soil Sci. Soc. Am. J. 60, 1248–1253. doi: 10.2136/sssaj1996.03615995006000040041x
Frostegård, A., and Bååth, E. (1996). The use of phospholipid fatty acid analysis to estimate bacterial and fungal biomass in soil. Biol. Fertil. Soils 22, 59–65. doi: 10.1007/BF00384433
Gayathri, T., Kavitha, S., Kumar, S. A., Kaliappan, S., Yeom, I. T., and Banu, J. R. (2015). Effect of citric acid induced deflocculation on the ultrasonic pretreatment efficiency of dairy waste activated sludge. Ultras. Sonochem. 22, 333–340 doi: 10.1016/j.ultsonch.2014.07.017
Gogoi, M., Biswas, T., Biswal, P., Saha, T., Modak, A., and Gantayet, L. M. (2021). A novel strategy for microbial conversion of dairy wastewater into biofertilizer. J. Clean. Prod. 293:126051. doi: 10.1016/j.jclepro.2021.126051
Goli, A., Shamiri, A., Khosroyar, S., Talaiekhozani, A., Sanaye, R., and Azizi, K. (2019). A review on different aerobic and anaerobic treatment methods in dairy industry wastewater. J. Environ. Treat. Tech. 6, 113–141. Available online at: https://www.cabdirect.org/cabdirect/abstract/20219917863
Gómez-Muñoz, B., Magid, J., and Jensen, L. S. (2017). Nitrogen turnover, crop use efficiency and soil fertility in a long-term field experiment amended with different qualities of urban and agricultural waste. Agric. Ecosyst. Environ. 240, 300–313. doi: 10.1016/j.agee.2017.01.030
González Jiménez, J. L., Healy, M. G., and Daly, K. (2019). Effects of fertiliser on phosphorus pools in soils with contrasting organic matter content: a fractionation and path analysis study. Geoderma 338, 128–135. doi: 10.1016/j.geoderma.2018.11.049
Gryta, A., Frac, M., and Oszust, K. (2014). The application of the Biolog EcoPlate approach in ecotoxicological evaluation of dairy sewage sludge. Appl. Biochem. Biotechnol. 174, 1434–1443. doi: 10.1007/s12010-014-1131-8
Hall, R. L., Staal, L. B., Macintosh, K. A., McGrath, J. W., Bailey, J., Black, L., et al. (2020). Phosphorus speciation and fertiliser performance characteristics: a comparison of waste recovered struvites from global sources. Geoderma 362:114096. doi: 10.1016/j.geoderma.2019.114096
Hallin, S., Jones, C. M., Schloter, M., and Philippot, L. (2009). Relationship between N-cycling communities and ecosystem functioning in a 50-year-old fertilization experiment. ISME J. 3, 597–605. doi: 10.1038/ismej.2008.128
Hansen, M. N., Sommer, S. G., Hutchings, N. J., and Sørensen, P. (2008). Emissionsfaktorer til Beregning af Ammoniakfordampning Ved Lagring og Udbringning af Husdyrgødning. Tjele: DJF Husdyrbrug.
Haraldsen, T. K., Pedersen, P. A., and Grønlund, A. (2011). “Mixtures of bottom wood ash and meat and bone meal as NPK fertilizer,” in Recycling of Biomass Ashes (Berlin: Springer), 33–44. doi: 10.1007/978-3-642-19354-5_3
Harter, J., Krause, H.-M., Schuettler, S., Ruser, R., Fromme, M., Scholten, T., et al. (2014). Linking N2O emissions from biochar-amended soil to the structure and function of the N-cycling microbial community. ISME J. 8, 660–674. doi: 10.1038/ismej.2013.160
He, Y., Zhou, X., Jiang, L., Li, M., Du, Z., Zhou, G., et al. (2017). Effects of biochar application on soil greenhouse gas fluxes: a meta-analysis. Glob. Chan. Biol. Bioener. 9, 743–755. doi: 10.1111/gcbb.12376
Heckrath, G., Brookes, P. C., Poulton, P. R., and Goulding, K. W. T. (1995). Phosphorus leaching from soils containing different phosphorus concentrations in the broadbalk experiment. J. Environ. Qual. 24, 904–910. doi: 10.2134/jeq1995.00472425002400050018x
Herzel, H., Krüger, O., Hermann, L., and Adam, C. (2016). Sewage sludge ash—a promising secondary phosphorus source for fertilizer production. Sci. Total Environ. 542, 1136–1143. doi: 10.1016/j.scitotenv.2015.08.059
Hobbie, J. E., and Hobbie, E. A. (2013). Microbes in nature are limited by carbon and energy: the starving-survival lifestyle in soil and consequences for estimating microbial rates. Front. Microbiol. 4:324. doi: 10.3389/fmicb.2013.00324
Houben, D., Michel, E., Nobile, C., Lambers, H., Kandeler, E., and Faucon, M.-P. (2019). Response of phosphorus dynamics to sewage sludge application in an agroecosystem in northern France. Appl. Soil Ecol. 137, 178–186. doi: 10.1016/j.apsoil.2019.02.017
Houot, S., Pons, M.-N., and Pradel, M. (2014). Valorisation des Matières Fertilisantes D'origine Résiduaire Sur les sols à Usage Agricole ou Forestier: Impacts Agronomiques, Environnementaux, Soci-Economiques. Synthèse du Rapport D'expertises. Available online at: https://www.inrae.fr/sites/default/files/pdf/mafor-synthese-vf-oct2014.pdf
Hudcová, H., Vymazal, J., and Rozkošný, M. (2019). Present restrictions of sewage sludge application in agriculture within the European union. Soil Wat. Res. 14, 104–120. doi: 10.17221/36/2018-SWR
Huygens, D., Saveyn, H. G. M., Tonini, D., Eder, P., and Delgado Sancho, L. (2019). Technical Proposals for Selected New Fertilising Materials Under the Fertilising Products Regulation (Regulation (EU) 2019/1009) - Process and Quality Criteria, and Assessment of Environmental and Market Impacts for Precipitated Phosphate Salts & Derivates, Thermal Oxidation Materials & Derivates and Pyrolysis & Gasification Materials, EUR 29841 EN. Luxembourg: European Union.
IPCC (2006). “2006 IPCC guidelines for national greenhouse gas inventories,” in The National Greenhouse Gas Inventories Programme, eds, H. S. Eggleston, L. Buendia, K. Miwa, T. Ngara, and K. Tanabe (IGES). Available online at: http://www.ipcc-nggip.iges.or.jp/public/2006gl/pdf/4_Volume4/V4_10_Ch10_Livestock.pdf
IPCC (2019). Refinement to the 2006 IPCC Guidelines for National Greenhouse Gas Inventories. Available online at: https://www.ipcc-nggip.iges.or.jp/
Jezierska-Tys, S., and Frac, M. (2009). Impact of dairy sewage sludge on enzymatic activity and inorganic nitrogen concentrations in the soils. Int. Agroph. 23, 31–37. Available online at: https://www.cabdirect.org/cabdirect/abstract/20093094438
Jorge-Mardomingo, I., Soler-Rovira, P., Casermeiro, M. Á., de la Cruz, M. T., and Polo, A. (2013). Seasonal changes in microbial activity in a semiarid soil after application of a high dose of different organic amendments. Geoderma 206, 40–48. doi: 10.1016/j.geoderma.2013.04.025
Kahiluoto, H., Kuisma, M., Ketoja, E., Salo, T., and Heikkinen, J. (2015). Phosphorus in manure and sewage sludge more recyclable than in soluble inorganic fertilizer. Environ. Sci. Techn. 49, 2115–2122. doi: 10.1021/es503387y
Kambo, H. S., and Dutta, A. (2015). A comparative review of biochar and hydrochar in terms of production, physico-chemical properties and applications. Ren. Sust. Energy Rev. 45, 359–378. doi: 10.1016/j.rser.2015.01.050
Karadag, D., Köroglu, O. E., Ozkaya, B., and Cakmakci, M. (2015). A review on anaerobic biofilm reactors for the treatment of dairy industry wastewater. Process Biochem. 50, 262–271. doi: 10.1016/j.procbio.2014.11.005
Khan, M. S., Zaidi, A., and Wani, P. A. (2009). “Role of phosphate solubilizing microorganisms in sustainable agriculture: a review,” in Sustainable Agriculture, eds E. Lichtfouse, M. Navarrete, P. Debaeke, S. Véronique, C. Alberola (Dordrecht: Springer).
Khiari, L., Joseph, C.-A., Gallichand, J., Beecher, N., and Bouslama, S. (2020). Classification and assessment models of first year biosolids phosphorus bioavailability. Waste Biom. Val. 11, 1443–1452. doi: 10.1007/s12649-018-0490-8
Kizilkaya, R., and Bayrakli, B. (2005). Effects of N-enriched sewage sludge on soil enzyme activities. Appl. Soil Ecol. 30, 192–202. doi: 10.1016/j.apsoil.2005.02.009
Knapp, B. A., and Insam, H. (2011). “Recycling of biomass ashes: current technologies and future research needs,” in Recycling of Biomass Ashes (Berlin: Springer), 1–16. doi: 10.1007/978-3-642-19354-5_1
Knödler, M., Berger, M., and Dobrindt, U. (2016). Long-term survival of the Shiga toxin-producing Escherichia coli O104:H4 outbreak strain on fenugreek seeds. Food Microb. 59, 190–195. doi: 10.1016/j.fm.2016.06.005
Kolev Slavov, A. (2017). General characteristics and treatment possibilities of dairy wastewater: a review. Food Tech. Biotech. 55, 14–28. doi: 10.17113/ftb.55.01.17.4520
Krogstad, T., Sogn, T. A., Asdal, Å., and Sæb,ø, A. (2005). Influence of chemically and biologically stabilized sewage sludge on plant-available phosphorous in soil. Ecol. Eng. 25, 51–60. doi: 10.1016/j.ecoleng.2005.02.009
Kuligowski, K., Poulsen, T. G., Rubæk, G. H., and Sørensen, P. (2010). Plant-availability to barley of phosphorus in ash from thermally treated animal manure in comparison to other manure based materials and commercial fertilizer. Euro. J. Agron. 33, 293–303 doi: 10.1016/j.eja.2010.08.003
Kumar, G. P., Yadav, S. K., Thawale, P. R., Singh, S. K., and Juwarkar, A. A. (2008). Growth of Jatropha curcas on heavy metal contaminated soil amended with industrial wastes and azotobacter – a greenhouse study. Biores. Techn. 99, 2078–2082 doi: 10.1016/j.biortech.2007.03.032
Kunito, T., Saeki, K., Goto, S., Hayashi, H., Oyaizu, H., and Matsumoto, S. (2001). Copper and zinc fractions affecting microorganisms in long-term sludge-amended soils. Biores. Techn. 79, 135–146. doi: 10.1016/S0960-8524(01)00047-5
Kushwaha, J. P., Srivastava, V. C., and Mall, I. D. (2011). An overview of various technologies for the treatment of dairy wastewaters. Critic. Rev. Food Sci. Nutr. 51, 442–452. doi: 10.1080/10408391003663879
Kuypers, M. M. M., Marchant, H. K., and Kartal, B. (2018). The microbial nitrogen-cycling network. Nat. Rev. Microbiol. 16, 263–276. doi: 10.1038/nrmicro.2018.9
Kuzyakov, Y., Subbotina, I., Chen, H., Bogomolova, I., and Xu, X. (2009). Black carbon decomposition and incorporation into soil microbial biomass estimated by 14C labeling. Soil Biol. Biochem. 41, 210–219. doi: 10.1016/j.soilbio.2008.10.016
Kwapinska, M., Horvat, A., Liu, Y., and Leahy, J. J. (2020). Pilot scale pyrolysis of activated sludge waste from milk processing factory. Waste Biom. Valor. 11, 2887–2903. doi: 10.1007/s12649-019-00596-y
Kyere, E. O., Foong, G., Palmer, J., Wargent, J. J., Fletcher, G. C., and Flint, S. (2019). Rapid attachment of listeria monocytogenes to hydroponic and soil grown lettuce leaves. Food Control 101, 77–80. doi: 10.1016/j.foodcont.2019.02.015
Lal, R. (2009). Soils and food sufficiency. A review. Agron. Sustain. Dev. 29, 113–133. doi: 10.1051/agro:2008044
Lalor, S., Schröder, J., Lantinga, E., Oenema, O., Kirwan, L., and Schulte, R. (2011). Nitrogen fertilizer replacement value of cattle slurry in grassland as affected by method and timing of application. J. Environ. Qual. 40, 362–373. doi: 10.2134/jeq2010.0038
Laperche, D. (2014). Boues des Step Urbaines: le Retour D'expérience des Adhérents D'AMORCE. 5/12/2012. Actu Environnement.com. Available online at: https://www.actu-environnement.com/ae/news/boues-step-urbaines-retour-experience-adherents-amorce-17228.php4 (accessed on July 27, 2021).
Lateef, S. K., Soh, B. Z., and Kimura, K. (2013). Direct membrane filtration of municipal wastewater with chemically enhanced backwash for recovery of organic matter. Biores. Techn. 150, 149–155. doi: 10.1016/j.biortech.2013.09.111
Le Noë, J., Garnier, J., and Billen, G. (2018). Phosphorus management in cropping systems of the Paris basin: from farm to regional scale. J. Environ. Man. 205, 18–28. doi: 10.1016/j.jenvman.2017.09.039
Lehmann, J., and Joseph, S. (2015). Biochar for Environmental Management - Science, Technology and Implementation, 2nd edn. New York, NY: Routledge.
Liao, X., Niu, Y., Liu, D., Chen, Z., He, T., Luo, J., et al. (2020). Four-year continuous residual effects of biochar application to a sandy loam soil on crop yield and N2O and NO emissions under maize-wheat rotation. Agric. Ecos. Environ. 302:107109. doi: 10.1016/j.agee.2020.107109
Liu, X., Liu, W., Wang, Q., Wu, L., Luo, Y., and Christie, P. (2017). Soil properties and microbial ecology of a paddy field after repeated applications of domestic and industrial sewage sludges. Environ. Sci. Pollut. Res. 24, 8619–8628. doi: 10.1007/s11356-017-8543-2
Liu, Y.-Y., and Haynes, R. J. (2010). Long-term irrigation with dairy factory wastewater influences soil quality world academy of science. Eng. Technol. Int. Sci. Index 46, 524–528. doi: 10.5281/zenodo.1075935
Liu, Y.-Y., and Haynes, R. J. (2011). Origin, nature, and treatment of effluents from dairy and meat processing factories and the effects of their irrigation on the quality of agricultural soils. Crit. Rev. Environ. Sci. Technol. 41, 1531–1599. doi: 10.1080/10643381003608359
Lizarralde, C. A., McDowell, R. W., Condron, L. M., and Brown, J. N. (2021). Potential phosphorus losses from grassland soils irrigated with dairy factory wastewater. Nutr. Cycl. Agroecosys. doi: 10.1007/s10705-021-10165-2
López-Mosquera, M., Moirón, C., and Carral, E. (2000). Use of dairy-industry sludge as fertiliser for grasslands in northwest Spain: heavy metal levels in the soil and plants. Resour. Conserv. Recycl. 30, 95–109. doi: 10.1016/S0921-3449(00)00058-6
Lorenz, K., and Lal, R. (2014). Biochar application to soil for climate change mitigation by soil organic carbon sequestration. J. Plant Nutrit. Soil Sci. 177, 651–670. doi: 10.1002/jpln.201400058
Lu, Q., He, Z. L., and Stoffella, P. J. (2012). Land application of biosolids in the USA: a review. Appl. Environ. Soil Sci. 2012:201462. doi: 10.1155/2012/201462
Luo, G., Ling, N., Nannipieri, P., Chen, H., Raza, W., Wang, M., et al. (2017). Long- term fertilisation regimes affect the composition of the alkaline phosphomonoesterase encoding microbial community of a vertisol and its derivative soil fractions. Biol. Fertil. Soils 53, 375–388. doi: 10.1007/s00374-017-1183-3
Maillard, E. M., and Angers, D. A. (2014). Animal manure application and soil organic carbon stocks: a meta-analysis. Glob. Chan. Biol. 20, 666–679. doi: 10.1111/gcb.12438
Major, J., Lehmann, J., Rondon, M., and Goodale, C. (2010). Fate of soil-applied black carbon: downward migration, leaching and soil respiration. Glob. Chan. Biol. 16, 1366–1379. doi: 10.1111/j.1365-2486.2009.02044.x
Major, N., Schierstaedt, J., Jechalke, S., Nesme, J., Ban, S. G., Cerne, M., et al. (2020). Composted sewage sludge influences the microbiome and persistence of human pathogens in soil. Microorganisms 8:1020. doi: 10.3390/microorganisms8071020
Majumder, S., Neogi, S., Dutta, T., Powel, M. A., and Banik, P. (2019). The impact of biochar on soil carbon sequestration: meta-analytical approach to evaluating environmental and economic advantages. J. Environ. Manag. 250:109466. doi: 10.1016/j.jenvman.2019.109466
Malghani, S., Gleixner, G., and Trumbore, S. E. (2013). Chars produced by slow pyrolysis and hydrothermal carbonization vary in carbon sequestration potential and greenhouse gases emissions. Soil Biol. Biochem. 62, 137–146. doi: 10.1016/j.soilbio.2013.03.013
Marklein, A. R., and Houlton, B. Z. (2012). Nitrogen inputs accelerate phosphorus cycling rates across a wide variety of terrestrial ecosystems. New Phytol. 193, 696–704 doi: 10.1111/j.1469-8137.2011.03967.x
Markowicz, A., Bondarczuk, K., Cycoń, M., and Sułowicz, S. (2021). Land application of sewage sludge: response of soil microbial communities and potential spread of antibiotic resistance. Environ. Pollut. 271, 116317. doi: 10.1016/j.envpol.2020.116317
Mayer, B. K., Baker, L. A., Boyer, T. H., Drechsel, P., Gifford, M., Hanjra, M. A., et al. (2016). Total value of phosphorus recovery. Environ. Sci. Technol. 50, 6606–6620. doi: 10.1021/acs.est.6b01239
Montgomery, M. B., Ohno, T., Griffin, T. S., Honeycutt, C. W., and Fernanadez, I. J. (2005). Phosphorus mineralization and availability in soil amended with biosolids and animal manures. Biol. Agric. Hort. 22, 321–334. doi: 10.1080/01448765.2005.9755295
Mottet, A., Francois, E., Latrille, E., Steyer, J. P., Déléris, S., Vedrenne, F., et al. (2010). Estimating anaerobic biodegradability indicators for waste activated sludge. Chem. Engin. J. 160, 488–496. doi: 10.1016/j.cej.2010.03.059
Murnane, J. G., Brennan, R. B., Fenton, O., and Healy, M. G. (2016). Zeolite combined with alum and aluminium chloride mixed with agricultural slurries reduces carbon losses in runoff from grassed soil boxes. J. Environ. Qual. 44, 1674–1683. doi: 10.2134/jeq2014.07.0319
Myhre, G., Shindell, D., Bréon, F.-M., Collins, W., Fuglestvedt, J., Huang, J., et al. (2013). “Anthropogenic and Natural Radiative Forcing,” in Climate Change 2013: The Physical Science Basis. Contribution of Working Group I to the Fifth Assessment Report of the Intergovernmental Panel on Climate Change, eds T. F. Stocker, D. Qin, G. K. Plattner, M. Tignor, S. K. Allen, J. Boschung, et al. Cambridge; New York, NY: Cambridge University Press.
Neal, C., and Heathwaite, A. L. (2005). Nutrient mobility within river basins: a European perspective. J. Hydrol. 304, 477–490 doi: 10.1016/j.jhydrol.2004.07.045
Nest, T. V., Amery, F., Fryda, L., Boogaerts, C., Bilbao, J., and Vandecasteele, B. (2021). Renewable P sources: P use efficiency of digestate, processed animal manure, compost, biochar and struvite. Sci. Tot. Environ. 750:141699. doi: 10.1016/j.scitotenv.2020.141699
Nicolás, C., Kennedy, J. N., Hernández, T., García, C., and Six, J. (2014). Soil aggregation in a semiarid soil amended with composted and non-composted sewage sludge—a field experiment. Geoderma 219, 24–31. doi: 10.1016/j.geoderma.2013.12.017
Nielsen, O.-K., Plejdrup, M. S., Winther, M., Nielsen, M., Gyldenkærne, S., and Mikkelsen, M. H. (2018). “Denmark's national inventory report 2018. Emission inventories 1990-2016 - submitted under the United Nations Framework convention on climate change and the kyoto protocol,” in DCE–Danish Centre for Environment and Energy. Scientific Report From DCE –Danish Centre for Environment and Energy No. 272. Aarhus University. Available online at: http://dce2.au.dk/pub/SR272.pdf
Olesen, J. E., Møller, H. B., Petersen, S. O., Sørensen, P., Nyord, T., and Sommer, S. G. (2020). Bæredygtig Biogas – Klima og Miljø-Effekter af Biogasproduktion. Aarhus Universitet; DCA–Nationalt Center for Fødevarer og Jordbrug. Available online at: https://dcapub.au.dk/djfpdf/DCArapport175.pdf
Omil, F., Garrido, J. M., Arrojo, B., and Méndez, R. (2003). Anaerobic filter reactor performance or the treatment of complex dairy wastewater at industrial scale. Water Res. 37, 4099–4108. doi: 10.1016/S0043-1354(03)00346-4
Oszust, K., Frac, M., and Lipiec, J. (2015). Soil microbial functionality in response to dairy sewage sludge and mineral fertilisers application under winter rape. Int. J. Environ. Sci. Technol. 12, 3675–3684. doi: 10.1007/s13762-015-0807-0
Ott, C., and Rechberger, H. (2012). The European phosphorus balance. Resour. Conser. Recyc. 60, 159–172. doi: 10.1016/j.resconrec.2011.12.007
Page, M. J., McKenzie, J. E., Bossuyt, P. M., Boutron, S. E., Hoffman, I., et al. (2021). The PRISMA 2020 statement: an updated guideline for reporting systematic reviews. BMJ 372:n71. doi: 10.31222/osf.io/v7gm2
Paredes, C., Menezes-Blackburn, D., Cartes, P., Gianfreda, L., and Mora, M. L. (2011). Phosphorus and nitrogen fertilization effect on phosphorus uptake and phosphatase activity in ryegrass and tall fescue grown in a Chilean Andisol. Soil Sci. 176, 245–251. doi: 10.1097/SS.0b013e3182147fd3
Parham, J., Deng, S., Raun, W., and Johnson, G. (2002). Long-term cattle manure application in soil. Biol. Fertil. Soils 35, 328–337. doi: 10.1007/s00374-002-0476-2
Pedersen, B. N., Eriksen, J., Christensen, B. T., and Sørensen, P. (2021). Fertilizer replacement value and leaching of nitrogen applied to spring barley in cattle deep litter: a 3-year lysimeter study. Soil Till. Res. 209:104954. doi: 10.1016/j.still.2021.104954
Peltre, C., Thuriès, L., Barthès, B., Brunet, D., Morvan, T., Nicolardot, B., et al. (2011). Near infrared reflectance spectroscopy: a tool to characterize the composition of different types of exogenous organic matter and their behaviour in soil. Soil Biol. Biochem. 43, 197–205. doi: 10.1016/j.soilbio.2010.09.036
Pérez, S., La Farré, M., García, J.M., and Barceló, D. (2001). Occurrence of polycyclic aromatic hydrocarbons in sewage sludge and their contribution to its toxicity in the ToxAlert® 100 bioassay. Chemosphere 45, 705–712. doi: 10.1016/S0045-6535(01)00152-7
Petersen, S. O., Olsen, A. B., Elsgaard, L., Triolo, J. M., and Sommer, S. G. (2016). Estimation of methane emissions from slurry pits below pig and cattle confinements. PLoS ONE 11 :e0160968. doi: 10.1371/journal.pone.0160968
Petersen, S. O., Petersen, J., and Rubæk, G. H. (2003). Dynamics and plant uptake of nitrogen and phosphorus in soil amended with sewage sludge. Appl. Soil Ecol. 24, 187–195. doi: 10.1016/S0929-1393(03)00087-8
Peyton, D. P., Healy, M. G., Fleming, G. T. A., Grant, J., Wall, D. P., and Morrison, L. (2016). Nutrient, metal and microbial loss in surface runoff following treated sludge and dairy cattle slurry application to an Irish grassland soil. Sci. Total Environ. 541, 218e229. doi: 10.1016/j.scitotenv.2015.09.053
Prasad, R., Pathak, S., and Patra, A, Shivay, Y. (2014). Textbook of Plant Nutrient Management. New Delhi: Indian Society of Agronomy.
Prasad, R., Shivay, Y. S., Majumdar, K., and Prasad, S. (2016). “Phosphorus management,” in Soil Phosphorus, eds R. Lal and B. A. Stewart (Boca Raton, FL: CRC Press), 81–113. doi: 10.1201/9781315372327
Rani, R. U., Kaliappan, S., Kumar, S. A., and Rajesh Banu, J. (2012). Combined treatment of alkaline and disperser for improving solubilization and anaerobic biodegradability of dairy waste activated sludge. Bioresou. Techn. 126, 107–116 doi: 10.1016/j.biortech.2012.09.027
Ravva, S. V., Sarreal, C. Z., Duffy, B., and Stanker, L. H. (2006). Survival of Escherichia coli O157:H7 in wastewater from dairy lagoons. J. Appl. Microbiol. 101, 891–902. doi: 10.1111/j.1365-2672.2006.02956.x
Rico Gutiérrez, J. L., Garcia, P., and Fdz-Polanco, F. (1991). Anaerobic treatment of cheese production wastewater using a UASB reactor. Biores. Tech. 37, 271–276. doi: 10.1016/0960-8524(91)90194-O
Rivas, J., Prazeres, A. R., Carvalho, F., and Beltrán, F. (2010). Treatment of cheese whey wastewater: combined coagulation–flocculation and aerobic biodegradation. J. Agric. Food Chem. 58, 7871–7877. doi: 10.1021/jf100602j
Rizzo, L., Manaia, C., Merlin, C., Schwartz, T., Dagot, C., Ploy, M. C., et al. (2013). Urban wastewater treatment plants as hotspots for antibiotic resistant bacteria and genes spread into the environment: a review. Sci. Total Environ. 447, 345–360. doi: 10.1016/j.scitotenv.2013.01.032
Rotz, C. A., and Hafner, S. D. (2011). “Whole farm impact of anaerobic digestion and biogas use on a New York dairy farm,” in American Society Agricultre Biological Engineers, 2011 (Louisville). doi: 10.13031/2013.37768
Roy, T., Biswas, D. R., Ghosh, A., Patra, A. K., Singh, R. D., Sarkar, A., et al. (2019). Dynamics of culturable microbial fraction in an Inceptisol under short-term amendment with municipal sludge from different sources. Appl. Soil Ecol. 136, 116–121. doi: 10.1016/j.apsoil.2018.12.024
Rumpel, C., Alexis, M., Chabbi, A., Chaplot, V., Rasse, D. P., Valentin, C., et al. (2006). Black carbon contribution to soil organic matter composition in tropical sloping land under slash and burn agriculture. Geoderma 130, 35–46. doi: 10.1016/j.geoderma.2005.01.007
Rutgersson, C., Ebmeyer, S., Lassen, S. B., Karkman, A., Fick, J., Kristiansson, E., et al. (2020). Long-term application of Swedish sewage sludge on farmland does not cause clear changes in the soil bacterial resistome. Environ. Int. 137:105339. doi: 10.1016/j.envint.2019.105339
Scholz, R. W., and Wellmer, F. W. (2013). Approaching a dynamic view on the availability of mineral resources: what we may learn from the case of phosphorus? Glob. Environ. Change 23, 11–27. doi: 10.1016/j.gloenvcha.2012.10.013
Schoumans, O. F., Bouraoui, F., Kabbe, C., Oenema, O., and van Dijk, K. C. (2015). Phosphorus management in Europe in a changing world. AMBIO 44, 180–192. doi: 10.1007/s13280-014-0613-9
Schröder, J. J., Uenk, D., and Hilhorst, G. J. (2007). Long-term nitrogen fertilizer replacement value of cattle manures applied to cut grassland. Plant Soil 299, 83–99. doi: 10.1007/s11104-007-9365-7
Science Communication Unit University of the West of England Bristol (2013). Science for Environment Policy in depth Report: Sustainable Phosphorus Use. Report produced for the European Commission DG Environment. Available online at: http://ec.europa.eu/science-environment-policy.
Scott, A., Ball, B. C., Crichton, I. J., and Aitken, M. N. (2000). Nitrous oxide and carbon dioxide emissions from grassland amended with sewage sludge. Soil Use Manage. 16, 36–41. doi: 10.1111/j.1475-2743.2000.tb00170.x
Shackley, S., Sohi, S., Brownsort, P., Carter, S., Cook, J., Cunningham, C., et al. (2010). An Assessment of the Benefits and Issues Associated With the Application of Biochar to Soil. London: Department for Environment, Food and Rural Affairs, UK Government.
Shakoor, A., Shahzad, S. M., Chatterjee, N., Arif, M. S., Farooq, T. H., Altaf, M. M., et al. (2021). Nitrous oxide emission from agricultural soils: application of animal manure or biochar? A global meta-analysis. J. Environ. Manag. 285:112170. doi: 10.1016/j.jenvman.2021.112170
Shi, W., Fenton, O., Ashekuzzaman, S. M., Daly, K., Leahy, J. J., Khalaf, N., et al. (2021b). An examination of maximum legal application rates of dairy processing and associated STRIBIAS fertilising products in agriculture. J. Environ. Manage. 301:113880. doi: 10.1016/j.jenvman.2021.113880
Shi, W., Healy, M. G., Ashekuzzaman, S. M., Daly, K., Leahy, J. J., and Fenton, O. (2021a). Dairy processing sludge and co-products: a review of present and future re-use pathways in agriculture. J. Clean. Prod. 281:128035. doi: 10.1016/j.jclepro.2021.128035
Siebielec, G., Siebielec, S., and Lipski, D. (2018). Long-term impact of sewage sludge, digestate and mineral fertilizers on plant yield and soil biological activity. J. Clean. Prod. 187, 372–379. doi: 10.1016/j.jclepro.2018.03.245
Sigurnjak, I., Brienza, C., Snauwaert, E., De Dobbelaere, A., De Mey, J., and Vaneeckhaute, C. (2019). Production and performance of bio-based mineral fertilizers from agricultural waste using ammonia (stripping-) scrubbing technology. Waste Manage. 89, 265–274. doi: 10.1016/j.wasman.2019.03.043
Singh, B. K., Quince, C., Macdonald, C. A., Khachane, A., Thomas, N., Al-Soud, W. A., et al. (2014). Loss of microbial diversity in soils is coincident with reductions in some specialized functions. Environ. Microbiol. 16, 2408–2420. doi: 10.1111/1462-2920.12353
Singh, R. P., and Agrawal, M. (2008). Potential benefits and risks of land application of sewage sludge. Waste Manag. 28, 347–358. doi: 10.1016/j.wasman.2006.12.010
Sivaprakasam, S., and Balaji, K. (2021). A review of upflow anaerobic sludge fixed film (UASFF) reactor for treatment of dairy wastewater materials today. Proceeding 43, 1879–1883. doi: 10.1016/j.matpr.2020.10.822
Smith, M. T. E., and Tibbett, M. (2004). Nitrogen dynamics under Lolium perenne after a single application of three different sewage sludge types from the same treatment stream. Bioresour. Technol. 91, 233–241. doi: 10.1016/S0960-8524(03)00205-0
Sobsey, M. D., Khatib, L. A., Hill, V. R., Alocilja, E., and Pillai, S. (2006). “Pathogens in animal wastes and the impacts of waste management practices on their survival, transport and fate,” in Animal Agriculture and the Environment, National Center for Manure & Animal Waste Management White Papers (Michigan: American Society of Agricultural and Biological Engineers).
Sommer, S. G., Christensen, M. L., Schmidt, T., and Jensen, L. S. (2013). Animal Manure Recycling: Treatment and Management, 1st Edn (Chichester: John Wiley & Sons).
Sommer, S. G., and Knudsen, L. (2021). Impact of Danish livestock and manure management regulations on nitrogen pollution, crop production, and economy. Front. Sustain. 2:658231. doi: 10.3389/frsus.2021.658231
Sommer, S. G., Schjørring, J. K., and Denmead, O. T. (2004). Ammonia volatilization from mineral fertilizers and plants in fields amended with ammoniacal fertilizers. Advan. Agron. 82, 557–662. doi: 10.1016/S0065-2113(03)82008-4
Sørensen, P., and Jensen, L. S. (2013). “Nutrient leaching and runoff from land application of animal manure and measures for reduction,” in Animal Manure Recycling: Treatment and Management, 1st edn. eds S. G. Sommer, M. L. Christensen, T. Schmidt, and L. S. Jensen (Chichester: John Wiley & Sons, Ltd).
Sørensen, P., and Rubæk, G. H. (2012). Leaching of nitrate and phosphorus after autumn and spring application of separated solid animal manures to winter wheat. Soil Use Manag. 28, 1–11. doi: 10.1111/j.1475-2743.2011.00382.x
Speir, T. W., van Schaik, A. P., Hunter, L. C., Ryburn, J. L., and Percival, H. J. (2007). Attempts to derive EC50 values for heavy metals from land-applied Cu-, Ni-, and Zn-spiked sewage sludge. Soil Biol. Biochem. 39, 539–549. doi: 10.1016/j.soilbio.2006.08.023
Spokas, K. A., Baker, J. M., and Reicosky, D. C. (2010). Ethylene: potential key for biochar amendment impacts. Plant Soil. 333, 443–452. doi: 10.1007/s11104-010-0359-5
Sri Shalini, S., Palanivelu, K., Ramachandran, A., and Raghavan, V. (2020). Biochar from biomass waste as a renewable carbon material for climate change mitigation in reducing greenhouse gas emissions—a review. Biomass Conver. Bioref. 11, 2247–2267. doi: 10.1007/s13399-020-00604-5
Stevenson, F. J., and Cole, M. A. (1999). Cycles of Soil: Carbon, Nitrogen, Phosphorus, Sulphur, Micronutrients, 2nd edn. New York, NY: John Wiley & Sons, Inc.
Subedi, R., Kammann, C., Pelissetti, S., Taupe, N., Bertora, C., Monaco, S., et al. (2015). Does soil amended with biochar and hydrochar reduce ammonia emissions following the application of pig slurry? Eur. J. Soil Sci. 66, 1044–1053. doi: 10.1111/ejss.12302
Sustarsic, M. (2009). “Waste water treatment: understanding the activated sludge process,” in Safety in Ammonia Plants and Related Facilities Symposium (San Francisco, CA), 26–29.
Taghizadeh-Toosi, A., Clough, T. J., Condron, L. M., Sherlock, R. R., Anderson, C. R., and Craigie, R. A. (2011). Biochar incorporation into pasture soil suppresses in situ nitrous oxide emissions from ruminant urine patches. J. Environ. Manag. 40, 468–476. doi: 10.2134/jeq2010.0419
Taghizadeh-Toosi, A., Clough, T. J., Sherlock, R. R., and Condron, L. M. (2012). Biochar adsorbed ammonia is bioavailable. Plant Soil 350, 57–69. doi: 10.1007/s11104-011-0870-3
Tchounwou, P. B., Yedjou, C. G., Patlolla, A. K., and Sutton, D. J. (2012). “Heavy metal toxicity and the environment. PMC 101, 133–164. doi: 10.1007/978-3-7643-8340-4_6
Thers, H., Abalos, D., Dörsch, P., and Elsgaard, L. (2020). Nitrous oxide emissions from oilseed rape cultivation were unaffected by flash pyrolysis biochar of different type, rate and field ageing. Sci. Tot. Environ. 724:138140. doi: 10.1016/j.scitotenv.2020.138140
Thomsen, I. K., Olesen, J. E., Møller, H. B., Sørensen, P., and Christensen, B. T. (2013). Carbon dynamics and retention in soil after anaerobic digestion of dairy cattle feed and faeces. Soil Biol. Biochem. 58, 82–87. doi: 10.1016/j.soilbio.2012.11.006
Thygesen, A. M., Wernberg, O., Skou, E., and Sommer, S. G. (2011). Effect of incineration temperature on phosphorus availability in bio-ash from manure. Environ. Techn. 32, 633–638. doi: 10.1080/09593330.2010.509355
Tian, G., Granato, T. C., Cox, A. E., Pietz, R. I., and Carlson, Jr. C. R. (2009). Soil carbon sequestration resulting from long-term application of biosolids for land reclamation. J. Environ. Qual. 38, 61–74. doi: 10.2134/jeq2007.0471
Torsvik, V., Daae, F. L., Sandaa, R. A., and Øvreås, L. (1998). Novel techniques for analysing microbial diversity in natural and perturbed environments. J. Biotechnol. 64, 53–62. doi: 10.1016/S0168-1656(98)00103-5
Urra, J., Alkorta, I., Mijangos, I., Epelde, L., and Garbisu, C. (2019). Application of sewage sludge to agricultural soil increases the abundance of antibiotic resistance genes without altering the composition of prokaryotic communities. Sci. Total Environ. 647, 1410–1420. doi: 10.1016/j.scitotenv.2018.08.092
Uusi-Kämppä, J., and Heinonen-Tanski, H. (2008). Evaluating slurry broadcasting and injection to ley for phosphorus losses and fecal microorganisms in surface runoff. J. Environ. Qual. 37, 2339–2350. doi: 10.2134/jeq2007.0428
Verma, A., and Singh, A. (2017). Physico-Chemical analysis of dairy industrial effluent. Int. J. Curr. Microbiol. App. Sci. 6, 1769–1775 doi: 10.20546/ijcmas.2017.607.213
Walstra, P., Wouters, J. T. M., and Geurts, T. J. (2005). Dairy Science and Technology, 2nd ed. Boca Raton, FL: CRC Press. doi: 10.1201/9781420028010
Wang, J. Y., Xiong, Z. Q., and Kuzyakov, Y. (2016). Biochar stability in soil: meta-analysis of decomposition and priming effects. GCB Bioenergy 8, 512–523. doi: 10.1111/gcbb.12266
Webb, J., Sørensen, P., Velthof, G., Amon, B., Pinto, M., Rodhe, L., et al. (2013). An assessment of the variation of manure nitrogen efficiency throughout Europe and an appraisal of means to increase manure-N efficiency. Adv. Agron. 119, 371–442. doi: 10.1016/B978-0-12-407247-3.00007-X
Wu, H., Lai, C., Zeng, G., Liang, J., Chen, J., Xu, J., et al. (2017). The interactions of composting and biochar and their implications for soil amendment and pollution remediation: a review. Crit. Rev. Biotech. 37, 754–764. doi: 10.1080/07388551.2016.1232696
Xing, J., Sun, S., Wang, H., Brookes, P. C., and Xu, J. (2020). Response of soil native microbial community to Eschericia coli O157:H7 invasion. Environ. Pollut. 261:114225. doi: 10.1016/j.envpol.2020.114225
Xu, H., He, P., Gu, W., Wang, G., and Shao, L. (2012). Recovery of phosphorus as struvite from sewage sludge ash. J. Environ. Sci. 24, 1533–1538. doi: 10.1016/S1001-0742(11)60969-8
Yadav, S. K., Juwarkar, A. A., Kumar, G. P., and Thawale, P. R. (2009). Bioaccumulation and phyto-translocation of arsenic, chromium and zinc by Jatropha curcas L.: impact of dairy sludge and biofertilizer. Biores. Techn. 100, 4616–4622. doi: 10.1016/j.biortech.2009.04.062
Yoshida, H., Nielsen, M. P., Scheutz, C., Jensen, L. S., Christensen, T. H., Nielsen, S., et al. (2015). Effects of sewage sludge stabilization on fertilizer value and greenhouse gas emissions after soil application. Acta Agric. Scan. Sect. B Soil Plant Sci. 65, 506–516. doi: 10.1080/09064710.2015.1027730
Yusiharni, B. E., Ziadi, H., and Gilkes, R. J. (2007). A laboratory and glasshouse evaluation of chicken litter ash, wood ash and iron smelting slag as liming agents and P fertilizers. Aust. J. Soil Res. 45, 374–389. doi: 10.1071/SR06136
Keywords: circular economy, phosphorus, environment–agriculture, bio-fertilizer, gaseous emissions (greenhouse gases and ammonia emissions)
Citation: Hu Y, Khomenko O, Shi W, Velasco-Sánchez Á, Ashekuzzaman SM, Bennegadi-Laurent N, Daly K, Fenton O, Healy MG, Leahy JJ, Sørensen P, Sommer SG, Taghizadeh-Toosi A and Trinsoutrot-Gattin I (2021) Systematic Review of Dairy Processing Sludge and Secondary STRUBIAS Products Used in Agriculture. Front. Sustain. Food Syst. 5:763020. doi: 10.3389/fsufs.2021.763020
Received: 23 August 2021; Accepted: 23 September 2021;
Published: 10 November 2021.
Edited by:
Shubiao Wu, Aarhus Institute of Advanced Studies, DenmarkReviewed by:
Jorge Paz-Ferreiro, RMIT University, AustraliaJosé L. S. Pereira, Instituto Politecnico de Viseu, Portugal
Copyright © 2021 Hu, Khomenko, Shi, Velasco-Sánchez, Ashekuzzaman, Bennegadi-Laurent, Daly, Fenton, Healy, Leahy, Sørensen, Sommer, Taghizadeh-Toosi and Trinsoutrot-Gattin. This is an open-access article distributed under the terms of the Creative Commons Attribution License (CC BY). The use, distribution or reproduction in other forums is permitted, provided the original author(s) and the copyright owner(s) are credited and that the original publication in this journal is cited, in accordance with accepted academic practice. No use, distribution or reproduction is permitted which does not comply with these terms.
*Correspondence: Owen Fenton, owen.fenton@teagasc.ie