- 1Ifremer, Université de la Polynésie Française, Institut Louis Malardé (ILM), Institut de recherche pour le développement (IRD), UMR 241 SECOPOL, Tahiti, French Polynesia
- 2Ifremer, Unité Recherches et Développements Technologiques (RDT), Plouzané, France
Microbial diversity plays key role in marine ecosystems, and quantifying the impact of plastic pollution on these organisms is essential to better anticipate and manage threats to these fragile ecosystems. In nine simplified tropical ecosystems (i.e. mesocosms), we tested a concentration gradient of macroplastics reflecting the amount of plastic released by pearl farms. In each mesocosm, we collected bacterial samples from three different compartments: macroplastics, water and animals, Tridacna maxima. The objective was to test how plastic concentration influences the bacterial community, whether certain bacteria respond similarly across these compartments, and to define a threshold concentration of plastic that would impact marine bacteria. We observed that over 70 % of the variability in the bacterial community was explained by the type of sample (51.8 %) and time (19.4 %). On a finer scale, we found that the abundance of 33 bacterial genera was significantly correlated with plastic pollution, with the highest concentration (4.05 g/L) accounting for the vast majority of the signal. The occurrence of these bacterial genera increased with high plastic concentrations, suggesting imbalanced competitive relationships favoring less pollutant-sensitive genera. Some of these bacteria were shared across compartments and have known ecological functions, including plastic degradation and pathogenicity. Our results align with prior studies that warn plastics can alter microbial interactions and promote the emergence of pathogenic families.
1 Introduction
The annual global production of plastic reached a record of 390 million tons in 2021 (Plastics Europe, 2022). A portion of this plastic will have a poorly managed end-of-life and will end up in the oceans. In 2023 alone, it is estimated that 0.5 million tons of plastics have surged from continents into the oceans (Kaandorp et al., 2023). Once at sea, this waste causes numerous disruptions to marine ecosystems and negatively impacts ecosystem services (Beaumont et al., 2019). Grim images of marine mammals, turtles, birds, and even sharks entangled in ghost nets or choking on food packaging are all too common (Laist, 1997; Gall and Thompson, 2015). However, these consequences represent only the emerged (albeit submerged) and highly publicized part of the iceberg of detrimental effects that plastics have on the marine environment. Plastic also affects the smallest life in our oceans, marine microbes that play a pivotal role in the marine food web, photosynthetic primary production, and biogeochemical cycling (Lear et al., 2021).
Research efforts on the species making up the "plastisphere", i.e. the collective organisms colonizing the surface of plastics, are currently underway, while only a few studies have examined the impact of plastics on marine microbial communities (Tetu et al., 2020). Most studies linking marine bacteria to plastic pollution primarily investigate the dynamics of bacterial colonization on plastics and/or the potential of specific bacterial genera to degrade polymers (Jacquin et al., 2019). A less studied, yet not harmless, effect of plastic is its ability to serve as a surface for the colonization and transportation of potentially pathogenic microbial species (Dussud et al., 2018; Lemonnier et al., 2022), a phenomenon referred to as the "raft effect". Understanding the impact of plastics on the bacterial community composition and identifying which pathogenic microbial groups use plastic as a new niche or benefit from plastic pollution will help to further pinpoint polluted ecosystems (Tetu et al., 2020). These gaps in our understanding become even more crucial when considering the significance of bacteria in all major marine biogeochemical cycles. For instance, a recent study demonstrated a significant decrease in the photosynthetic activity of the ocean’s most abundant photosynthetic bacteria, Prochlorococcus, due to a range of plastic leachate dilutions, from 3.125 % to 50 % HDPE (high density polyethylene) and from 0.25 to 10 % PVC (polyvinyl chloride) in relation to the volumetric ratio of seawater (Tetu et al., 2019).
In Polynesia, the pearl farming sector represents the country’s second largest economic resource after tourism (DRM, 2022). Most of the installations used in pearl farms are made of plastic. The extensive use of plastic, coupled with poor waste management, has made pearl farming one of the identified sources of macro and microplastic litter (< 5 mm) in Polynesian atolls (Andréfouët et al., 2014). A recent study quantifying the amount of waste generated annually by pearl farming practices revealed that 369 tons per year of plastic waste were generated by this activity on Mangareva atoll alone in the Gambier archipelago (Crusot et al., 2023). The deleterious effects of physical pollution caused by the presence of plastic microbeads have already been demonstrated on energy balance, gametogenesis and transcriptomic disturbances in Pinctada margaritifera, the Polynesian pearl oyster (Gardon et al., 2018, 2020b). Chemical pollution induced by the leaching products of new pearl farming plastics on marine organisms has also been singled out, causing an increased mortality and malformations during larval development in tropical species (Gardon et al., 2020a). As pearl farming occurs in the vast majority of Polynesian lagoons, it is essential to characterize the potential impacts of chemical pollution generated by this activity on the marine ecosystem. Moreover, identifying a sentinel species exhibiting early markers of exposure to contaminants released by plastics is crucial for setting up a future monitoring network to assess the state of pollution in lagoons (Multisanti et al., 2022).
To date, studies investigating the impact of chemical pollution arising from pearl farming materials have focused on pearl oysters, Pinctada margaritifera (Gardon et al., 2020a). No study has yet addressed the impact of plastic concentration on taxonomic composition and abundance of marine bacteria communities in the context of pearl farming. To conduct our experiments, we established nine mesocosms representing the marine ecosystem of French Polynesia since mesocosms has been proved to be an efficient tool to investigate plastic impact on the marine ecosystem (Lott et al., 2020). Mesocosm, i.e. defined as a “bounded and partially enclosed outdoor experiment to bridge the gap between the laboratory and the real world in environmental science” (Odum, 1984; Crossland and La Point, 1992; Bruckner et al., 1995) approaches offer the possibility to implement scenarios that are more realistic than in vitro experiments, while enabling better control and understanding, or even minimization of the variations observed in natural environments (Joachim et al., 2009; Venkatarama Sharma et al., 2021). This facilitates the interpretation of results following the introduction of pollutants, represented here by pearl farming plastics.
In this study, we first sought to evaluate the influence of plastic pollution on the bacterial community associated with the plastic substrate, the water, and the microbiome of the giant clam. Our second objective was to identify bacteria whose abundance varies significantly with plastic concentration, either by being favored or inhibited by plastic, and to investigate whether some of these bacteria are shared between plastic substrate, water, and the microbiome of the giant clam. Overall, our study aims to fill the gap in understanding the impact of plastic pollution on bacterial community assemblages in both inert and living environments, from bacteria to entire communities.
2 Materials and methods
2.1 Setting up the mesocosms
Nine mesocosms with a volume of 275 L for the main tank and 70 L for the settling tank were designed following the Jaubert method (Jaubert, 2013). The Jaubert method uses a large quantity of substrate to promote biological water filtration through the activity of an active bacterial community. Here, the substrate consisted of sand and coral stones taken from Vairao lagoon (Tahiti, French Polynesia). After sterilizing the sand and rocks through successive treatments with fresh water and UV radiation, we added 65 L of sand and 17 kg of rocks (10 kg in the main tank and 7 kg in the settling tank) to each mesocosm. Following the addition of sand and rocks, we calculated the available water volume in each tank to be 270 L. The parameters of the tanks were maintained at the following values, the temperature was 27 ± 0.3°C, the pH was 8 ± 0.1, and the salinity was 36.1 ± 0.2 psu. The lighting was turned on for 8 hours per day. After one year of cycling, allowing for the establishment of denitrifying bacteria in the substrates, we introduced marine organisms. In each mesocosm six giant clams Tridacna maxima (shell length: 4 ± 1 cm) were added. To rid them of potential parasites, the clams underwent a one-minute freshwater bath before being placed in the mesocosms. After observing several cases of fireworm predation during the acclimation phases which appear to originate from an initial batch of giant clams that had not undergone parasite removal, we decided to suspend all the animals to prevent direct contact with the substrate (Figure 1). The concentrations of plastics per mesocosm are shown in Table 1.
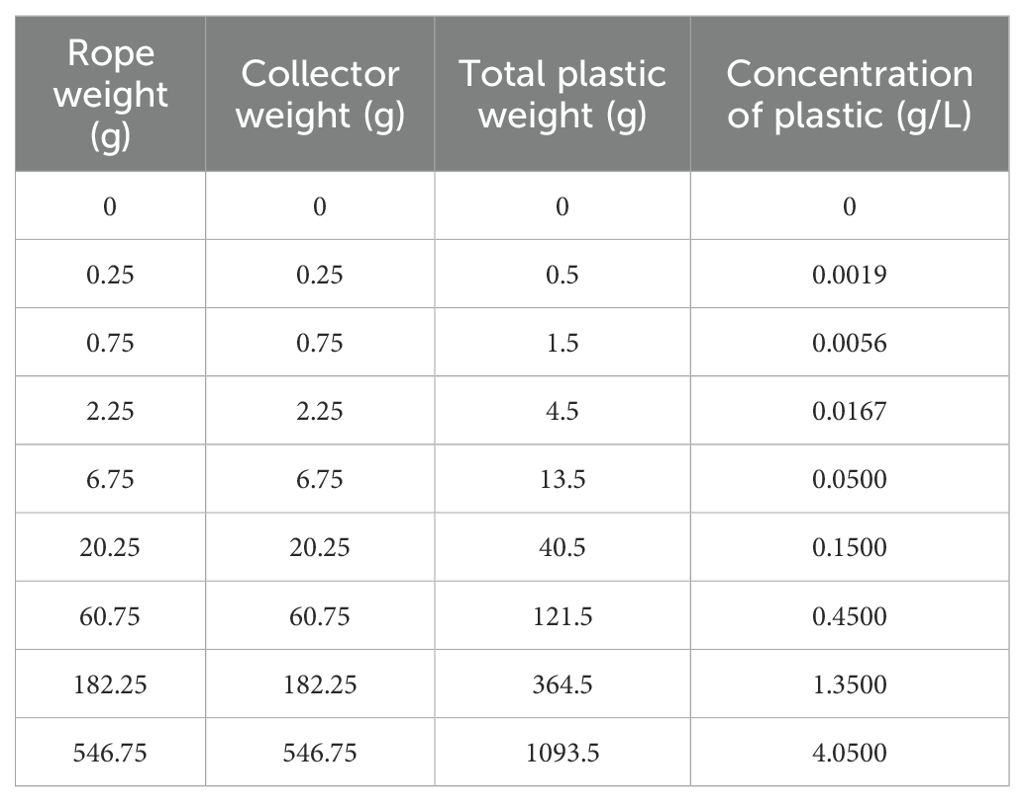
Table 1. Weight of the rope and collector and corresponding plastic concentration based on a mobilised water volume of 270 L per mesocosm.
2.2 Species of interest
Bivalves have already proven to be reliable bio-indicators for assessing the pollution status of environments (Ding et al., 2021; Prokić et al., 2021; Multisanti et al., 2022). Sentinel species must meet the following criteria: wide distribution range, a well-known biology, sedentary nature, ability to provide early alerts, key function in the ecosystem, homogeneous response to pollutants, and the existence of identifiable toxic effects associated with the degree of pollution (Li et al., 2019). By meeting several of these criteria, Tridacna maxima, a tropical giant clam, may be an ideal sentinel species. This sessile filter-feeder species is widely distributed in French Polynesia (Andréfouët et al., 2005; Gilbert et al., 2005). Tridacna maxima is already employed as a sentinel for Metallic Trace Elements (MTE) in Polynesia (Godéré et al., 2023). Furthermore, research teams have demonstrated that in polluted areas, the quantity of zooxanthellae in the mantle and the frequency of giant clam valve movements, as monitored by valvometry, serve as reliable indicators of environmental pollution by contaminants such as lead (Yaqin et al., 2020; Killam et al., 2023). It has also been demonstrated that this species is capable of bioaccumulating microplastics (Arossa et al., 2019). Negative effects, such as oxidative stress and physiological alteration resulting from plastics, have been observed in other bivalve species exposed to contaminated environments (Sureda et al., 2018). Moreover, the giant clam requires a limited amount of nutrient, which aligns with the Jaubert method used here (i.e., low water renewal).
2.3 Plastics used and concentration calculations for mesocosms
The lowest concentrations in this range (i.e., 0.0019 g/L) were estimated based on the quantities of waste produced by pearl farming in certain Polynesian atolls (DRM, 2015). However, the distribution of plastics in lagoons can be highly heterogeneous due to complex lagoon hydrodynamics (Bruyère et al., 2024). As a result, local plastic concentrations may be significantly higher than global estimates at the lagoon scale, with certain plastic accumulation zones acting as hotspots for chemical release (Gardon et al., 2021). To encompass a broad spectrum of potential plastic concentrations in pearl farming lagoons, we selected a range from 0.0019 to 4.0500 g/L, using a base-3 logarithmic interval between each concentration (Table 1). This range includes plastic concentrations typically tested in toxicological studies on plastic leaching (Ke et al., 2019; Gardon et al., 2020a; Klein et al., 2021; Wang et al., 2023).
The plastics used in this experiment were supplied by a pearl farm on the Mangareva atoll in the Gambier Archipelago and consisted of standard new ropes and collectors commonly used in pearl farms across French Polynesia. The collectors are spongy plastic structures used for recruiting oyster spat. Previous RAMAN analyses confirmed that these materials are composed of polyethylene (PE) and polypropylene (PP) (Gardon et al., 2020a).
2.4 Chemical analysis
After two months of experimentation, we collected 500 mL of seawater from each mesocosm. The samples were subjected to chemical characterization in duplicate at CEDRE (Center for Documentation, Research, and Experimentation on Accidental Water Pollution, Brest, France) employing gas chromatography (HP 7890N, multifunction injection Combipal MPS2, Gerstel) coupled with tandem mass spectrometry (GC/MSMS). The interface temperature was maintained at 300°C, with a pre-programmed injection ranging from 50°C (0.5 min) to 280°C (6 min) at a rate of 15°C/min. This setup was integrated with a temperature-programmed injector (Cooling Injection Device, Gerstel: -10°C (0.05 min) to 300°C (10 min) at 12°C/s). The oven temperature program started at 70°C (0.5 min) to 150°C at 20°C/min, followed by an increase to 320°C (5 min) at 7°C/min. Helium served as the carrier gas, and the capillary column employed was an RXi 5-ms (Restek, Bellefonte, USA). The chromatograph was linked to a tandem mass spectrometry detector (Agilent 7000 Triple Quad).
Quantitative analysis of Polycyclic Aromatic Hydrocarbons (PAHs), Polychlorinated Biphenyls (PCBs), Polybrominated Diphenyl Ethers (PBDEs), pesticides, and additives was carried out using internal standard calibration in Multiple Reaction Monitoring (MRM) mode, incorporating two transitions for each compound (Quantifier/Qualifier). The acquisition frequency for each fragment was set at 2 cycles per second. MassHunter Workstation V10.0 software (Agilent Technologies) used to reprocess of chromatograms in the analysis of organic compounds.
2.5 Experimental design
Prior to introducing the plastic into the mesocosms, two liters of water from each mesocosm were collected, filtered through 0.22 µm pore size polycarbonate membrane filters, and stored in lysis buffer (2 % CTAB, 1.4 M NaCI, 100 mm Tris-HCl pH 8, 20 mM EDTA pH 8 and 200 μg/mL) at -80°C after grinding and digestion with proteinase K. One giant clam from each mesocosm was also sacrificed at T0, a portion of their muscle was extracted and frozen at -20°C while their gills were preserved in lysis buffer CTAB 2 % at -80°C. Growth rate, a biological parameter of bivalves known to be impacted by the presence of plastic in the environment (Détrée and Gallardo-Escárate, 2018), was measured by recording the shell length of all the giant clams at different time points during the experiment using a caliper. The growth rate was calculated as the difference between initial and final shell lengths divided by the number of days in the experiment. The plastics were then randomly immersed in the mesocosms, making the initial time of the experiment (T0). Every fifteen days (i.e. T1, T2, T3), and continuing until the end of the experiment, two liters of water from each mesocosm were collected and processed as described above. After 60 days of plastic immersion, the experiment was stopped (i.e. T4). All remaining giant clams were sacrificed, and their gills and muscles were collected and preserved as previously. Samples were also collected from the macroplastics (ropes and collectors) and the water in each mesocosm. All samples were stored at -80°C in 2 % CTAB lysis buffer awaiting DNA extraction.
2.6 Glycogen quantification
The presence of plastic in the environment can result in adverse health impacts on the energy balance of marine organisms such as pearl oyster Pinctada margaritifera (Gardon et al., 2018). One of the proxies chosen to assess the health of the giant clams was the quantification of their muscle glycogen reserves. After thawing the giant clam muscles, 100 mg were sampled, transferred to Eppendorf tubes to which 500 µL of Trichloroacetic Acid (TCA) and 3 stainless steel beads were added. The samples were ground for 5 minutes with a grinder set to 30 beats per second. Once the sample was ground, 2.5 mL of 15 % TCA was added. The sample was then incubated at 4°C for one hour. The tube was then centrifuged for 10 minutes at 3 000 g and at a temperature of 4°C. After centrifugation, 1.5 mL of the supernatant was recovered from the tube and supplemented with 12 mL of 96 % ethanol. The samples were then stored at 4°C for 12 hours with agitation (shaking platform). The next day, the tubes were centrifuged at 4°C for 30 minutes at 4 000 g. The supernatant was removed, and 200 µL of pure water was added to the pellet before a brief vortex to re-solubilize the pellet (containing glycogen). Glycogen quantification was performed through a colorimetric reaction with anthrone-sulfuric acid. The absorbance of the resulting colored complex was measured at a wavelength of 620 nm (FLUOstar, BMG Labtech).
2.7 Statistical analyses
The normality of the data was tested using a Shapiro-Wilk test, and homoscedasticity was assessed using a Levene test on the growth rate and glycogen concentration in the muscle tissues of giant clam individuals. As normality conditions were not met in our datasets, we conducted a Kruskal-Wallis test to determine if there were significant differences in the growth rate and glycogen concentration among the giant clams subjected to various plastic concentrations. The significance level chosen for these analyses was a P-value < 0.05. All these analyses were performed using R v4.0.5.
2.8 DNA extraction, library preparation and 16S rRNA gene sequencing
Bacterial assemblages were assessed for three compartments: the surface of plastics, the water column and the giant clams (Tridacna maxima). Samples for DNA extraction consisted of polycarbonate PCTE membrane filters of 2 L of water, 50 to 300 mg of gills tissue, and plastic smears respectively.
DNA was extracted using CTAB (cetyl trimethylammonium bromide)-based extraction protocol adapted from (Winnepennickx et al., 1993). Briefly, 600 μL of CTAB 2 % buffer was added to each sample tubes (filters, cotton swabs and clam gill tissue) as well as three sterile stainless-steel balls of 3 mm-diameter. Tubes were then immediately placed in a vibrating ball mill (Mixer Mill MM400, Retsch, Haan, Germany) for grinding at 30 Hz for 3 minutes and further incubated overnight at 60°C. After thawing the samples at room temperature, an equal volume of phenol-chloroform-isosoamyl alcohol (25:24:1) was then added to each sample. After gentle inversion of tubes manually samples tubes were centrifuged for 10 minutes at 12 000 g and 400 µl of the aqueous phase transferred to a new tube. DNA was precipitated by adding an equal volume of isopropanol and incubated at room temperature 10 minutes after gentle inversion of tubes. After centrifugation for 20 min at 12 000 g, the supernatant was discarded and the pellet washed with 70 % ethanol. The pellet was air-dried for 10 minutes and resuspended in 50 µl of DNase-free water.
After DNA extraction, the V4 region of the bacterial 16S SSU rRNA gene was amplified using the primers 515F (5’-GTGYCAGCMGCCGCGGTAA-3’) (Parada et al., 2016) and 806R (5’-GGACTACNVGGGTWTCTAAT-3’) (Apprill et al., 2015). PCR products were submitted for 2x250 paired-end sequencing on an Illumina MiSeq platform at the Genome Quebec platform, Montréal (Canada). Samples with fewer than 10 000 reads were not considered for subsequent analyses. Sequences have been deposited in the ENA database (BioProject: PRJEB74057).
2.9 Data processing
The fastq files generated by Illumina MiSeq were processed using QIIME2 v2022.11 (Bolyen et al., 2019) and DADA2 (Callahan et al., 2016). Primers were removed from the demultiplexed sequences using Cutadapt (Martin, 2011) (via q2-cutadapt). DADA2 parameters were selected using the FIGARO tool [44]: trim Position [221,229] and max Expected Error [1,1]. The following steps of the DADA2 pipeline were performed using the default parameters (via q2-dada2). Amplicon sequence variants (ASVs) clustering was performed using dbOTU3 (Olesen et al., 2017) with the default settings (via q2-dbOTU3). Taxonomy was assigned to ASVs using the q2‐feature‐classifier (Bokulich et al., 2018) classify‐sklearn naive Bayes taxonomy classifier against the SILVA138 (Quast et al., 2013) pre-trained classifier for the V4 region of the 16S gene.
2.10 Principal coordinates analysis
Eukaryotic, mitochondrial, chloroplast and unidentified sequences were removed from the dataset. ASVs with a relative abundance greater than 0.5 % and present in fewer than three samples were filtered out using the phyloseq v1.42.0 R package and a custom function. First, we estimated the Bray-Curtis distance matrix from the relative abundance dataset (samples in rows, ASVs in columns) based on the recommendation of Laporte et al. (2021) for eDNA metabarcoding. Then, we performed a Principal Coordinate Analysis (PCoA) using the pcoa function available in ape v.5.7-1 R package with Lingoes correction of negative eigenvalues, as recommended by Legendre and Anderson (1999). The percentage of variation and significance of each PCoA axis were assessed using the pcoa.sig function available in the R package PCPS v1.0.7.
2.11 Distanced-based redundancy analysis
We conducted a distance-based Redundancy Analysis (db-RDA), a statistical tool developed by Legendre and Anderson (1999), to assess the influence of each experimental factor - i.e. time of the experiment, type of the sample, plastic concentration - on community assemblage variation. The following ‘time of the experiment’ and ‘type of the sample’ were transformed into dummy variables using the R package fastDummies v.1.7.3. Collinearity among explanatory variables was assessed before running the models. Both an empty model and a complete model were built to select the significant variables to retain, using the ordistep function (with the direction set to “both”), available in the R package vegan v2.6-4. For the final db-RDA model, significance of each variable and axis was assessed using the anova function with 10 000 permutations. The global adjusted R2 value and significance were reported for the final model. All statistical analyses were conducted in R 4.2.3 (R Core Team, 2022).
2.12 Linear regression analysis to detect ASV with relative abundance influenced by plastic concentration
By considering each type of sample separately (i.e. plastics, water and the giant clams), we conducted a linear regression analysis to test the relationship between the relative abundance of each ASV and the gradient of plastic concentration. From this analysis, we selected only the ASVs showing a significant correlation with the cor.test function (P-value < 0.01) and a high R2 (R2 > 0.4). These ASVs will be referred to as candidate bacteria for the plastic response. We extracted the genus of each candidate to evaluate the representativity of the genus and to determine if some candidate’s genus were shared among different type of samples. The results were represented using the ggplot2 v.3.4.2 R package. To evaluate the number of ASVs shared among all samples and those unique to each sample, we used the UpSet R v.1.4.0 R package (Conway et al., 2017).
2.13 Dendrogram
The pairwise distances of the DNA sequences associated with the selected candidate bacteria were calculated using the “K80” model with the dist.dna function available in the ape v.5.7-1 R package. The dendrogram was then constructed using the hclust and as.dendrogram functions from the stats v4.2.2 R package. We used FigTree v1.4.4, a graphical viewer, to visualize the tree (http://tree.bio.ed.ac.uk/software/figtree/).
3 Results
3.1 Global bacterial community diversity
The overall dataset comprises 104 samples (16, 42 and 46 samples of plastic, water and T. maxima, respectively) with sufficient sequencing depth (> 10 000 reads) (Supplementary Figure S1) including a total of 10 710 bacterial ASVs. To keep only representative ASVs for describing bacterial community, we selected 1 716 ASV present in relatively high abundance (i.e. relative abundance > 0.5) and in at least three out of 104 samples. A Principal Coordinates Analysis (PCoA) was then performed to reflect the overall variation of the bacterial community (Figure 2). On the first significant Principal Coordinate axis (PCo1; 19.62 %), water samples were delineated from Tridacna maxima samples, while on PCo2 (12.38 %) plastic samples were distinct from water samples. Notably, we also observed that T. maxima samples formed two separate groups along PCo1 and PCo2 (Figure 2). The first group of T. maxima individuals, located on the right of PCo1 (PCo1 > 0.4 and PCo2 < 0.00), corresponds to samples with low bacterial diversity and two dominant ASVs (ASV1 and ASV2: both from the Endozoicomonadaceae family) (Figure 2, Supplementary Figure S2). The second group (PCoA1 < 0.4 and PCo2 > 0.00), located on the left of the PCo1 axis, comprises T. maxima samples with more diverse and evenly distributed bacterial communities (Figure 2, Supplementary Figure S2). These two groups include giant clams from different plastic concentrations and time points (i.e. T0, T4), indicating that neither plastic concentration nor time explains observed the pattern. Kruskal-Wallis statistical tests on growth rate and muscle glycogen concentration (i.e. proxies for the physiological status of our animals) between these groups were not significant (P > 0.01, Supplementary Figures S3, S4). Similarly, the correlation between bacterial diversity and growth rate was non-significant (R2 = -0.22, P-value = 0.25) as was the correlation with muscle glycogen concentration (R2 = 0.12, P-value = 0.56).
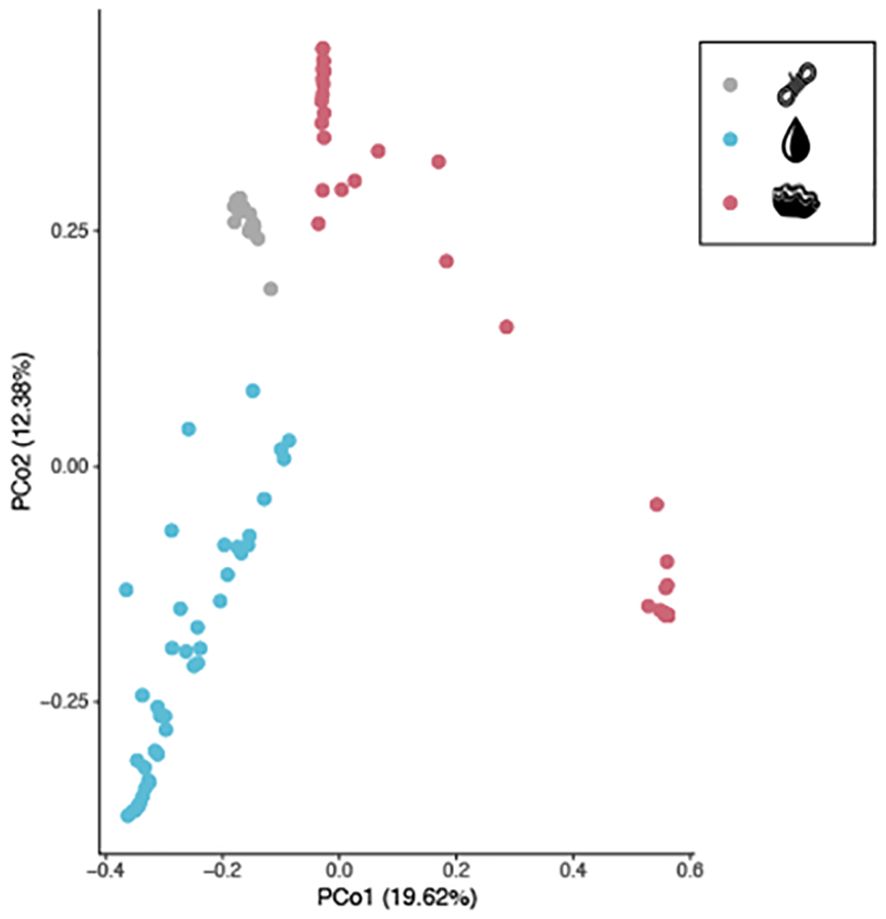
Figure 2. Variation in Bacterial Communities across plastic, water, and Tridacna maxima samples. Principal Coordinates Analysis (PCoA) on ASVs, each point represents a sample coloured according to the compartment where it was collected: in grey for plastic, in blue for water, in pink for Tridacna maxima.
3.2 Testing the influence of plastic concentration, on the variation of community assemblage
We tested the influence of plastic concentration on a broad scale using a distanced-based Redundancy Analysis (db-RDA) on the six significant PCoA axes (Supplementary Figure S5). The analysis revealed that plastic concentration did not significantly influence the variation in community assemblage (P-value = 0.418). In contrast, and consistent with the PCoA results (i.e. plastics, water column and the giant clams; Figure 2), the majority of the variation was significantly influenced by the type of sample (adjusted R2 = 51.8 %; P-value < 0.001). We also checked whether the type of plastic used in pearl farms (i.e. ropes and collectors) influenced community variation and found no significant difference in community assemblage between the two types of plastic (P-value = 0.3). To investigate the influence of the temporal scale on the bacterial community, we retained only the water samples, as they were collected at five different time points (i.e. T0, T1, T2, T3, T4). Sampling time significantly influenced bacterial community assemblages (P-value < 0.001), accounting for up to 19.4 % of the global variation. Each sampling time was significant, suggesting regular changes in the bacterial communities over time.
3.3 Continuity in a bacterial community structure across different type of eDNA samples
We identified 1 180, 1 251 and 820 ASVs specifically associated with plastics, water, and giant clams, respectively (Figure 3). We emphasized the specificity of the bacterial community associated with each compartment, as at least 30.2 % of the ASVs were unique to one of the compartments. The strongest continuity in community structure was found between water and plastic samples, with 514 shared ASVs, while the lowest continuity was found between Tridacna maxima and water samples, with only 80 shared ASV (Figure 3).
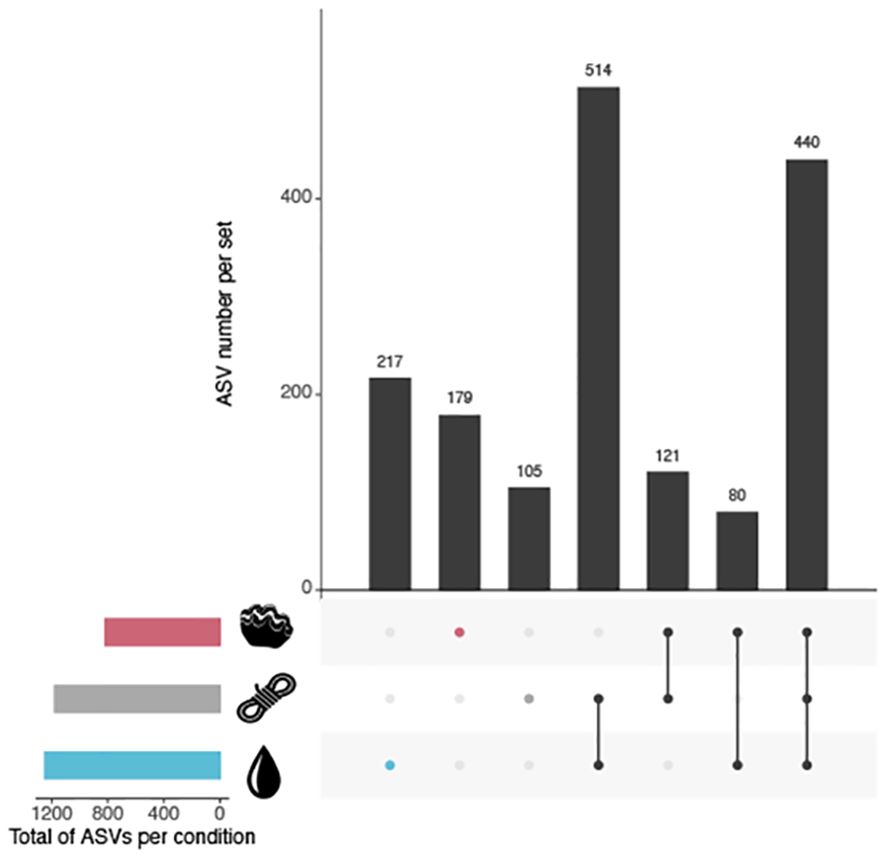
Figure 3. UpSet diagram showing the size of the intersection (i.e. number of ASV shared) across the types of samples (plastic, water, and Tridacna maxima). The size of each set is shown by the horizontal bars on the left.
3.4 Chemical analysis
Except for the mesocosm with the highest plastic concentration (4.05 g/L), chemical analyses revealed that the concentrations of the tested chemical components were below the limits of detection (LD) or quantification (LQ) (Table 2). In the mesocosm with 4.05 g of plastic, gas chromatography coupled with tandem mass spectrometry (GC/MSMS) indicated the presence of several pesticides such as Beta-BHC, Delta-BHC, acetochlor, metolachlor, and chlorpyrifos. The sum of pesticides reached 20.69 ng/L and 1.13 ng/L in the first and second replicates, respectively. Nonylphenols, belonging to the alkylphenols class, were also detected in one of the two replicates at a level of 13.55 ng/L of water in the same mesocosm (Table 2).
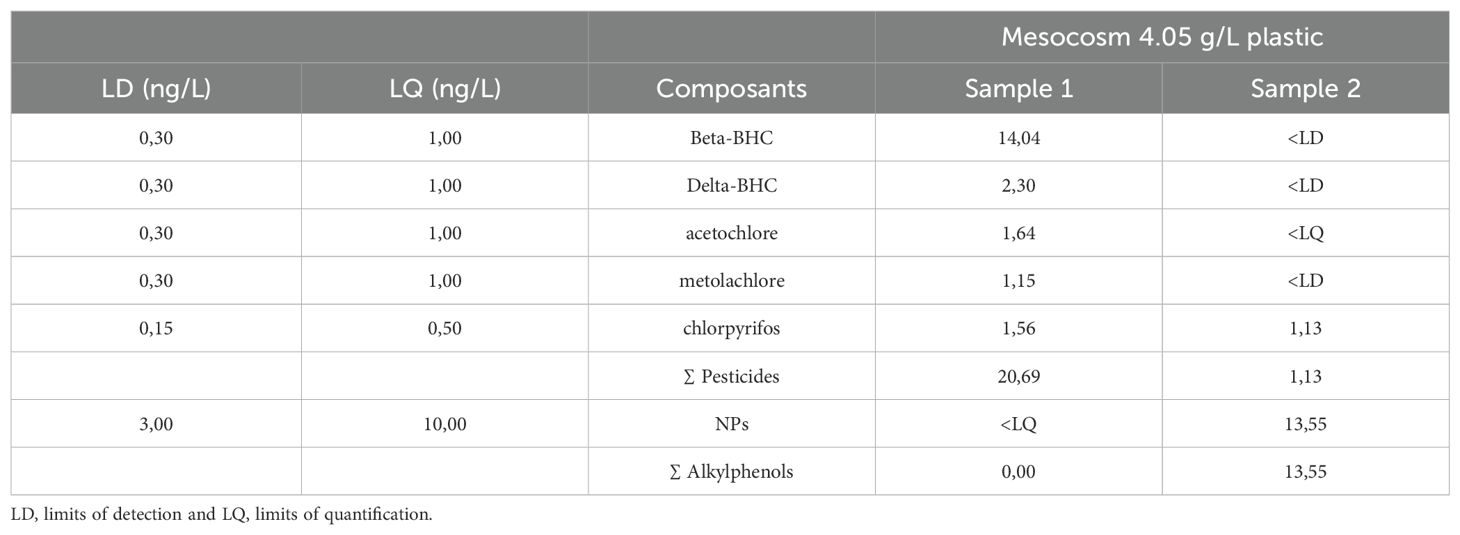
Table 2. Chemical compositions of the water in the most concentrated mesocosm, obtained through gas chromatography coupled with tandem mass spectrometry (GC/MSMS) conducted by CEDRE in Brest (Center for Documentation, Research, and Experimentation on Accidental Water Pollution).
3.5 Identifying candidate bacteria associated with plastic
For each type of sample (i.e. plastic, water, and T. maxima), the linear regression between the relative abundance of an ASV and plastic concentration detected a total of 33 candidates ASVs (R2 > 0.4 or R2 < -0.4 and P-value < 0.01). Candidate ASVs were also required to be present in at least three samples from the same environment. All the selected candidates had a positive R2, revealing a consistently positive relationship between relative abundance and plastic concentration (Supplementary Figure S6). Comparing the bacterial abundances in mesocosms with plastic concentrations ranging from 0 to 1.35 g/L, abundance tended to be similar; however, at 4.05 g/L, the abundance was higher, suggesting that the linear regression is largely driven by this unique extreme point in the distribution, regardless of the sample type (Supplementary Figure S6).
3.6 Detecting a threshold for the impact of plastic
The mean abundances of all candidate bacteria obtained for plastic support, water and T. maxima samples at low plastic concentrations (ranges from 0 to 1.35 g/L) were 0.0007, 0.0018, 0.0001 while at high concentrations (4.05 g/L) these abundances were 0.0063, 0.0069, 0.0019 respectively. This represents 9, 3.8 and 19-fold shift in the abundance of candidate bacteria on plastic, water and T. maxima, respectively, with T. maxima showing the highest shift (Figure 4). These differences in bacterial candidate abundance between concentrations of 0 to 1.35 g/L and the highest concentration at 4.05 g/L of plastic were significant across all three sample types (Wilcoxon test; P-value < 0.01).
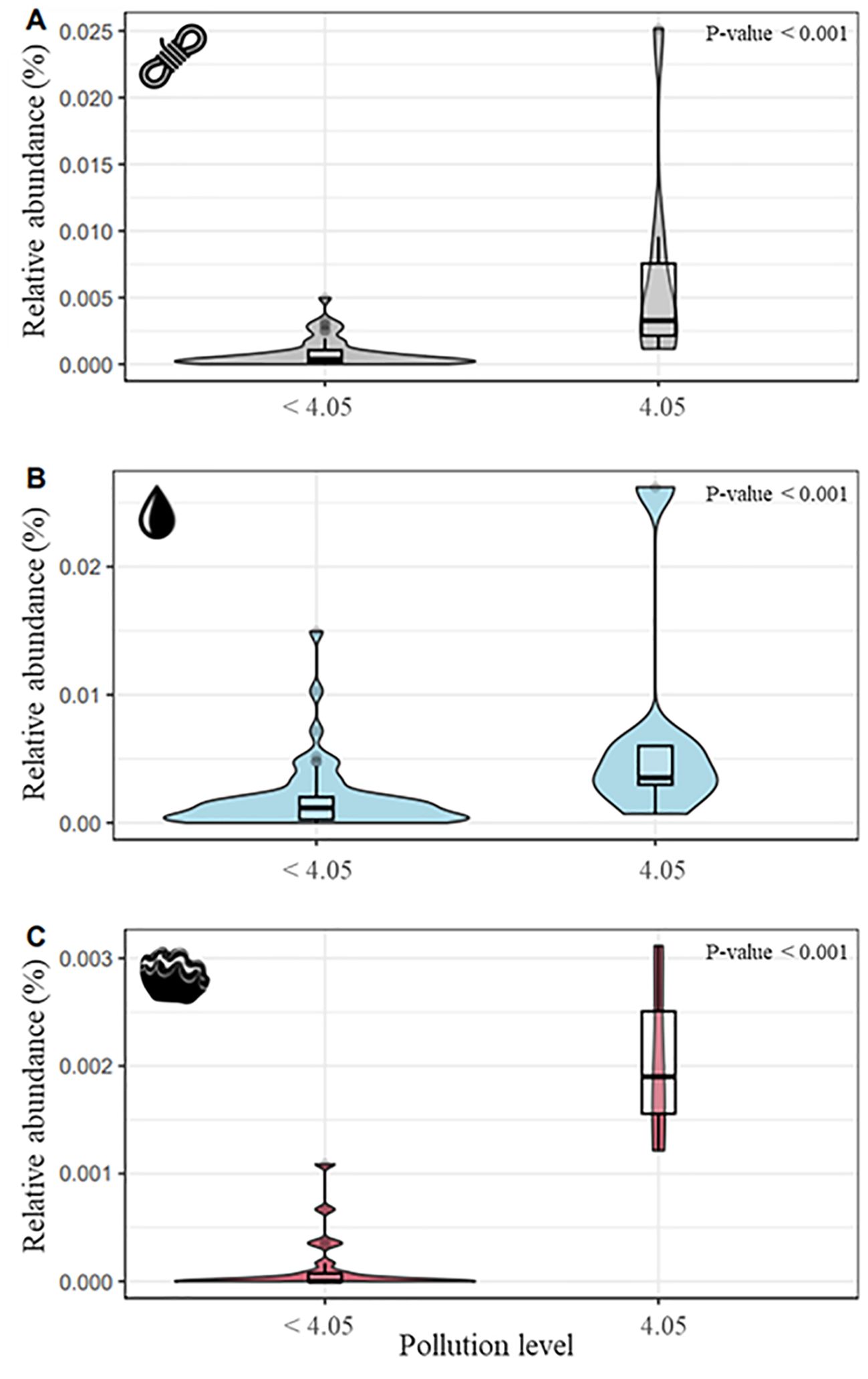
Figure 4. Average of relative percentages of abundance of candidates ASVs for concentrations below 4.05 g/L and for the maximum plastic concentration of 4.05 g/L for each type of sample. [(A) plastic, (B) water, (C) Tridacna maxima].
3.7 Shared candidate among samples
Among these 33 candidates ASVs, some are specific to a particular type of sample. Specifically, four candidates ASVs are exclusively present in clams, nine are specific to the seawater compartment, and 18 are specific to the plastic. Notably, two candidates ASVs shared between water and plastic (Figure 5) corresponding to Sulfurospirillum (Class: Campylobacteria) and Filomicrobium (Class: Alphaproteobacteria) (Table 3).
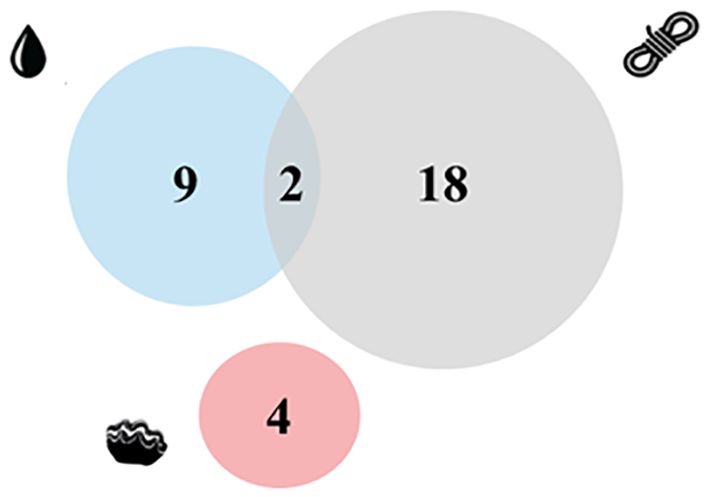
Figure 5. Venn diagram of candidate bacteria shared between plastic, water, and Tridacna maxima (grey: plastic, blue: water, pink: Tridacna maxima).
3.8 Candidate bacteria genetic similarity
Highlighting the genetic similarity across ASVs candidates reveals that the 33 identified bacterial genera are distributed into five classes, namely Campylobacteria, Bacteroidia, Planctomycetes, Alphaproteobacteria, and Gammaproteobacteria (Table 3, Figure 6). The Campylobacteria class is represented by a single genus, Sulfurospirillum, while other classes, such as Alphaproteobacteria, are overrepresented, encompassing at least four candidate families of bacteria associated with plastic concentration.
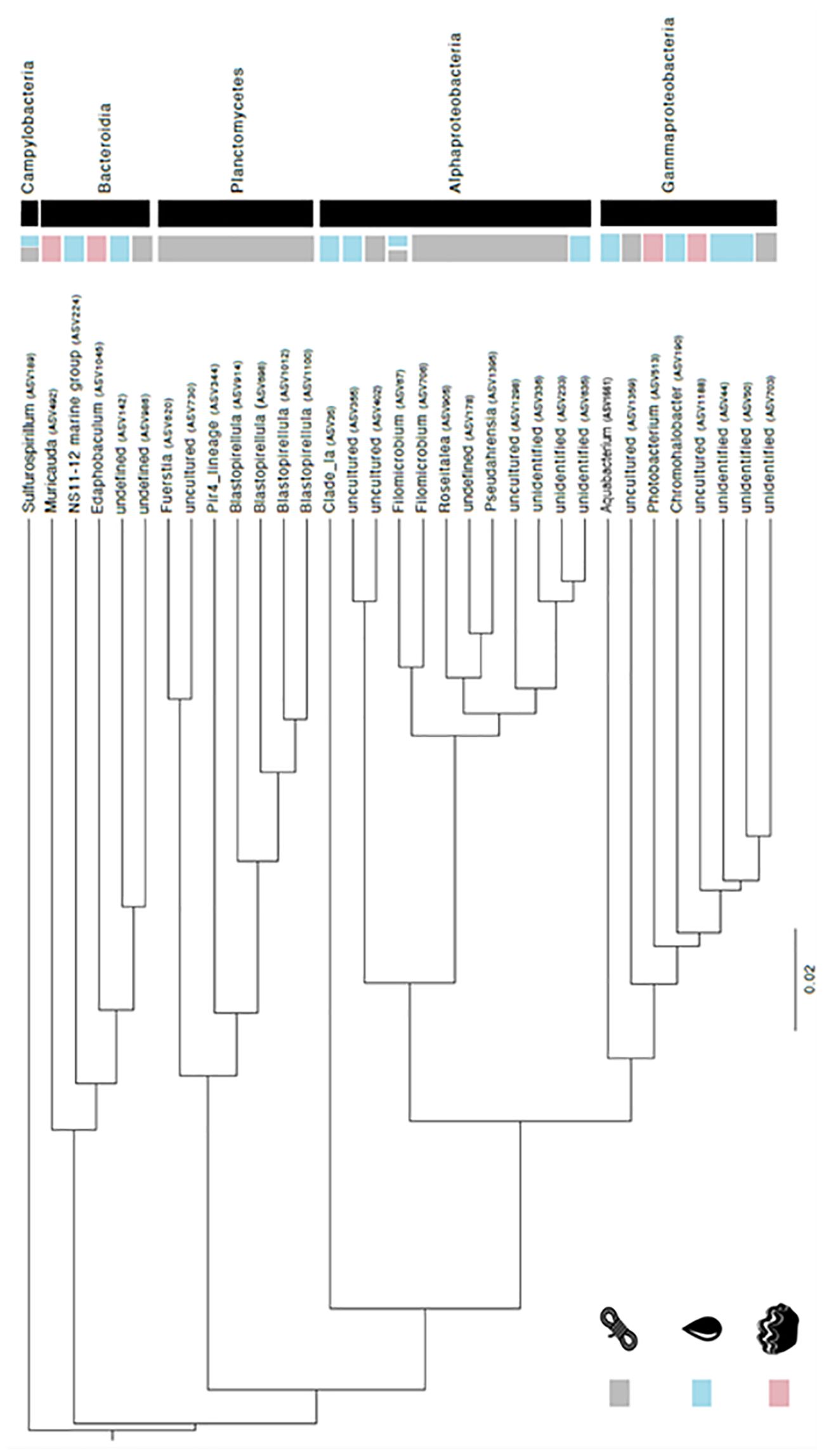
Figure 6. Left panel – Phylogenetic tree based on the V4 region of the bacterial 16S SSU rRNA gene for each ASV candidate; the ASVs are coloured accordingly to the type of sample where they were detected as candidates (grey: plastic, blue: water, pink: Tridacna maxima).
However, the identification of candidate ASVs in one type of sample does not imply their absence in other environments. Table 3 shows that most of the candidate ASVs identified in one environment are also present in other environments without a significant impact on their abundance. In total, 11 ASVs (ASV635, ASV355, ASV905, ASV189, ASV1359, ASV513, ASV44, ASV703, ASV914, ASV730, ASV620) are shared among the three compartments (i.e., plastic support, water, and T. maxima). Additionally, 15 candidate ASVs from plastic substrate or water are shared between these two same environments, further emphasizing the bacterial continuity between them. Only five candidate ASVs are present exclusively in a single environment, namely ASV1045 (T. maxima), ASV492 (T. maxima), ASV1661 (water), ASV190 (water) and ASV1012 (plastic).
4 Discussion
A future projection estimates that by 2060, between 155 and 265 million metric tons of plastic waste will be generated globally each year (Lebreton and Andrady, 2019). Ineffective management of this waste will increase the already massive amounts of plastics in the marine environment, highlighting the urgent need to anticipate the potential consequences of plastic on marine ecosystems. Our study aligns with these future concerns and seeks to delineate the impact of chemical plastic pollution on the marine bacterial compartment. By designing a mesocosm that reflects the French Polynesia ecosystem, impacted by pearl farming - the major source of marine plastic pollution in this region (Andréfouët et al., 2014; Crusot et al., 2023) - we identified the bacteria affected by plastic pollution, considering the various levels of organization within the marine ecosystem, from animals to the water column and the plastic substrate. From a practical standpoint, we provide a list of bacteria potentially impacted by plastic pollution, which should be monitored to assess the possible repercussions of bacterial imbalances resulting from high concentrations of plastics in the environment.
Plastics play a crucial role in the dissemination of pathogens by providing durable surfaces that facilitate the formation and long-distance transport of biofilms, which can harbor pathogenic microorganisms (Goldstein et al., 2014; Dussud et al., 2018; Lemonnier et al., 2022),. This was demonstrated, for instance, by Kirstein, who showed that microplastics sampled from the North and Baltic Seas were covered with biofilms containing potentially pathogenic species (Kirstein et al., 2016). Here, we identified five candidate bacteria, whose abundance was significantly affected by plastic pollution on plastic substrate and in the water, from the Rhodobacteraceae family. This family includes species known to be associated with diseases in sponges (Sweet et al., 2015, algae (Fernandes et al., 2012), oysters (Boettcher et al., 2005) and corals (Cooney et al., 2002; Pantos et al., 2003). We also identified two candidate bacteria from the Flavobacteriaceae family in water samples and in giant clams, which includes pathogens responsible for the "Suminori" disease in the red alga Porphyra sp. (Kusuda et al., 1992) and tenacibaculosis, a disease affecting a wide range of commercially valuable fish species (Nowlan et al., 2020; Lopez et al., 2022). We then recommend the monitoring of these bacterial, whose presence is facilitated by plastic pollution, as they can significantly disrupt marine food chain, impact the economies of industries relying on marine resources, and pose risks to human health such disease transmission and metabolic disorders (Rivas et al., 2013; Junaid et al., 2022).
Investigating changes in bacterial composition in a microbiome under environmental stressors can offer valuable insights into ecosystem health, given bacteria’s short generation time and their critical role, which perform essential functions within marine organisms (Sehnal et al., 2021). Here, we found one candidate bacteria belonging to Vibrionaceae in the giant clam microbiome, specifically in the Photobacterium genus, which includes highly pathogenic bacterial isolates affecting various marine organisms and humans (Rivas et al., 2013). For instance, an in situ incubation experiment showed that the Photobacterium pathogens were favored by microplastics substrates, underlying ecological risks of microplastics in mariculture industry (Hou et al., 2021). These mesocosm observations demonstrate for the first time the increased health risks that plastic-polluted pearl farming lagoons may face. In severe cases, an overabundance of pathogenic bacteria could lead to marine epizootics. Heavily polluted lagoons may cause bacterial imbalances, which, in the most pessimistic scenarios, could trigger cascading effects impacting not only pearl farming and aquaculture sectors in French Polynesia, but also the entire surrounding ecosystem.
Giant clams, as living organisms, can actively select the bacteria that colonize them – through mechanisms such as their immune system - unlike plastic and water, which are inert environments where bacterial colonization is largely passive (Wright et al., 2020). Indeed, microbiota have evolved over time in interaction with their host animals, forming mutualistic relationships (Apprill, 2017). Furthermore, the low bacterial diversity (with an overrepresentation of Endozoicomonadaceae) observed in the giant clam microbiome demonstrates the animal's ability to selectively permit or reject bacteria. The high abundance of Endozoicomonadaceae found in some T. maxima individuals aligns with the study of Rossbach et al. (2019) which reported the apparent dominance of this family in the gills of T. maxima. These selection mechanisms are primarily driven by innate immunity (Allam and Espinosa, 2015), but the delicate balance between the giant clam and its microbiota can be influenced by external abiotic stressors, such as rising temperature and pollution (Guibert et al., 2020; Yaqin et al., 2020). In this study, we did not observe plastic-induced dysbiosis in the gill microbiota of the giant clam microbiome, in contrast to findings from other organisms. For example, Jin et al. (2019) demonstrated intestinal dysbiosis and gut barrier dysfunction in male mice exposed to polystyrene microplastics and nanoplastics for six weeks, with a significant decrease in Actinobacteria. Lu et al. (2018) reported shifts in gut microbiota proportions that may contribute to disease development in male mice exposed to micro and nanoplastics. In fish, Qiao et al. (2019) found that Danio rerio exposed to polystyrene microplastics for 24 hours experienced a significant reduction in Proteobacteria and an increase in Fusobacteria, while Kurchaba et al. (2020) observed increased abundance of Bacteroidetes in zebrafish larvae exposed to polyethylene microplastics for 10 days. These results suggest that the absence of dysbiosis observed in the giant clam may be species-specific, or could have been influenced by the design limitations of our experiment. Specifically, the choice to monitor the microbiome of the gills rather than the digestive gland may have limited our ability to detect dysbiosis, as this organ could be more responsive to plastic-induced changes, as previously observed in other terrestrial and marine species. Moreover, the duration of exposure in our experiment may have been insufficient to reveal potential microbiome disturbances, which might only manifest over a longer time frame. A prolonged study and focus on other organs, such as the digestive gland, would be valuable in future investigations to better understand the impacts of microplastic exposure on the giant clam microbiome. Further studies on other filter-feeding organisms are needed to determine whether this resistance to dysbiosis is widespread or unique, while increasing the sample size of giant clams would help confirm these observations and provide a better understanding of the mechanisms underlying the stability of their microbiome in the face of plastic pollution.
Plastics introduce an additional source of carbon into the environment as they degrade or leach additives, increasing carbon availability in areas where plastics accumulate (Sheridan et al., 2022). Additives released, such as pesticides, phthalates, alkylphenols and polycyclic aromatic hydrocarbons (PAHs), released in large quantities when new pearl plastics are immersed in water may then play a significant role in bacterial selection (Gardon et al., 2020a; Goulais et al., 2024). For instance, only bacteria capable of absorbing these leached additives were able to develop first (Pinto et al., 2019). For example, certain bacterial taxa, such as members of the Alteromonadaceae and Oceanospirillaceae families, have been shown to thrive in the presence of phthalates, while others are inhibited by these compounds (Iwaki et al., 2012). Chemical interactions between bacteria and leached additives may then contribute to bacterial abundance shifts as observed in our study. Furthermore, differences in the types of additives present in different polymers, such as HDPE or PP, have been linked to variations in bacterial colonization patterns (Romera-Castillo et al., 2018). For instance, plastics exposed to solar radiation tend to release more dissolved organic carbon (DOC), which further stimulates bacterial growth (Romera-Castillo et al., 2018). In our study, we documented that all candidate bacteria influenced by plastic pollution were more abundancy in high plastic concentrations compared to low concentrations. This suggests that the increase in available carbon in plastic-polluted areas promotes bacterial biomass and growth (Sheridan et al., 2022). Moreover, after two months of exposure to plastics, the dominant bacterial classes in our samples were Gammaproteobacteria and Alphaproteobacteria. This observation aligns with findings from recent studies showing that these classes tend to dominate in specific polymer environments. For instance, a study highlighted that Gammaproteobacteria and Alphaproteobacteria significantly dominated mesoplastics made of poly(ethylene terephthalate) and polystyrene, indicating a specific affinity for colonizing these polymers (Debroas et al., 2017). The predominance of these bacterial groups in our samples raises questions about their potential resistance or utilization of plastic leaching products. While Gammaproteobacteria and Alphaproteobacteria are commonly found in water columns, their significant association with plastics suggest their ability to thrive in environments altered by plastic pollution. On the other hand, carbon is not the only chemical element affected by the presence of plastics. A microcosm study demonstrated that microplastics, depending on their type, size, and concentration, can influence microbial processes that also control the nitrogen cycle in sediments. Microplastics such as polyurethane foam (PUF) and polylactic acid (PLA) promote nitrification (the conversion of NH4+ (ammonium) to NO3- (nitrate) and NO2- (nitrite)), while polyvinyl chloride (PVC) inhibits it, leading to an accumulation of NH4+ (Seeley et al., 2020). This modulation of the nitrogen cycle by microplastics could have significant implications for marine ecosystems. For instance, heterotrophic bacteria, such as the candidate bacteria belonging to Burkholderiales identified in our mesocosms, may utilize this altered nitrogen source. Indeed, Burkholderiales possess functional denitrifying genes (Usyskin-Tonne et al., 2021), which enable them to benefit from the shifts in the C/N ratio induced by the presence of plastics in the environment.
Exploring the microbial communities’ response to pollution along the marine continuum is crucial for defining which habitats are most affected by pollution, how one habitat may influence another and thus tracking plastic pollution holistically (Rezaei Somee et al., 2021). In our study, we highlighted a strong exchange of bacteria between habitats, as nearly 70% of the microbial community was shared by at least two habitat types. Consequently, bacteria whose abundance is boosted by increasing plastic concentration in one compartment could follow this continuum and become more abundant in another connected habitat, potentially leading to its colonization. As expected, the plastic and water compartments shared the largest bacterial community, as both planktonic and plastic-binding bacteria have the ability to associate and form biofilms (Dang and Lovell, 2016). Floating marine plastics create a new colonizable niche in the water column, which is conducive to the establishment of certain microbial primary producers (Wright et al., 2020). Since water transports both bacteria and the chemical compounds released by plastics, plastic pollution in this habitat can have far-reaching effects on the bacterial community within the mesocosm scale, potentially inducing changes in bacterial abundance. These impacts can extend beyond habitats directly in contact with plastics.
To date, our study is the first to demonstrate that while plastic pollution may not initially appear to impact the bacterial community on a large scale, its effect is subtler and manifest in specific bacterial genera across different compartments - from plastic substrates to the water column and marine animals. Plastic pollution can influence particular bacteria, including photosynthetic (Tetu et al., 2019) and pathogenic species (Vlaanderen et al., 2023), indicating that its impact extends beyond mere colonization surfaces. Chemical analyses conducted on all the mesocosms after two months of plastic immersion identified pesticides and alkylphenols exclusively in the mesocosm with the highest plastic concentration (4.05 g/l, Table 2). Concurrently, we observed shifts in the abundance of certain bacteria, specifically between mesocosms with plastic concentrations below 4.05 g/l and above 4.05 g/l. Remarkably, 20 of the 33 candidates ASVs are associated with the 'plastic' compartment. The increased abundance of these ASVs is likely due to the greater surface area available for bacterial colonization. This suggest that plastic additives may play a role in shaping microbial communities within plastispheres (Pinto et al., 2019). Our study is the first to directly link plastic concentration in the marine environment with changes in the abundance of specific marine bacteria, some of which may serve as bio-indicators. We found that certain bacterial families show a significant increase in abundance in response to high plastic concentrations in specific ecosystem compartments, though these families are not universally impacted across all environments. This underscores the specific impact of plastic pollution on bacterial genera associated with habitats.
Given the plastic pollution crisis, various strategies are being explored to reduce plastic waste. Among these, polymer degradation by bacteria emerges as a promising solution. Certain bacteria can use plastics as a carbon source, transforming these synthetic materials into biodegradable matter (Wright et al., 2020). In our study, we identified Photobacterium and Aquabacterium as candidate bacteria for this purpose. These genera have been previously identified for their plastic-degrading capacities, supporting the development of controlled biodegradation methods. For instance, Photobacterium has been shown to degrade PET bottles effectively; a recent study reported a 35 % degradation of PET bottles after six weeks of exposure to this bacterium (Sarkhel et al., 2020). Similarly, Aquabacterium is known for metabolizing recalcitrant substances, including plastics such as PVC and PHB polymers (Kalmbach et al., 2000; Martínez-Campos et al., 2021).
One of the fundamental principles of genetics is that a monophyletic group of organisms share one or more unique derived characters (Ashlock, 1971). These shared genetic traits often indicate similar functional roles across different species. In our study, the phylogenetic tree revealed that Photobacterium and Aquabacterium belong to the same monophyletic group within the class Gammaproteobacteria and are capable of degrading plastic polymers. A review by Gambarini et al. (2021) highlighted Gammaproteobacteria are notably represented among bacterial classes with plastic-degrading capacities, comprising 17.7 % of described plastic-degrading bacteria and capable of degrading over 43 plastic polymers. This suggests that the ability to degrade plastic polymers might be a trait shared by genera within this monophyletic group. Interestingly, no studies have yet reported plastic degradation by Chromohalobacters. Therefore, it would be valuable for future research to investigate the potential plastic-degrading abilities of Chromohalobacters and other genera within this monophyletic group that have not yet been described (i.e., ASV 1359, ASV 1188, ASV44, ASV50, and ASV703).
5 Conclusion
Our study contributes to the growing body of research on the impact of plastic pollution on marine ecosystems. By analyzing bacterial communities in mesocosms that mimic simplified tropical marine ecosystems, we sought to understand the effects of chemical pollution from leaching pearl plastics on marine bacteria. We did not observe widespread dysbiosis associated with gradual plastic pollution, our findings identified specific bacterial genera that are affected by plastic pollution. The phylogenetic analysis of these bacteria indicates that some of them are known pathogens or have plastic-degrading capacities. Additionally, several bacteria within the same monophyletic group have not yet been extensively studied. It remains possible that these bacteria could also be pathogenic or capable of utilizing plastic polymers as a carbon source. To advance our understanding, future research should focus on:
1. Exploring the potential pathogenicity: Conduct targeted studies to determine if the bacterial genera identified in our study that are affiliated with known pathogens also exhibit pathogenic traits. This could involve assessing their virulence factors and interactions with marine organisms to understand potential risks.
2. Investigating plastic degradation abilities: Examine the plastic-degrading capabilities of the identified bacteria, particularly those within the same monophyletic group as Photobacterium and Aquabacterium. This includes testing their ability to degrade various plastic polymers and assessing their efficiency in transforming plastics into biodegradable compounds.
3. Conducting field validation: Validate the mesocosm findings through field studies in natural marine environments to confirm if the observed patterns and impacts of plastic pollution hold true in more complex and variable real-world conditions.
Data availability statement
The datasets presented in this study can be found in online repositories. The names of the repository/repositories and accession number(s) can be found below: https://www.ebi.ac.uk/ena, PRJEB74057.
Ethics statement
The manuscript presents research on animals that do not require ethical approval for their study.
Author contributions
MG: Writing – review & editing, Writing – original draft, Methodology, Investigation, Formal analysis, Data curation, Conceptualization. SD-T: Writing – review & editing, Formal analysis, Data curation. GM: Writing – review & editing, Validation, Supervision, Methodology, Funding acquisition, Conceptualization. FG: Writing – review & editing, Validation, Supervision, Methodology. DS: Writing – review & editing, Validation, Supervision, Methodology, Investigation, Funding acquisition, Conceptualization. LB: Writing – review & editing, Writing – original draft, Visualization, Validation, Supervision, Methodology, Formal analysis, Data curation.
Funding
The author(s) declare financial support was received for the research, authorship, and/or publication of this article. This work was supported by the Direction des Ressources Marines (DRM) of French Polynesia and Ifremer. MG was granted an Ifremer scholarship.
Acknowledgments
We would like to thank Thibaud MEURILLON, Baptiste FLEUROT, Nono LEWIS TETAURA, Jérémy LE LUYER, Oriane CHEVALIER, Caline BASSET and Corinne FALCHETTO, for their invaluable assistance during the experiments.
Conflict of interest
The authors declare that the research was conducted in the absence of any commercial or financial relationships that could be construed as a potential conflict of interest.
Publisher’s note
All claims expressed in this article are solely those of the authors and do not necessarily represent those of their affiliated organizations, or those of the publisher, the editors and the reviewers. Any product that may be evaluated in this article, or claim that may be made by its manufacturer, is not guaranteed or endorsed by the publisher.
Supplementary material
The Supplementary Material for this article can be found online at: https://www.frontiersin.org/articles/10.3389/fmars.2024.1486802/full#supplementary-material
References
Allam B., Espinosa E. P. (2015). “Mucosal immunity in mollusks,” in Mucosal Health in Aquaculture (Academic Press), pp. 325–370. doi: 10.1016/B978-0-12-417186-2.00012-1
Andréfouët S., Gilbert A., Yan L., Remoissenet G., Payri C., Chancerelle Y. (2005). The remarkable population size of the endangered clam Tridacna maxima assessed in Fangatau Atoll (Eastern Tuamotu, French Polynesia) using in situ and remote sensing data. ICES J. Mar. Sci. 62, 1037–1048. doi: 10.1016/j.icesjms.2005.04.006
Andréfouët S., Thomas Y., Lo C. (2014). Amount and type of derelict gear from the declining black pearl oyster aquaculture in Ahe atoll lagoon, French Polynesia. Mar. pollut. Bull. 83, 224–230. doi: 10.1016/j.marpolbul.2014.03.048
Apprill A. (2017). Marine animal microbiomes: Toward understanding host-microbiome interactions in a changing ocean. Front. Mar. Sci. 4. doi: 10.3389/fmars.2017.00222
Apprill A., Mcnally S., Parsons R., Weber L. (2015). Minor revision to V4 region SSU rRNA 806R gene primer greatly increases detection of SAR11 bacterioplankton. Aquat. Microbial. Ecol. 75, 129–137. doi: 10.3354/ame01753
Arossa S., Martin C., Rossbach S., Duarte C. M. (2019). Microplastic removal by Red Sea giant clam (Tridacna maxima). Environ. pollut. 252, 1257–1266. doi: 10.1016/j.envpol.2019.05.149
Beaumont N. J., Aanesen M., Austen M. C., Börger T., Clark J. R., Cole M., et al. (2019). Global ecological, social and economic impacts of marine plastic. Mar. pollut. Bull. 142, 189–195). doi: 10.1016/j.marpolbul.2019.03.022
Boettcher K. J., Geaghan K. K., Maloy A. P., Barber B. J. (2005). Roseovarius crassostreae sp. nov., a member of the Roseobacter clade and the apparent cause of juvenile oyster disease (JOD) in cultured Eastern oysters. Int. J. System. Evol. Microbiol. 55, 1531–1537. doi: 10.1099/ijs.0.63620-0
Bokulich N. A., Kaehler B. D., Rideout J. R., Dillon M., Bolyen E., Knight R., et al. (2018). Optimizing taxonomic classification of marker-gene amplicon sequences with QIIME 2’s q2-feature-classifier plugin. Microbiome 6, 1–17. doi: 10.1186/s40168-018-0470-z
Bolyen E., Rideout J. R., Dillon M. R., Bokulich N. A., Abnet C. C., Al-Ghalith G. A., et al. (2019). Reproducible, interactive, scalable and extensible microbiome data science using QIIME 2. Nat. Biotechnol. 37, 852–857. doi: 10.1038/s41587-019-0209-9
Bruckner A., Wright J., Kampichler C., Bauer R., Kandeler E. (1995). A method of preparing mesocosms for assessing complex biotic processes in soils. Biol. Fertil. Soils 19, 257–262. doi: 10.1007/BF00336169
Bruyère O., Le Gendre R., Liao V., Andréfouët S. (2024). Lagoon hydrodynamics of pearl farming islands: The case of Gambier (French Polynesia). Earth Sys. Sci. Data 16, 667–679. doi: 10.5194/essd-16-667-2024
Callahan B. J., McMurdie P. J., Rosen M. J., Han A. W., Johnson A. J. A., Holmes S. P. (2016). DADA2: High-resolution sample inference from Illumina amplicon data. Nat. Methods 13, 581–583. doi: 10.1038/nmeth.3869
Conway J. R., Lex A., Gehlenborg N. (2017). UpSetR: An R package for the visualization of intersecting sets and their properties. Bioinformatics 33, 2938–2940. doi: 10.1093/bioinformatics/btx364
Cooney R. P., Pantos O., Le Tissier M. D. A., Barer M. R., O’Donnell A. G., Bythell J. C. (2002). Characterization of the bacterial consortium associated with black band disease in coral using molecular microbiological techniques. Environ. Microbiol. 4, 401–413. doi: 10.1046/j.1462-2920.2002.00308.x
Crossland N. O., La Point T. W. (1992). The design of mesocosm experiments. Environ. Toxicol. Chem. 11, 1–4. doi: 10.1002/etc.5620110101
Crusot M., Gaertner J.-C., Rodriguez T., Lo C., Gaertner-Mazouni N. (2023). Assessment of plastic waste generated by the aquaculture industry: The case study of pearl farming in French Polynesia. J. Clean. Prod. 425, 138902. doi: 10.1016/j.jclepro.2023.138902
Dang H., Lovell C. R. (2016). Microbial surface colonization and biofilm development in marine environments. Microbiol. Mol. Biol. Rev. 80, 91–138. doi: 10.1128/mmbr.00037-15
Debroas D., Mone A., Ter Halle A. (2017). Plastics in the North Atlantic garbage patch: a boat-microbe for hitchhikers and plastic degraders. Sci. total Environ. 599, 1222–1232. doi: 10.1016/j.scitotenv.2017.05.059
Détrée C., Gallardo-Escárate C. (2018). Single and repetitive microplastics exposures induce immune system modulation and homeostasis alteration in the edible mussel Mytilus galloprovincialis. Fish Shellfish Immunol. 83, 52–60. doi: 10.1016/j.fsi.2018.09.018
Ding J., Sun C., He C., Li J., Ju P., Li F. (2021). Microplastics in four bivalve species and basis for using bivalves as bioindicators of microplastic pollution. Sci. Total Environ. 782, 146830. doi: 10.1016/j.scitotenv.2021.146830
DRM (Direction des Ressources Marines) (2015). Rapport de repérage et d’évaluation des déchets immergés identification des hoa à curer, lagon de Takaroa, BC n°28469, Décembre 2015 22.
DRM (Direction des Ressources Marines) (2022). Statistiques 2022: La perliculture. Available online at: https://www.ressources-marines.gov.pf/2023/06/12/statistiques-la-perliculture/.
Dussud C., Hudec C., George M., Fabre P., Higgs P., Bruzaud S., et al. (2018). Colonization of non-biodegradable and biodegradable plastics by marine microorganisms. Front. Microbiol. 9. doi: 10.3389/fmicb.2018.01571
Fernandes N., Steinberg P., Rusch D., Kjelleberg S., Thomas T. (2012). Community structure and functional gene profile of bacteria on healthy and diseased thalli of the red seaweed delisea pulchra. PloS One 7, 1–8. doi: 10.1371/journal.pone.0050854
Gall S. C., Thompson R. C. (2015). The impact of debris on marine life. Mar. pollut. Bull. 92, 170–179. doi: 10.1016/j.marpolbul.2014.12.041
Gambarini V., Pantos O., Kingsbury J. M., Weaver L., Handley K. M., Lear G. (2021). Phylogenetic distribution of plastic-degrading microorganisms. MSystems 6 (1), 10–1128. doi: 10.1128/msystems.01112-20
Gardon T., El Rakwe M., Paul-Pont I., Le Luyer J., Thomas L., Prado E., et al. (2021). Microplastics contamination in pearl-farming lagoons of French Polynesia. J. Hazard. Mater. 419, 126396. doi: 10.1016/j.jhazmat.2021.126396
Gardon T., Huvet A., Paul-Pont I., Cassone A. L., Sham Koua M., Soyez C., et al. (2020a). Toxic effects of leachates from plastic pearl-farming gear on embryo-larval development in the pearl oyster Pinctada margaritifera. Water Res. 179, 115890. doi: 10.1016/j.watres.2020.115890
Gardon T., Morvan L., Huvet A., Quillien V., Soyez C., Le Moullac G., et al. (2020b). Microplastics induce dose-specific transcriptomic disruptions in energy metabolism and immunity of the pearl oyster Pinctada margaritifera. Environ. pollut. 266, 115180. doi: 10.1016/j.envpol.2020.115180
Gardon T., Reisser C., Soyez C., Quillien V., Le Moullac G. (2018). Microplastics affect energy balance and gametogenesis in the pearl oyster pinctada margaritifera. Environ. Sci. Technol. 52, 5277–5286. doi: 10.1021/acs.est.8b00168
Gilbert A., Yan L., Remoissenet G., Andréfouët S., Payri C., Chancerelle Y. (2005). Extraordinarily high giant clam density under protection in Tatakoto atoll (Eastern Tuamotu archipelago, French Polynesia). Coral Reefs 24, 495. doi: 10.1007/s00338-005-0494-2
Godéré I., Gaertner J. C., Dassié E. P., Belamy T., Maihota N., Baudrimont M., et al. (2023). Metallic trace element contamination of the giant clam Tridacna maxima in French Polynesia. Mar. pollut. Bull. 196, 115639. doi: 10.1016/j.marpolbul.2023.115639
Goldstein M. C., Carson H. S., Eriksen M. (2014). Relationship of diversity and habitat area in North Pacific plastic-associated rafting communities. Mar. Biol. 161, 1441–1453. doi: 10.1007/s00227-014-2432-8
Goulais M., Saulnier D., Rouxel J., Galgani F. (2024). Bioindicator species of plastic toxicity in tropical environments. Sci. total Environ. 954. doi: 10.1016/j.scitotenv.2024.176185
Guibert I., Lecellier G., Torda G., Pochon X., Berteaux-Lecellier V. (2020). Metabarcoding reveals distinct microbiotypes in the giant clam Tridacna maxima. Microbiome 8, 1–14. doi: 10.1186/s40168-020-00835-8
Hou D., Hong M., Wang Y., Dong P., Cheng H., Yan H., et al. (2021). Assessing the risks of potential bacterial pathogens attaching to different microplastics during the summer–autumn period in a mariculture cage. Microorganisms 9, 1909. doi: 10.3390/microorganisms9091909
Iwaki H., Nishimura A., Hasegawa Y. (2012). Isolation and characterization of marine bacteria capable of utilizing phthalate. World J. Microbiol. Biotechnol. 28, 1321–1325. doi: 10.1007/s11274-011-0925-x
Jacquin J., Cheng J., Odobel C., Pandin C., Conan P., Pujo-Pay M., et al. (2019). Microbial ecotoxicology of marine plastic debris: A review on colonization and biodegradation by the “plastisphere. Front. Microbiol. 10. doi: 10.3389/fmicb.2019.00865
Jaubert J. M. (2013). Scientific considerations on a technique of ecological purification that made possible the cultivation of reef-building corals in Monaco, Vol. 2. Eds. Leewis R. J., Janse M., 115–126.
Jin Y., Lu L., Tu W., Luo T., Fu Z. (2019). Impacts of polystyrene microplastic on the gut barrier, microbiota and metabolism of mice. Sci. total Environ. 649, 308–317. doi: 10.1016/j.scitotenv.2018.08.353
Joachim S., Morin A., Thybaud É. (2009). Effet du cuivre sur la structure et le fonctionnement des écosystèmes aquatiques. Actual. Chimique 334, 55–57.
Junaid M., Siddiqui J. A., Sadaf M., Liu S., Wang J. (2022). Enrichment and dissemination of bacterial pathogens by microplastics in the aquatic environment. Sci. total Environ. 830, 154720. doi: 10.1016/j.scitotenv.2022.154720
Kaandorp M. L. A., Lobelle D., Kehl C., Dijkstra H. A., van Sebille E. (2023). Global mass of buoyant marine plastics dominated by large long-lived debris. Nat. Geosci. 16, 689–694. doi: 10.1038/s41561-023-01216-0
Kalmbach S., Manz W., Bendinger B., Szewzyk U. (2000). In situ probing reveals Aquabacterium commune as a widespread and highly abundant bacterial species in drinking water biofilms. Water Res. 34, 575–581. doi: 10.1016/S0043-1354(99)00179-7
Ke A. Y., Chen J., Zhu J., Wang Y. H., Hu Y., Fan Z. L., et al. (2019). Impacts of leachates from single-use polyethylene plastic bags on the early development of clam Meretrix meretrix (Bivalvia: Veneridae). Mar. pollut. Bull. 142, 54–57. doi: 10.1016/j.marpolbul.2019.03.029
Killam D., Thompson D., Morgan K., Russell M. (2023). Giant clams as open-source, scalable reef environmental biomonitors. PloS One 18, 1–22. doi: 10.1371/journal.pone.0278752
Kirstein I. V., Kirmizi S., Wichels A., Garin-Fernandez A., Erler R., Löder M., et al. (2016). Dangerous hitchhikers? Evidence for potentially pathogenic Vibrio spp. on microplastic particles. Mar. Environ. Res. 120, 1–8. doi: 10.1016/j.marenvres.2016.07.004
Klein K., Heß S., Schulte-Oehlmann U., Oehlmann J. (2021). Locomotor behavior of Neocaridina palmata : a study with leachates from UV-weathered microplastics. PeerJ 9, e12442. doi: 10.7717/peerj.12442
Kurchaba N., Cassone B. J., Northam C., Ardelli B. F., LeMoine C. M. (2020). Effects of mp polyethylene microparticles on microbiome and inflammatory response of larval zebrafish. Toxics 8, 55. doi: 10.3390/toxics8030055
Kusuda R., Kawai K., Salati F., Kawamura Y., Yamashita Y. (1992). Characteristics of Flavobacterium sp. causing “Suminori“ disease in cultivated Porphyra. Aquacult. Sci. 40, 457–461. doi: 10.11233/aquaculturesci1953.40.457
Laist D. W. (1997). Impacts of marine debris: entanglement of marine life in marine debris including a comprehensive list of species with entanglement and ingestion records. In Marine debris: sources, impacts, and solutions. New York, NY: Springer New York, 99–139. doi: 10.1007/978-1-4613-8486-1_10
Laporte M., Reny-Nolin E., Chouinard V., Hernandez C., Normandeau E., Bougas B., et al. (2021). Proper environmental DNA metabarcoding data transformation reveals temporal stability of fish communities in a dendritic river system. Environ. DNA 3, 1007–1022. doi: 10.1002/edn3.224
Lear G., Kingsbury J. M., Franchini S., Gambarini V., Maday S. D. M., Wallbank J. A., et al. (2021). Plastics and the microbiome: impacts and solutions. Environ. Microb. 16, 1–19. doi: 10.1186/s40793-020-00371-w
Lebreton L., Andrady A. (2019). Future scenarios of global plastic waste generation and disposal. Palgrave Commun. 5, 1–11. doi: 10.1057/s41599-018-0212-7
Legendre P., Anderson M. J. (1999). Distance-based redundancy analysis: Testing multispecies responses in multifactorial ecological experiments. Ecol. Monogr. 69, 1–24. doi: 10.1890/0012-9615(1999)069[0001:DBRATM]2.0.CO;2
Lemonnier C., Chalopin M., Huvet A., Le Roux F., Labreuche Y., Petton B., et al. (2022). Time-series incubations in a coastal environment illuminates the importance of early colonizers and the complexity of bacterial biofilm dynamics on marine plastics. Environ. pollut. 312, 119994. doi: 10.1016/j.envpol.2022.119994
Li J., Lusher A. L., Rotchell J. M., Deudero S., Turra A., Bråte I. L. N., et al. (2019). Using mussel as a global bioindicator of coastal microplastic pollution. Environ. pollut. 244, 522–533. doi: 10.1016/j.envpol.2018.10.032
Lopez P., Saulnier D., Swarup-Gaucher S., David R., Lau C., Taputuarai R., et al. (2022). First Isolation of Virulent Tenacibaculum maritimum Isolates from Diseased Orbicular Batfish (Platax orbicularis) Farmed in Tahiti Island. Pathogens 11 (2), 131. doi: 10.3390/pathogens11020131
Lott C., Eich A., Unger B., Makarow D., Battagliarin G., Schlegel K., et al. (2020). Field and mesocosm methods to test biodegradable plastic film under marine conditions. PloS One 15, 1–26. doi: 10.1371/journal.pone.0236579
Lu L., Wan Z., Luo T., Fu Z., Jin Y. (2018). Polystyrene microplastics induce gut microbiota dysbiosis and hepatic lipid metabolism disorder in mice. Sci. Total Environ. 631, 449–458.
Martin M. (2011). Cutadapt removes adapter sequences from high-throughput sequencing reads. EMBnet. J. 7, 2803–2809. doi: 10.14806/ej.17.1.200
Martínez-Campos S., González-Pleiter M., Fernández-Piñas F., Rosal R., Leganés F. (2021). Early and differential bacterial colonization on microplastics deployed into the effluents of wastewater treatment plants. Sci. Total Environ. 757, 143832. doi: 10.1016/j.scitotenv.2020.143832
Multisanti C. R., Merola C., Perugini M., Aliko V., Faggio C. (2022). Sentinel species selection for monitoring microplastic pollution: A review on one health approach. Ecol. Indic. 145, 109587. doi: 10.1016/j.ecolind.2022.109587
Nowlan J. P., Lumsden J. S., Russell S. (2020). Advancements in characterizing Tenacibaculum infections in Canada. Pathogens 9, 1–35. doi: 10.3390/pathogens9121029
Olesen S. W., Duvallet C., Alm E. J. (2017). DbOTU3: A new implementation of distribution-based OTU calling. PloS One 12, 1–13. doi: 10.1371/journal.pone.0176335
Pantos O., Cooney R. P., Le Tissier M. D. A., Barer M. R., O’Donnell A. G., Bythell J. C. (2003). The bacterial ecology of a plague-like disease affecting the Caribbean coral Montastrea annularis. Environ. Microbiol. 5, 370–382. doi: 10.1046/j.1462-2920.2003.00427.x
Parada A. E., Needham D. M., Fuhrman J. A. (2016). Every base matters: Assessing small subunit rRNA primers for marine microbiomes with mock communities, time series and global field samples. Environ. Microbiol. 18, 1403–1414. doi: 10.1111/1462-2920.13023
Pinto M., Langer T. M., Hüffer T., Hofmann T., Herndl G. J. (2019). The composition of bacterial communities associated with plastic biofilms differs between different polymers and stages of biofilm succession. PloS One 14, 1–20. doi: 10.1371/journal.pone.0217165
Plastics Europe (2022). Plastics – the facts 2022. Available online at: https://plasticseurope.org/fr/knowledge-hub/plastics-the-facts-2022/ (Accessed October 2022).
Prokić M. D., Gavrilović B. R., Radovanović T. B., Gavrić J. P., Petrović T. G., Despotović S. G., et al. (2021). Studying microplastics: Lessons from evaluated literature on animal model organisms and experimental approaches. J. Hazard. Mater. 414, 125476. doi: 10.1016/j.jhazmat.2021.125476
Qiao R., Sheng C., Lu Y., Zhang Y., Ren H., Lemos B. (2019). Microplastics induce intestinal inflammation, oxidative stress, and disorders of metabolome and microbiome in zebrafish. Sci. Total Environ. 662, 246–253. doi: 10.1016/j.scitotenv.2019.01.245
Quast C., Pruesse E., Yilmaz P., Gerken J., Schweer T., Yarza P., et al. (2013). The SILVA ribosomal RNA gene database project: Improved data processing and web-based tools. Nucleic Acids Res. 41, 590–596. doi: 10.1093/nar/gks1219
Rezaei Somee M., Dastgheib S. M. M., Shavandi M., Ghanbari Maman L., Kavousi K., Amoozegar M. A., et al. (2021). Distinct microbial community along the chronic oil pollution continuum of the Persian Gulf converge with oil spill accidents. Sci. Rep. 11, 1–15. doi: 10.1038/s41598-021-90735-0
Rivas A. J., Lemos M. L., Osorio C. R. (2013). Photobacterium damselae subsp. a bacterium pathogenic for marine animals and humans. Front. Microbiol. 4. doi: 10.3389/fmicb.2013.00283
Romera-Castillo C., Pinto M., Langer T. M., Álvarez-Salgado X. A., Herndl G. J. (2018). Dissolved organic carbon leaching from plastics stimulates microbial activity in the ocean. Nat. Commun. 9, 1430. doi: 10.1038/s41467-018-03798-5
Rossbach S., Cardenas A., Perna G., Duarte C. M., Voolstra C. R. (2019). Tissue-specific microbiomes of the red sea giant clam tridacna maxima highlight differential abundance of endozoicomonadaceae. Front. Microbiol. 10. doi: 10.3389/fmicb.2019.02661
Sarkhel R., Sengupta S., Das P., Bhowal A. (2020). Comparative biodegradation study of polymer from plastic bottle waste using novel isolated bacteria and fungi from marine source. J. Polymer Res. 27, 1–8. doi: 10.1007/s10965-019-1973-4
Seeley M. E., Song B., Passie R., Hale R. C. (2020). Microplastics affect sedimentary microbial communities and nitrogen cycling. Nat. Commun. 11, 2372. doi: 10.1038/s41467-020-16235-3
Sehnal L., Brammer-Robbins E., Wormington A. M., Blaha L., Bisesi J., Larkin I., et al. (2021). Microbiome composition and function in aquatic vertebrates: small organisms making big impacts on aquatic animal health. Front. Microbiol. 12. doi: 10.3389/fmicb.2021.567408
Sheridan E. A., Fonvielle J. A., Cottingham S., Zhang Y., Dittmar T., Aldridge D. C., et al. (2022). Plastic pollution fosters more microbial growth in lakes than natural organic matter. Nat. Commun. 13, 4175. doi: 10.1038/s41467-022-31691-9
Sureda A., Capó X., Busquets-Cortés C., Tejada S. (2018). Acute exposure to sunscreen containing titanium induces an adaptive response and oxidative stress in Mytillus galloprovincialis. Ecotoxicol. Environ. Saf. 149, 58–63. doi: 10.1016/j.ecoenv.2017.11.014
Sweet M., Bulling M., Cerrano C. (2015). A novel sponge disease caused by a consortium of micro-organisms. Coral Reefs 34, 871–883. doi: 10.1007/s00338-015-1284-0
Tetu S. G., Sarker I., Moore L. R. (2020). How will marine plastic pollution affect bacterial primary producers? Commun. Biol. 3, 20–23. doi: 10.1038/s42003-020-0789-4
Tetu S. G., Sarker I., Schrameyer V., Pickford R., Elbourne L. D. H., Moore L. R., et al. (2019). Plastic leachates impair growth and oxygen production in Prochlorococcus, the ocean’s most abundant photosynthetic bacteria. Commun. Biol. 2, 1–9. doi: 10.1038/s42003-019-0410-x
Usyskin-Tonne A., Hadar Y., Yermiyahu U., Minz D. (2021). Elevated CO2 and nitrate levels increase wheat root-associated bacterial abundance and impact rhizosphere microbial community composition and function. ISME J. 15, 1073–1084. doi: 10.1038/s41396-020-00831-8
Venkatarama Sharma K., Kumar Sarvalingam B., Rudragouda Marigoudar S. (2021). A review of mesocosm experiments on heavy metals in marine environment and related issues of emerging concerns. Environ. Sci. pollut. Res. 28, 1304–1316. doi: 10.1007/s11356-020-11121-3
Vlaanderen E. J., Ghaly T. M., Moore L. R., Focardi A., Paulsen I. T., Tetu S. G. (2023). Plastic leachate exposure drives antibiotic resistance and virulence in marine bacterial communities. Environ. pollut. 327, 121558. doi: 10.1016/j.envpol.2023.121558
Wang H., Liu H., Zhang Y., Zhang L., Wang Q., Zhao Y. (2023). The toxicity of microplastics and their leachates to embryonic development of the sea cucumber Apostichopus japonicus. Mar. Environ. Res. 190, 106114. doi: 10.1016/j.marenvres.2023.106114
Winnepennickx B., Backeljau T., De Wachter R. (1993). Technical tips: Extraction of high molecular weight DNA from molluscs. Tig 9, 407. https://hdl.handle.net/10067/53200151162165141.
Wright R. J., Erni-Cassola G., Zadjelovic V., Latva M., Christie-Oleza J. A. (2020). Marine plastic debris: A new surface for microbial colonization. Environ. Sci. Technol. 54, 11657–11672. doi: 10.1021/acs.est.0c02305
Keywords: plastic pollution, bacteria, abundance, mesocosms, pearl farming
Citation: Goulais M, Darinot-Thomas S, Mitta G, Galgani F, Saulnier D and Benestan L (2024) Plastic is in the details: the impact of plastic pollution through a mesocosm experiment. Front. Mar. Sci. 11:1486802. doi: 10.3389/fmars.2024.1486802
Received: 26 August 2024; Accepted: 28 October 2024;
Published: 21 November 2024.
Edited by:
Teresa Bottari, National Research Council (CNR), ItalyReviewed by:
Fabiana Corami, National Research Council (CNR), ItalySun Cuici, Chinese Academy of Sciences (CAS), China
Copyright © 2024 Goulais, Darinot-Thomas, Mitta, Galgani, Saulnier and Benestan. This is an open-access article distributed under the terms of the Creative Commons Attribution License (CC BY). The use, distribution or reproduction in other forums is permitted, provided the original author(s) and the copyright owner(s) are credited and that the original publication in this journal is cited, in accordance with accepted academic practice. No use, distribution or reproduction is permitted which does not comply with these terms.
*Correspondence: Maeva Goulais, TWFldmEuR291bGFpc0BpZnJlbWVyLmZy