- MOE Key Laboratory of Thermo-Fluid Science and Engineering, Xi’an Jiaotong University, Xi’an, Shaanxi, China
With increasing population and urbanization, the amount of municipal sewage sludge generated is huge and growing rapidly. In order to minimize resource inputs and pollutant emissions in the sludge disposal process, it is crucial to carry out an environmental impact analysis and sustainability assessment of different strategies based on life cycle assessment (LCA). LCA provides a flexible framework for quantifying the consumption and emissions of different processes to determine the energy consumption and environmental impact of sewage sludge treatment and disposal. The aim of this review is to compare the energy consumption and GHG emissions of existing sludge management options for energy and nutrient recovery. At the same time, the characteristics of sewage sludge and the potential to convert sludge from waste to valuable products were assessed. While the excessive moisture content and ash content make sludge unsuitable for use as fuel, and the potential risk of contamination with heavy metals makes it less suitable for use as organic fertilizer, energy and material recovery during disposal can reduce disposal costs and environmental impacts. In the context of the current limitations reviewed, the level of potential GHG emissions of existing sludge treatment and disposal routes is: composting > anaerobic digestion > pyrolysis > incineration. With suitable sludge treatment routes, negative GHG emissions could potentially be achieved by substituting fossil fuels for heat and electricity generation, or as a fertilizer substitute. The development and application of future sludge management strategies should aim at reduction and harmless disposal, thereby reducing the operating costs and environmental burdens through resources.
1 Introduction
Wastewater treatment plants (WWTPs) are facilities used to treat domestic and industrial wastewater. Various processes (e.g., physical, chemical, and biological) are combined to remove pollutants from industrial or human activities to minimize environmental and human health damage caused by wastewater from WWTPs (Hreiz et al., 2015). Sewage sludge is the waste residue from primary/secondary sedimentation tanks and other linked equipment in the wastewater treatment process (Bolobajev et al., 2014), which has potential ecological hazards due to the residual organic matter and heavy metals from treated wastewater. Without proper management, it can lead to serious environmental issues, such as odors, disease transmission, heavy metal bioaccumulation and greenhouse gas (GHG) emissions (Singh et al., 2011). Consequently, it is imperative to follow a series of treatment and disposal procedures, e.g., thickening, anaerobic digestion (AD), dewatering, thermal drying, incineration, agricultural use, landfill, etc. Dewatering is the primary step in minimizing the water content and volume of sludge (Wu et al., 2020; Yu et al., 2023a). The essential requirements for stable and harmless treatment are the decomposition of perishable organic pollutants and the removal of microorganisms such as pathogenic bacteria and viruses. Meanwhile, sludge, which is part of organic waste containing renewable organic matter, can be considered a sustainable resource in terms of nutrients or energy recovery (Pradel et al., 2016), thereby achieving the ultimate goal of resource utilization.
In addition, population growth, urbanization and industrialization, coupled with mismanagement, have heightened the urgent need and concern for effective sludge disposal. According to Eurostat statistics, sewage sludge production in the European Union amounts to about 15 million tons of dry solids (DS) in 2020 (Eurostat, 2022). Statistics from “Report on the State of the Ecology and Environment in China 2022”showed that the total capacity of China’s urban WWTPs was 58.46 billion cubic meters in 2021. The annual production of urban sewage sludge in China was approximately 13.6 million tons (DS). Currently, agriculture use and incineration are the main disposal methods in European countries, accounting for 32.96% and 32.56% of total disposal, respectively (Eurostat, 2022). Incineration, land application, landfills, and building materials production are the most widely used sludge disposal methods in China, with 26.7%, 29.3%, 20.1% and 15.9%, respectively (Lu, 2019).
The priority objectives of sludge disposal are to reduce, optimize management costs and/or produce a clean material that can be safely used to recover energy and valuable materials. Sewage sludge, defined as urban biomass, can be converted into energy, enabling the resourceful use of sludge as an alternative fuel (Guo et al., 2022) (Zhang et al., 2021), potentially reducing fossil fuel consumption and CO2 emissions (Nakatsuka et al., 2020; Zhao et al., 2022). It can also recover effective elements to replace chemical fertilizers. It should be noted that the carbon in sewage sludge is regarded as 100% biogenic based on the Intergovernmental Panel on Climate Change (IPCC) guidelines (IPCC, 2000). Thus, sludge can be considered as a carbon-neutral biomass fuel in the energy recovery process via combustion. Meanwhile, sludge is a potential phosphorus resource, with relevant studies showing that P accounts for 2%–10% of dried sludge (Lee et al., 2020). Thermal processing of sewage sludge, i.e., incineration, pyrolysis and hydrothermal treatment, can convert phosphorus to various species to improve its bioavailability (Kosacka et al., 2021). Crystallization, bio-leaching and acid/base leaching are applied for P recovery from sludge (Li et al., 2019), (Biswas et al., 2009; Mehta et al., 2015; Lee et al., 2018).
Sludge has the dual nature of waste and potential resource, allowing the recovery of valuable elements and carbon-neutral energy during disposal. However, the paramount problem in extracting usable energy from sludge is the consumption of large amounts of fossil fuels for drying (Nakatsuka et al., 2020; Yu et al., 2023b), as the moisture content of sludge can only be reduced to 70%–80% by conventional mechanical dewatering technique (Bianchini et al., 2015). Therefore, thermal drying is essential to reduce the moisture content considerably. Moreover, biological methods such as anaerobic digestion require supplementary heat to maintain the reaction temperatures (35°C, mesophilic conditions). Thus, it is necessary to clarify when sludge is waste (net energy consumption) and when it is product (net energy/valuable product output) in the different disposal routes, so that the environmental burden could be saved in a reasonable way (Pradel et al., 2016). Achieving safe and low-carbon disposal of sludge has become one of the most challenging topics in solid waste disposal.
Life cycle assessment (LCA) of sludge treatment and disposal has been broadly applied in recent years. It can quantify the environmental impact of energy and resource consumption over the life cycle of the waste management technology to select the resource recovery technology with the minimum environmental load (Lin et al., 2016). Academics at home and abroad have applied the LCA method to critically analyze and study the environmental impact of sludge disposal. LCA provided a quantitative evaluation method for environmental impacts from process selection to policy assessment and highlights the life cycle impact assessment methods on environmental emissions (Yoshida et al., 2013). However, no detailed investigation of their study has been carried out. Pradel et al. (Pradel et al., 2016) reviewed 44 papers on wastewater treatment life cycle assessment and determined the impact of sludge conversion from “waste” to “product” on the GOAL and SCOPE of LCA. The system boundaries may become unequal between “waste to product” of sludge and nutrient/material recovery scenarios. Kacprzak et al. (Kacprzak et al., 2017; Fang et al., 2023) outlined some basic criteria for judging the maximization of the circular economy “waste to resource” concept. Tech et al. (Teoh and Li, 2020) assessed and reviewed 67 research breakthroughs (including 32 LCA-related literature reviews) published between 2000 and 2018 using a semi-quantitative assessment approach. The reduction of sludge volume/weight and the environmental effects of various sludge treatment methods (including biological, chemical, thermal and thermochemical) were determined. Ding et al. (Ding et al., 2021) analyzed each LCA procedure (goal, scope, inventory, impact assessment methodology and interpretation) to identify differences in factors and evaluate sludge disposal technologies based on nutrient/energy recovery-oriented sludge disposal LCA. In past published reviews, numerous scholars have focused on LCA models and approaches for sludge management strategies, as well as the overall impact of different sludge disposal methods on climate change, human health, ecosystem quality, and resources. Few works paid attention to the detailed impacts of greenhouse gas (GHG) emissions and energy consumption on the LCA of sludge treatment and disposal routes.
To this end, this review is structured as follows: 1) an overview of existing mainstream sludge treatment and disposal routes and LCA methods; 2) summarize sewage sludge characteristics from the published literature to evaluate the conversion potential from waste to valuable products; 3) analysis of GHG emissions and energy consumption of sewage sludge treatment and disposal routes; 4) describe the main challenges and outline development prospects.
2 Overview of LCA on sludge treatment and disposal routes
2.1 Main steam sludge treatment and disposal routes
Land application, composting, incineration, anaerobic digestion, pyrolysis and landfilling are the most commonly used sludge management methods, to recover energy and nutrients from sludge (Liu et al., 2017). All sludge treatment and disposal methods have their advantages and disadvantages (Table 1).
2.1.1 Composting
Composting of organic waste has long been considered an attractive method of sludge management for nutrient recovery (Nguyen and Shima, 2019). Sewage sludge and its compost contain valuable nutrients and humus substances that can be used as organic fertilizers. Compost is used as a fertilizer substitute, offsetting the equivalent amount of chemical fertilizer based on the phosphorus content (Sablayrolles et al., 2010). Apart from providing plant nutrition (phosphorus, nitrogen and potassium), the organic substance contained in compost stabilizes and/or improves soil humus content (Shaddel et al., 2019). To increase the carbon content of sludge compost, thickened sludge is normally mixed with wood chips or sawdust (Tarpani et al., 2020).
2.1.2 Anaerobic digestion
Anaerobic digestion (AD) is a biochemical process that converts organic matter from waste (sludge, agro-industrial wastes) into biogas, biochar, and liquid that can be used for methane-rich bioenergy, soil conditioners, and liquid fertilizer, respectively (Bernard et al., 2020). AD is commonly used to stabilize sludge, a natural process of microbial decomposition of organic matter under anaerobic conditions (Linyi et al., 2020; Sheng et al., 2022), at mesophilic (around 30°C–37°C) or thermophilic (around 50°C–55°C) conditions (Hidaka et al., 2016) in sealed vessel reactors of various shapes and sizes specific to the site and feedstock conditions.
2.1.3 Pyrolysis
Pyrolysis refers to the decomposition of organic matter under medium and high temperatures (between 300°C and 1,300°C), in the absence of oxygen and under substantially dry conditions. Pyrolysis process generally operates under atmospheric pressure (Ghodke et al., 2021), 0.1–0.2 bar pressure (vacuum pyrolysis) (Murwanashyaka et al., 2001) or 50–200 bar pressures (hydro-pyrolysis) (Frišták et al., 2018). Syngas and bio-oil, the basic products of the sludge pyrolysis process, can be used as bioenergy, replacing fossil fuels, while biochar can be used as a carbon-rich product for soil amendment or fertilizer to increase carbon sequestration in the soil (Akhter et al., 2015; Karer et al., 2015; Rajec et al., 2016).
2.1.4 Incineration
The organic compounds of sludge are entirely oxidized by incineration at high temperatures (Tyagi and Lo, 2016). In this process, sludge is burned in the combustion chamber supplied with excess air (oxygen) to convert the organic carbon, nitrogen, sulfur, nitrogen and phosphorus into gas and mineral solid products (Cui et al., 2006). Models of sludge incineration include mono- and co-incineration. Wet or dried sludge is incinerated in fluidized bed combustors (FBCs) at 850°C, with the addition of auxiliary fuels to maintain combustion (Hospido et al., 2005). Alternatively, sludge is co-combusted in coal-fired boilers and cement kilns as an additional fuel. The process of wet sludge drying has elevated energy consumption in terms of water removal, the dewatered sludge (80–85 wt% moisture content) needs to be further dried up to 10–30 wt% in the traditional energy-consuming thermal drying methods to meet combustion requirements (Ma et al., 2016; Raja and Alphin, 2020). Due to the low calorific value of sludge, mono-incineration and co-incineration process is commonly supplemented by additional fuels (coal, oil or natural gas) (Cieślik et al., 2015; Liang et al., 2021; Zamparas, 2021).
2.2 Life cycle assessment method
Life cycle assessment (LCA) is a globally recognized analysis method for assessing the environmental impacts of systems and is standardized by the International Standardization Organization (ISO). LCA is typically conducted through four phases: goal and scope definition, inventory analysis, impact assessment, and interpretation (Godlee, 2006).
The goal of LCA indicates the intended application and the reason for conducting the study, as well as the audience and comparison assertions. The scope definition is sufficient to ensure the breadth, depth and detail of the research to meet the stated goals. The functional unit (FU), as a measure of the output function of the product system, is to supply a reference benchmark for related inputs and outputs. FU is a crucial basis for the simultaneous comparison and analysis of optional scenarios (Hertwich et al., 2001; Teoh and Li, 2020). In previous LCA studies of sewage sludge treatment units, the specified amounts of sewage or sludge mass is a common FU (Hospido et al., 2004; Yoshida et al., 2013; Ding et al., 2021).
System boundary defines the boundary of the basic flow unit process of inputs and outputs, which have a significant impact on LCA (Finnveden et al., 2009; Pradel et al., 2016). Life cycle inventory analysis covers energy and material requirements, pollutant emissions and environmental hazards resulting from raw materials mining, refining, product manufacturing, transportation, sales, consumption and disposal, and is referred to as foreground data, followed by raw material data form processing technologies (Pradel et al., 2016). Data for treatment techniques are commonly sourced from literature, field test data and LCI database, such as Econinvent (Yoshida et al., 2013; Ding et al., 2021). The construction and decommissioning of treatment plants are generally excluded, previous studies have shown their contribution to the impact to be negligible (Fransson et al., 2008; Yoshida et al., 2013). In general, LCI plays an essential role in LCA analysis, and LCI data can affect the quality and uncertainty of LCA results.
3 Characteristics of sewage sludge
3.1 Sewage sludge source
Sludge is a by-product of sewage treatment in wastewater treatment plants (WWTPs) that receive wastewater from several municipalities, including domestic, industrial, commercial, hospital, road and agricultural water, human excreta, personal care products, detergents, disinfectants, pharmaceuticals, sediments, etc (Cantinho et al., 2016). Most conventional WWTPs normally have the following steps: preliminary treatment, primary treatment and secondary treatment, the layout of which is shown in Figure 1.
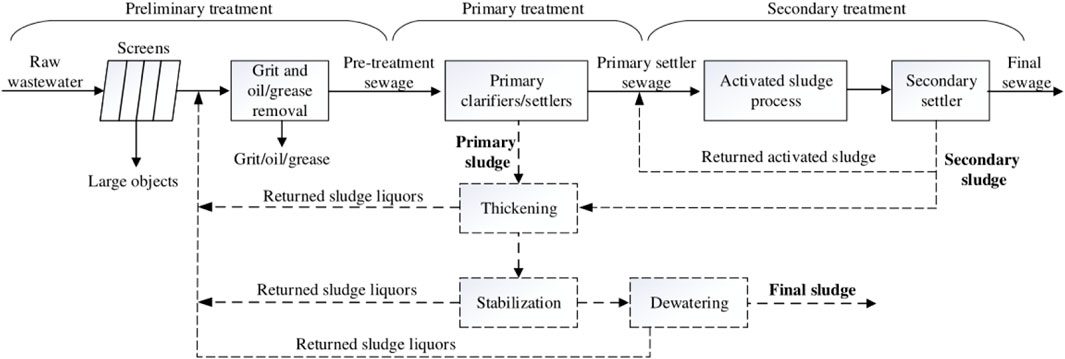
FIGURE 1. The treatment of municipal wastewater and the production of sewage sludge (Cantinho et al., 2016).
In preliminary processing, large objects in raw wastewater are filtered through a grating screen. Due to the difference in gravity, sand and grit settle in the gravel channel for treatment. In the primary treatment, grease and oil rise to the surface and are skimmed off in the primary clarifier/sedimentation tank, while sludge (in the form of semi-solids/slurry) settles at the bottom of the tank. The primary stage removes 50%–70% of the total suspended solids from the raw wastewater and the sludge produced in this stage is referred to as primary sludge (Pathak et al., 2009).
Secondary treatment is a biological process that uses microorganisms to stabilize organic constituents and remove non-colloidal solids. Activated sludge processes and settling tanks are commonly used for secondary treatment, producing secondary sludge. Additional treatment (tertiary treatment for nutrient removal) can be added to improve the sewage quality (Syed-Hassan et al., 2017). Sludge from WWTPs is generally treated in a sequence of thickening, stabilization/conditioning and dewatering to reduce the total weight and volume for transportation and additional disposal (Yoshida et al., 2013; Zhang et al., 2014).
3.2 Proximate and ultimate analysis
Proximate analysis includes moisture content (M), volatile content (V), fixed carbon content (FC) and ash content (A), and the moisture content of sewage sludge is extremely variable and shows large fluctuation. After thickening, the sludge mass is generally reduced by 70% and the moisture content of sludge is decreased to 97%–98% (Stefanakis et al., 2014). The moisture is significantly reduced to about 80% by dewatering (belt filter press or centrifuge) (Kumar and Kumar, 2018). Figure 2 showed the results of the dry basis proximate analysis (d in form of subscripts) obtained from previous literature (Domínguez et al., 2008; Zhai et al., 2012; Xiong et al., 2013; Zhang L. et al., 2015; Zhang Y. et al., 2015; Kan et al., 2016; Lishan et al., 2018). Sludge possessed a relatively stable Ad content of approximately 31.2–57.2 wt%, which is domainated by inorganic components left over from the wastewater treatment process. The volatile content of the sludge is extremely variable, i.e. 38.6–60 wt%. The fixed carbon content in the sludge is roughly below 11.2%. The proximate analysis of sludge varies widely compared to alternative fuels (biomass or coal), and sludge is considered a solid fuel with high ash and volatility (Zhu et al., 2017; Usman et al., 2020).
The ultimate analysis determines the content of C, H, O, N and S, Figure 3 shows the ultimate analysis data for the dried ash-free base of sludge reported in the literature (Domínguez et al., 2008; Zhai et al., 2012; Xiong et al., 2013; Zhang L. et al., 2015; Zhang Y. et al., 2015; Kan et al., 2016; Lishan et al., 2018). The C contents in sludge is dominant with about 40.6–57.4 wt%. The content of H in sludge is 3.7–10.6 wt% (average content of 7.4 wt%). The total content of O, N and S in sludge ranged from 34.6 to 54 wt%, with average levels of 31.3 wt%, 7.1 wt% and 2.6 wt% for O, N and S, respectively.
3.3 Heavy metals content
Figure 4 shows several typical heavy metal concentrations for different sewage sludge. The hollow symbols indicate the heavy content of different sludge from previous literature (Dai et al., 2007). And the solid symbols and error bars represent the average and maximum, minimum contents of heavy metals (Cheng et al., 2022). These data were obtained from the database of sewage sludge data containing heavy metals concentrations established by analyzing 104 data sets selected from 420 references on the China National Knowledge Infrastructure (CNKI). The heavy metal thresholds of sludge for agricultural use (Leval A) in “Control standards of pollutants in sludge for agricultural use (GB 4282–2018)" are listed as dotted line for reference. The avarage values of the eight typical heavy metals in the #1∼#6 sludge and the average content in the above sludge database are below the threshold, while the contents of As, Ni, Zn and Cu have the potential to exceed the threshold based on the previous statistical data.
Previous literature indicated that about 50%–80% of heavy metals (i.e., Cd, Hg, Pb, Cr, As, Ni, Zn and Cu) in wastewater enter suspended solids through physicochemical and biological interactions (passive cellular adsorption, i.e., complex and inorganic microprecipitation reactions, and active cellular uptake, i.e., bioaccumulation of heavy metals by biochemical reactions with intracellular compounds) (Pagnanelli et al., 2009; Choińska-Pulit et al., 2018; Huang et al., 2018; Qin et al., 2019; Wang et al., 2020; Yang et al., 2020). In particular, suspended sediments containing large amounts of organic matter, such as proteins, polysaccharides and humic substances, readily interact with heavy metals (Chojnacka, 2010; Qin et al., 2019). Cr, Cd and Cu originate mainly from pipeline sediments, Zn and As are mainly from agriculture and domestic sources, Ni is mainly industry and Pb originates from fuel combustion and traffic (Cheng et al., 2022).
4 Energy consumption and GHG emission
Due to the elevated moisture content of sludge, its reduction and harmless treatment processes require energy consumption such as electricity and fuel, which is relevant for the economic operation of sludge disposal. According to IPCC guidelines, the carbon in sewage sludge is considered to be biogenic, but the consumption of fossil fuels in sludge disposal leads to GHG emissions. Given the potential for global warming, a more accurate calculation of direct GHG emissions over the lifetime of the disposal is essential. GHG emissions from the sewage-treatment industry account for 1%–2% of total emissions in China (Xu et al., 2021). Life cycle inventory broadly plays an influential role in LCA analysis of energy consumption and GHG emissions. For example, the energy conversion capacity of sludge is extremely dependent on its organic matter content, presenting a state of fluctuating between 30%–80% (Li et al., 2017). In the case of incineration, the self-sustaining combustibility and calorific value of sludge are inversely proportional to the moisture content but directly proportional to organic matter content (Komilis et al., 2014), which causes the distinction in energy recovery efficiency and GHG emissions of different disposal methods under different inventory data.
4.1 Composting
Composting is an aerobic process in which most of the degradable organic carbon in waste is converted into CO2. CH4 is formed in the anaerobic processes, and is mostly oxidized in aerobic. CH4 released into the atmosphere accounts for between one and several percent of the initial carbon content of the sludge. N2O is also emitted during composting, ranging from 0.5% to 5% of the initial nitrogen content. Operating electricity for auxiliary machinery such as mixers and conveyors is the main energy consumption in the composting process. The electricity consumption of the compost mixing process was 33.2 kW h/t DS and that of the fermentation and maturation process was 501 kW h/t DS (Tarpani et al., 2020).
The environmental impact of the agricultural application of composted sludge was investigated and the net GHG emissions were 197 kg CO2-eq/t DS. The main contribution of different life cycle stages to GHG emissions is sludge treatment (according to 75.25% of the total emissions), that is, CH4 emissions from sludge composting and CO2 emissions corresponding to electricity consumption. The credits for avoiding CO2 emissions accounted for −19.54%, mainly due to compost being used as a fertilizer substitute. Piippo et al. (Piippo et al., 2017) assessed the GHG emissions from composting in the form of “with CO2” and “without CO2,” which depends on whether the biological CO2 is considered. The net GHG emission from composting is 2047.1 kg CO2-eq/t DS, or 1113.8 kg CO2-eq/t DS excluding biological CO2. Biological CO2 is mainly expelled during composting, and CH4 and N2O are mainly produced during the composting process. The avoided CO2 emission is 288 kg CO2-eq/t DS. By using compost as fertilizer, it can replace some chemical fertilizers and reduce the emissions in their production. Lishan et al. (Lishan et al., 2018) assessed the CO2-eq emissions of composting. The amount of CO2-eq produced in the composting process is 1097.9 kgCO2-eq/t DS, and the disposal process is a major contributor to CO2 emissions compared to transport and dewatering.
The GHG emission during composting is about 180–1100 kg CO2-eq/t DS (Hong et al., 2009; Sablayrolles et al., 2010; Lombardi et al., 2017), which is mainly affected by different profiles of grid electricity and alternative schemes of compost.
4.2 Anaerobic digestion
Anaerobic digestion, a low-energy biochemical process, is recognized as a cost-effective method for solid waste disposal and resource recovery. During anaerobic digestion of sludge, biodegradable waste is decomposed into biogas, which is a mixture of CO2, CH4 and a slight amount of addition gases (Yan et al., 2021).
Anaerobic digestion process, belt filter dewatering after anaerobic digestion, storage and agricultural application consume 88.6 kW h, 49.1 kW h and 58.5 kW h electricity respectively (Tarpani et al., 2020). Digestion can replace 50–100 kg of equivalent synthetic NPK 15-15-15 fertilizer, depending on the phosphorus content. Biogas is used to maintain the digester at 35°C (mesophilic conditions), and the excess is used for electricity generation of 397–794 kW h. Li and Feng (Li and Feng, 2018) compared the integrated approach with anaerobic digestion and pyrolysis in terms of life cycle environmental impact and energy efficiency. The energy consumption of the anaerobic digestion process is 2.8 GJ/t DS of heat and 50 kW h/t DS of electricity. The energy consumption of thermal drying is 2940 MJ/t H2O of heat and 40 kW h/t H2O of electricity.
Tarpani et al. (Tarpani et al., 2020) assessed the GHG emissions from anaerobic digestion, and the results showed that the main contributors were CH4 emissions from agricultural application (80 kg CO2-eq/t DS) and fossil CO2 from emissions from grid electricity generation (59 kg CO2-eq/t DS). The credits GHG emissions mainly include digestion as substitute fertilizer (−127 kg CO2-eq/t DS) and biogas for electricity generation (−239 kg CO2-eq/t DS). Piippo et al. (Piippo et al., 2017) assessed the GHG emissions from anaerobic digestion. The net GHG emission is 141.15 kg CO2-eq/t DS, which is −341.3 kg CO2-eq/t DS without accounting for biological CO2. Biolohical CO2 is mainly expelled during the utilization process of biogas in the CHP process and abiotic CO2 is mainly produced during the utilization process of digestion. The avoided CO2 emission is −565.7 kg CO2-eq/t DS by replacing some chemical fertilizers with compost as fertilizer and reducing CO2 emissions. The GHG emission of the anaerobic digestion and agricultural application route is −280–650 kg CO2eq/t DS (Murray et al., 2008; Peters and Rowley, 2009; Gourdet et al., 2016). Variation is mainly due to different sludge treatment conditions and the amount of electricity generated and fertilizer avoided.
4.3 Pyrolysis
Pyrolysis of dried sludge produces syngas, tar and/or biochar, and the drying process and pyrolysis require energy consumption. The syngas generated is used to aid the pyrolytic process and the excess can be exported. The production of tar and char is also credited with producing comparable amounts of fossil fuels such as heavy fuel oil and coal.
The filter press process, the thermal drying process and the pyrolysis process of the pyrolysis route consume 40, 118 and 244 kW h of electricity, respectively. During the thermal drying process, 1638 kW h of heat is supplied from a natural gas industrial furnace. In the pyrolysis process, 115–230 kg of biochar and 20–40 kg of bio-oil can be recovered, and 2.1–4.2 kW h of heat can also be recovered by pyrolysis gas (Tarpani et al., 2020). In the pyrolysis pathway, electricity of 77.78 kW h/t DS is used for the operation of reactors, conveyors and accessory devices and natural gas is used as the complementary energy of 800–1,320 MJ/t DS for dewatered sludge (60%wt moisture content) pyrolysis, which is depended on the organic content and pyrolysis condition, e.g., 1,180 MJ/t DS at 550°C (Hossain et al., 2010), 1,147 MJ/t DS at 500°C (Agarwal et al., 2015) and 950 MJ/t DS at 450°C (Tomasi Morgano et al., 2018).
The GHG emission from the pyrolysis route is 315 kg CO2-eq/t DS, of which 422 kg CO2-eq/t DS can be produced from the gas consumed in the thermal drying process. The utilization of pyrolysis gas, biochar and bio-oil can reduce GHG emissions (−251 kg CO2-eq/t DS) by the avoidance of heat and fuels. Barry et al. (Barry et al., 2019) assessed the GHG emissions in different application scenarios of biochar from sludge pyrolysis. The GHG emissions from incineration without energy recovery and incineration with organic Rankine cycle energy recovery ash were 646.98 and 238.28 kg CO2-eq/t DS, respectively. Agricultural applications and fuel utilization of biochar showed reduced global warming potential, with credit GHG emissions of −87.14 and −495.46 kg CO2-eq/t DS respectively. Agricultural land applications of biochar show a reduced potential for global warming. This is accomplished by recycling the energy from the pyrolysis process, reducing the need for fossil fuels, displacing mineral fertilizers, and carbon sequestration in the biochar. The use of biochar in cement kilns shows a significant reduction in global warming potential. This is partly due to the re-use of energy from the pyrolysis process, but primarily due to the replacement of lignite coal as a fuel. The GHG emission is about −495–500 kg CO2-eq/t DS (Hospido et al., 2005; Houdkova et al., 2008). This is achieved by recovering energy during pyrolysis, reducing the need for fossil fuels, replacing mineral fertilizers and sequestering carbon in biochar.
4.4 Incineration
In the sludge incineration process, the organic compounds of sludge are oxidated at elevated temperatures, and biomass energy is converted into heat and can be further converted into electricity, biosolids are burned to form mainly biological CO2 and water, leaving only inert material (ash). The calorific value of dried sewage sludge is the same as that of lignite, and it can be used for sludge drying, heat generation and power generation through energy recovery.
In the sludge incineration route, 52.5 kW h of electricity is consumed during the centrifuge dewatering of the sludge to 75% moisture content, and 31 kg/t DS diesel heavy oil is burned in the furnace to be used as an auxiliary fuel. 227–454 kW h of electricity and 12–24 kW h of heat can be recovered by sludge incineration (Tarpani et al., 2020).
The dewatered sludge is incinerated in fluidized bed boiler at 850°C, with the addition of fuel and lime to improve the combustion efficiency and to control acid gases, respectively. The main contributors of GHG emissions (39.8 kg CO2-eq/t DS) from sludge incineration are the CO2 emissions of heavy oil (108 kg CO2-eq/t DS) and credit emission from energy recovery (94% electricity and 6% heat) reduce the emissions (−147 kg CO2-eq/t DS) (Tarpani et al., 2020).
The GHG emission from thermal drying-incineration is 103 kg CO2-eq/t DS. Most of the heat generated by dried sludge incineration is used for thermal drying, and the main contribution of CO2 emission is transportation, as the moisture content of sludge after the belt filter press is 81.8%, the sludge reduction is not sufficient (Zhang et al., 2019). Compared to thermal, electro-dewatering consumes 223 kg CO2-eq/t DS, with a credit emission of −312 kg CO2-eq by displacing the fossil fuel. Therefore, the GHG emission from electro-dewatering-incineration is −32 kg CO2-eq/t DS.
Piippo et al. (Piippo et al., 2017) assessed the GHG emissions from incineration with mechanical drying and with thermal drying. The net GHG emission from incineration with mechanical drying is 1002.7 kg CO2-eq/t DS, and that from incineration with thermal drying is 924.01 kg CO2-eq/t DS. Regardless of biogenic CO2, the net GHG emissions from incineration with mechanical drying and thermal drying are −617.7 kg CO2-eq/t DS and −874.7 kg CO2-eq/t DS, respectively. Biological CO2 is mainly expelled during the combustion of sludge. Electricity consumption in the drying process and supplement fuel consumption are major contributors to abiotic CO2 GHG emissions from incineration with mechanical drying, while electricity and steam consumption are major contributors to abiotic CO2 GHG emissions from thermal drying. The avoided CO2 emissions from mechanical drying and thermal drying are −942.5 kg CO2-eq/t DS and −1,047 kg CO2-eq/t DS respectively. CO2 emissions from fossil fuels can be reduced by using dry sludge as fuel in power plants.
The GHG emission of sludge incineration is −617–670 kg CO2-eq/t DS (Houdkova et al., 2008; Hong et al., 2009; Lombardi et al., 2017), which is mainly affected by the sludge drying method and/or the addition amount of auxiliary fuel and energy recovery.
5 Future challenges of sludge diaposal
Energy and/or valuable elements in sludge can be recovered through composting, anaerobic digestion, pyrolysis, and incineration (Table 2). However, due to the fluctuating nature of sludge, the energy consumption and GHG emissions of the disposal routes vary widely. For example, the amount of energy recovered depends on the organic matter content, which directly affects the energy consumption and directly contributes to the GHG emissions throughout the disposal process.
When sludge is considered as a useful product, it denotes that sludge disposal needs to be integrated with water treatment strategy, i.e., the water treatment process produces two by-products, namely, sludge and “clean water.” In the context of carbon neutrality, future sludge treatment and disposal should be aimed at energy consercation and energy resource recovery. In large municipal WTTPs with large sludge production, the primary objective is to reduce the amount of sludge and maximize energy conversion efficiency. The focus should be on improving the efficiency of energy recovery and the output of high-quality products in medium and small-sized WTTPs.
With respect to the future challenges of sludge disposal, the current review summarizes the main points that emerge from the analysis.
1) In terms of the environment, reduce secondary waste generation and achieve environmentally sustainable operation of the disposal process based on ensuring harmless and efficient disposal. In the context of controlling carbon emissions, consideration of GHG emissions during disposal is also an essential aspect to reduce pollutant emissions during sludge disposal. While CO2 emissions from the sludge oxidation process are not counted in the inventory of GHG emissions, they need to be counted in the information reports of the energy sector and the waste disposal sector.
2) In terms of economics, a favorable compromise should be found between the resource recovery and material consumption to reduce the resource consumption (including electric energy, thermal energy, auxiliary fuel, etc.). Reduce the operational cost of the disposal process while achieving the disposal target. Local constraints from policies, geographic factors, economics, climate, etc. should be assessed, as well as the balance between the local applicability and cost of traditional and emerging treatment technologies.
3) At the societal level, sewage sludge used to grow food crops has strict systemic controls given the potential threat of pathogens and contaminant migration. This is essential for the protection of public health and safety. Timely development (revision) of standards for land use, drying and incineration, anaerobic digestion and co-processing of sludge. In addition to the formulation of a number of local sludge treatment and disposal standards, price subsidies and alternative mechanisms for sludge disposal and resource utilization will also be improved.
6 Conclusion
This study provides an overview of existing mainstream sludge treatment and disposal strategies, summarizes the characteristics of sewage sludge, and assesses the potential of converting sludge from waste to valuable products. The energy consumption and GHG emissions of sewage sludge treatment and disposal are analyzed through LCA practices, depending on the physicochemical properties, the specific process selection, and the associated cost to the environmental measures of most sludge management strategies. Variability in the LCA results reported by different studies weakens the comparability of results across studies, so most studies focus on comparing scenarios.
As a carbon-neutral biomass-based fuel, sludge has properties that distinguish it from other biomass fuels and fossil fuels, namely, excess ash, elevated volatile and low fixed carbon content. Moreover, its extreme moisture content in raw sludge makes it necessary to consume electrical or thermal energy for drying before it can be used as fuel. At the same time, due to its richness in N, P, and K, sludge can be applied as a raw material for the production of agricultural fertilizers. As a result, energy or nutrient recovery and comparison of conventional or promising alternatives are the main drivers in most studies of LCA-based sludge management options. Sludge disposal systems generate associated added value while achieving reduced and harmless disposal.
The level of potential GHG emissions of existing sludge treatment and disposal routes is: composting > anaerobic digestion > pyrolysis > incineration. However, the actual emission can vary due to LCI differences. There are numerous factors that affect the results, for instance, the form of energy supplementation for treatment process (thermal or electrical energy), the option of the specific process flow, and the final disposal scheme of the products (fuels to produce electricity or heat, alternatives to fertilizers) and quality of the products. A common direction for future research should be to develop strategies that minimize environmental implications, including GHG emissions, to recover energy and/or resources from sludge while achieving sludge reduction and harmless sustainable operation.
Author contributions
SY, Data processing, Investigation, Writing—original draft. SD, Writing—review and editing. AZ, Investigation, Modifying and editing. XW, Project administration. HT, Supervision, Funding acquisition. All authors contributed to the article and approved the submitted version.
Funding
This work was supported by the National Key Research and Development Program of China (NO.2020YFC1910000).
Conflict of interest
The authors declare that the research was conducted in the absence of any commercial or financial relationships that could be construed as a potential conflict of interest.
Publisher’s note
All claims expressed in this article are solely those of the authors and do not necessarily represent those of their affiliated organizations, or those of the publisher, the editors and the reviewers. Any product that may be evaluated in this article, or claim that may be made by its manufacturer, is not guaranteed or endorsed by the publisher.
References
Agarwal, M., Tardio, J., and Venkata Mohan, S. (2015). Pyrolysis of activated sludge: Energy analysis and its technical feasibility. Bioresour. Technol. 178, 70–75. doi:10.1016/j.biortech.2014.09.134
Akhter, A., Hage-Ahmed, K., Soja, G., and Steinkellner, S. (2015). Compost and biochar alter mycorrhization, tomato root exudation, and development of Fusarium oxysporum f. sp. lycopersici. Front. Plant Sci. 6, 529. doi:10.3389/fpls.2015.00529
Barry, D., Barbiero, C., Briens, C., and Berruti, F. (2019). Pyrolysis as an economical and ecological treatment option for municipal sewage sludge. Biomass Bioenergy 122, 472–480. doi:10.1016/j.biombioe.2019.01.041
Bernard, S. S., Srinivasan, T., Suresh, G., Ivon Paul, A., Fowzan, K. M., and Kishore, V. A. (2020). Production of biogas from anaerobic digestion of vegetable waste and cow dung. Mater. Today Proc. 33, 1104–1106. doi:10.1016/j.matpr.2020.07.129
Bianchini, A., Bonfiglioli, L., Pellegrini, M., and Saccani, C. (2015). Sewage sludge drying process integration with a waste-to-energy power plant. Waste Manag. 42, 159–165. doi:10.1016/j.wasman.2015.04.020
Biswas, B. K., Inoue, K., Harada, H., Ohto, K., and Kawakita, H. (2009). Leaching of phosphorus from incinerated sewage sludge ash by means of acid extraction followed by adsorption on orange waste gel. J. Environ. Sci. 21 (12), 1753–1760. doi:10.1016/S1001-0742(08)62484-5
Bolobajev, J., Kattel, E., Viisimaa, M., Goi, A., Trapido, M., Tenno, T., et al. (2014). Reuse of ferric sludge as an iron source for the Fenton-based process in wastewater treatment. Chem. Eng. J. 255, 8–13. doi:10.1016/j.cej.2014.06.018
Cantinho, P., Matos, M., Trancoso, M., and Santos, M. (2016). Behaviour and fate of metals in urban wastewater treatment plants: A review. Int. J. Environ. Sci. Technol. 13, 359–386. doi:10.1007/s13762-015-0887-x
Cheng, X., Wei, C., Ke, X., Pan, J., Wei, G., Chen, Y., et al. (2022). Nationwide review of heavy metals in municipal sludge wastewater treatment plants in China: Sources, composition, accumulation and risk assessment. J. Hazard. Mater. 437, 129267. doi:10.1016/j.jhazmat.2022.129267
Choińska-Pulit, A., Sobolczyk-Bednarek, J., and Łaba, W. (2018). Optimization of copper, lead and cadmium biosorption onto newly isolated bacterium using a Box-Behnken design. Ecotoxicol. Environ. Saf. 149, 275–283. doi:10.1016/j.ecoenv.2017.12.008
Chojnacka, K. (2010). Biosorption and bioaccumulation the prospects for practical applications. Environ. Int. 36, 299–307. doi:10.1016/j.envint.2009.12.001
Cieślik, B. M., Namieśnik, J., and Konieczka, P. (2015). Review of sewage sludge management: Standards, regulations and analytical methods. J. Clean. Prod. 90, 1–15. doi:10.1016/j.jclepro.2014.11.031
Cui, H., Ninomiya, Y., Masui, M., Mizukoshi, H., Sakano, T., and Kanaoka, C. (2006). Fundamental behaviors in combustion of raw sewage sludge. Energy and Fuels 20 (1), 77–83. doi:10.1021/ef050188d
Dai, J., Xu, M., Chen, J., Yang, X., and Ke, Z. (2007). PCDD/F, PAH and heavy metals in the sewage sludge from six wastewater treatment plants in Beijing, China. Chemosphere 66 (2), 353–361. doi:10.1016/j.chemosphere.2006.04.072
Ding, A., Zhang, R., Ngo, H. H., He, X., Ma, J., Nan, J., et al. (2021). Life cycle assessment of sewage sludge treatment and disposal based on nutrient and energy recovery: A review. Sci. Total Environ. 769, 144451. doi:10.1016/j.scitotenv.2020.144451
Domínguez, A., Fernández, Y., Fidalgo, B., Pis, J. J., and Menéndez, J. A. (2008). Bio-syngas production with low concentrations of CO2 and CH4 from microwave-induced pyrolysis of wet and dried sewage sludge. Chemosphere 70 (3), 397–403. doi:10.1016/j.chemosphere.2007.06.075
Eurostat, S. O. E. U. (2022). Sewage sludge production and disposal. [Online]. Available: https://appsso.eurostat.ec.europa.eu/nui/show.do?dataset=env_ww_spd&lang=en.
Fang, Y.-K., Wang, H.-C., Fang, P.-H., Liang, B., Zheng, K., Sun, Q., et al. (2023). Life cycle assessment of integrated bioelectrochemical-constructed wetland system: Environmental sustainability and economic feasibility evaluation. Resour. Conservation Recycl. 189, 106740. doi:10.1016/j.resconrec.2022.106740
Finnveden, G., Hauschild, M. Z., Ekvall, T., Guinée, J., Heijungs, R., Hellweg, S., et al. (2009). Recent developments in life cycle assessment. J. Environ. Manage 91 (1), 1–21. doi:10.1016/j.jenvman.2009.06.018
Fransson, K., Perzon, M., Fröling, M., Mossakowska, A., and Svanström, M. (2008). Sewage sludge handling with phosphorus utilization – life cycle assessment of four alternatives. J. Clean. Prod. 16, 135–151. doi:10.1016/j.jclepro.2006.12.004
Frišták, V., Pipíška, M., and Soja, G. (2018). Pyrolysis treatment of sewage sludge: A promising way to produce phosphorus fertilizer. J. Clean. Prod. 172, 1772–1778. doi:10.1016/j.jclepro.2017.12.015
Ghodke, P. K., Sharma, A. K., Pandey, J. K., Chen, W.-H., Patel, A., and Ashokkumar, V. (2021). Pyrolysis of sewage sludge for sustainable biofuels and value-added biochar production. J. Environ. Manag. 298, 113450. doi:10.1016/j.jenvman.2021.113450
Godlee, F. (2006). An international standard for disclosure of clinical trial information. BMJ 332 (7550), 1107–1108. doi:10.1136/bmj.332.7550.1107
Gourdet, C., Girault, R., Berthault, S., Richard, M., Tosoni, J., and Pradel, M. (2016). In quest of environmental hotspots of sewage sludge treatment combining anaerobic digestion and mechanical dewatering: A life cycle assessment approach. J. Clean. Prod. 143, 1123–1136. doi:10.1016/j.jclepro.2016.12.007
Guo, B., Wang, Y., Zhou, H., and Hu, F. (2022). Can environmental tax reform promote carbon abatement of resource-based cities? Evidence from a quasi-natural experiment in China. Environ. Sci. Pollut. Res. 2022. doi:10.1007/s11356-022-23669-3
Hertwich, E. G., Mateles, S. F., Pease, W. S., and McKone, T. E. (2001). Human toxicity potentials for life-cycle assessment and toxics release inventory risk screening. Environ. Toxicol. Chem. 20 (4), 928–939. doi:10.1002/etc.5620200431
Hidaka, T., Wang, F., Sakurai, K., Tsumori, J., and Minamiyama, M. (2016). Anaerobic codigestion of grass and sewage sludge: Laboratory experiments and feasibility analysis. Water Environ. Res. a Res. Publ. Water Environ. Fed. 88, 2070–2081. doi:10.2175/106143016X14733681695320
Hong, J., Hong, J., Otaki, M., and Jolliet, O. (2009). Environmental and economic life cycle assessment for sewage sludge treatment processes in Japan. Waste Manag. 29 (2), 696–703. doi:10.1016/j.wasman.2008.03.026
Hospido, A., Moreira, M. T., Fernández-Couto, M., and Feijoo, G. (2004). Environmental performance of a municipal wastewater treatment plant. Int. J. Life Cycle Assess. 9 (4), 261. doi:10.1007/BF02978602
Hospido, A., Moreira, T., Martín, M., Rigola, M., and Feijoo, G. (2005). Environmental evaluation of different treatment processes for sludge from urban wastewater treatments: Anaerobic digestion versus thermal processes (10 pp). Int. J. Life Cycle Assess. 10 (5), 336–345. doi:10.1065/lca2005.05.210
Hossain, M. K., Strezov, V., Yin Chan, K., and Nelson, P. F. (2010). Agronomic properties of wastewater sludge biochar and bioavailability of metals in production of cherry tomato (Lycopersicon esculentum). Chemosphere 78 (9), 1167–1171. doi:10.1016/j.chemosphere.2010.01.009
Houdkova, L., Borán, J., Ucekaj, V., Elsä, , and Stehlík, P. (2008). Thermal processing of sewage sludge - II. Appl. Therm. Eng. 28, 2083–2088. doi:10.1016/j.applthermaleng.2008.04.005
Hreiz, R., Latifi, M. A., and Roche, N. (2015). Optimal design and operation of activated sludge processes: State-of-the-art. Chem. Eng. J. 281, 900–920. doi:10.1016/j.cej.2015.06.125
Huang, F., Wang, Z. H., Cai, Y. X., Chen, S. H., Tian, J. H., and Cai, K. Z. (2018). Heavy metal bioaccumulation and cation release by growing Bacillus cereus RC-1 under culture conditions. Ecotoxicol. Environ. Saf. 157, 216–226. doi:10.1016/j.ecoenv.2018.03.077
IPCC (2000). Good practice guidance and uncertainty management in national greenhouse gas inventories. Switzerland: IPCC.
Kacprzak, M., Neczaj, E., Fijałkowski, K., Grobelak, A., Grosser, A., Worwag, M., et al. (2017). Sewage sludge disposal strategies for sustainable development. Environ. Res. 156, 39–46. doi:10.1016/j.envres.2017.03.010
Kan, T., Strezov, V., and Evans, T. (2016). Effect of the heating rate on the thermochemical behavior and biofuel properties of sewage sludge pyrolysis. Energy and Fuels 30 (3), 1564–1570. doi:10.1021/acs.energyfuels.5b02232
Karer, J., Wawra, A., Zehetner, F., Dunst, G., Wagner, M., Pavel, P.-B., et al. (2015). Effects of biochars and compost mixtures and inorganic additives on immobilisation of heavy metals in contaminated soils. Water, Air, and Soil Pollut. 226 (10), 342. doi:10.1007/s11270-015-2584-2
Komilis, D., Kissas, K., and Symeonidis, A. (2014). Effect of organic matter and moisture on the calorific value of solid wastes: An update of the Tanner diagram. Waste Manag. (New York, N.Y.) 34, 249–255. doi:10.1016/j.wasman.2013.09.023
Kosacka, A., Niedbała, G., Kolarzyk, P., and Ristvej, J. (2021). “Phosphorus recovery from sewage sludge,” in Advances in environmental engineering research in Poland (England,UK: Routledge).
Kumar, D., and Kumar, D. (2018). Water circuits design and tailings management. Sustain. Manag. Coal Prep., 293–320. doi:10.1016/B978-0-12-812632-5.00014-8
Lee, C.-G., Alvarez, P. J. J., Kim, H.-G., Jeong, S., Lee, S., Lee, K. B., et al. (2018). Phosphorous recovery from sewage sludge using calcium silicate hydrates. Chemosphere 193, 1087–1093. doi:10.1016/j.chemosphere.2017.11.129
Lee, Y., Sethurajan, M., van de Vossenberg, J., Meers, E., and van Hullebusch, E. D. (2020). Recovery of phosphorus from municipal wastewater treatment sludge through bioleaching using Acidithiobacillus thiooxidans. J. Environ. Manag. 270, 110818. doi:10.1016/j.jenvman.2020.110818
Li, B., Boiarkina, I., Yu, W., Huang, H. M., Munir, T., Wang, G. Q., et al. (2019). Phosphorous recovery through struvite crystallization: Challenges for future design. Sci. Total Environ. 648, 1244–1256. doi:10.1016/j.scitotenv.2018.07.166
Li, H., and Feng, K. (2018). Life cycle assessment of the environmental impacts and energy efficiency of an integration of sludge anaerobic digestion and pyrolysis. J. Clean. Prod. 195, 476–485. doi:10.1016/j.jclepro.2018.05.259
Li, H., Jin, C., and Mundree, S. (2017). Hybrid environmental and economic assessment of four approaches recovering energy from sludge with variant organic contents. J. Clean. Prod. 153, 131–138. doi:10.1016/j.jclepro.2017.03.167
Liang, Y., Xu, D., Feng, P., Guo, Y., and Wang, S. (2021). Municipal sewage sludge incineration and its air pollution control. J. Clean. Prod. 295, 126456. doi:10.1016/j.jclepro.2021.126456
Lin, Y.-P., Wang, W.-H., Pan, S.-Y., Ho, C.-C., Hou, C.-J., and Chiang, P.-C. (2016). Environmental impacts and benefits of organic Rankine cycle power generation technology and wood pellet fuel exemplified by electric arc furnace steel industry. Appl. Energy 183, 369–379. doi:10.1016/j.apenergy.2016.08.183
Linyi, C., Yujie, Q., Buqing, C., Chenglong, W., Shaohong, Z., Renglu, C., et al. (2020). Enhancing degradation and biogas production during anaerobic digestion of food waste using alkali pretreatment. Environ. Res. 188, 109743. doi:10.1016/j.envres.2020.109743
Lishan, X., Tao, L., Yin, W., Zhilong, Y., and Jiangfu, L. (2018). Comparative life cycle assessment of sludge management: A case study of xiamen, China. J. Clean. Prod. 192, 354–363. doi:10.1016/j.jclepro.2018.04.171
Liu, Z., Singer, S., Zitomer, D., and McNamara, P. J. (2017). Can autocatalytic pyrolysis of wastewater biosolids be energy neutral and generate value-added products? Proc. Water Environ. Fed. 2017, 360. doi:10.2175/193864717821496392
Lombardi, L., Nocita, C., Bettazzi, E., Fibbi, D., and Carnevale, E. (2017). Environmental comparison of alternative treatments for sewage sludge: An Italian case study. Waste Manag. 69, 365–376. doi:10.1016/j.wasman.2017.08.040
Lu, J. (2019). “Carbon footprint and reduction potential of Chinese wastewater treatment sector,” (Beijing, China: University of Chinese Academy of Sciences). Master.
Ma, J., Zhang, L., and Li, A. (2016). Energy-efficient co-biodrying of dewatered sludge and food waste: Synergistic enhancement and variables investigation. Waste Manag. 56, 411–422. doi:10.1016/j.wasman.2016.06.007
Mehta, C., Khunjar, W., Nguyen, V., Tait, S., and Batstone, D. (2015). Technologies to recover nutrients from waste streams: A critical review. Crit. Rev. Environ. Sci. Technol. 45, 385–427. doi:10.1080/10643389.2013.866621
Murray, A., Horvath, A., and Nelson, K. (2008). Hybrid life-cycle environmental and cost inventory of sewage sludge treatment and end-use scenarios: A case study from China. Environ. Sci. Technol. 42, 3163–3169. doi:10.1021/es702256w
Murwanashyaka, J. N., Pakdel, H., and Roy, C. (2001). Step-wise and one-step vacuum pyrolysis of birch-derived biomass to monitor the evolution of phenols. J. Anal. Appl. Pyrolysis 60 (2), 219–231. doi:10.1016/S0165-2370(00)00206-0
Nakatsuka, N., Kishita, Y., Kurafuchi, T., and Akamatsu, F. (2020). Integrating wastewater treatment and incineration plants for energy-efficient urban biomass utilization: A life cycle analysis. J. Clean. Prod. 243, 118448. doi:10.1016/j.jclepro.2019.118448
Nguyen, T.-B., and Shima, K. (2019). Composting of sewage sludge with a simple aeration method and its utilization as a soil fertilizer. Environ. Manag. 63 (4), 455–465. doi:10.1007/s00267-017-0963-8
Pagnanelli, F., Mainelli, S., Bornoroni, L., Dionisi, D., and Toro, L. (2009). Mechanisms of heavy-metal removal by activated sludge. Chemosphere 75 (8), 1028–1034. doi:10.1016/j.chemosphere.2009.01.043
Pathak, A., Dastidar, M. G., and Sreekrishnan, T. R. (2009). Bioleaching of heavy metals from sewage sludge: A review. J. Environ. Manag. 90 (8), 2343–2353. doi:10.1016/j.jenvman.2008.11.005
Peters, G., and Rowley, H. (2009). Environmental comparison of biosolids management systems using life cycle assessment. Environ. Sci. Technol. 43, 2674–2679. doi:10.1021/es802677t
Piippo, S., Lauronen, M., and Postila, H. (2017). Greenhouse gas emissions from different sewage sludge treatment methods in north. J. Clean. Prod. 177, 483–492. doi:10.1016/j.jclepro.2017.12.232
Pradel, M., Aissani, L., Villot, J., Baudez, J.-C., and Laforest, V. (2016). From waste to added value product: Towards a paradigm shift in life cycle assessment applied to wastewater sludge – a review. J. Clean. Prod. 131, 60–75. doi:10.1016/j.jclepro.2016.05.076
Qin, H., Tianjue, H., Zhai, Y., Lu, N., and Aliyeva, J. (2019). The improved methods of heavy metals removal by biosorbents: A review. Environ. Pollut. (Barking, Essex 1987) 258, 113777. doi:10.1016/j.envpol.2019.113777
Raja, S., and Alphin, M. S. (2020). Systematic effects of Fe doping on the activity of V2O5/TiO2-carbon nanotube catalyst for NH3-SCR of NOx</sub>. J. Nanoparticle Res. Interdiscip. Forum Nanoscale Sci. Technol. 22 (7), 190. doi:10.1007/s11051-020-04919-2
Rajec, P., Rosskopfová, O., Galamboš, M., Frišták, V., Soja, G., Dafnomili, A., et al. (2016). Sorption and desorption of pertechnetate on biochar under static batch and dynamic conditions. J. Radioanalytical Nucl. Chem. 310 (1), 253–261. doi:10.1007/s10967-016-4811-8
Sablayrolles, C., Gabrielle, B., and Montrejaud-Vignoles, M. (2010). Life cycle assessment of biosolids land application and evaluation of the factors impacting human toxicity through plant uptake. J. Industrial Ecol. 14 (2), 231–241. doi:10.1111/j.1530-9290.2010.00222.x
Shaddel, S., Bakhtiary-Davijany, H., Kabbe, C., Dadgar, F., and Østerhus, S. W. (2019). Sustainable sewage sludge management: From current practices to emerging nutrient recovery technologies. Sustainability 11 (12), 3435. doi:10.3390/su11123435
Sheng, X., Wang, J., Cui, Q., Zhang, W., and Zhu, X. (2022). A feasible biochar derived from biogas residue and its application in the efficient adsorption of tetracycline from an aqueous solution. Environ. Res. 207, 112175. doi:10.1016/j.envres.2021.112175
Singh, R. P., Singh, P., Araujo, A. S. F., Hakimi Ibrahim, M., and Sulaiman, O. (2011). Management of urban solid waste: Vermicomposting a sustainable option. Resour. Conservation Recycl. 55 (7), 719–729. doi:10.1016/j.resconrec.2011.02.005
Stefanakis, A., Akratos, C. S., and Tsihrintzis, V. A. (2014). General aspects of sludge management. Vertical Flow Constructed Wetlands, 181–189. doi:10.1016/b978-0-12-404612-2.00009-x
Syed-Hassan, S. S. A., Wang, Y., Hu, S., Su, S., and Xiang, J. (2017). Thermochemical processing of sewage sludge to energy and fuel: Fundamentals, challenges and considerations. Renew. Sustain. Energy Rev. 80, 888–913. doi:10.1016/j.rser.2017.05.262
Tarpani, R. R. Z., Alfonsín, C., Hospido, A., and Azapagic, A. (2020). Life cycle environmental impacts of sewage sludge treatment methods for resource recovery considering ecotoxicity of heavy metals and pharmaceutical and personal care products. J. Environ. Manag. 260, 109643. doi:10.1016/j.jenvman.2019.109643
Teoh, S. K., and Li, L. Y. (2020). Feasibility of alternative sewage sludge treatment methods from a lifecycle assessment (LCA) perspective. J. Clean. Prod. 247, 119495. doi:10.1016/j.jclepro.2019.119495
Tomasi Morgano, M., Leibold, H., Richter, F., Stapf, D., and Seifert, H. (2018). Screw pyrolysis technology for sewage sludge treatment. Waste Manag. 73, 487–495. doi:10.1016/j.wasman.2017.05.049
Tyagi, V. K., and Lo, S. L. (2016). “Chapter 10 - energy and resource recovery from sludge: Full-scale experiences,” in Environmental materials and waste. Editors M. N. V. Prasad, and K. Shih (United States: Academic Press), 221–244.
Usman, M., Shi, Z.-J., Ren, S., Ngo, H., Luo, G., and Zhang, S. (2020). Hydrochar promoted anaerobic digestion of hydrothermal liquefaction wastewater: Focusing on the organic degradation and microbial community. Chem. Eng. J. 399, 125766. doi:10.1016/j.cej.2020.125766
Wang, X., Zhu, Y., Hu, Z., Zhang, L., Yang, S., Ruan, R., et al. (2020). Characteristics of ash and slag from four biomass-fired power plants: Ash/slag ratio, unburned carbon, leaching of major and trace elements. Energy Convers. Manag. 214, 112897. doi:10.1016/j.enconman.2020.112897
Wu, B., Dai, X., and Chai, X. (2020). Critical review on dewatering of sewage sludge: Influential mechanism, conditioning technologies and implications to sludge re-utilizations. Water Res. 180, 115912. doi:10.1016/j.watres.2020.115912
Xiong, S., Zhuo, J., Zhang, B., and Yao, Q. (2013). Effect of moisture content on the characterization of products from the pyrolysis of sewage sludge. J. Anal. Appl. Pyrolysis 104, 632–639. doi:10.1016/j.jaap.2013.05.003
Xu, Y., Liu, R., Yang, D., and Dai, X. (2021). Sludge treatment and resource recovery towards carbon neutrality in China: Current status and future perspective. Blue-Green Syst. 3 (1), 119–127. doi:10.2166/bgs.2021.115
Yan, B., Liu, C., Xu, S., Zhou, J., Wong, J., and Zhang, J. (2021). Bioconversion technologies: Anaerobic digestion of food waste. Curr. dev. Biotechnol. Bioeng., 163–204. doi:10.1016/B978-0-12-819148-4.00006-3
Yang, W., Song, W., Li, J., and Zhang, X. (2020). Bioleaching of heavy metals from wastewater sludge with the aim of land application. Chemosphere 249, 126134. doi:10.1016/j.chemosphere.2020.126134
Yoshida, H., Christensen, T. H., and Scheutz, C. (2013). Life cycle assessment of sewage sludge management: A review. Waste Manag. Res. 31 (11), 1083–1101. doi:10.1177/0734242x13504446
Yu, D., Guo, J., Meng, J., and Sun, T. (2023a). Biofuel production by hydro-thermal liquefaction of municipal solid waste: Process characterization and optimization. Chemosphere 328, 138606. doi:10.1016/j.chemosphere.2023.138606
Yu, D., Wan, X., and Gu, B. (2023b). Bi-objective optimization of biomass solid waste energy system with a solid oxide fuel cell. Chemosphere 323, 138182. doi:10.1016/j.chemosphere.2023.138182
Zamparas, M. (2021). The role of resource recovery technologies in reducing the demand of fossil fuels and conventional fossil-based mineral fertilizers. Low Carbon Energy Technol. Sustain. Energy Syst., 3–24. doi:10.1016/B978-0-12-822897-5.00001-8
Zhai, Y., Peng, W., Zeng, G., Fu, Z., Lan, Y., Chen, H., et al. (2012). Pyrolysis characteristics and kinetics of sewage sludge for different sizes and heating rates. J. Therm. Analysis Calorim. 107, 1015–1022. doi:10.1007/s10973-011-1644-0
Zhang, H., Rigamonti, L., Visigalli, S., Turolla, A., Gronchi, P., and Canziani, R. (2019). Environmental and economic assessment of electro-dewatering application to sewage sludge: A case study of an Italian wastewater treatment plant. J. Clean. Prod. 210, 1180–1192. doi:10.1016/j.jclepro.2018.11.044
Zhang, L.-H., Li, Z., Yang, S.-M., and Duan, F. (2021). Dried sludge reburning blended with calcium magnesium acetate addition in a fluidized bed combustor. Waste Manag. 123, 120–130. doi:10.1016/j.wasman.2021.01.028
Zhang, L., Duan, F., and Huang, Y. (2015a). Effect of organic calcium compounds on combustion characteristics of rice husk, sewage sludge, and bituminous coal: Thermogravimetric investigation. Bioresour. Technol. 181, 62–71. doi:10.1016/j.biortech.2015.01.041
Zhang, L., Xu, C. C., Champagne, P., and Mabee, W. (2014). Overview of current biological and thermo-chemical treatment technologies for sustainable sludge management. Waste Manag. Res. 32 (7), 586–600. doi:10.1177/0734242x14538303
Zhang, Y., Zhang, L., Duan, F., Jiang, X., Sun, X., and Chyang, C. (2015b). Co-combustion characteristics of sewage sludge with different rank bituminous coals under the O2/CO2 atmosphere. J. Therm. Analysis Calorim. 121 (2), 729–736. doi:10.1007/s10973-015-4582-4
Zhao, L., Du, M., Du, W., Guo, J., Liao, Z., Kang, X., et al. (2022). Evaluation of the carbon sink capacity of the proposed kunlun mountain national park. Int. J. Environ. Res. Public Health 19 (16), 9887. doi:10.3390/ijerph19169887
Keywords: sewage sludge, LCA, energy consumption, GHG emission, disposal
Citation: Yu S, Deng S, Zhou A, Wang X and Tan H (2023) Life cycle assessment of energy consumption and GHG emission for sewage sludge treatment and disposal: a review. Front. Energy Res. 11:1123972. doi: 10.3389/fenrg.2023.1123972
Received: 14 December 2022; Accepted: 31 May 2023;
Published: 09 June 2023.
Edited by:
ZhaoYang Dong, Nanyang Technological University, SingaporeReviewed by:
Hari Sharma, Sikkim Manipal University, IndiaMuslum Demir, Osmaniye Korkut Ata University, Türkiye
Mohamed Salem, University of Science Malaysia (USM), Malaysia
Copyright © 2023 Yu, Deng, Zhou, Wang and Tan. This is an open-access article distributed under the terms of the Creative Commons Attribution License (CC BY). The use, distribution or reproduction in other forums is permitted, provided the original author(s) and the copyright owner(s) are credited and that the original publication in this journal is cited, in accordance with accepted academic practice. No use, distribution or reproduction is permitted which does not comply with these terms.
*Correspondence: Houzhang Tan, hzt@xjtu.edu.cn