- 1Guang'anmen Hospital, China Academy of Chinese Medical Sciences, Beijing, China
- 2The Second People's Hospital of Jiaozuo, Jiaozuo, China
- 3Department of Breast Surgery, Xingtai People's Hospital, Xingtai, China
- 4Beijing University of Chinese Medicine, Beijing, China
- 5The First Clinical Medical College of Shaanxi University of Chinese Medicine, Taiyuan, China
As a result of ongoing breakthroughs in cancer therapy, cancer patients' survival rates have grown considerably. However, cardiotoxicity has emerged as the most dangerous toxic side effect of cancer treatment, negatively impacting cancer patients' prognosis. In recent years, the link between non-coding RNAs (ncRNAs) and cancer therapy-induced cardiotoxicity has received much attention and investigation. NcRNAs are non-protein-coding RNAs that impact gene expression post-transcriptionally. They include microRNAs (miRNAs), long non-coding RNAs (lncRNAs), and circular RNAs (circRNAs). In several cancer treatments, such as chemotherapy, radiotherapy, and targeted therapy-induced cardiotoxicity, ncRNAs play a significant role in the onset and progression of cardiotoxicity. This review focuses on the mechanisms of ncRNAs in cancer therapy-induced cardiotoxicity, including apoptosis, mitochondrial damage, oxidative stress, DNA damage, inflammation, autophagy, aging, calcium homeostasis, vascular homeostasis, and fibrosis. In addition, this review explores potential ncRNAs-based biomarkers and therapeutic strategies, which may help to convert ncRNAs research into clinical practice in the future for early detection and improvement of cancer therapy-induced cardiotoxicity.
Introduction
In the last decade, significant advancements in cancer treatment have been made, including anthracycline chemotherapy, molecular targeted therapy, and radiotherapy, which have considerably improved the survival rates of cancer patients, lengthened survival time, and enhanced their quality of life. The National Cancer Institute estimates that at least 16.9 million cancer survivors will be alive in the US in 2019 and that the number will be nearly 22.1 million by 2030 (1). Cardiovascular hazards connected with cancer treatment, such as arrhythmia, arterial hypertension, thromboembolic ischemia, myocardial fibrosis, and heart failure, are growing as cancer treatment improves (2, 3). Cardiotoxicity is one of the primary causes of morbidity and death in patients with cancer, posing a severe danger to the treatment's lengthy effectiveness and life quality (4). An observational study comparing the general US population with 3,234,256 US cancer survivors (1973–2012) found that 38.0% of patients died from cancer and 11.3% died from cardiovascular disease (CVD) (5). Furthermore, patients with cancer from diagnosis to surviving cancer (all sites) had a higher risk of dying from CVD compared to the general US population. The current diagnosis and management of cancer therapy-induced cardiotoxicity are hampered by several factors. The link between cancer therapy and cardiotoxicity is complex, especially due to our incomplete understanding of the underlying pathogenesis, resulting in a lack of effective and specific early diagnosis methods and treatment strategies. As a result, it is vital to understand the pathophysiology and underlying molecular processes of cancer therapy-induced cardiotoxicity, so that novel diagnostics and therapeutic targets may be developed.
Non-coding RNAs (ncRNAs) are a class of genetic, epigenetic, and translational regulators, mainly composed of microRNAs (miRNAs), long non-coding RNAs (lncRNAs), and circular RNAs (circRNAs) (6) (Figure 1). MiRNAs are short ncRNAs with a length of 19–24 nucleotides that regulate post-transcriptional gene expression via sticking to the 3′ untranslated region (3′-UTR) of target mRNAs and restricting their stability and translation, thus playing a role in a variety of physiological processes including cell development, cell death, proliferation, and signaling (7, 8). Unlike miRNAs, lncRNAs are ncRNAs over 200 nucleotides in length with mRNA-like structures that can control the structure and transcription of the nucleus and influence mRNA stability or serve as competing endogenous RNAs (ceRNAs) interact with miRNAs to regulate mRNA translation (9). CircRNAs are newly discovered ncRNAs whose 5′ and 3′ ends are covalently closed by a back-splicing reaction and have been shown to exert their functions through a variety of mechanisms, including miRNA sponges, protein interactions, protein translation, and parental gene control (10). Because of their important regulatory roles in multiple biological processes of disease development, ncRNAs have great potential as biomarkers and therapeutic targets. Accumulating evidence suggests that ncRNAs have a critical role in the occurrence and development of cardiotoxicity generated by cancer treatment, as well as in pathological conditions such as oxidative damage, mitochondrial damage, apoptosis, dysregulation of calcium homeostasis, and dysregulation of vascular homeostasis (11–13). Therefore, this review summarizes the mechanisms of three ncRNAs (miRNAs, lncRNAs, and circRNAs) in cancer therapy-induced cardiotoxicity and discusses their diagnostic and therapeutic potential in this disease.
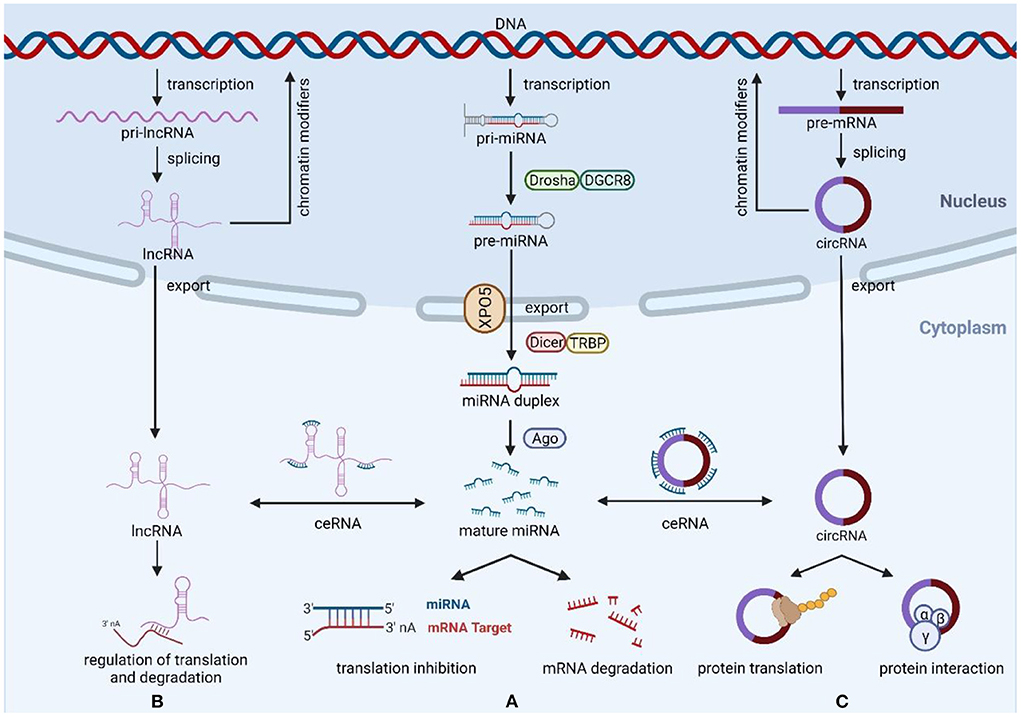
Figure 1. Biogenesis and function of miRNAs, lncRNAs, and circRNAs. (A) The ncRNAs genes are transcribed to generate pri-miRNAs, Drosha, and DGCR8 process pri-RNAs to pre-miRNA, then export the pre-miRNAs from nucleus to cytoplasm via exportin-5, and generate miRNA duplexes by Dicer and TRBP processing. Mature miRNAs regulate the expression of target mRNAs through degradation or translational repression. (B) Most transcribed lncRNAs are polyadenylated at 3′, 5′ capping and splicing. LncRNAs exert their functions mainly through three mechanisms of action, including miRNA spongeization, regulation of translation and degradation, and modifiers of parental gene expression. (C) CircRNAs are formed by back-splicing of pre-mRNA, and their main functions include miRNA sponges, protein interactions, protein translation, and regulation of parental genes. Created with BioRender.com.
ncRNAs in cancer therapy-induced cardiotoxicity
There is increasing evidence that the dysregulation of ncRNAs is associated with cancer therapy-induced cardiotoxicity and plays a crucial role by affecting apoptosis, mitochondrial damage, oxidative stress, inflammation, autophagy, calcium homeostasis, vascular homeostasi, fibrosis, etc. (Figure 2). The molecular mechanisms and functional importance of known ncRNAs implicated in cancer therapy-induced cardiotoxicity are summarized in Table 1.
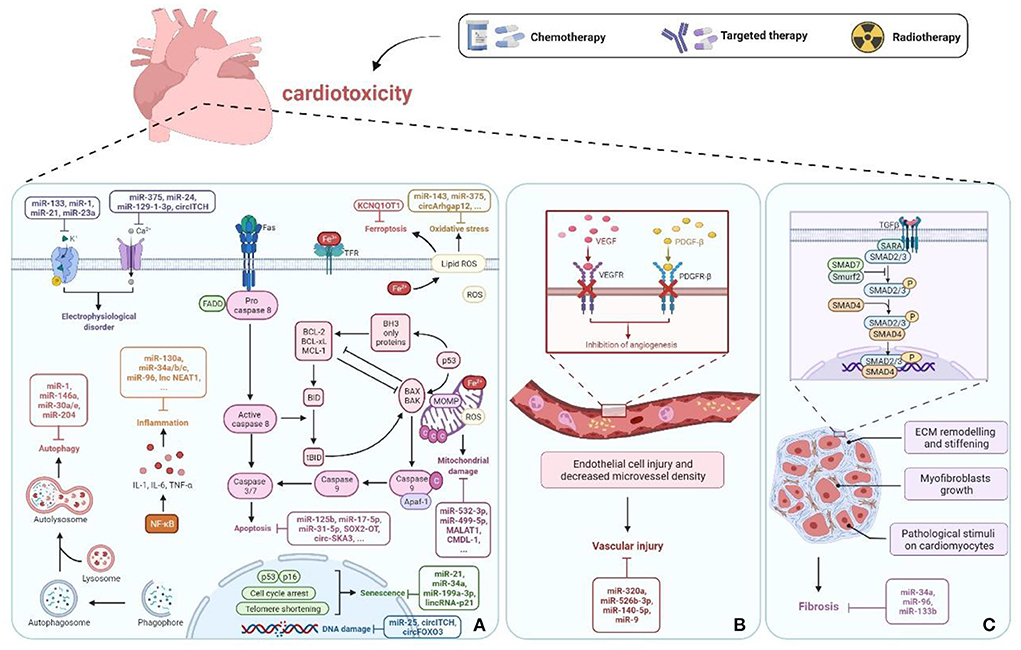
Figure 2. ncRNAs in cancer therapy-induced cardiotoxicity. (A) ncRNAs cause cell damage or death through regulation of apoptosis, mitochondrial damage, oxidative stress, DNA damage, inflammation, autophagy, senescence, electrophysiological disorders, and ferroptosis pathways. (B) Vascular homeostasis disorder. (C) Fibrosis. Created with BioRender.com.
Anthracyclines
Doxorubicin (DOX), pirarubicin (THP), and epirubicin (EPI) are among the most often used anthracyclines, which are used to treat breast cancer (BC), gastric cancer, acute lymphoblastic leukemia, and lymphoma. However, anthracyclines' clinical use is restricted due to their harmful effects on normal tissues, particularly cardiotoxicity. To date, a large number of studies have confirmed that ncRNAs are abnormally expressed and participate in anthracycline-induced cardiotoxicity by regulating molecular signaling pathways. However, where miRNAs have been extensively studied, lncRNAs and circRNAs are still in their infancy. In-depth knowledge of how these ncRNAs contribute to anthracycline-induced cardiotoxicity will help to identify novel biomarkers or therapeutic targets and provide a more thorough theoretical foundation and evidence base for clinical applications.
miRNA
Apoptosis
Apoptosis, which is separated into exogenous and endogenous apoptosis, is the best researched programmed cell death route (95). The Fas-involved pathway, which triggers caspase-8, is primarily responsible for exogenous apoptosis. Endogenous apoptosis, on the other hand, is mainly associated with the proapoptotic proteins Bax/Bak of the Bcl-2 protein family, inducing mitochondrial outer membrane permeability and cytochrome c release. Both pathways activate the caspase cascade, which results in cardiomyocyte death. The endogenous apoptotic pathway is currently the most studied in ncRNA- and anthracycline-induced cardiotoxicity. In DOX-treated rat myocardial, for example, miR-34a-5p was upregulated, enhancing Bax expression and mitochondrial depolarization while inhibiting Bcl-2 expression (20). Sirt1, which has miR-34a-5p binding sites in its 3′-UTR, is its downstream mediator. Sirt1 is a NAD-dependent deacetylase that affects mitochondrial function, apoptosis, and inflammatory responses (96, 97). After DOX therapy, Sirt1 expression declined, and its target gene P66shc was negatively regulated, enhancing apoptosis produced by the p66shc-mediated mitochondrial death pathway. As a result, activation of the Sirt1/p66shc pathway is responsible for miR-34a-5p's influence on cardiomyocyte apoptosis. Interestingly, the activation of the NF-κB/p65 signaling pathway was discovered to promote the elevation of miR-34a-5p in DOX-treated myocardial cells, which suggests that the upstream mechanism of miR-34a-5p has to be further researched in the future.
Cardiomyocyte atrophy and apoptosis were increased in DOX-induced human-induced pluripotent stem cell-derived cardiomyocytes (hiPSC-CMs) and primary neonatal rat cardiomyocytes, while upregulation of the miR-212/132 cluster protected against DOX-treated atrophy and apoptosis (19). Upregulation of the miR-212/132 cluster by the adeno-associated virus (AAV) 9 improved cardiac ejection fraction and myocardial wall thickness in DOX mice, alleviating cardiac dysfunction. Overexpression of Fitm2, a downstream target of the pro-hypertrophic miR-212/132 family, partially reversed the anti-apoptotic and anti-atrophic functions of the miR-212/132 cluster, which has been identified as localized in the endoplasmic reticulum and implicated in lipid droplet production. According to a recent study, miR-125b was elevated in DOX-caused cardiomyocytes, and miR-125b suppression diminished apoptosis and ameliorated myocardial damage (16). In DOX-treated cardiomyocytes, miR-125b was increased, and miR-125b inhibition reduced apoptosis and improved cardiac damage. STARD13 was a straight target of miR-125b, which could inhibit the nucleocytoplasmic translocation of YesaSociated protein (YAP) by targeting STARD13. Therefore, miR-125b can play a proapoptotic role in DOX-caused cardiotoxicity by modulating the STARD13/YAP axis, whereas the exact molecular mechanism remains unclear. This study also showed that mesenchymal stem cell-derived small extracellular vesicles (MSC-sEVs) protect the heart against DOX-induced apoptosis, with miR-199a-3p playing a significant role (14). Through the Akt-Sp1/p53 signal pathway, miR-199a-3p in MSC-sEVs upregulated the production of anti-apoptotic proteins survivin and Bcl-2, inhibiting DOX-induced cardiomyocyte apoptosis and improving cardiac contractile function.
Mitochondrial damage
Mitochondria are the prominent organelles for DOX-induced cardiomyocyte injury (98). DOX can remain in the mitochondrial inner membrane, creating an irreversible binding with cardiolipin and destroying the normal structure and function of mitochondria. To sustain proper metabolism, the mitochondrial structure undergoes continual fusion and fission, and abnormal mitochondrial fission causes cardiomyocyte death (99, 100). DOX therapy, for example, induced mitochondrial fission and apoptosis in cardiomyocytes via upregulating miR-532-3p and adversely controlling the translation of apoptosis repressor with caspase recruitment domain (ARC) in a time-dependent way (40). Overexpression of ARC, on the other hand, prevented DOX-induced mitochondrial fission, reducing cardiomyocyte death. Similarly, miR-499-5p overexpression lowered DOX cardiotoxicity by silencing p21 and inhibiting mitochondrial fission and cell death in myocardial cells (41). Nevertheless, neither of these studies delved into the specific molecular mechanisms by which ARC or p21 control mitochondrial fission.
DOX-related mitochondrial dysfunction is characterized by the loss of mitochondrial membrane permeability and cytochrome c release (101). The cationic drug DOX binds to the negatively charged phospholipids in the inner mitochondrial membrane to form an irreversible DOX cardiolipin complex, which disrupts the electron transport chain (98). Decreased free cardiolipin leads to the detachment of cytochrome c from the mitochondrial membrane, activating caspase-dependent death. In addition, the proapoptotic protein Bax is overexpressed after DOX treatment, and high levels of Bax protein affect mitochondrial membrane permeability by binding to porins on the mitochondrial membrane, promoting mitochondrial release of apoptotic protein cytochrome c, thereby inducing caspase-dependent apoptosis (102, 103). For instance, miR-29b was downregulated in DOX-treated cardiomyocytes and inversely impacted Bax transcription via specifically attacking the 3′-UTR of Bax (39). MiR-29b agomir inhibited cytochrome c release, mitochondrial membrane depolarization, and caspase activation while decreasing the proapoptotic protein Bax, lowering mitochondria-dependent apoptotic pathways, and ameliorating DOX-induced cardiac injury. Another study showed that miR-23a and p-Drp1 were significantly increased and PGC-1α was considerably decreased in DOX-treated cardiomyocytes (38). Previous studies have demonstrated that miR-23a directly reduces cardiomyocyte PGC-1α levels by binding to the 3′-UTR of PGC-1α, which can inhibit DOX-induced mitochondrial dysfunction (104, 105). Furthermore, Drp1 is a dynamin-related protein that mediates the mitochondrial outer membrane fission and regulates mitochondrial dynamics (106). MiR-23a inhibitor restored PGC-1α and p-Drp1 levels, which in turn improved mitochondrial membrane potential and inhibited oxidative stress and mitochondria-dependent apoptosis pathways. In contrast, PGC-1α siRNA silencing eliminated the effect of miR-23a inhibitors on PGC-1α and p-Drp1 and the protective effect on cardiomyocytes. Taken together, the knockdown of miR-23a attenuated DOX-induced mitochondrial dysfunction by restoring the PGC-1α/p-Drp1 pathway. Furthermore, Bmpr1a might be a possible target of miR-15b-5p, a crucial regulator in maintaining cardiomyocyte integrity (37). By upregulating miR-15b-5p or suppressing Bmpr1a, DOX-induced oxidative stress, mitochondrial membrane potential damage, and apoptosis might be exacerbated.
Oxidative stress
The principal mechanism of anthracycline-induced cardiotoxicity has been considered oxidative stress mediated via excess reactive oxygen species (ROS). ROS comprises hydroxyl radical (·OH), singlet oxygen (1O2), superoxide anion (O2·-), and hydrogen peroxide (H2O2), whose excessive production causes lipid peroxidation and structural changes in biomolecules, disrupting the structural integrity of cell membranes and ultimately leading to cardiomyocyte death (107). MiRNA expression is upregulated or suppressed in anthracycline-treated cardiomyocytes, affecting the expression level of their target gene proteins and participating in anthracycline cardiotoxicity owing to oxidative damage, according to several studies. For example, the expression of miR-143 was upregulated after DOX treatment, and miR-143 induced myocardial injury by inhibiting AKT signaling pathway to increase oxidative damage and apoptosis (32). DOX-caused apoptosis and oxidative stress have been demonstrated to be inhibited by activating the AKT signal pathway in previous research (108). Inhibition of miR-143 or activation of AKT reversed DOX-induced myocardial injury. However, the detailed molecular mechanism through which miR-143 and AKT interact is unknown. Conversely, another study confirmed that miR-375 exerted a similar effect by directly targeting 3-phosphoinositide-dependent kinase 1 (PDK1), activating the AKT signaling path (29).
Furthermore, miRNAs have been identified as downstream targets in controlling cardiotoxicity generated by DOX. Nucleolin is a downstream target of miRNA-21, the most numerous RNA-binding protein (RBP) in the nucleolus (56). Following the DOX treatment, the translation of nucleolar protein elevated, as did the expression of miRNA-21, which in turn inhibited ROS generation and lipid peroxidation, thus shielding cardiomyocytes from DOX-induced oxidative damage. This protection could be reversed by knocking down the nucleolar protein. THP-induced cardiotoxicity has been demonstrated to be ameliorated by rutin (RUT), an essential dietary flavonoid (66). After THP treatment, miR-125b-1-3p was upregulated, the JunD expression level decreased, and ROS and apoptosis levels increased in HL-1 cells. JunD, a member of the activator protein-1 transcription factor family, is a key regulator of oxidative stress levels (109). By GO enrichment analysis and TargetScan database screening, a potential miR-125b-1-3p-binding site was found on the 3′-UTR of JunD mRNA. MiR-125b-1-3p may decrease JunD by direct targeting, increasing THP-induced oxidative damage and apoptosis. In contrast, RUT can reverse this effect and thus attenuate THP damage to cardiomyocytes. RUT may also prevent THP-caused cell death and oxidative damage by boosting miR-22-5p expression and blocking the Rap1a-mediated RAP1/ERK signaling pathway, according to a recent study (65).
DNA damage
The DNA damage occurs early in DOX-caused cardiac cell death, which might be attributable to direct or indirect ROS generation (110, 111). MiR-25 was shown to be dose-dependently upregulated following DOX treatment, accompanied by an increase in ROS and γ-H2AX (a DNA damage marker) (42). PTEN is a dual lipid/protein phosphatase that has previously been implicated in significantly impacting DNA repair (112). Upregulation of miR-25 causes cardiomyocyte death by interacting with the PTEN 3′-UTR and lowering PTEN levels, resulting in increased ROS generation and DNA damage. Inhibition of miR-25 can restore the expression of PTEN, alleviate DOX-induced cardiotoxicity, and does not affect the anticancer ability of DOX, suggesting that this might be a future therapeutic option for DOX-generated cardiotoxicity.
Inflammation
As shown by the increases in proinflammatory cytokines, inflammation response is assumed to be intimately linked to DOX-induced cardiotoxicity (113). Nuclear factor κB (NF-κB), a crucial determinant in the management of inflammation, secretes a variety of proinflammatory cytokines, including interleukin-6 (IL-6), interleukin-1 (IL-1), and tumor necrosis factor alpha (TNF-α) (114). The expression of miR-130a, for example, was dramatically enhanced following DOX therapy and was inversely linked with the expression of PPARγ (46). PPARγ was shown to upregulate the anti-apoptotic protein BCL2, prevent apoptosis, and block NF-κB-mediated cardiomyocyte enlargement, all of which are significant in anti-apoptosis and inflammation (115, 116). Inhibition of miR-130a improved DOX-induced inflammatory response and apoptosis by restoring PPARγ expression, which in turn enhanced BCL2 and lowered NF-κB. MiR-34a,−34b, and−34c are three highly homologous miRNAs that make up the miRNA-34 family. Further research revealed that miR-34b/c increased the secretion of proinflammatory cytokines and aggravated the inflammatory reaction via ITCH/NF-κB signaling pathway (47). MiR-34b/c antagomir was targeted to inhibit the expression of miR-34b/c, lower inflammatory cytokine translation, and reverse the cardiotoxicity generated by DOX. AntimiR-34a targeted the inhibition of miR-34a, upregulated Bcl-2 and SIRT1 expression, reduced acetylation levels of p53, SMAD2/3, and NF-κB, as well as reduced inflammatory response, senescence, fibrosis, and apoptosis (51). Furthermore, upregulation of miR-96 alleviated DOX-generated cardiotoxicity via blocking the Rac1/NF-κB signal pathway as well as attenuating oxidative stress, inflammatory response, and fibrosis (64).
Inflammation and accumulation of ROS activate caspase-3 and aggravate cell death (101). DOX inhibited the expression of miR-425 and increased the levels of IL-1β, IL-6, TNF-α, and ROS in cardiomyocytes (45). RIPK1 is a Ser/Thr kinase containing a death domain that controls inflammatory signaling and the activation of multiple cell death pathways (117). RIPK1 was found to be a direct target of miR-425 with a miR-425-binding site on its 3′-UTR. MiR-425 overexpression protected cardiomyocytes from DOX-induced inflammation, oxidative stress, and apoptosis by reducing RIPK1 expression. Under normal settings, Nrf2 attaches to the Keap1 protein; however, during stress, it is activated, liberated from Keap1, and binds to antioxidant response elements, controlling various detoxification enzymes and antioxidant genes (118). Overexpression of miR-152 could attach to the 3′-UTR of Keap1 straightly, activating antioxidant and anti-inflammatory actions mediated by Nrf2, as well as reducing cell death (43). The miR-200b production was found to be decreased in cardiomyocytes treated with trophoblast stem cell-derived exosomes (TSC-Exos) in a recent study (44). Myocardial cell inflammatory response and apoptosis were both lowered by TSC-Exos and miR-200b inhibitors. TSC-Exos has anti-inflammatory and anti-apoptotic characteristics and may restore Zeb1 expression by suppressing miR-200b. Nevertheless, the specific method by which TSC-Exos controls miR-200b is uncertain.
Autophagy
Autophagy is essential for cardiomyocyte homeostasis, whereas it is disrupted when external stimuli are overwhelming, resulting in cell death (119). Autophagy was dramatically reduced in EPI-treated cardiomyocytes, worsening the apoptotic process (68). Paeonol, a Chinese medicinal extract from Moutan Cortex, can reduce EPI-induced heart damage, boost miR-1 upregulation, and block the PI3K/AKT signal pathway. NF-κB and mTOR are critical downstream targets of PI3K/AKT that significantly impact restoring autophagy levels and reducing myocardial cell death. Another study found that miR-146a, which targeted the TAF9b/P53 pathway, improved autophagy impairment and inhibited apoptosis, ameliorating DOX-induced myocardial injury (26). TAF9b is a co-activator of P53 and mediates autophagy impairment and apoptosis levels by affecting the stability of P53 (120).
Interestingly, recent studies have shown that DOX increases cardiac function impairment by inducing excessive autophagy in cardiomyocytes. The levels of autophagy markers LC3-II/LC3-I and autophagy were raised in DOX-treated rat hearts, while the expressions of both miR-30a and miR-30e were lowered (22, 48). Beclin 1, a downstream target of miR-30, mediates excessive autophagy in cardiomyocytes (121). Enhancing miR-30a/e expression can reduce Beclin 1 translation, inhibit increased cardiomyocytes autophagy, and improve myocardial injury. Another study showed that DOX decreased miR-204 levels in vivo and in vitro, increased HMGB1 protein expression levels, and promoted the formation of autophagic vesicles in the nucleus (49). HMGB1 is a DNA-binding nuclear protein involved in the regulation of apoptosis/autophagy balance (122). HMGB1 3′-UTR has a miR-204-binding site, and overexpression of miR-204 significantly attenuated DOX-induced autophagy levels and improved myocardial function by directly inhibiting the HMGB1 pathway.
Senescence
Cellular senescence has been characterized as a stable cell cycle arrest with the termination of cellular value addition in response to diverse stresses (123). In a DOX-induced cardiomyocyte senescence model, senescence-associated β-galactosidase (SA-β-gal) activity was increased, senescence gene indicators were deregulated, and miR-21 expression was increased (57). The downstream target gene of miR-21, PTEN, has been confirmed. MiR-21 can speed up cardiomyocyte aging by targeting to increase the expression of PTEN, whereas inhibiting miR-21 expression can play an antiaging effect.
The bulk of current research is focused on miR-34a, which has been shown to function on multiple target genes. AntimiR-34a inhibited the expression of miR-34a, which negatively regulated Bcl-2 and SIRT1 expression and delayed cellular senescence (51). SIRT1 is a NAD-dependent sirtuin that mediates cellular senescence by reducing the acetylation level of the senescence-related gene P53 (124). Another study showed that the expression of p16 and p53 was significantly elevated, telomerase activity decreased, telomere length shortened, and cell viability and proliferation decreased after DOX treatment (53). Telomeres are a key marker of cellular senescence, preventing DNA damage at the end of the eukaryotic chromosomes (125). SIRT1 is essential for the elongation of telomeres and the stability of the genome (126). Mesenchymal stem cells (MSCs) can inhibit miR-34a, increase SIRT1 expression, improve telomerase activity and telomere length, restore cell viability and proliferation, and serve as an antiaging agent (53). As a result, MSCs may hold significant therapeutic potential in the therapy of DOX-treated cardiac aging. Furthermore, serum extracellular vesicles (EVs) can delay cardiomyocyte senescence, in which miR-34a plays a vital role (52). Telomere attrition, DNA damage, and apoptosis have been demonstrated to be reduced by PNUTS, a miR-34a target gene (127). Serum EVs inhibit the miR-34a production, which negatively regulates PNUTS expression, thereby playing an antiaging effect.
According to a recent study, miR-199a-3p not only attenuated DOX-induced senescence in cardiomyocytes but also reduced the spread of senescence (15). GATA4 is a critical factor regulating programmed senescence and injury-induced senescence, and miR-199a-3p reduces its translation by binding straightly to its 3′-UTR, which has an antiaging impact. MiR-199a-3p can also inhibit the generation of SASP factors, thus acting as an inhibitor of the spread of senescence. SASP is composed of chemokines, proinflammatory cytokines as well as other factors with deleterious paracrine and systemic effects that contribute to the senescence of otherwise healthy cardiomyocytes (128).
Calcium homeostasis
Disruption of calcium homeostasis has been suggested as another critical pathogenic mechanism leading to cardiac injury from anthracyclines (129). Calumenin is a calcium-binding protein located in the endoplasmic reticulum of mammalian tissues and is a crucial participant in calcium ion (Ca2+) homeostasis in the endoplasmic reticulum (130). The quantities of calumenin mRNA and protein rose when miR378* was overexpressed. Silencing miR378* inhibited this enhanced expression, suggesting that calumenin might be a miR378* target (60). By inhibiting the expression of calumenin, miR378* may alter endoplasmic reticulum stress and promote the endoplasmic reticulum stress-mediated apoptosis pathway; however, the exact molecular mechanism is still unclear. Another research discovered that suppression of miR-375 alleviated DOX-caused contractile dysfunction in vivo and in vitro models in which Ca2+ has an essential impact on modulating myocardial cell contractility (29). In previous studies, ROS production disrupted cardiomyocyte calcium homeostasis, leading to contractile dysfunction (131). By stimulating the PDK1/AKT signaling pathway, inhibiting miR-375 lowers oxidative damage and enhances heart systolic performance. Furthermore, in cardiac failure caused by DOX, miR-24 expression was considerably enhanced, negatively regulating its downstream factor JP-2 (59). JP-2 is a structural protein that modulates Ca2+ signaling by linking the transverse tubule to the sarcoplasmic reticulum, vital for excitation-contraction (EC) coupling (132, 133). The EC-coupling structure was destabilized by the downregulation of JP-2, resulting in decreased Ca2+ release and lower myocardial contractility. Inhibition of miR-24 expression and restoration of JP-2 expression can ameliorate DOX-induced heart failure.
In a THP-induced myocardial injury model, the expression of miR-129-1-3p was recently discovered to be downregulated (67). GRIN2D is a subunit of the N-methyl-D-aspartate (NMDA) receptor complex that forms a ligand-gated ion channel with high calcium permeability (134). MiR-129-1-3p negatively modulated GRIN2D expression via straightly attaching the GRIN2D 3′-UTR, leading cardiomyocyte Ca2+ signaling to become overactive, resulting in calcium overload and cell death. The regulation of calcium homeostasis by overexpression of miR-129-1-3p may offer a novel strategy for treating THP cardiotoxicity.
Vascular homeostasis
The maintenance of vascular homeostasis is achieved by the joint action of vascular endothelial cells, fibroblasts, and vascular smooth muscle cells to generate vascular endothelial growth factor (VEGF), nitric oxide, and other vasoactive substances (135). Among them, VEGF-A mediates the development and stabilization of blood vessels, which is essential for maintaining vascular homeostasis (136). Decreased microvessel density and endothelial cell injury lead to the disruption of vascular homeostasis, inducing the development of DOX cardiotoxicity. For example, in DOX-treated mouse hearts, the vascular endothelium was damaged, nitric oxide release, tube formation, and migration of endothelial cells were impaired, the microvessel density markers CD31 and CD34 were decreased, and cardiac microvessels were damaged, accompanied by a significant upregulation of miR-320a (62). Similar to miR-320a treatment, targeting VEGF-A-specific siRNA promotes endothelial dysfunction and perturbs vascular homeostasis. This suggests that VEGF-A is a target of miR-320a and is adversely influenced by miR-320a. MiR-320a inhibition can restore VEGF-A expression, allowing cardiovascular homeostasis to be maintained. Conversely, another study found that miR-526b-3p was associated with DOX-treated dysfunction of vascular homeostasis, but not via directly targeting the VEGF-A 3′-UTR to inhibit VEGFA expression (61). This study proposes that miR-526b-3p directly targets STAT3, and the VEGF-A promoter of STAT3 has effective binding sites for BS1 and BS2, to inhibit the transcription of VEGF-A.
Fibrosis
Doxorubicin has been linked to cardiac fibrosis in several investigations, and it has been shown to cause fibrous disorganization and accumulation of collagen fibers. DOX-induced ROS production has been reported to trigger the TGF-β route, which stimulates cardiac fibroblasts and promotes fibrosis (137). TGF-β, a pro-fibrotic marker, and its downstream effector, phosphorylated SMAD3, both rose significantly in DOX-induced rat cardiomyocytes (51). Pro-fibrotic and NF-κB-mediated inflammatory factors impact the amount of myocardial fibrosis. AntimiR-34a upregulated Bcl-2 and SIRT1 expression as well as reduced acetylation levels of SMAD2/3 and NF-κB, thus reducing inflammation and fibrosis while also improving cardiac diastolic and systolic function.
Another study showed that miR-96, derived from MSC-Exos, enhanced systolic and diastolic functions as well as attenuated cardiac damage in DOX-treated rat hearts by blocking the Rac1/NF-κB signaling pathway and reducing oxidative stress markers (GSH-Px, SOD, and malondialdehyde), inflammatory cytokines (IL-1β, TNF-α, and IL-6), and collagen fiber accumulation (64). Moreover, overexpression of miR-133b targeted the 3′-UTR of PTBP1 and TAGLN2, negatively regulated their translation levels, and inhibited myocardial cell death and collagen accumulation, thus alleviating DOX-induced myocardial fibrosis (63). PTBP1 belongs to a subfamily of ubiquitously expressed heterogeneous nuclear ribonucleoproteins and is a multifunctional RNA-binding protein involved in many biological processes, including maintenance of cellular structure and motility, immunity, protein metabolism, and the cell cycle (138). TAGLN2 is an actin-binding protein involved in the regulation of cell morphology, motility, and cellular transformation (139). Upregulation of PTBP1 or TAGLN2 may counteract the miR-133b influence on cell death and collagen buildup.
lncRNA
Apoptosis
The lncRNA Mhrt, which is downregulated in failing cardiac tissue, was first used to investigate the role of lncRNAs in DOX-caused cardiotoxicity (78). Mhrt affects Nrf2 expression levels via regulating the formation of the H3 histone and Nrf2 promoter complex. By regulating Nrf2 and enhancing the activity of the proapoptotic protein caspase-3, downregulation of Mhrt induces cardiomyocyte death. By overexpressing the Mhrt-Nrf2 signaling pathway, obstatin was able to reverse these effects. A large body of evidence has demonstrated that lncRNAs might operate as ceRNAs to control miRNA production, therefore participating in DOX-induced apoptosis. For example, a previous study identified LINC00339 as a miR484 target through bioinformatics and luciferase reporter analyses (77). As an endogenous “sponge” of miR484, LINC00339 may be involved in cardiomyocyte apoptosis via directly targeting and regulating miR484 production, and its knockdown could reverse the effect on cardiomyocyte apoptosis. Moreover, the production of lnc SNHG1 was diminished in DOX-treated AC16 cells (76). SNHG1 adversely regulated miR-195 production via serving as a ceRNA. Bcl-2 was shown to be a miR-195 target gene, known to be a crucial regulator in mediating apoptosis (140). Overexpression of SNHG1 alleviated DOX-induced apoptosis by reducing miR-195 expression, increasing Bcl-2 protein quantities, and inhibiting caspase-3 cleavage.
Similarly, the lncRNA NEAT1 was linked to apoptosis and its expression was lowered considerably following DOX therapy (75). Let-7f-2-3p expression was elevated by the lncRNA NEAT1, which was discovered to be an endogenous sponge RNA of let-7f-2-3p. XPO1 is critical for controlling nuclear protein export, which distributes various anti-apoptotic proteins such as HAX-1 from the nucleus to the cytoplasm to regulate apoptosis (141, 142). Let-7f-2-3p inhibits XPO1 production through targeting its 3′-UTR, inhibiting XPO1-mediated nuclear export of HAX-1, and increasing myocardial enzyme release and cell death. DOX-induced myocardial damage was attenuated without compromising its anticancer activity when NEAT1 was overexpressed or let-7f-2-3p was knocked down. Furthermore, lnc SOX2-OT and DP5 were significantly upregulated after DOX treatment, whereas miR-942-5p was considerably downregulated (71). SOX2-OT acted as a miR-942-5p sponge, affecting its production. MiR-942-5p negatively regulated DP5 expression, an apoptosis-related tumor suppressor gene. Therefore, SOX2-OT suppression diminishes myocardial cell death and prevents cardiac function damage via modulating the miR-942-5p/DP5 axis.
Chinese herbal extracts have been demonstrated to defend against cardiotoxicity caused by DOX via modulating specific lncRNAs in a recent study (69). Salvianolic acid A (SalA), the effective constituent of Chinese medicine Salvia miltiorrhiza, has an apoptotic inhibitory effect by blocking NFKB1 transcriptional activation and downregulating lncRNA PVT1 production. DOX was reported to enhance PVT1 expression, decrease cell viability, and aggravate apoptosis rate (70). Through adsorbing miR-187-3p via sponge action, PVT1 lowered its level. AGO1 is a well-known cell death regulator that controls cell death through numerous pathways (143, 144). AGO1 is a downstream target of miR-187-3p whose expression levels are inversely linked. As a result, the highly expressed PVT1 induced cardiomyocyte death by enhancing AGO1 expression through sponge adsorption of miR-187-3p.
Mitochondrial damage
Hypoxia treatment of human adipose-derived MSCs resulted in the accumulation of the lncRNA MALAT1 in secreted exosomes (80). Silencing MALAT1 in MSCs or overexpressing miR-92a-3p in myocardial cells increased Fabp3, Fabp4, and Mtfp1 expressions while decreased Cox4i2, Hspa1a, and Atp1b2 expressions, resulting in mitochondrial metabolic disorders. MALAT1 was discovered to be an exosomal transfer RNA that inhibits the production of miR-92a-3p, which targets and binds to the 3′-UTR of ATG4a. MALAT1 is a ceRNA that binds to miR-92a-3p and activates ATG4a, thereby enhancing mitochondrial metabolism, restoring cardiomyocyte viability, promoting rejuvenation, and inhibiting DOX-induced cardiac aging, according to this study.
A recent study showed that among the lncRNAs with differing expressions, CMDL-1 was the most dramatically attenuated lncRNA in myocardial cells following DOX injection (79). Drp1 is a cytoplasmic protein whose function is decreased and mitochondrial fission is prevented by phosphorylation of its serine residue at position 637 (145). Drp1-mediated mitochondrial fission and apoptosis may be inhibited by the upregulation of CMDL-1, which regulates Drp1 phosphorylation. Drp1 knockdown partially suppressed CMDL-1's anti-mitochondrial fission and anti-apoptotic actions, but not completely. This suggests that CMDL-1 might control Drp1's function rather than transcription; however, the exact molecular mechanism remains further studied.
Oxidative stress and senescence
In the DOX cell model, lincRNA-p21 is thought to regulate oxidative stress and may be involved in cellular senescence by inducing ROS production and oxidative damage (82, 146). In HL-1 cells treated with DOX, enhanced lincRNA-p21 expression was accompanied by decreased mitochondrial transmembrane potential and SOD activity, as well as increased ROS production and MDA activity. Moreover, the age-related genes p16 and p53 were upregulated, and telomere length and telomerase activity were decreased. Antioxidant treatment inhibited oxidative stress, reduced ROS production, improved cell viability, and delayed cellular senescence considerably. Similarly, knocking down lincRNA-p21 also helped to reduce oxidative stress and alleviate DOX-induced cardiac aging. The Wnt/β-catenin signal pathway is known to have a role in heart regeneration and aging, and knocking down lincRNA-p21 exerts cardioprotection by activating the Wnt/β-catenin signal pathway (147).
Ferroptosis
Ferroptosis has been identified as a unique cell death process that is iron-dependent rather than apoptosis and is defined by a substantial intracellular accumulation of free iron and lipid ROS (148). In the DOX cell model, the lncRNA KCNQ1OT1 is a crucial lncRNA involved in the mechanism of ferroptosis (73). In DOX-treated cardiomyocytes, free iron and lipid ROS were dramatically increased, and METTL14 was upregulated and catalyzed the m6A modification of KCNQ1OT1. MiR-7-5p has been discovered as a direct site of KCNQ1OT1, which binds to the 3′-UTR of the transferrin receptor (TFRC) and controls its expression. TFRC is required for cellular uptake of the transferrin-iron complex, and its overexpression promotes iron uptake and lipid ROS production, ultimately leading to cardiomyocyte ferroptosis (149). Interestingly, miR-7-5p was also involved in the targeted repression of METTL14 expression, implying the existence of an additional feedforward mechanism in the ferroptosis mechanism. In conclusion, the METTL14/KCNQ1OT1/miR-7-5p/TFRC axis plays an essential role in DOX-induced ferroptosis.
CircRNA
Apoptosis
Qki5 is known to be an RBP that governs various circRNAs (150). According to previous studies, Qki5 regulates a group of circRNAs produced by the genes Fhod3, Ttn, and Strn3, which mediate cardiomyocyte apoptosis (85). MiR-31-5p was discovered to adversely affect the development of circPan3 by directly targeting QKI in recent research (18). By silencing QKI and upregulating circPan3, inhibition of miR-31-5p alleviated apoptosis. However, the downstream molecular processes of circPan3 were not investigated further in this study.
CircRNAs can behave as miRNA sponges, generating anti-apoptotic effects, according to another research. For example, the expression of circ-SKA3 and TLR4 was enhanced following DOX treatment (84). In contrast, the expression of miR-1303 was lowered, which was followed by reduced cell viability and increased proapoptotic proteins. The expression of TLR4 was mediated by Circ-SKA3, which behaved like a sponge for miR-1303. TLR4 is known to belong to the toll-like receptor (TLR) family, which regulates inflammatory and immune responses (151). Circ-SKA3 knockdown attenuated DOX-induced cardiotoxicity via the miR-1303/TLR4 axis. Interestingly, extracellular circ-SKA3 was revealed to be bundled into exosomes in this investigation. Exosomal circ-SKA3 may be taken up and internalized by target cells AC16, potentially affecting the RNA expression and function of AC16 cells. Moreover, mmu_circ_0002106, mmu_circ_0016006, and mmu_circ_00115773 were upregulated after DOX treatment, suggesting that these three circRNAs may contribute to myocardial injury by promoting apoptosis, albeit the exact process has to be investigated further (83).
Oxidative stress
CircITCH, an E3-ubiquitin protein ligase that modulates tumor suppressor stability, is engaged in oxidative stress generated by DOX (152). In hiPSC-CMs treated by DOX and cancer sufferers' postmortem tissues with DOX heart disease, circITCH expression has been reported to be downregulated (87). CircITCH functions as a natural sponge for miR-330-5p, targeting and regulating it, which in turn controls the interaction between miR-330-5p and the 3′-UTR of SIRT6, BIRC5, and ATP2A2 mRNAs. As previously documented, SIRT6 regulates oxidative stress and DNA damage, BIRC5-encoded survivin regulates apoptosis, and ATP2A2-encoded SERCA2a regulates cardiomyocyte contractile performance and calcium regulation (153–156). CircITCH overexpression ameliorated DOX-induced cardiac dysfunction through modulating the miR-330-5p-SIRT6/BIRC5/ATP2A2 axis, whereas CircITCH knockdown abolished these effects. CircITCH is a broad-spectrum tumor suppressor that also alleviates DOX-induced cardiotoxicity, suggesting that overexpression of CircITCH might be a valuable method to cure DOX-generated cardiotoxicity.
Another study showed that circArhgap12 expression was elevated and ROS generation increased after DOX treatment, which led to cardiomyocyte death (86). CircArhgap12 served as a sponge for miR-135a-5p and inhibiting it alleviated oxidative stress and cell death by controlling miR-135a-5p production. Furthermore, ADCY1 mRNA may contain possible target locations for miR-135a-5p, and the downstream molecular mechanism must be investigated further.
Radiotherapy
Radiation therapy provides benefits in terms of lowering cancer recurrence and mortality, but at the same time leads to an increased risk of cardiovascular dysfunction (157). It has been reported that miR-34a is significantly upregulated in cardiomyocytes after exposure to radiation, with increased expression of aging-related genes Cdkn1a and Cdkn2c and decreased length and activity of telomere (89). Macrophage migration inhibitory factor (MIF) is a multifunctional cytokine found throughout the body that plays a significant role in cardio-metabolism (158). Exogenous MIF significantly inhibited the radiation-induced expression of miR-34a, which in turn restored SIRT1-mediated antiaging properties. It is well known that SIRT1 is a miR-34a target that has been associated with cellular senescence (124). Oxidative stress hastens the aging of cardiomyocytes (159). Further research revealed that MIF inhibited cellular aging by preventing radiation-induced cardiomyocyte ROS and MDA production via regulating oxidative stress. MIF's antioxidant action was abolished when miR-34a was overexpressed or SIRT1 was knocked down. As a result, by regulating the miR-34a/SIRT1 signaling pathway and avoiding oxidative stress-mediated cellular senescence, MIF might be crucial in treating the radiation-induced cardiac injury.
Additionally, circFOXO3 was remarkably upregulated after radiation to promote DNA repair and limit the apoptosis rate to protect cardiomyocytes (88). The effects were abolished when circFOXO3 was knocked out, but the specific chemical mechanism remains unclear.
Arsenic trioxide
Although arsenic trioxide (As2O3) is good therapy for acute promyelocytic leukemia and other malignant tumors, its broad clinical applicability is limited due to cardiotoxicity adverse effects (160). In As2O3-treated guinea pigs, there was a dose-dependent lengthening of the QRS complex and QT interval, which resulted in increased mortality (92). Further studies discovered that As2O3 upregulated miR-133 and miR-1 production while decreasing ether-a-go-go-related gene (ERG) and Kir2.1 protein levels. Kir2.1 is a potassium channel subunit that is essential to sustain the voltage of the resting membrane and create the final repolarization. It predominantly mediates the inward rectifying potassium current IK1 (161). Long QT syndrome is known to be caused by reduced IKr current density and human ERG (hERG) channel production in As2O3-treated patients (162). Another research demonstrated that miR-21 and miR-23a inhibited hERG expression and thereby caused As2O3-induced electrophysiological disturbances (91). As2O3 increased NF-κB phosphorylation, which upregulated miR-21 and inhibited the expression of Sp1, a transcription factor required for driving transcription from the ERG promoter (163). Additionally, lncRNA NEAT1 plays a crucial role in As2O3-induced cardiotoxicity (90). By blocking the miR-124/NF-κB signal path and reducing inflammatory response and apoptosis, NEAT1 overexpression protects cardiac cells against As2O3 injury.
Targeted molecular drugs
Bevacizumab (BVZ), a monoclonal antibody targeting VEGF ligands, achieves antitumor effects by suppressing tumor angiogenesis and lowering neovascularization. However, cardiotoxic adverse effects may occur after long-term treatment (164, 165). BVZ causes cardiomyocyte mortality that is dose- and duration-dependent, leading to cardiac damage, according to a recent study (93). Previously, miR-140-5p was thought to have a role in DOX-caused oxidative stress (35, 36). After BVZ treatment, miR-140-5p was upregulated and reduced VEGFA expression by targeting its 3′-UTR. The expression of 14-3-3γ, which is linked to oxidative stress and apoptosis in cardiomyocytes, was considerably reduced when VEGFA expression was silenced (166). MiR-140-5p inhibition, which targets the VEGFA/14-3-3γ signaling pathway, lowered MDA and ROS levels and mitigated BVZ-induced oxidative damage. Cardiotoxicity has also been connected to antitumor medicines that target the platelet-derived growth factor receptor (PDGFR). MiR-9 has been reported to regulate PDGFR-β-mediated compensatory angiogenesis by targeting the 3′-UTR of PDFGR-β and adversely regulating its expression (94).
ncRNAs as biomarkers in cancer therapy-induced cardiotoxicity
Non-coding RNAs have been identified as prospective novel biomarkers for early identification of cardiotoxicity generated by cancer treatment, in addition to the functions and mechanisms of ncRNAs outlined above. Table 2 shows a list of potential ncRNA biomarkers.
Anthracyclines
Earlier studies focused on animal models' screening for differential miRNA expression in DOX-induced cardiac tissue. For instance, one study showed that 24 miRNAs were differently expressed in DOX-induced mouse hearts, with miR-34a being the only miRNA significantly upregulated across all DOX doses and miR-34a expression level increasing with DOX dosages overall (174). Furthermore, the lowest cumulative DOX dose that caused miR-34a elevation was 6 mg/kg, which occurred well before cardiac troponin T (cTnT) changes and histopathological damage. In hiPSC-CMs treated with DOX, another study corroborated the early elevation of miR-34a-3p (173). According to these findings, a rise in miR-34a might be a precursor to DOX-induced cardiac injury. Early miRNA changes in the heart may be useful in developing biomarkers to identify patients with DOX-induced cardiotoxicity. However, the invasive nature of tissue biopsy limits its use in clinical research.
Due to the stable expression of miRNAs in nearly all body fluids (blood, serum, saliva, etc.) and the development of detection techniques, circulating miRNAs (c-miRNAs) have recently been viewed as promising early biomarkers for the detection of cardiotoxicity induced by cancer therapy (182). Although miR-208a expression is known to be reduced in DOX-damaged mouse hearts, plasma miR-208a could not be detected in research on DOX-caused BC individuals, even when troponin levels were at their peak after 12 weeks, implying that DOX-damaged hearts do not release miR-208a into the bloodstream (172, 175, 183). MiR-34a-5p expression was upregulated in DOX-treated mice's plasma, whereas seven miRNAs, including miR-133a-3p and miR-1-3p, were suppressed. In this investigation, however, no plasma levels of cardiac troponin I (cTnI) or brain natriuretic peptide were detected (171). Another research in DOX-treated rats found increased expression of plasma miR-133b, miR-1, and miR-133a 24 h following the administration (184). However, there were no noticeable changes in circulating cTnI and cTnT levels or histopathology. It is possible that DOX has not yet caused cardiac injury in rats, and that the acute toxic effects of DOX occur in skeletal muscle before cardiac injury. The circulating levels of miR-1 and−133b were shown to be higher in BC individuals treated with DOX in a study (172). Taken together, it may suggest that varied expression of c-miRNAs is connected to the differences in experimental animal models, species, and levels of DOX treatment and that changes shown in animal models are not always transferable to the human clinical setting.
In recent years, the bulk of studies on c-miRNAs profiles has focused on BC individuals undergoing anthracycline therapy. For instance, four congestive heart failure-related c-miRNAs (miR-423-5p, miR-34a-5p, miR-126-3p, and miR-199a-3p) were identified in patients with BC treated with anthracyclines, whereas another systematic analysis investigated five c-miRNAs (miR-126, miR-210, miR-20a, Let-7f, and miR-1) (167, 176). In a study of epirubicin/cyclophosphamide followed by docetaxel (EC-D)-induced cardiotoxicity in BC individuals, miR-17-5p and miR-20a expression levels were lowered (176). The two miRNAs have a good forecast value for predicting a lower risk of cardiotoxicity, according to receiver operating characteristic (ROC) curves. These findings potentially point to miRNAs as possible biomarkers for predicting anthracycline-induced cardiotoxicity in patients with BC.
Furthermore, heart-related plasma miR-29b and miR-499 levels were considerably elevated 6–24 h following the anthracycline dosing in children and young patients with a drug dose–response relationship, which may facilitate early diagnosis of myocardial injury (169). Consistent with the results, another study showed substantial differences in the levels of 17 miRNAs in children receiving anthracycline chemotherapy in comparison with normal children (168). Among them, miR-499a-5p and miR-29c-3p were found to be considerably elevated following the beginning and finish of anthracycline treatment, respectively.
In a dog sarcoma model receiving only DOX treatment, four chosen EV-miRNAs appeared variably expressed (miR-181d, miR-502, miR-107, and miR-146a) (170). Further studies revealed that miR-181d was upregulated in individuals with a low left ventricular ejection fraction, and miR-502 was upregulated after only two doses of DOX, far ahead of changes in cTnI and echocardiographic parameters. These results may imply that EV-miRNAs can be employed as the biomarkers for early cardiotoxicity identification, although larger sample sizes are needed for future validation.
Radiotherapy
In patients with BC treated with radiotherapy, the production of miRNAs-221,−222,−146a, and−155 in the blood is elevated, which are known to be associated with the progression of CVD (179). These miRNA expression levels were strongly connected with pre-radiotherapy control and post-radiotherapy and adversely correlated with age. Moreover, CVD-related risk variables such as dyslipidemia, therapy being taken, and one side of breast cancer, affected alterations in their levels. These findings may suggest that alterations in these miRNAs may be linked to the progression of radiotherapy-induced CVD in patients with BC. In patients with non-small cell lung cancer (NSCLC) who received irradiation in an investigation, pretreatment c-miRNA signatures were found to be predictive of radiation-induced cardiotoxicity grade 3 or above (G3+) (178). The signatures consisted of 14 c-miRNA species whose serum concentrations changed as G3+ radiation-induced cardiotoxicity progressed.
A recent study screened mouse cardiac tissue for the changes in mRNAs, miRNAs, and lncRNAs levels that predict radiation-induced heart damage after exposing mice to 1, 2, 4, 8, and 12 Gray (Gy) whole-body irradiation for 48 h (177). The study found that the expression alterations in numerous mRNAs and ncRNAs at different doses, such as LncRNA Abhd11os, Pvt1, Trp53cor1, and Dino, rose as the radiation dose increased. Surprisingly, significant upregulation of miRNAs was only found at 1 and 12 Gy, and no miRNAs were detected to be consistently upregulated or downregulated at all doses. This is likely owing to the early time point and rigorous statistical analysis.
Targeted molecular drugs
In patients with BVZ-treated colorectal cancer, both plasma miR1254 and miRNA579 expression levels were raised, and miRNA1254 was unaffected by different patient characteristics and corresponded most strongly with the clinical diagnosis of BVZ-induced cardiotoxicity (181). This suggests that the specific elevations of miR1254 and miRNA579 may be the important indicators of BVZ-induced cardiotoxicity, allowing BVZ-induced cardiotoxicity to be distinguished from other cardiovascular disorders. Another research of patients with NSCLC receiving BVZ chemotherapy discovered that blood miR-30c expression rose with treatment duration and was positively correlated with cardiotoxicity before and during chemotherapy (180). According to a ROC curve investigation evaluating the area under curve sensitivity and specificity of changes in blood miR-30c levels, miR-30c may be a sensitive biomarker for diagnosing cardiotoxicity in patients with NSCLC treated with BVZ.
ncRNAs as therapeutic targets in cancer therapy-induced cardiotoxicity
Numerous studies have revealed that ncRNAs play a role in the molecular signal pathways regulating cancer therapy-induced cardiotoxicity. As a result, ncRNAs are being considered potential therapeutic targets for cancer therapy-induced cardiotoxicity, with therapeutic strategies targeting ncRNAs showing promise. Further studies looked at miRNA antagonists and mimic to boost or suppress miRNA expression. For example, as we discussed above, therapeutic silencing of miR-34a expression levels by antimiR-34a and miR-29b overexpression after miR-29b agomir treatment attenuated DOX-induced cardiotoxicity in rats (39, 51). In addition, viral vectors (e.g., adenovirus, AAV, and lentivirus) can be employed to transport ncRNAs to cells and boost their expression levels. For instance, AAV9-based overexpression of miR-200a and lentiviral overexpression of CMDL-1 prevented DOX-induced cardiomyocyte injury, and lentiviral overexpression of circFOXO3 reduced radiation-induced DNA damage and apoptosis (34, 79, 88). Furthermore, siRNA and antisense nucleotides are used to silence ncRNAs, such as knockdown of SOX2-OT and circSKA3, to enhance cardiac function (71, 84).
Cell-based treatments, particularly MSCs, hold considerable promise for the treatment of cardiovascular diseases because of their regeneration capabilities and established biosafety (185). MSCs-based therapies can ameliorate DOX-induced cardiac aging, according to a recent study, and further research has linked MSCs antiaging properties to the regulation of the miR-34a-SIRT1 axis (53). However, stem cell therapy is limited by inconsistent supply, infusion toxicity, poor survival, and immune rejection. Excitingly, exosome-based cell-free therapy is considered to be a promising therapeutic approach, especially MSC-sEVs due to their regenerative and remodeling abilities for cardiac injury (186). Several recent studies have discovered that stem cell-secreted miRNA-rich exosomes can suppress DOX-induced oxidative stress, inflammatory responses, fibrosis, and apoptosis, hence functioning as cardioprotective agents (14, 28, 64). Another study found that MSCs-derived exosomal hypoxia-derived lncRNA MALAT might improve mitochondrial metabolism and promote regeneration in a synergistic manner, ultimately alleviating cardiac aging (80). Notably, the latest study proposes a new local delivery technique based on exosomes and ultrasound, which improves cardiac drug delivery efficiency dramatically (55). Exosomes containing miRNA mimics were administered into experimental animals, and ultrasound-targeted microbubble destruction was done in the heart area. As a result, exosome-mediated miRNA distribution to the heart was greatly enhanced, successfully lowering DOX-induced cardiotoxicity. Therefore, further research is required to generate repeatable and stable MSC-EV products for clinical applications, as the current studies have not looked at clinically meaningful doses.
Several medications have been demonstrated to regulate ncRNA production and signaling pathways to reduce cardiotoxicity caused by cancer therapy, thereby exerting cardioprotective effects. For example, by regulating the miR-30e/Beclin 1 signaling route, ACE2 suppresses DOX-induced cardiac autophagy, whereas dexrazoxane prevents cardiomyocyte death via controlling the miR-17-5p/PTEN signaling network (17, 50). In addition, herbal medicines have cardioprotective benefits against cancer-related cardiotoxicity. Chinese medicine Salvia miltiorrhiza's active ingredient, SalA, for instance, is involved in reducing lncRNA PVT1 expression; the Chinese medicine formula Shenmai injection, on the other hand, can control the miR-30a/Beclin 1 pathway and ameliorate heart injury (48, 69). To summarize, ncRNAs have been discovered to have therapeutic promise, but current studies are still in the preclinical stage, and more research is needed in the future.
Conclusion
One of the most hazardous side effects of cancer treatment is cardiotoxicity, which seriously affects cancer patients' quality of survival. Recently, research on ncRNAs in cancer therapy-induced cardiotoxicity has made substantial progress and is regarded as a promising actor with promising application prospects. This review outlines recent developments in the study of the ncRNAs involved in cancer therapy-induced cardiotoxicity. Apoptosis, mitochondrial damage, oxidative stress, DNA damage, inflammation, autophagy, aging, calcium homeostasis, vascular homeostasis, and fibrosis are all regulated by ncRNAs and play a role in the occurrence and progression of cancer therapy-induced cardiotoxicity. Circulating ncRNAs are being evaluated as potential new biomarkers for detecting cancer therapy-induced cardiotoxicity early, which is crucial for a better patient prognosis. Because circulating ncRNAs are highly stable and specific, tests for them are inexpensive, rapid, and noninvasive, and changes in their expression levels may be beneficial for detecting cardiotoxicity in patients with cancer. Furthermore, ncRNAs have therapeutic potential, with numerous studies confirming that ncRNAs may be used as therapeutic targets to relieve the cardiotoxicity caused by cancer therapy.
To date, researchers have carried out a large number of studies on miRNAs, while understanding of lncRNAs and circRNAs is still in the preliminary exploration stage. There is also a lack of studies on ncRNAs in cardiotoxicity induced by other cancer treatments, such as platinum and paclitaxel used in first-line chemotherapy, and more work focusing on these areas is still needed in the future. In addition, despite the prospective clinical applications of ncRNAs and the great progress gained, many challenges and limits remain. First and foremost, the fundamental problems of ncRNA-based therapeutics are dependable delivery systems and potential off-target consequences, with tissue-specific delivery with long-term on-target effects being a major concern. Second, because cancer therapy-induced cardiotoxicity impacts the expression of numerous ncRNAs implicated in different signaling pathways simultaneously, ncRNAs as therapeutic targets may lack therapeutic specificity, limiting the comprehension of the pharmacological mechanism. Another disadvantage is that most research is conducted on healthy cells or animal models. However, healthy cardiomyocytes cannot represent patients of all ages, and there are species differences in ncRNAs between experimental animals and human clinical environments. Tumors themselves may lead to differential expression of ncRNAs, even among patients from different tumor types. Tumor patient therapy is a dynamic process accompanied by dynamic ncRNA expression. In addition, it is unclear whether ncRNA therapy promotes tumor growth or impacts the effectiveness of cancer treatment. To address these challenges, more research is required in the future. In conclusion, the study of ncRNAs in cancer therapy-induced cardiotoxicity not only contributes to a deeper grasp of the molecular pathways underlying oncological cardiology but also offers a new promising detection method and therapeutic strategy, giving cancer therapy-induced cardiotoxicity patients new hope.
Author contributions
YX and ZS designed this study. WS wrote the first draft of this manuscript and created figures. JX, LW, YJ, and JC participated in discussions and improved pictures related to the manuscript. XS, FY, and LT critically revised the manuscript. All authors contributed to the article and approved the submitted version.
Funding
This work was supported by the National Natural Science Foundation of China (Grant Nos. 81725024 and 81430098), the CACMS Innovation Fund (Grant No. CI2021A00919), and the National Key R&D Program of China (Grant Nos. 2018YFC1704901 and 2018YFC1704900).
Conflict of interest
The authors declare that the research was conducted in the absence of any commercial or financial relationships that could be construed as a potential conflict of interest.
Publisher's note
All claims expressed in this article are solely those of the authors and do not necessarily represent those of their affiliated organizations, or those of the publisher, the editors and the reviewers. Any product that may be evaluated in this article, or claim that may be made by its manufacturer, is not guaranteed or endorsed by the publisher.
References
1. Miller KD, Nogueira L, Mariotto AB, Rowland JH, Yabroff KR, Alfano CM, et al. Cancer treatment and survivorship statistics, 2019. CA Cancer J Clin. (2019) 69:363–85. doi: 10.3322/caac.21565
2. Curigliano G, Cardinale D, Dent S, Criscitiello C, Aseyev O, Lenihan D, et al. Cardiotoxicity of anticancer treatments: epidemiology, detection, and management. CA Cancer J Clin. (2016) 66:309–25. doi: 10.3322/caac.21341
3. Moslehi JJ. Cardiovascular toxic effects of targeted cancer therapies. N Engl J Med. (2016) 375:1457–67. doi: 10.1056/NEJMra1100265
4. Dent S, Liu P, Brezden-Masley C, Lenihan D. Cancer and cardiovascular disease: the complex labyrinth. J Oncol. (2015) 2015:516450. doi: 10.1155/2015/516450
5. Sturgeon KM, Deng L, Bluethmann SM, Zhou S, Trifiletti DM, Jiang C, et al. A population-based study of cardiovascular disease mortality risk in US cancer patients. Eur Heart J. (2019) 40:3889–97. doi: 10.1093/eurheartj/ehz766
6. Mattick JS, Makunin IV. Non-coding RNA. Hum Mol Genet. (2006) 15(Spec No 1):R17–29. doi: 10.1093/hmg/ddl046
7. Bartel DP. MicroRNAs: target recognition and regulatory functions. Cell. (2009) 136:215–33. doi: 10.1016/j.cell.2009.01.002
8. Griffiths-Jones S, Grocock RJ, van Dongen S, Bateman A, Enright AJ. miRBase: microRNA sequences, targets and gene nomenclature. Nucleic Acids Res. (2006) 34(Database issue):D140–4. doi: 10.1093/nar/gkj112
9. Yao RW, Wang Y, Chen LL. Cellular functions of long noncoding RNAs. Nat Cell Biol. (2019) 21:542–51. doi: 10.1038/s41556-019-0311-8
10. Qu S, Yang X, Li X, Wang J, Gao Y, Shang R, et al. Circular RNA: A new star of noncoding RNAs. Cancer Lett. (2015) 365:141–8. doi: 10.1016/j.canlet.2015.06.003
11. Chen L, Xu Y. MicroRNAs as biomarkers and therapeutic targets in doxorubicin-induced cardiomyopathy: a review. Front Cardiovasc Med. (2021) 8:740515. doi: 10.3389/fcvm.2021.740515
12. Fa HG, Chang WG, Zhang XJ, Xiao DD, Wang JX. Noncoding RNAs in doxorubicin-induced cardiotoxicity and their potential as biomarkers and therapeutic targets. Acta Pharmacol Sin. (2021) 42:499–507. doi: 10.1038/s41401-020-0471-x
13. Song L, Qiao G, Xu Y, Ma L, Jiang W. Role of non-coding RNAs in cardiotoxicity of chemotherapy. Surg Oncol. (2018) 27:526–38. doi: 10.1016/j.suronc.2018.06.003
14. Lee JY, Chung J, Byun Y, Kim KH, An SH, Kwon K. Mesenchymal stem cell-derived small extracellular vesicles protect cardiomyocytes from doxorubicin-induced cardiomyopathy by upregulating survivin expression via the miR-199a-3p-Akt-Sp1/p53 signaling pathway. Int J Mol Sci. (2021) 22:102. doi: 10.3390/ijms22137102
15. Xia W, Chang B, Li L, Hu T, Ye J, Chen H, et al. MicroRNA therapy confers anti-senescent effects on doxorubicin-related cardiotoxicity by intracellular and paracrine signaling. Aging. (2021) 13:25256–70. doi: 10.18632/aging.203743
16. Jin X, Yu W, Ye P. MiR-125b enhances doxorubicin-induced cardiotoxicity by suppressing the nucleus-cytoplasmic translocation of YAP via targeting STARD13. Environ Toxicol. (2021). doi: 10.1002/tox.23438
17. Yu X, Ruan Y, Shen T, Qiu Q, Yan M, Sun S, et al. Dexrazoxane protects cardiomyocyte from doxorubicin-induced apoptosis by modulating miR-17-5p. Biomed Res Int. (2020) 2020:5107193. doi: 10.1155/2020/5107193
18. Ji X, Ding W, Xu T, Zheng X, Zhang J, Liu M, et al. MicroRNA-31-5p attenuates doxorubicin-induced cardiotoxicity via quaking and circular RNA Pan3. J Mol Cell Cardiol. (2020) 140:56–67. doi: 10.1016/j.yjmcc.2020.02.009
19. Gupta SK, Garg A, Avramopoulos P, Engelhardt S, Streckfuss-Bomeke K, Batkai S, et al. miR-212/132 cluster modulation prevents doxorubicin-mediated atrophy and cardiotoxicity. Mol Ther. (2019) 27:17–28. doi: 10.1016/j.ymthe.2018.11.004
20. Zhu JN, Fu YH, Hu ZQ Li WY, Tang CM, Fei HW, et al. Activation of miR-34a-5p/Sirt1/p66shc pathway contributes to doxorubicin-induced cardiotoxicity. Sci Rep. (2017) 7:11879. doi: 10.1038/s41598-017-12192-y
21. Razavi-Azarkhiavi K, Jaafari MR, Abnous K, Razavi BM, Jafarian AH, Hassani FV, et al. The cardiotoxic mechanism of doxorubicin (DOX) and pegylated liposomal DOX in mice bearing C-26 colon carcinoma: a study focused on microRNA role for toxicity assessment of new formulations. Pharm Res. (2017) 34:1849–56. doi: 10.1007/s11095-017-2194-3
22. Novak J, Sana J, Stracina T, Novakova M, Slaby O. Doxorubicin and liposomal doxorubicin differentially affect expression of miR-208a and let-7g in rat ventricles and atria. Cardiovasc Toxicol. (2017) 17:355–9. doi: 10.1007/s12012-016-9393-8
23. Beji S, Milano G, Scopece A, Cicchillitti L, Cencioni C, Picozza M, et al. Doxorubicin upregulates CXCR4 via miR-200c/ZEB1-dependent mechanism in human cardiac mesenchymal progenitor cells. Cell Death Dis. (2017) 8:e3020. doi: 10.1038/cddis.2017.409
24. Tony H, Yu K, Qiutang Z. MicroRNA-208a silencing attenuates doxorubicin induced myocyte apoptosis and cardiac dysfunction. Oxid Med Cell Longev. (2015) 2015:597032. doi: 10.1155/2015/597032
25. Horie T, Ono K, Nishi H, Nagao K, Kinoshita M, Watanabe S, et al. Acute doxorubicin cardiotoxicity is associated with miR-146a-induced inhibition of the neuregulin-ErbB pathway. Cardiovasc Res. (2010) 87:656–64. doi: 10.1093/cvr/cvq148
26. Pan JA, Tang Y, Yu JY, Zhang H, Zhang JF, Wang CQ, et al. miR-146a attenuates apoptosis and modulates autophagy by targeting TAF9b/P53 pathway in doxorubicin-induced cardiotoxicity. Cell Death Dis. (2019) 10:668. doi: 10.1038/s41419-019-1901-x
27. Pan Y, Pan YM, Liu FT, Xu SL, Gu JT, Hang PZ, et al. MicroRNA-98 ameliorates doxorubicin-induced cardiotoxicity via regulating caspase-8 dependent Fas/RIP3 pathway. Environ Toxicol Pharmacol. (2021) 85:103624. doi: 10.1016/j.etap.2021.103624
28. Henderson J, Dubey PK, Patil M, Singh S, Dubey S, Namakkal Soorappan R, et al. microRNA-377 Signaling Modulates Anticancer Drug-Induced Cardiotoxicity in Mice. Front Cardiovasc Med. (2021) 8:737826. doi: 10.3389/fcvm.2021.737826
29. Zhang H, Tian Y, Liang D, Fu Q, Jia L, Wu D, et al. The Effects of Inhibition of MicroRNA-375 in a Mouse Model of Doxorubicin-Induced Cardiac Toxicity. Med Sci Monit. (2020) 26:e920557. doi: 10.12659/MSM.920557
30. Xu C, Liu CH, Zhang DL. MicroRNA-22 inhibition prevents doxorubicin-induced cardiotoxicity via upregulating SIRT1. Biochem Biophys Res Commun. (2020) 521:485–91. doi: 10.1016/j.bbrc.2019.10.140
31. Liu Y, Li Y, Ni J, Shu Y, Wang H, Hu T. MiR-124 attenuates doxorubicin-induced cardiac injury via inhibiting p66Shc-mediated oxidative stress. Biochem Biophys Res Commun. (2020) 521:420–6. doi: 10.1016/j.bbrc.2019.10.157
32. Li XQ, Liu YK Yi J, Dong JS, Zhang PP, Wan L, et al. MicroRNA-143 Increases oxidative stress and myocardial cell apoptosis in a mouse model of doxorubicin-induced cardiac toxicity. Med Sci Monit. (2020) 26:e920394. doi: 10.12659/MSM.920394
33. Li J, Wan W, Chen T, Tong S, Jiang X, Liu W. miR-451 Silencing inhibited doxorubicin exposure-induced cardiotoxicity in mice. Biomed Res Int. (2019) 2019:1528278. doi: 10.1155/2019/1528278
34. Hu X, Liu H, Wang Z, Hu Z, Li L. miR-200a attenuated doxorubicin-induced cardiotoxicity through upregulation of Nrf2 in mice. Oxid Med Cell Longev. (2019) 2019:1512326. doi: 10.1155/2019/1512326
35. Zhao L, Tao X, Qi Y, Xu L, Yin L, Peng J. Protective effect of dioscin against doxorubicin-induced cardiotoxicity via adjusting microRNA-140-5p-mediated myocardial oxidative stress. Redox Biol. (2018) 16:189–98. doi: 10.1016/j.redox.2018.02.026
36. Zhao L, Qi Y, Xu L, Tao X, Han X, Yin L, et al. MicroRNA-140-5p aggravates doxorubicin-induced cardiotoxicity by promoting myocardial oxidative stress via targeting Nrf2 and Sirt2. Redox Biol. (2018) 15:284–96. doi: 10.1016/j.redox.2017.12.013
37. Wan GX, Cheng L, Qin HL, Zhang YZ, Wang LY, Zhang YG. MiR-15b-5p is Involved in doxorubicin-induced cardiotoxicity via inhibiting Bmpr1a signal in H9c2 cardiomyocyte. Cardiovasc Toxicol. (2019) 19:264–75. doi: 10.1007/s12012-018-9495-6
38. Du J, Hang P, Pan Y, Feng B, Zheng Y, Chen T, et al. Inhibition of miR-23a attenuates doxorubicin-induced mitochondria-dependent cardiomyocyte apoptosis by targeting the PGC-1alpha/Drp1 pathway. Toxicol Appl Pharmacol. (2019) 369:73–81. doi: 10.1016/j.taap.2019.02.016
39. Jing X, Yang J, Jiang L, Chen J, Wang H. MicroRNA-29b regulates the mitochondria-dependent apoptotic pathway by targeting Bax in doxorubicin cardiotoxicity. Cell Physiol Biochem. (2018) 48:692–704. doi: 10.1159/000491896
40. Wang JX, Zhang XJ, Feng C, Sun T, Wang K, Wang Y, et al. MicroRNA-532-3p regulates mitochondrial fission through targeting apoptosis repressor with caspase recruitment domain in doxorubicin cardiotoxicity. Cell Death Dis. (2015) 6:e1677. doi: 10.1038/cddis.2015.41
41. Wan Q, Xu T, Ding W, Zhang X, Ji X, Yu T, et al. miR-499-5p attenuates mitochondrial fission and cell apoptosis via p21 in doxorubicin cardiotoxicity. Front Genet. (2018) 9:734. doi: 10.3389/fgene.2018.00734
42. Li Z, Li H, Liu B, Luo J, Qin X, Gong M, et al. Inhibition of miR-25 attenuates doxorubicin-induced apoptosis, reactive oxygen species production and DNA damage by targeting PTEN. Int J Med Sci. (2020) 17:1415–27. doi: 10.7150/ijms.41980
43. Zhang WB, Lai X, Guo XF. Activation of Nrf2 by miR-152 Inhibits Doxorubicin-induced cardiotoxicity via attenuation of oxidative stress, inflammation, and apoptosis. Oxid Med Cell Longev. (2021) 2021:8860883. doi: 10.1155/2021/8860883
44. Ni J, Liu Y, Kang L, Wang L, Han Z, Wang K, et al. Human trophoblast-derived exosomes attenuate doxorubicin-induced cardiac injury by regulating miR-200b and downstream Zeb1. J Nanobiotechnol. (2020) 18:171. doi: 10.1186/s12951-020-00733-z
45. Guo L, Zheng X, Wang E, Jia X, Wang G, Wen J. Irigenin treatment alleviates doxorubicin (DOX)-induced cardiotoxicity by suppressing apoptosis, inflammation and oxidative stress via the increase of miR-425. Biomed Pharmacother. (2020) 125:109784. doi: 10.1016/j.biopha.2019.109784
46. Pakravan G, Foroughmand AM, Peymani M, Ghaedi K, Hashemi MS, Hajjari M, et al. Downregulation of miR-130a, antagonized doxorubicin-induced cardiotoxicity via increasing the PPARgamma expression in mESCs-derived cardiac cells. Cell Death Dis. (2018) 9:758. doi: 10.1038/s41419-018-0797-1
47. Zhang WC, Yang JH, Liu GH, Yang F, Gong JL, Jia MG, et al. miR-34b/c regulates doxorubicin-induced myocardial cell injury through ITCH. Cell Cycle. (2019) 18:3263–74. doi: 10.1080/15384101.2019.1673618
48. Zhang X, Lv S, Zhang W, Jia Q, Wang L, Ding Y, et al. Shenmai injection improves doxorubicin cardiotoxicity via miR-30a/Beclin 1. Biomed Pharmacother. (2021) 139:111582. doi: 10.1016/j.biopha.2021.111582
49. Du Y, Liu G, Zhao L, Yao R. Protective Effect of miR-204 on doxorubicin-induced cardiomyocyte injury via HMGB1. Oxid Med Cell Longev. (2020) 2020:8819771. doi: 10.1155/2020/8819771
50. Lai L, Chen J, Wang N, Zhu G, Duan X, Ling F. MiRNA-30e mediated cardioprotection of ACE2 in rats with doxorubicin-induced heart failure through inhibiting cardiomyocytes autophagy. Life Sci. (2017) 169:69–75. doi: 10.1016/j.lfs.2016.09.006
51. Piegari E, Cozzolino A, Ciuffreda LP, Cappetta D, De Angelis A, Urbanek K, et al. Cardioprotective effects of miR-34a silencing in a rat model of doxorubicin toxicity. Sci Rep. (2020) 10:12250. doi: 10.1038/s41598-020-69038-3
52. Liu Y, Liu Z, Xie Y, Zhao C, Xu J. Serum extracellular vesicles retard H9C2 cell senescence by suppressing miR-34a expression. J Cardiovasc Transl Res. (2019) 12:45–50. doi: 10.1007/s12265-018-9847-4
53. Xia W, Hou M. Mesenchymal stem cells confer resistance to doxorubicin-induced cardiac senescence by inhibiting microRNA-34a. Oncol Lett. (2018) 15:10037–46. doi: 10.3892/ol.2018.8438
54. Piegari E, Russo R, Cappetta D, Esposito G, Urbanek K, Dell'Aversana C, et al. MicroRNA-34a regulates doxorubicin-induced cardiotoxicity in rat. Oncotarget. (2016) 7:62312–26. doi: 10.18632/oncotarget.11468
55. Sun W, Zhao P, Zhou Y, Xing C, Zhao L, Li Z, et al. Ultrasound targeted microbubble destruction assisted exosomal delivery of miR-21 protects the heart from chemotherapy associated cardiotoxicity. Biochem Biophys Res Commun. (2020) 532:60–7. doi: 10.1016/j.bbrc.2020.05.044
56. Sun H, Tong Z, Fang Y, Jiang B, Liang P, Tang Y, et al. Nucleolin protects against doxorubicin-induced cardiotoxicity via upregulating microRNA-21. J Cell Physiol. (2018) 233:9516–25. doi: 10.1002/jcp.26854
57. Bei Y, Wu X, Cretoiu D, Shi J, Zhou Q, Lin S, et al. miR-21 suppression prevents cardiac alterations induced by d-galactose and doxorubicin. J Mol Cell Cardiol. (2018) 115:130–41. doi: 10.1016/j.yjmcc.2018.01.007
58. Tong Z, Jiang B, Wu Y, Liu Y, Li Y, Gao M, et al. MiR-21 Protected Cardiomyocytes against Doxorubicin-Induced Apoptosis by Targeting BTG2. Int J Mol Sci. (2015) 16:14511–25. doi: 10.3390/ijms160714511
59. Younis NN, Salama A, Shaheen MA, Eissa RG. Pachymic acid attenuated doxorubicin-induced heart failure by suppressing miR-24 and preserving cardiac junctophilin-2 in rats. Int J Mol Sci. (2021) 22:e10710. doi: 10.3390/ijms221910710
60. Wang Y, Cui X, Wang Y, Fu Y, Guo X, Long J, et al. Protective effect of miR378* on doxorubicin-induced cardiomyocyte injury via calumenin. J Cell Physiol. (2018) 233:6344–51. doi: 10.1002/jcp.26615
61. Zhang L, Liu L, Li X. MiR-526b-3p mediates doxorubicin-induced cardiotoxicity by targeting STAT3 to inactivate VEGFA. Biomed Pharmacother. (2020) 123:109751. doi: 10.1016/j.biopha.2019.109751
62. Yin Z, Zhao Y, Li H, Yan M, Zhou L, Chen C, et al. miR-320a mediates doxorubicin-induced cardiotoxicity by targeting VEGF signal pathway. Aging. (2016) 8:192–207. doi: 10.18632/aging.100876
63. Li Z, Ye Z, Ma J, Gu Q, Teng J, Gong X. MicroRNA133b alleviates doxorubicininduced cardiomyocyte apoptosis and cardiac fibrosis by targeting PTBP1 and TAGLN2. Int J Mol Med. (2021) 48:4958. doi: 10.3892/ijmm.2021.4958
64. Lei B, Wu X, Xia K, Sun H, Wang J. Exosomal Micro-RNA-96 derived from bone marrow mesenchymal stem cells inhibits doxorubicin-induced myocardial toxicity by inhibiting the Rac1/Nuclear factor-kappaB signaling pathway. J Am Heart Assoc. (2021) 10:e020589. doi: 10.1161/JAHA.120.020589
65. Qin M, Li Q, Wang Y, Li T, Gu Z, Huang P, et al. Rutin treats myocardial damage caused by pirarubicin via regulating miR-22-5p-regulated RAP1/ERK signaling pathway. J Biochem Mol Toxicol. (2021) 35:e22615. doi: 10.1002/jbt.22615
66. Li Q, Qin M, Li T, Gu Z, Tan Q, Huang P, et al. Rutin protects against pirarubicin-induced cardiotoxicity by adjusting microRNA-125b-1-3p-mediated JunD signaling pathway. Mol Cell Biochem. (2020) 466:139–48. doi: 10.1007/s11010-020-03696-9
67. Li Q, Qin M, Tan Q, Li T, Gu Z, Huang P, et al. MicroRNA-129-1-3p protects cardiomyocytes from pirarubicin-induced apoptosis by down-regulating the GRIN2D-mediated Ca(2+) signalling pathway. J Cell Mol Med. (2020) 24:2260–71. doi: 10.1111/jcmm.14908
68. Wu J, Sun C, Wang R, Li J, Zhou M, Yan M, et al. Cardioprotective effect of paeonol against epirubicin-induced heart injury via regulating miR-1 and PI3K/AKT pathway. Chem Biol Interact. (2018) 286:17–25. doi: 10.1016/j.cbi.2018.02.035
69. Wu Y, Xiu W, Wu Y. Salvianolic Acid A Protects H9C2 Cardiomyocytes from doxorubicin-induced damage by inhibiting NFKB1 expression thereby downregulating long-noncoding RNA (lncRNA) plasmacytoma variant translocation 1 (PVT1). Med Sci Monit. (2021) 27:e929824. doi: 10.12659/MSM.929824
70. Zhan J, Hu P, Wang Y. lncRNA PVT1 aggravates doxorubicin-induced cardiomyocyte apoptosis by targeting the miR-187-3p/AGO1 axis. Mol Cell Probes. (2020) 49:101490. doi: 10.1016/j.mcp.2019.101490
71. Wang H, Lin X, Li J, Zeng G, Xu T. Long noncoding RNA SOX2-OT aggravates doxorubicin-induced apoptosis of cardiomyocyte by targeting miR-942-5p/DP5. Drug Des Devel Ther. (2021) 15:481–92. doi: 10.2147/DDDT.S267474
72. Lai L, Xu Y, Kang L, Yang J, Zhu G. LncRNA KCNQ1OT1 contributes to cardiomyocyte apoptosis by targeting FUS in heart failure. Exp Mol Pathol. (2020) 115:104480. doi: 10.1016/j.yexmp.2020.104480
73. Zhuang S, Ma Y, Zeng Y, Lu C, Yang F, Jiang N, et al. METTL14 promotes doxorubicin-induced cardiomyocyte ferroptosis by regulating the KCNQ1OT1-miR-7-5p-TFRC axis. Cell Biol Toxicol. (2021). doi: 10.1007/s10565-021-09660-7
74. Chen H, Liu J, Wang B, Li Y. Protective effect of lncRNA CRNDE on myocardial cell apoptosis in heart failure by regulating HMGB1 cytoplasm translocation through PARP-1. Arch Pharm Res. (2020) 43:1325–34. doi: 10.1007/s12272-020-01290-7
75. Liu Y, Duan C, Liu W, Chen X, Wang Y, Liu X, et al. Upregulation of let-7f-2-3p by long noncoding RNA NEAT1 inhibits XPO1-mediated HAX-1 nuclear export in both in vitro and in vivo rodent models of doxorubicin-induced cardiotoxicity. Arch Toxicol. (2019) 93:3261–76. doi: 10.1007/s00204-019-02586-4
76. Chen S, Wang J, Zhou Y. Long non-coding RNA SNHG1 protects human AC16 cardiomyocytes from doxorubicin toxicity by regulating miR-195/Bcl-2 axis. Biosci Rep. (2019) 39:BSR20191050. doi: 10.1042/BSR20191050
77. Li J, Li L, Li X, Wu S. Long noncoding RNA LINC00339 aggravates doxorubicin-induced cardiomyocyte apoptosis by targeting MiR-484. Biochem Biophys Res Commun. (2018) 503:3038–43. doi: 10.1016/j.bbrc.2018.08.090
78. Li HQ, Wu YB, Yin CS, Chen L, Zhang Q, Hu LQ. Obestatin attenuated doxorubicin-induced cardiomyopathy via enhancing long noncoding Mhrt RNA expression. Biomed Pharmacother. (2016) 81:474–81. doi: 10.1016/j.biopha.2016.04.017
79. Aung LHH, Chen X, Cueva Jumbo JC Li Z, Wang SY, Zhao C, et al. Cardiomyocyte mitochondrial dynamic-related lncRNA 1 (CMDL-1) may serve as a potential therapeutic target in doxorubicin cardiotoxicity. Mol Therapy Nucleic acids. (2021) 25:638–51. doi: 10.1016/j.omtn.2021.08.006
80. Xia W, Chen H, Xie C, Hou M. Long-noncoding RNA MALAT1 sponges microRNA-92a-3p to inhibit doxorubicin-induced cardiac senescence by targeting ATG4a. Aging. (2020) 12:8241–60. doi: 10.18632/aging.103136
81. Zhang S, Yuan Y, Zhang Z, Guo J, Li J, Zhao K, et al. LncRNA FOXC2-AS1 protects cardiomyocytes from doxorubicin-induced cardiotoxicity through activation of WNT1-inducible signaling pathway protein-1. Biosci Biotechnol Biochem. (2019) 83:653–8. doi: 10.1080/09168451.2018.1553606
82. Xie Z, Xia W, Hou M. Long intergenic noncoding RNAp21 mediates cardiac senescence via the Wnt/betacatenin signaling pathway in doxorubicin-induced cardiotoxicity. Mol Med Rep. (2018) 17:2695–704. doi: 10.3892/mmr.2017.8169
83. Xing X, Tan Z, Zhi X, Sun H, Yang J, Li L, et al. Integrating analysis of circular RNA and mRNA expression profiles in doxorubicin induced cardiotoxicity mice. J Appl Toxicol. (2021). doi: 10.1002/jat.4257
84. Li B, Cai X, Wang Y, Zhu H, Zhang P, Jiang P, et al. Circ-SKA3 Enhances doxorubicin toxicity in AC16 cells through miR-1303/TLR4 axis. Int Heart J. (2021) 62:1112–23. doi: 10.1536/ihj.20-809
85. Gupta SK, Garg A, Bar C, Chatterjee S, Foinquinos A, Milting H, et al. Quaking inhibits doxorubicin-mediated cardiotoxicity through regulation of cardiac circular RNA expression. Circ Res. (2018) 122:246–54. doi: 10.1161/CIRCRESAHA.117.311335
86. Wang X, Cheng Z, Xu J, Feng M, Zhang H, Zhang L, et al. Circular RNA Arhgap12 modulates doxorubicin-induced cardiotoxicity by sponging miR-135a-5p. Life Sci. (2021) 265:118788. doi: 10.1016/j.lfs.2020.118788
87. Han D, Wang Y, Wang Y, Dai X, Zhou T, Chen J, et al. The tumor-suppressive human circular RNA CircITCH sponges miR-330-5p to ameliorate doxorubicin-induced cardiotoxicity through upregulating SIRT6, Survivin, and SERCA2a. Circ Res. (2020) 127:e108–e25. doi: 10.1161/CIRCRESAHA.119.316061
88. Qiu Y, Xie X, Lin L. circFOXO3 protects cardiomyocytes against radiation induced cardiotoxicity. Mol Med Rep. (2021) 23:e11816. doi: 10.3892/mmr.2020.11816
89. Hu Y, Xia W, Hou M. Macrophage migration inhibitory factor serves a pivotal role in the regulation of radiation-induced cardiac senescence through rebalancing the microRNA-34a/sirtuin 1 signaling pathway. Int J Mol Med. (2018) 42:2849–58. doi: 10.3892/ijmm.2018.3838
90. Chen XX, Jiang YJ, Zeng T, Li JJ. Overexpression of the long noncoding RNA NEAT1 protects against As2O3-induced injury of cardiomyocyte by inhibiting the miR-124/NF-kappaB signaling pathway. Eur Rev Med Pharmacol Sci. (2020) 24:1378–90. doi: 10.26355/eurrev_202002_20195
91. Zhao X, Shi YQ, Yan CC, Feng PF, Wang X, Zhang R, et al. Up-regulation of miR-21 and miR-23a contributes to As2 O3-induced hERG channel deficiency. Basic Clin Pharmacol Toxicol. (2015) 116:516–23. doi: 10.1111/bcpt.12348
92. Shan H, Zhang Y, Cai B, Chen X, Fan Y, Yang L, et al. Upregulation of microRNA-1 and microRNA-133 contributes to arsenic-induced cardiac electrical remodeling. Int J Cardiol. (2013) 167:2798–805. doi: 10.1016/j.ijcard.2012.07.009
93. Chen XY, Huang WL, Peng XP, Lv YN Li JH, Xiong JP. miR-140-5p mediates bevacizumab-induced cytotoxicity to cardiomyocytes by targeting the VEGFA/14-3-3gamma signal pathway. Toxicol Res. (2019) 8:875–84. doi: 10.1039/c9tx00100j
94. Zhang J, Chintalgattu V, Shih T, Ai D, Xia Y, Khakoo AY. MicroRNA-9 is an activation-induced regulator of PDGFR-beta expression in cardiomyocytes. J Mol Cell Cardiol. (2011) 51:337–46. doi: 10.1016/j.yjmcc.2011.05.019
95. Wenningmann N, Knapp M, Ande A, Vaidya TR, Ait-Oudhia S. Insights into doxorubicin-induced cardiotoxicity: molecular mechanisms, preventive strategies, and early monitoring. Mol Pharmacol. (2019) 96:219–32. doi: 10.1124/mol.119.115725
96. Poulose N, Raju R. Sirtuin regulation in aging and injury. Biochim Biophys Acta. (2015) 1852:2442–55. doi: 10.1016/j.bbadis.2015.08.017
97. Migliaccio E, Giorgio M, Mele S, Pelicci G, Reboldi P, Pandolfi PP, et al. The p66shc adaptor protein controls oxidative stress response and life span in mammals. Nature. (1999) 402:309–13. doi: 10.1038/46311
98. Goormaghtigh E, Chatelain P, Caspers J, Ruysschaert JM. Evidence of a complex between adriamycin derivatives and cardiolipin: possible role in cardiotoxicity. Biochem Pharmacol. (1980) 29:3003–10. doi: 10.1016/0006-2952(80)90050-7
99. Chen Y, Liu Y, Dorn GW 2nd. Mitochondrial fusion is essential for organelle function and cardiac homeostasis. Circul Res. (2011) 109:1327–31. doi: 10.1161/CIRCRESAHA.111.258723
100. Suen DF, Norris KL, Youle RJ. Mitochondrial dynamics and apoptosis. Genes Dev. (2008) 22:1577–90. doi: 10.1101/gad.1658508
101. Childs AC, Phaneuf SL, Dirks AJ, Phillips T, Leeuwenburgh C. Doxorubicin treatment in vivo causes cytochrome C release and cardiomyocyte apoptosis, as well as increased mitochondrial efficiency, superoxide dismutase activity, and Bcl-2:Bax ratio. Cancer Res. (2002) 62:4592–8. Available online at: https://aacrjournals.org/cancerres/article/62/16/4592/509147/Doxorubicin-Treatment-in-Vivo-Causes-Cytochrome-c
102. Kuwana T, Newmeyer DD. Bcl-2-family proteins and the role of mitochondria in apoptosis. Curr Opin Cell Biol. (2003) 15:691–9. doi: 10.1016/j.ceb.2003.10.004
103. Park S, Jang JW, Moon EY. BAFF attenuates oxidative stress-induced cell death by the regulation of mitochondria membrane potential via Syk activation in WiL2-NS B lymphoblasts. Sci Rep. (2020) 10:1–12. doi: 10.1038/s41598-020-68628-5
104. Liu D, Ma Z, Di S, Yang Y, Yang J, Xu L, et al. AMPK/PGC1alpha activation by melatonin attenuates acute doxorubicin cardiotoxicity via alleviating mitochondrial oxidative damage and apoptosis. Free Radic Biol Med. (2018) 129:59–72. doi: 10.1016/j.freeradbiomed.2018.08.032
105. Sun LY, Wang N, Ban T, Sun YH, Han Y, Sun LL, et al. MicroRNA-23a mediates mitochondrial compromise in estrogen deficiency-induced concentric remodeling via targeting PGC-1alpha. J Mol Cell Cardiol. (2014) 75:1–11. doi: 10.1016/j.yjmcc.2014.06.012
106. Frank S, Gaume B, Bergmann-Leitner ES, Leitner WW, Robert EG, Catez F, et al. The role of dynamin-related protein 1, a mediator of mitochondrial fission, in apoptosis. Dev Cell. (2001) 1:515–25. doi: 10.1016/S1534-5807(01)00055-7
107. Battogtokh G, Choi YS, Kang DS, Park SJ, Shim MS, Huh KM, et al. Mitochondria-targeting drug conjugates for cytotoxic, anti-oxidizing and sensing purposes: current strategies and future perspectives. Acta Pharm Sin B. (2018) 8:862–80. doi: 10.1016/j.apsb.2018.05.006
108. Zhang X, Hu C, Kong CY, Song P, Wu HM, Xu SC, et al. FNDC5 alleviates oxidative stress and cardiomyocyte apoptosis in doxorubicin-induced cardiotoxicity via activating AKT. Cell Death Differ. (2020) 27:540–55. doi: 10.1038/s41418-019-0372-z
109. Hussain S, Khan AW, Akhmedov A, Suades R, Costantino S, Paneni F, et al. Hyperglycemia induces myocardial dysfunction via epigenetic regulation of JunD. Circ Res. (2020) 127:1261–73. doi: 10.1161/CIRCRESAHA.120.317132
110. L'Ecuyer T, Sanjeev S, Thomas R, Novak R, Das L, Campbell W, et al. DNA damage is an early event in doxorubicin-induced cardiac myocyte death. Am J Physiol Heart Circ Physiol. (2006) 291:H1273–80. doi: 10.1152/ajpheart.00738.2005
111. Minotti G, Menna P, Salvatorelli E, Cairo G, Gianni L. Anthracyclines: molecular advances and pharmacologic developments in antitumor activity and cardiotoxicity. Pharmacol Rev. (2004) 56:185–229. doi: 10.1124/pr.56.2.6
112. Bassi C, Ho J, Srikumar T, Dowling RJ, Gorrini C, Miller SJ, et al. Nuclear PTEN controls DNA repair and sensitivity to genotoxic stress. Science. (2013) 341:395–9. doi: 10.1126/science.1236188
113. Pecoraro M, Del Pizzo M, Marzocco S, Sorrentino R, Ciccarelli M, Iaccarino G, et al. Inflammatory mediators in a short-time mouse model of doxorubicin-induced cardiotoxicity. Toxicol Appl Pharmacol. (2016) 293:44–52. doi: 10.1016/j.taap.2016.01.006
114. Gordon JW, Shaw JA, Kirshenbaum LA. Multiple facets of NF-kappaB in the heart: to be or not to NF-kappaB. Circ Res. (2011) 108:1122–32. doi: 10.1161/CIRCRESAHA.110.226928
115. Ren Y, Sun C, Sun Y, Tan H, Wu Y, Cui B, et al. PPAR gamma protects cardiomyocytes against oxidative stress and apoptosis via Bcl-2 upregulation. Vascul Pharmacol. (2009) 51:169–74. doi: 10.1016/j.vph.2009.06.004
116. Yamamoto K, Ohki R, Lee RT, Ikeda U, Shimada K. Peroxisome proliferator-activated receptor gamma activators inhibit cardiac hypertrophy in cardiac myocytes. Circulation. (2001) 104:1670–5. doi: 10.1161/hc4001.097186
117. Ofengeim D, Yuan J. Regulation of RIP1 kinase signalling at the crossroads of inflammation and cell death. Nat Rev Mol Cell Biol. (2013) 14:727–36. doi: 10.1038/nrm3683
118. Balogun E, Hoque M, Gong P, Killeen E, Green CJ, Foresti R, et al. Curcumin activates the haem oxygenase-1 gene via regulation of Nrf2 and the antioxidant-responsive element. Biochem J. (2003) 371(Pt 3):887–95. doi: 10.1042/bj20021619
119. Bravo-San Pedro JM, Kroemer G, Galluzzi L. Autophagy and mitophagy in cardiovascular disease. Circ Res. (2017) 120:1812–24. doi: 10.1161/CIRCRESAHA.117.311082
120. Simabuco FM, Morale MG, Pavan ICB, Morelli AP, Silva FR, Tamura RE. p53 and metabolism: from mechanism to therapeutics. Oncotarget. (2018) 9:23780–823. doi: 10.18632/oncotarget.25267
121. Pan W, Zhong Y, Cheng C, Liu B, Wang L, Li A, et al. MiR-30-regulated autophagy mediates angiotensin II-induced myocardial hypertrophy. PLoS ONE. (2013) 8:e53950. doi: 10.1371/journal.pone.0053950
122. Ulloa L, Messmer D. High-mobility group box 1 (HMGB1) protein: friend and foe. Cytokine Growth Factor Rev. (2006) 17:189–201. doi: 10.1016/j.cytogfr.2006.01.003
123. Venkatachalam G, Surana U, Clement MV. Replication stress-induced endogenous DNA damage drives cellular senescence induced by a sub-lethal oxidative stress. Nucleic Acids Res. (2017) 45:10564–82. doi: 10.1093/nar/gkx684
124. Yi J, Luo J. SIRT1 and p53, effect on cancer, senescence and beyond. Biochim Biophys Acta. (2010) 1804:1684–9. doi: 10.1016/j.bbapap.2010.05.002
125. Bernadotte A, Mikhelson VM, Spivak IM. Markers of cellular senescence. Telomere shortening as a marker of cellular senescence. Aging. (2016) 8:3–11. doi: 10.18632/aging.100871
126. De Bonis ML, Ortega S, Blasco MA. SIRT1 is necessary for proficient telomere elongation and genomic stability of induced pluripotent stem cells. Stem Cell Rep. (2014) 2:690–706. doi: 10.1016/j.stemcr.2014.03.002
127. Boon RA, Iekushi K, Lechner S, Seeger T, Fischer A, Heydt S, et al. MicroRNA-34a regulates cardiac ageing and function. Nature. (2013) 495:107–10. doi: 10.1038/nature11919
128. Lewis-McDougall FC, Ruchaya PJ, Domenjo-Vila E, Shin Teoh T, Prata L, Cottle BJ, et al. Aged-senescent cells contribute to impaired heart regeneration. Aging Cell. (2019) 18:e12931. doi: 10.1111/acel.12931
129. Lim CC, Zuppinger C, Guo X, Kuster GM, Helmes M, Eppenberger HM, et al. Anthracyclines induce calpain-dependent titin proteolysis and necrosis in cardiomyocytes. J Biol Chem. (2004) 279:8290–9. doi: 10.1074/jbc.M308033200
130. Mazzorana M, Hussain R, Sorensen T. Ca-dependent folding of human calumenin. PLoS ONE. (2016) 11:e0151547. doi: 10.1371/journal.pone.0151547
131. Zhang M, Prosser BL, Bamboye MA, Gondim ANS, Santos CX, Martin D, et al. Contractile function during angiotensin-II activation: increased Nox2 activity modulates cardiac calcium handling via phospholamban phosphorylation. J Am Coll Cardiol. (2015) 66:261–72. doi: 10.1016/j.jacc.2015.05.020
132. Guo A, Wang Y, Chen B, Wang Y, Yuan J, Zhang L, et al. E-C coupling structural protein junctophilin-2 encodes a stress-adaptive transcription regulator. Science. (2018) 362:6421. doi: 10.1126/science.aan3303
133. Bers DM. Cardiac excitation-contraction coupling. Nature. (2002) 415:198–205. doi: 10.1038/415198a
134. Hajdu T, Juhasz T, Szucs-Somogyi C, Racz K, Zakany R. NR1 and NR3B composed intranuclear N-methyl-d-aspartate receptor complexes in human melanoma cells. Int J Mol Sci. (2018) 19:1929. doi: 10.3390/ijms19071929
135. Flood EC, Hajjar KA. The annexin A2 system and vascular homeostasis. Vascul Pharmacol. (2011) 54:59–67. doi: 10.1016/j.vph.2011.03.003
136. Taimeh Z, Loughran J, Birks EJ, Bolli R. Vascular endothelial growth factor in heart failure. Nat Rev Cardiol. (2013) 10:519–30. doi: 10.1038/nrcardio.2013.94
137. Cappetta D, Esposito G, Piegari E, Russo R, Ciuffreda LP, Rivellino A, et al. SIRT1 activation attenuates diastolic dysfunction by reducing cardiac fibrosis in a model of anthracycline cardiomyopathy. Int J Cardiol. (2016) 205:99–110. doi: 10.1016/j.ijcard.2015.12.008
138. Chen J, Wu Y, Luo X, Jin D, Zhou W, Ju Z, et al. Circular RNA circRHOBTB3 represses metastasis by regulating the HuR-mediated mRNA stability of PTBP1 in colorectal cancer. Theranostics. (2021) 11:7507–26. doi: 10.7150/thno.59546
139. Kim HR, Park JS, Park JH, Yasmin F, Kim CH, Oh SK, et al. Cell-permeable transgelin-2 as a potent therapeutic for dendritic cell-based cancer immunotherapy. J Hematol Oncol. (2021) 14:43. doi: 10.1186/s13045-021-01058-6
140. Cory S, Adams JM. The Bcl2 family: regulators of the cellular life-or-death switch. Nat Rev Cancer. (2002) 2:647–56. doi: 10.1038/nrc883
141. Grzybowska EA, Zayat V, Konopinski R, Trebinska A, Szwarc M, Sarnowska E, et al. HAX-1 is a nucleocytoplasmic shuttling protein with a possible role in mRNA processing. FEBS J. (2013) 280:256–72. doi: 10.1111/febs.12066
142. Jans DA, Martin AJ, Wagstaff KM. Inhibitors of nuclear transport. Curr Opin Cell Biol. (2019) 58:50–60. doi: 10.1016/j.ceb.2019.01.001
143. Parisi C, Giorgi C, Batassa EM, Braccini L, Maresca G, D'Agnano I, et al. Ago1 and Ago2 differentially affect cell proliferation, motility and apoptosis when overexpressed in SH-SY5Y neuroblastoma cells. FEBS Lett. (2011) 585:2965–71. doi: 10.1016/j.febslet.2011.08.003
144. Wang M, Zhang L, Liu Z, Zhou J, Pan Q, Fan J, et al. AGO1 may influence the prognosis of hepatocellular carcinoma through TGF-beta pathway. Cell Death Dis. (2018) 9:324. doi: 10.1038/s41419-018-0338-y
145. Cereghetti GM, Stangherlin A, Martins de, Brito O, Chang CR, Blackstone C, Bernardi P, et al. Dephosphorylation by calcineurin regulates translocation of Drp1 to mitochondria. Proc Natl Acad Sci USA. (2008) 105:15803–8. doi: 10.1073/pnas.0808249105
146. Przybylska D, Janiszewska D, Gozdzik A, Bielak-Zmijewska A, Sunderland P, Sikora E, et al. NOX4 downregulation leads to senescence of human vascular smooth muscle cells. Oncotarget. (2016) 7:66429–43. doi: 10.18632/oncotarget.12079
147. Schworer S, Becker F, Feller C, Baig AH, Kober U, Henze H, et al. Epigenetic stress responses induce muscle stem-cell ageing by Hoxa9 developmental signals. Nature. (2016) 540:428–32. doi: 10.1038/nature20603
148. Dixon SJ, Lemberg KM, Lamprecht MR, Skouta R, Zaitsev EM, Gleason CE, et al. Ferroptosis: an iron-dependent form of nonapoptotic cell death. Cell. (2012) 149:1060–72. doi: 10.1016/j.cell.2012.03.042
149. Gao M, Monian P, Quadri N, Ramasamy R, Jiang X. Glutaminolysis and transferrin regulate ferroptosis. Mol Cell. (2015) 59:298–308. doi: 10.1016/j.molcel.2015.06.011
150. Conn SJ, Pillman KA, Toubia J, Conn VM, Salmanidis M, Phillips CA, et al. The RNA binding protein quaking regulates formation of circRNAs. Cell. (2015) 160:1125–34. doi: 10.1016/j.cell.2015.02.014
151. Kuzmich NN, Sivak KV, Chubarev VN, Porozov YB, Savateeva-Lyubimova TN, Peri F. TLR4 signaling pathway modulators as potential therapeutics in inflammation and sepsis. Vaccines (Basel). (2017) 5:34. doi: 10.3390/vaccines5040034
152. Jahng JWS, Liu L, Wu JC. Tumor repressor circular RNA as a new target for preventative gene therapy against doxorubicin-induced cardiotoxicity. Circ Res. (2020) 127:483–5. doi: 10.1161/CIRCRESAHA.120.317568
153. Del Monte F, Harding SE, Schmidt U, Matsui T, Kang ZB, Dec GW, et al. Restoration of contractile function in isolated cardiomyocytes from failing human hearts by gene transfer of SERCA2a. Circulation. (1999) 100:2308–11. doi: 10.1161/01.CIR.100.23.2308
154. Levkau B, Schafers M, Wohlschlaeger J, von Wnuck Lipinski K, Keul P, Hermann S, et al. Survivin determines cardiac function by controlling total cardiomyocyte number. Circulation. (2008) 117:1583–93. doi: 10.1161/CIRCULATIONAHA.107.734160
155. Mao Z, Hine C, Tian X, Van Meter M, Au M, Vaidya A, et al. SIRT6 promotes DNA repair under stress by activating PARP1. Science. (2011) 332:1443–6. doi: 10.1126/science.1202723
156. Pan H, Guan D, Liu X, Li J, Wang L, Wu J, et al. SIRT6 safeguards human mesenchymal stem cells from oxidative stress by coactivating NRF2. Cell Res. (2016) 26:190–205. doi: 10.1038/cr.2016.4
157. Darby SC, Cutter DJ, Boerma M, Constine LS, Fajardo LF, Kodama K, et al. Radiation-related heart disease: current knowledge and future prospects. Int J Radiat Oncol Biol Phys. (2010) 76:656–65. doi: 10.1016/j.ijrobp.2009.09.064
158. Merk M, Mitchell RA, Endres S, Bucala R. D-dopachrome tautomerase (D-DT or MIF-2): doubling the MIF cytokine family. Cytokine. (2012) 59:10–7. doi: 10.1016/j.cyto.2012.03.014
159. Zhang C, Wang J, Ma X, Wang W, Zhao B, Chen Y, et al. ACE2-EPC-EXs protect ageing ECs against hypoxia/reoxygenation-induced injury through the miR-18a/Nox2/ROS pathway. J Cell Mol Med. (2018) 22:1873–82. doi: 10.1111/jcmm.13471
160. Westervelt P, Brown RA, Adkins DR, Khoury H, Curtin P, Hurd D, et al. Sudden death among patients with acute promyelocytic leukemia treated with arsenic trioxide. Blood. (2001) 98:266–71. doi: 10.1182/blood.V98.2.266
161. Dhamoon AS, Jalife J. The inward rectifier current (IK1) controls cardiac excitability and is involved in arrhythmogenesis. Heart Rhythm. (2005) 2:316–24. doi: 10.1016/j.hrthm.2004.11.012
162. Curran ME, Splawski I, Timothy KW, Vincent GM, Green ED, Keating MT, et al. molecular basis for cardiac arrhythmia: HERG mutations cause long QT syndrome. Cell. (1995) 80:795–803. doi: 10.1016/0092-8674(95)90358-5
163. Zhang Y, Dong Z, Jin L, Zhang K, Zhao X, Fu J, et al. Arsenic trioxide-induced hERG K(+) channel deficiency can be rescued by matrine and oxymatrine through up-regulating transcription factor Sp1 expression. Biochem Pharmacol. (2013) 85:59–68. doi: 10.1016/j.bcp.2012.09.002
164. Economopoulou P, Kotsakis A, Kapiris I, Kentepozidis N. Cancer therapy and cardiovascular risk: focus on bevacizumab. Cancer Manag Res. (2015) 7:133–43. doi: 10.2147/CMAR.S77400
165. Jain RK. Normalizing tumor vasculature with anti-angiogenic therapy: a new paradigm for combination therapy. Nat Med. (2001) 7:987–9. doi: 10.1038/nm0901-987
166. Kleppe R, Martinez A, Doskeland SO, Haavik J. The 14-3-3 proteins in regulation of cellular metabolism. Semin Cell Dev Biol. (2011) 22:713–9. doi: 10.1016/j.semcdb.2011.08.008
167. Freres P, Bouznad N, Servais L, Josse C, Wenric S, Poncin A, et al. Variations of circulating cardiac biomarkers during and after anthracycline-containing chemotherapy in breast cancer patients. BMC Cancer. (2018) 18:102. doi: 10.1186/s12885-018-4015-4
168. Oatmen KE, Toro-Salazar OH, Hauser K, Zellars KN, Mason KC, Hor K, et al. Identification of a novel microRNA profile in pediatric patients with cancer treated with anthracycline chemotherapy. Am J Physiol Heart Circ Physiol. (2018) 315:H1443–H52. doi: 10.1152/ajpheart.00252.2018
169. Leger KJ, Leonard D, Nielson D, de Lemos JA, Mammen PP, Winick NJ. Circulating microRNAs: Potential markers of cardiotoxicity in children and young adults treated with anthracycline chemotherapy. J Am Heart Assoc. (2017) 6:e004653. doi: 10.1161/JAHA.116.004653
170. Beaumier A, Robinson SR, Robinson N, Lopez KE, Meola DM, Barber LG, et al. Extracellular vesicular microRNAs as potential biomarker for early detection of doxorubicin-induced cardiotoxicity. J Vet Internal Med. (2020) 34:1260–71. doi: 10.1111/jvim.15762
171. Ruggeri C, Gioffre S, Chiesa M, Buzzetti M, Milano G, Scopece A, et al. A Specific circulating microRNA cluster is associated to late differential cardiac response to doxorubicin-induced cardiotoxicity in vivo. Dis Markers. (2018) 2018:8395651. doi: 10.1155/2018/8395651
172. Rigaud VO, Ferreira LR, Ayub-Ferreira SM, Avila MS, Brandao SM, Cruz FD, et al. Circulating miR-1 as a potential biomarker of doxorubicin-induced cardiotoxicity in breast cancer patients. Oncotarget. (2017) 8:6994–7002. doi: 10.18632/oncotarget.14355
173. Chaudhari U, Nemade H, Gaspar JA, Hescheler J, Hengstler JG, Sachinidis A. MicroRNAs as early toxicity signatures of doxorubicin in human-induced pluripotent stem cell-derived cardiomyocytes. Arch Toxicol. (2016) 90:3087–98. doi: 10.1007/s00204-016-1668-0
174. Desai VG, J CK, Vijay V, Moland CL, Herman EH, Lee T, et al. Early biomarkers of doxorubicin-induced heart injury in a mouse model. Toxicol Appl Pharmacol. (2014) 281:221–9. doi: 10.1016/j.taap.2014.10.006
175. Vacchi-Suzzi C, Bauer Y, Berridge BR, Bongiovanni S, Gerrish K, Hamadeh HK, et al. Perturbation of microRNAs in rat heart during chronic doxorubicin treatment. PLoS ONE. (2012) 7:e40395. doi: 10.1371/journal.pone.0040395
176. Qin X, Chang F, Wang Z, Jiang W. Correlation of circulating pro-angiogenic miRNAs with cardiotoxicity induced by epirubicin/cyclophosphamide followed by docetaxel in patients with breast cancer. Cancer Biomark. (2018) 23:473–84. doi: 10.3233/CBM-181301
177. Aryankalayil MJ, Martello S, Bylicky MA, Chopra S, May JM, Shankardass A, et al. Analysis of lncRNA-miRNA-mRNA expression pattern in heart tissue after total body radiation in a mouse model. J Transl Med. (2021) 19:336. doi: 10.1186/s12967-021-02998-w
178. Hawkins PG, Sun Y, Dess RT, Jackson WC, Sun G, Bi N, et al. Circulating microRNAs as biomarkers of radiation-induced cardiac toxicity in non-small-cell lung cancer. J Cancer Res Clin Oncol. (2019) 145:1635–43. doi: 10.1007/s00432-019-02903-5
179. Esplugas R, Arenas M, Serra N, Belles M, Bonet M, Gascon M, et al. Effect of radiotherapy on the expression of cardiovascular disease-related miRNA-146a,−155,−221 and−222 in blood of women with breast cancer. PLoS One. (2019) 14:e0217443. doi: 10.1371/journal.pone.0217443
180. Zhou F, Lu X, Zhang X. Serum miR-30c level predicted cardiotoxicity in non-small cell lung cancer patients treated with bevacizumab. Cardiovasc Toxicol. (2018) 18:284–9. doi: 10.1007/s12012-018-9457-z
181. Zhao Z, He J, Zhang J, Liu M, Yang S, Li N, et al. Dysregulated miR1254 and miR579 for cardiotoxicity in patients treated with bevacizumab in colorectal cancer. Tumour Biol. (2014) 35:5227–35. doi: 10.1007/s13277-014-1679-5
182. Mitchell PS, Parkin RK, Kroh EM, Fritz BR, Wyman SK, Pogosova-Agadjanyan EL, et al. Circulating microRNAs as stable blood-based markers for cancer detection. Proc Natl Acad Sci U S A. (2008) 105:10513–8. doi: 10.1073/pnas.0804549105
183. Oliveira-Carvalho V, Ferreira LR, Bocchi EA. Circulating mir-208a fails as a biomarker of doxorubicin-induced cardiotoxicity in breast cancer patients. J Appl Toxicol. (2015) 35:1071–2. doi: 10.1002/jat.3185
184. Nishimura Y, Kondo C, Morikawa Y, Tonomura Y, Torii M, Yamate J, et al. Plasma miR-208 as a useful biomarker for drug-induced cardiotoxicity in rats. J Appl Toxicol. (2015) 35:173–80. doi: 10.1002/jat.3044
185. Ezquer F, Gutierrez J, Ezquer M, Caglevic C, Salgado HC, Calligaris SD. Mesenchymal stem cell therapy for doxorubicin cardiomyopathy: hopes and fears. Stem Cell Res Ther. (2015) 6:116. doi: 10.1186/s13287-015-0109-y
186. Arslan F, Lai RC, Smeets MB, Akeroyd L, Choo A, Aguor EN, et al. Mesenchymal stem cell-derived exosomes increase ATP levels, decrease oxidative stress and activate PI3K/Akt pathway to enhance myocardial viability and prevent adverse remodeling after myocardial ischemia/reperfusion injury. Stem Cell Res. (2013) 10:301–12. doi: 10.1016/j.scr.2013.01.002
Keywords: non-coding RNAs, cancer therapy, cardiotoxicity, biomarkers, therapeutic strategies
Citation: Sun W, Xu J, Wang L, Jiang Y, Cui J, Su X, Yang F, Tian L, Si Z and Xing Y (2022) Non-coding RNAs in cancer therapy-induced cardiotoxicity: Mechanisms, biomarkers, and treatments. Front. Cardiovasc. Med. 9:946137. doi: 10.3389/fcvm.2022.946137
Received: 17 May 2022; Accepted: 28 July 2022;
Published: 23 August 2022.
Edited by:
Emma Louise Robinson, University of Colorado, United StatesReviewed by:
Yang Shao, Fudan University, ChinaJunlian Gu, Shandong University, China
Tarun Pant, Medical College of Wisconsin, United States
Copyright © 2022 Sun, Xu, Wang, Jiang, Cui, Su, Yang, Tian, Si and Xing. This is an open-access article distributed under the terms of the Creative Commons Attribution License (CC BY). The use, distribution or reproduction in other forums is permitted, provided the original author(s) and the copyright owner(s) are credited and that the original publication in this journal is cited, in accordance with accepted academic practice. No use, distribution or reproduction is permitted which does not comply with these terms.
*Correspondence: Yanwei Xing, eGluZ3lhbndlaTEyMzQ1JiN4MDAwNDA7MTYzLmNvbQ==; Zeyu Si, emV5dV9zJiN4MDAwNDA7MTYzLmNvbQ==
†These authors have contributed equally to this work and share first authorship