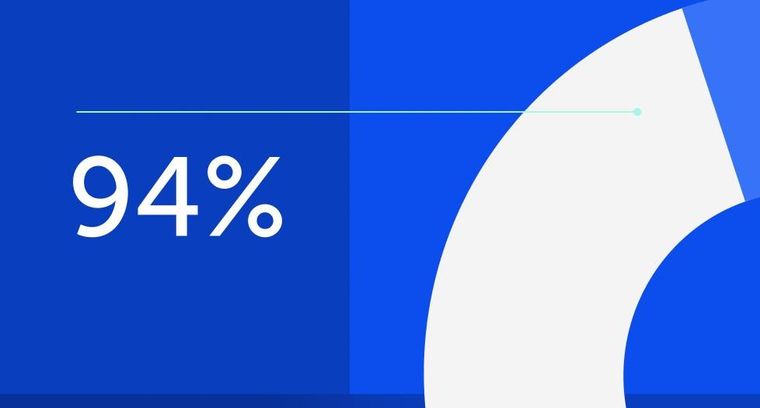
94% of researchers rate our articles as excellent or good
Learn more about the work of our research integrity team to safeguard the quality of each article we publish.
Find out more
REVIEW article
Front. Sustain. Food Syst., 20 September 2018
Sec. Waste Management in Agroecosystems
Volume 2 - 2018 | https://doi.org/10.3389/fsufs.2018.00059
This article is part of the Research TopicNew processes for Nutrient Recovery from WastesView all 14 articles
In this chapter the relevance of microalgae-based processes for the recovery of nutrients contained in wastewaters is reviewed. The fundamentals of the process are discussed from the biological and engineering standpoints and it is shown that on this type of processes the nutrient recovery capacity is mainly a function of solar radiation availability. If adequately designed and operated up to 450 tCO2, 25 tN, and 2.5 tP per hectare and per year can be fixed, producing up to 200 t/year of valuable microalgae biomass. The utilization of microalgae-based processes reduces to half the energy consumption of conventional wastewater treatment and allows recovering up to 90% of the nutrients contained into wastewater. Still the technology available (photobioreactors, harvesting, downstream) must be improved to reduce the land requirement and the hydraulic retention time, but current technology is ready to be demonstrated at large scale, so that the first initial facilities based on this technology have been recently developed. Moreover, this technology must be adapted to the different wastewater types, from sewage to manure. The major advantage of microalgae-based processes is the production of large amounts of valuable biomass, useful for the production of biofuels but much more interesting for animal feeding and agriculture uses, thus enhancing the productivity and sustainability of foods production.
Microalgae have been proposed for a wide range of applications, such as the production of pharmaceuticals and nutraceuticals, the production of biofuels, and depuration processes to carry out the treatment of effluents as flue gases and wastewater (Spolaore et al., 2006; Acién Fernández et al., 2012b). The reasons why microalgae are considered fit for such different applications include (i) its high growth rate, over 1 day−1, (ii) being capable to growth in widely different environments, from warm areas such as the trophic or deserts to cold areas in high mountains and poles, (iii) their valuable biochemical composition, as its biomass is rich in proteins and lipids among others valuable compounds, and (iv) they do not require fertile land or usable water, growing even in wastewaters (Chisti, 2012). When using wastewaters microalgae allows recovering the nutrients contained in those (Olguín, 2012).
Microalgae are photoautotrophic microorganisms, so they use solar energy to reduce inorganic nutrients to organic matter thus producing biomass. Reported biomass productivity of microalgae cultures varies as a function of reactor technology, microalgae strain and environmental conditions, but values ranging from 40 to 150 tn/ha·year (dry matter) have been reported (Benemann, 2003; Chisti, 2012). However, to achieve high productivity values large amounts of nutrients are required. For instance, to produce 100 tn of microalgae biomass up to 200 of CO2, 10 tn of N, and 1 tn of P are consumed. Usually CO2 is supplied as pure food grade CO2, whereas N is supplied as nitrate salts, and P is supplied as phosphate salts. To supply these nutrients chemical fertilizers are usually utilized. Those are based on fossil energy and have a minimum production cost of around 0.5 €/kg. To reduce this production cost the utilization of flue gases and wastewater as nutrients source is mandatory (Acién et al., 2012a). Moreover, the recovery of nutrients from wastewaters produces an economic benefit that make the process really profitable.
Production of microalgae coupled to the treatment of wastewater has been widely reported, including for largely different effluents as sewage, centrate, manure, etc. (Olguín, 2012; Craggs et al., 2013; Hernández et al., 2013). According to the composition of the effluent the overall process must be adequately designed and operated. Producing microalgae on these effluents allows recovering the N and P contained on these effluents as valuable biomass, thus up to 1 kg of dry microalgae biomass can be produced per m3 of sewage, whereas more than 10 kg of dry microalgae biomass can be produced per m3 of manure. That is the reason why microalgae have been also proposed as a nutrient recovery technology. In this case the nutrient recovery capacity is limited by the microalgae biomass production, the higher the biomass productivity the larger the nutrients recovery capacity, with maximum theoretical values of 10 tN/ha·year and 2 tP/ha·year reported as possible (Acién et al., 2016).
In this chapter the capacity of microalgae to contribute to nutrients recovery processes is revised, in addition to the technologies currently used, the application of these processes to different effluents and finally the potential uses of the released biomass. This is a fast growing field on which numerous research groups and companies are now involved, due to the high potential of microalgae based processes and interesting applications of produced biomass. This field is directly related with the bioeconomy sector and the concept of circular economy, because the aim is to recover and recycle nutrients from wastewaters to make human activities more sustainable, especially wastewater treatment and foods production if the produced biomass is used as biofertilizer or for animal feeding.
As microalgae can recover nutrients from wastewaters they have been proposed for wastewater treatment as alternative to conventional technologies such as those based on activated sludge. However, when considering the utilization of microalgae for wastewater treatment it must be bore in mind that what there exists is actually a consortium of microalgae and bacteria (Muñoz et al., 2006). As in these systems no sterile conditions are possible, the consortium that finally prevail in the reactors will be that naturally occurring as a function of the wastewater composition, environmental conditions, reactor design, and operation conditions. Regardless the biological composition of the consortium a general pattern is assumed (Figure 1). Thus, bacteria existing in the culture oxidize the organic matter to inorganic compounds, consuming oxygen in this step, whereas microalgae use the light to uptake the inorganic nutrients that have been released by the bacteria and produce biomass, during this step releasing in turn the oxygen required by the bacteria for the oxidizing step (Muñoz et al., 2006). According to this scheme a “natural” equilibrium between microalgae in bacteria stablishes whatever the conditions on the reactor. However, the composition of the consortium in this equilibrium can differ widely depending on the conditions prevailing in the reactor. Thus the relative composition of the consortium determines the relative relevance of the phenomena (oxygen production, CO2 consumption, nitrogen and phosphorous fixation, etc.), finally occurring in this type of processes (García et al., 2017).
Figure 1. Scheme of the main biological phenomena taking place when using microalgae/bacteria consortia for nutrients recovery from wastewaters.
The performance of microalgae-based wastewater treatment processes is mainly a function of light availability in the reactor, moreover it is mainly a function of solar radiation availability (a function of the location, date, and solar hour) and culture depth. Due to the presence of biomass in the culture the light impinging on the reactor surface is attenuated along the culture depth, the average irradiance at which the cells are exposed the culture thus becomes a function of culture depth (Molina Grima et al., 1996). The relevance of this parameter the performance of microalgae-based wastewater treatment has been discussed at great length. General agreement exists about that fixation of nutrients by microalgae, mainly nitrogen and phosphorous, is a direct function of average irradiance. If this is so, then the shorter the culture depth the higher the average irradiance is and thus the higher the nitrogen and phosphorous fixation rate (Olguín, 2012; Cabanelas et al., 2013a; Posadas et al., 2014; Acién et al., 2016). On the other hand, the shorter the culture depth the smaller the volume of the reactor is and this decreases the amount of wastewater that can be treated per reactor surface unit. Thus, an optimum culture depth that balances both bactors must be defined.
Optimizing the water depth in wastewater treatment processes using microalgae is not easy because it is a function of major objective of the facility, such as to produce high quality biomass or to treat larger volume of wastewater as possible. Thus, to maximize the recovery of nutrients and the production of microalgae biomass the culture depth must be short, <0.2 m, whereas to alternatively to maximize the capacity of wastewater treatment the culture depth must be large, more than 0.3 m. It must be highlighted that in an extreme scenario of large culture depth, the light availability could be enough to produce enough oxygen for the oxidation of the organic matter by the bacteria, but in these conditions it is possible that not enough light is available for the microalgae to take up the released inorganic compounds, then nitrification and denitrification would take place in large extension (Sutherland et al., 2014). Concluding, at these extreme conditions nitrogen and phosphorous would be not recovered but released to the atmosphere (N) or precipitated (P) with the sludge, thus approximating to the phenomena taking place in conventional activated sludge systems (Morales-Amaral et al., 2015a). To maximize the efficient recovery of nutrients it is mandatory to reduce the culture depth below 0.2 m. The sjorter the culture depth the higher the proportion of nutrients finally fixed as valuable microalgae biomass.
The coupling of wastewater treatment and microalgae production was initially proposed as alternative for reducing the microalgae production cost, but today it is considered as an alternative to the utilization of conventional wastewater treatment systems. The main reason for this is that the utilization of microalgae allows recovering nutrients carried by the wastewater, while minimizing the emissions of greenhouse gases and saving energy (Olguín, 2012; Craggs et al., 2013). Conventional technologies require expending large amounts of energy, up to 0.5 kWh/m3, that costs up to 0.2 €/m3 to remove the contaminants from the wastewater and to release clean water. In addition, the treatment cost can be higher if advanced oxidation processes for the removal of nitrogen as Anammox and others are applied, up to 5–8 €/kg (Aqualia, personal communication). Most of the compounds contained into the wastewater are then released to the atmosphere, carbon as CO2, and nitrogen as N2, whereas P is precipitated and usually removed with digested sludge. Thus, in the case of FCC Aqualia, one of the largest wastewater treatment company in Europe, this company annually treat up to 500 Mm3 of wastewater, from this treatment dissipating more than 1,000 kt/year of CO2, 25 kt/year of nitrogen, 5 kt/year of phosphorous. These amounts are largely relevant as shows the fact that they are equivalent to 11 and 26% of the overall nitrogen and phosphorous based fertilizers produced by Fertiberia, the largest fertilizer-producing company in Spain. If the treatment of this large amount of wastewater were done using microalgae it would be possible to produce up to 500 kt/year of microalgae biomass, avoiding the emission of the corresponding amount of CO2 and other greenhouse gases produced in conventional wastewater treatment plants. Moreover, processes based on microalgae requires much less energy, as little as 0.2 kWh/m3, saving more than a half of the energy currently spent in conventional wastewater treatment processes.
The capacity of microalgae to growth and to produce biomass is a function of solar radiation availability and photosynthetic efficiency. Daily solar radiation is mainly a function of location and day of the year, ranging from 150 to 350 W/m2. Regarding the photosynthetic efficiency, it is a function of how close the culture conditions (temperature, pH, etc.) are to the optimum required by the strain used. Photosynthetic efficiency values reported ranged from maximum of 5% achieved in optimized reactors, normally operated in perfect controlled conditions at laboratory or pilot scale, to minimum of 1% achieved in open reactors and large facilities where the control of culture conditions is very difficult (Tredici et al., 1997; Tredici, 2010). On the basis of solar radiation availability and photosynthetic efficiency, and considering the consumption of nutrients by microalgae per unit mass produced, the nutrients recovery capacity by microalgae-based processes can be estimated (Figure 2). Results shows how the CO2 fixation capacity ranges from 40 to 200 t/ha·year in locations with low solar radiation, and from 70 to 450 t/ha·year in tropical areas, when the photosynthetic efficiency modifies from 1 to 5%. Regarding N recovery, it ranges from 2 to 10 t/ha·year in locations with low solar radiation, and from 5 to 25 t/ha·year in tropical areas, when the photosynthetic efficiency modifies from 1 to 5%. On the same way, the P recovery range from 0.2 to 1.1 t/ha·year in locations with low solar radiation, and from 0.5 to 2.5 t/ha·year in tropical areas, when the photosynthetic efficiency modifies from 1 to 5% (Figure 2). These are the limit values of nutrients recovery capacity when using microalgae bases processes, and although this has been reported at laboratory and pilot scale, real facilities must still be developed to demonstrate the feasibility of this technology at commercial scale (Posadas et al., 2013; Ledda et al., 2015; Morales-Amaral et al., 2015b; Sepúlveda et al., 2015).
Figure 2. Variation of CO2, N, and P recovery capacity as a function of solar radiation availability and photosynthetic efficiency achieved in the production system. (A) CO2 fixation capacity, (B) N recovery capacity, (C) P recovery capacity.
Nutrients recovered by microalgae-based processes can partially replace the production of synthetic N and P based fertilizers. Thus, soluble forms of N and P are produced at large scale worldwide because they are the pillars underpinning food production by agriculture. Regarding nitrogen, the actual production systems use atmospheric nitrogen and large amounts of energy (10–15 kWh/kgN) to transform it into ammonia and nitrate by the Haber process, which makes this process a large contributor to the global warming effect. Regarding phosphorous, the reservoirs of this nutrient are limited and some reports have been warning for some time about a crash of actual food production system based on phosphorous before the end of this century (Cordell et al., 2009). The process of transforming P-rich rocks into fertilizers also needs huge amounts of energy (5–10 kWh/kgP). To avoid these problems it is necessary to enforce the recovery of nitrogen and phosphorous from wastes and residual streams, and microalgae can naturally carry out these processes (Craggs et al., 1996). Thus microalgae are capable to completely remove N and P contained in wastewater streams, using only using solar energy in the process and producing valuable biomass at the same time. The development of microalgae-based treatment processes is a key issue in this field (Acién et al., 2016).
In spite of the great advantages of microalgae-based processes for recovering nutrients from wastewater, this technology still has not been extensively used, only a few examples are in operation worldwide. The reason for this is the limited performance of the technology currently available. The microalgae-based wastewater treatment technology still must be improved. The actual technology for microalgae culturing requires long hydraulic residence times, of up to 7–10 days, and extensive surfaces, up to 10 m2 per equivalent person (Matamoros et al., 2015). To make this technology more suitable for wastewater depuration at industrial scale these parameters need to be largely optimized, decreasing the hydraulic retention time to <1 day and the required surface to 1 m2 per equivalent person. Due to the relevance of wastewater treatment field, large companies focus their efforts on this challenge. In this sense, FCC Aqualia recently inaugurated the first demonstration facility of wastewater treatment based on microalgae using optimized technology requiring <2 days of hydraulic retention time and 2 m2 per equivalent person. This facility of 10 ha will be able to process the wastewater from the town of Chiclana in the South of Spain and has been supported by the EU Commission through the ALLGAS project (FCC Aqualia, 2018). Other relevant projects on this field includes SABANA project (www.eu-sabana.eu) focused into the production of biofertilizers and Aquafeed from wastewater using seawater, or AlgaeBioGas project (www.algaebiogas.eu) focused into the treatment of biogas digestate using microalgae. In spite of this successful examples, there is still room for improvement of the technology, and specially it must be demonstrated in different locations and using wastewaters with different compositions. The technology used in this type of processes and the influence of wastewater composition into the performance of the system are analyzed next.
The recovery of nutrients by using microalgae is a process that must be carefully planned and performed. The main steps involved in a microalgae-based process for the recovery of nutrients are: (i) pre-treatment of effluent, (ii) recovery of nutrients and production of biomass in the photobioreactor, (iii) harvesting of biomass, (iv) treatment of used water for recirculation or disposal, and (v) transformation of the biomass into end products. Pre-treatment required is similar to that performed in conventional wastewater treatment plants, so it is necessary to carry out filtration steps in order to minimize the total solids content and maximize the transparency of the wastewater. The photobioreactor and the harvesting are the most relevant steps into the process. Treatment of used water is habitually not necessary if the harvesting step is enough efficient in the removal of solids. Moreover, as microalgae processes remove pathogens and micro-contaminants quite efficiently, no additional UV or ozone treatment are necessary for water disposal or reuse (Matamoros et al., 2015). Finally, the downstream process will be a function of the final uses of the biomass, always related to human uses. The relevance of photobioreactor and harvesting technologies are discussed next.
The core of the process is the photobioreactor in which the microalgae biomass is produced. Many bibliographic references are already available about the design and operation of various photobioreactors but for wastewater treatment and nutrients recovery mainly open raceway reactors are used (Posten, 2009; Lundquist et al., 2010; Craggs et al., 2012).
Open raceway reactors consist of a low depth carrousel in which the water is recirculated mainly using paddlewheels (Figure 3). They are basically large water reservoirs with low depth to facilitate light penetration and thus increase the biomass productivity. Details about the construction of this type of reactors has been extensively published, although it is still a “hot research field” due to the relevance of this technology not only for wastewater treatment, but also for other microalgae-based processes. Thus, raceway reactors are the most extended microalgae production technology with more than 90% of total worldwide microalgae production being done in those systems. The performance of open raceways for the recovery of nutrients from wastewater has been confirmed but it can be further improved. Major efforts on this field are focused on the reduction of energy consumption by optimizing its fluid-dynamics (Sompech et al., 2012; Chiaramonti et al., 2013; Mendoza et al., 2013a), the improvement of mass transfer capacity (Li et al., 2013; Mendoza et al., 2013b; de Godos et al., 2014), and development of overall performance models (Fernández et al., 2016; Huesemann et al., 2016; Solimeno et al., 2017).
Figure 3. Image of raceway reactors operated by FCC AQualia in ALLGAS project for wastewater treatment and nutrients recovery using microalgae. Left, a regular raceway reactor, Right, the LEAR system.
The most relevant facts about these reactors are that the energy consumption must be lower than 10 W/m3, and that the culture depth can be modified from 0.4 to 0.1 m. When using for wastewater treatment the energy consumption must be lower as possible, always lower than 5 W/m3, and the culture depth ranges from 0.2 to 0.4 m. Recently FCC Aqualia patented a new design of LEAR reactor consuming <2 W/m3, which is a really low energy requirement (Figure 3). The main advantages of the raceway reactors are its low cost, below 10 €/m2, and its scale up potential. Currently, single units of up to 5,000 m2 are been used at commercial scale. Another advantage of this technology is its low energy consumption, that makes them particularly suitable for the production of biofuels (Chisti, 2013). The main disadvantages of raceway reactors are related with the poor control of culture conditions and low productivity. The size of a single open raceway reactor is up to 5.000 m2, but large facilities up to 20 ha exist that have been built by installing multiple reactors.
Harvesting of microalgae biomass is a critical step in every microalgae based-process. The main reasons for that are that microalgae cells are small in size (2–20 μm), have a density similar to water and their concentration in the cultures is rather low, ranging from 0.5 to 3.0 g/L. Thus, recovering efficiently and completely the biomass from large culture volumes is a challenge. It has been reported that separation of the biomass from microalgae cultures can sum up to 30% of the total biomass production cost (Grima et al., 2005). When recovering nutrients from wastewaters only low energy-demanding and low-cost technologies can be considered. Fortunately, the wastewater treatment industry has ample experience in the removal of low concentration small solids from large volumes of water and thus the technologies from the wastewater treatment sector are recommendable.
In conventional microalgae-based processes centrifugation is the most extended technology for the recovery of biomass from microalgae cultures. The is technology already available for this application, including some especial equipment being developed from companies as GEA Westfalia. Using conventional self-discharge centrifuges the energy consumption approximates to 1 kWh/m3, whereas using nozzle separators it can be reduced to 0.4 kWh/m3 (GEA communication). For the separation to be energetically positive, the energy consumption of harvesting step must be minimized below 0.1 kWh/m3. Considering a biomass concentration of 1 g/L and energy consumption of harvesting step of 1 kWh/m3, the specific energy consumption is of 3.6 MJ/kg, close to 20% of the total energy content of produced biomass. When using nozzle separator, the specific energy consumption reduces till 7% but still this energy consumption needs to be reduced. The answer to this necessity has been the development of two steps processes. In these, a first step carries out a low cost preconcentration of the biomass in the culture broth while a second step a more complete dewatering is performed to achieve the final biomass concentration targeted, over 100 g/L. Cheap and low energy demanding technologies proposed for microalgae harvesting includes sedimentation and flotation, including the utilization of coagulants/flocculants to modify the physical properties of microalgae biomass (Udom et al., 2013; Gutiérrez et al., 2015). The utilization of dissolved air flotation is one of most extensively recommended, allowing to pre-concentrate the microalgae biomass up to 40 g/L sludge, releasing clean water free of solids then accomplishing regulation (Bare et al., 1975). Using these technologies, the energy consumption can be greatly reduced below 0.1 kWh/m3, so the specific energy consumption of the harvesting step is reduced to <2% of total energy content of the biomass. Regardless of the harvesting technology finally used, it must be efficient enough to bring the final concentration of biomass in the outlet stream up to code with the regulations.
Each effluent is different and its composition and properties can change as a function of time and operational conditions. The mean composition of most relevant effluents is showed in Table 1. It can be seen how large differences exist between the (i) effluents from farms, mainly manure and centrate from anaerobic digestion of manure and other residuals, and (ii) the effluents from sewage, including the raw sewage and centrate produced from anaerobic digestion of activated sludge. The composition of other effluents from agroindustry also detailed on this table are in the middle between these two extreme scenarios. The main characteristics of an effluent that need to be taken into account when considering nutrient recovery from these effluents using microalgae are discussed next.
Table 1. Proximate composition of different effluents that can be processed by microalgae to recover nutrients and to produce biomass.
Light penetration is a critical parameter in any microalgae-based process, as it reduces sharply as the turbidity increases due to the solids content of the effluent. Values over than 3,000 NTU, equivalent to 1,000 mg/L, largely reduce the performance of microalgae cultures (Ledda et al., 2015). To solve this problem, a pre-treatment of the effluent is necessary either by filtration, or alternatively by dilution of the effluent with water but in this last case the total volume of effluent to be processed increases, making this alternative not recommendable. It is important to note that the suspended solids are finally hydrolyzed in the microalgae reactor, but this is a slow process and these solids remain a long time, thus to maximize the capacity of the microalgae process it is recommendable to minimize the presence of suspended solids in the inlet water (Ledda et al., 2016).
Nitrogen is a major nutrient for microalgae production, but it is normally supplied as nitrate in concentration of 50 mgN/L when preparing artificial culture medium. When using effluents nitrogen is present mainly as ammonia, and at concentrations ranging from 65 to over 9,000 mgN/L. It has been widely reported that ammonia is toxic at concentrations higher than 100 mg/L, but some strains are more tolerant than others and this tolerance is also modulated by the culture conditions (Collos and Harrison, 2014; Morales-Amaral et al., 2015a). It is important to notice that because microalgae reactors are operated in continuous mode, the real concentration of ammonium at which the cells are exposed inside the reactor is not the same as the one of the inlet effluent. Thus, the real concentration inside the culture is a function of the ammonium concentration in the inlet, of the imposed dilution rate and of the biomass productivity as this determines the nutrients removal capacity. It has been demonstrated that even with ammonium inlet concentrations over than 600 mg/L the ammonium concentration into the reactor can be lower than 100 mg/L (Morales-Amaral et al., 2015b; Sepúlveda et al., 2015).
Phosphorous is the other major nutrient required for microalgae production. In effluents, phosphorous is normally found as phosphate or in organic compounds, but in the microalgae reactors it is always oxidized to phosphate due to the high oxidative conditions prevailing. There are not reports on phosphorous toxicity for microalgae cultures probably because always low concentrations are always provided. The most relevant problem concerning phosphorous management in microalgae reactors is the precipitation of calcium phosphate that happens in alkaline conditions (Morales-Amaral et al., 2015a). To minimize this problem, it is necessary to limit the presence of calcium in the culture medium and to reduce the pH during the operation of the reactor (Posadas et al., 2015). In any case, the precipitation of phosphorous is not a problem if finally it is harvested with the biomass and adequately used in the final application of the biomass.
The organic matter contained in the effluent is the major contaminant to be removed in whatever wastewater treatment process. Biodegradable compounds are included into the biological oxygen demand (BOD), while chemical oxygen demand (COD) includes the total degradable compounds. Although heterotrophic and even mixotrophic growth of microalgae have been reported, it has been demonstrated only using low molecular weight molecules such as glycerol, glucose, etc., thus it cannot be assumed that microalgae are able to degrade large organic molecules (Cerón García et al., 2000; Ren et al., 2014). This role is performed in microalgae/bacteria consortia by the heterotrophic bacteria, so the higher the concentration of organic matter in the wastewater to be treated the higher the population of bacteria in the final biomass produced will be. It is important to note that bacteria prevailing in microalgae/bacteria consortia are not pathogenic, so the competence reduces the presence of E. coli and Clostridium with respect to that contained into the inlet effluent (Ouali et al., 2015). When using effluents with high concentration of organic matter larger hydraulic retention times must be used to allows the complete degradation of these contaminants. In any case, the rate at which the organic matter is oxidized to inorganic compounds by the heterotrophic bacteria is faster (hours) than the rate it takes to the microalgae to fix the released compounds (days) (Gómez-Serrano et al., 2015).
Sewage is produced by human activities. The total amount of sewage produced is continuously increasing due to the population rise and the improvement of life style. Microalgae can be used for the recovery of nutrients contained in the sewage, but the final process must be properly designed according to the composition of wastewater to be used. Thus, different possible schemes exist. When considering the processes performed in a conventional wastewater treatment plant three main streams are identified: (i) wastewater after primary treatment it containing all the soluble compounds (organic and inorganic), (ii) wastewater after secondary treatment it containing only a minor concentration of inorganic compounds but not organic matter, and (iii) centrate from anaerobic digestion of sludge that contains both organic and inorganic compounds but in reduced state (Figure 4, Table 2).
Figure 4. Scheme of a conventional wastewater treatment process. Composition of wastewater at different points inside the wastewater treatment plant is included in Table 2.
Table 2. Composition of microalgae culture medium and effluents from wastewater treatment plants, nutrient limiting the production of microalgae biomass when using each one, and the maximal achievable biomass concentration according to the concentration of the limiting nutrient.
Comparing the composition of microalgae culture medium with that of different effluents from conventional wastewater treatment plants it is concluded that all of them contain the same components that are also the main elements of the biomass such as carbon, nitrogen and phosphorous (Table 2). However, the total concentration, and in some cases the relative concentration of each one of these compounds is not the same among the different mediums. as it can be seen, when using microalgae to treat wastewater after primary or secondary treatment the nutrient limiting the production of microalgae is N, whereas when centrate from anaerobic digestion of sludge is used the nutrient limiting the production of microalgae is P (Acién et al., 2016). Also the maximal microalgae biomass concentration achievable using the different effluents depends on its total nutrients concentration, resulting that centrate from anaerobic digestion is the richest in nutrients allowing to achieve the highest microalgae biomass concentration, up to 1.2 g/L. The second best is wastewater after primary treatment that allows to achieve up to 0.65 g/L of microalgae biomass, and finally the wastewater after secondary treatment is the poorest effluent only being able to achieve a microalgae biomass concentration of 0.2 g/L.
The recovery of nutrients from wastewater after primary treatment is the most extended scenario. This scenario is equivalent to replace conventional activated sludge systems by microalgae-based technologies, so that the process becomes cheaper and less energy-consuming than conventional systems. In this case up to 1 kg of microalgae biomass can be produced per m3 of processed wastewater. Since the water at the end of the process must accomplish the EU regulation, the nitrogen concentration must be lower than 10 mg/L and P concentration must be below 2 mg/L. The entire process must be designed and operated to accomplish these criteria. Some works demonstrated that this is a highly interesting and feasible strategy (Cabanelas et al., 2013a; Craggs et al., 2014).
The recovery of nutrients from wastewaters after secondary treatment has been proposed as alternative to tertiary treatment, thus allowing to reduce the nitrogen and phosphorus concentration in the effluent from conventional wastewater treatment processes (Cabanelas et al., 2013b). The main challenge in this case is the low concentration of nutrients in treated wastewater that limits the microalgae biomass production capacity. To solve this problem the utilization of membranes to separate the hydraulic and the cellular retention times has been proposed. Using membranes, it is possible to maintain the cellular retention times at the optimum value of 3–4 days, whereas reducing the hydraulic retention time up to 1 day so largely increasing the capacity of wastewater treatment (Marbelia et al., 2014; Gómez-Serrano et al., 2015).
The recovery of nutrients from centrate obtained after anaerobic digestion of activated sludge is a relevant issue. Centrate from anaerobic digestion contains up to 1,000 mgN/L and 30 mgP/L and its removal in wastewater treatment plants entails a high cost and energy consumption. The flow of centrate in wastewater treatment plants is not a negligible issue, as it can be up to 2% of total wastewater flow, which is recirculated to the process increasing the cost and energy consumption of wastewater treatment processes. For a medium size conventional plant processing 50,000 m3/day of wastewater, avoiding the necessity of recirculate and treat this centrate can allow a reduction in the power consumption up to 12,000 kWh/day, with an annual cost reduction of 430 k€/year (FCC Aqualia, personal communication). Microalgae can recover nutrients from this centrate, but it must be added to the photobioreactor carefully to avoid excess of turbidity and ammonium. The dilution of centrate prior to its addition to the photobioreactor is usually done, but to make the process more sustainable the water used for the dilution of the centrate can be recirculated from the effluent (Morales-Amaral et al., 2015a). Due to the high nutrients content of centrate from conventional wastewater treatment plants, it has been proposed also as a suitable single nutrients source to produce marine strains (Ledda et al., 2015; Sepúlveda et al., 2015).
Livestock agriculture in Europe has developed into an efficient industry during the last decades, but its future is tarnished by several environmental problems (e.g., water, air, and soil pollution) that stem from the large quantities of wastes produced within several intensively farming regions. The entire manure production in the EU that potentially is available for manure processing is estimated to 1.4 billion tons. Germany is the first producer with up to 202 million tons, whereas Spain produced 117 million tons, and Italy up to 88 million tons per year. Most of the manure is stored and land spread contributing to contamination of water reservoirs and causing emissions to the atmosphere. Alternatively, manure can be processed to change its physical and/or chemical properties as an objective itself, or in order to recover energy from the livestock manure, make the livestock manure more stable, or remove nutrients from the main stream (Ledda et al., 2016). In this scenario farms are obliged to develop and apply specific solutions adapted to the various types of manure produced and the local situation. Different technologies can be applied to manage manure, but no universal solutions exist. Processed and unprocessed manures are usually land spread, but production of these residues overpasses the capacity of the arable land available. Also, only a small fraction of the nutrients presents in the slurry (particularly N and P) are consumed by plants, causing enormous environmental problems related to leaching, pollution of surface waters, emissions of noxious gases into the atmosphere and surface water eutrophication. Moreover, the Nitrates Directive (1991) establishes action programs to cut down nitrogen pollution and states the limit of 0.170 tonN/ha·year from livestock manure to control pollution, improve water quality, and protect human health.
The application of microalgae-bacteria consortiums allows using solar energy to transform manure nutrients (C, N, P) into valuable biomass that can be used to produce commodities as feed and biofertilizers. The feasibility of this process has been demonstrated at small scale it being reclaimed as a suitable solution for mild climates, allowing to valorize the manure as raw material for the production of valuable compounds instead of as a residual (Godos et al., 2009; Min et al., 2014). The main disadvantage of manure for microalgae production is the excess of ammonium contained on this effluent, that makes necessary to supply this effluent carefully as nutrients source to microalgae cultures (Liao et al., 1995; Min et al., 2014). Recently a demonstration project performed in Spain titled “PURALGA” has demonstrated the feasibility of microalgae based processes for the production of biofertilizers from raw manure. Techno economic analysis of the process show that using microalgae it is possible to treat the manure at cost below 2 €/m3, but even more interesting is that the incomes from biofertilizers produced are much higher than the operation cost of the treatment plant, thus achieving a profitable process instead a of costly process (unpublished data).
When coupling the production of microalgae with the recovery of nutrients form wastewater using the microalgae biomass for human related applications it is not allowed. However, it could be used for energy, animal or agriculture uses. The major factor limiting today the widespread utilization of microalgae biomass on these markets is the enormous amounts of biomass required for these markers, overpassing by several order of magnitude the actual microalgae production capacity of 30 kt/year worldwide (Benemann, 2013). Coupling the recovery of nutrients from wastewaters with the production of microalgae will allow to greatly increase the availability of microalgae biomass for these non-human related applications.
Energy demand is continuously increasing and the utilization of renewable energy is being enforced by most of the countries in worldwide. Among different alternatives, microalgae have been proposed as highly interesting for the production of biofuels, mainly biodiesel (Chisti, 2007). The reasons for that include the relatively high lipid content of microalgae biomass in addition to its high production capacity per land surface. However, if using clean water and fertilizers the microalgae production cost is too high to enter the energy market. Only coupling the production of microalgae with nutrients recovery from wastewater the microalgae biomass production cost can be reduced below 1 €/kg, which is the limit for suitable processes (Acién et al., 2017). Moreover, although microalgae can be used to produce biodiesel from the lipid fraction, it sums up no more than 30% of the total biomass which makes necessary to develop biorefinery schemes that can process and use the remaining 70% of the biomass.
Microalgae-based biorefineries have been studied for a long time. Numerous references have been published in this field (Vanthoor-Koopmans et al., 2013; Trivedi et al., 2015; Moreno-Garcia et al., 2017). As a general conclusion it can be summarized that to produce biofuels the best option is to produce biogas. The production of biogas from microalgae biomass allows transforming up to 65% of the total biomass into final product biogas, whereas for bioethanol only a maximum of 40% of the total biomass can be transformed, and focusing on biodiesel the limit decreases to a maximum of 30% of the biomass that can be transformed (Vanthoor-Koopmans et al., 2013). Other biofuels or intermediates as bio-oil among others, give similar results and imply more complex processes. The development of biorefineries involving different steps as oil extraction to produce biodiesel, carbohydrates extraction to produce bioethanol, and finally the waste biomass being used to produce biogas has been also proposed but the complexity of the process is excessive and the losses of raw materials in each step reduce the overall performance of the global process (Ortiz Montoya et al., 2013). Production of biogas from anaerobic digestion is a well-stablished technology, including the possibility of upgrading the biogas to biomethane for automotive uses (Figure 5). From microalgae biomass more than 200 L of biomethane can be produced per kg of microalgae biomass. Bearing this is mind, only FCC Aqualia wastewater treatment plants would be able to produce up to 100 Mm3 of biomethane if actual conventional wastewater treatment were replaced by microalgae wastewater treatment schemes, this would be enough to fuel 65,000 cars each year on a fully sustainable mode (FCC AQUALIA, personal communication).
Figure 5. Scheme of a conventional process for the production of biogas from microalgae biomass produced by recovering nutrients from wastewater.
Regarding animal uses, the valuable composition of microalgae biomass is highly attractive for this application (Yaakob et al., 2014). The most traditional use of microalgae for feeding is related with aquaculture. On this field, the microalgae are used for feeding the first stage of larvae and juvenile, then it is replaced by fish-oil and fishmeal because of its lower cost. In the final stages even fish oil and fish meal are replaced by cereals and other raw materials to keep the price of the feed as low as possible (Shah et al., 2018). If microalgae were available at low price and in enough amounts to be competitive in this market it would be widely used because of its superior nutritional valuable and acceptance by the animals. The strains used for aquaculture include marine strains such as Nannochloropsis, but also freshwater strains such as Chlorella, Spirulina, or Scenedesmus among others (Muller-Feuga, 2000). Using only microalgae for feeding in aquaculture is not possible due to the enormous size of this market compared to the current microalgae production capacity, but it has been widely reported that the inclusion of 1–10% of microalgae biomass in the Aquafeed greatly improves the quality of feed and the final health of animals produced (de Cruz et al., 2018). For this market the value of microalgae biomass can be higher than 1 €/kg, which means that since up to 1 kg of microalgae biomass can be produced per m3 of wastewater, a revenue of 1 €can be potentially obtained per m3 of wastewater. Still these figures must be demonstrated in real scale processes.
Concerning animal uses, an equivalent scenario has been proposed. In addition to proteins, carbohydrates and lipids, microalgae contain vitamins, minerals, antioxidants, and other valuable components that can be included in animal diets (Brune et al., 2009). The benefits of incorporating microalgae in animal feeding has been widely reported to be related with the improvement of the health of the animals and the improvement of color and other properties of the end products obtained from these animals (meat, eggs, etc.) (Becker, 2007; Yaakob et al., 2014). Just as in the case of aquaculture, the major limitation to the incorporation of microalgae in animal feeding is the current high price of this raw material and its low availability. If the price were reduced and the availability increased it could be incorporated initially in the feed of young animals and later in the feed for adults. A way to reduce the cost of microalgae biomass for animal feeding is to combine the extraction of lipids for biodiesel with the utilization of protein-rich remaining biomass for animal feeding, thus combining to applications requiring large amounts of biomass (Gatrell et al., 2014).
It is important to mention that for this application it the use of the microalgae biomass is allowed even if produced from wastewaters, as long as it is demonstrated that the biomass is safe, free of pathogens, etc. Moreover, the utilization of microalgae biomass on these markets is the most efficient strategy in terms of sustainability and nutrients recovery, because the nutrients recovered from the wastewater are directly used as feed for animals, avoiding the need to produce plants or vegetables in an intermediate step. For these reasons the development of this scheme is likely to become a reality in the next future.
Regarding the products to be obtained for agricultural uses, the current increasing world food demand and the contamination effect brought about by the increased use of chemical fertilizers to enhance crop yields highlight the importance of products such as biostimulants, biopesticides, and biofertilizers as interesting candidates in sustainable agriculture. These bioproducts are fully biodegradable and non-toxic both to plants and their consumers. As a result, there are no toxicity problems or ecotoxicity or harmful residues. Additionally, these formulations are safe from an operational point of view (Bhardwaj et al., 2014). Microalgae have been reported to be a source of valuable compounds for agriculture uses. Marine algae have been shown to have bactericidal, antifungal, and insecticidal activities (Goud et al., 2007; Hernández-Carlos and Gamboa-Angulo, 2011; Najdenski et al., 2013). Green microalgae genera like Nannochloropsis, Chlorella, and Scenedesmus can produce phytohormones including indol acetic acid (IAA), cytokinins, gibberellins, absicisic acid (ABA), and/or jasmonic acid as well as other substances with biostimulant activity (Lu and Xu, 2015). On the other hand, some cyanobacteria like Arthrospira (Spirulina) are able to produce IAA, cytokinins and/or jasmonic acid, and including are very effective “biopesticide” agents (Tarakhovskaya et al., 2007).
Controlling the microalgae growing in wastewater treatment processes is not easy, but by the modification of the culture conditions such as pH, nutrients supply, and other key parameters it is possible to favor a given type of microalgae and cyanobacteria strain. Regardless of the photosynthetic microorganism produced, it would be rich in proteins in any case and thus rich in valuable amino acids in addition to carbohydrates and lipids. These compounds confer interesting properties to microalgae-based products for agricultural uses. The processing of microalgae biomass to obtain the final product is a key step. Enzymatic hydrolysis under mild conditions is highly recommendable although other alternatives such as chemical hydrolysis or other downstream processing strategies have been proposed (Romero García et al., 2012). The quality of microalgae-based biofertilizers is largely a function of the downstream processing as well as of the quality of the microalgae biomass used. In this process it is not necessary to use dry biomass, but a cell disruption step is mandatory and the dosage the optimum type of enzymes needs to be chosen carefully (Figure 6). The final separation of solids and stabilization of the product are also key challenges that are kept secret by the companies. This type of microalgae-based products is more frequent in agriculture by the day and will continue to grow due to its demonstrated positive effects in the enhancement of growth and production of plants.
Figure 6. Scheme of the enzymatic hydrolysis process for the production of amino acids concentrates from microalgae biomass produced by recovering nutrients from wastewater.
The recovery of nutrients from wastewaters using microalgae is a reliable process, which can be applied to different wastewater types from sewage to centrate and manure. If adequately designed and operated up to 450 tCO2, 25 tN, and 2.5 tP per hectare and per year can be fixed, producing up to 200 t/year of valuable microalgae biomass. To achieve these values, the technology actually used must be improved, taking into account the existence of microalgae/bacteria consortia and their different requirements. To maximize the light utilization is a major challenge on these systems. Coupling microalgae production with wastewater treatment has a large impact in the reduction of treatment cost and the increase of sustainability of wastewater treatment processes, treatment cost reducing to half and sustainability increasing by reduction of energy requirement and greenhouse gases emissions. The major challenge today is to demonstrate this type of processes at large scale, at different conditions and using different wastewater types, different industrial scale projects being in progress. The produced biomass is a valuable resource for different applications. Although the production of biofuels is always attractive, microalgae are much more interesting for chemicals, animal feeding, and agricultural uses. Thus, biodiesel and bioethanol can be produced from microalgae biomass in addition to biogas/biomethane, this last being the most recommendable. Additionally, microalgae biomass can be used for the production of intermediates for chemical synthesis. However, the recommended use of microalgae biomass is for animal feeding and agriculture. Microalgae contains valuable compounds (proteins, fatty acids, biostimulants, etc.) that allows the enhancement of yield, in addition to improvement of sustainability and economic balance, of foods production processes. There are no doubts that in the next years more microalgae-based processes will be installed coupling the recovery of nutrients with biomass production, thus expanding the relevance of microalgae biotechnology.
FA is the leader of the research group performing this research in the last years. CG-S is the researcher in charge of most of the experimental work performed. JF-S is co-leader of the group and responsible of modeling and data analysis.
The authors declare that the research was conducted in the absence of any commercial or financial relationships that could be construed as a potential conflict of interest.
This work was supported by the European Union's Horizon 2020 Research and Innovation program under the Grant Agreement No. 727874 SABANA, and ERANET LAC GREENBIOREFINERY ELAC 2014/BEE-0357, in addition to Ministerio de Ciencia, Innovacion y Universidades – Gobierno de España, project GREENFARM, grant number CTQ2017-84006-C3-3-R.
Acién Fernández, F. G., González-López, C. V., Fernández-Sevilla, J. M., and Molina-Grima, E. (2012b). Conversion of CO2 into biomass by microalgae: how realistic a contribution may it be to significant CO2 removal? Appl. Microbiol. Biotechnol. 96, 577–586. doi: 10.1007/s00253-012-4362-z
Acién, F. G., Fernández, J. M., Magán, J. J., and Molina, E. (2012a). Production cost of a real microalgae production plant and strategies to reduce it. Biotechnol. Adv. 30, 1344–1353. doi: 10.1016/j.biotechadv.2012.02.005
Acién, F. G., Gómez-Serrano, C., Morales-Amaral, M. M., Fernández-Sevilla, J. M., and Molina-Grima, E. (2016). Wastewater treatment using microalgae: how realistic a contribution might it be to significant urban wastewater treatment? Appl. Microbiol. Biotechnol. 100, 9013–9022. doi: 10.1007/s00253-016-7835-7
Acién, F. G., Molina, E., Fernández-Sevilla, J. M., Barbosa, M., Gouveia, L., Sepúlveda, C., et al. (2017). “Economics of microalgae production,” in Microalgae-Based Biofuels Bioproduction From Feedstock Cultivation to End-Products, eds R. Muñoz and C. Gonzalez-Fernandez (Duxford: Woodhead Publishing).
Bare, W. F. R., Jones, N. B., and Middlebrooks, E. J. (1975). Algae removal using dissolved air flotation. J. Water Pollut. Control Fed. 47, 153–169. Available online at: http://www.jstor.org/stable/25038604
Becker, E. W. (2007). Micro algae as a source of protein. Biotechnol. Adv. 25, 207–210. doi: 10.1016/j.biotechadv.2006.11.002
Benemann, J. (2013). Microalgae for biofuels and animal feeds. Energies 6, 5869–5886. doi: 10.3390/en6115869
Benemann, J. R. (2003). “Biofixation of CO2 and greenhouse gas abatement with microalgae,” in 6th Asia-Pacific Conference on Algal Biotechnology, (Laguna) 1–29.
Bhardwaj, D., Ansari, M. W., Sahoo, R. K., and Tuteja, N. (2014). Biofertilizers function as key player in sustainable agriculture by improving soil fertility, plant tolerance and crop productivity. Microb. Cell Fact. 13:66. doi: 10.1186/1475-2859-13-66
Brune, D. E., Lundquist, T. J., and Benemann, J. R. (2009). Microalgal biomass for greenhouse gas reductions: potential for replacement of fossil fuels and animal feeds. J. Environ. Eng. 135, 1136–1144. doi: 10.1061/(ASCE)EE.1943-7870.0000100
Cabanelas, I. T., Ruiz, J., Arbib, Z., Chinalia, F. A., Garrido-Pérez, C., Rogalla, F., et al. (2013b). Comparing the use of different domestic wastewaters for coupling microalgal production and nutrient removal. Bioresour. Technol. 131, 429–436. doi: 10.1016/j.biortech.2012.12.152
Cabanelas, I. T. D., Arbib, Z., Chinalia, F. A., Souza, C. O., Perales, J. A., Almeida, P. F., et al. (2013a). From waste to energy: microalgae production in wastewater and glycerol. Appl. Energy 109, 283–290. doi: 10.1016/j.apenergy.2013.04.023
Cerón García, M. C., Fernández Sevilla, J. M., Acién Fernández, F. G., Molina Grima, E., and García Camacho, F. (2000). Mixotrophic growth of Phaeodactylum tricornutum on glycerol: growth rate and fatty acid profile. J. Appl. Phycol. 12, 239–248. doi: 10.1023/A:1008123000002
Chiaramonti, D., Prussi, M., Casini, D., Tredici, M. R., Rodolfi, L., Bassi, N., et al. (2013). Review of energy balance in raceway ponds for microalgae cultivation: re-thinking a traditional system is possible. Appl. Energy 102, 101–111. doi: 10.1016/j.apenergy.2012.07.040
Chisti, Y. (2007). Biodiesel from microalgae. Biotechnol. Adv. 25, 294–306. doi: 10.1016/j.biotechadv.2007.02.001
Chisti, Y. (2012). “Raceways-based production of algal crude oils,” in Microalgal Biotechnology Potential Production, eds C. Posten and C. Walter (Berlin: DeGruyter), 113–146.
Chisti, Y. (2013). Raceways-based production of algal crude oil. Green 3, 195–216. doi: 10.1515/green-2013-0018
Collos, Y., and Harrison, P. J. (2014). Acclimation and toxicity of high ammonium concentrations to unicellular algae. Mar. Pollut. Bull. 80, 8–23. doi: 10.1016/j.marpolbul.2014.01.006
Cordell, D., Drangert, J. O., and White, S. (2009). The story of phosphorus: global food security and food for thought. Glob. Environ. Chang. 19, 292–305. doi: 10.1016/j.gloenvcha.2008.10.009
Craggs, R., Park, J., Heubeck, S., and Sutherland, D. (2014). High rate algal pond systems for low-energy wastewater treatment, nutrient recovery and energy production. N. Z. J. Bot. 52, 60–73. doi: 10.1080/0028825X.2013.861855
Craggs, R., Sutherland, D., and Campbell, H. (2012). Hectare-scale demonstration of high rate algal ponds for enhanced wastewater treatment and biofuel production. J. Appl. Phycol. 24, 329–337. doi: 10.1007/s10811-012-9810-8
Craggs, R. J., Adey, W. H., Jenson, K. R., St. John, M. S., Green, F. B., and Oswald, W. J. (1996). Phosphorus removal from wastewater using an algal turf scrubber. Water Sci. Technol. 33, 191–198. doi: 10.2166/wst.1996.0138
Craggs, R. J., Lundquist, T. J., and Benemann, J. R. (2013). “Wastewater treatment and algal biofuel production,” in Algae for Biofuels and Energy, eds M. A. Borowitzka and N. R. Moheimani (Dordrecht: Spinger), 153–163. doi: 10.1007/978-94-007-5479-9_9
de Cruz, C. R., Lubrano, A., and Gatlin, D. M. (2018). Evaluation of microalgae concentrates as partial fishmeal replacements for hybrid striped bass Morone sp. Aquaculture 493, 130–136. doi: 10.1016/j.aquaculture.2018.04.060
de Godos, I., Mendoza, J. L., Acién, F. G., Molina, E., Banks, C. J., Heaven, S., et al. (2014). Evaluation of carbon dioxide mass transfer in raceway reactors for microalgae culture using flue gases. Bioresour. Technol. 153, 307–314. doi: 10.1016/j.biortech.2013.11.087
FCC Aqualia (2018). Home - All-Gas. Available online at: http://www.all-gas.eu/en/
Fernández, I., Acién, F. G., Guzmán, J. L., Berenguel, M., and Mendoza, J. L. (2016). Dynamic model of an industrial raceway reactor for microalgae production. Algal Res. 17, 67–78. doi: 10.1016/j.algal.2016.04.021
García, D., Posadas, E., Blanco, S., Acién, G., García-Encina, P., Bolado, S., et al. (2017). Evaluation of the dynamics of microalgae population structure and process performance during piggery wastewater treatment in algal-bacterial photobioreactors. Bioresour. Technol. 248, 120–126. doi: 10.1016/j.biortech.2017.06.079
Gatrell, S., Lum, K., Kim, J., and Lei, X. G. (2014). Nonruminant nutrition symposium: potential of defatted microalgae from the biofuel industry as an ingredient to replace corn and soybean meal in swine and poultry diets. J. Anim. Sci. 92, 1306–1314. doi: 10.2527/jas.2013-7250
Godos, I., de Blanco, S., García-Encina, P. A., Becares, E., and Muñoz, R. (2009). Long-term operation of high rate algal ponds for the bioremediation of piggery wastewaters at high loading rates. Bioresour. Technol. 100, 4332–4339. doi: 10.1016/j.biortech.2009.04.016
Gómez-Serrano, C., Morales-Amaral, M. M., Acién, F. G., Escudero, R., Fernández-Sevilla, J. M., and Molina-Grima, E. (2015). Utilization of secondary-treated wastewater for the production of freshwater microalgae. Appl. Microbiol. Biotechnol. 99, 6931–6944. doi: 10.1007/s00253-015-6694-y
Goud, M. J. P., Seshikala, D., and Charya, M. A. S. (2007). Anitbacterial activity and biomolecular composition of certain fresh water micro-algae collected from River Godavari (India). Sci. World J. 9, 350–358. doi: 10.1615/InterJAlgae.v9.i4.40
Grima, E. M., Fernández, F. G. A., and Medina, A. R. (2005). “Downstream processing of cell-mass and products,” in Handbook of Microalgal Culture: Biotechnology and Applied Phycology, ed A. Richmond (Oxford, UK: Blackwell Science Ltd.), 215–252.
Gutiérrez, R., Passos, F., Ferrer, I., Uggetti, E., and García, J. (2015). Harvesting microalgae from wastewater treatment systems with natural flocculants: effect on biomass settling and biogas production. Algal Res. 9, 204–211. doi: 10.1016/j.algal.2015.03.010
Hernández, D., Riaño, B., Coca, M., and García-González, M. C. (2013). Treatment of agro-industrial wastewater using microalgae-bacteria consortium combined with anaerobic digestion of the produced biomass. Bioresour. Technol. 135, 598–603. doi: 10.1016/j.biortech.2012.09.029
Hernández-Carlos, B., and Gamboa-Angulo, M. M. (2011). Metabolites from freshwater aquatic microalgae and fungi as potential natural pesticides. Phytochem. Rev. 10, 261–286. doi: 10.1007/s11101-010-9192-y
Huesemann, M., Crowe, B., Waller, P., Chavis, A., Hobbs, S., Edmundson, S., et al. (2016). A validated model to predict microalgae growth in outdoor pond cultures subjected to fluctuating light intensities and water temperatures. Algal Res. 13, 195–206. doi: 10.1016/j.algal.2015.11.008
Ledda, C., Romero Villegas, G. I., Adani, F., Acién Fernández, F. G., and Molina Grima, E. (2015). Utilization of centrate from wastewater treatment for the outdoor production of Nannochloropsis gaditana biomass at pilot-scale. Algal Res. 12, 17–25. doi: 10.1016/j.algal.2015.08.002
Ledda, C., Schievano, A., Scaglia, B., Rossoni, M., Acién Fernández, F. G., and Adani, F. (2016). Integration of microalgae production with anaerobic digestion of dairy cattle manure: an overall mass and energy balance of the process. J. Clean. Prod. 112, 103–112. doi: 10.1016/j.jclepro.2015.07.151
Li, S., Luo, S., and Guo, R. (2013). Efficiency of CO2 fixation by microalgae in a closed raceway pond. Bioresour. Technol. 136, 267–272. doi: 10.1016/j.biortech.2013.03.025
Liao, P. H., Chen, A., and Lo K, V. (1995). Removal of nitrogen from swine manure wastewaters by ammonia stripping. Bioresour. Technol. 54, 17–20. doi: 10.1016/0960-8524(95)00105-0
Lu, Y., and Xu, J. (2015). Phytohormones in microalgae: a new opportunity for microalgal biotechnology? Trends Plant Sci. 20, 273–282. doi: 10.1016/j.tplants.2015.01.006
Lundquist, T. J., Woertz, I., and Benemann, J. R. (2010). “Microalgae for wastewater treatment and biofuels production,” in ACS National Meeting Abstracts (Boston, MA).
Marbelia, L., Bilad, M. R., Passaris, I., Discart, V., Vandamme, D., Beuckels, A., et al. (2014). Membrane photobioreactors for integrated microalgae cultivation and nutrient remediation of membrane bioreactors effluent. Bioresour. Technol. 163, 228–235. doi: 10.1016/j.biortech.2014.04.012
Matamoros, V., Gutiérrez, R., Ferrer, I., García, J., and Bayona, J. M. (2015). Capability of microalgae-based wastewater treatment systems to remove emerging organic contaminants: a pilot-scale study. J. Hazard. Mater. 288, 34–42. doi: 10.1016/j.jhazmat.2015.02.002
Mendoza, J. L., Granados, M. R., de Godos, I., Acién, F. G., Molina, E., Banks, C., et al. (2013a). Fluid-dynamic characterization of real-scale raceway reactors for microalgae production. Biomass Bioenergy 54, 267–275. doi: 10.1016/j.biombioe.2013.03.017
Mendoza, J. L., Granados, M. R., de Godos, I., Acién, F. G., Molina, E., Heaven, S., et al. (2013b). Oxygen transfer and evolution in microalgal culture in open raceways. Bioresour. Technol. 137, 188–195. doi: 10.1016/j.biortech.2013.03.127
Min, M., Hu, B., Mohr, M. J., Shi, A., Ding, J., Sun, Y., et al. (2014). Swine manure-based pilot-scale algal biomass production system for fuel production and wastewater treatment–a case study. Appl. Biochem. Biotechnol. 172, 1390–1406. doi: 10.1007/s12010-013-0603-6
Molina Grima, E., Fernández Sevilla, J. M., Sánchez Pérez, J. A., García Camacho, F., Molina-Grima, E., Sevilla, J. M. F., et al. (1996). A study on simultaneous photolimitation and photoinhibition in dense microalgal cultures taking into account incident and averaged irradiances. J. Biotechnol. 45, 59–69. doi: 10.1016/0168-1656(95)00144-1
Morales-Amaral, M. M., Gómez-Serrano, C., Acién, F. G., Fernández-Sevilla, J. M., and Molina-Grima, E. (2015a). Outdoor production of Scenedesmus sp. in thin-layer and raceway reactors using centrate from anaerobic digestion as the sole nutrient source. Algal Res. 12, 99–108. doi: 10.1016/j.algal.2015.08.020
Morales-Amaral, M. M., Gómez-Serrano, C., Acién, F. G., Fernández-Sevilla, J. M., and Molina-Grima, E. (2015b). Production of microalgae using centrate from anaerobic digestion as the nutrient source. Algal Res. 9, 297–305. doi: 10.1016/j.algal.2015.03.018
Moreno-Garcia, L., Adjallé, K., Barnabé, S., and Raghavan, G. S. V. (2017). Microalgae biomass production for a biorefinery system: recent advances and the way towards sustainability. Renew. Sustain. Energy Rev. 76, 493–506. doi: 10.1016/j.rser.2017.03.024
Muller-Feuga, A. (2000). The role of microalgae in aquaculture: situation and trends. J. Appl. Phycol. 12, 527–534. doi: 10.1023/A:1008106304417
Muñoz, R., Guieysse, B., Muñoz, R., and Guieysse, B. (2006). Algal-bacterial processes for the treatment of hazardous contaminants: a review. Water Res. 40, 2799–2815. doi: 10.1016/j.watres.2006.06.011
Najdenski, H. M., Gigova, L. G., Iliev, I. I., Pilarski, P. S., Lukavský, J., Tsvetkova, I. V., et al. (2013). Antibacterial and antifungal activities of selected microalgae and cyanobacteria. Int. J. Food Sci. Technol. 48, 1533–1540. doi: 10.1111/ijfs.12122
Olguín, E. J. (2012). Dual purpose microalgae-bacteria-based systems that treat wastewater and produce biodiesel and chemical products within a Biorefinery. Biotechnol. Adv. 30, 1031–1046. doi: 10.1016/j.biotechadv.2012.05.001
Ortiz Montoya, E. Y., Llamas Moya, B., Molina Grima, E., García Cuadra, F., Fernández Sevilla, J. M., and Acien Fernandez, F. G. (2013). Method for the Valorisation of Photosynthetic Microorganisms for Integral Use of Biomass. Patent WO 2014/122331 Al.
Ouali, A., Jupsin, H., Vasel, J. L., and Ghrabi, A. (2015). Removal of E. coli and enterococci in maturation pond and kinetic modelling under sunlight conditions. Desalin. Water Treat. 53, 1068–1074. doi: 10.1080/19443994.2013.856350
Posadas, E., Bochon, S., Coca, M., García-González, M. C., García-Encina, P. A., and Muñoz, R. (2014). Microalgae-based agro-industrial wastewater treatment: a preliminary screening of biodegradability. J. Appl. Phycol. 26, 2335–2345. doi: 10.1007/s10811-014-0263-0
Posadas, E., García-Encina, P. A., Soltau, A., Domínguez, A., Díaz, I., and Muñoz, R. (2013). Carbon and nutrient removal from centrates and domestic wastewater using algal-bacterial biofilm bioreactors. Bioresour. Technol. 139, 50–58. doi: 10.1016/j.biortech.2013.04.008
Posadas, E., Morales, M. M., Gomez, C., Acién, F. G., and Muñoz, R. (2015). Influence of pH and CO2 source on the performance of microalgae-based secondary domestic wastewater treatment in outdoors pilot raceways. Chem. Eng. J. 265, 239–248. doi: 10.1016/j.cej.2014.12.059
Posten, C. (2009). Design principles of photo-bioreactors for cultivation of microalgae. Eng. Life Sci. 9, 165–177. doi: 10.1002/elsc.200900003
Ren, H. Y., Liu, B. F., Kong, F., Zhao, L., Xie, G. J., and Ren, N. Q. (2014). Energy conversion analysis of microalgal lipid production under different culture modes. Bioresour. Technol. 166, 625–629. doi: 10.1016/j.biortech.2014.05.106
Romero García, J. M., Acién Fernández, F. G., and Fernández Sevilla, J. M. (2012). Development of a process for the production of l-amino-acids concentrates from microalgae by enzymatic hydrolysis. Bioresour. Technol. 112, 164–170. doi: 10.1016/j.biortech.2012.02.094
Sepúlveda, C., Acién, F. G., Gómez, C., Jiménez-Ruíz, N., Riquelme, C., and Molina-Grima, E. (2015). Utilization of centrate for the production of the marine microalgae Nannochloropsis gaditana. Algal Res. 9, 107–116. doi: 10.1016/j.algal.2015.03.004
Shah, M. R., Lutzu, G. A., Alam, A., Sarker, P., Kabir Chowdhury, M. A., Parsaeimehr, A., et al. (2018). Microalgae in aquafeeds for a sustainable aquaculture industry. J. Appl. Phycol. 30, 197–213. doi: 10.1007/s10811-017-1234-z
Solimeno, A., Gabriel, F., and García, J. (2017). Mechanistic model for design, analysis, operation and control of microalgae cultures: calibration and application to tubular photobioreactors. Algal Res. 21, 236–246. doi: 10.1016/j.algal.2016.11.023
Sompech, K., Chisti, Y., and Srinophakun, T. (2012). Design of raceway ponds for producing microalgae. Biofuels 3, 387–397. doi: 10.4155/bfs.12.39
Spolaore, P., Joannis-Cassan, C., Duran, E., and Isambert, A. (2006). Commercial applications of microalgae. J. Biosci. Bioeng. 101, 87–96. doi: 10.1263/jbb.101.87
Sutherland, D. L., Turnbull, M. H., and Craggs, R. J. (2014). Increased pond depth improves algal productivity and nutrient removal in wastewater treatment high rate algal ponds. Water Res. 53, 271–281. doi: 10.1016/j.watres.2014.01.025
Tarakhovskaya, E. R., Maslov, Y. I., and Shishova, M. F. (2007). Phytohormones in algae. Russ. J. Plant Physiol. 54, 163–170. doi: 10.1134/S1021443707020021
Tredici, M. R. (2010). Photobiology of microalgae mass cultures: understanding the tools for the next green revolution. Biofuels 1, 143–162. doi: 10.4155/bfs.09.10
Tredici, M. R., Zittelli, G. C., and Autotrofi, M. (1997). Efficiency of sunlight utilization: tubular versus flat photobioreactors. Biotech. Bioeng. 57, 187–197. doi: 10.1002/(SICI)1097-0290(19980120)57:2<187::AID-BIT7>3.0.CO;2-J
Trivedi, J., Aila, M., Bangwal, D. P., Kaul, S., and Garg, M. O. (2015). Algae based biorefinery - How to make sense? Renew. Sustain. Energy Rev. 47, 295–307. doi: 10.1016/j.rser.2015.03.052
Udom, I., Zaribaf, B. H., Halfhide, T., Gillie, B., Dalrymple, O., Zhang, Q., et al. (2013). Harvesting microalgae grown on wastewater. Bioresour. Technol. 139, 101–106. doi: 10.1016/j.biortech.2013.04.002
Vanthoor-Koopmans, M., Wijffels, R. H., Barbosa, M. J., and Eppink, M. H. (2013). Biorefinery of microalgae for food and fuel. Bioresour. Technol. 135, 142–149. doi: 10.1016/j.biortech.2012.10.135
Keywords: microalgae, nutrients recovery, wastewater treatment, biomass, bioenergy, biofertilizers
Citation: Acién Fernández FG, Gómez-Serrano C and Fernández-Sevilla JM (2018) Recovery of Nutrients From Wastewaters Using Microalgae. Front. Sustain. Food Syst. 2:59. doi: 10.3389/fsufs.2018.00059
Received: 14 May 2018; Accepted: 24 August 2018;
Published: 20 September 2018.
Edited by:
Maria Cruz Garcia Gonzalez, Instituto Tecnológico Agrario de Castilla y León, SpainReviewed by:
Valeria Reginatto, Universidade de São Paulo, BrazilCopyright © 2018 Acién Fernández, Gómez-Serrano and Fernández-Sevilla. This is an open-access article distributed under the terms of the Creative Commons Attribution License (CC BY). The use, distribution or reproduction in other forums is permitted, provided the original author(s) and the copyright owner(s) are credited and that the original publication in this journal is cited, in accordance with accepted academic practice. No use, distribution or reproduction is permitted which does not comply with these terms.
*Correspondence: Francisco Gabriel Acién Fernández, ZmFjaWVuQHVhbC5lcw==
Disclaimer: All claims expressed in this article are solely those of the authors and do not necessarily represent those of their affiliated organizations, or those of the publisher, the editors and the reviewers. Any product that may be evaluated in this article or claim that may be made by its manufacturer is not guaranteed or endorsed by the publisher.
Research integrity at Frontiers
Learn more about the work of our research integrity team to safeguard the quality of each article we publish.