- 1Department of Agronomy, Indian Agricultural Research Institute, New Delhi, India
- 2ICAR-Research Complex for North Eastern Hill Region, Meghalaya, India
- 3Department of Agronomy, Rajiv Gandhi University, Arunachal Pradesh, India
- 4Department of Microbiology, Indian Agricultural Research Institute, New Delhi, India
- 5ICAR-Indian Institute of Rapeseed-Mustard Research, Rajasthan, India
- 6Chaudhary Charan Singh Haryana Agricultural University, Hisar, Haryana, India
- 7Crop Production, Indian Grassland and Fodder Research Institute, Jhansi, Uttar Pradesh, India
- 8Indian Institute of Maize Research, Ludhiana, Punjab, India
- 9Borlaug Institute for South Asia, Samastipur, Bihar, India
In South Asian regions, the traditional maize–mustard rotation (MMR) has become less profitable and unsustainable due to inappropriate fertilization practices and the degradation of soil properties. Therefore, climate-smart and sustainable farm practices are necessary to mitigate production risks and improve soil properties. This study evaluated the long-term impacts of conservation tillage and nutrient management on equivalent yields, soil microbial properties, and water-energy savings. A long-term field experiment was initiated 9 years ago, using the split-plot design to evaluate the three conservation tillage (CA)-based crop establishment practices, i.e., zero tillage (ZT) and conventional tillage (CT), permanent beds (PNB). Each practice was accompanied by the recommended dose of fertilizer (RDF), improved RDF (RDFI), and nutrient expert-guided (NEI) fertilization. CA-based tillage (ZT or PNB) resulted in 24.4–25.2% greater maize grain equivalent yields (EY) compared to the CT, while the NEI and RDFI produced statistically (p = 0.05) identical EY, being 26.6–30.3% greater than the RDF. These practices substantially reduced the water footprints, besides 11.9–12.9% and 23.4–26.6% (9-yrs average) greater water productivity compared to CT and RDF, respectively. In fact, at 0–45 cm soil depth, residues retained ZT or PNB had 31.9–42.2%, 56.5–67.2%, and 16.5–18.3% more bacterial (107), fungi (104), and actinomycetes (104) populations, respectively. Across soil depths, ZT or PNB recorded 7.65–11% and 23.2–31.9% greater soil microbial biomass-C and -P, respectively. Compared to CT-based practices, these practices also improved soil mineralizable N (NO3− N/NH4+ N). The conventionally tilled plots consumed greater direct and indirect non-renewable energy than the CA-based residue-retaining practices. By virtue of residue retention, the PNB and ZT had ~108% greater energy input (EI) than the CT, whereas it was vice versa in terms of the energy output (EO). The NEI registered a 7.6–28.7% higher EO than the RDFI and RDF. These long-term field studies demonstrated that adopting CA-based ZT, or PNB, in combination with precise nutrient management would enhance equivalent yields and soil microbial dynamics, besides improving water-energy footprints in maize–mustard growing ecologies.
1 Introduction
Conservation agriculture and precise nutrient management practices offer several benefits, such as reducing soil erosion, enhancing soil fertility, and boosting crop yields (Brouder and Gomez-Macpherson, 2014; Stevenson et al., 2014). Maize (Zea mays L.), also known as corn or the queen of cereals, is a staple food crop used for human consumption, animal feed, and industrial purposes (Shiferaw et al., 2011). It is a rich source of carbohydrates, dietary fiber, vitamins, and minerals and is a key component of traditional diets in the United States, Mexico, and parts of the African continent (Adamtey et al., 2016). In India, the area under maize cultivation is ~9.9 million ha, with annual production of ~31.5 million tons (GoI, 2020–2021), and Indian states like Andhra Pradesh, Karnataka, Rajasthan, Maharashtra, Bihar, Uttar Pradesh, Madhya Pradesh, and Himachal Pradesh are the dominant maize-growing states that contribute ~80% of the total maize production in the country (Biswakarma et al., 2020). In contrast, mustard (Brassica juncea L.) is an amphidiploid species (AABB; 2n = 36), which is grown either under irrigated or rainfed conditions that require the average external inputs and contributes ~25% to the Indian oilseed supply (Limbalkar et al., 2021). In the food industry, it is widely used for its flavor and nutritional properties because it is an excellent source of essential fatty acids, proteins, vitamins, and minerals (Jat et al., 2011).
The maize–mustard rotation (MMR) is a productive and highly resilient system under variable climatic aberrations. Many smallholders in South Asia rely on MMR for food security and regular income. Several issues have arisen due to the continuous adoption of this rotation following traditional practices and inefficient management (Pradhan et al., 2018). The main drawbacks are lower yield and resource use efficiency. Farmers often mismanage crop residues in conventional MMR because maize residues, though more palatable than rice residues, are still burned or are not returned back to the soil. However, mustard residues, which are unpalatable to the livestock, are often used as fuel at home (Jat et al., 2017; Pooniya et al., 2021). Thus, on-farm and efficient residue management holds the key to increasing soil organic matter (SOM) and nitrogen (N), especially in regions with low fertility soils (Biswakarma et al., 2021, 2023).
In maize-based rotations, the integration of conservation agriculture and precise nutrient management practices has been promising and may result in improved productivity, profitability, and resource use efficiency. The adoption of CA practices, such as no-till and permanent beds, enhances soil properties and reduces environmental impacts (Pradhan et al., 2018). With long-term residue retention, CA practices, like ZT or PNB, can lead to a positive soil carbon (C) and nitrogen (N) budget and enhance soil biological and physical properties (Jantalia et al., 2007; Melero et al., 2011; Pooniya et al., 2022).
Soil microorganisms play vital roles in agroecosystems and cope with biotic and abiotic stresses (Janvier et al., 2007). Moreover, microbial communities can rapidly adjust their biomass and composition in response to environmental changes (Schloter et al., 2003). Thus, analyzing microbial characteristics is a useful approach for evaluating the effects of agricultural management practices (Gil-Sotres et al., 2005). Microbial biomass carbon (C) and phosphorus (P), which reflect soil capacity to retain and recycle nutrients and organic matter, are essential indicators of soil health. Rhizosphere fungi are essential to soil–plant interactions, and inappropriate fertilization can reduce bacterial diversity (Zhou et al., 2015). The management of soil fertility, residue, and tillage has an impact on both the edaphic and biological aspects of soil, as well as the composition and activities of microbial communities (Degrune et al., 2016). Temperature, soil moisture, nutrients, organic matter, and soil texture are other factors affecting soil microbial populations (Brockett et al., 2012; Leff et al., 2015). In addition to the soil’s physical and chemical properties, the soil microbiome is an important indicator of soil quality (Schloter et al., 2018).
The Nutrient Expert (NE) tool was developed by the International Plant Nutrition Institute (IPNI) and the International Maize and Wheat Improvement Centre (CIMMYT). The system is designed to help users make nutrient-related decisions by using a computer-based decision-support system and is based on the principles of location-specific nutrient applications (Pampolino et al., 2012; Gaire et al., 2016). In the NE, the determination of nutrient requirements relies on the assessment of internal nutrient efficiency, which is estimated using the QUEFTS model, Quantitative Evaluation of the Fertility of Tropical Soils (Satyanarayana et al., 2013; Majumdar et al., 2013). It takes account of targeted agronomic efficiency and yield responses, as well as the supply of nutrients from indigenous sources, to maintain yields and promote the restoration of soil fertility (Sapkota et al., 2014; Pooniya et al., 2015). The All-India Coordinated Research Projects (AICRP) and IPNI conducted multi-location field trials (n = 104), and the use of NEI-based fertilization has been shown to have the potential to increase crop yields, optimize nutrient utilization, and minimize fertilizer application (Satyanarayana et al., 2013). In addition, being very exhaustive, the MMR may lead to widespread nutrient deficiencies in soils and crops because this rotation removes a large amount of nutrients (Jat et al., 2013). This is where the combined application of CA and efficient nutrient management practices should be explored for their usability. The present study was conducted for 9 consecutive years in the IGP region to answer the following research questions: (i) How do long-term CA practices affect soil microbial dynamics? (ii) What is the impact of NE-guided nutrient management on equivalent yields and water-energy footprints? (iii) How do CA and precise nutrient management affect soil fertility? The findings of this study will provide valuable insights into the benefits of long-term CA and NE-guided nutrient management practices in the MMR and can help farmers, policymakers, and other stakeholders make informed decisions about sustainable agriculture practices.
2 Materials and methods
2.1 Experimental details and weather
The long-term field investigation on conservation agriculture (CA)-based maize–mustard rotation (MMR) was initiated in the rainy season of 2013 at the ICAR-Indian Agricultural Research Institute, which is situated at 28°38′ N; 77°11′ E, New Delhi, India. The experimental location has a sub-tropical, semi-arid environment, distinguished by its scorching summers and chilly winters. The average annual precipitation fluctuates between 697 and 1,541 mm, of which ~80% occurs between July and September. The minimum and maximum temperatures and relative humidity ranges between 5°C–26°C, 20°C-40°C, and 65–83%, respectively (Supplementary Table 1). Maize was grown from July to October, and mustard is grown from October to April every year. According to pre-experiment chemical analysis of the upper (0.00–0.15 m) soil depth, the soil was alluvium-derived sandy loam (Typic Haplustepts) with a pH of 7.3, Walkley-Black C (oxidizable SOC) 0.40%; KMnO4 oxidizable N 159.9 kg ha−1 Subbiah and Asija (1956); 0.5 M NaHCO3 extractable P 15.6 kg ha−1 Olsen et al. (1954a,b), and 1 N NH4OAc extractable K 161.3 kg ha−1 Hanway and Heidel (1952).
2.2 Treatment description and experimental design
The combinations of different tillage and nutrient management practices were assessed for the 9 years (2013–21) continuously in a fixed plot employing the split-plot design and replicated thrice. Each experimental unit was 20.0 m × 8.5 m in size, resulting in a 170 m2 area. Tillage practices (03), i.e., double zero-tilled flatbed (ZTFB), permanent beds (PNB), and conventional tillage (CT), were allocated in the main plots, and nutrient management practices (03), i.e., (i) farmers’ fertilizer practice followed by, the recommended fertilization (RDF): FFP (7y) fb RDF (2y)—(⅓ N basal + surface banding of ⅓ N at knee-high stage and ⅓ N at tasseling); (ii) RDF followed by RDF improved: RDF (7y) fb RDFI (2y)—[⅓ N basal + ⅓ N sub-surface banding (SSB) at knee-high and ⅓ N surface banding at tasseling]; (iii) nutrient expert-guided (NE) fertilization followed by NE improved: NE (7y) fb NEI (2y)—(⅓ N basal + ⅓ N SSB at knee-high and ⅓ N surface banding at tasseling) were allocated in the sub-plots (Table 1). After harvesting of the crops in each season, all the aboveground residues were removed from the conventional plots, whereas, in the ZT and PNB plots, maize stubbles and mustard stalks (~2.5 Mg ha−1 on a dry weight basis, each crop) were recycled continuously for the 9 years (Figures 1a, 1b; Supplementary Figure 1); the excess stover/stalks were utilized for animal feeding and household purposes.
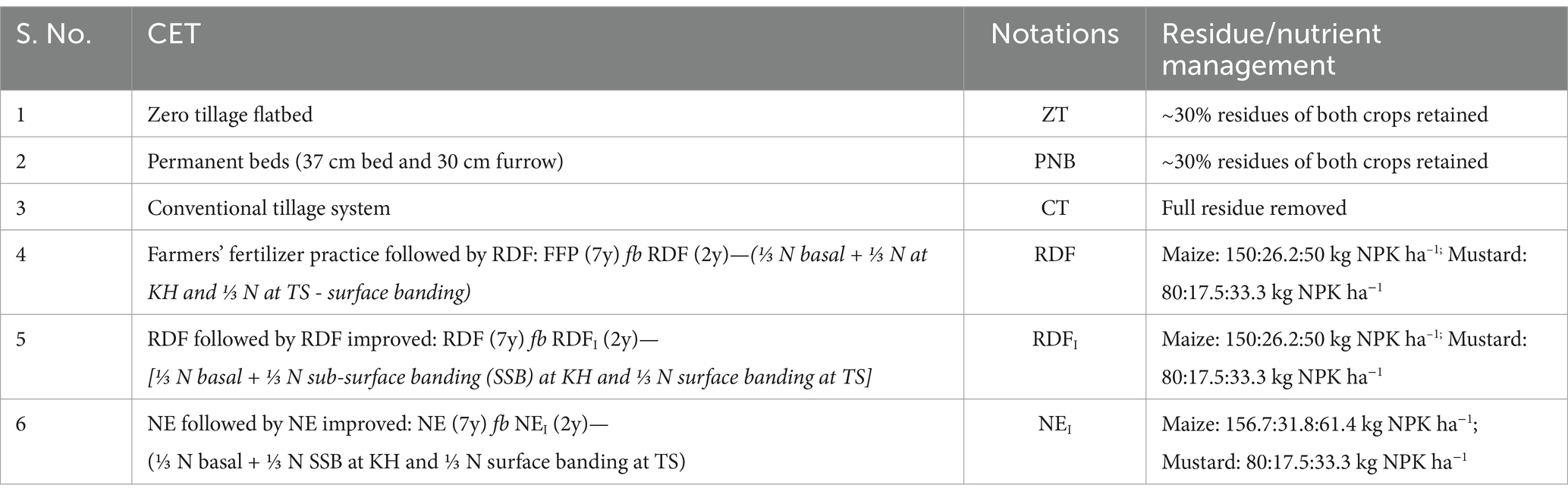
Table 1. Description of tillage, crop establishment (CET), and nutrient management practices adopted for 9 years in a maize–mustard rotation (MMR).
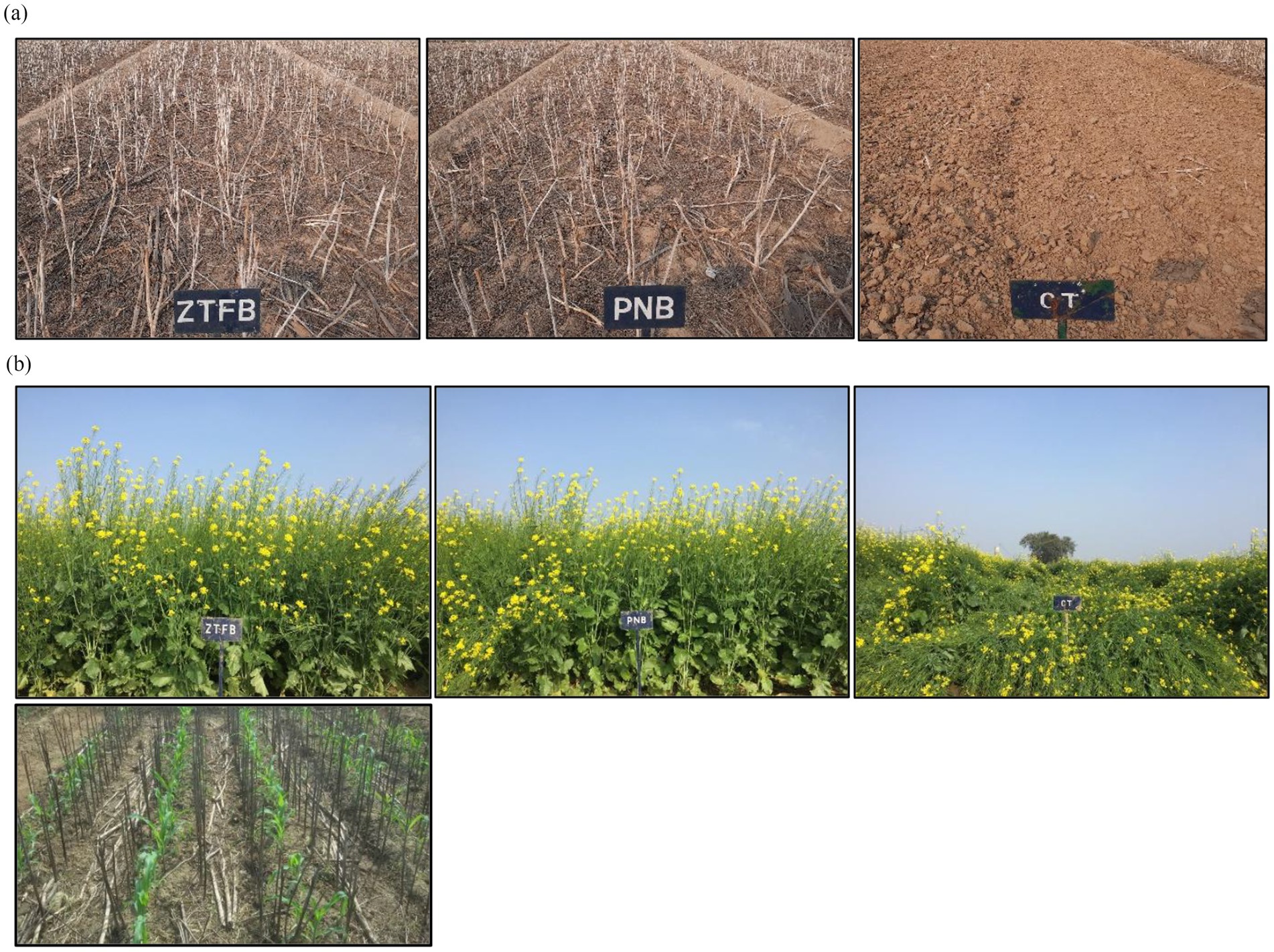
Figure 1. (a) Nine years old residue retained ZT flatbed (left side), PNB (middle) and conventionally tilled (right side) plots under maize-mustard rotation. Pictures were clicked after the harvest of the 9th winter season mustard crop. (b) Flowered mustard under CA and nutrient management-based 9-years old experiment; ZT mustard (top left); PNB (top middle); lodged down CT grown mustard (top right); PNB planted maize with mustard residues (bottom left side).
2.3 Cultural practices and agronomic management
In the CT plots, the experimental field was deep-ploughed to 0.3 m using a disc plough and then pulverized twice with a harrow, followed by leveling using a laser-equipped land leveler for the final seedbed preparation. Similarly, in the PNB (0.30 m furrow and 0.37 m bed width), reshaping and sowing were performed simultaneously using a raised bed planter, followed by packing using a disc coulter. In contrast, in the ZTFB, the crop seeds were drilled using a drill equipped with inverted T-type furrow openers (Supplementary Figure 2). During each season, the high-quality maize genotype ‘HQPM 1’ was sown in the 1st fortnight of July at a spacing of 0.67 m × 0.20 m using 20 kg seed ha−1, but later this genotype was replaced by the recent ‘PMH-1’ and ‘PJHM-1’ genotypes during the years 2017 and 2021, respectively. In contrast, after harvesting maize in early October, the popular mustard genotype ‘Pusa Vijay’ (4 kg seed ha−1) was sown at the second fortnight of October every year. A description of the fertilizer management protocols or practices used in each treatment is described in Table 1. At sowing, the maize crop received ⅓ of nitrogen (N), total phosphorus (P), and potassium (K) as basal in the form of urea CO (NH₂)2, di-ammonium phosphate ((NH4)2HPO4), and potassium chloride (KCL), respectively. The remaining N was applied as per treatment through subsurface banding at knee-high (KH) and tasseling (TS). However, in mustard, half N was applied at the seeding, and the half was top-dressed at the first watering. Since a nutrient expert (NE) for the mustard is not available, the fertilizers were given as per the RDF in NEI-based units. In conservation tillage (ZT or PNB), glyphosate (1 kg a.i. ha−1) was sprayed 1 week before seeding, followed by the application of atrazine/pendimethalin (as pre-emergence, 1–3 days after sowing). In contrast, in the CT plots, weeds were controlled by two- to three-hand weeding. Irrigation water was applied considering the quantity of precipitation and duration of the dry spell during the cropping cycles. Furthermore, the total quantity of water (precipitation + irrigation) applied in maize–mustard rotation was 1,561, 1,167, 1,032, 1,483, 1,045, 1,042, 1,081, 1,117, and 1758 mm in the respective years during the experimentation. In general, mustard is irrigated two to three times per season, whereas maize is irrigated four to five times per season. Need-based plant protection measures were employed to control insect pests and diseases.
2.4 Yield measurements
The samples for yield assessment were taken from a central net plot (9 × 8 m = 72 m2) leaving border rows from each side of the experimental plot. During every October and April, the maize and mustard crops were harvested, leaving ~0.20 m and ~ 0.45 m stubbles from the ground level, respectively. After harvesting, the produce was sun-dried, threshed, and cleaned, and the moisture content was adjusted to ~15% for maize and ~ 12% for mustard to report the grain yield (Ghosh et al., 2022). Furthermore, the stover/stalk yields were measured by deducting the grain weight from the respective total biomass yield (Mg ha−1). Additionally, for a detailed comprehension of yield efficiency on a cropping system level, individual crop yields were converted to system productivity in terms of maize grain equivalents (EY) at the market price scale using the formula described by Biswakarma et al. (2021).
Wherein, EY = Grain equivalents of maize (Mg ha−1), Ymaize = Grain yield of maize (Mg ha−1), Ymustard = Seed yield of mustard (Mg ha−1), Pmaize = Maize grain price (US$ Mg−1), and Pmustard = Mustard grain price (US$ Mg−1).
2.5 Soil N, nitrate-N (NO3−-N), and ammonium-N (NH4+-N)
The Kjeldahl method (DISTYL EMS, KEL PLUS) using alkaline permanganate was used to determine soil nitrogen (Subbiah and Asija, 1956). Nitrate-N (NO3−-N) and ammonium-N (NH4+-N) were quantified via UV spectrophotometry (U128, Hitachi, Fukuoka, Japan). Briefly, 10 g of soil was combined with 100 mL of 2 M KCl and shaken on a mechanical shaker for 1 h at 200 r/m and 25°C, and then the supernatant was filtered. The NO3− -N and NH4+-N contents were determined using a suitable volume of aliquot of the above extract following the procedure described by Jackson (1958) and Schuffelen et al. (1961), respectively.
2.6 Soil microbial properties
A serial dilution method and standard plate count method were used to count the different groups of microbes. A nutrient agar medium was used for total bacterial counts (TBCs), potato dextrose agar medium for total fungal counts (TFCs), and actinomycete isolation agar medium (HiMedia, India) for actinomycetes. A fumigation extraction method was used to measure soil microbial biomass-C (MBC) (Vance et al., 1987). Pre-weighed soil samples from various soil depths were fumigated for 24 h with ethanol-free chloroform. The non-fumigated set was also maintained separately. Furthermore, a solution of 0.5 M K2SO4 (soil to extractant ratio of 1: 4) was added and subjected to reciprocal shaking for a duration of 30 min and subsequently filtered using a filter paper, i.e., Whatman No. 42. The soil OC content of the filtrate was determined using the dichromate digestion method followed by back titration with a (p = 0.05) N solution of ferrous ammonium sulfate. The MBC was then determined using the provided equation:
Where, EC = (Corg in fumigated soil–Corg in non-fumigated soil), and expressed in μg C g−1 soil.
Soil microbial biomass-P (MBP) was measured using the procedure (Brookes et al., 1982). The fumigation extraction process was performed as in MBC. Each soil sample was duplicated; one was fumigated, and the other was not. Olsen’s method (Olsen et al., 1954a,b) was then used to estimate soil-available phosphorus from both fumigated and non-fumigated samples. The soil MBP was determined by subtracting the phosphorous values of the fumigated and non-fumigated samples.
2.7 Water footprints
The water velocity was monitored using a water meter placed in the main channel. In general, the maize and mustard crops received 3–5 and 2–3 irrigations, respectively, based on the critical growth stages and occurrence of the rainfall events during the cropping cycle. The amount of total water and depth was computed by equations as suggested by Jat et al. (2009).
Where, F is the flow rate (m3 s−1), t is time (s) taken in each irrigation in each plot, and A is plot area (m2).
Additionally, the data pertaining to the amount of rainfall received during the growing season was obtained from the neighboring agro-meteorological station (Division of Agricultural Physics, IARI, New Delhi). The effective rainfall was calculated using the established methodology outlined by Mohammad et al. (2018). The cumulative irrigation input for each experimental unit was determined by adding the amount of irrigation water applied and the effective rainfall. The water productivity, expressed in kg grains ha−1 mm−1 of water, was calculated using the method outlined by Bhushan et al. (2007).
Furthermore, the system’s water productivity was determined by adding the water productivity of the maize and mustard crops. The Parihar et al. (2022) formula was used to calculate the water footprint (WF).
2.8 Energy calculations
Energy is the key to optimizing the crop productivity and farm income. In crop production, energy input includes both direct (i.e., labor, fuel, and electricity) and indirect (i.e., seed, fertilizers, and chemicals) sources. This was later categorized into the renewable and non-renewable energy sources for further computation and interpretation. The energy balance of the maize–mustard rotation was determined using the standard conversion equivalent given by Saad et al. (2016) and the energy output by multiplying the corresponding energy coefficients with the system yield (Jat et al., 2020). To evaluate the energy efficiency of individual management practices, various energy indices were calculated using the (Equations 1–10) (Ghosh et al., 2022).
2.9 Statistical analysis
An analysis of variance was used to determine treatment effects (Gomez and Gomez, 1984). A post hoc test of the difference of means was performed using Tukey’s honestly significant difference test (p = 0.05) using SAS 9.3 (SAS Institute, Cary, NC). A pooled analysis was performed because the coefficients of variance of the main and interaction effects varied significantly between years, with no significant year and treatment interactions.
3 Results
3.1 Description of weather parameters
The years 2021–22 had the most precipitation, 1,608 mm from July to April, followed by 1,368 mm during 2013–14 and 1,230 mm during 2016–17, whereas only 600–996 mm was recorded during the other years. The western disturbances in northwest India caused low rainfall during the winter. During the November–April, 2019–20 and 2014–15 had the highest rainfall, which was 306 mm and 315 mm, respectively, and the 2 years with the least rain, 2015–16 and 2017–18, had 22 and 39 mm, respectively. Most of the rainfall during the study period was received during the monsoon period from July to September (Supplementary Table 1).
3.2 Nine-year individual crop yield trends
As the experiment progressed, the grain yield under PNB and ZT conditions was found to be statistically (p = 0.05) identical. Across years, maize grain yield showed an increase of 4–29% and 3.9–28.7% under the PNB and ZT, respectively, compared to the CT (Supplementary Figure 3a). However, RDFI and NEI recorded 20.9–48.6% and 10.3–50.5% higher grain yields than the RDF, respectively (Supplementary Figure 3b). The NEI consistently produced the highest stover yield and was similar to the RDFI for five of the 9 years studied (Supplementary Figures 3c,d). ZT and PNB had 12% greater pooled yields than the CT (Figure 2a), but the NEI and RDFI stover yields increased by 32 and 26%, respectively, over the RDF (Figure 2b).
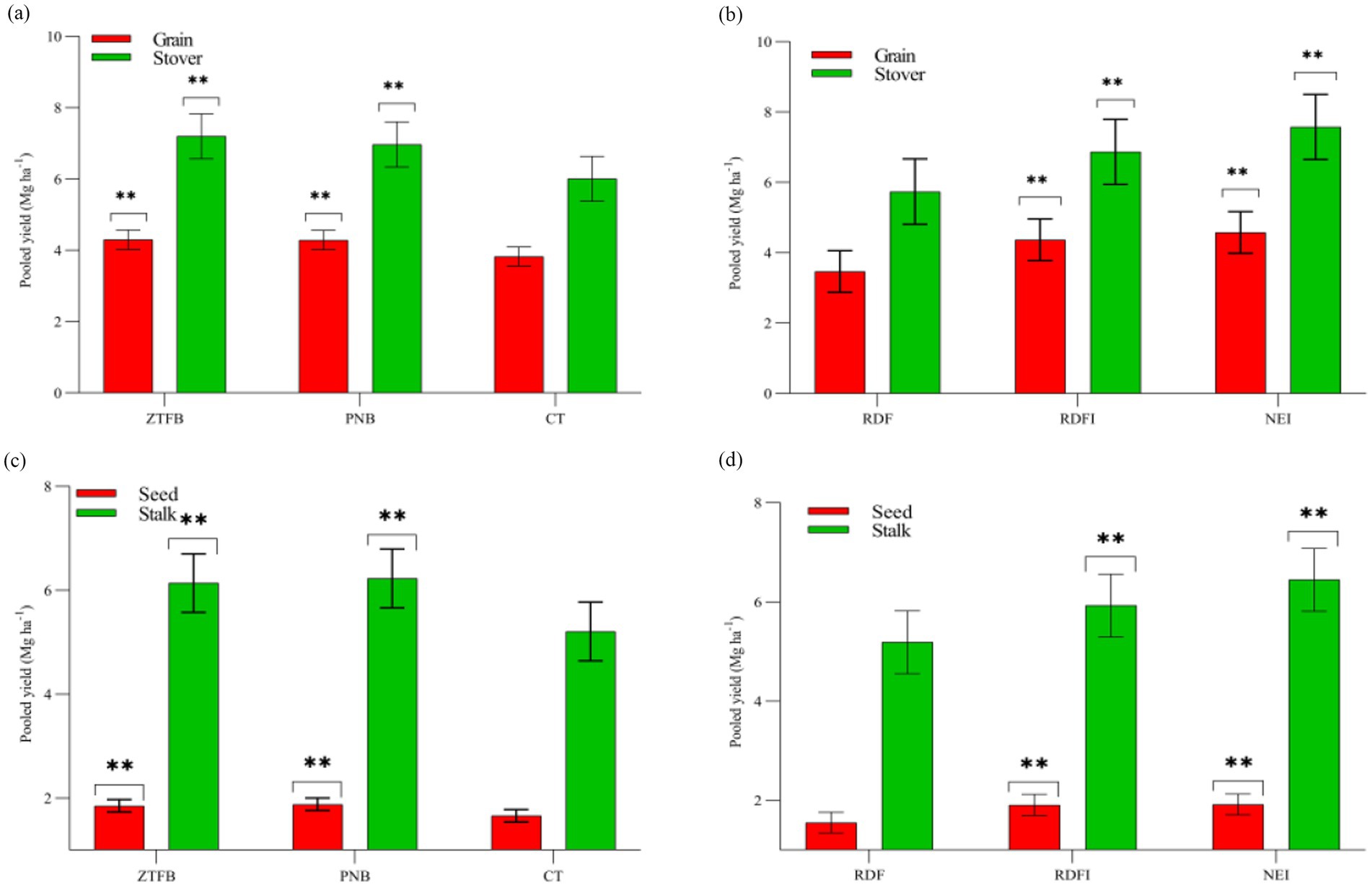
Figure 2. Nine-years pooled grain/stover (a,b) and seed/stalk yields (c,d) (Mg ha−1) of maize and mustard crops under different tillage and nutrient management practices. The asterisk (**) indicates LSD at p0.05.
During the 2021–22 season, the PNB yielded 4.2 and 21.3% higher mustard yields than the ZT and the CT, respectively (Supplementary Figure 4a). On average, the mustard yield was 29.8 and 27.3% higher under the NEI and the RDFI, respectively, than under the RDF (Supplementary Figure 4b). The stalk yield was higher under the ZT and the PNB conditions than under the CT. The NEI also produced a significantly higher stalk yield than the RDFI and the RDF (Supplementary Figures 4c,d). Furthermore, the pooled mustard grain and stalk yields were 13.3 and 19.6% higher, respectively, under the PNB over the CT (Figure 2c). The NEI produced 23.9 and 24.3% higher grain and stalk yields, respectively, over the RDF (Figure 2d).
3.3 Nine-year equivalent yield trends
Long-term tillage significantly influenced the system yields, measured in respect of the maize grain equivalents (EY), in 5 out of the 9 study years, wherein ZT and the PNB improved the EY by 7–20% over the CT (Figure 3a). Regarding the nutrient management practices, the EY was the same between the NEI and the RDFI, but being 26.6–30.3% higher than the RDF (Figure 3b). A significant interaction between tillage practices and nutrient management practices was observed in the EY. The highest EY was recorded with the PNB-NEI during 2013–14, 2018–19, and 2020–21; CT-NEI during 2014–15; ZT-NEI during 2015–16, 2017–18, and 2019–20; ZT-RDFI during 2016–17; and PNB-RDFI during 2021–22 (Table 2).
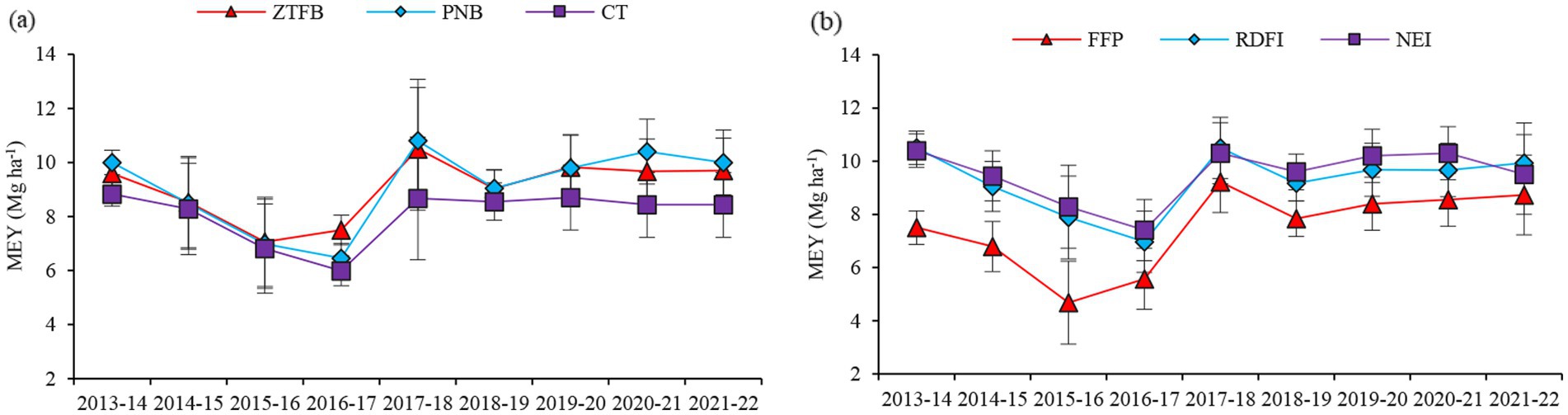
Figure 3. Nine-years trend in the maize equivalent yields under different tillage (a) and nutrient management (b) practices in maize–mustard rotation. The vertical bars indicate LSD at p0.05.
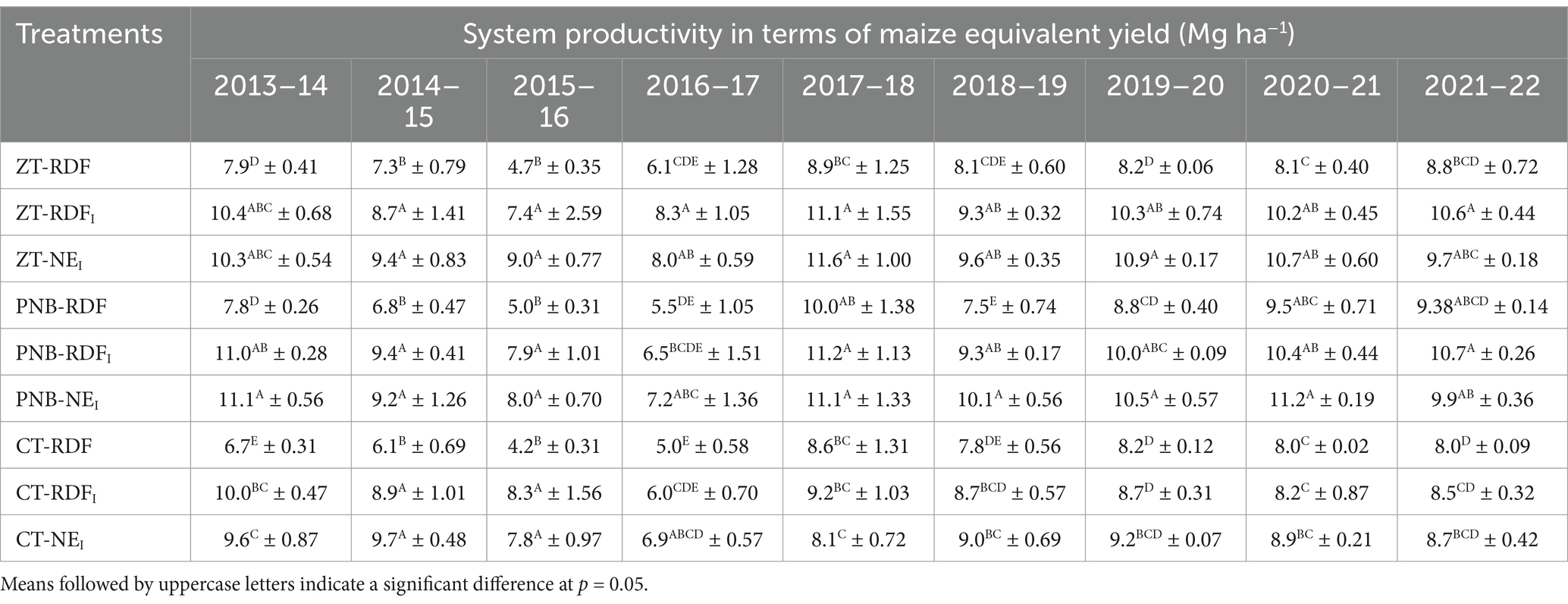
Table 2. Interaction effects of different tillage and nutrient management practices on maize grain equivalents (±S.E.).
3.4 Water footprints
The effects of tillage practices on water footprints were significant (p = 0.05), with the lowest water footprint recorded under the PNB (139 liter kg−1 ha−1) closely fb ZT (140.5) liter kg−1 ha−1 and the highest under the CT (156.7) liter kg−1 ha−1 (Supplementary Figure 5). The NEI (132.4 liter kg−1 ha−1) and RDFI (135.7 liter kg−1 ha−1) resulted in significantly lower water footprints than the RDF (168.1 liter kg−1 ha−1). On average, the system water productivity was the highest under PNB and fb ZT, which were 12.9 and 11.9% higher than the CT, respectively. The NEI and the RDFI-based nutrient management practices improved the system water productivity by 26.6 and 23.4% over the RDF, respectively. There was a significant interaction between tillage and nutrient management practices in terms of water productivity, which followed the same trend as the EY (Table 3).
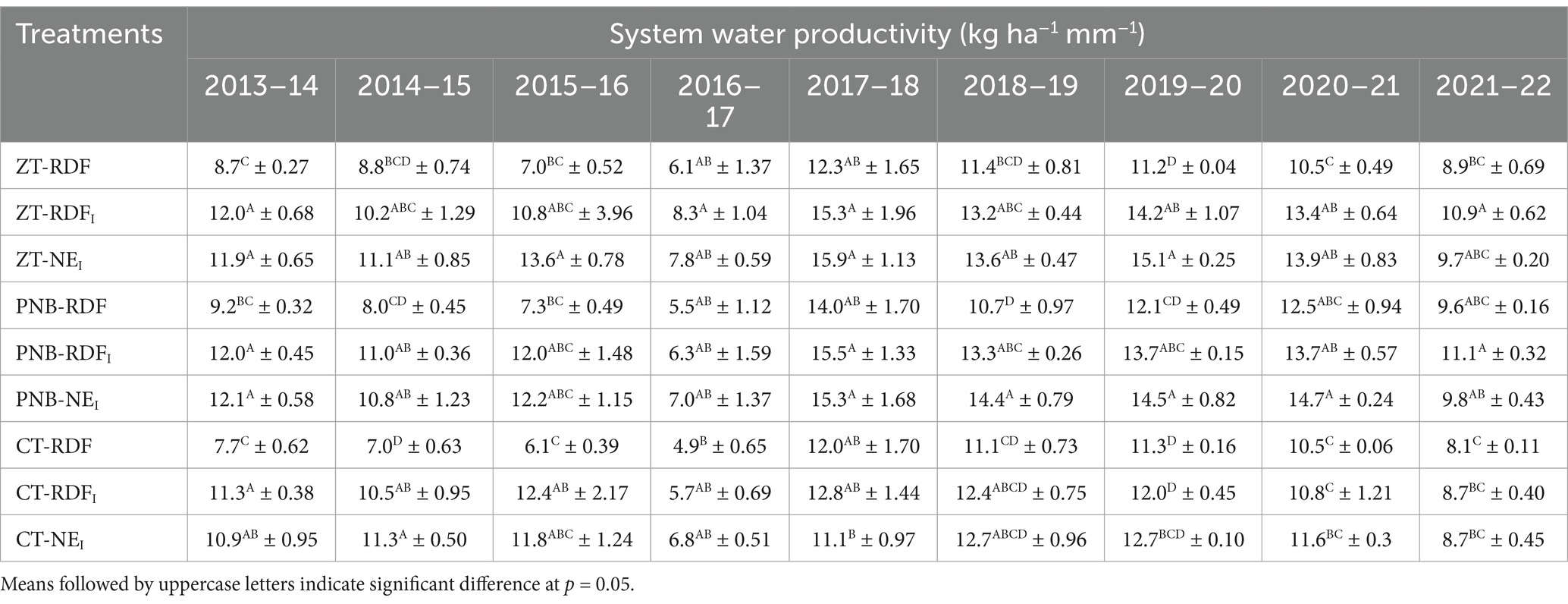
Table 3. Interaction effects of different tillage and nutrient management practices on water productivity (±S.E.) of MMR.
3.5 Soil available N, nitrate-N, and ammonium-N
At tasseling of ninth season maize, sampling was performed from a 0–45 cm soil profile. Among the tillage practices, a significant difference in soil available N was recorded up to 30 cm depth. ZT and PNB had a greater supply of N than CT at 5 cm. However, in 5–15 cm, PNB had a significantly higher N than ZT and CT. Again, in 15–30 m soil depth, PNB was similar to ZT but significantly higher than CT. The soil N did not differ between 30 and 45 cm soil depths due to tillage practices. RDFI and NEI indicate more available soil N in 0–30 cm soil depth. However, at 30–45 cm soil depth, NEI recorded higher soil N than RDFI and RDF (Figures 4a,b). Across different soil depths, ZT and PNB had significantly higher levels of soil NO3− N than CT; however, NEI and RDFI recorded higher levels of NO3− N than RDF (Figures 4c,d). In addition, CA-based tillage (ZT/PNB) had higher levels of soil NH4+-N in 0–30 cm soil depth than CT but was similar in 30–45 cm depth. In the top (0–5 cm) and bottom (30–45 cm) soil layers, nutrient management practices recorded similar soil NH4+-N. However, at 5–30 cm soil depth, NEI and RDFI were significantly superior to RDF (Figures 4e,f).
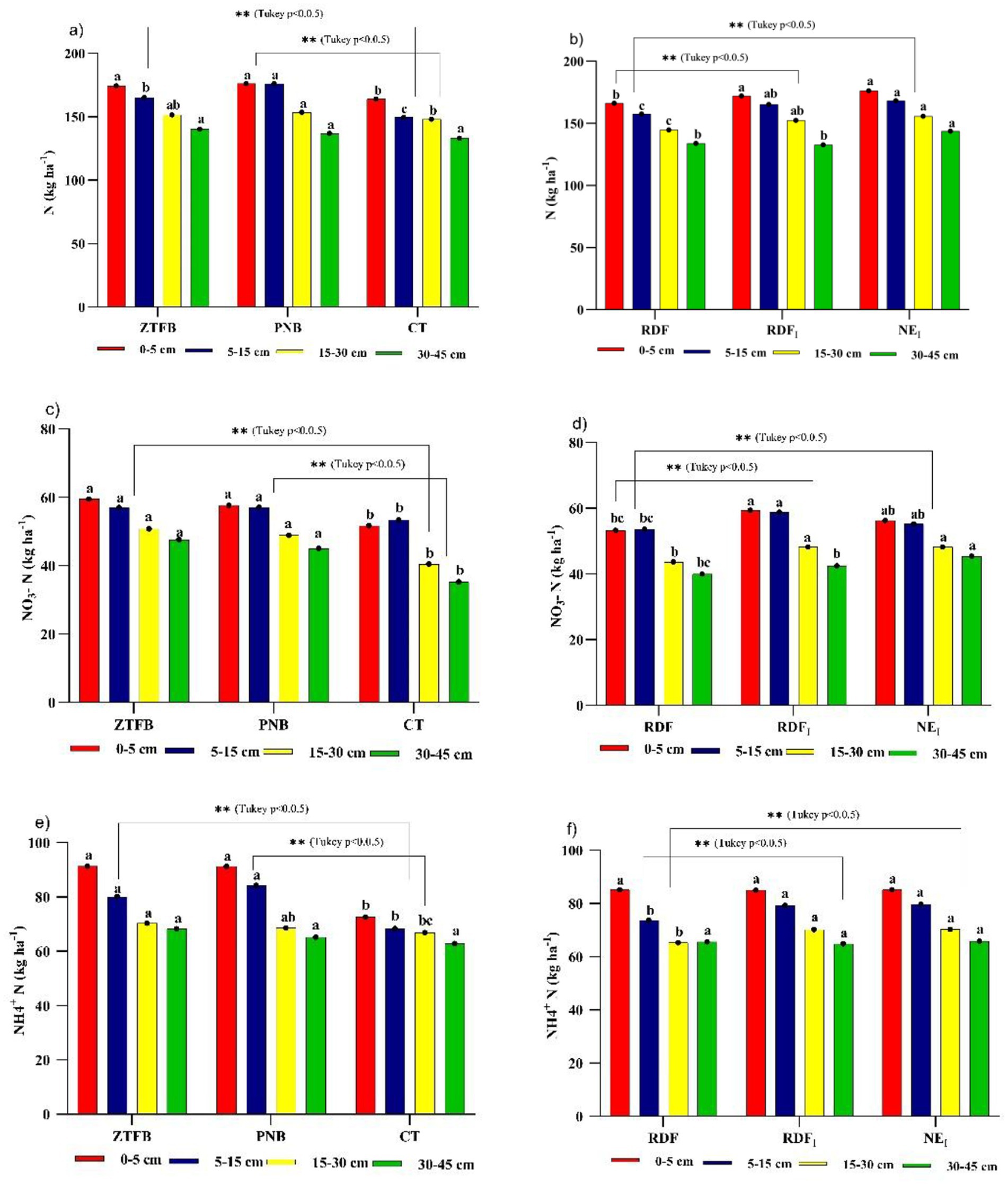
Figure 4. Long-term effect of tillage and nutrient management practices on soil available N (a,b), nitrate-N (NO3–-N) (c,d) and ammonical-N (NH4 + -N) (e,f) at tasseling of 9th season maize under maize-mustard rotation. Means for each parameter with at least one letter common are not significantly different at p0.05 level of significance.
3.6 Soil microbial dynamics
ZT had a larger bacterial population (107), similar to PNB, but significantly greater than CT. Again, in nutrient management, NEI and RDFI showed much larger bacterial counts than RDF plots. Interestingly, the 5–30 cm soil depth had 8.7 and 38.6% more bacterial populations than the 0–5 cm and 30–45 cm soil depths, as a result of adequate moisture (Figures 5a,b). In addition, these CA-based practices recorded significantly higher fungi (104) and actinomycetes (104) counts than the CT-based. However, NEI and RDFI had higher fungal counts than the RDF plots (Figures 5c–f). At 0–5 cm soil depth, PNB had the highest microbial biomass-P (MBP), fb ZT, and CT. At the next soil depths, both ZT and PNB had similar MBPs and were significantly larger than the CT plots. Across soil depths, NEI and RDFI-dominated RDF plots (Figures 6a,b). Furthermore, the PNB plots recorded increased soil microbial biomass-C (MBC) at 0–5 cm depth compared to the ZT and CT plots. However, in subsequent layers (5–45 cm), ZT resulted in more MBC activities than PNB and CT plots. NEI facilitated greater MBC at 0–5 cm soil depth. On the contrary, RDFI had greater MBC at 5–45 cm soil depth than NEI and RDF plots (Figures 6c,d).
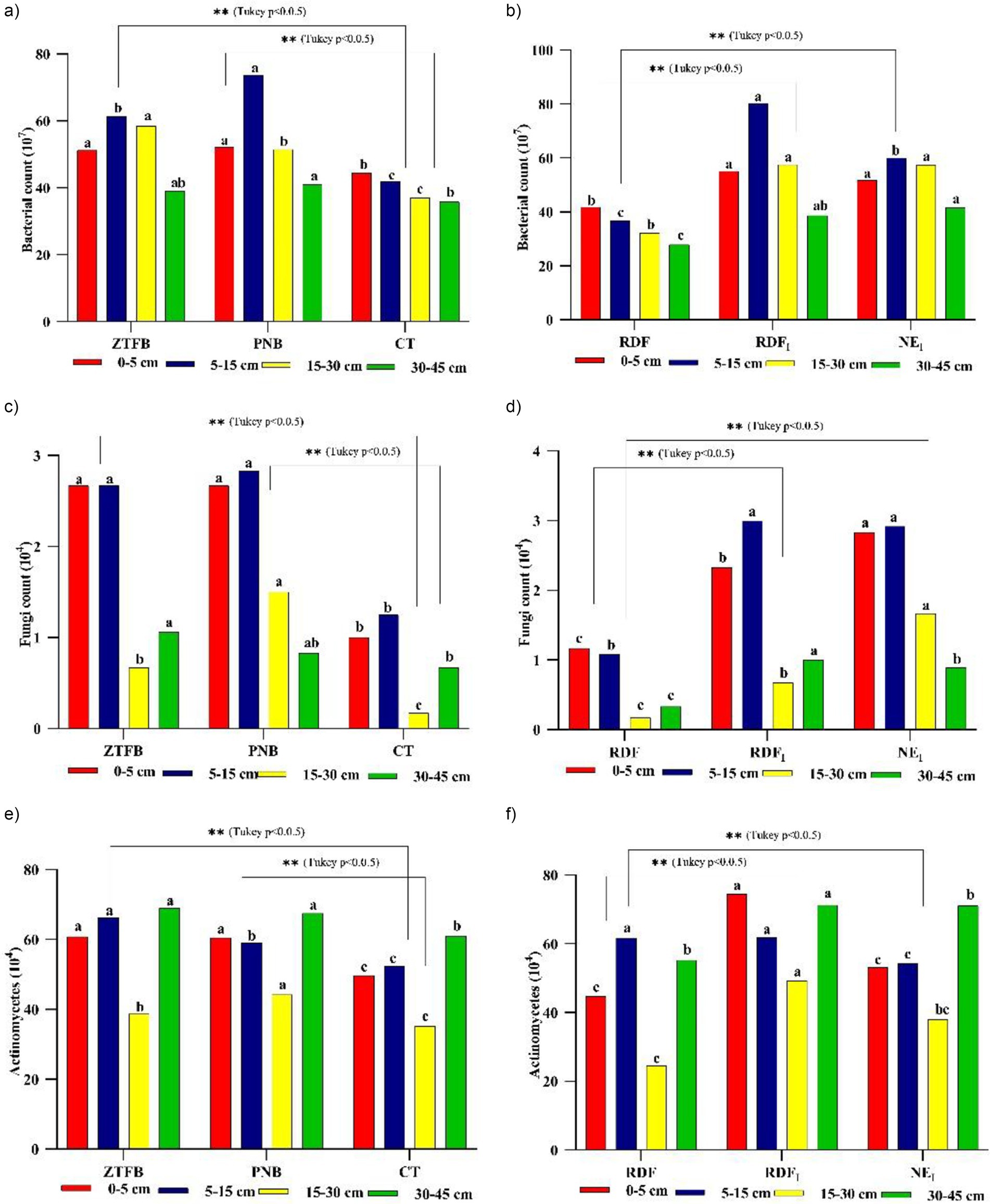
Figure 5. Long-term effect of tillage and nutrient management practices on (a,b), (c,d) and (e,f) counts and at tasseling of 9th season maize under maize-mustard rotation. Means for each parameter with at least one letter common are not significantly different at p0.05 level of significance.
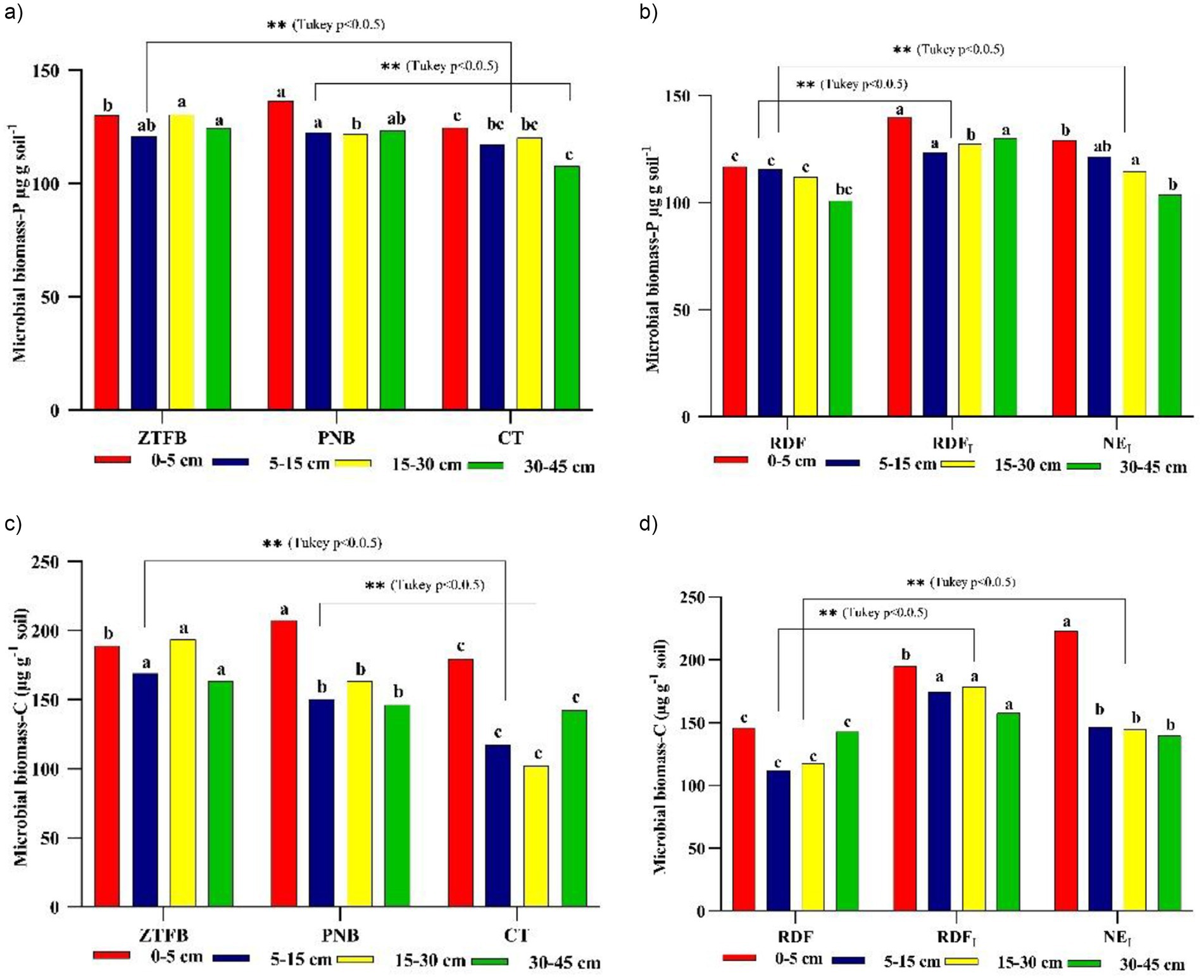
Figure 6. Long-term effect of tillage and nutrient management practices on microbial (a,b) and (c,d) at tasseling of 9th season maize under maize-mustard rotation. Means for each parameter with at least one letter common are not significantly different at p0.05 level of significance.
3.7 Energy footprints
The maximum contribution to non-renewable inputs in the system was from indirect sources, such as the fertilizers and the pesticides, accounting for ~75% of the total. Direct non-renewable inputs, such as diesel and electricity, were the second largest contributors under the CT. In contrast, indirect renewable inputs, including crop seeds and residue, accounted for ~57% of the inputs used under PNB and ZT conditions. The CT required more inputs, such as land preparation, labor use, irrigation, and electricity, than the ZT and PNB (Supplementary Figures 6a,b). The energy input (EI) was highest under PNB (110,585 MJ ha−1), fb the ZT (110,173 MJ ha−1), whereas it was lowest under CT (53,036 MJ ha−1). However, the energy output (EO) was found to be the highest under ZT conditions and was similar to that under PNB conditions, whereas it was the lowest under CT conditions due to the lower system biological yields. The NEI registered the maximum EO at 7.6 and 28.7% higher than the RDFI and RDF, respectively. Furthermore, the net energy (NE), energy use efficiency (EUE), and energy productivity (EP) were found to be higher under the CT than under the PNB and ZT. Similarly, among the nutrient management practices, the NE, EUE, EP, and the non-renewable energy ratio (NRER) were the highest under the NEI, followed by the RDFI and the least under the RDF. The specific energy (SE) was the highest under the ZTFB (18.2 MJ kg−1) and RDF (17.8 MJ kg−1). The interaction between tillage and nutrient management practices indicated that the maximum EI and EO were recorded under PNB-NEI, NE, EUE, and the EP under the CT-NEI, NRER under ZT-NEI, and SE under PNB-RDF treatment combinations (Table 4).
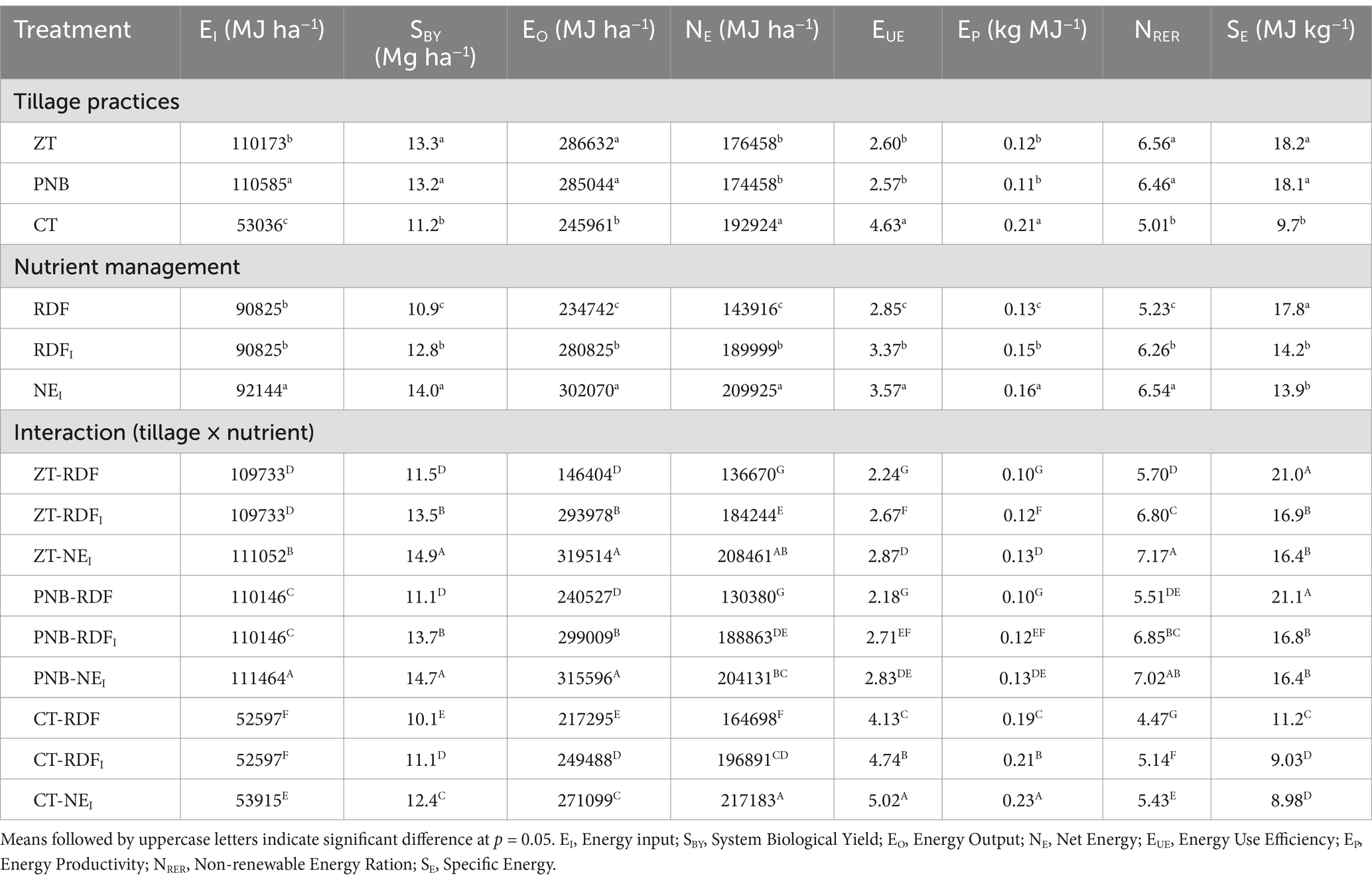
Table 4. Energy dynamics of MMR under different tillage and nutrient management practices (9 yrs. mean).
4 Discussion
The South Asian agro-ecologies are the major vulnerable hotspots for existing and impending climate variability. Conservation tillage (CA) has been considered the most resource-efficient and climate-smart production system to address the enduring challenges of agricultural sustainability (Allen et al., 2011; Jat et al., 2017; Pradhan et al., 2018). Thus, under changing climatic scenarios, the CA-based maize–mustard rotation in conjugation with a user-friendly nutrient expert-assisted (NEI) and recommended fertilization (RDFI) method could be a striking substitute. Our nine-year field analysis of the maize–mustard rotation showed significantly (p = 0.05) greater equivalent yields under ZT/PNB than under CT practices. This might be due to the favorable microclimate resulting from the incessant implementation of CA-based tillage along with a balanced fertilization (Jat et al., 2017; Pooniya et al., 2021). Furthermore, the residue retention under the ZT and the PNB provides a continuous supply of organic matter, thereby improving the soil properties governing the physical environments (Pooniya et al., 2012; Govaerts et al., 2007; Jat et al., 2018), reducing the crop-weed interference (Jat et al., 2019), minimizing the evaporation losses, and improving the water/nutrient extraction pattern due to a more complex root-based mechanism. In fact, we observed greater equivalent yields under CA-based tillage in both excess and deficit rainfall years. The combined effect of residue retention and the growth of maize and mustard crops on the beds may have helped to avoid the adverse effects of short-term waterlogging during heavy rains (Li et al., 2011). Additionally, this approach supports better conservation practices and promotes more efficient use of irrigation water during dry seasons (Thierfelder et al., 2012).
This investigation reinforces the previous findings that adopting the NEI/RDFI would increase the equivalent yields (EY) over the RDF in adjoining regions (Majumdar et al., 2013). The higher EY (26.6–30.3%) under the NEI and RDFI may be attributed to the optimal and balanced application of nutrients during the critical crop growth stages to align with the demand–supply dynamics of the plant nutrients required for higher yields (Pampolino et al., 2012). Additionally, native nutrient sources are considered in the NEI along with yield responses and agronomic efficiencies, and a systematic approach is used to gather site-specific information (Satyanarayana et al., 2013; Pooniya et al., 2015). Maize-based systems generally extract a larger amount of nutrients from the soil profile (Kaiser et al., 2014; Gathala et al., 2015; Zhiipao et al., 2023). Thus, our study reveals the importance of balanced and precise application of nutrients to achieve higher resource use efficiency and improve the yields of the MMR system.
The CA-based residue retention practices combined with efficient nutrient management strategies, such as NEI/RDFI resulted in greater NO3−-N and NH4+-N contents in the upper 30 cm of the soil profile. The increased mineralizable nitrogen in no-tillage systems suggests that soil nitrogen reserves can be improved by reducing or eliminating tillage (Malhi et al., 2018). Since nitrogen is the major limiting factor in crop production and most available nitrogen is used for plant biomass synthesis, soil organic nitrogen should be considered when determining the nutrient requirements of crops. In our study, CA-based tillage and precise nutrient management resulted in a larger microbial population. This can likely attributed to the well-established role of residue-retention no-till practices in nutrient cycling and supporting soil microbes (He et al., 2007). In no-till soils, fungal and bacterial species are dominant (Nicolardot et al., 2007) and are associated with greater diversity (Helgason et al., 2010). As soil fungi are extremely sensitive to physical disturbances, they form hyphal networks that facilitate the translocation of nutrients under no-tillage (Klein and Paschke, 2004). There were also higher amounts of actinomycetes in residue-retained zero- or no-till plots. Crop detritus retention is a carbon source and provides favorable conditions for microbial growth (Salinas-Garcia et al., 2002). Under permanent beds, we observed an increased amount of MBC and MBP. However, in the subsequent layers, ZT plots showed comparable levels of MBC or MBP. Interestingly, the soil depth of 5–30 cm had 38.6% more bacterial populations than the soil depth of 30–45 cm. As soil depth is a critical factor influencing soil microbial communities, their populations may differ with varying depths (Helgason et al., 2009), and the microbial communities sampled from deeper soil profiles may exhibit distinct characteristics compared to those sampled from the surface of the soil. The decrease in SOC and N at lower depths under no-till systems may be a significant factor in the decrease in soil microbial biomass. Substrate availability, soil moisture, temperature, and aeration may collectively influence variations in microbial community across different soil depths.
The declining water table and fluctuating rainfall patterns rambled the search for climate-smart alternative crops and, of course, water-saving technologies. The adoption of CA-based (ZT or PNB) practices coupled with precise nutrient management saved 23.4–26.6% water over CT-based practices. It has been reasoned out that residue retention reduces evaporation from the soil surface and minimizes transpiration losses because of reduced weed growth (Jat et al., 2019). Accordingly, it has a positive effect on soil moisture regimes, saves water, and enhances total water-use efficiency (Biswakarma et al., 2021). Furthermore, the PNBs act as a passage for disposing of excess drainage water conserving soil moisture during dry spells. However, the greater equivalent yields and sufficient nutrient supply under the NEI/RDFI condition elucidate the enhancement in water productivity and thus lower the water footprint.
The study of energy relationships and analysis is key to all farming systems for achieving environmental sustainability and societal welfare (Ghosh et al., 2022). As a developing country, India should shift its focus more toward resource-conservative technologies and depend on renewable energy sources to ensure food and nutritional security amid looming climate changes. Our results revealed that the highest contribution to non-renewable inputs, such as diesel, electricity, and fertilizers, occurred under CT practices. This increase can be attributed to the greater use of machinery and labor for land preparation, pumping of groundwater, and the greater use of agrochemicals under the CT practices. In contrast, CA-based practices indirectly consumed more renewable energy (57%), primarily from crop seeds and residues. This might be due to the higher energy equivalence of crop residues (Chaudhary et al., 2009), indicating that residue retention is the most energy-demanding operation in crop production. Furthermore, the greater energy input (~108%) under the CA-based plots was mainly ascribed to the retention of huge quantities of the residue for the 9 consecutive years compared with the CT plots. However, the greater energy output under ZT/PNB in the main plots and NEI in the sub-plots was primarily due to the greater system equivalent yields (Jat et al., 2019), unlike the reverse in terms of the CT practices. Likewise, the higher net energy (NE), energy use efficiency (EUE), and energy productivity (EP) in the CT and the NEI were due to the zero recycling of the crop residues and the balanced fertilization, respectively, unlike the ZT/PNB plots, which consumed a huge amount of crop residues in the last 9 years (Saad et al., 2016; Jat et al., 2020).
5 Conclusion
Based on the results of a nine-year-long-term field study, the CA-based tillage practices, i.e., ZT or PNB and NEI, and the RDFI enabled the production of greater maize grain equivalents (EY) over the CT and RDF. These long-term tillage practices with precise nutrient management (RDFI or NEI) can substantially reduce water footprints and save system water use compared to CT and RDF plots. Furthermore, these practices had greater bacterial (107), fungi (104), and actinomycetes (104) counts in addition to improved soil microbial biomass-C and -P across soil depths. CT practices consumed greater direct and indirect non-renewable energy than the residue-retained CA-based tillage practices. CA-based practices had greater energy input (EI) than the CT practices, but it was vice versa in terms of the energy output (EO). NEI-guided fertilization registered higher EO than RDFI and RDF. Hence, the residue-retained zero tillage and the permanent bed system in conjugation with expert-guided precise nutrient application (NEI) or improved RDFI is recommended to enhance equivalent yields and soil microbial diversity. This approach also contributes to water-energy savings in the maize–mustard rotation within South Asian agro-ecologies. The future directions of conservation agriculture (CA)-based maize–mustard production include systematic estimation of greenhouse gas emissions and carbon footprints. This will help devise mitigation and adaptation strategies, promote circular or green economies, and achieve the Sustainable Development Goals (SDGs).
Data availability statement
The original contributions presented in the study are included in the article/Supplementary material, further inquiries can be directed to the corresponding authors.
Author contributions
KL: Conceptualization, Data curation, Formal analysis, Investigation, Methodology, Validation, Writing – original draft. VP: Conceptualization, Data curation, Investigation, Methodology, Project administration, Resources, Supervision, Validation, Visualization, Writing – original draft, Writing – review & editing. NB: Data curation, Formal analysis, Investigation, Methodology, Project administration, Supervision, Validation, Visualization, Writing – original draft, Writing – review & editing. RZ: Conceptualization, Data curation, Formal analysis, Investigation, Methodology, Validation, Visualization, Writing – original draft, Writing – review & editing. AS: Formal analysis, Writing – review & editing. DK: Project administration, Resources, Writing – review & editing. YS: Project administration, Resources, Supervision, Writing – review & editing. PG: Investigation, Methodology, Supervision, Validation, Writing – review & editing. RB: Investigation, Writing – review & editing. SSR: Project administration, Resources, Supervision, Writing – review & editing. PU: Data curation, Methodology, Supervision, Visualization, Writing – review & editing. AB: Formal analysis, Methodology, Validation, Writing – original draft. KS: Methodology, Resources, Writing – review & editing. RC: Visualization, Writing – review & editing. RDJ: Resources, Supervision, Writing – review & editing. SR: Methodology, Validation, Writing – review & editing. SJ: Methodology, Resources, Supervision, Writing – review & editing. RJ: Methodology, Writing – review & editing.
Funding
The author(s) declare that no financial support was received for the research and/or publication of this article.
Acknowledgments
We thank the ICAR-Indian Agricultural Research Institute and the Indian Council of Agricultural Research, New Delhi for providing the necessary field and laboratory facilities during the experimentation.
Conflict of interest
The authors declare that the research was conducted in the absence of any commercial or financial relationships that could be construed as a potential conflict of interest.
Publisher’s note
All claims expressed in this article are solely those of the authors and do not necessarily represent those of their affiliated organizations, or those of the publisher, the editors and the reviewers. Any product that may be evaluated in this article, or claim that may be made by its manufacturer, is not guaranteed or endorsed by the publisher.
Supplementary material
The Supplementary material for this article can be found online at: https://www.frontiersin.org/articles/10.3389/fsufs.2025.1359281/full#supplementary-material
References
Adamtey, N., Musyoka, M. W., Zundel, C., Cobo, J. G., Karanja, E., Fiaboe, K. K. M., et al. (2016). Productivity, profitability and partial nutrient balance in maize-based conventional and organic farming systems in Kenya. Agric. Ecosyst. Environ. 235, 61–79. doi: 10.1016/j.agee.2016.10.001
Allen, D. E., Singh, B. P., and Dalal, R. C. (2011). Soil health indicators under climate change: a review of current knowledge in soil health and climate change. Heidelberg: Springer-Verlag, 25–45.
Bhushan, L., Ladha, J. K., Gupta, R. K., Singh, S., Tirol‐Padre, A., Saharawat, Y. S., et al. (2007). Saving of water and labor in a rice–wheat system with no‐tillage and direct seeding technologies. Agron. J. 99, 1288–1296. doi: 10.2134/agronj2006.0227
Biswakarma, N., Pooniya, V., and Zhiipao, R. R. (2020). Assessing productivity and profitability of maize (Zea mays) under conservation tillage and nutrient management. Indian J. of Agron. 65, 368–371. doi: 10.59797/ija.v65i3.2979
Biswakarma, N., Pooniya, V., Zhiipao, R. R., Kumar, D., Shivay, Y. S., das, T. K., et al. (2023). Identification of a resource-efficient integrated crop management practice for the rice-wheat rotations in south Asian indo-Gangetic Plains. Agric. Ecosyst. Environ. 357:108675. doi: 10.1016/j.agee.2023.108675
Biswakarma, N., Pooniya, V., Zhiipao, R. R., Kumar, D., Verma, A. K., Shivay, Y. S., et al. (2021). Five years integrated crop management in direct seeded rice-zero till wheat rotation of North-Western India: effects on soil carbon dynamics, crop yields, water productivity and economic profitability. Agric. Ecosyst. Environ. 318:107492. doi: 10.1016/j.agee.2021.107492
Brockett, B. F. T., Prescott, C. E., and Grayston, S. J. (2012). Soil moisture is the major factor influencing microbial community structure and enzyme activities across seven biogeoclimatic zones in western Canada. Soil Biol. Biochem. 44, 9–20. doi: 10.1016/j.soilbio.2011.09.003
Brookes, P. C., Powlson, D. S., and Jenkinson, D. S. (1982). Measurement of microbial biomass phosphorus in soil. Soil Biol. Biochem. 14, 319–329. doi: 10.1016/0038-0717(82)90001-3
Brouder, S. M., and Gomez-Macpherson, H. (2014). The impact of conservation agriculture on smallholder agricultural yields: a scoping review of the evidence. Agric. Ecosyst. Environ. 187, 11–32. doi: 10.1016/j.agee.2013.08.010
Chaudhary, V. P., Gangwar, B., Pandey, D. K., and Gangwar, K. S. (2009). Energy auditing of diversified rice-wheat cropping systems in indo-Gangetic plains. Energy 34, 1091–1096. doi: 10.1016/j.energy.2009.04.017
Degrune, F., Theodorakopoulos, N., Dufrene, M., Colinet, G., Bodson, B., Hiel, M. P., et al. (2016). No favorable effect of reduced tillage on microbial community diversity in a silty loam soil (Belgium). Agric. Ecosyst. Environ. 224, 12–21. doi: 10.1016/j.agee.2016.03.017
Gaire, A., Koirala, S., Shrestha, R. K., and Amgain, L. P. (2016). Growth and productivity of different cultivars of rice under nutrient expert© and other fertilizer management practices at Lamjung. Int. J. Appl. Sci. Biotechnol. 4, 178–182. doi: 10.3126/ijasbt.v4i2.14974
Gathala, M. K., Jagadish, M. S., Rahman, M. M., Hossain, M. I., Harun, M., Ghosh, A. K., et al. (2015). Conservation agriculture-based tillage and crop establishment options can maintain farmers' yields and increase profits in South Asia’s rice–maize systems: evidence from Bangladesh. Field Crop Res. 172, 85–98. doi: 10.1016/j.fcr.2014.12.003
Ghosh, S., Das, T. K., Rana, K. S., Biswas, D. R., Das, D. K., Singh, G., et al. (2022). Energy budgeting and carbon footprint of contrasting tillage and residue management scenarios in rice-wheat cropping system. Soil Till. Res. 223:105445. doi: 10.1016/j.still.2022.105445
Gil-Sotres, F., Trasar-Cepeda, C., Leiros, M. C., and Seoane, S. (2005). Different approaches to evaluating soil quality using biochemical properties. Soil Bio. Biochem. 37, 877–887. doi: 10.1016/j.soilbio.2004.10.003
GoI (2020–2021). Agricultural statistics division. New Delhi, India: Directorate of Economics and Statistics, Department of Agriculture, Cooperation and Farmers Welfare, Government of India.
Gomez, K. A., and Gomez, A. A. (1984). “Statistical procedures for agricultural research” in An international rice research institute book. 2nd ed (New York: Wiley-Inter-Science, John Wiley & Sons).
Govaerts, B., Sayre, K. D., Lichter, K., Dendooven, L., and Deckers, J. (2007). Influence of permanent bed planting and residue management on physical and chemical soil quality in rainfed maize-wheat system. Plant Soil 291, 39–54. doi: 10.1007/s11104-006-9172-6
Hanway, J. J., and Heidel, H. (1952). Soil analysis methods as used in Iowa state college soil testing laboratory, bulletin 57. USA: Iowa State College of Agriculture, 131.
He, J. Z., Shen, J. P., Zhang, L. M., Zhu, Y. G., Zheng, Y. M., Xu, M. G., et al. (2007). Quantitative analyses of the abundance and composition of ammonia-oxidizing bacteria and ammonia-oxidizing archaea of a Chinese upland red soil under long-term fertilization practices. Environ. Microbiol. 9, 2364–2374. doi: 10.1111/j.1462-2920.2007.01358.x
Helgason, B. L., Walley, F. L., and Germida, J. J. (2009). Fungal and bacterial abundance in long-term no-till and intensive-till soils of the Northern Great Plains. Soil Sci. Soc. Am. J. 73, 120–127. doi: 10.2136/sssaj2007.0392
Helgason, B. L., Walley, F. L., and Germida, J. J. (2010). Long-term no-till management affects microbial biomass but not community composition in Canadian prairie agroecosystems. Soil Biol. Biochem. 42, 2192–2202. doi: 10.1016/j.soilbio.2010.08.015
Jackson, M. L. (1958). Soil chemical analysis. Englewood Cliffs, New Jersey: Prentice Hall, 199–201.
Jantalia, C. P., Resck, D. V. S., Alves, B. J. R., Zotarelli, L., Urquiaga, S., and Boddey, R. M. (2007). Tillage effect on carbon stocks of a clayey oxisol under a soybean-based crop rotation in the Brazilian Cerrado region. Soil Till. Res. 95, 97–109. doi: 10.1016/j.still.2006.11.005
Janvier, C., Villeneuve, F., Alabouvette, C., Edel-Herman, V., Mateille, T., and Steinberg, C. (2007). Soil health through soil disease suppression: which strategy from descriptors to indicators? Soil biol. Biochemist 39, 1–23. doi: 10.1016/j.soilbio.2006.07.001
Jat, M. L., Balyan, J. K., Kumar, V., Rathi, Y. S., Chary, G. R., and Sharma, R. K. (2017). Crop residue management for sustainable production of maize in dryland ecosystem. Chem. Sci. Rev. Lett. 6, 1681–1686.
Jat, M. L., Gathala, M. K., Ladha, J. K., Saharawat, Y. S., Jat, A. S., Kumar, V., et al. (2009). Evaluation of precision land leveling and double zero-till systems in the rice-wheat rotation: water use, productivity, profitability and soil physical properties. Soil Till. Res. 105, 112–121. doi: 10.1016/j.still.2009.06.003
Jat, M. L., Gathala, M. K., Saharawat, Y. S., Tetarwal, J. P., Gupta, R., and Yadvinder, S. (2013). Double no-till and permanent raised beds in maize-wheat rotation of north-western indo-Gangetic plains of India: effects on crop yields, water productivity, profitability and soil physical properties. Field Crop Res. 149, 291–299. doi: 10.1016/j.fcr.2013.04.024
Jat, R. D., Jat, H. S., Nanwal, R. K., Yadav, A. K., Bana, A., Choudhary, K. M., et al. (2018). Conservation agriculture and precision nutrient management practices in maize-wheat system: effects on crop and water productivity and economic profitability. Field Crop Res. 222, 111–120. doi: 10.1016/j.fcr.2018.03.025
Jat, H. S., Kumar, V., Datta, A., Choudhary, M., Singh, Y., Kakraliya, S. K., et al. (2020). Designing profitable, resource-use efficient and environmentally sound cereal-based systems for western indo-Gangetic plains. Sci. Rep. 10:19267. doi: 10.1038/s41598-020-76035-z
Jat, S. L., Parihar, C. M., Singh, A. K., Jat, M. L., Jat, R. K., Singh, D. K., et al. (2011). Conservation agriculture in maize production systems. DMR technical bulletin 2011/4. New Delhi: Directorate of Maize Research, 25.
Jat, S. L., Parihar, C. M., Singh, A. K., Kumar, B., Choudhary, M., Nayak, H. S., et al. (2019). Energy auditing and carbon footprint under long-term conservation agriculture-based intensive maize systems with diverse inorganic nitrogen management options. Sci. Total Environ. 664, 659–668. doi: 10.1016/j.scitotenv.2019.01.425
Kaiser, M., Piegholdt, C., Andruschkewitsch, R., Linsler, D., Koch, H., and Ludwig, B. (2014). Impact of tillage intensity on carbon and nitrogen pools in surface and sub-surface soils of three long-term field experiments. Eur. J. Soil Sci. 65, 499–509. doi: 10.1111/ejss.12146
Klein, D. A., and Paschke, M. W. (2004). Filamentous fungi: the indeterminate lifestyle and microbial ecology. Microb. Ecol. 47, 224–235. doi: 10.1007/s00248-003-1037-4
Leff, J. W., Jones, S. E., Prober, S. M., Barberan, A., Borer, E. T., Firn, J. L., et al. (2015). Consistent responses of soil microbial communities to elevated nutrient inputs in grasslands across the globe. Proc. Natl. Acad. Sci. U. S. A. 112, 10967–10972. doi: 10.1073/pnas.1508382112
Li, L. L., Huang, G. B., Zhang, R. Z., Bill, R., Guangdi, L., and Kwong, Y. C. (2011). Benefits of conservation agriculture on soil and water conservation and its progress in China. Agric. Sci. China 10, 850–859. doi: 10.1016/S1671-2927(11)60071-0
Limbalkar, O. M., Singh, R., Kumar, P., Nanjundan, J., Parihar, C. M., Vasisth, P., et al. (2021). Deployment of Brassica carinata A. Braun derived Brassica juncea (L.) Czern. Lines for improving heterosis and water use efficiency under water deficit stress conditions. Front. Plant Sci. 12:765645. doi: 10.3389/fpls.2021.765645
Majumdar, K., Satyanarayana, T., Pampolino, M., Dutta, S., Jat, M. L., Sulewski, G., et al. (2013). Nutrient expert® for hybrid maize (version 1.1). A decision support tool for providing field specific fertilizer recommendations for tropical hybrid maize. India: International Plant Nutrition Institute and International Maize and Wheat Improvement Centre, 52.
Malhi, S. S., Légère, A., Vanasse, A., and Parent, G. (2018). Effects of long-term tillage, terminating no-till and cropping system on organic C and N, and available nutrients in a Gleysolic soil in Québec, Canada. J. Agri. Sci. 156, 472–480. doi: 10.1017/S0021859618000448
Melero, S., Lopez-Bellido, R. J., Lopez-Bellido, L., Munoz-Romero, V., Moreno, F., and Murillo, J. M. (2011). Long-term effect of tillage, rotation and nitrogen fertilizer on soil quality in a Mediterranean vertisol. Soil Till. Res. 114, 97–107. doi: 10.1016/j.still.2011.04.007
Mohammad, A., Sudhishri, S., Das, T. K., Singh, M., Bhattacharyya, R., Dass, A., et al. (2018). Water balance in direct seeded rice under conservation agriculture in north-western indo-Gangetic Plains of India. Irrig. Sci. 36, 381–393. doi: 10.1007/s00271-018-0590-z
Nicolardot, B., Bouziri, L., Bastian, F., and Ranjard, L. (2007). A microcosm experiment to evaluate the influence of location and quality of plant residues on residue decomposition and genetic structure of soil microbial communities. Soil Biol. Biochem. 39, 1631–1644. doi: 10.1016/j.soilbio.2007.01.012
Olsen, S. R., Cole, C. V., Watanabe, F. S., and Dean, L. (1954a). Estimation of available phosphorus in soil by extraction with sodium carbonate. Washington: USDA, 933 (OCoLC) 755265710.
Olsen, S. R., Cole, C. V., Watanabe, F. S., and Dean, L. A. (1954b). Estimation of available phosphorus in soils by extraction with sodium bicarbonate. Washington, DC: US Dept Agric Circ 939.
Pampolino, M., Majumdar, K., Jat, M. L., Satyanarayana, T., Kumar, A., Shahi, V. B., et al. (2012). Development and evaluation of nutrient expert for wheat in South Asia (special issue): nutrient management for wheat. Better Crops 96, 29–31.
Parihar, C. M., Meena, B. R., Nayak, H. S., Patra, K., Sena, D. R., Singh, R., et al. (2022). Co-implementation of precision nutrient management in long-term conservation agriculture-based systems: a step towards sustainable energy-water-food nexus. Energy 254:124243. doi: 10.1016/j.energy.2022.124243
Pooniya, V., Jat, S. L., Choudhary, A. K., Singh, A. K., Parihar, C. M., Bana, R. S., et al. (2015). ‘Nutrient expert’ assisted site-specific-nutrient-management: an alternative precision fertilization technology for maize-wheat cropping system in South-Asian Indo-Gangetic Plains. Indian J. Agril. Sci. 85, 996–1002. doi: 10.56093/ijas.v85i8.50796
Pooniya, V., Shivay, Y. S., Rana, A., Nain, L., and Prasanna, R. (2012). Enhancing soil nutrient dynamics and productivity of basmati rice through residue incorporation and zinc fertilization. Eur. J. Agron. 41, 28–37. doi: 10.1016/j.eja.2012.03.004
Pooniya, V., Zhiipao, R. R., Biswakarma, N., Jat, S. L., Kumar, D., Parihar, C. M., et al. (2021). Long-term conservation agriculture and best nutrient management improves productivity and profitability coupled with soil properties of a maize-chickpea rotation. Sci. Rep. 11:10386. doi: 10.1038/s41598-021-89737-9
Pooniya, V., Zhiipao, R. R., Biswakarma, N., Kumar, D., Shivay, Y. S., Babu, S., et al. (2022). Conservation agriculture based integrated crop management sustains productivity and economic profitability along with soil properties of the maize-wheat rotation. Sci. Rep. 12:1962. doi: 10.1038/s41598-022-05962-w
Pradhan, A., Chan, C., Roul, P. K., Halbrendt, J., and Sipes, B. (2018). Potential of conservation agriculture (CA) for climate change adaptation and food security under rainfed uplands of India: a transdisciplinary approach. Agric. Syst. 163, 27–35. doi: 10.1016/j.agsy.2017.01.002
Saad, A. A., Das, T. K., Rana, D. S., Sharma, A. R., Bhattacharyya, R., and Lal, K. (2016). Energy auditing of a maize-wheat-greengram cropping system under conventional and conservation agriculture in irrigated north-western indo-Gangetic Plains. Energy 116, 293–305. doi: 10.1016/j.energy.2016.09.115
Salinas-Garcia, J. R., Velazquez-Garcia, J. D., Gallardo-Valdez, A., Diaz-Mederos, P., Caballero-Hernandez, F., Tapia-Vargas, L. M., et al. (2002). Tillage effects on microbial biomass and nutrient distribution in soils under rain-fed corn production in Central-Western Mexico. Soil Till. Res. 66, 143–152. doi: 10.1016/S0167-1987(02)00022-3
Sapkota, T. B., Majumder, K., Jat, M. L., Kumar, A., Bishnoi, D. K., Mcdonald, A. J., et al. (2014). Precision nutrient management in conservation agriculture-based wheat production system of north-west India: profitability, nutrient use efficiency and environmental footprint. Field Crop Res. 155, 233–244. doi: 10.1016/j.fcr.2013.09.001
Satyanarayana, T., Majumdar, K., Pampolino, M., Johnston, A. M., and Jat, M. L. (2013). Nutrient expert™: a tool to optimize nutrient use and improve productivity of maize. Better Crops 97, 21–24.
Schloter, M., Dilly, O., and Munch, J. C. (2003). Indicators for evaluating soil quality. Agric. Ecosyst. Environ. 98, 255–262. doi: 10.1016/S0167-8809(03)00085-9
Schloter, M., Nannipieri, P., Sørensen, S. J., and Van Elsas, J. D. (2018). Microbial indicators for soil quality. Biol. Fertil. Soils 54, 1–10. doi: 10.1007/s00374-017-1248-3
Schuffelen, A. C., Muller, A., and Van Schouwenburg, J. C. (1961). Quick-tests for-soil and plant analysis used by small laboratories. Netherlands J. Agri. Sci. 9, 2–16. doi: 10.18174/njas.v9i1.17630
Shiferaw, B., Prasanna, B. M., Hellin, J., and Banziger, M. (2011). Crops that feed the world 6. Past successes and future challenges to the role played by maize in global food security. Food Secur. 3, 307–327. doi: 10.1007/s12571-011-0140-5
Stevenson, J. R., Serraj, R., and Cassman, K. G. (2014). Evaluating conservation agriculture for small-scale farmers in sub-Saharan Africa and South Asia. Agric. Ecosyst. Environ. 187, 1–10. doi: 10.1016/j.agee.2014.01.018
Subbiah, B. V., and Asija, G. L. (1956). A rapid procedure for the estimation of available nitrogen in soils. Curr. Sci. 25, 259–260.
Thierfelder, C., Cheesman, S., and Rusinamhodzi, L. (2012). A comparative analysis of conservation agriculture systems: benefits and challenges of rotations and intercropping in Zimbabwe. Field Crop Res. 137, 237–250. doi: 10.1016/j.fcr.2012.08.017
Vance, E. D., Brookes, P. C., and Jenkinson, D. S. (1987). An extraction method for measuring soil microbial biomass carbon. Soil Biol. Biochem. 19, 703–704.
Zhiipao, R. R., Pooniya, V., Biswakarma, N., Kumar, D., Shivay, Y. S., Dass, A., et al. (2023). Timely sown maize hybrids improve the post-anthesis dry matter accumulation, nutrient acquisition and crop productivity. Sci. Rep. 13:1688. doi: 10.1038/s41598-023-28224-9
Keywords: conservation tillage, soil microbial dynamics, nutrient expert-system, water-energy footprints, equivalent yields
Citation: Lakhena KK, Pooniya V, Biswakarma N, Zhiipao RR, Singh A, Kumar D, Shivay YS, Govindasamy P, Bana RS, Rathore SS, Upadhayay PK, Barman A, Swarnalakshmi K, Choudhary RL, Jat RD, Ramakrishnan S, Jat SL and Jat R (2025) Impact of nine years of conservation tillage and precise nutrient management on equivalent yields, soil microbial dynamics, and water-energy footprints of the maize–mustard rotation. Front. Sustain. Food Syst. 9:1359281. doi: 10.3389/fsufs.2025.1359281
Edited by:
Vassilios D. Litskas, Independent Researcher, Lefkosia, CyprusReviewed by:
Luis F. Goulao, University of Lisbon, PortugalJuan Fernando Hirzel, Agricultural Research Institute, Chile
Copyright © 2025 Lakhena, Pooniya, Biswakarma, Zhiipao, Singh, Kumar, Shivay, Govindasamy, Bana, Rathore, Upadhayay, Barman, Swarnalakshmi, Choudhary, Jat, Ramakrishnan, Jat and Jat. This is an open-access article distributed under the terms of the Creative Commons Attribution License (CC BY). The use, distribution or reproduction in other forums is permitted, provided the original author(s) and the copyright owner(s) are credited and that the original publication in this journal is cited, in accordance with accepted academic practice. No use, distribution or reproduction is permitted which does not comply with these terms.
*Correspondence: Vijay Pooniya, dnBvb25peWFAZ21haWwuY29t; Niraj Biswakarma, Ymlzd2FuaXJhajY2NkBnbWFpbC5jb20=; R. R. Zhiipao, emhpaXBvcnVzdHVtQGdtYWlsLmNvbQ==; Arjun Singh, YXJqdW5zaWFyaUBnbWFpbC5jb20=