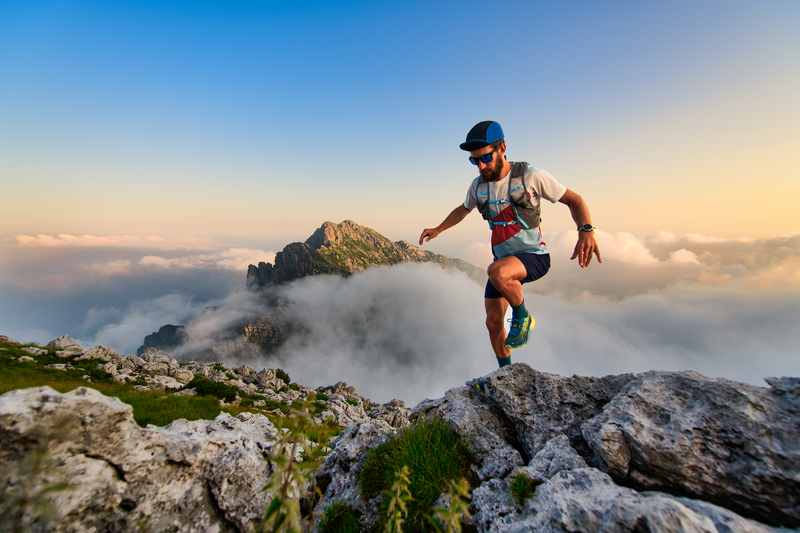
95% of researchers rate our articles as excellent or good
Learn more about the work of our research integrity team to safeguard the quality of each article we publish.
Find out more
REVIEW article
Front. Sustain. Food Syst. , 08 January 2025
Sec. Sustainable Food Processing
Volume 8 - 2024 | https://doi.org/10.3389/fsufs.2024.1506573
This article is part of the Research Topic Sustainable Functional Food’s Sourcing, Production, And Process View all 5 articles
Fish are an important source of animal protein, providing 17% of the world's meat consumption. More than 3 billion people rely on fish for 20 % of their daily protein intake. In addition to a high protein content, fish contain essential fatty acids which are beneficial to human health. However, this key food source will be depleted by 2048 if current global trends of climate change, overfishing, and pollution continue. Considering the threat to sustainability and the need for marine ecosystem recovery, “clean fish”—grown in a laboratory—could be a potential solution. Since cell-based fish are produced in clean rooms where airborne particulates, contaminants, and pollutants are kept within strict limits, these engineered fish do not contain any health-risking factors. Therefore, clean fish can provide people with sustainable and nutritional diets while contributing to the recovery of the marine ecosystem. This review will discuss topics, including cell-based fish, the edible part of fish, technology, and commercialization.
Fish is an essential food source, providing the world population with high-quality nutrients such as animal protein, micronutrients, and essential fatty acids naturally produced essentially only in the marine environment (Kawarazuka and Béné, 2010; Hicks et al., 2019; Pal et al., 2018; Balami et al., 2019). According to the Food and Agriculture Organization of the United Nations (FAO), fish protein accounts for ~17% of the animal protein consumed by the world's population (FAO, 2020). In particular, the reliance upon the consumption of fish is greater in regions where livestock is relatively short in supply, including Africa (17%) and Asia (26%) (FAO, 2000). It is estimated that a population of 1 billion people are relying on fish as the primary animal protein source (FAO, 2000). However, this key food source will be depleted by 2048 if current global trends of climate change, overfishing, and pollution continue (Worm et al., 2006).
At the same time, the global population is expected to increase from 7.7 billion (2019) to 9.7 billion (2050) (Maja and Ayano, 2021). The rapidly growing population will cause food scarcity since the supply will not be able to satisfy the demand. Billions of people are already consuming more resources than the Earth can provide. Therefore, each new-born individual adds to the threat of food insecurity and the pressure on the world food supply. This pressure is the primary cause of global issues, such as global warming, climate change, ocean acidification, and species extinction.
Because of the increased demand for seafood, the ocean is overfished ~3 times more than it can sustain. Moreover, most of the seafood consumed is contaminated by human-caused pollution, including microplastics, mercury, and toxins (Danopoulos et al., 2020; de Almeida Rodrigues et al., 2019; Marques et al., 2010). Consequently, human health is threatened by eating contaminated fish, which is otherwise a valuable source of nutrients. Besides pollution, fisheries also damage the habitat of various marine creatures (Green et al., 2014). This results in damaged ecosystems, further threatening marine life.
In addition to their nutritional value, fish are a source of bioactive compounds that exhibit significant therapeutic potential. Recent studies have demonstrated the health-promoting attributes of fish-based bioactives, including peptides, polyunsaturated fatty acids, and antioxidants, which have anti-inflammatory, antimicrobial, and cardiovascular benefits. Furthermore, bioactive molecules isolated from fish mucus have shown antibacterial and antibiofilm properties, highlighting their potential in preventing bacterial infections and supporting wound healing (Patel et al., 2020). Probiotic strains such as Enterococcus durans F3, derived from the gut of freshwater fish, have also demonstrated benefits for gut health and immune function (Alshammari et al., 2019). These findings underscore the therapeutic importance of fish products and the need to preserve their availability for both nutritional and medical applications.
Fish farming has arisen as a solution to the abovementioned issues. Although this alternative has somewhat narrowed the gap between the demand and supply, it has also created other problems. These farmed fish are kept in high density where diseases are rapidly spread. To avoid the spreading of infectious diseases, various chemicals, including antibiotics, are used (Cabello, 2006). Furthermore, the escapees can spread the disease to wild animals, which can threaten the survival of the native populations (Yang et al., 2019). Fish farming was introduced to help the recovery of the marine ecosystem. However, the irony is that aquacultured fish are fed with feed derived from wild-caught fish (Merino et al., 2012).
Another alternative introduced was plant-based protein, which eliminates animals from the manufacturing process. The consumption of plant-based protein, such as tofu, was first documented as early as 965 CE in China (Shurtleff and Aoyagi, 2013). Recently, plant-based (meatless) meat has gained popularity among consumers concerned about environmental and ethical issues. Unlike conventional plant-based protein (tofu), plant-based meat aims to mimic the appearance, texture, and taste of real meat. However, meeting consumer expectations remains challenging, as plant-based diets often lack certain essential nutrients critical for a healthy life (Neufingerl and Eilander, 2021; Gorissen and Witard, 2018; Gibson and Ferguson, 1998).
In the midst of all this, 70% more food must be produced by 2050 to satisfy the world's demand (FAO, 2011). Is there any hope for overcoming this world crisis using the remaining natural resources?
Once thought to be an inexhaustible food source, the ocean is now being overexploited. Considering the threat to sustainability and the need for marine ecosystem recovery, “clean fish” might be a potential solution. The concept of “clean fish” is to produce edible fish based on tissue engineering (TE) techniques. Briefly, fish cells are isolated from the fish tissue and cultured under appropriate conditions. Then, the cells are cultured on three-dimensional (3D)-shaped structures called scaffolds and induced to mature into whole tissue (Figure 1). Since cell-based fish are produced in clean rooms where airborne particulates, contaminants, and pollutants are kept within strict limits, these engineered fish do not contain any health-risk factors. Therefore, clean fish can provide people with sustainable and nutritional diets while contributing to the recovery of the marine ecosystem. However, the impact of clean fish on ecosystems requires a more cautious outlook. While this approach could reduce overfishing pressures, the timeline for noticeable ecological recovery remains uncertain. Moreover, challenges related to scalability and production costs must be addressed before these benefits can be fully realized. Extensive research is necessary to substantiate claims regarding its ecosystem impact. As an example, during the COVID-19 lockdown, unforeseen changes were observed in wildlife due to restricted human activities (Manenti et al., 2020; Arora et al., 2020; Rupani et al., 2020). Although this pandemic was a human tragedy, the “Great Pause” has benefited wildlife. Likewise, while clean fish could play a role in alleviating marine exploitation, substantial efforts will be required to achieve this on a meaningful scale.
The creation of lab-grown fish starts with the preparation of the cells. Cells are the smallest building blocks of all living organisms. An ensemble of cells with similar structure and function makes up a tissue (Farley et al., 2012). In fish, cells are isolated from the edible part, which is the skeletal muscle tissue, which strongly affects the quality of the fish flesh. This protein source is composed of highly dense and closely packed muscle fibers. The longitudinal muscle fibers are organized into myofibers, which are produced through the fusion of the primary cells known as myosatellite cells (Figure 2) (Frontera and Ochala, 2015). Myosatellite cells are stem cells capable of developing into skeletal muscle tissue. Therefore, cells should be isolated from the muscle tissue of the target fish type. The isolation and culture conditions of the muscle cells vary depending on the fish type. There are various studies on the isolation of primary muscle cells (Table 1).
Cells alone do not have the ability to develop into functional tissue. Accordingly, a supportive platform has been introduced to guide the cells to mature into a whole tissue. In the field of TE, this platform is called a scaffold (Chan and Leong, 2008). Researchers developing lab grown fish have acquired TE techniques, including the fabrication of scaffolds. The isolated cells are grown on scaffolds to develop 3D-shaped tissues. The characteristics of the scaffold may affect the structure and texture quality of the final product. Therefore, the material and design of the scaffolds should be carefully considered. Figure 3 shows the type of scaffolds used to develop lab-grown meat or fish. Scaffolds are designed in various ways, including fibrous, sponge, mesh, and cell-laden types.
Since cells are essential in lab-grown fish, the cell culture medium is one of the most important factors. The early types of culture medium were composed of a balanced solution with pH, osmolarity, and salt concentration similar to that of bodily fluids (Xu et al., 2020; Yao and Asayama, 2017). Gradually, the culture medium was further developed by adding essential components, including glucose, amino acids, inorganic salts, vitamins, and buffers (Eagle, 1955). This is called the basal medium. However, additional supplements are required for long-term cell culture. The most common constituent is fetal bovine serum (FBS), which is derived from unborn calves at the slaughterhouse (Gstraunthaler, 2003). FBS is obtained from the fetuses of pregnant cows during the slaughter process. Blood is extracted using a vacuum pump, gravity, or massage by inserting a needle into the heart of the fetus, without anesthesia. This process, carried out within 5–35 min after slaughter, results in the fetus's brain function remaining intact due to their higher resistance to anoxia compared to adults. Consequently, it is highly likely that the fetuses survive and experience pain during the blood collection process (Jochems et al., 2002). This action raised ethical concerns related to animal welfare.
Collecting blood from a living fetus during the final third of its development violates EU Directive 2010/63/EU regulations. However, if the fetus is considered already dead prior to blood collection, its death by asphyxiation without anesthesia or euthanasia raises significant animal welfare concerns (Weber and Wagner, 2021). Other problematic issues regarding FBS are the large variability in quality and composition, the possibility of contamination, and the containment of toxic factors (van der Valk, 2022). The composition of FBS is not precisely defined and varies between batches. Furthermore, it is susceptible to contamination by viruses, mycoplasma, and prions. These factors present significant scientific challenges concerning the reproducibility of experiments and the safety of products utilizing FBS (Van der Valk et al., 2004).
Therefore, a replacement is necessary for the development of edible cell-cultured fish. Another reason for the need for an alternative to FBS is to reduce the production cost, which directly affects the price of the final product. The production cost of cultured meat is heavily influenced by the culture medium, which constitutes ~55 to 95% of the total cost. Containing 10–20% FBS, priced at approximately $1,000 per liter, this medium poses a major challenge to the commercial scalability of cultured meat production. Professor Mark Post has estimated that around 50 liters of FBS would be needed to produce a single cultured meat patty weighing 140 grams (Hubalek et al., 2022). As much as 30% of the lab-grown fish production cost can be attributed to the cost of the cell culture media (Garrison et al., 2022). So far, no corporation has formulated a fish cell culture medium with an FBS alternative.
To address these challenges, researchers are exploring alternatives such as plant-based proteins and recombinant proteins. Protein-rich sources, including soybeans, soy, and wheat, present a safe, environmentally sustainable, and nutritionally valuable alternative. Plant-derived peptides serve as an abundant source of amino acids, which are critical for protein synthesis and cellular function. These peptides contribute to enhancing cell growth, performance, differentiation, and sustainability in serum-free media (Amirvaresi and Ovissipour, 2024). However, the sensory characteristics (appearance, aroma, and flavor) are less preferred by consumers, necessitating additional processes to improve these attributes (Rubio et al., 2020).
Recombinant proteins also offer potential solutions. The technology of genetically engineering microorganisms to produce growth factors enables the large-scale production of essential components for cell culture. While this approach is promising, there are potential risks associated with genetic engineering, and further research is required to enhance production efficiency.
An example of these advancements is the use of rapeseed protein isolate (RPI) derived from four types of plants as a replacement for expensive recombinant albumin. This method has shown promise in culturing and proliferating bovine satellite cells at a lower cost, demonstrating the feasibility of plant-based alternatives for serum-free media (Stout et al., 2023).
Skeletal muscle tissues are composed of specialized cells with the ability to shorten or contract, generating movement of the body. Unlike mammalian muscle structure, fish lack the tendon tissue that connects the muscle to the bone. Instead, fish skeletal muscle tissue is directly anchored to the skin and bone. Fish skeletal muscle can be divided into two different types: white muscle and red muscle (Figure 4). The color variation strongly depends on the amount of myoglobin present; red muscle has a high myoglobin content. Myoglobin is the main heme sarcoplasmic protein responsible for oxygen transport. The portion of red muscle in fish only accounts for < 10% of the total muscle mass. In most fish types, the largest red muscle mass is located near the lateral line below the skin. This muscle is recruited during slow to moderate continuous swimming speeds. In contrast to red muscle, white muscle is almost devoid of myoglobin, resulting in reduced oxygen-transport ability. Therefore, white muscle is used for fast, burst swimming, including predation and escape behavior, and fatigues rapidly. The fast muscle tissue corresponds to 70–90% of the entire muscle mass. This part of the fish becomes commercially important since it is the edible portion.
A fish filet is composed of W-shaped myomeres, which are muscle segments divided by myosepta, a connective tissue membrane consisting mainly of collagen fibers (Gemballa and Vogel, 2002; Van Leeuwen, 1999) (Figure 5). The myosepta dissolve easily upon cooking and turn into gelatin, which is why the “flakes” (muscle bundles in the myomere) tend to fall apart after cooking (Bricard et al., 2014) (Figure 6). Each myomere consists of muscle fibers (cells) organized in the form of separate bundles arranged in parallel. Compared to mammals, fish muscle fibers are relatively short. In fish, a single muscle fiber is approximately 20–100 mm in diameter and made up of myofibrils (1–2 mm in diameter). The unit of the myofibril is the sarcomere, which contains two sets of filaments, actin (thin filament) and myosin (thick filament). Not only the contractility of the muscle, but also the texture of fish strongly depends on the myofibrillar proteins, such as myosin and actin (Hatae et al., 1984, 1990; Rayment et al., 1993).
As mentioned above, fish is an essential part of a healthy diet, providing important nutrients, including protein and polyunsaturated fatty acids (PUFA), eicosapentaenoic acid (EPA), and docosahexaenoic acid (DHA) (Li and Hu, 2009). Fish are the most diverse group among vertebrates, and the nutrient content can vary due to the species of fish, ecological factors (e.g., diet, environment, trophic level, metabolism), and sociocultural factors (e.g., cooking method, preparation method, parts consumed).
The consumer's decision to purchase meat depends on its quality, which can be sensed by factors such as appearance, taste, and freshness. In general, the meat quality is determined by the muscle fibers, intramuscular connective tissue, and intramuscular fat (Listrat et al., 2016). Because of the bonding of muscle fibers by the connective tissue, the softening of the fish meat is highly dependent on the extracellular matrix (ECM) components of the connective tissue, which is mainly collagen. Specifically, collagen type V in the ECM of the connective tissue is solubilized in postmortem fish muscle (Sato et al., 1997). Although the biochemical activity behind this postmortem tenderization is still unclear, Kubota et al. (2001) demonstrated that metalloproteinases are responsible for the postmortem tenderization of fish muscles. For these reasons, the storage method is of great importance.
Interest in future food, such as animal protein substitutes, has increased rapidly over the past decade. The global plant-based meat market is projected to grow from $4.6 billion (2018) to $85 billion (2030). Meanwhile, cell-based substitutes are commercially available only in Singapore. However, research and development (R&D) are proceeding rapidly. Recently, 3D bioprinting has gained much attention among cell-based meat companies.
The conventional method of manufacturing cell-based meat is based on the self-assembly of the cells. When cells are co-cultured in a suitable environment, such as in bioreactors, cells tend to organize and arrange themselves into complex structures without external manipulation. This approach has the potential to enhance the efficiency and scalability of cell-based meat production, making it more commercially viable. While self-assembling techniques show promise, ongoing research is needed to optimize the process and further improve the quality, taste, and nutritional composition of cell-based meat products.
3D bioprinting is an emerging field that holds great potential for various applications in medicine and TE. While significant progress has been made in the bioprinting of human tissues and organs, such as skin, cartilage, and bone, bioprinting of cell-cultured products is still in the early stages of development.
Research on 3D bioprinting of cultured meat has been gaining attention in recent years as a potential solution to address sustainability and ethical concerns associated with traditional livestock farming. However, there have been limited studies on the 3D bioprinting of cell-based fish or meat. Jeong et al. (2022) used a digital light processing-based (DLP) printing technology to produce steak-type cultured meat. Bovine embryonic fibroblasts in the gelatin methacryloyl (GelMA) scaffold were differentiated into muscle cells and adipocytes to resemble real meat. The cultured meat maintained its shape after frying and cutting. In another study, Kang et al. (2021) endeavored to create meat-like tissue with a hierarchical structure resembling natural meat by assembling cell fibers. In this manufacturing process, tendon-gel integrated bioprinting technology was used to fabricate cultured steak composed of muscle, fat, and vascular tissue. This approach allowed for the precise placement of cell fibers to mimic the arrangement found in real meat. According to Ben-Arye et al. (2020), a 3D-printed scaffold composed of soy protein can support bovine satellite cells to become bovine muscle tissue for cell-based meat. Not only provided the 3D scaffold a supportive platform, but it also offered an environment for the cells to grow and organize into muscle tissue.
It is important to note that 3D bioprinting of cultured meat is still in its early stages, and there are many technical and regulatory challenges to overcome. The complexity of mimicking the intricate structure and texture of conventional meat poses significant hurdles. However, ongoing R&D efforts are expected to advance the field and pave the way for the commercial production of cultured meat in the future. Table 2 summarizes the advantages and limitations of conventional methods and 3D bioprinting in the production of cultured meat and fish.
In 2013, a Dutch company called Mosa Meat, led by Dr. Mark Post, showcased the world's first cell-cultured burger created using muscle cells extracted from a cow and grown in a laboratory, marking a significant milestone in the development of cultured meat. In December 2020, the Singapore Food Agency (SFA) became the first regulatory body to approve a cultured meat product, granting Eat Just permission to sell its cell-cultured chicken. This lab-grown chicken nugget, available at Huber's Butchery in Singapore for around $14, underwent a regulatory review process lasting over 2 years.
Since then, progress has continued worldwide. In June 2023, the United States Department of Agriculture (USDA) approved the sale of cell-cultured chicken produced by Upside Foods and Eat Just, representing a major step toward commercialization in the U.S. market. In January 2024, Israel approved a cell-cultured beef product developed by Aleph Farms, expanding global acceptance of cultured meat. Additionally, Vow, an Australian start-up, developed a cultured quail meat product that received approval from the Singapore Food Agency in April 2024, marking Singapore's second approval and the fourth globally for cultured meat.
Despite these advancements, cell-based fish, including cell-based seafood, has not yet received regulatory approval in any country. Research and development efforts continue, and regulatory approval for such products is expected as the technology and frameworks evolve.
According to consulting firm AT Kearney, cell-based meat could substitute 30% of the global meat market by 2030 and increase to 60% by 2040, while the conventional meat supply could drop more than 33%. The Good Food Institute (GFI), a non-profit organization focused on alternative proteins, estimates that the global cultivated meat market could reach $25 billion by 2030. It is important to note that these Figures and projections are subject to change as the market evolves, and various factors can influence the actual market size, including regulatory developments, consumer acceptance, production scalability, and R&D investment. Experts envision that this invisible market could become a reality if price parity and consumer acceptance can be overcome.
The price of a product strongly affects the willingness of customers to buy it and, in turn, consumers' faith in a business enterprise. The cost of cultured meat is expected to decrease over time as the industry progresses and technology advances. Several factors contribute to cost reduction in the production of cultured meat.
• Scale-up and economies of scale: As production facilities scale up their operations, they can take advantage of economies of scale, which can lead to cost savings. Larger production volumes can help spread out fixed costs, such as facility expenses and equipment investments, over a greater number of products.
• Process optimization: As companies gain more experience and knowledge in the field of cultured meat production, they can refine and optimize their processes. This includes improving cell culture techniques, developing more efficient media formulations, and streamlining production workflows. Process optimization can lead to higher yields, reduced production time, and decreased resource consumption, ultimately lowering costs.
• Technological innovations: Advances in biotechnology, TE, and automation can contribute to cost reduction. New technologies and innovations can streamline and automate certain aspects of cultured meat production, reducing labor costs and improving overall efficiency.
• Alternative culture media: Culture media, which provide the necessary nutrients for cell growth, can be a significant expense in cultured meat production. Companies are actively researching and developing cost-effective and sustainable alternatives to traditional culture media, such as plant-based or recombinant protein-based media. Switching to more affordable and readily available media components can help reduce production costs.
• Biomass production and sourcing: The cost of acquiring and maintaining a sustainable and reliable cell source is an important consideration. Companies are exploring various approaches, such as developing cell lines with high growth rates and low production costs or using alternative cell sources to optimize biomass production and sourcing, thereby impacting overall costs.
To reduce the unit production cost of cultured meat, it is essential to develop large-scale production technologies utilizing bioreactors or microcarriers. Additionally, automation of production processes is necessary to lower labor costs and maximize operational efficiency (Pajčin et al., 2022).
While regulatory milestones, such as approvals by the SFA, USDA, and FDA, mark significant progress, resistance remains. Shortly after the U.S. approvals in 2023, seven states introduced legislation banning cultured meat, citing ethical and economic concerns. Similarly, Italy and France have proposed bills prohibiting the production and sale of lab-grown meat. These contrasting regulatory stances highlight the complex socio-political and cultural challenges facing cultured meat.
Additionally, discussions are ongoing regarding the safety of cultured meat products and their production facilities, which could significantly impact the development and commercialization of the cultured meat industry. For cell-based seafood, current efforts focus on high-value species such as fish maw. Despite cost reductions, production costs remain significantly higher than those of traditional seafood products, limiting commercialization potential (Rubio et al., 2019; Stephens et al., 2018).
While the field of cell-based meat holds great promise, several hurdles need to be overcome for successful commercialization.
• Scaling up production to meet consumer demand is a significant challenge. Currently, the production of cell-based meat is carried out in small-scale laboratory settings. Developing large-scale bioreactor systems and optimizing cell culture techniques for mass production are critical for commercial viability.
• Cell-based meat is a novel food product that falls under the jurisdiction of regulatory bodies responsible for food safety and labeling. Establishing clear and appropriate regulations and obtaining regulatory approval can be a complex process, requiring extensive safety assessments and compliance with existing food standards.
• The success of cell-based meat depends on consumer acceptance and willingness to embrace this novel food source. Primary factors influencing consumer acceptance of cultured meat include concerns about safety, taste, and familiarity. For instance, consumers unfamiliar with cultured meat report a rejection rate of 60%, while even those familiar with it show a rejection rate of 36% (Hadi and Brightwell, 2021).
Current cultured meat technology struggles to mimic thick cell layers and tissue structures. Moreover, the absence of co-culture systems for multiple cell types that compose meat makes it challenging to replicate the texture of real meat (Fraeye et al., 2020).
Consumers view FBS usage, which is essential for cultured meat production, as unsafe due to concerns about viral or prion infection risks and potential genetic modifications in recombinant proteins used as FBS alternatives (Hadi and Brightwell, 2021).
Addressing concerns related to taste, texture, safety, and perceived naturalness of the product is crucial for widespread adoption. Researchers, entrepreneurs, and regulatory bodies are actively working to address these hurdles and advance the commercialization of cell-based meat. Collaboration between industry stakeholders, technological advancements, and ongoing R&D efforts will play a crucial role in overcoming these challenges and bringing cell-based meat to the market.
In conclusion, the production of clean fish through TE techniques offers a promising solution to address the challenges of overfishing, pollution, and the increasing global demand for food, in particular, protein. The concept of clean fish involves culturing fish cells in controlled environments, free from pollutants and contaminants, to produce edible fish without health risk factors. Producing clean fish will provide sustainable and nutritional diets while contributing to the recovery of the marine ecosystem.
Despite its promise, the journey toward the commercialization of clean fish faces significant hurdles. These include the need to scale up production technology to achieve cost-effective mass production, develop sustainable and affordable alternatives to FBS, and design scaffolds that replicate the texture and structure of conventional fish. Additionally, the absence of regulatory approval and the psychological and cultural barriers to consumer acceptance remain critical challenges. Addressing concerns about taste, safety, and the perceived naturalness of lab-grown fish is vital for widespread adoption.
Technological advancements such as 3D bioprinting offer a promising pathway for overcoming some of these obstacles, enabling the creation of complex tissue structures that mimic natural fish. However, achieving price parity with conventional fish will require continuous process optimization, Cost reductions, and innovative approaches to production.
Further research and collaboration across academia, industry, and regulatory bodies are essential to address these challenges. By advancing scaling technologies, reducing production costs, and improving consumer perceptions, clean fish has the potential to transform the future of sustainable seafood and contribute to global food security. While the road ahead is complex, the promise of clean fish makes it a pursuit worth advancing.
DK: Funding acquisition, Visualization, Writing – original draft. J-HC: Writing – original draft. Y-GP: Writing – review & editing. HC: Writing – original draft. S-HM: Conceptualization, Funding acquisition, Writing – review & editing. GY: Conceptualization, Visualization, Writing – review & editing.
The author(s) declare financial support was received for the research, authorship, and/or publication of this article. This work was supported by the Korea Institute of Marine Science and Technology Promotion (KIMST) funded by the Ministry of Oceans and Fisheries, Korea (RS-2023-00235057) and National Research Foundation of Korea (NRF) funded by the Korea government (MSIT) (No. 2022R1A2C1006622), and Chung-Ang University Graduate Research Scholarship (Academic scholarship for College of Biotechnology and Natural Resources) in 2023.
DK was employed by Baobab Healthcare Inc.
The remaining authors declare that the research was conducted in the absence of any commercial or financial relationships that could be construed as a potential conflict of interest.
The author(s) declare that no Gen AI was used in the creation of this manuscript.
All claims expressed in this article are solely those of the authors and do not necessarily represent those of their affiliated organizations, or those of the publisher, the editors and the reviewers. Any product that may be evaluated in this article, or claim that may be made by its manufacturer, is not guaranteed or endorsed by the publisher.
Alshammari, E., Patel, M., Sachidanandan, M., Kumar, P., and Adnan, M. (2019). Potential evaluation and health fostering intrinsic traits of novel probiotic strain Enterococcus durans F3 isolated from the gut of fresh water fish Catla catla. Food Sci. Anim. Resour. 39:844. doi: 10.5851/kosfa.2019.e57
Amirvaresi, A., and Ovissipour, R. (2024). Assessment of plant-and microbial-derived protein hydrolysates as sustainable for fetal bovine serum in seafood cell culture media. Fut. Foods 10:100443. doi: 10.1016/j.fufo.2024.100443
Arora, S., Bhaukhandi, K. D., and Mishra, P. K. (2020). Coronavirus lockdown helped the environment to bounce back. Sci. Total Environ. 742:140573. doi: 10.1016/j.scitotenv.2020.140573
Balami, S., Sharma, A., and Karn, R. (2019). Significance of nutritional value of fish for human health. Malays. J. Halal Res. 2, 32–34. doi: 10.2478/mjhr-2019-0012
Ben-Arye, T., Shandalov, Y., Ben-Shaul, S., Landau, S., Zagury, Y., Ianovici, I., et al. (2020). Textured soy protein scaffolds enable the generation of three-dimensional bovine skeletal muscle tissue for cell-based meat. Nat. Food 1, 210–220. doi: 10.1038/s43016-020-0046-5
Bricard, Y., Ralliere, C., Lebret, V., Lefevre, F., and Rescan, P.-Y. (2014). Early fish myoseptal cells: insights from the trout and relationships with amniote axial tenocytes. PLoS ONE 9:e91876. doi: 10.1371/journal.pone.0091876
Cabello, F. C. (2006). Heavy use of prophylactic antibiotics in aquaculture: a growing problem for human and animal health and for the environment. Environ. Microbiol. 8, 1137–1144. doi: 10.1111/j.1462-2920.2006.01054.x
Chan, B., and Leong, K. (2008). Scaffolding in tissue engineering: general approaches and tissue-specific considerations. Eur. Spine J. 17, 467–479. doi: 10.1007/s00586-008-0745-3
Danopoulos, E., Jenner, L. C., Twiddy, M., and Rotchell, J. M. (2020). Microplastic contamination of seafood intended for human consumption: a systematic review and meta-analysis. Environ. Health Perspect. 128:126002. doi: 10.1289/EHP7171
de Almeida Rodrigues, P., Ferrari, R. G., Dos Santos, L. N., and Junior, C. A. C. (2019). Mercury in aquatic fauna contamination: a systematic review on its dynamics and potential health risks. J. Environ. Sci. 84, 205–218. doi: 10.1016/j.jes.2019.02.018
Dutta, S. D., Ganguly, K., Jeong, M.-S., Patel, D. K., Patil, T. V., Cho, S.-J., et al. (2022). Bioengineered lab-grown meat-like constructs through 3D bioprinting of antioxidative protein hydrolysates. ACS Appl. Mater. Inter. 14, 34513–34526. doi: 10.1021/acsami.2c10620
Eagle, H. (1955). Nutrition needs of mammalian cells in tissue culture. Science 122, 501–504. doi: 10.1126/science.122.3168.501
FAO (2011). The State of the World's Land and Water Resources for Food and Agriculture: Managing Systems at Risk. Rome: FAOand Earthscan from Routledge.
Farley, A., McLafferty, E., and Hendry, C. (2012). Cells, tissues, organs and systems. Nurs. Stand. 26:40. doi: 10.7748/ns.26.52.40.s46
Fraeye, I., Kratka, M., Vandenburgh, H., and Thorrez, L. (2020). Sensorial and nutritional aspects of cultured meat in comparison to traditional meat: much to be inferred. Front. Nutr. 7:35. doi: 10.3389/fnut.2020.00035
Frontera, W. R., and Ochala, J. (2015). Skeletal muscle: a brief review of structure and function. Calcif. Tissue Int. 96, 183–195. doi: 10.1007/s00223-014-9915-y
Gao, Y., Zhou, H., Gao, Z., Jiang, H., Wang, X., Mai, K., et al. (2019). Establishment and characterization of a fibroblast-like cell line from the muscle of turbot (Scophthalmus maximus L.). Fish Physiol. Biochem. 45, 1129–1139. doi: 10.1007/s10695-019-00628-3
Garrison, G. L., Biermacher, J. T., and Brorsen, B. W. (2022). How much will large-scale production of cell-cultured meat cost? J. Agric. Food Res. 10:100358. doi: 10.1016/j.jafr.2022.100358
Gemballa, S., and Vogel, F. (2002). Spatial arrangement of white muscle fibers and myoseptal tendons in fishes. Comp. Biochem. Physiol. Part A 133, 1013–1037. doi: 10.1016/S1095-6433(02)00186-1
Gibson, R. S., and Ferguson, E. L. (1998). Nutrition intervention strategies to combat zinc deficiency in developing countries. Nutr. Res. Rev. 11, 115–131. doi: 10.1079/NRR19980008
Gorissen, S. H., and Witard, O. C. (2018). Characterising the muscle anabolic potential of dairy, meat and plant-based protein sources in older adults. Proc. Nutr. Soc. 77, 20–31. doi: 10.1017/S002966511700194X
Green, A. L., Fernandes, L., Almany, G., Abesamis, R., McLeod, E., Aliño, P. M., et al. (2014). Designing marine reserves for fisheries management, biodiversity conservation, and climate change adaptation. Coast. Manag. 42, 143–159. doi: 10.1080/08920753.2014.877763
Gstraunthaler, G. (2003). Alternatives to the use of fetal bovine serum: serum-free cell culture. ALTEX Altern. Anim. Exp. 20, 275–281. doi: 10.14573/altex.2003.4.257
Hadi, J., and Brightwell, G. (2021). Safety of alternative proteins: technological, environmental and regulatory aspects of cultured meat, plant-based meat, insect protein and single-cell protein. Foods 10:1226. doi: 10.3390/foods10061226
Hatae, K., Yoshimatsu, F., and Matsumoto, J. J. (1984). Discriminative characterization of different texture profiles of various cooked fish muscles. J. Food Sci. 49, 721–726. doi: 10.1111/j.1365-2621.1984.tb13195.x
Hatae, K., Yoshimatsu, F., and Matsumoto, J. J. (1990). Role of muscle fibers in contributing firmness of cooked fish. J. Food Sci. 55, 693–696. doi: 10.1111/j.1365-2621.1990.tb05208.x
Hicks, C. C., Cohen, P. J., Graham, N. A., Nash, K. L., Allison, E. H., D'Lima, C., et al. (2019). Harnessing global fisheries to tackle micronutrient deficiencies. Nature 574, 95–98. doi: 10.1038/s41586-019-1592-6
Hubalek, S., Post, M. J., and Moutsatsou, P. (2022). Towards resource-efficient and cost-efficient cultured meat. Curr. Opin. Food Sci. 47:100885. doi: 10.1016/j.cofs.2022.100885
Ianovici, I., Zagury, Y., Redenski, I., Lavon, N., and Levenberg, S. (2022). 3D-printable plant protein-enriched scaffolds for cultivated meat development. Biomaterials 284:121487. doi: 10.1016/j.biomaterials.2022.121487
Jeong, D., Seo, J. W., Lee, H. G., Jung, W. K., Park, Y. H., Bae, H., et al. (2022). Efficient myogenic/adipogenic transdifferentiation of bovine fibroblasts in a 3D bioprinting system for steak-type cultured meat production. Adv. Sci. 9:2202877. doi: 10.1002/advs.202202877
Jochems, C. E., Van Der Valk, J. B., Stafleu, F. R., and Baumans, V. (2002). The use of fetal bovine serum: ethical or scientific problem? Altern. Lab. Anim. 30, 219–227. doi: 10.1177/026119290203000208
Jones, N. (2023). Lab-grown meat: the science of turning cells into steaks and nuggets. Nature 619, 22–24. doi: 10.1038/d41586-023-02095-6
Kang, D.-H., Louis, F., Liu, H., Shimoda, H., Nishiyama, Y., Nozawa, H., et al. (2021). Engineered whole cut meat-like tissue by the assembly of cell fibers using tendon-gel integrated bioprinting. Nat. Commun. 12:5059. doi: 10.1038/s41467-021-25236-9
Kawarazuka, N., and Béné, C. (2010). Linking small-scale fisheries and aquaculture to household nutritional security: an overview. Food Sec. 2, 343–357. doi: 10.1007/s12571-010-0079-y
Kubota, M., Kinoshita, M., Kubota, S., Yamashita, M., Toyohara, H., Sakaguchi, M., et al. (2001). Possible implication of metalloproteinases in postmortem tenderization of fish muscle. Fish. Sci. 67, 965–968. doi: 10.1046/j.1444-2906.2001.00347.x
Kumar, A., Singh, N., Goswami, M., Srivastava, J., Mishra, A. K., Lakra, W., et al. (2016). Establishment and characterization of a new muscle cell line of Zebrafish (Danio rerio) as an in vitro model for gene expression studies. Anim. Biotechnol. 27, 166–173. doi: 10.1080/10495398.2016.1147455
Li, D., and Hu, X. (2009). Fish and its multiple human health effects in times of threat to sustainability and affordability: are there alternatives? Asia Pac. J. Clin. Nutr. 18, 553–563. Available at: https://search.informit.org/doi/10.3316/ielapa.713463137280633
Listrat, A., Lebret, B., Louveau, I., Astruc, T., Bonnet, M., Lefaucheur, L., et al. (2016). How muscle structure and composition influence meat and flesh quality. Sci. World J. 2016:3182746. doi: 10.1155/2016/3182746
Liu, Y., Wang, R., Ding, S., Deng, L., Zhang, Y., Li, J., et al. (2022). Engineered meatballs via scalable skeletal muscle cell expansion and modular micro-tissue assembly using porous gelatin micro-carriers. Biomaterials 287:121615. doi: 10.1016/j.biomaterials.2022.121615
Maja, M. M., and Ayano, S. F. (2021). The impact of population growth on natural resources and farmers' capacity to adapt to climate change in low-income countries. Earth Syst. Environ. 5, 271–283. doi: 10.1007/s41748-021-00209-6
Manenti, R., Mori, E., Di Canio, V., Mercurio, S., Picone, M., Caffi, M., et al. (2020). The good, the bad and the ugly of COVID-19 lockdown effects on wildlife conservation: Insights from the first European locked down country. Biol. Conserv. 249:108728. doi: 10.1016/j.biocon.2020.108728
Marques, A., Nunes, M. L., Moore, S. K., and Strom, M. S. (2010). Climate change and seafood safety: human health implications. Food Res. Int. 43, 1766–1779. doi: 10.1016/j.foodres.2010.02.010
Matschak, T., and Stickland, N. (1995). The growth of Atlantic salmon (Salmo salar L.) myosatellite cells in culture at two different temperatures. Experientia 51, 260–266. doi: 10.1007/BF01931109
Merino, G., Barange, M., Blanchard, J. L., Harle, J., Holmes, R., Allen, I., et al. (2012). Can marine fisheries and aquaculture meet fish demand from a growing human population in a changing climate? Glob. Environ. Change 22, 795–806. doi: 10.1016/j.gloenvcha.2012.03.003
Montserrat, N., Sánchez-Gurmaches, J., García De La Serrana, D., Navarro, M., and Gutiérrez, J. (2007). IGF-I binding and receptor signal transduction in primary cell culture of muscle cells of gilthead sea bream: changes throughout in vitro development. Cell Tissue Res. 330, 503–513. doi: 10.1007/s00441-007-0507-2
Mulvaney, D. R., and Cyrino, J. E. (1995). Establishment of channel catfish satellite cell cultures. Basic Appl. Myol. 5, 65–70.
Neufingerl, N., and Eilander, A. (2021). Nutrient intake and status in adults consuming plant-based diets compared to meat-eaters: a systematic review. Nutrients 14:29. doi: 10.3390/nu14010029
Pajčin, I., KneŽić, T., Savic Azoulay, I., Vlajkov, V., Djisalov, M., Janjušević, L., et al. (2022). Bioengineering outlook on cultivated meat production. Micromachines 13:402. doi: 10.3390/mi13030402
Pal, J., Shukla, B., Maurya, A. K., Verma, H. O., Pandey, G., Amitha, A., et al. (2018). A review on role of fish in human nutrition with special emphasis to essential fatty acid. Int. J. Fish. Aquat. Stud. 6, 427–430.
Patel, M., Ashraf, M. S., Siddiqui, A. J., Ashraf, S. A., Sachidanandan, M., Snoussi, M., et al. (2020). Profiling and role of bioactive molecules from puntius sophore (freshwater/brackish fish) skin mucus with its potent antibacterial, antiadhesion, and antibiofilm activities. Biomolecules 10:920. doi: 10.3390/biom10060920
Peng, L.-M., Zheng, Y., You, F., Wu, Z.-H., Tan, X., Jiao, S., et al. (2016). Comparison of growth characteristics between skeletal muscle satellite cell lines from diploid and triploid olive flounder Paralichthys olivaceus. PeerJ 4:e1519. doi: 10.7717/peerj.1519
Rayment, I., Holden, H. M., Whittaker, M., Yohn, C. B., Lorenz, M., Holmes, K. C., et al. (1993). Structure of the actin-myosin complex and its implications for muscle contraction. Science 261, 58–65. doi: 10.1126/science.8316858
Rubio, N., Datar, I., Stachura, D., Kaplan, D., and Krueger, K. (2019). Cell-based fish: a novel approach to seafood production and an opportunity for cellular agriculture. Front. Sustain. Food Syst. 3:435832. doi: 10.3389/fsufs.2019.00043
Rubio, N. R., Xiang, N., and Kaplan, D. L. (2020). Plant-based and cell-based approaches to meat production. Nat. Commun. 11, 1–11. doi: 10.1038/s41467-020-20061-y
Rupani, P. F., Nilashi, M., Abumalloh, R., Asadi, S., Samad, S., Wang, S., et al. (2020). Coronavirus pandemic (COVID-19) and its natural environmental impacts. Int. J. Environ. Sci. Technol. 17, 4655–4666. doi: 10.1007/s13762-020-02910-x
Sato, K., Ando, M., Kubota, S., Origasa, K., Kawase, H., Toyohara, H., et al. (1997). Involvement of type V collagen in softening of fish muscle during short-term chilled storage. J. Agric. Food Chem. 45, 343–348. doi: 10.1021/jf9606619
Schätzlein, E., and Blaeser, A. (2022). Recent trends in bioartificial muscle engineering and their applications in cultured meat, biorobotic systems and biohybrid implants. Commun. Biol. 5:737. doi: 10.1038/s42003-022-03593-5
Seiliez, I., Sabin, N., and Gabillard, J.-C. (2012). Myostatin inhibits proliferation but not differentiation of trout myoblasts. Mol. Cell. Endocrinol. 351, 220–226. doi: 10.1016/j.mce.2011.12.011
Shurtleff, W., and Aoyagi, A. (2013). History of Tofu and Tofu Products (965 CE to 2013). Soyinfo Center. Available at: https://books.google.co.kr/books?hl=ko&lr=&id=gGrUNvZt0_YC&oi=fnd&pg=PA1&ots=ILhfNRA8H5&sig=ZYdDHl-hKamckOm8f5j3Im5FW9c#v=onepage&q&f=false
Stephens, N., Di Silvio, L., Dunsford, L., Ellis, I., Glencross, M., and Sexton, A. (2018). cultured meat to market: technical, socio-political, and regulatory challenges in cellular agriculture. Trends Food Sci. Technol. 78, 155–166. doi: 10.1016/j.tifs.2018.04.010
Stout, A. J., Rittenberg, M. L., Shub, M., Saad, M. K., Mirliani, A. B., Dolgin, J., et al. (2023). A Beefy-R culture medium: replacing albumin with rapeseed protein isolates. Biomaterials 296:122092. doi: 10.1016/j.biomaterials.2023.122092
Tanaka, R.-i., Sakaguchi, K., Yoshida, A., Takahashi, H., Haraguchi, Y., and Shimizu, T. (2022). Production of scaffold-free cell-based meat using cell sheet technology. npj Sci. Food 6:41. doi: 10.1038/s41538-022-00155-1
van der Valk, J. (2022). Fetal bovine serum—A cell culture dilemma. Science 375, 143–144. doi: 10.1126/science.abm1317
Van der Valk, J., Mellor, D., Brands, R., Fischer, R., Gruber, F., Gstraunthaler, G., et al. (2004). The humane collection of fetal bovine serum and possibilities for serum-free cell and tissue culture. Toxicol. In Vitro 18, 1–12. doi: 10.1016/j.tiv.2003.08.009
Van Leeuwen, J. L. A. (1999). mechanical analysis of myomere shape in fish. J. Exp. Biol. 202, 3405–3414. doi: 10.1242/jeb.202.23.3405
Waltz, E. (2021a). Club-goers take first bites of lab-made chicken. Nat. Biotechnol. 39, 257–259. doi: 10.1038/s41587-021-00855-1
Waltz, E. (2021b). No bones, no scales, no eyeballs: appetite grows for cell-based seafood. Nat. Biotechnol. 39, 1483–1485. doi: 10.1038/d41587-021-00022-6
Wang, L., Cao, Z., Liu, Y., Xiang, Y., Sun, Y., Zhou, Y., et al. (2020). Establishment and characterization of a new cell line from the muscle of humpback grouper (Cromileptes altivelis). Fish Physiol. Biochem. 46, 1897–1907. doi: 10.1007/s10695-020-00841-5
Weber, T., and Wagner, K. (2021). Replacing fetal bovine serum (FBS) in research and testing. ALTEX Altern. Anim. Exp. 38, 163–164. doi: 10.14573/altex.2012141
Worm, B., Barbier, E. B., Beaumont, N., Duffy, J. E., Folke, C., Halpern, B. S., et al. (2006). Impacts of biodiversity loss on ocean ecosystem services. Science 314, 787–790. doi: 10.1126/science.1132294
Xu, W., Yuan, J., Gong, W., Xie, G., Ma, Y., Zhang, J., et al. (2020). Cell culture media chronicle. Biomed. Pharmacol. J. 13, 551–554. doi: 10.13005/bpj/1917
Yang, L., Waples, R. S., and Baskett, M. L. (2019). Life history and temporal variability of escape events interactively determine the fitness consequences of aquaculture escapees on wild populations. Theor. Popul. Biol. 129, 93–102. doi: 10.1016/j.tpb.2018.12.006
Keywords: clean fish, cell-based fish, protein, commercialization, sustainable food
Citation: Kang D, Choi J-H, Park Y-G, Choi HK, Moon S-H and Yang GH (2025) Not just fish, but the future. Front. Sustain. Food Syst. 8:1506573. doi: 10.3389/fsufs.2024.1506573
Received: 05 October 2024; Accepted: 23 December 2024;
Published: 08 January 2025.
Edited by:
Swati Sharma, Integral University, IndiaReviewed by:
José Lino Vieira De Oliveira Costa, University of Lisbon, PortugalCopyright © 2025 Kang, Choi, Park, Choi, Moon and Yang. This is an open-access article distributed under the terms of the Creative Commons Attribution License (CC BY). The use, distribution or reproduction in other forums is permitted, provided the original author(s) and the copyright owner(s) are credited and that the original publication in this journal is cited, in accordance with accepted academic practice. No use, distribution or reproduction is permitted which does not comply with these terms.
*Correspondence: Sung-Hwan Moon, bW9vbnNoQGNhdS5hYy5rcg==; Gi Hoon Yang, dG9ueUB0b2ZmbW9iaWxpdHkuY29t
†These authors have contributed equally to this work
Disclaimer: All claims expressed in this article are solely those of the authors and do not necessarily represent those of their affiliated organizations, or those of the publisher, the editors and the reviewers. Any product that may be evaluated in this article or claim that may be made by its manufacturer is not guaranteed or endorsed by the publisher.
Research integrity at Frontiers
Learn more about the work of our research integrity team to safeguard the quality of each article we publish.