- 1Natural Resources and Sustainable Development Laboratory, Faculty of Sciences, Ibn Tofail University, Kenitra, Morocco
- 2Faculty of Sciences, Arab American University, Ramallah, Palestine
- 3Center of Innovation and Technology Transfer, Moulay Ismail University, Meknes, Morocco
- 4Bioactives and Environmental Health Laboratory, Faculty of Sciences, Moulay Ismail University, Meknes, Morocco
- 5Department of Pharmacognosy, College of Pharmacy, King Saud University, Riyadh, Saudi Arabia
- 6National Center for Research in Human Genomics, Evry Courcouronnes, France
Introduction: In the global field of nanotechnology, the production of silver nanoparticles (AgNPs) is an environmentally beneficial technique. To prevent the diseases caused by many commonly used pesticides, it is essential to synthesize safe alternatives from low-cost sources.
Material and methods: This study focuses on the eco-friendly extracellular production of AgNPs using an aqueous extract of Ulva lactuca as a reducing agent in a silver nitrate solution. The biosynthetic reaction was confirmed through various analytical techniques, including ultraviolet–visible (UV–Vis) spectroscopy, Fourier transform infrared spectroscopy (FT-IR), scanning electron microscope (SEM), and energy dispersive X-ray analysis (EDAX). Moreover, antioxidant activity was confirmed. It has also been evaluated against a variety of bacteria and fungi that have an impact on humans, animals, and plants.
Results and discussion: The mean size of the nanoparticles (NPs) varied from 45–61 nm. The disk diffusion testing results show that, in comparison to the tested antibiotics, Staphylococcus aureus, Escherichia coli, Staphylococcus epidermidis, and Klebsiella pneumoniae were more responsive to U. lactuca-silver nanoparticles (U-AgNPs) extract. eldor 50% sc fungicide was used at the prescribed dose of 0.5 μL/mL, and the antifungal activity of aqueous and U-AgNPs extracts was examined at three concentrations of 0.62, 1.25, and 2.5 mg/mL. Through the suppression of spore germination, in vitro investigations demonstrated that U-AgNPs at varying doses greatly hindered the process of reproductive growth of Botrytis cinerea. Comparing U-AgNPs to the standard synthetic fungicide Teldor 50% sc, it is noteworthy to observe that a substantial control efficacy against tomato and strawberry gray mold was noticed, especially at concentrations of 2.5mg/mL on the entire tomato and strawberry plants and also on the detached leaves and fruits without causing any appearance damage. In summary, this study advances the field of agronanotechnology by demonstrating the potential application of a fungicide based on NPs to prevent gray mold on tomato and strawberry plants in greenhouse environments and throughout the postharvest phase.
Introduction
Natural or artificial substances known as pesticides prevent, manage, and eliminate weeds, insects, and other pests that interfere with plant growth. These substances are categorized based on their chemical composition, application, dangers, and mechanism of action (Ahamad and Kumar, 2023; Khan et al., 2023). Global pesticide use has increased dramatically and consistently since the mid-1940s, mostly as a result of commercial farming (Singh et al., 2020; Elbialy et al., 2021; Trellu et al., 2021; Umapathi et al., 2022). Pesticide use has gone out of control, contaminating food and causing damage to the environment, agriculture, and waterways (Cech et al., 2023). Pesticide residues can be found in soil, water, air, fruits, vegetables, and processed foods. Agricultural pesticide exposure and its acute and long-term health consequences on the diet are major public health concerns, particularly in developing nations. Chemical pesticides can potentially be cytotoxic, mutagenic, and carcinogenic to humans (Fang et al., 2020). Due to their non-species-specific mechanism of action, pesticides often kill or damage organisms other than pests, including people. A WHO and United Nations Environment Programme (UNEP) research states that 3 million people are poisoned and 200,000 people die globally as a result of pesticide exposure, with poorer nations accounting for the majority of these deaths (Boedeker et al., 2020).
As the agricultural sector seeks to balance productivity with environmental and health considerations, it is essential to address the challenges associated with current pesticide utilization; the need for innovative, environmentally safe antipathogenic, antifoulant, and anticorrosion materials is constantly growing. Learning from the improvements that natural goods offer after lengthy periods of evolutionary growth is an appealing option in producing such materials (Krishnan et al., 2018).
Seaweeds can be grown sustainably and have a high growth rate, allowing for the quick production of biomass. Because of its scalability, seaweed-based green technology is rapidly gaining traction as a sustainable solution across several industries. This trend draws attention to the ability of seaweeds to produce very efficient biosorbents, such as the ultrasonic seaweed-based biosorbent (Kumar et al., 2022), as well as biopesticides, an environmentally benign substitute for traditional chemical pesticides (Pérez-de-Luque, 2020).
Nanotechnology in agriculture has concentrated on several nanoparticle (NP) types, each with unique and intriguing characteristics, such as metal NPs, such as gold and silver, which are well-known for having antibacterial properties (Singh et al., 2020). Since nanotechnology has produced nanoagrochemicals to increase global food production, it has become a bio-stimulant for smart agriculture (Hanif et al., 2024; Waqif et al., 2024). Compared to their conventional counterparts, these nanoagrochemicals have demonstrated a 20–30% boost in production; nevertheless, their effects on environmental sustainability differ (Kah et al., 2018).
Researchers’ interest in the marine algae-mediated production of AgNPs is piqued by these algae’s year-round availability and ease of harvesting. Researchers have demonstrated that producing AgNPs from algae is economical and environmentally benign because it does not involve hazardous chemicals (Abdel-Raouf et al., 2018; Choudhary et al., 2023). In this work, we have created AgNPs by reducing aqueous AgNO3 using U. lactuca marine algae extract.
To decrease the pollution associated with older pesticides and to highlight the development of new, safe, and effective synthetic pesticides, we highlight the potential applications of nanotechnology in agriculture. This technology could produce safe pesticides that do not harm the environment or human health.
Materials and methods
Sample collection and preparation
Fresh seaweed was gathered by hand from the Mehdia coast (latitude: 9,440 N and longitude: 79,000 E), cleaned thoroughly in seawater to remove external matter such as sand, epiphytes, and other algae contaminants; and then placed in polyethylene plastic bags and transported to the laboratory promptly to minimize deterioration (Muthukumar et al., 2015). In the laboratory, the samples were rewashed three times with tap water and one more time with distilled water. The samples were dried at 40 ± 2°C for 78 h, and then kept in dark bottles at 4°C, after being mechanically blended into a fine powder for future analysis and applications.
Aqueous extract of Ulva lactuca
A measure of 100 mL of distilled water and 5 g of seaweed were mixed, stirred for 1 h at 6,000 rpm, and permitted to sit at ambient temperature for 24 h. Following filtration, the extract was kept in the refrigerator in dark bottles for later use (Amin, 2019).
Silver nanoparticle biosynthesis using Ulva lactuca
A measure of 10 mL of U. lactuca’s aqueous extract and 1 mM of silver nitrate (AgNO3) were combined, stirred, and heated to 100°C for 24 h. When the reaction’s color shifts from translucent to brown, the reaction is deemed successful (Bhimba and Kumari, 2014).
UV–vis spectroscopic characterization of synthesized nanoparticles
The effectiveness of the bioreduction of AgNO3 by U. lactuca’s aqueous extract into AgNPs was demonstrated using UV–Vis (Schimatzu UV-1800). At intervals of 100 nm, the absorbance spectrum was measured over a wavelength range of 200–1000 nm.
Fourier-transform infrared spectroscopy
The Bruker FT-IR instrument was utilized to analyze the sample-synthesized NPs to ascertain the functional groups of the AgNPs. The RockSolid permanently aligned interferometer and the DigiTect integrated detection system, with a 24-bit analog-to-digital converter (ADC), was utilized at the University Center of Analysis, Expertise, Technology Transfer and Incubator within the Faculty of Science at Ibn Tofail University, Kenitra, Morocco. The spectral analysis was focused on the range of 400–4,000 cm−1.
X-ray diffraction analysis
The crystallographic data of the dried silver nitrate solution were obtained after bioreduction. The X’Pert3 materials research diffractometer was used to record the XRD patterns. The samples were scanned using a 2θ range of 10°–70°.
Scanning electron microscope
The SEM analysis was carried out on an SEM JEOL. The Center for Innovation and technological by technology: Center of Innovation and Technology Transfer of Moulay Ismail University, CITT -UMI in Meknes City, Morocco established the size and shape of the AgNPs.
Antioxidant activity of AgNPs
DPPH activity
The method suggested by Aouji et al. (2023) was adjusted to assess the seaweed extracts’ ability to scavenge free radicals: 2.5 mL of a methanol solution containing 0.2-mM 2,2-diphenyl-1-picrylhydrazyl (DPPH) was combined with a 0.5 mL every extract (at doses of 15.62 to 1,000 μg/mL); the combination was then incubated for 30 min at ambient temperature sheltered from the light. At 517 nm, absorbance was then noted. The DPPH solution served as a control and ascorbic acid as a standard to determine the extent to which samples and the reference standard had scavenged radicals. Using Equation 1, the fraction of DPPH scavenging was determined.
In this equation, DOsample represents the absorbance of the test sample (the sample mixed with the DPPH solution), while DO control represents the absorbance of the control (DPPH without sample). The inhibitory concentration at which DPPH radicals were 50% scavenged was measured as the half-maximal inhibitory concentration (IC50) value (μg/mL).
Ferric reducing antioxidant power
The reducing power of the extracts was ascertained using the Oyaizu method (Oyaizu, 1986). Potassium ferricyanide (1%, w/v) was incorporated into 2.5 mL of phosphate buffer (0.2 M, pH 6.6) after the extracted sample (1 mL) had been combined with it. For 20 min, the combined solution was incubated at 50°C. Then, 2.5 mL of 10% w/v trichloroacetic acid was incorporated, and the mixture was centrifuged at 3000 rpm for 10 min. A measure of 2.5 mL of the supernatant was combined with 0.5 mL of ferric chloride (0.1%, w/v) and 2.5 mL of distilled water. After 10 min of incubation, the absorbance was determined at 700 nm. The reaction mixture’s increased absorbance demonstrated a higher reducing power.
Antibacterial activity of aqueous and U-AgNP extracts
Agar diffusion method
Staphylococcus aureus, Escherichia coli, Staphylococcus epidermidis, Enterobacter cloacae, Acinetobacter baumannii, Klebsiella pneumoniae, and methicillin-resistant S. aureus were gathered from the Kenitra Center for Medical Analyses and kept in a Muller–Hinton medium to evaluate the activities of the produced NPs and aqueous extract. Antibacterial activity was assessed using the Muller–Hinton agar diffusion method (Oubihi et al., 2020). A microbial suspension with an optical density of 1 McFarland was spread over the surface of an agar medium in a Petri dish. Ulva extracts (30 mg/mL) were dissolved in 1 mL of dimethyl sulfoxide (DMSO). Whatman absorbent paper disks, measuring 6 mm in diameter, were autoclaved and then soaked in the extracts for testing. The disks were then placed on the seeded agar’s surface and incubated for 24 h at 37°C. DMSO’s inhibitory action was also examined. Each test was carried out in triplicates under sterile conditions. Ampicillin (10 μg/disk) and streptomycin (100 μg/disk) were utilized as an antibacterial positive control; however, DMSO was utilized as a negative control. Antibiotics, aqueous, and U-AgNP inhibition zones were assessed and represented in millimeters (zone of inhibition ± standard deviation [SD]). As an alternative to Agar-based procedures, agar-based techniques such as disk diffusion can be useful because they are quicker and easier to apply (Liu et al., 2016; Schumacher et al., 2018).
Determination of the minimum inhibitory concentration
The MIC is the lowest inhibitory concentration (MIC) at which no visible growth was seen. This experiment was performed in triplicate, employing the macrodilution method described by Tarfaoui et al. (2022) to determine the MICs. The extracts were dissolved in DMSO, a solvent with no known antibacterial properties (Rahmi and Putri, 2020). Serial dilutions of the extracts were prepared in agar solutions (2–100 μL/mL).
Antifungal activity of aqueous and U-AgNPs
In vitro antifungal activity
Using the agar plate methodology, AgNPs’ antifungal activity was assessed (Nwosu and Okafor, 1995), against Aspergillus versicolor, Alternaria alternata, Penicillium spp., Fusarium equiseti, and B. cinerea, isolated and identified by The Laboratory of Plant, Animal and Agro-industry Production, Department of Biology, Ibn Tofail University, Kenitra, Morocco.
Potato dextrose agar (PDA) medium with a volume of 95 mL was stored at 40°C after autoclaving. Weighted at 62, 125, and 250 mg, the dried aqueous and NP extracts were reconstituted with 5 mL of DMSO and then put into 95 mL of PDA medium. The final concentrations (0.62, 1.25, and 2.5 mg/mL) were then transferred to PDA Petri plates (12 mL/plate). The 7-day-old fungal cultures’ mycelium disks, which had a diameter of 5 mm, were moved to Petri plates. Then incubated for 7 days at 22 ± 2°C (Memmert Group, Model-High Precision Incubator), with daily observations of fungal development made (Onaran and Yilar, 2012). Teldor 50% sc was used as a standard antifungal. Utilizing DMSO (% 5) served as the negative control. The inhibitory zones were identified and noted after incubation. The inhibition rate was calculated using Equation 2:
where R denotes the radial growth of the fungal mycelium in control plates, and r refers to the fungal mycelium’s radial growth in aqueous and U-AgNP-treated plates (Bharose et al., 2024).
Antifungal activity of aqueous and U-AgNP extracts in vivo
The Solanum lycopersicum variety was chosen for the experiment. In a greenhouse, tomato seedlings were planted in 15 by 15 cm plastic pots filled with 1:1 black dirt and peat soil for the vegetable mix, under 16 h of photoperiod. When necessary, plants were watered (Yousef et al., 2021). In the Moulay Bouselham region, we purchased strawberry plants from a nearby nursery. Two months after planting, tomato plants were moved (among the strawberry plants) into a controlled environment with a temperature of 21°C and 70% humidity for acclimatization. To provide ideal circumstances for the inoculation of gray mold, the humidity was raised to 100%, and the plants were covered with agrofilm 24 h prior to the inoculation. The plants were treated by spraying the leaves with 1 mL of aqueous and U-AgNP extracts after 24 h. Each tomato and strawberry trifoliate had three wounds made on its center leaves. On each wound, 2 mm of B. cinerea mycelium was applied. The plants were once more wrapped with agrofilm and stored for 48 h at 21°C and 100% humidity. After 48 h, the agrofilm was removed. The plants were frequently watered, and the severity and emergence of infection signs were observed. Teldor 50% sc was used as a standard antifungal. Utilizing DMSO (% 5) served as the negative control. Each treatment has three duplicates or three plants. All evaluations were conducted 3, 6, and 8 days after inoculation.
Measuring leaves’ and fruits’ response to aqueous and U-AgNP extracts
The uniform and healthy fruits of tomatoes (harvested from the developing plants) and strawberries (picked from a local store), were sterilized with ethanol at 70° and dried using a sterile filter paper after being rinsed three times with sterile distilled water. At three distinct locations throughout the equatorial zone, 2-mm diameter needles were used to create 3-mm deep wounds. A 20-μL drop of each extract was then applied to the wounds (Hassan et al., 2021). A 2-mm diameter mycelial explant was also inserted into the incisions after 2 h. The same protocol was applied to tomato and strawberry leaves. After that, the fruits and leaves were coated with plastic covers and retained in a dark and humid environment.
Evaluating the severity of the infection
The scale of infection was assessed 8 days after treatment of the plants intentionally infected with B. cinerea mycelium. The percentage of affected leaf area on randomly chosen plants was used to determine the severity of the disease. It was estimated using a disease rating scale of 1–9, recommended by Notteghem et al. (1980). Table 1 presents the results.
Equation 3 was used to convert the severity scale into a Percentage Severity Index (SI) for analysis:
where Nt is the total number of plants noticed, Xi is the disease severity scale, ni is the number of damaged plants (leaves or fruits) with a rating of i, and 9 is the maximum disease severity scale.
Data analysis
The data were analyzed using a one-way analysis of variance (ANOVA) followed by Tukey’s test (α = 0.05) to determine significance levels. The results of three repetitions are presented by mean ± SD. A p < 0.05 was considered statistically significant.
Results and discussion
Characterization of U-AgNP extract
The reaction mixture’s color shifted from light green to dark brown, which was the first sign that AgNPs were being formed (Hashemabadi et al., 2021).
After being refined, AgNPs were tested using UV spectrophotometry. Figure 1 shows a distinct and unique characteristic peak at 440 nm, which corresponds to the plasmon absorbance of the AgNP. In contrast, the characteristic peak was registered at 401 nm for the aqueous extract.
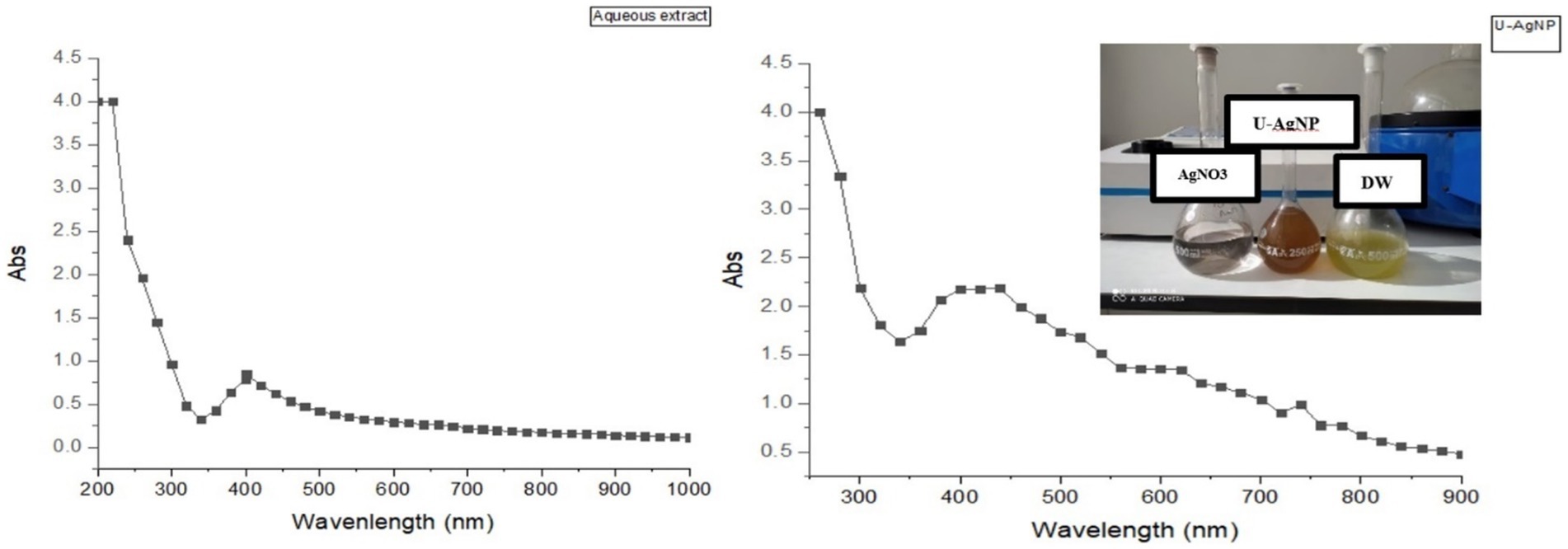
Figure 1. Ultraviolet–visible (UV–Vis) absorption spectrum of aqueous Distilled Water (DW) and U-AgNPs derived from Ulva lactuca.
The chemical composition of the AgNPs’ surface is examined using FT-IR spectroscopy, which also permits the identification of potential biomolecules that may be in charge of the Ag2+ ion reduction and capping of the bioreduced AgNPs produced by U. lactuca extract. The absorption bands were visible in the FT-IR spectrum at 465.35, 1082.33, 1408.34, 1536.401, 1631.31, 2348.97, 2916.90, and 3271.40 cm−1 (Figure 2).
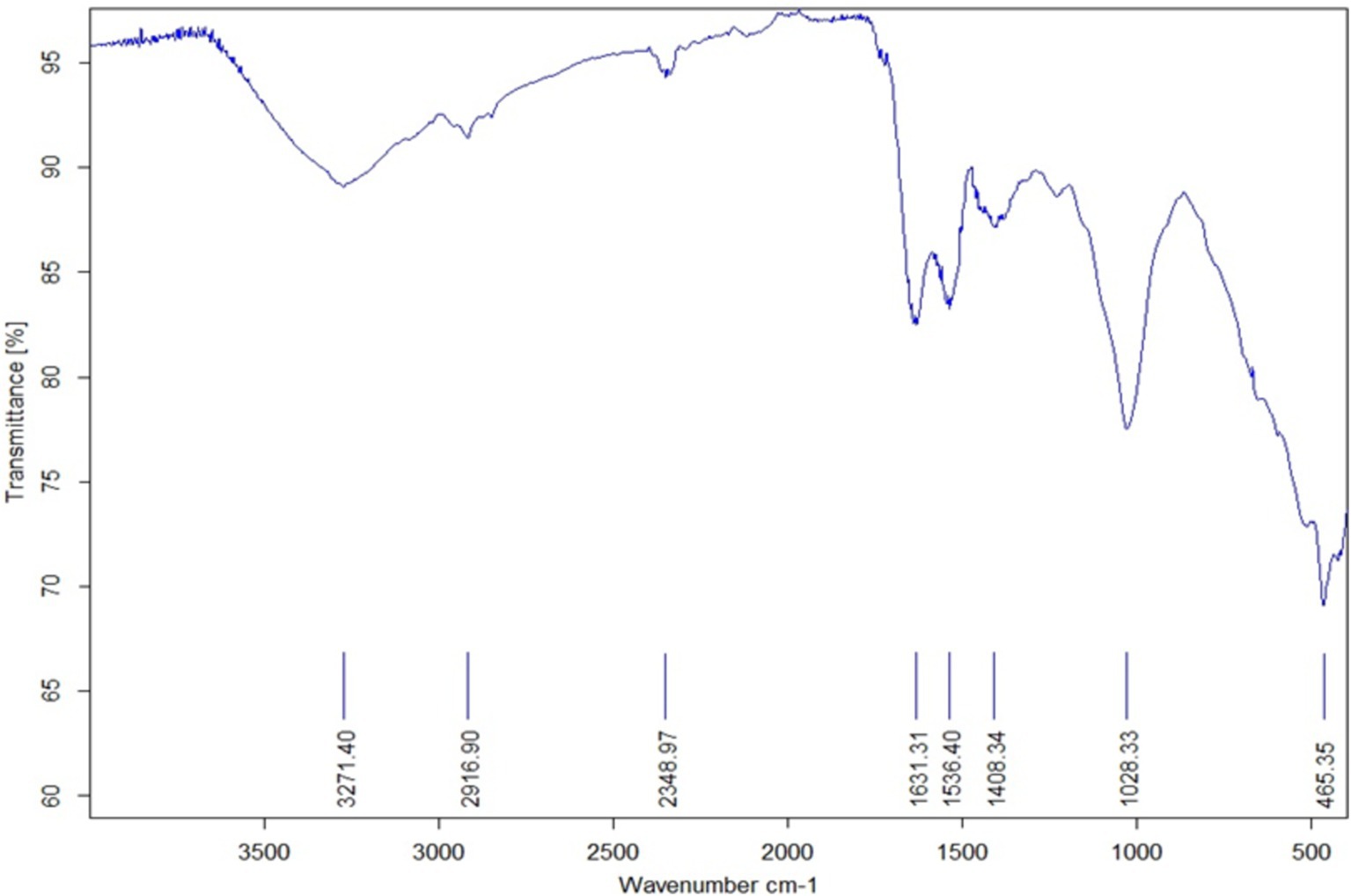
Figure 2. Fourier transform infrared spectroscopic (FT-IR) data of U-AgNPs derived from Ulva lactuca.
The peak at 465.35 cm−1 is attributed to the coupling of O–Si–O bending and K–O stretching vibrations (Theodosoglou et al., 2010). The peak at 1082 cm−1 is assigned to the C–N stretch of aliphatic amines, while the peak at 1408 cm−1 is associated with C–N stretching vibrations of aromatic and aliphatic amines. The peak at 1536 cm−1 is linked to the N–O asymmetric stretching of nitro compounds, and the peak at 1631 cm−1 is attributed to the protein amide I bond, resulting from a carbonyl stretch in proteins. This peak was relatively close to the native protein reported by Macdonald and Smith (1996), which implies that although proteins and AgNPs are conversing, their secondary structure is unaffected by the binding process or the subsequent Ag nanoparticle-protein interaction (Fayaz et al., 2010).
The study demonstrated that amino acid residues’ carbonyl groups have a great affinity for metal, which may lead to the creation of a layer that covers metal NPs and serves as a capping agent to prevent agglomeration and stabilize the medium (Al-Fa’ouri et al., 2021). These findings support the hypothesis that certain proteins function as stabilizing and reducing agents for AgNPs. The peak at 2348 cm−1 refers to the asymmetric vibration of C–H, while the peak at 2916 cm−1 is caused by the symmetric and asymmetric stretching of alkenes along the C–H bond. The peak at 3271 cm−1 is associated with the O–H stretching of water molecules, alcohols, or phenols, as well as the presence of N–H amides stretching vibration. Thus, it can be assumed that the plant extract’s saponins, tannins, glycosides, flavonoids, and polyphenols give the NPs their additional stability and are compatible with the findings of Khalifa et al. (2016). The existence of organic molecules such as alkenes, alkanes, primary amines, ester, and nitro groups is confirmed by FT-IR spectral analysis, which also demonstrates the significance of U. lactuca extract in the environmentally friendly synthesis of AgNPs.
The XRD examination indicates the formation of crystalline silver particles, wherein nanocrystals are created, as indicated by the peaks at 2θ values of 27.93°, 32.41°, 38.40°, 46.29°, 57.51°, and 78.6° corresponding to (110), (111), (122), (200), (220), and (311) crystal planes of the crystalline structure (Figure 3).
Green-produced AgNPs were examined for surface morphology using a scanning electron microscope. The SEM of AgNPs revealed a spherical shape with a size range of 45–61 nm, involving agglomeration caused by U. lactuca’s organics acting as stabilizing agents (Figure 4; Harinee et al., 2019).
Energy dispersive X-ray spectroscopy (EDAX) analysis was performed to verify the existence of AgNPs and offer the chemical purity of the produced NPs (Figure 5), revealing peaks between 0 and 4 kV. The optical absorption peak indicates that nanocrystalline elemental silver is occurring, which was noticed at approximately 3 keV, which is characteristic of the metallic silver nanocrystals’ absorption owing to surface plasma resonance. Strong silver signals and weak carbon and oxygen peaks are visible in the spectrum; these could result from biomolecules attached to the surface of the AgNPs. The presence of salt or protein residue in the marine environment may lead to the discovery of additional substances such as Si and Ca (Amin, 2019).
Evaluation of antioxidant activity
DPPH and FRAP assays
Naturally occurring antioxidants, such as phenolics, terpenoids, and alkaloids, play a highly important role in enhancing human health. They help prevent or treat a variety of diseases by minimizing genetic mutations, preventing cell damage, and detoxifying oxygen-free radicals (García-Sánchez et al., 2020).
The DPPH and FRAP tests were used in this investigation to assess the antioxidant activity of U-AgNPs and the aqueous extract of U. lactuca. The aqueous extract, U-AgNP, and ascorbic acid as the standard were used to measure the DPPH scavenging activity at various doses (15.62–1,000 μg/mL; Figure 6). The color change was employed to ascertain the DPPH scavenging activity, which increased dose-dependently. We noticed that the biosynthesized AgNPs were more effective antioxidants than U. lactuca’s aqueous extract; in other terms, the DPPH radical inhibition of U-AgNPs (IC50 = 23.03 ± 0.015 μg/mL), was higher than that of the aqueous extract (IC50 = 38.20 ± 0.02 μg/mL) and analogous to the ascorbic acid standard (IC50 = 13.49 ± 0.353 μg/mL) as mentioned in Figure 6.
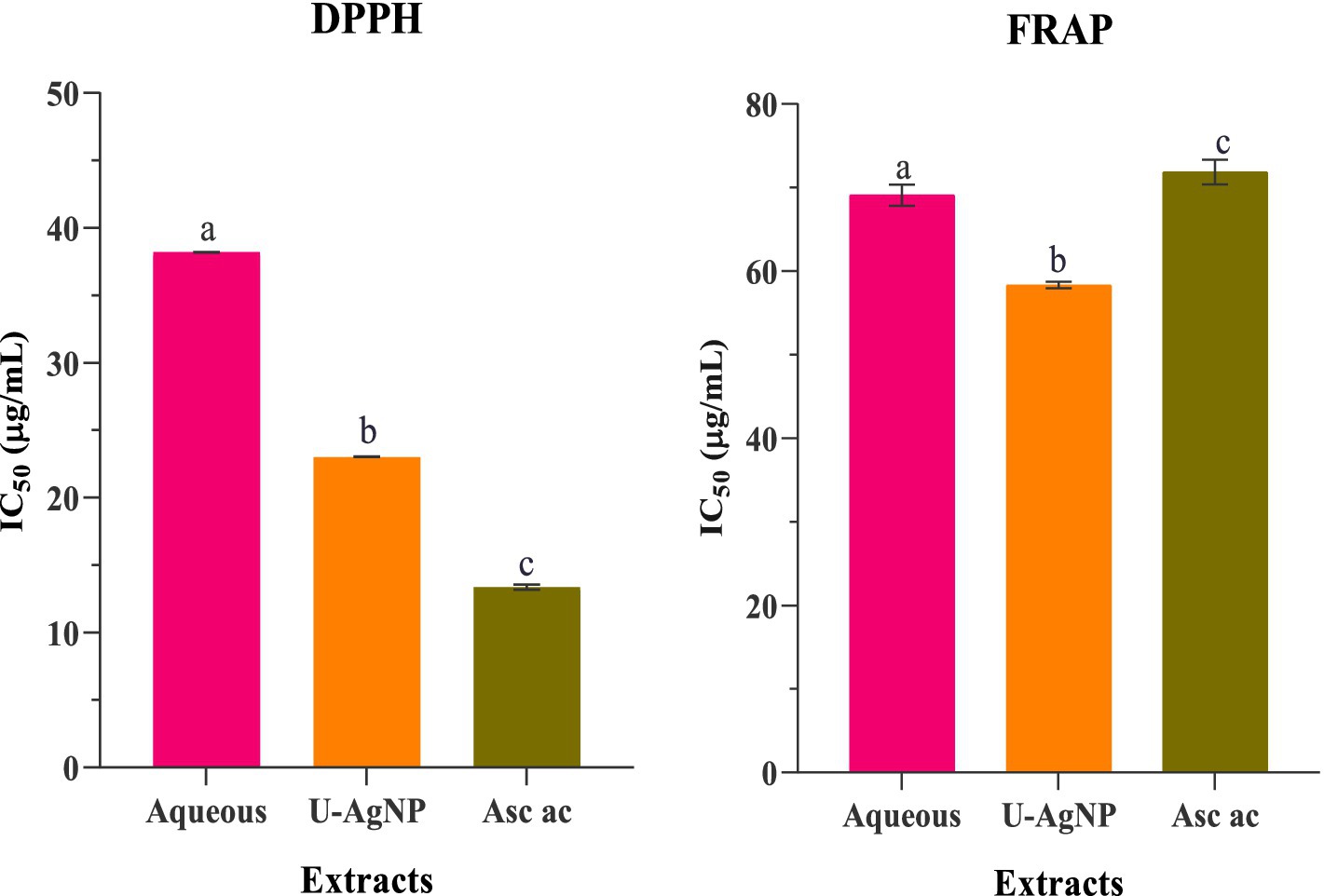
Figure 6. Antioxidant activity using the DPPH and FRAP methods, demonstrating the IC50 values for standard ascorbic acid, U-AgNP, and aqueous extracts. Columns marked with different alphabets (a, b, and c) indicate a statistically significant difference between the samples (p < 0.05).
The significant difference (p < 0.05) is illustrated by the letters a, b, and c.
Furthermore, the FRAP experiment demonstrated that tested extracts had concentration-dependent action at various concentrations (15.62–1,000 μg/mL). The reducing activity of the U-AgNP extract was significantly higher than that of the aqueous, with an IC50 value of 58.08 ± 1.25 μg/mL. Ascorbic acid (71.86 ± 1.48 μg/mL) was used as a reference antioxidant to compare the reduction activity (Figure 6).
The exceptional antioxidant activity of U-AgNPs, as demonstrated by the FRAP and DPPH tests, can be attributed to their diverse phytochemical composition, particularly the presence of flavonoids and phenolic acids. These phytochemicals work as a capping agent by adhering to AgNP surfaces, interacting and efficiently scavenging free radicals. Our results align with those of who reported that the green-produced AgNPs from the marine seaweed Gracilaria edulis’ aqueous extract showed an increased DPPH radical scavenging activity with an IC50 value of 30.71 ± 0.22 μg/mL. In comparison, at 400 mg/mL of NPs, AgNPs manufactured utilizing green algae Spirogyra hyalina showed strong antioxidant activity and effectively scavenged the DPPH free radicals (Abdullah et al., 2021). AgNPs have demonstrated a remarkable ability to scavenge DPPH free radicals, making them a viable option for utilization in antioxidant tests, AgNPs are also strong antioxidants (Mittal et al., 2012).
Another explanation provided by Vijayan et al. (2019) suggests that the stickiness of bioactive substances to the spherically structured NPs is responsible for the enhanced antioxidant activities of NPs over extract the bioactive compounds’ adhesion to the spherically shaped NPs is what gives the NPs extract its increased antioxidant activity. Comparatively, AgNPs made from the extract of Melia azedarach showed increased antioxidant capacity, as per the extract (Elemike et al., 2017). Researchers claim that flavonoids, terpenoids, and phenolic compounds found in plants are what give AgNPs their antioxidant capacity. These chemicals also enable AgNPs to serve as reducing agents, hydrogen donors, and singlet oxygen quenchers (Elemike et al., 2017). Moreover, green-synthesized AgNPs revealed excellent antioxidant activity (Yaglioglu et al., 2022; Erenler and Gecer, 2022).
The ability of structurally distinct phenolic compounds to function as antioxidants was assessed. The formation of AgNPs seemed to be significantly influenced by the hydroxylation of the aromatic ring. Phenolic compounds’ high degree of hydroxylation demonstrated both a strong ability to scavenge radicals and a propensity to convert Ag+ into AgNPs (Docea et al., 2021). The functional groups that cling to AgNPs from algal extract are thought to be responsible for their antioxidant activity. These groups suppress molecular oxidation by preventing the start of a chain reaction, which results in the creation of stable, non-reactive radicals (Arif and Uddin, 2020).
According to these techniques, Ag+ and Ag2+ are the two oxidation states in which silver can exist, depending on the circumstances of the reaction, and the synthesized AgNPs are possibly capable of squelching free radicals by either giving or receiving electrons. This is the mechanism by which AgNPs exert their antioxidant effect (Shanmugasundaram et al., 2013; Bedlovicˇová et al., 2020). The consequent antioxidant activity of AgNPs mostly depends on the extract’s reducing agents bound or capped to the NPs’ surface. AgNPs’ antioxidant properties have been found to depend on their size, surface coating, surface charge, stability, nature, and chemical composition (Khalil et al., 2020).
Antibacterial activity of aqueous and U-AgNP extracts
Agar diffusion method
Using the agar disk diffusion method, the antibacterial activity of U. lactuca aqueous extract and the produced AgNPs were examined against the human pathogenic bacterial isolates. Table 2 summarizes the results. The zone of inhibition formed by the produced AgNPs demonstrated strong antibacterial activity. The most significant inhibition zone of 8.7 mm was observed in the aqueous extract of U. lactuca. In comparison, the mean zone of inhibition was 8.4, 8.2, and 8 mm against S. aureus, S. epidermidis, and K. pneumoniae, respectively. Among the examined microorganisms, the AgNPs produced from U. lactuca exhibited a 22.5-mm zone of inhibition against E. coli, followed by S. aureus, S. epidermidis, and K. pneumoniae. The least susceptible microorganism was E. cloacae. Additionally, E. coli was more responsive to aqueous and AgNP extracts. The current investigation discovered that U. lactuca was efficient against Gram-positive and Gram-negative bacteria. Ulva fasciata mediates AgNPs’ antibacterial activity against E. coli, as revealed by Ragaa et al. (2018). The antibacterial activity of U. fasciata’s aqueous extract against E. coli (3 mm), S. aureus (3 mm), and K. pneumoniae (3 mm) was reported by Chellaram et al. (2015). DMSO, distilled water, and AgNO3 showed no antibacterial activity in the experiments.
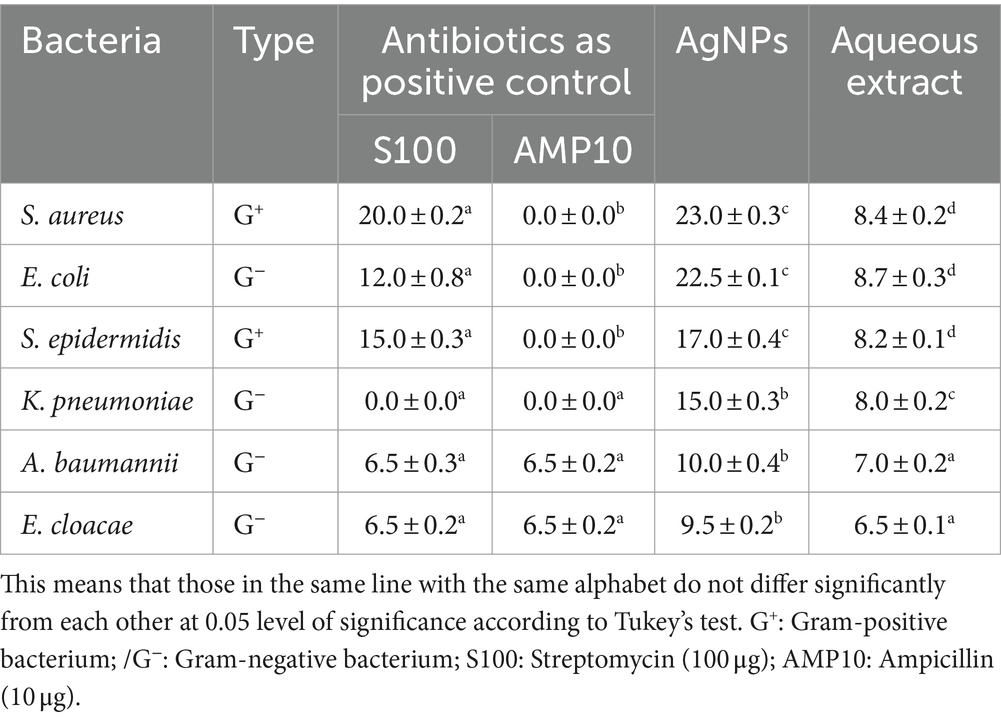
Table 2. Antibacterial activity of aqueous and synthesized AgNPs from Ulva lactuca expressed in inhibition zone (mm).
MIC determination
The MIC value, which is the lowest concentration of an antimicrobial agent (MIC) that stops a microorganism from developing, can be used to assess the effectiveness of NPs (Parvekar et al., 2020). The findings in Table 3 show that up to 2 μL/mL of U-AgNP extract impeded the development of S. aureus and E. coli. In comparison, this extract at a concentration of 4 μL/mL was able to block the development of S. epidermidis. AgNPs were examined for antibacterial activity, and according to Kamble et al. (2023), K. pneumoniae revealed a MIC value of 25 μL/mL, and E. coli demonstrated a MIC value of 50 μL/mL.
The results of the study indicate that U-AgNPs exhibited a greater significant antibacterial ability compared to the aqueous extract, indicating that Ag ions are better agents for antimicrobial activity (Tamayo et al., 2014; Rather et al., 2019). U-AgNPs were also found to have greater bacterial potency, as well as various S. aureus and E. coli strains. This may be due to their enhanced encounters with the proteins and polysaccharides on cell walls (Prasher et al., 2018).
It is interesting to note that the functions of bacteria can be altered by the concentration of silver nitrate (Pandian et al., 2010). At a concentration of 1 mM silver nitrate, AgNPs were biosynthesized, according to Kalimuthu et al. (2008). Furthermore, the bacteria continued to survive while the AgNPs were being synthesized and started growing again after the silver was taken out of their surroundings (Kalimuthu et al., 2008).
In vitro antifungal analysis of aqueous and U-AgNPs extracts
A. alternata, A. versicolor, B. cinerea, F. equiseti, and Penicillium spp. were the antifungal agents against which aqueous and U-AgNPs were tested in vitro (Table 4). The antifungal activity of different concentrations, including 2.5, 1.25, and 0.62 mg/mL, was examined. The growth capacity of several fungi was dose-dependently reduced by aqueous or U-AgNP extracts. Additionally, AgNPs have shown efficacy and provide the most promising antifungal action against B. cinerea, F. equiseti, A. alternata, Penicillium spp., and A. versicolor, with inhibition rates of 100, 88, 68.45, 58.33, and 50%, respectively, at the dosage of 2.5 mg/mL (Table 4). When compared to the standard reference antifungal drug, Teldor 50% sc, we found good antifungal activity, when compared to the negative control, all the tested extract dosages demonstrated an antifungal effect on the tested pathogens. Aqueous extract showed the lowest activity against A. versicolor and Penicillium spp.; A. alternata was moderately sensitive to aqueous extract.
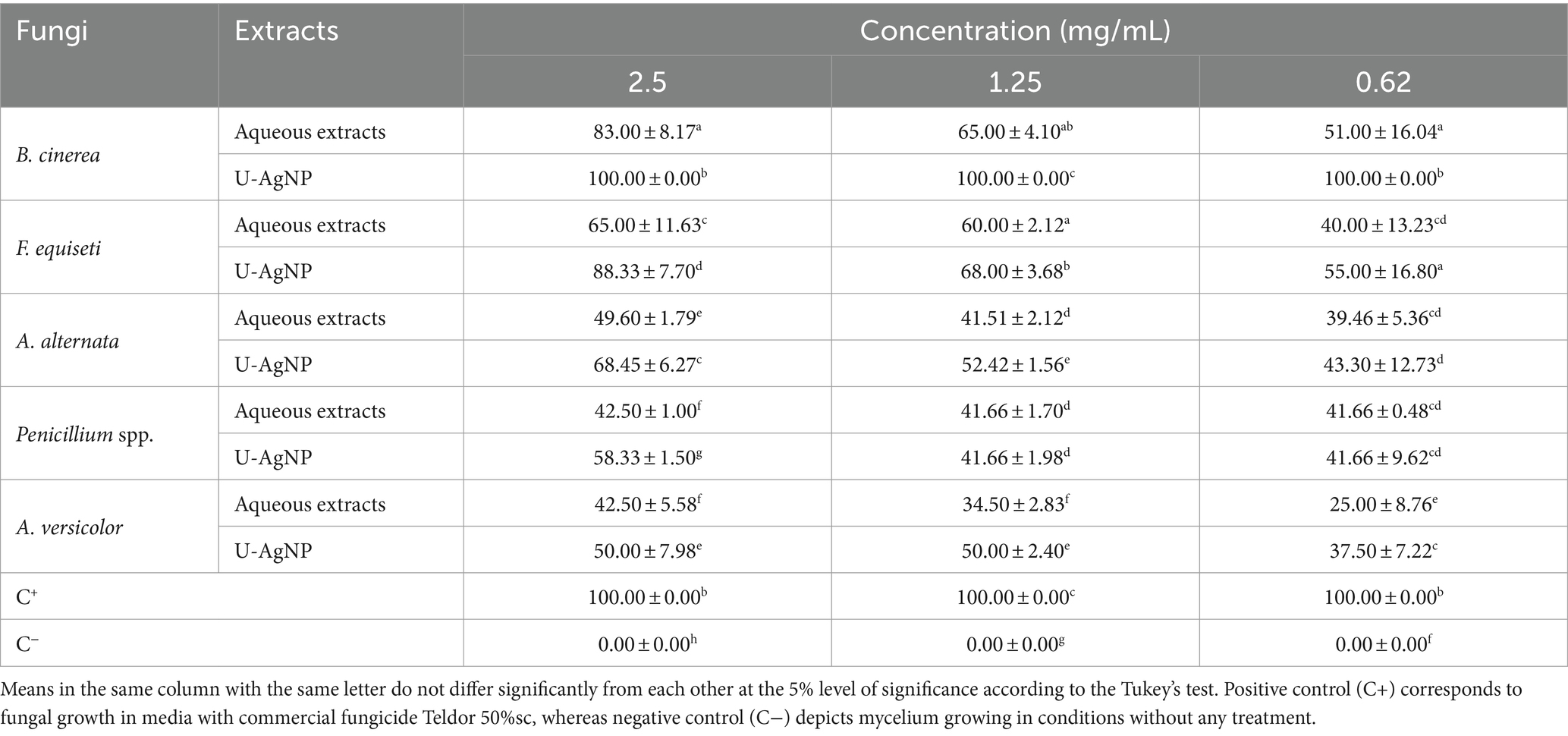
Table 4. Antifungal activity of U-AgNP and aqueous extracts on different doses of fungi expressed in percentage of inhibition (%).
In similar studies, the fungi used in our study have been tested by different algae species extracts for identifying antifungal activities by several researchers, such as Fusarium sp. (Shanmugam, 2014), A. alternata (Vehapi et al., 2020), Penicillium spp. (Lagrouh et al., 2017) and B. cinerea (El-Bilawy et al., 2022). According to published research, this study is the first to look into the antifungal properties of the aqueous and AgNP extracts of U. lactuca against A. versicolor and F. equiseti. This feature makes the study more original.
The findings point to the existence of bioactive substances in both kinds of extracts that can prevent the growth of certain fungi. Since it contains phytochemical components, which include protein, lipids, pigments, polyphenols, polysaccharides, alkaloids, terpenes, chlorophylls, and carotenoids that may be utilized as antioxidants and antimicrobials, U. lactuca has wide range of biological activity (Chojnacka et al., 2012; Sakthieaswari and Srisudha, 2016; Manchu et al., 2014). However, the effectiveness of AgNPs against fungal infections is only partially supported by research Vivek et al. (2011) investigated the red alga Gelidiella acerosa extract’s role in the creation, characterization, and use of biologically produced nanomaterials. They found that AgNPs had a significant antifungal effect when compared to conventional antifungal medications. While extensive research has been conducted on AgNPs’ effects on bacteria, their function in antifungal effects has not garnered as much attention (Bruna et al., 2021; Khaldoun et al., 2024). On the contrary, AgNPs’ microbial agent mechanism is still up for debate and is not entirely understood (Rafińska et al., 2019); It is commonly known that when fungal cells are exposed to AgNP solution, pits form on the membrane’s surface, destroying the integrity of the cells (Wen et al., 2022). Moreover, the Ag NPs’ antifungal activity is probably closely related to their smaller size and shape because of their increased surface area, which enhances their antimicrobial activity (Osonga et al., 2020). Additionally, based on experimental evidence, it has been demonstrated that exposure to silver ions causes DNA to lose its ability to replicate, leading to a reduction in cell viability and, ultimately, death (Takáč et al., 2023).
The fungicidal effect of U-AgNPs on detached leaves, fruits, and plants: mitigating/managing gray mold disease
To investigate whether the extracts include any characteristics that could shield tomato and strawberry leaves, fruits, and petioles against B. cinerea, the treatments with aqueous and U-AgNP extracts were applied before pathogen challenge at various doses (0.62, 1.25, and 2.5 mg/mL).
At concentrations of 1.25, and 2.5 mg/mL, U-AgNPs considerably inhibited the growth of B. cinerea’s mycelia, but at 0.62 mg/mL, the effect of the aqueous extract was significantly diminished. Based on the suppressed effects on mycelia growth and the antifungal effects of U-AgNPs on PDA, we selected a concentration of 1.25 and 2.5 mg/mL for our next fungal infection trials on detached leaves, fruits, and plants.
The aqueous extract did not produce any decrease in the degree of damage in leaves following infection by pathogens. On tomato leaves, the aqueous extract showed no considerable inhibition; however, at concentrations of 2.5 and 1.25 mg/mL, strawberry leaves showed moderate inhibition (77 and 79%) compared to the negative control (100%). However, U-AgNP extract appears to decrease the injury in tomato and strawberry leaves inoculated with B. cinerea (Figure 7). U-AgNP extract appears to be more effective at producing the protective effect, leading to a decrease in the extent of damages created after the pathogen infection compared to the negative control.
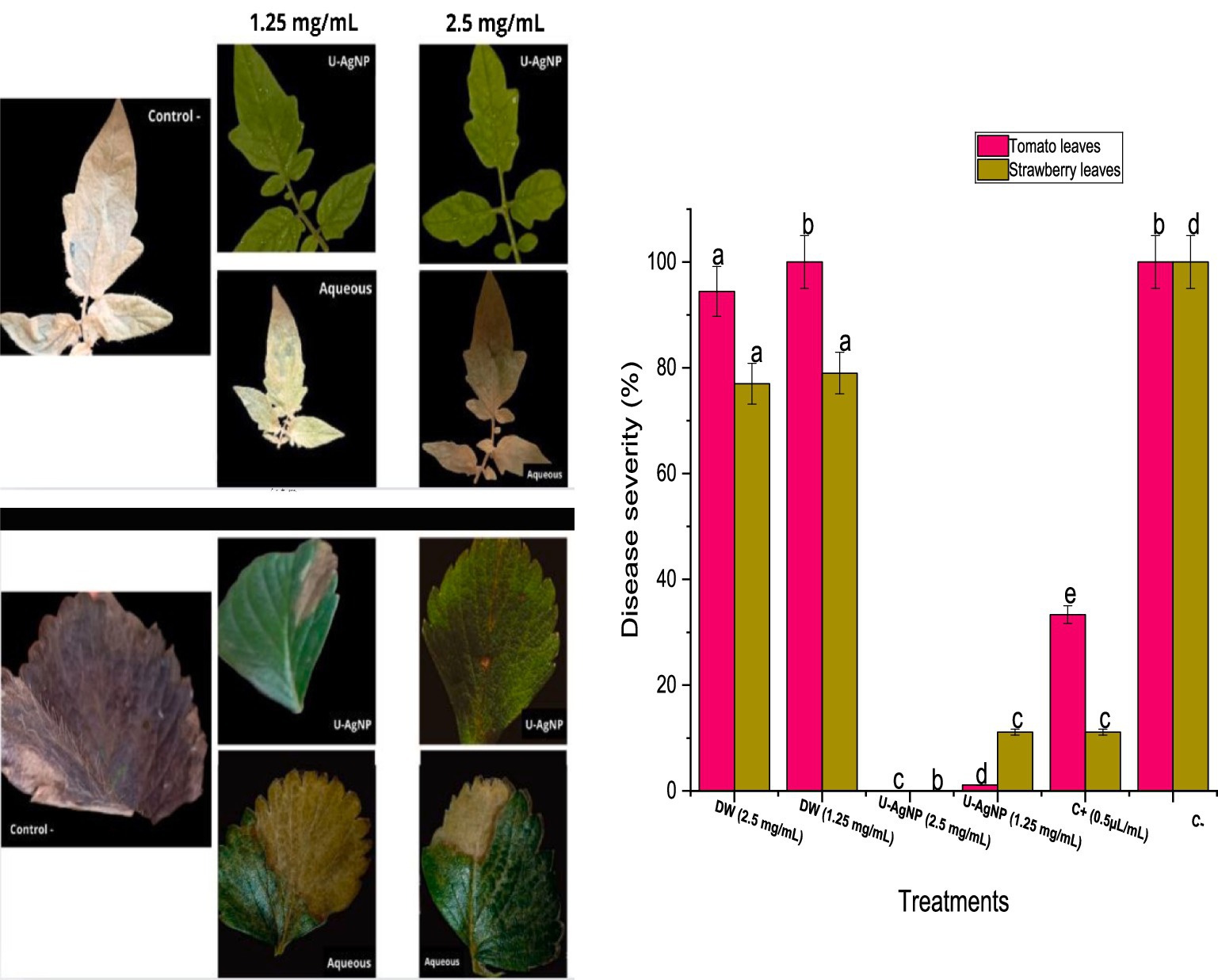
Figure 7. U-AgNPs and aqueous extracts possess antifungal properties. Tomato and strawberry leaves treated with varying concentrations of aqueous or U-AgNPs (1.25, 2.5 mg/mL) were used in the in vivo antifungal activity. Columns marked with different letters (a–e) indicate a statistically significant difference between the samples (p < 0.05).
Additionally, our research looked into U-AgNPs’ possible ability to prevent gray mold on strawberries and tomatoes (Figure 8). On the outer layer of the infected tomato and strawberry fruits treated with U-AgNPs at the examined concentrations, we did not find any B. cinerea lesions during this experiment (Figure 8), at concentrations of 1.25 and 2.5 mg/mL, however, a percentage of 33% of disease severity were seen in contrast when it comes to the fruits sprayed with the commercial fungicide. This indicates that U-AgNPs, at concentrations of 1.25 and 2.5 mg/mL, greatly controlled or decreased the damage that B. cinerea does to fruits. Conventional fungicide treatment of tomato and strawberry fruits also decreased disease symptoms, although not to the same “a lesser extent” as the U-AgNP treatment, at concentrations of 1.25 and 2.5 mg/mL. However, the aqueous extract had practically an inhibitory effect on B. cinerea in tomato fruits despite significant deviations from the negative control; on strawberry fruits, however, the aqueous extract had a disease severity percentage that was slightly similar to the negative control (94%), particularly at the concentration of 1.25 mg/mL (88%) and a lower value at the concentration of 2.5 mg/mL (66%). This implies that B. cinerea induced lesions on fruits, which U-AgNPs greatly reduced.
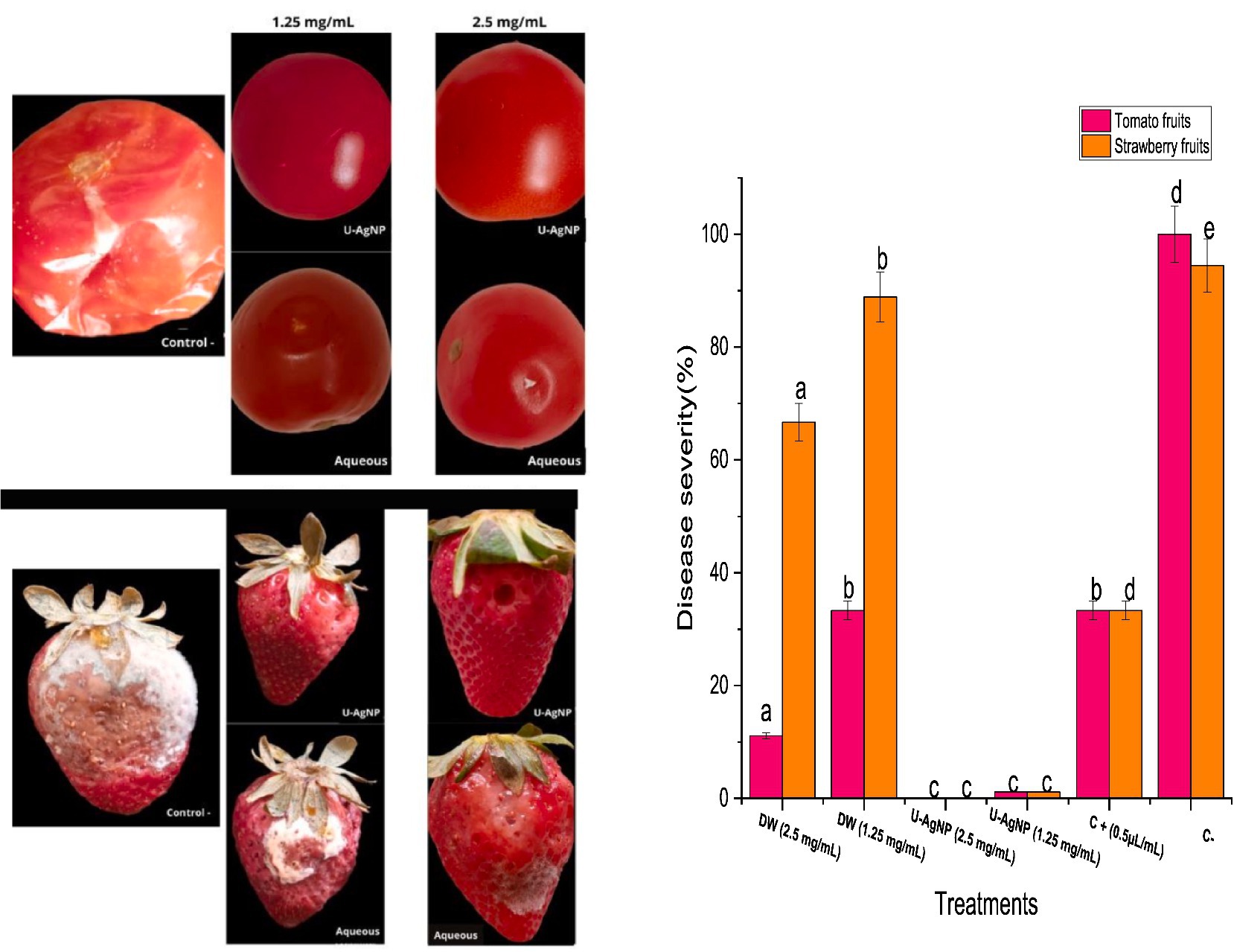
Figure 8. Aqueous and U-AgNP treatments were examined for their capacity to provide protection utilizing tomato and strawberry fruits with varying concentrations of aqueous or U-AgNPs (1.25, 2.5 mg/mL). Columns marked with different letters (a–e) indicate a statistically significant difference between the samples (p < 0.05).
Moreover, the tomato and strawberry plants were used to assess U-AgNPs’ ability to prevent gray mold. In strawberry plants, the U-AgNP extract was more effective than the commercial fungicide; however, in tomato plants, the U-AgNP extract outperformed the commercial fungicide. The results showed 100% efficiency (0% disease severity percentage) against B. cinerea at the concentration of 2.5 mg/mL, which was more potency than the commercial fungicide (Figure 9).
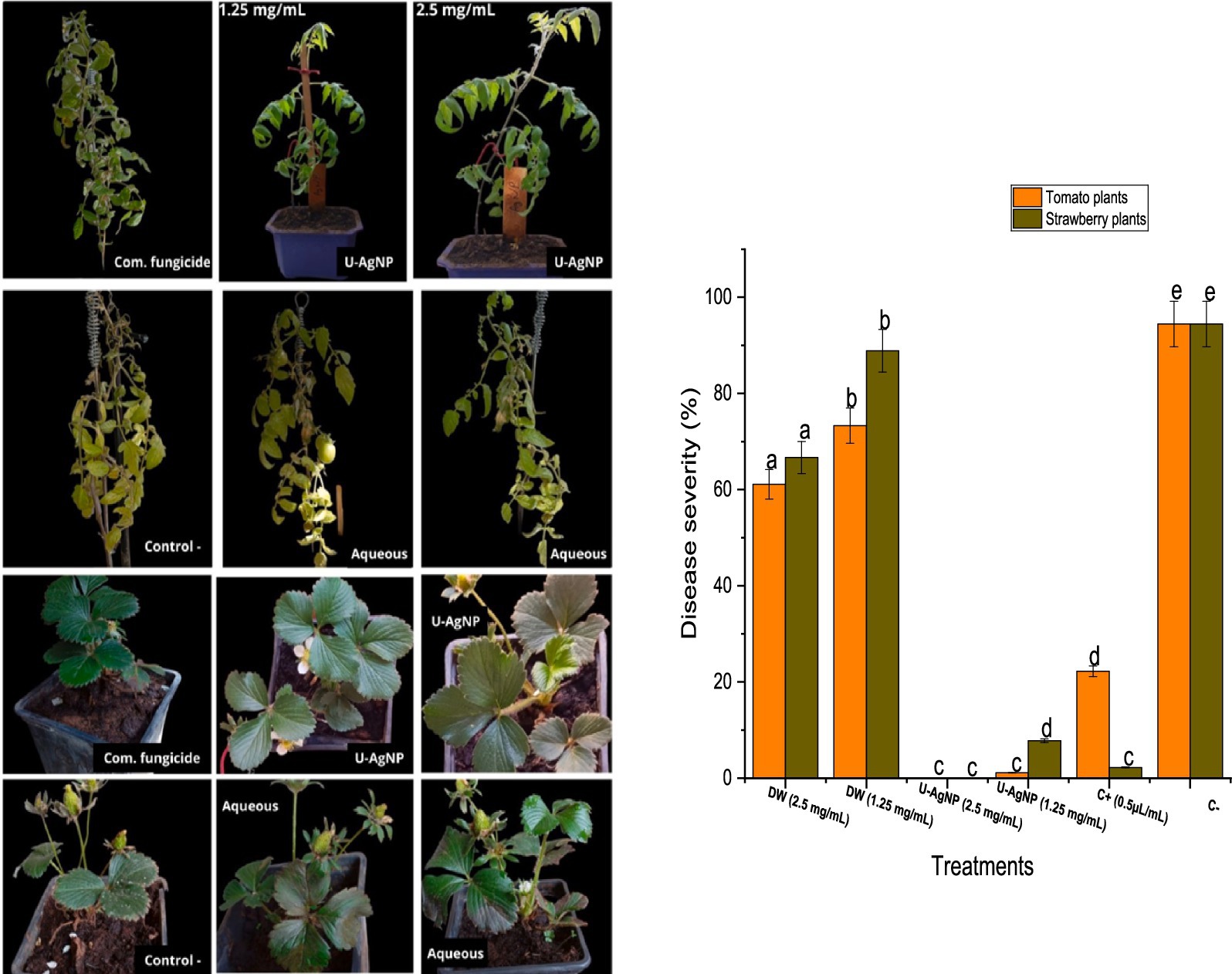
Figure 9. The severity of the gray mold illness on the entire tomato plant and how well they respond to treatment with aqueous and U-AgNPs at 1.25 and 2.5 mg/mL concentrations and Teldor 50% sc as commercial fungicide. Columns marked with different letters (a–e) indicate a statistically significant difference between the samples (p < 0.05).
The protective capacity of the U-AgNP extract was more effective at 2.5 mg/mL, obtaining a 100% level of protection across the whole plant, including fruits and leaves. At a lower concentration of 1.25 mg/mL, the extract decreased the amount of damage to the leaves (1 and 11% for tomato and strawberry leaves, respectively) compared to 100% damage in the negative control (Figure 7). The findings indicate that U-AgNP extract contains one or more active principles that, in a dose-dependent manner, may protect leaves from the pathogenic fungus B. cinerea.
Using fungicides is the main strategy for managing plant disease. However, research is necessary to produce novel, biodegradable, effective, and safe products; nevertheless, this pathogen has developed resistance to single-site inhibitors. In this study, we conducted both in vivo and in vitro experiments to investigate the antifungal activity of U-AgNPs against B. cinerea, the primary cause of the gray mold. Nevertheless, there has not been much research conducted on AgNPs’ in vivo antifungal activity. According to Elmer et al., NPs may penetrate root cells and migrate to various areas of the treated plants leading to resistance throughout the system (Elmer and White, 2016).
Due to their unique qualities, NPs have sparked a lot of concern last 10 years. These particles can swiftly pierce the matrix because they are reactive. To create green NPs, non-toxic and ecologically safe processes are employed. For NP biosynthesis, plant extracts are utilized in the bioreduction of metal ions, which is a quick, inexpensive, bacteria-free, and easily scaled-up process (Qayyum and Khan, 2016). As a result, the findings of our in vivo antifungal assay strongly support the creation of agrochemical NP agents on a platform that is both affordable and environmentally friendly. Overall, U. lactuca extract-based “green synthesis” of AgNPs eliminates the risks associated with using toxic reducing/capping agents and is a viable, affordable, and ecologically friendly method (Haseeb et al., 2019). This would significantly help reduce the use of dangerous pesticides, which are extremely detrimental when used near food crops such as fruits and vegetables (Venkatesan et al., 2016). According to current evidence, U-AgNPs can effectively replace conventional fungicides in agricultural pest management.
Ensuring the safe application of AgNPs in agricultural contexts requires thorough risk evaluations and continuous monitoring of AgNPs’ impact on human health and the environment. Regulations and guidelines can be implemented to help minimize the hazards and maximize the advantages of these cutting-edge nanomaterials. Although biosynthesized AgNPs have benefits, the environmental and health effects of using them in agrochemicals must be carefully considered. The biosynthesis process should be optimized to create AgNPs that exhibit decreased toxicity while retaining their antimicrobial efficacy. Our future goal is to reduce negative interactions with non-target organisms and minimize potential dangers to ecosystems and animal health by concentrating on developing safer AgNP formulations that can support sustainable farming practices through thorough testing and assessment.
Conclusion
Implementing nanotechnology in agriculture may offer a secure and efficient means of eradicating plant diseases. AgNPs from U. lactuca were studied using FTIR, XRD, UV–vis spectrophotometry, and EDX. Ag+ was discovered to be reduced to Ag, with a corresponding shift in the UV–vis spectral peak from 401 nm to 440 nm. AgNP functional groups were identified through FTIR analysis, and the crystalline structure of the biosynthesized AgNPs was demonstrated through XRD investigation. SEM analysis revealed that the AgNPs were spherical, with diameters ranging from 45 to 61 nm. The presence of Ag in the biosynthesized AgNPs was confirmed through EDX analysis, while the DPPH and FRAP tests verified the antioxidant qualities of the material. AgNPs that were biologically produced have shown antibacterial efficacy against both Gram-positive and Gram-negative bacteria. Through both in vivo and in vitro experiments, the antifungal activity of the nanoparticles was validated. Even at extremely low concentrations, U-AgNPs exhibit remarkable antifungal efficacy. When combined, U-AgNPs have the potential to act as a fungistat by efficiently suppressing B. cinerea infections and improving the quality of detached leaves, fruits, and plants at comparatively low concentrations (2.5 mg/mL). Because of their antifungal characteristics, NPs have a great deal of promise for use in horticulture, as our study demonstrated.
Data availability statement
The datasets presented in this study can be found in online repositories. The names of the repository/repositories and accession number(s) can be found in the article/supplementary material.
Ethics statement
The manuscript presents research on animals that do not require ethical approval for their study.
Author contributions
MT: Data curation, Conceptualization, Formal analysis, Investigation, Project administration, Validation, Writing – original draft. MA: Formal analysis, Methodology, Writing – review & editing. HI: Data curation, Writing – review & editing. FA: Data curation, Methodology, Writing – review & editing. AsO: Methodology, Writing – review & editing. AbO: Formal analysis, Software, Writing – review & editing. LH: Funding acquisition, Resources, Writing – review & editing. AS: Data curation, Funding acquisition, Resources, Writing – review & editing. ON: Data curation, Funding acquisition, Resources, Writing – review & editing. MT: Data curation, Writing – review & editing. RB: Investigation, Project administration, Resources, Supervision, Validation, Writing – review & editing. OH: Investigation, Validation, Writing – review & editing.
Funding
The author(s) declare that financial support was received for the research, authorship, and/or publication of this article. Researchers supporting project RSPD2024R1057, Kind Saud university, Riyadh, Saudi Arabi.
Acknowledgments
The authors extend their appreciation to the Researchers Supporting project number (RSPD2024R1057), King Saud University, Riyadh, Saudi Arabia. The authors also wish to thank Professor Douira Allal from the Laboratory of Animal Production and AgroIndustry, Faculty of Sciences, Ibn Tofail University, Kenitra, Morocco, and Professor Chakir Said from the Laboratory of Valorization of Medicinal and Aromatic Plants and Environment, Faculty of Sciences, Moulay Ismail University, Meknes, Morocco, for their technical assistance.
Conflict of interest
The authors declare that the research was conducted in the absence of any commercial or financial relationships that could be construed as a potential conflict of interest.
Publisher’s note
All claims expressed in this article are solely those of the authors and do not necessarily represent those of their affiliated organizations, or those of the publisher, the editors and the reviewers. Any product that may be evaluated in this article, or claim that may be made by its manufacturer, is not guaranteed or endorsed by the publisher.
References
Abdel-Raouf, N., Al-Enazi, N. M., Ibraheem, I. B. M., Alharbi, R. M., and Alkhulaifi, M. M. (2018). Biosynthesis of silver nanoparticles by using of the marine brown alga Padina pavonia and their characterization. Saudi J Biol Sci. 26, 1207–1215. doi: 10.1016/j.sjbs.2018.01.007
Abdullah, N. S., Al-Radadi, T., Faisalc, H., and Shaha, S. A. R. (2021). Novel biosynthesis, characterization and bio-catalytic potential of green algae (Spirogyra hyalina) mediated silver nanomaterials. Saudi J. Biolog. Sci. 29, 411–419. doi: 10.1016/j.sjbs.2021.09.013
Ahamad, A., and Kumar, J. (2023). Pyrethroid pesticides: an overview on classification, toxicological assessment and monitoring. J Hazardous Materials Advan. 10:100284. doi: 10.1016/j.hazadv.2023.100284
Al-Fa’ouri, M., Abu-Kharma, M. H., and Awwad, A. M. (2021). Green synthesis of copper oxide nanoparticles using Bougainvillea leaves aqueous extract and antibacterial activity evaluation. Chem. Int. 7, 155–162. doi: 10.5281/zenodo.4899095
Amin, H. H. (2019). Ulva lactuca as a cheap and safe bio pesticide in fields and its chemical composition (in vitro). Egyptian J. Aquatic Biol. Fisheries 23, 415–428. doi: 10.21608/ejabf.2019.67227
Aouji, M., Imtara, H., Rkhaila, A., Bouhaddioui, B., Alahdab, A., Parvez, M. K., et al. (2023). Nutritional composition, fatty acids profile, mineral content, antioxidant activity and acute toxicity of the flesh of Helix aspersa Müller. Molecules 28:6323. doi: 10.3390/molecules28176323
Arif, R., and Uddin, R. (2020). A review on recent developments in the biosynthesis of silver nanoparticles and its biomedical applications. Medical Devices and Sensors 4:e10158. doi: 10.1002/mds3.10158
Bedlovicˇová, Z., Strapácˇ, I., Baláž, M., and Salayová, A. (2020). A brief overview on antioxidant activity determination of silver nanoparticles. Molecules 25:3191. doi: 10.3390/molecules25143191
Bharose, A. A., Hajare, S. T., Soni, M., Prajapati, K. K., Singh, S. C., and Upadhye, V. (2024). Bacteria-mediated green synthesis of silver nanoparticles and their antifungal potentials against aspergillus flavus. PLoS One 19:e0297870. doi: 10.1371/journal.pone.0297870
Bhimba, B. V., and Kumari, P. R. (2014). Photosynthesis of silver nanoparticles from the extracts of seaweed Ulva Lactuca and its antimicrobial activity. Int J Pharm. Bio. Sci 5, 666–677.
Boedeker, W., Watts, M., Clausing, P., and Marquez, E. (2020). The global distribution of acute unintentional pesticide poisoning: estimations based on a systematic review. BMC Public Health 20, 1–19. doi: 10.1186/s12889-020-09939-0
Bruna, T., Maldonado-Bravo, F., Jara, P., and Caro, N. (2021). Silver nanoparticles and their antibacterial applications. Int. J. Mol. Sci. 22:7202. doi: 10.3390/ijms22137202
Cech, R., Zaller, J. G., Lyssimachou, A., Clausing, P., Hertoge, K., and Linhart, C. (2023). Pesticide drift mitigation measures appear to reduce contamination of nonagricultural areas, but hazards to humans and the environment remain. Sci. Total Environ. 854:158814. doi: 10.1016/j.scitotenv.2022.158814
Chellaram, C., Raja, P., and Diraviya, R. K. (2015). Medicinal properties of Ulva fasciata and Ulva reeticulate. Int. J. Res. Pharmaceutical Sci. 6, 1–6.
Chojnacka, K., Saeid, A., Witkowska, Z., and Tuhy, L. (2012). Biologically active compounds in seaweed extracts - the prospects for the application. Open Conference Proceed J 3, 20–28. doi: 10.2174/1876326X01203020020
Choudhary, S., Kumawat, G., Khandelwal, M., Khangarot, R. K., Sangela, V., Kumar, M., et al. (2023). Sustainable phyco-fabrication of silver nanoparticles using Coelastrella terrestris and their multiple downstream applications. Biocatal. Agric. Biotechnol. 53:102854. doi: 10.1016/j.bcab.2023.102854
Docea, A. O., Calina, D. A. M., Buga, O., Zlatian, M. M. B., Paoliello, G. D., Mogosanu, C. T., et al. (2021). The effect of silver nanoparticles on antioxidant/pro-oxidant balance in a murine model. Int. J. Mol. Sci. 21, 1233–1239. doi: 10.3390/ijms21041233
Elbialy, Z. I., Assar, D. H., Abdelnaby, A., Asa, S. A., Abdelhiee, E. Y., Ibrahim, S. S., et al. (2021). Healing potential of Spirulina platensis for skin wounds by modulating bFGF, VEGF, TGF-ß1 and α-SMA genes expression targeting angiogenesis and scar tissue formation in the rat model. Biomed. Pharmacother. 137:111349. doi: 10.1016/j.biopha.2021.111349
El-Bilawy, E. H., Al-Mansori, A. N. A., Alotibi, F. O., Al-Askar, A. A., Arishi, A. A., Teiba, I. I., et al. (2022). Antiviral and antifungal of Ulva fasciata extract: HPLC analysis of polyphenolic compounds. Sustain. For. 14:12799. doi: 10.3390/su141912799
Elemike, E. E., Fayemi, O. E., Ekennia, A. C., Onwudiwe, D. C., and Ebenso, E. E. (2017). Silver nanoparticles mediated by costus afer leaf extract: synthesis, antibacterial, antioxidant and electrochemical properties. Molecules 22:701. doi: 10.3390/molecules22050701
Elmer, W. H., and White, J. C. (2016). The use of metallic oxide nanoparticles to enhance growth of tomatoes and eggplants in disease infested soil or soilless medium. Environ. Sci. Nano 3, 1072–1079. doi: 10.1039/C6EN00146G
Erenler, R., and Gecer, E. N. (2022). Synthesis of silver nanoparticles using Sideritis montana L. leaf extract: characterization, catalytic degradation of methylene blue and antioxidant activity. J. Nano Res. 75, 17–28. doi: 10.4028/p-333bjm
Fang, L., Liao, X., Jia, B., Shi, L., Kang, L., Zhou, L., et al. (2020). Recent progress in immunosensors for pesticides. Biosens. Bioelectron. 164:112255. doi: 10.1016/j.bios.2020.112255
Fayaz, A. M., Balaji, K., Girilal, M., Yadav, R., Kalaichelvan, P. T., and Venketesan, R. (2010). Biogenic synthesis of silver nano- particles and their synergistic effect with antibiotics: a study against gram-positive and gramnegative bacteria. Nanomedicine 6, 103–109. doi: 10.1016/j.nano.2009.04.006
García-Sánchez, A., Miranda-Díaz, A. G., and Cardona-Muñoz, E. G. (2020). The role of oxidative stress in physiopathology and pharmacological treatment with pro- and antioxidant properties in chronic diseases. Oxidative Med. Cell. Longev. 16:2082145. doi: 10.1155/2020/2082145
Hanif, M., Munir, N., Abideen, Z., Yong, J. W. H., El-Keblawy, A., and El-Sheikh, M. A. (2024). Synthesis and optimization of nanoparticles from Phragmites karka improves tomato growth and salinity resilience. Biocatal. Agric. Biotechnol. 55:102972. doi: 10.1016/j.bcab.2023.102972
Harinee, S., Muthukumar, K., Dahms, H. U., Koperuncholan, M., Vignesh, S., Banu, R. J., et al. (2019). Biocompatible nanoparticles with enhanced photocatalytic and antimicrofouling potential. Int. Biodeterior. Biodegradation 145:104790. doi: 10.1016/j.ibiod.2019.104790
Haseeb, M., Khan, M. S., Baker, A., Khan, I., Wahid, I., and Jaabir, M. S. M. (2019). Anticancer and antibacterial potential of MDR Staphylococcus aureus mediated synthesized silver nanoparticles. Biosci. Biotechnol. Res. Commun. 12, 26–35. doi: 10.21786/bbrc/12.1/4
Hashemabadi, M., Sasan, H., Amandadi, M., Mohammadinejad, R., Farnoosh, G., Azimzadeh, M., et al. (2021). Natural gum as bio-reductant to green synthesize silver nanoparticles: assessing the apoptotic efficacy on MCF-7 and SH-SY5Y cell lines and their antimicrobial potential. Polym. Bull. 78, 2867–2886. doi: 10.1007/s00289-020-03238-9
Hassan, H., Mohamed, M., Yusoff, S., Hata, E., and Tajidin, N. (2021). Selecting antagonistic yeast for postharvest biocontrol of Colletotrichum gloeosporioides in papaya fruit and possible mechanisms involved. Agronomy 11:760. doi: 10.3390/agronomy11040760
Kah, M., Kookana, R. S., Gogos, A., and Bucheli, T. D. (2018). A critical evaluation of nanopesticides and nanofertilizers against their conventional analogues. Nat. Nanotechnol. 13, 677–684. doi: 10.1038/s41565-018-0131-1
Kalimuthu, K., Suresh Babu, R., Venkataraman, D., Bilal, M., and Gurunathan, S. (2008). Biosynthesis of silver nanocrystals by Bacillus licheniformis. Colloids Surf. B: Biointerfaces 65, 150–153. doi: 10.1016/j.colsurfb.2008.02.018
Kamble, P. S., Gadade, J. P., Patil, D. S., Jagtap, M. A., Jayannawar, D. P., Nimbalkar, M. S., et al. (2023). Biosynthesis of silver nanoparticles using Drimia indica and exploring its antibacterial profile. J. Nanosci. Nanotechnol. Res. 7:4. doi: 10.12769/ipnnr-23.7.01
Khaldoun, K., Khizar, S., and Saidi-Besbes, S. (2024). Synthesis of silver nanoparticles as an antimicrobial mediator. J Umm Al-Qura University of Appl Sci. doi: 10.1007/s43994-024-00159-5
Khalifa, K. S., Hamouda, R. A., Hanafy, D., and Hamza, A. (2016). In vitro antitumor activity of silver nanoparticles biosynthesized by marine algae. Dig. J. Nanomater. Biostruct. 11, 213–221.
Khalil, I., Yehye, W. A., Etxeberria, A. E., Alhadi, A. A., Dezfooli, S. M., Julkapli, N. B. M., et al. (2020). Nanoantioxidants: recent trends in antioxidant delivery applications. Antioxidants 9:24. doi: 10.3390/antiox9010024
Khan, B. A., Nadeem, M. A., Nawaz, H., Amin, M. M., Abbasi, G. H., Nadeem, M., et al. (2023). Pesticides: impacts on agriculture productivity, environment, and management strategies. Emerging Contaminants and Plants: interactions, Adaptations and Remediation Technologies. 10, 109–134. doi: 10.1007/978-3-031-22269-6_5
Krishnan, M., Subramanian, H., Dahms, H. U., Sivanandham, V., Seeni, P., Gopalan, S., et al. (2018). Biogenic corrosion inhibitor on mild steel protection in concentrated HCl medium. Nat. Sci. Rep. 8:2609. doi: 10.1038/s41598-018-20718-1
Kumar, S., Manokar, S. N., Thirunavookarasu, N., Nivethitha, V., Nidhusri, T. N., Niranjana, T., et al. (2022). Characterization of power ultrasound modified kappaphycus alvarezii biosorbent and its modeling by artificial neural networks. Air Soil Pollu. 233:234. doi: 10.1007/s11270-022-05690-x
Lagrouh, F., Dakka, N., and Bakri, Y. (2017). The antifungal activity of Moroccan plants and the mechanism of action of secondary metabolites from plants. J. Medical Mycol. 27, 303–311. doi: 10.1016/j.mycmed.2017.04.008
Liu, H., Taylor, T. H. Jr., Pettus, K., Johnson, S., Papp, J. R., and Trees, D. (2016). Comparing the disk-diffusion and agar dilution tests for Neisseria gonorrhoeae antimicrobial susceptibility testing. Antimicrob. Resist. Infect. Control 5:46. doi: 10.1186/s13756-016-0148-x
Macdonald, I. D. G., and Smith, W. E. (1996). Orientation of cytochrome C adsorbed on a citrate-reduced silver colloid surface. Langmuir 12, 706–713. doi: 10.1021/la950256w
Manchu, N., Melpha, Y., and James, J. E. (2014). Phytochemical investigation of three species of Ulva from Rasthacaud coast, Tamil Nadu, India. J. Chem. Pharm. Res. 6, 570–574.
Mittal, A. K., Kaler, A., and Banerjee, U. C. (2012). Free radical scavenging and antioxidant activity of silver nanoparticles synthesized from flower extract of Rhododendron dauricum. NANO Biomed. Eng. 4, 114–128. doi: 10.5101/nbe.v4i3.p118-124
Muthukumar, K., Vignesh, S., Dahms, H. U., Gokul, M. S., Palanichamy, S., Subramanian, G., et al. (2015). Antifouling assesments on biogenic nanoparticles: a filed study from polluted offshore platform. Mar. Pollut. Bull. 73, 743–755. doi: 10.1016/j.msec.2016.12.062
Notteghem, J. L., Andriatompo, G. M., Chatel, M., and Dechanet, R. (1980). Techniques utilisées pour la sélection de variétés de riz possédant la résistance horizontale à la pyriculariose. Annales de Phytopathologie 12, 199–266.
Nwosu, M. O., and Okafor, J. I. (1995). Preliminary studies of the antifungal activities of some medical plants against Basidiobolus and some other pathogenic Fungi. Mycoses 38, 191–195. doi: 10.1111/j.1439-0507.1995.tb00048.x
Onaran, A., and Yilar, M. (2012). Antifungal activity of Trachystemon orientalis L. aqueous extract against plant pathogens. J Food, Agricul Environ 10, 278–291. doi: 10.13140/RG.2.1.1368.1765
Osonga, F. J., Akgul, A., Yazgan, I., Akgul, A., Eshun, G. B., Sakhaee, L., et al. (2020). Size and shape-dependent antimicrobial activities of silver and gold nanoparticles: a model study as potential fungicides. Molecules (Basel, Switzerland) 25:2682. doi: 10.3390/molecules25112682
Oubihi, A., Ouryemchi, I., Nounah, I., Tarfaoui, K., Harhar, H., Ouhssine, M., et al. (2020). Chemical composition, antibacterial and antifungal activities of Thymus leptobotrys Murb essential oil. Adv. Tradit. Med. 20, 673–679. doi: 10.1007/s13596-020-00488-w
Oyaizu, M. (1986). Studies on products of Browning reactions: Antioxidative activities of product of Browning reaction prepared from glucosamine. Japan J Nutrit 44, 307–315. doi: 10.5264/eiyogakuzashi
Pandian, K. S. R., Deepak, V., Kalishwaralal, K., Viswanathan, P., and Gurunathan, S. (2010). Mechanism of bactericidal activity of silver nitrate - a concentration dependent bi-functional molecule. Brazilian J. Microbiol. Pub. Brazilian Society for Microbiol 41, 805–809. doi: 10.1590/S1517-83822010000300033
Parvekar, P., Palaskar, J., Metgud, S., Maria, R., and Dutta, S. (2020). The minimum inhibitory concentration (MIC) and minimum bactericidal concentration (MBC) of silver nanoparticles against Staphylococcus aureus. Biomaterial Investigations in Dentistry 7, 105–109. doi: 10.1080/26415275.2020.1796674
Pérez-de-Luque, A. (2020). Guest edited collection: nanotechnology in agriculture. Sci. Rep. 10:15738. doi: 10.1038/s41598-020-73198-7
Prasher, P., Singh, M., and Mudila, H. (2018). Silver nanoparticles as antimicrobial therapeutics: current perspectives and future challenges. 3Biotech 8:411. doi: 10.1007/s13205-018-1436-3
Qayyum, S., and Khan, A. U. (2016). Nanoparticles vs. biofilms: a Battle against another paradigm of antibiotic resistance. Med. Chem. Commun. 7, 1479–1498. doi: 10.1039/c6md00124f
Rafińska, K., Pomastowski, P., and Buszewski, B. (2019). Study of Bacillus subtilis response to different forms of silver. Sci. Total Environ. 661, 120–129. doi: 10.1016/j.scitotenv.2018.12.139
Ragaa, A. F. H., Abd El-Mongy, M., and Fatehy Eid, K. (2018). Antibacterial activity of silver nanoparticles using Ulva fasciata extracts as reducing agent and sodium dodecyl sulfate as stabilizer. Int. J. Pharmacol. 14, 359–368. doi: 10.3923/ijp.2018.359.368
Rahmi, M., and Putri, D. H. (2020). The antimicrobial activity of DMSO as a natural extract solvent. SERAMBI BIOLOGI 5, 56–58. doi: 10.24036/5909RF00
Rather, R. A., Sarwara, R. K., Das, N., and Pal, B. (2019). Impact of reducing and capping agents on carbohydrates for the growth of ag and cu nanostructures and their antibacterial activities. Particuology 43, 219–226. doi: 10.1016/j.partic.2018.01.004
Sakthieaswari, P., and Srisudha, S. (2016). Preliminary study on phytochemical analysis, mineral composition and antibacterial properties of Amphiroafragilissima (LINNAEUS) J V LAMOROUX and Ulva Reticulata Forsskal collected from mandapam coast, Tamil Nadu. Int. J. Recent Scientific Res. 7, 12084–12089.
Schumacher, A., Vranken, T., and Malhotra, A. (2018). In vitro antimicrobial susceptibility testing methods: agar dilution to 3D tissue-engineered models. Eur. J. Clin. Microbiol. Infect. Dis. 37, 187–208. doi: 10.1007/s10096-017-3089-2
Shanmugam, G. (2014). Review of research in internal-wave and internal-tide deposits of China: discussion. J. Palaeogeogr. 3, 2–20. doi: 10.3724/SP.J.1261.2014.00060
Shanmugasundaram, T., Radhakrishnan, M., and Gopikrishnan, V. (2013). A study of the bactericidal, anti-biofouling, cytotoxic and antioxidant properties of actinobacterially synthesized silver nanoparticles. Colloids Surf. B: Biointerfaces 111, 680–687. doi: 10.1016/j.colsurfb.2013.06.045
Singh, R. P., Handa, R., and Manchanda, G. (2020). Nanoparticles in sustainable agriculture: an emerging opportunity. J. Control. Release. 329, 1234–1248. doi: 10.1016/j.jconrel.2020.10.051
Singh, R., Kumar, N., Mehra, R., Kumar, H., and Singh, V. P. (2020). Progress and challenges in the detection of residual pesticides using nanotechnology based colorimetric techniques. Trends in Environmental Analytical Chemistry. 26:e00086. doi: 10.1016/j.teac.2020.e00086
Takáč, P., Michalková, R., Čižmáriková, M., Bedlovičová, Z., Balážová, Ľ., and Takáčová, G. (2023). The role of silver nanoparticles in the diagnosis and treatment of Cancer: are there any perspectives for the future? Life (Basel, Switzerland) 13:466. doi: 10.3390/life13020466
Tamayo, L. A., Zapata, P. A., Vejar, N. D., Azócar, M. I., Gulppi, M. A., Zhou, X., et al. (2014). Release of silver and copper nanoparticles from polyethylene nanocomposites and their penetration into Listeria monocyto-genes. Mater. Sci. Eng. C 40, 24–31. doi: 10.1016/j.msec.2014.03.037
Tarfaoui, K., Brhadda, N., Ziri, R., Oubihi, A., Imtara, H., Haida, S., et al. (2022). Chemical profile, antibacterial and antioxidant potential of Zingiber officinale roscoe and Elettaria cardamomum (L.) Maton Essential Oils and Extracts. Plan. Theory 11:1487. doi: 10.3390/plants11111487
Theodosoglou, E., Koroneos, A., Soldatos, T., Zorba, T., and Paraskevopoulos, K. M. (2010). Comparative Fourier transform infrared and X-ray powder diffraction analysis of naturally occurred K-feldspars. Bull. Geol. Soc. Greece 43, 2752–2761. doi: 10.12681/bgsg.11681
Trellu, C., Vargas, H. O., Mousset, E., Oturan, N., and Oturan, M. A. (2021). Electrochemical technologies for the treatment of pesticides. Curr. Opin. Electrochem. 26:100677. doi: 10.1016/j.coelec.2020.100677
Umapathi, R., Ghoreishian, S. M., Sonwal, S., Rani, G. M., and Huh, Y. S. (2022). Portable electrochemical sensing methodologies for on-site detection of pesticide residues in fruits and vegetables. Coord. Chem. Rev. 453:214305. doi: 10.1016/j.ccr.2021.214305
Vehapi, M., Koçer, A. T., Yılmaz, A., and Özçimen, D. (2020). Investigation of the antifungal effects of algal extracts on apple-infecting Fungi. Arch. Microbiol. 202, 455–471. doi: 10.1007/s00203-019-01760-7
Venkatesan, J., Kim, S. K., and Shim, M. S. (2016). Antimicrobial, antioxidant, and anticancer activities of biosynthesized silver nanoparticles using marine algae ecklonia cava. Nano 6:235. doi: 10.3390/nano6120235
Vijayan, S., Koilparambil, D., and Shanavas, J. (2019). In vitro anticancer evaluation of chitosan/biogenic silver nanoparticle conjugate on Si ha and MDA MB cell lines. Appl. Nanosci. 10, 715–728. doi: 10.1007/s13204-019-01151-w
Vivek, M., Kumar, P. S., Steffi, S., and Sudha, S. (2011). Biogenic silver nanoparticles by Gelidiella acerosa extract and their antifungal effects. Avicenna J. Medical Biotechnol. 3, 143–148
Waqif, H., Munir, N., Farrukh, M. A., Hasnain, M., Sohail, M., and Abideen, Z. (2024). Algal macromolecular mediated synthesis of nanoparticles for their application against citrus canker for food security. Int. J. Biol. Macromol. 263:130259. doi: 10.1016/j.ijbiomac.2024.130259
Wen, H., Shi, H., Jiang, N., Qiu, J., Lin, F., and Kou, Y. (2022). Antifungal mechanisms of silver nanoparticles on mycotoxin producing rice false smut fungus. iScience 26:105763. doi: 10.1016/j.isci.2022.105763
Yaglioglu, A. S., Erenler, R., Gecer, E. N., and Genc, N. (2022). Biosynthesis of silver nanoparticles using Astragalus flavesces leaf: identification, antioxidant activity, and catalytic degradation of methylene blue. J. Inorg. Organomet. Polym. Mater. 32, 3700–3707. doi: 10.1007/s10904-022-02362-5
Keywords: Ulva lactuca, silver nanoparticles, spectroscopy, safe synthetic pesticides, biosynthesis, antioxidant activity, antimicrobial activity
Citation: Taibi M, Aouji M, Imtara H, Abujaber F, Oubihi A, Ouannou A, Hajji L, Shahat AA, Noman OM, Tarayrah M, Bengueddour R and Hassani O (2024) Novel biosynthesis of silver nanoparticles using Ulva lactuca and their potential toward environment and agricultural purposes. Front. Sustain. Food Syst. 8:1490880. doi: 10.3389/fsufs.2024.1490880
Edited by:
M. Leonor Faleiro, University of Algarve, PortugalReviewed by:
Maria Miguel, University of Algarve, PortugalVignesh Sivanandham, Indian Institute of Food Processing Technology, India
Copyright © 2024 Taibi, Aouji, Imtara, Abujaber, Oubihi, Ouannou, Hajji, Shahat, Noman, Tarayrah, Bengueddour and Hassani. This is an open-access article distributed under the terms of the Creative Commons Attribution License (CC BY). The use, distribution or reproduction in other forums is permitted, provided the original author(s) and the copyright owner(s) are credited and that the original publication in this journal is cited, in accordance with accepted academic practice. No use, distribution or reproduction is permitted which does not comply with these terms.
*Correspondence: Hamada Imtara, SGFtYWRhLnRhcmF5cmFoQGdtYWlsLmNvbQ==