- 1Department of Soil Science, Luiz de Queiroz College of Agriculture, University of São Paulo, São Paulo, Brazil
- 2Center for Carbon Research in Tropical Agriculture (CCARBON), University of São Paulo, São Paulo, Brazil
- 3Inter-American Institute for Cooperation on Agriculture, San José, Costa Rica
- 4CFAES Rattan Lal Center for Carbon Management and Sequestration, School of Environment and Natural Resources, The Ohio State University, Columbus, OH, United States
Soil represents Earth’s largest terrestrial reservoir of carbon (C) and is an important sink of C from the atmosphere. However, the potential of adopting best management practices (BMPs) to increase soil C sequestration and offset greenhouse gas (GHG) emissions in agroecosystems remains unclear. Synthesizing available information on soil C sink capacity is important for identifying priority areas and systems to be monitored, an essential step to properly estimate large-scale C sequestration potential. This study brings an overview of thousands of research articles conducted in the Americas and presents the current state-of-the-art on soil C research. Additionally, it estimates the large-scale BMPs adoption impact over soil C dynamics in the region. Results indicated that soil C-related terms are widely cited in the literature. Despite that, from a total of ~13 thousand research articles recovered in the systematic literature review, only 9.2% evaluated soil C (at any depth), and only 4.6% measured soil C for the 0–30 cm soil layer, mostly conducted in North and South America regions. Literature review showed a low occurrence of terms related to BMPs (e.g., cover cropping), suggesting a research gap on the subject. Estimates revealed that upscaling of BMPs over 30% of agricultural land area (334 Mha) of the Americas can lead to soil C sequestration of 13.1 (±7.1) Pg CO2eq over 20 years, offsetting ~39% of agricultural GHG emissions over the same period. Results suggest that efforts should be made to monitor the impact of cropping system on soil C dynamics on the continents, especially in regions where data availability is low (e.g., Central, Caribbean, and Andean regions). Estimating the available degraded area for the continent and the soil C sequestration rates under BMPs adoption for Central, Andean, and Caribbean regions were major shortcomings encountered in our analysis. Thus, it is expected that some degree of uncertainty may be associated with the obtained results. Despite these limitations, upscaling of BMPs across the Americas suggests having great potential for C removal from the atmosphere and represents a global positive impact in terms of climate change mitigation and adaptation.
1 Introduction
Soil is the basic resource for maintenance of the life-supporting processes in the Earth’s Critical Zone (Brantley et al., 2007; Kraamwinkel et al., 2021). Soil’s multifunctionality is critical to strengthening essential ecosystem services for human well-being through the provisioning of food, feed, fiber, and energy; storage and purification of water; neutralization, filtering, and buffering of pollutants; and regulation of climate (Pereira et al., 2018). Being the planet’s largest reservoir of terrestrial carbon (C), soil can be both a source and a sink of CO2 (Lal, 2004). In natural ecosystems, the direction of C transfer between the pedosphere and atmosphere is regulated by natural factors such as temperature and rainfall, vegetation changes, fire events, and mineral weathering (Berner, 2003; Doetterl et al., 2015; Lal, 2005; Li et al., 2021). In managed ecosystems, anthropogenic factors are also important drivers, especially in increasing greenhouse gas (GHG) emissions by the retrieval and burning of fossil fuels, land-use change, increased livestock populations and overgrazing, and large-scale soil mobilization (conventional tillage and mining) (Le Quéré et al., 2009; Smith, 2008; Smith et al., 2014; Herrero et al., 2013). Thus, one priority is to find alternatives to reverse the direction of excess C flow by using plants to capture atmospheric CO2 and the soils to sequester it for the long term (Bossio et al., 2020; Lal et al., 2021; Sá et al., 2017).
The agricultural sector accounts for 21–35% of total anthropogenic GHG emissions (Crippa et al., 2021; Hong et al., 2021; Smith et al., 2014). Land use change, fertilizer application, enteric fermentation of ruminants, and soil tillage are major sources of nitrous oxide (N2O), methane (CH4), and carbon dioxide (CO2) emissions worldwide (Karakurt et al., 2012; Snyder et al., 2009). Rapidly rising demand for agricultural products due to the increase in human and livestock population and that of the purchasing power pressures the sector to increase production and making the task of reducing net anthropogenic emissions more urgent and dauntingly difficult (Stavi and Lal, 2013). However, unlike other sectors (i.e., energy, transportation, and industry) which must reduce emissions and offset GHGs (Amelung et al., 2020; Wollenberg et al., 2016), the agricultural sector can both reduce GHG emissions and capture and sequester C in the soil through the intensification and adoption of BMPs (e.g., no-till, pasture reclamation, and integrated agricultural systems).
The adoption of BMPs can increase land productivity, facilitate land-sparing and ecosystem restoration, reduce emissions, preserve natural ecosystems, and sequester C in soil concomitantly (Lal, 2015). In addition, BMPs improve soil health and reverse ecosystem degradation (IPBES, 2019; IPCC, 2022; Yang et al., 2024). Because soils have a finite capacity to stabilize C, decision-making must rely on real-world data of the potential for C sequestration in agricultural soils by adopting these practices (Stewart et al., 2007). In addition, establishing evidence-based soil C references tied to the adoption of BMPs could catalyze the availability of resources to farm systems adopt sustainable land management practices and generate, as a consequence, basis for the establishment of references for the C credit markets as by quantifying the C sequestration potential of soils. This, in turn, would benefit farmers and landowners who adopts BMPs, as they could monetize their efforts through the sale of carbon credits in these emerging markets as a co-product generated by the adoption of BMP’s in agrifood systems. Ultimately, this approach would not only drive the adoption of climate-resilient agricultural practices but also support global climate goals, aligning with the objectives of the Paris Agreement by reducing GHG emissions and enhancing C sinks.
The availability of field information on soil C data under cropping systems strategies is essential towards establishing public policies and large-scale initiatives for soil C sequestration. The Americas region, with an agricultural area of about 1.11 billion hectares (World Bank, 2023), is foreseen to present a great potential for contributing to climate change mitigation actions through BMPs expansion in agroecosystems. Despite that, little is known about the current state-of-the-art on the subject in the region, which represents a challenge for decision-making processes and the implementation of field initiatives. Therefore, the present study involves the Americas region as a case study for identifying the current available literature data on soil C and for estimating the potential of C sequestration in agricultural systems. Specifically, this article presents the current knowledge on C sequestration by BMPs produced in the Americas and highlight the existing gaps and the significant opportunities for further in-depth research in the topic. Additionally, it presents the estimates of the potential for C sequestration in the soil by the widespread use of BMPs in the agricultural sector, as well as the area required to mitigate agricultural GHG emissions.
2 Materials and methods
2.1 Bibliometric review
In the first part of the study, publications were searched on the Web of Science® platform. The query string was defined considering terms related to SOM dynamics in different agroecosystems, such as annual cropping systems, pasture, and integrated agricultural systems, as well as diverse management practices (e.g., tillage) that may occur within these systems. The search considered terms mentioned in each record’s “title, abstract, and keywords” (Topic Field), and it was limited to peer-reviewed articles, as they represent most high-quality published documents. The query string used was as follows:
“TS = “soil carbon stock* OR soil carbon OR soil organic* AND “agroforestry” OR “integrated systems”* OR “integrated crop-livestock”* OR “integrated crop-livestock-forestry”* OR silvopastoral* OR agropastoral* OR agrosilvopastoral* OR ICL* OR ICF* OR ICLF OR Past* OR grass OR rangeland OR “pasture management” OR “well-managed pasture” OR “nominal pasture” OR “improved pasture” OR “degraded pasture” OR “recovery pasture” OR “pasture restoration” OR “management system”* OR no-till* OR “conventional tillage” OR “zero-till*” OR “strip-till*” OR “reduced till*” OR “mulch based” OR “straw mulch based” OR “subsoiling” OR “plow” OR “disk” OR “chisel plow” OR “Ridge Tillage” AND CU = (34 member countries).”
The search was restricted to the American region (countries from Canada to Argentina, including the Caribbean) using the “countries/region” filtering tool available on the platform. The obtained documents were manually analyzed to quantify (i) how many were conducted in the region and (ii) how many assessed soil C stocks. Studies meeting both selection criteria that covered the first 30 cm soil profile depth were identified. This information was used to build up a spreadsheet accounting for the number of studies that measured C stocks in the continent.
2.2 Mitigation scenarios
2.2.1 Prediction of soil C sequestration capacity in the Americas and Caribbean regions
The agricultural land area of each country was delineated for estimating the soil C sequestration capacity through the implementation of BMPs in agriculture. The data on land area was obtained from World Bank (2023). Then, the proportion of this area that could be converted into BMPs was estimated by assuming areas under some level of degradation or underuse. In this study, we used the definition of land degradation based on a temporary or permanent reduction in the productive capacity of the land resulting from erosion, salinization, compaction, acidification, and chemical pollution of soils (FAO, 2015; UNEP, 1992). Based on the state of agricultural soils worldwide (FAO, 2015); information presented in the Soil Atlas of Latin America and the Caribbean (Gardi et al., 2013); and an assessment presented by the United Nations Convention to Combat Desertification in Latin America and the Carribean (UNCCD, 2019), we found that the proportion of degraded land varies from 26 to 33%. Therefore, it was assumed that around 30% of the total agricultural area of each country could be potentially improved, being the area adopted for further calculations (FAO, 2015; Gardi et al., 2013; UNCCD, 2019). The adoption of three potential strategies were identified: improved pasture (IP), conservation agriculture (CA), and integrated agricultural systems (IAS). For these three systems, different rates of C sequestration potential were attributed according to the country/region based on published data, including both literature reviews and results of field studies (Table 1). For the regions with no available information, the rates of the closest region were assumed (in terms of geography, climate, and agricultural practices; Supplementary Figure S3). The Supplementary Figure S4 illustrates the geographic location of the studies considered to extract the soil C sequestration rates.
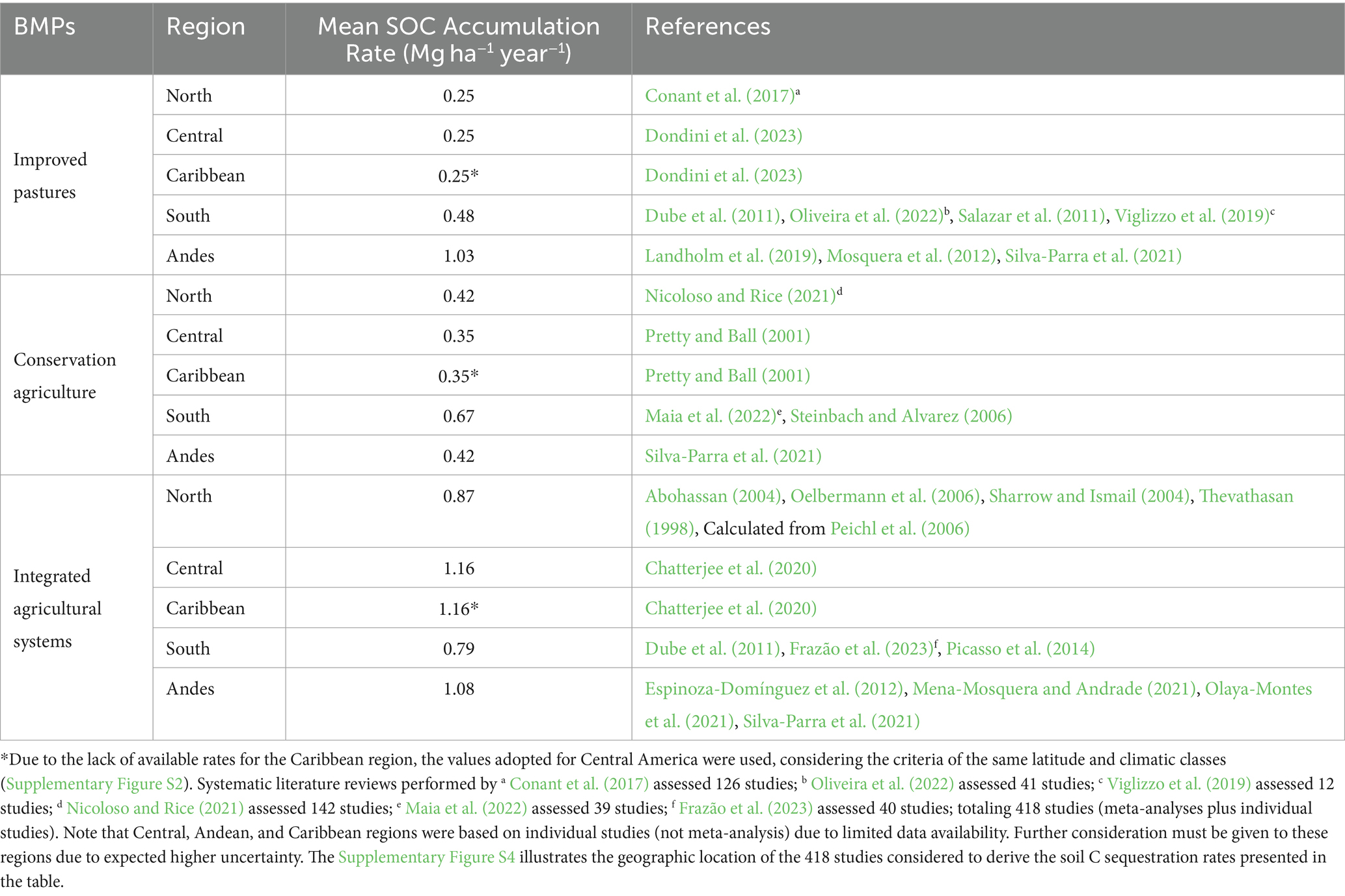
Table 1. Mean soil organic carbon accumulation rates by adoption of best management practices obtained from literature data for the Americas.
The soil C sequestration potential was estimated as the product of the available area and the annual C sequestration rates obtained in the literature. Two scenarios were projected, considering a timespan of eight (short-term scenario) and 20 years (maximum time of response up to steady state, by IPCC, 2019a), and a linear response for soil C accumulation for the 0–30 cm layer (plow layer). The short-term scenario (8 years) was projected to provide an estimate about soil C sequestration until 2030 (2022–2030), as many countries from the region presented GHG emissions reduction targets for 2030 (ClimateWatch database; https://www.climatewatchdata.org/). The 20-year scenario is referred to as 2050, assuming the 2030–2050 period. Since there is no standardization for the potential of BMPs implementation in the Americas, the proportions adopted in the Brazilian Sector Plan for Mitigation and Adaptation to Climate Change for the Consolidation of a Low-Carbon Economy in Agriculture (ABC Plan) were used. The ABC plan was released in 2010 (currently called RenovAgro) and was responsible for the mitigation of 131–169 MtCO2eq from 2010 to 2020 (Manzatto et al., 2020); and has a goal to mitigate other 1,076 MtCO2eq by 2030 Brazil (Frazão et al., 2023). The estimates were based on the implementation of 55% under IP, 25% under CA, and 20% under IAS over the available agricultural area of each country (i.e., 30% of the total agricultural area). The ABC Plan defined these proportions according to the required technical and monetary resources needed (i.e., low-demanding to high-demanding practices). The soil C sequestration potential was calculated following Equation 1:
where CSP is carbon sequestration potential, A is the area, ACSR is the annual C sequestration rates, and T is the timespan considered for the calculations, i.e., 8 and 20 years, which is the maximum response rate until steady state (IPCC, 2019a).
2.2.2 The need to expand BMPs to mitigate GHG emissions
The GHG emission datasets for the target countries were divided into economic sectors available in the ClimateWatch database. Total annual emissions were calculated by the agricultural sector of the most recent five-year period available (2015–2019) and calculated the mean annual emissions for each country individually. These datasets created two simulated scenarios for GHG emissions mitigation by adoption of BMPs, with 2030 and 2050 temporal targets, which would give short-and long-term overviews necessary to decision making. The soil C sequestration rates were then used under BMPs previously calculated to estimate the area needed to offset GHG emissions in both scenarios. The target area was calculated by dividing the mean annual GHG emissions by the mean annual SOC sequestration potential of the BMPs. For the 2030 scenario, the target was the reduction by 50% of the GHG emissions associated with the agricultural sector in each country to accelerate the mitigation process by the end of the decade (which would continue until 20 years by 2042). For the 2050 scenario, the target set was neutrality (offsetting 100% of emissions). The areas for each region of the continent were calculated as the sum of the area of each country in the region. The calculations were performed following Equation 2:
where A is the target area of BMP implementation necessary to offset agricultural emissions, GHG(2015–2019) is the mean annual greenhouse gas emissions from agricultural sector calculated for the 2015–2019 period, and SOCseq is the mean annual soil organic carbon sequestration potential of the BMPs.
The Supplementary Figure S1 summarizes the steps taken to perform the estimates presented in the study.
3 Results
3.1 Soil carbon stock available data and bibliometric analysis
The literature review suggests that, despite many documents retrieved, only a little information is available on C sequestration in agroecosystems of the Americas. From more than 13 thousand papers considered initially for this study, only around 1,189 documents (<9.2%) presented data on C stocks in the soil for the three evaluated systems (Figure 1); and the number was even lower for those reporting the data to 30 cm depth, i.e., 598 documents or 4.6% (Table 2). The bibliometric analysis extracted from the literature review revealed a set of 100 most used keywords, distributed in 5 groups, as shown in the keyword network co-occurrence diagram (Supplementary Tables S1, S2). When describing the studies, the authors used the terms and topics including soil organic matter, soil organic carbon, soil carbon, carbon sequestration, land use change, climate change, no-tillage, carbon dioxide, nitrogen, and phosphorus with greater frequency and intensity. Therefore, these terms are crucial and central to the topic addressed.
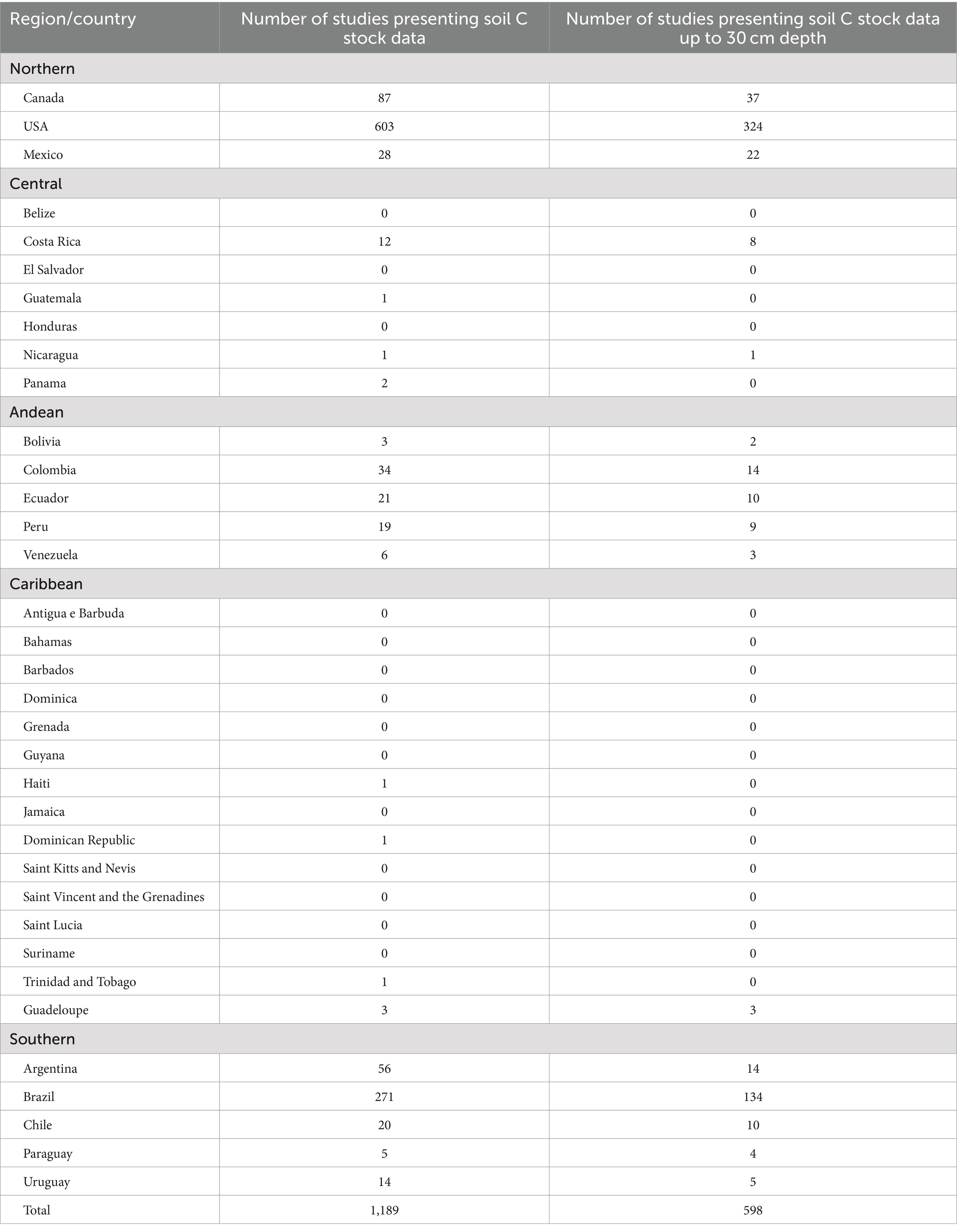
Table 2. The total number of studies returned by the search filtered C stock data for all depths, and 0–30 cm for the Americas and Caribbean.
3.1.1 North America
The North America region (i.e., Canada, USA, and Mexico) had the greatest number of studies, i.e., 718; 60.4% of total, presenting soil C data (Table 2). Considering the 0–30 cm soil layer, the region had 64% of the total number of studies (598) that measured soil C. The USA, specifically, presented 83.4 and 84.5% of the total amount of studies recovered in the North region considering overall soil layers and the complete first 30 cm soil layer, respectively (Table 2). The network co-occurrence diagram of the most cited keywords (not shown) was like the one observed for the whole dataset, with SOM-related keywords being dominant; besides other terms related to nutrient cycling (e.g., phosphorus, nitrogen, nutrients), GHG emissions, and soil biology. Best management practices-related keywords were restricted to “no-till,” which had a significant size contribution; and other keywords such as “agroforestry,” “conservation agriculture,” and “soil health/quality,” which showed a low contribution to the diagram (data not shown). Relevant studies identified in the search were the ones published by Conant et al. (2017) and Nicoloso and Rice (2021), which are extensive meta-analyses that focused soil C sequestration on pasture and no-tillage systems, respectively.
3.1.2 Central, Caribbean, and Andean regions
The Central and Caribbean regions showed the lowest number of studies that measured C stocks in the Americas, representing only 1.8% (both regions) of the total (Table 2). A total of 16 studies measured soil C stocks in the Central region, with most of them (i.e., 12) being conducted in Costa Rica. When considering the top 30 cm soil layer, however, only nine studies were identified, where eight were reported in Costa Rica and one study was conducted in Nicaragua (Table 2). In the Caribbean region, only six studies presented C stocks at any layer, and three studies were recovered presenting soil C stocks for the complete top 30 cm layer (Table 2), with most of them conducted in Guadaloupe. Due to the reduced number of studies, the network co-occurrence diagrams (not shown) revealed little information about the research published in these regions. Some of the few keywords highlighted were “agroforestry,” “coffee,” “carbon sequestration,” and “ecosystem services.” Relevant studies identified were the ones published by Chatterjee et al. (2020), Pretty and Ball (2001), and Dondini et al. (2023).
In the Andean region, the number of studies recovered in the search was higher than the observed for the Caribbean and Central areas, but still was reduced compared to the other regions assessed in the search. On total, 83 studies were recovered (7% of total) when considering any depths, and 38 studies were identified when the full top 30 cm layer was considered (Table 2). Colombia was the country that presented the greatest number of studies, 40.9 and 36.8% of the total number observed in the region for any depths and the full 30 cm layer, respectively. The co-occurrence diagram (not shown) suggested that research topics are mostly focused on “agroforestry,” “land conversion,” “carbon sequestration,” and “pasture.” Important studies identified in our research were the ones published by Landholm et al. (2019), Mosquera et al. (2012), and Silva-Parra et al. (2021).
3.1.3 South region
The southern region ranks second in the number of studies in the Americas, totaling 366 (Table 2). This total, which corresponds to 31% of studies carried out in the Americas, is distributed between Brazil, which holds 74% of studies (271), Argentina (56), Chile (20), Uruguay (14) and Paraguay (5). Around 46% of studies in the region (167) measured carbon in the 0–30 cm layer, while for Brazil and Chile, this percentage was 50%, with 134 and 10 studies, respectively (Table 2). The keyword co-occurrence diagram for the southern region (not shown) was like those obtained for the entire database (Figure 1) and the northern region, observing the predominance of keywords pertinent to SOM. Similarly, the word “no-till” was the only one that demonstrated a significant size among the terms related to best management practices, while for nutrient cycling, the most notable contribution was from “nitrogen.” Comprehensive studies involving meta-analysis were identified for the southern region, such as that of Viglizzo et al. (2019), which addressed the potential for carbon sequestration in pastures in Argentina, Brazil, Paraguay, and Uruguay. Maia et al. (2022) and Oliveira et al. (2022), conducted research in Brazil on the potential for C sequestration in no-till and pasture, respectively.
3.2 Soil organic carbon accumulation rates
Due to limited data availability for conducting a meta-analysis, specific studies were identified within our database that provided the C sequestration rates needed to perform further estimates for the studied region. More specifically, for regions where meta-analysis are available in the literature (e.g., North and South America), C sequestration rates obtained in these studies were prioritized (Conant et al., 2017; Frazão et al., 2023; Nicoloso and Rice, 2021; Viglizzo et al., 2019; Maia et al., 2022). For regions where meta-analysis were not available, and the identified studies were not sufficient to proceed with our originally chosen approach, C sequestration rates reported in specific (individual) studies were adopted (Table 1). It was found that the highest rates of soil C sequestration are obtained in integrated agricultural systems (IAS), especially in the Central and Andean regions (Table 1). Accumulation rates (Mg ha−1 year−1) for IAS ranged from 0.79 to 1.16 for the five regions, 0.25 to 1.03 for improved pastures (IP), and 0.42 to 0.67 for conservation agriculture (CA). The average rates of SOC accumulation (Mg ha−1 year−1) for the three selected BMPs were 0.50 (IP), 0.46 (CA), and 0.97 (IAS). Given the lack of data for the Caribbean region, rates for this region were assumed to be the same as those for the Central region, considering the criteria of the same geographic position (latitude) and climate classes (Supplementary Figure S2). Furthermore, the values of the C sequestration rates were extracted from the documents which either contained these data, or provided the necessary information (e.g., timespan of management) to calculate the rates. The Supplementary Figure S4 illustrates the geographic location of the studies used to derive the soil C sequestration rates.
3.3 Soil carbon sequestration potential in the Americas and Caribbean regions
Upscaling BMPs over 334 Mha in the Americas (30% of the land dedicated to agriculture and livestock, presumed degraded for the region) would sequester up to 5.2 (±2.9) Pg CO2eq by 2030 (8-year period from 2022 to 2030) and would present an overall potential of 13.1 (±7.1) Pg CO2eq over 20 years (Figure 2). The northern and southern regions had the greatest potential for soil C sequestration, contributing to 38.9 and 38.6% of the total C sink capacity, respectively. The highest potential of soil C sequestration in the southern region was observed in Brazil, comprising 3.07 Pg CO2eq over 20 years (60.4% of the total potential for the southern region; Supplementary Table S2). In the northern region, the USA has the greatest C sink capacity, with a potential of 3.7 Pg CO2eq (72.3% of the total capacity of the region). Together, Brazil and USA represent 51.5% of the total capacity of the Americas (13.1 Pg CO2eq; Supplementary Table S2). The Andean region has the third largest potential (20.6%), followed by the central (1.4%) and the Caribbean regions (0.5%; Figure 2).
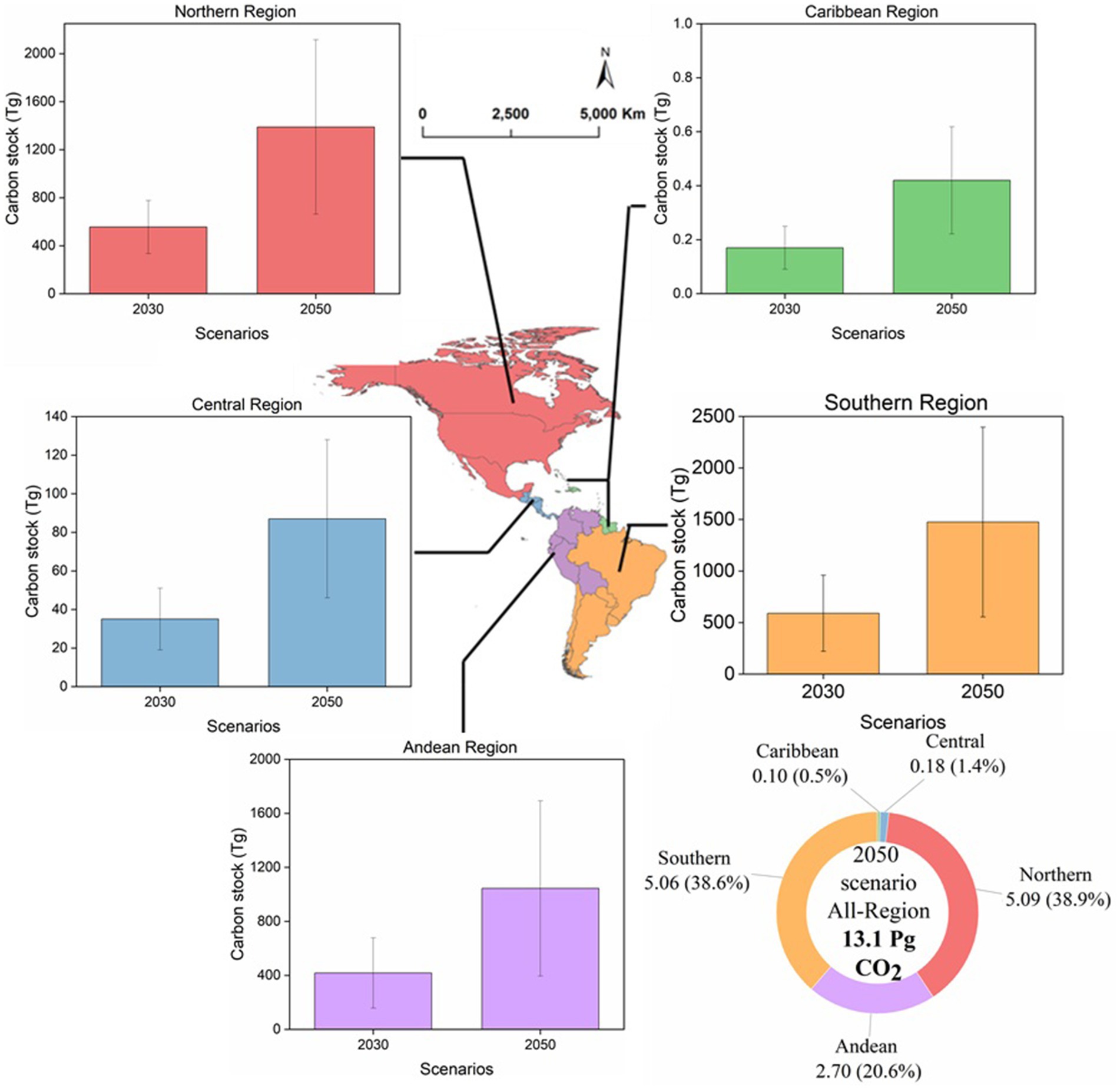
Figure 2. Estimated soil C sequestration capacity (Mt) for the 0–30 cm depth up to 2030 (2030 scenario) and 2050 (2050 scenario). The 2030 scenario was calculated considering a timespan of 8 years (2022–2030), while the 2050 scenario was calculated considering a timespan of 20 years, which is the maximum time of response for soil C under the application of a determined management practice (IPCC, 2019a,b). The estimated potential for soil C sequestration was based on a combination of pasture reclamation, no-tillage, and integrated systems (e.g., agroforestry, silvopastoral, integrated crop-livestock forest systems) expansion. The estimates were calculated according to the agricultural area of each country, assuming the availability of 30% of the total area to be recovered through the implementation of BMPs. Obs.: Following the Table 1, calculated estimates for Central, Andean, and Caribbean regions were performed using individual studies due to lack of meta-analysis data. For this reason, these regions may present a higher level of uncertainty in the estimates compared to the South and North regions.
3.4 Neutrality of GHG emissions from the agricultural sector in the Americas and the Caribbean region
There is little information regarding the status of soil degradation in the Americas. However, the few information available indicates that around 30% of the land is degraded in the region, which could be restored and increase soil C sequestration over time (FAO, 2015; Gardi et al., 2013; UNCCD, 2019). In such case, the adoption of BMP in those 30% of the land dedicated with agricultural practices (crops and livestock, i.e., 334 Mha) would offset 39.2% (i.e., 0.66 Pg CO2eq year−1) of the annual agricultural GHG emissions (i.e., 1.68 Pg CO2eq year−1) over 20 years (Figure 3). For a complete offset of annual emissions, however, it would be necessary to produce with the selected BMPs in 915 Mha (Figure 3), corresponding to 82.4% of the total agricultural area (i.e., 1.11 B ha, per the World Bank, 2023). The south and north regions have the greatest need for expansion of the BMP to achieve neutrality of total GHG emissions from agriculture, i.e., 323 and 507 Mha, respectively (Figure 4). On the other hand, although the Caribbean and the Central regions require a smaller area (11 and 23 Mha, respectively) to mitigate the sector’s emissions, these exceed the agricultural area available in these regions by 30 and 74%, respectively. Among all regions, the Andean region requires the least area to completely offset emissions from agriculture, needing about 36% of the available agricultural area (50.5 Mha; Supplementary Table S2), exceeding by only 6% of the baseline value adopted in this study (30% of the total agricultural area) as an available area for upscaling of BMPs. In contrast, the northern and southern regions need approximately 90 and 83%, respectively. For a short-term mitigation scenario, with 50% GHG reductions by 2030, the Andean region is the only one that could reach the target without exceeding the reference area (Figure 3; Supplementary Table S2). It is worth noting that the higher the level of adoption of BMPs in the region, the shorter would be the time needed to achieve net-zero emissions targets, as the need to scale up the selected technologies depends on the current level in the region.
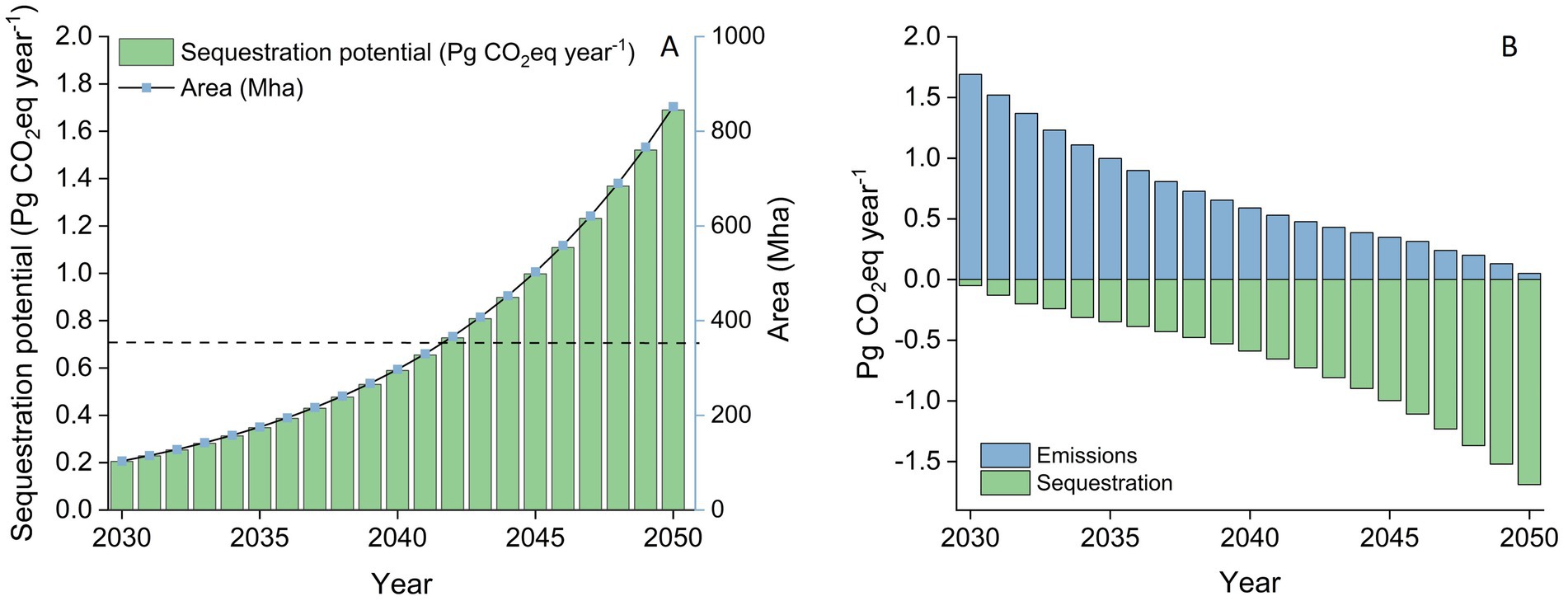
Figure 3. Simulated evolution of the potential for CO2eq sequestration in the soil due to the expansion of BMPs in agricultural areas in the Americas (A); and estimated impact of increasing soil CO2eq sequestration over agricultural emissions (B). Dashed lines represent the available estimated agricultural area that could be restored through BMPs in each country/region, which was assumed to be 30% of the total agricultural area (FAO, 2015). Note that graph “A” represents the relationship between the area implemented under BMPs and the soil C sequestration potential that would be achieved. The curve shape represents a hypothetical scenario of BMPs expansion for achieving C neutrality in the agricultural sector within a 20-year period (i.e., timespan to reach steady state).
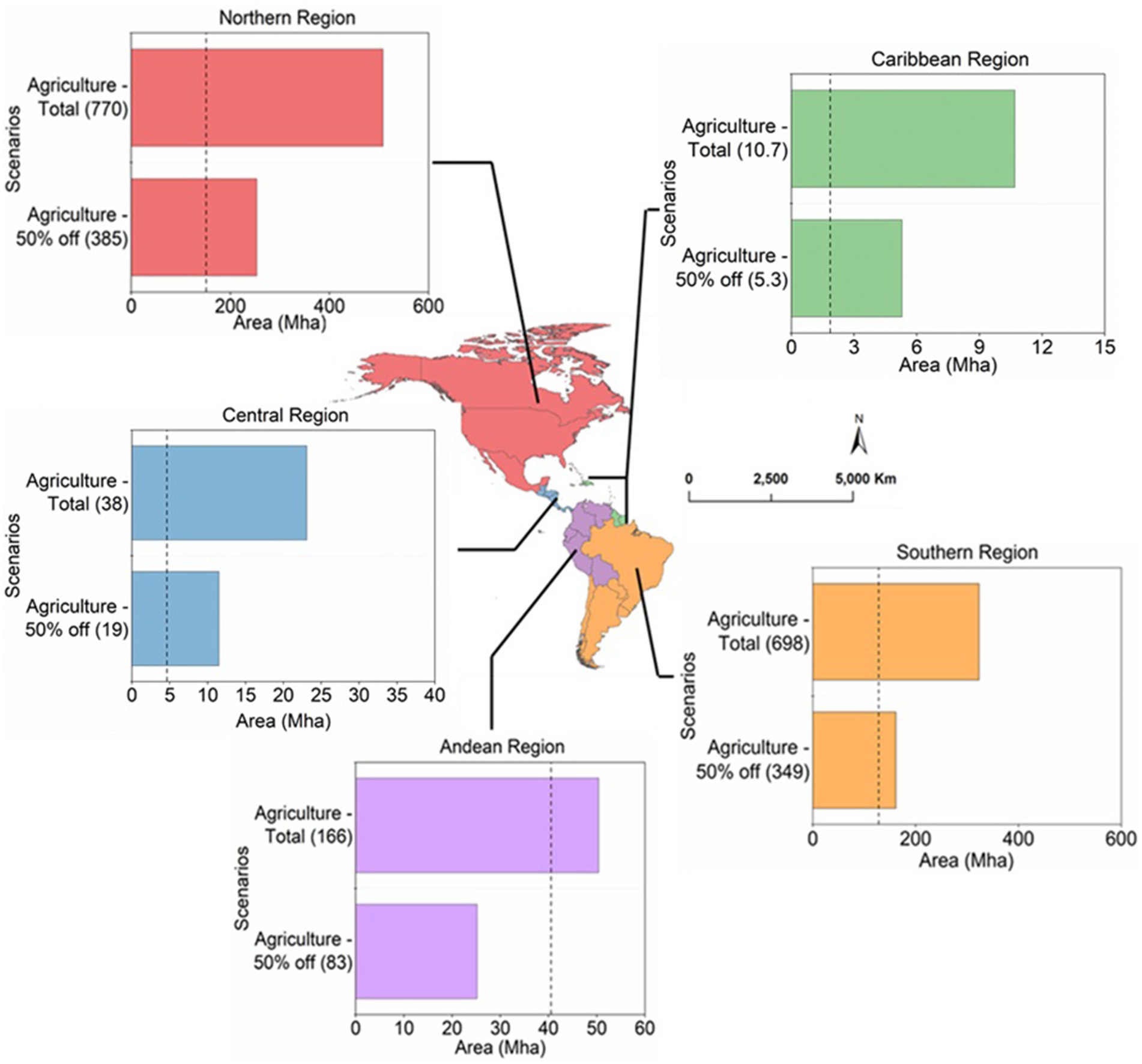
Figure 4. Estimated required area (Mha) to compensate agricultural emissions for each region in the Americas. The 2030 scenario was calculated considering a 50% reduction target for short-term results by the end of the decade (average CO2eq emissions between 2015 and 2019 were used as the baseline and a timespan of 8 years (2022–2030)). The 2050 scenario was calculated considering the neutrality target and a period of 20 years. The required estimated area to compensate for emissions was based on a combination of pasture reclamation, no-tillage, and integrated systems (e.g., agroforestry, silvopastoral, integrated crop-livestock forest systems) expansion. Values in parentheses on the y-axis represent the CO2eq emissions (MtCO2eq year−1) to be compensated and estimated for each scenario. Dashed lines represent the available estimated agricultural area that could be restored through BMPs in each country/region, which was assumed to be 30% of the total agricultural area (FAO, 2015; Gardi et al., 2013; UNCCD, 2019).
4 Discussion
4.1 Data availability on soil C dynamics in the Americas
The systematic literature review showed that despite the large number of studies retrieved in the search, only a small fraction performed field measurements for soil C data evaluation, mostly conducted in the north and south regions (Table 2). This discrepancy suggests that despite SOM-related terms being systematically mentioned in scientific research articles, the fraction of studies that measure it in the field is limited and does not present a good coverage of the whole continent. Indeed, the agricultural area in the Americas is largely represented by the South and North regions (952 Mha; World Bank, 2023), which helps to justify the high concentration of studies in these areas. Nevertheless, despite the lowest individual contribution, Central, Caribbean, and Andean regions sum together 161 Mha of agricultural land (World Bank, 2023), therefore accounting for a significant land proportion. Furthermore, the variability for soil, climate, and agroecosystems management encountered in these regions are considerable high (Alavi-Murillo et al., 2022; Owens et al., 2020), which requires an appropriate sampling density for properly identifying the impact of different management/climate conditions on soil C dynamics.
In addition to the reduced number of studies identified, lack of sampling standardization was also a major limitation observed in the dataset, since only 4.6% (out of the 13 thousand studies retrieved in the search) of the studies measured soil C up to 30 cm soil deep. The IPCC recommendations for soil C evaluation suggests a minimum sampling depth considering the first 30 cm of the soil (IPCC, 2006), since this layer is frequently the most affected one under cropping systems areas and is predicted to account for ~45% of total soil C stocks found in the first 1 m of soil (1,505 Pg of C; Lal, 2018). Moreover, new studies have recently suggested more detailed approaches, emphasizing the need for sampling deeper soil layers (Button et al., 2022; Olson and Al-Kaisi, 2015). The need for more detailed sampling protocols relies on the fact that studies showed that cropping management can affect soil C in deeper layers (Olson and Al-Kaisi, 2015). In the same line, C sequestration in the sub-soil layers have been advocated as a promising strategy for long-term soil C stabilization (Button et al., 2022); since deeper soil layer have a reduced microbial activity and a higher C saturation deficit, therefore favoring C binding onto mineral structures and protection against microbial oxidation (Chenu et al., 2019; Sokol and Bradford, 2019).
The search also suggested that a reduced number of studies focused on BMPs, as indicated by the co-occurrence map of the keywords. The most cited terms were related to SOM and overall processes such as “land use change,” suggesting that this is a largely studied subject. Apart from that, only “no-tillage,” which is a BMP, had a significant contribution/interaction in the map. Other important keywords such as “agroforestry” and “cover crops” appeared but indicated to have a small contribution. This highlights that despite the wide recognition that these practices are great strategies for promoting soil C accrual and restore soil health (Jian et al., 2020; Lange et al., 2015; Nwaogu and Cherubin, 2024; Steinfeld et al., 2023; Yang et al., 2024), the number of research in these topics is still limited. Unlocking the potential of the Americas for improving the sustainability of its agricultural areas depends on obtaining high-quality field data for soil C sequestration strategies. Best management practices (e.g., cover cropping, crop diversification) are suggested to be a key-tool for achieving these goals, and the literature review suggests that this is a path that still needs to be paved.
4.2 Carbon sequestration capacity and the need for expanding best management practices to mitigate agricultural GHG emissions
The calculations exercises present a first attempt to estimate both the capacity of agricultural land from the Americas to sequester C and the necessary area needed to completely offset GHG emissions using only three nature-based solutions or technologies: IP, CA, and IAS. The estimated soil C sequestration potential (13.1 ± 7.1 Pg CO2eq) supports the previous studies (Lal, 2004, 2003; Minasny et al., 2017; Paustian et al., 2016; Smith, 2008), which showed that BMPs are efficient strategies of promoting soil C sequestration and are a potential tool to mitigate the anthropogenic climate change. Adoption of BMPs could sequester up to 8 Pg CO2eq year−1 globally (Paustian et al., 2016) and have a large positive impact on GHG mitigation (IPCC, 2022). Other researchers have estimated a mitigation capacity of 1.5 to 5.3 Pg CO2eq year−1 (Smith, 2008). The estimate presented herein suggests that up to 1.02 (0.66 ± 0.36) Pg CO2eq year−1 would be mitigated in the Americas considering only one-third of the available agricultural area, which represents roughly 12 to 44% of the global estimated capacity according to Smith et al. (2008), and ~ 8% according to Paustian et al. (2016). The given scenario places the agri-food systems of the Americas as a major component to mitigate climate change as well as farmers as a key actor to enhance global food security.
The greatest potential of soil C sequestration observed in southern and northern regions depends on the available agricultural area in the countries of these regions. For the Andean region, however, despite the lower contributions compared to southern and northern regions, the reviewed rates for soil C sequestration are considerably high, especially for the practice of IAS, which also presented high values for this modality (1.16 Mg ha−1 year−1) in the central region. This highlights the potential for soil C sequestration for this region when considering each area unit. In practical terms, these higher rates for soil C sequestration may be explained by two main reasons: (i) the Andean region is comprised of high-altitude area which contribute to promoting soil C accrual (Alavi-Murillo et al., 2022; Segnini et al., 2011); (ii) the studies recovered in the present search for the Andean region assessed mostly agroforestry systems for the IAS modality. Agroforestry systems are generally more complex when compared to other IAS studies that were considered in the search for other regions (e.g., integrated crop-livestock systems). The high complexity of these systems may contribute to increasing soil C sequestration potential, as stated by Steinfeld et al. (2023) and Lange et al. (2015). Once it promotes a greater capacity for C accrual in the soil, this feature is highly interesting in the context of C market initiatives. In general terms, the high rates for soil C sequestration increase the region’s capacity for mitigation of GHG emissions. Despite the smaller contribution of each country when compared to the other regions, Andean, Caribbean, and the Central American countries together contribute up to 23.6% of the total potential, representing 3.1 Pg CO2eq.
The predicted potential was based on C sequestration rates reported in the literature (Table 1), where three different strategies were adopted in proportions that are more feasible to be implemented in the field, i.e., 56% of IP, 24% of CA, and 20% IAS. Due to this conservative scenario, a significant proportion of the agricultural area in the continents (i.e., 915 Mha – 82.4% of the total) would be needed to completely offset agricultural GHG emissions. Prioritizing strategies that are more efficient in promoting soil C sequestration, like IAS (Table 1), could be beneficial for reducing the required area to achieve C neutrality since it could increase the capacity for promoting CO2 removal from the atmosphere. In the same line, combining other approaches not considered in the calculations presented here, such as biochar, could also be beneficial. Biochar has been reported to greatly contribute to soil C sequestration, besides reducing N2O emissions and improving soil health (Beillouin et al., 2023; Kaur et al., 2023; Mukherjee and Lal, 2013). Available meta-analyses indicated increases on soil C levels ranging from 39 to 64% under biochar application (Bai et al., 2019; Beillouin et al., 2023; Chagas et al., 2022). Alongside, other conservation strategies such as reduced tillage (or minimum tillage) also can potentially contribute as a BMP strategy (Jordon et al., 2022; Krauss et al., 2017). The soil C sequestration potential calculations performed here were based on NT practice due to the great data availability (i.e., soil C sequestration rates) in the literature. Nevertheless, adopting other intermediate strategies such as minimum tillage could also be positive, especially in field conditions where management or technical restrictions prevent the application of more robust approaches such as NT.
Furthermore, due to limited data availability, these estimates considered only the potential for soil C sequestration in the soil, and do not include the aboveground C sequestration (e.g., in the trees of IASs) and the potential reduction of GHG emissions from the agricultural sector. The present study also did not include the potential positive impacts on agronomic productivity, soil health, and other benefits (Kopittke et al., 2022). The actions taken for climate change mitigation must involve multiple efforts, which would comprise strategies specifically focused on reducing GHG emissions in the productive chain of the agricultural sector, therefore enhancing the mitigation capacity (Lal, 2022). This could lead to a carbon-positive scenario, where the agri-food systems could mitigate more GHG than they generate. For example, the Global Methane Pledge, launched at COP26, in Glasgow and ratified by 30 countries of the Americas, seeks to establish volunteer actions to reduce 30% of CH4 emissions from 2020 levels until 2030. This target could be achieved using different tools with the potential to reduce GHG emissions allied to soil C sequestration technologies (Johnson et al., 2007; Lal, 2022; Nayak et al., 2015). For example, using additives in ruminants’ diets could reduce enteric CH4 production by ~34% (±15) (MAPA, 2020; Roque et al., 2019; van Wesemael et al., 2019). This management practice can significantly impact the mitigation of GHG emissions from the livestock sector in the Americas, where five (i.e., Brazil, USA, Argentina, Mexico, Uruguay) of the ten largest cattle herds in the world are present (i.e., ~366 million head, per Souza Filho et al., 2019).
Improved grazing management practices could also be beneficial to reducing enteric CH4 production (Souza Filho et al., 2019), besides being an effective strategy for increasing nutrient use efficiency (NUE) (Löw et al., 2020). A greater NUE can be beneficial: (i) to increase forage/crop yield and consequently C accrual, and (ii) to reduce the surplus of nutrients in the soil solution that could be leached or converted into GHG like N2O in the case of N (Venterea et al., 2016). Specifically, for N fertilization, inhibitors can also reduce the production of N2O (e.g., nitrification inhibitors like dicyandiamide - DCD; Liu et al., 2013). Similarly, crop diversification strategies like crop rotation could improve nutrient cycling, consequently leading to better nutrient usage and reducing the demand for external inputs (Lehman et al., 2017). In more biodiverse cropping systems, the inclusion of cover crops associated with no-till can significantly improve soil physical and biological quality (Blanco-Canqui and Ruis, 2020; Lange et al., 2015; Williams et al., 2020). Soil with better physical conditions can increase air and water diffusion, thus reducing the occurrence of anaerobic sites that are prone to denitrification or methanogenesis processes (Blanco-Canqui and Ruis, 2020). Biologically, a more diverse microbial community can prevent the dominance of specific groups that can be more efficient in producing N2O and CH4 (Cezar et al., 2021). Lastly, maintaining healthy soil could increase CO2 capture in living biomass and, therefore, enhance C sequestration.
4.3 Study limitations
The systematic literature review indicated that despite BMPs-related terms are frequently mentioned in the literature, the number of studies that assess soil C under these strategies is reduced. The review also indicated the urgency for standardization of the sampling depth, which should minimally include the top 30 cm layer and preferentially reach 100 cm depth (at least). Evidence suggest that assessing soil C considering only the utmost soil layer may not represent soil C dynamics properly, as the impact of cropping systems can affect deeper layers (Smith et al., 2020). A significant gap on soil C data was observed specifically for Central, Caribbean, and Andean regions. The low availability of data for these regions, combined with the lack of technical criteria, prevented the extraction of C accumulation rates via meta-analysis (Bown and Sutton, 2010; Hansen et al., 2022). Therefore, the scenarios were generated from an extensive literature review focused on identifying C sequestration rates in regions with data availability (Table 1 and Supplementary Figure S4). Most of the extracted rates were obtained from meta-analysis studies per se, as for North (Conant et al., 2017; Nicoloso and Rice, 2021) and South (Viglizzo et al., 2019; Maia et al., 2022; Frazão et al., 2023) America. But for other regions (Central, Caribbean, and Andean), isolated studies were adopted. The use of individual studies for Central, Caribbean, and Andean regions is a shortcoming encountered in the analysis, which unfortunately, represents a bias in the reported data (Lin and Chu, 2018; Rothstein et al., 2005).
Another challenge was identifying the real extension and the most promising location (within each landscape/region) of degraded agricultural areas where implementation of BMPs would be more technical and socio-economically feasible for increasing soil C sequestration. Due to a lack of data, these estimates are restricted to the extension of 30% of the available agricultural area, an average estimate of the degraded land proportion (see Materials and Methods, section 2.2.1). It is uncertain whether this value is below or above the true extent. Further limitations associated with this study are related to the poor availability of soil C data across the region, which would be necessary to account for soil C saturation status. As expected, the reported soil C sequestration rates (Table 1) may not be fully achieved depending on the current soil C levels. The absence of these data prevents obtaining precise estimates of the C sequestration potential, which is an essential step towards establishing public policies aimed at mitigating GHG emissions through agriculture for addressing anthropogenic climate change. Despite this, we consider the results presented here valuable and timely because it provides an order of magnitude of the mitigation potential with the expansion of BMPs in the Americas based on evidence. Such approach can be improved at the pace that more information is generated for the region, enhancing the quality of the analysis.
5 Conclusion
This study provides a first assessment of the current knowledge regarding the research on SOC-related subjects in the Americas. Overall, the results demonstrate an urgent need for experimental standardization in soil C research, with the establishment of a minimal sampling depth; and a significant territorial blind spot in the continent. Our current estimate of soil C sequestration potential may be refined in the future as more C data become available in these regions and as the accuracy of land use and soil degradation in the agroecosystems improves. Despite that, these estimates reveal that a considerable amount of C (i.e., 13.1 Pg CO2eq over a 20-year period) would be sequestered under the expansion of pasture reclamation strategies, no-tillage system, and integrated agricultural systems across 30% of the agricultural area (334 Mha). The effectiveness of these technologies would offset roughly 39% of the GHG emissions originating from the agricultural sector. An additional area of 581 Mha would be needed to be managed under those BMP’s to compensate the total emissions originating from the sector to achieve net zero targets (mainly adopted by countries for 2050). The need to upscale the adoption of such technologies depends on the current level of existent across the region. The data presented in Table 1 could be used as a reference for establishing national policies aimed at improving soil C sequestration by using BMPs, such as the ABC+ Plan/RenovAgro in Brazil (Brazil, 2023). Also, these data provides reference numbers for soil C sequestration based on published information, making the Americas’ region a Tier 2 level considering the IPCC methodology for national GHG inventories (IPCC, 2006). Moreover, according to the IPCC Special Report on climate change, desertification, land degradation, sustainable land management, food security, and greenhouse gas fluxes in terrestrial ecosystems (IPCC, 2019b), the agriculture sector of the region is a global player in positive terms of GHG mitigation, adaptation, food security and combat to desertification and land degradation. This is an outstanding achievement for the region, and for the climate change and food security agenda. Finally, it also represents a major step in supporting countries to advance with the adoption of climate-smart agriculture technologies towards the net-zero agenda by the middle of the century, a relevant accomplishment to turn down the climate crisis.
Data availability statement
The original contributions presented in the study are included in the article/Supplementary material, further inquiries can be directed to the corresponding author.
Author contributions
CC: Conceptualization, Data curation, Formal analysis, Funding acquisition, Investigation, Methodology, Project administration, Resources, Supervision, Validation, Visualization, Writing – original draft, Writing – review & editing. MRC: Conceptualization, Data curation, Formal analysis, Funding acquisition, Investigation, Methodology, Project administration, Resources, Supervision, Validation, Visualization, Writing – original draft, Writing – review & editing. JV: Conceptualization, Data curation, Formal analysis, Investigation, Methodology, Supervision, Validation, Visualization, Writing – original draft, Writing – review & editing. JL: Conceptualization, Data curation, Formal analysis, Investigation, Methodology, Supervision, Validation, Visualization, Writing – original draft, Writing – review & editing. MLC: Conceptualization, Data curation, Formal analysis, Investigation, Methodology, Supervision, Validation, Visualization, Writing – original draft, Writing – review & editing. FV: Conceptualization, Data curation, Formal analysis, Funding acquisition, Investigation, Methodology, Project administration, Resources, Supervision, Validation, Visualization, Writing – original draft, Writing – review & editing. FC: Conceptualization, Data curation, Formal analysis, Funding acquisition, Investigation, Methodology, Project administration, Resources, Supervision, Validation, Visualization, Writing – original draft, Writing – review & editing. MI: Conceptualization, Data curation, Formal analysis, Funding acquisition, Investigation, Methodology, Project administration, Resources, Supervision, Validation, Visualization, Writing – original draft, Writing – review & editing. RL: Conceptualization, Data curation, Formal analysis, Funding acquisition, Investigation, Methodology, Project administration, Resources, Supervision, Validation, Visualization, Writing – original draft, Writing – review & editing.
Funding
The author(s) declare that no financial support was received for the research, authorship, and/or publication of this article.
Acknowledgments
We would like to thank the Inter-American Institute for Cooperation on Agriculture (IICA) and all the public and private partners supporting the Living Soils of the Americas initiative, especially Director General Manuel Otero for his leadership and guidance. In addition, we thank the support of the São Paulo Research Foundation (FAPESP) for providing scholarships (JV - FAPESP grant #2023/09533-3; JL - FAPESP grant #2021/14989-0; MLC - FAPESP grant #2022/13531-3) and funding through the Center for Carbon Research in Tropical Agriculture (CCARBON) – FAPESP grant #2021/10573-4. MRC thanks the CNPq for his Research Productivity Fellowship (grant #311787/2021-5).
Conflict of interest
The authors declare that the research was conducted in the absence of any commercial or financial relationships that could be construed as a potential conflict of interest.
Publisher’s note
All claims expressed in this article are solely those of the authors and do not necessarily represent those of their affiliated organizations, or those of the publisher, the editors and the reviewers. Any product that may be evaluated in this article, or claim that may be made by its manufacturer, is not guaranteed or endorsed by the publisher.
Supplementary material
The Supplementary material for this article can be found online at: https://www.frontiersin.org/articles/10.3389/fsufs.2024.1481005/full#supplementary-material
Abbreviations
Best management practices, BMPs; Greenhouse gas, GHG; Soil organic matter, SOM; Conservation agriculture, CA; Integrated agroforestry systems, IAS; Improved pasture, IP; Soil organic carbon, SOC; Intergovernmental Panel on Climate Change, IPCC; Nutrient use efficiency, NUE; Dicyandiamide, DCD; Integrated crop-livestock, ICL; Integrated crop-forestry, ICF; integrated crop-livestock-forestry, ICLF.
References
Abohassan, R. A. (2004). Carbon dynamics in a temperate agroforestry system in southern Ontario, Canada. Guelph (ON): University of Guelph.
Alavi-Murillo, G., Diels, J., Gilles, J., and Willems, P. (2022). Soil organic carbon in Andean high-mountain ecosystems: importance, challenges, and opportunities for carbon sequestration. Reg. Environ. Chang. 22:128. doi: 10.1007/s10113-022-01980-6
Amelung, W., Bossio, D., Vries, W., Kögel-Knabner, I., Lehmann, J., Amundson, R., et al. (2020). Towards a global-scale soil climate mitigation strategy. Nat. Commun. 11:5427. doi: 10.1038/s41467-020-18887-7
Bai, X., Huang, Y., Ren, W., Coyne, M., Jacinthe, P. A., Tao, B., et al. (2019). Responses of soil carbon sequestration to climate-smart agriculture practices: a meta-analysis. Glob. Chang. Biol. 25, 2591–2606. doi: 10.1111/gcb.14658
Beillouin, D., Corbeels, M., Demenois, J., Berre, D., Boyer, A., Fallot, A., et al. (2023). A global meta-analysis of soil organic carbon in the Anthropocene. Nat. Commun. 14:3700. doi: 10.1038/s41467-023-39338-z
Berner, R. A. (2003). The long-term carbon cycle, fossil fuels and atmospheric composition. Nature 426, 323–326. doi: 10.1038/nature02131
Blanco-Canqui, H., and Ruis, S. J. (2020). Cover crop impacts on soil physical properties: a review. Soil Sci. Soc. Am. J. 84, 1527–1576. doi: 10.1002/saj2.20129
Bossio, D. A., Cook-Patton, S. C., Ellis, P. W., Fargione, J., Sanderman, J., Smith, P., et al. (2020). The role of soil carbon in natural climate solutions. Nat. Sustain. 3, 391–398. doi: 10.1038/s41893-020-0491-z
Bown, M. J., and Sutton, A. J. (2010). Quality control in systematic reviews and Meta-analyses. Eur. J. Vasc. Endovasc. Surg. 40, 669–677. doi: 10.1016/j.ejvs.2010.07.011
Brantley, S. L., Goldhaber, M. B., and Ragnarsdottir, K. V. (2007). Crossing disciplines and scales to understand the critical zone. Elements 3, 307–314. doi: 10.2113/gselements.3.5.307
Brazil. (2023). Agricultura de Baixa Emissão de Carbono. Available at: https://www.gov.br/agricultura/pt-br/assuntos/sustentabilidade/agricultura-de-baixa-emissao-de-carbono (Accessed September 26, 2024).
Button, E. S., Pett-Ridge, J., Murphy, D. V., Kuzyakov, Y., Chadwick, D. R., and Jones, D. L. (2022). Deep-C storage: biological, chemical and physical strategies to enhance carbon stocks in agricultural subsoils. Soil Biol. Biochem. 170:108697. doi: 10.1016/j.soilbio.2022.108697
Cezar, R. M., Vezzani, F. M., Kaschuk, G., Balsanelli, E., Souza, E. M., Vargas, L. K., et al. (2021). Crop rotation reduces the frequency of anaerobic soil bacteria in red latosol of Brazil. Braz. J. Microbiol. 52, 2169–2177. doi: 10.1007/s42770-021-00578-0
Chagas, J. K. M., Figueiredo, C. C. D., and Ramos, M. L. G. (2022). Biochar increases soil carbon pools: evidence from a global meta-analysis. J. Environ. Manag. 305:114403. doi: 10.1016/j.jenvman.2021.114403
Chatterjee, N., Nair, P. K. R., Nair, V. D., Bhattacharjee, A., Virginio Filho, E. M., Muschler, R. G., et al. (2020). Do coffee agroforestry systems always improve soil carbon stocks deeper in the soil?-a case study from Turrialba. Costa Rica. Forests 11:49. doi: 10.3390/f11010049
Chenu, C., Angers, D. A., Barré, P., Derrien, D., Arrouays, D., and Balesdent, J. (2019). Increasing organic stocks in agricultural soils: knowledge gaps and potential innovations. Soil Tillage Res. 188, 41–52. doi: 10.1016/j.still.2018.04.011
Conant, R. T., Cerri, C. E. P., Osborne, B. B., and Paustian, K. (2017). Grassland management impacts on soil carbon stocks: a new synthesis. Ecol. Appl. 27, 662–668. doi: 10.1002/eap.1473
Crippa, M., Solazzo, E., Guizzardi, D., Monforti-Ferrario, F., Tubiello, F. N., and Leip, A. (2021). Food systems are responsible for a third of global anthropogenic GHG emissions. Nat. Food 2, 198–209. doi: 10.1038/s43016-021-00225-9
Doetterl, S., Stevens, A., Six, J., Merckx, R., Van Oost, K., Pinto, M. C., et al. (2015). Soil carbon storage controlled by interactions between geochemistry and climate. Nat. Geosci. 8, 780–783. doi: 10.1038/ngeo2516
Dondini, M., Martin, M., Camillis, C., Uwizeye, A., Soussana, J. F., Robinson, T., et al. (2023). Global assessment of soil carbon in grasslands: From current stock estimates to sequestration potential. Rome, Italy: FAO.
Dube, F., Thevathasan, N. V., Zagal, E., Gordon, A. M., Stolpe, N. B., and Espinosa, M. (2011). “Carbon sequestration potential of Silvopastoral and other land use Systems in the Chilean Patagonia” in Carbon sequestration potential of agroforestry systems: Opportunities and challenges. eds. B. M. Kumar and P. K. R. Nair (Dordrecht: Springer Netherlands), 101–127.
Espinoza-Domínguez, W., Krishnamurthy, L., Vázquez-Alarcón, A., and Torres-Rivera, A. (2012). Almacén de carbono en sistemas agroflorestales con café. Rev. Chapingo Ser. Cienc. For. Ambiente 18, 57–70. doi: 10.5154/r.rchscfa.2011.04.030
Frazão, L. A., Ferreira, E. A., Oliveira, W. R., Freitas, I. C., Cerri, C. E., Vilela, J. M., et al. (2023). Sustainable intensification of agriculture and livestock production in Brazil: A meta-analysis of soil C changes in integrated systems (No. EGU23-1998). Presented at the EGU23, Copernicus Meetings.
Gardi, C., Angelini, M., Comerma, J., Gaistardo, C. C., Rojas, A. E., Jones, A., et al. (2013). Atlas de suelos de America Latina y el Caribe. Luxembourg: Oficina de Publicaciones de la Union Europea.
Hansen, C., Steinmetz, H., and Block, J. (2022). How to conduct a meta-analysis in eight steps: a practical guide. Manag. Rev. Q. 72, 1–19. doi: 10.1007/s11301-021-00247-4
Herrero, M., Havlík, P., Valin, H., Notenbaert, A., Rufino, M. C., Thornton, P. K., et al. (2013). Biomass use, production, feed efficiencies, and greenhouse gas emissions from global livestock systems. Proc. Natl. Acad. Sci. USA 110, 20888–20893. doi: 10.1073/pnas.1308149110
Hong, C., Burney, J. A., Pongratz, J., Nabel, J. E. M. S., Mueller, N. D., Jackson, R. B., et al. (2021). Global and regional drivers of land-use emissions in 1961–2017. Nature 589, 554–561. doi: 10.1038/s41586-020-03138-y
IPBES (2019). Summary for policymakers of the global assessment report on biodiversity and ecosystem services of the intergovernmental science-policy platform on biodiversity and ecosystem services. Popul. Dev. Rev. 45, 680–681. doi: 10.1111/padr.12283
IPCC. (2006). IPCC guidelines for National Greenhouse gas Inventories, prepared by the National Greenhouse gas Inventories Programme.
IPCC (2019a). Technical summary, in: Climate change and land: IPCC special report on climate change, desertification, land degradation, sustainable land management, food security, and greenhouse gas fluxes in terrestrial ecosystems. Cambridge: Cambridge University Press.
IPCC (2019b). Summary for policymakers. In: Climate change and land: An IPCC special report on climate change, desertification, land degradation, sustainable land management, food security, and greenhouse gas fluxes in terrestrial ecosystems. Cambridge: Cambridge University Press.
IPCC (2022). Climate change and land: IPCC special report on climate change, desertification, land degradation, sustainable land management, food security, and greenhouse gas fluxes in terrestrial ecosystems. Cambridge: Cambridge University Press.
Jian, J., Du, X., Reiter, M. S., and Stewart, R. D. (2020). A meta-analysis of global cropland soil carbon changes due to cover cropping. Soil Biol. Biochem. 143:107735. doi: 10.1016/j.soilbio.2020.107735
Johnson, J. M. F., Franzluebbers, A. J., Weyers, S. L., and Reicosky, D. C. (2007). Agricultural opportunities to mitigate greenhouse gas emissions. Environ. Pollut. 150, 107–124. doi: 10.1016/j.envpol.2007.06.030
Jordon, M. W., Smith, P., Long, P. R., Bürkner, P. C., Petrokofsky, G., and Willis, K. J. (2022). Can regenerative agriculture increase national soil carbon stocks? Simulated country-scale adoption of reduced tillage, cover cropping, and ley-arable integration using Roth C. Sci. Total Environ. 825:153955. doi: 10.1016/j.scitotenv.2022.153955
Karakurt, I., Aydin, G., and Aydiner, K. (2012). Sources and mitigation of methane emissions by sectors: a critical review. Renew. Energy 39, 40–48. doi: 10.1016/j.renene.2011.09.006
Kaur, N., Kieffer, C., Ren, W., and Hui, D. (2023). How much is soil nitrous oxide emission reduced with biochar application? An evaluation of meta-analyses. GCB Bioenergy 15, 24–37. doi: 10.1111/gcbb.13003
Kopittke, P. M., Berhe, A. A., Carrillo, Y., Cavagnaro, T. R., Chen, D., Chen, Q. L., et al. (2022). Ensuring planetary survival: the centrality of organic carbon in balancing the multifunctional nature of soils. Crit. Rev. Environ. Sci. Technol. 52, 4308–4324. doi: 10.1080/10643389.2021.2024484
Kraamwinkel, C. T., Beaulieu, A., Dias, T., and Howison, R. A. (2021). Planetary limits to soil degradation. Commun. Earth Environ. 2:249. doi: 10.1038/s43247-021-00323-3
Krauss, M., Ruser, R., Müller, T., Hansen, S., Mäder, P., and Gattinger, A. (2017). Impact of reduced tillage on greenhouse gas emissions and soil carbon stocks in an organic grass-clover ley - winter wheat cropping sequence. Agric. Ecosyst. Environ. 239, 324–333. doi: 10.1016/j.agee.2017.01.029
Lal, R. (2003). Global potential of soil carbon sequestration to mitigate the greenhouse effect. Crit. Rev. Plant Sci. 22, 151–184. doi: 10.1080/713610854
Lal, R. (2004). Soil carbon sequestration to mitigate climate change. Geoderma 123, 1–22. doi: 10.1016/j.geoderma.2004.01.032
Lal, R. (2005). Forest soils and carbon sequestration. For. Ecol. Manag. 220, 242–258. doi: 10.1016/j.foreco.2005.08.015
Lal, R. (2015). Restoring soil quality to mitigate soil degradation. Sustain. For. 7, 5875–5895. doi: 10.3390/su7055875
Lal, R. (2018). Digging deeper: a holistic perspective of factors affecting soil organic carbon sequestration in agroecosystems. Glob. Chang. Biol. 24, 3285–3301. doi: 10.1111/gcb.14054
Lal, R. (2022). Reducing carbon footprints of agriculture and food systems. Carbon. Footpr. 1:3. doi: 10.20517/cf.2022.05
Lal, R., Bouma, J., Brevik, E., Dawson, L., Field, D. J., and Glaser, B. (2021). Soils and sustainable development goals of the United Nations: an International Union of Soil Sciences perspective. Geoderma Reg. 25:e00398. doi: 10.1016/j.geodrs.2021.e00398
Landholm, D. M., Pradhan, P., Wegmann, P., Sánchez, M. A. R., Salazar, J. C. S., and Kropp, J. P. (2019). Reducing deforestation and improving livestock productivity: greenhouse gas mitigation potential of silvopastoral systems in Caquetá. Environ. Res. Lett. 14:114007. doi: 10.1088/1748-9326/ab3db6
Lange, M., Eisenhauer, N., Sierra, C. A., Bessler, H., Engels, C., Griffiths, R. I., et al. (2015). Plant diversity increases soil microbial activity and soil carbon storage. Nat. Commun. 6:6707. doi: 10.1038/ncomms7707
Le Quéré, C., Raupach, M. R., Canadell, J. G., Marland, G., Bopp, L., Ciais, P., et al. (2009). Trends in the sources and sinks of carbon dioxide. Nat. Geosci. 2, 831–836. doi: 10.1038/ngeo689
Lehman, R. M., Osborne, S. L., and Duke, S. E. (2017). Diversified no-till crop rotation reduces nitrous oxide emissions, increases soybean yields, and promotes soil carbon accrual. Soil Sci. Soc. Am. J. 81, 76–83. doi: 10.2136/sssaj2016.01.0021
Li, J., Pei, J., Liu, J., Wu, J., Li, B., Fang, C., et al. (2021). Spatiotemporal variability of fire effects on soil carbon and nitrogen: a global meta-analysis. Glob. Change Biol. 27, 4196–4206. doi: 10.1111/gcb.15742
Lin, L., and Chu, H. (2018). Quantifying publication bias in meta-analysis. Biometrics 74, 785–794. doi: 10.1111/biom.12817
Liu, C., Wang, K., and Zheng, X. (2013). Effects of nitrification inhibitors (DCD and DMPP) on nitrous oxide emission, crop yield and nitrogen uptake in a wheat–maize cropping system. Biogeosciences 10, 2427–2437. doi: 10.5194/bg-10-2427-2013
Löw, P., Karatay, Y. N., and Osterburg, B. (2020). Nitrogen use efficiency on dairy farms with different grazing systems in northwestern Germany. Environ. Res. Commun. 2:105002. doi: 10.1088/2515-7620/abc098
Maia, S. M. F., Medeiros, A. S., Santos, T. C., Lyra, G. B., Lal, R., Assad, E. D., et al. (2022). Potential of no-till agriculture as a nature-based solution for climate-change mitigation in Brazil. Soil Tillage Res. 220:105368. doi: 10.1016/j.still.2022.105368
Manzatto, C. V., Araujo, L. S., Assad, E. D., Sampaio, F. G., Sotta, E. D., Vicente, L. E., et al. (2020). Mitigação das emissões de Gases de Efeitos Estufa pela adoção das tecnologias do Plano ABC: estimativas parciais. Jaguariúna, SP: Embrapa Meio Ambiente.
MAPA (2020). Coletânea de fatores de emissão e remoção de gases de efeito estufa da pecuária brasileira. Pecuária e Abastecimento: Ministério da Agricultura.
Mena-Mosquera, V. E., and Andrade, H. J. (2021). Valuation of carbon sequestration and storage ecosystem Services in a Tropical Moist Forest of Chocó, Colombia. Floresta Ambient. 28:e20200088. doi: 10.1590/2179-8087-FLORAM-2020-0088
Minasny, B., Malone, B. P., McBratney, A. B., Angers, D. A., Arrouays, D., Chambers, A., et al. (2017). Soil carbon 4 per mille. Geoderma 292, 59–86. doi: 10.1016/j.geoderma.2017.01.002
Mosquera, O., Buurman, P., Ramirez, B. L., and Amezquita, M. C. (2012). Carbon stocks and dynamics under improved tropical pasture and silvopastoral systems in Colombian Amazonia. Geoderma 189-190, 81–86. doi: 10.1016/j.geoderma.2012.04.022
Mukherjee, A., and Lal, R. (2013). Biochar impacts on soil physical properties and greenhouse gas emissions. Agronomy 3, 313–339. doi: 10.3390/agronomy3020313
Nayak, D., Saetnan, E., Cheng, K., Wang, W., Koslowski, F., Cheng, Y. F., et al. (2015). Management opportunities to mitigate greenhouse gas emissions from Chinese agriculture. Agric. Ecosyst. Environ. 209, 108–124. doi: 10.1016/j.agee.2015.04.035
Nicoloso, R. S., and Rice, C. W. (2021). Intensification of no-till agricultural systems: an opportunity for carbon sequestration. Soil Sci. Soc. Am. J. 85, 1395–1409. doi: 10.1002/saj2.20260
Nwaogu, C., and Cherubin, M. R. (2024). “Integrated agricultural systems: the 21st century nature-based solution for resolving the global FEEES challenges” in Advances in agronomy. ed. D. L. Sparks (Cambridge, MA: Academic Press Inc), 1–73.
Oelbermann, M., Voroney, R. P., Thevathasan, N. V., Gordon, A. M., Kass, D. C. L., and Schlönvoigt, A. M. (2006). Soil carbon dynamics and residue stabilization in a Costa Rican and southern Canadian alley cropping system. Agrofor. Syst. 68, 27–36. doi: 10.1007/s10457-005-5963-7
Olaya-Montes, A., Llanos-Cabrera, M. P., Cherubin, M. R., Herrera-Valencia, W., Ortiz-Morea, F. A., and Silva-Olaya, A. M. (2021). Restoring soil carbon and chemical properties through silvopastoral adoption in the Colombian Amazon region. Land Degrad. Dev. 32, 3720–3730. doi: 10.1002/ldr.3832
Oliveira, D. C., Maia, S. M. F., Freitas, R. C. A., and Cerri, C. E. P. (2022). Changes in soil carbon and soil carbon sequestration potential under different types of pasture management in Brazil. Reg. Environ. Chang. 22:87. doi: 10.1007/s10113-022-01945-9
Olson, K. R., and Al-Kaisi, M. M. (2015). The importance of soil sampling depth for accurate account of soil organic carbon sequestration, storage, retention and loss. Catena 125, 33–37. doi: 10.1016/j.catena.2014.10.004
Owens, P. R., Dorantes, M. J., Fuentes, B. A., Libohova, Z., and Schmidt, A. (2020). Taking digital soil mapping to the field: lessons learned from the water smart agriculture soil mapping project in Central America. Geoderma Reg. 22:e00285. doi: 10.1016/j.geodrs.2020.e00285
Paustian, K., Lehmann, J., Ogle, S., Reay, D., Robertson, G. P., and Smith, P. (2016). Climate-smart soils. Nature 532, 49–57. doi: 10.1038/nature17174
Peichl, M., Thevathasan, N. V., Gordon, A. M., Huss, J., and Abohassan, R. A. (2006). Carbon sequestration potentials in temperate tree-based intercropping systems, southern Ontario, Canada. Agrofor. Syst. 66, 243–257. doi: 10.1007/s10457-005-0361-8
Pereira, P., Bogunovic, I., Muñoz-Rojas, M., and Brevik, E. C. (2018). Soil ecosystem services, sustainability, valuation and management. Curr. Opin. Environ. Sci. Health 5, 7–13. doi: 10.1016/j.coesh.2017.12.003
Picasso, V. D., Modernel, P. D., Becoña, G., Salvo, L., Gutiérrez, L., and Astigarraga, L. (2014). Sustainability of meat production beyond carbon footprint: a synthesis of case studies from grazing systems in Uruguay. Meat Sci. 98, 346–354. doi: 10.1016/j.meatsci.2014.07.005
Pretty, J., and Ball, A. (2001). Agricultural influences on carbon emissions and sequestration: A review of evidence and the emerging trading options. Wivenhoe Park: University of Essex.
Roque, B. M., Salwen, J. K., Kinley, R., and Kebreab, E. (2019). Inclusion of Asparagopsis armata in lactating dairy cows’ diet reduces enteric methane emission by over 50 percent. J. Clean. Prod. 234, 132–138. doi: 10.1016/j.jclepro.2019.06.193
Rothstein, H. R., Sutton, A. J., and Borenstein, M. (2005). “Publication Bias in Meta-analysis” in Publication Bias in Meta-analysis. eds. H. R. Rothstein, A. J. Sutton, and M. Borenstein (Hoboken, NJ: Wiley), 1–7.
Sá, J. C. M., Lal, R., Cerri, C. C., Lorenz, K., Hungria, M., and Carvalho, P. C. F. (2017). Low-carbon agriculture in South America to mitigate global climate change and advance food security. Environ. Int. 98, 102–112. doi: 10.1016/j.envint.2016.10.020
Salazar, O., Casanova, M., and Kätterer, T. (2011). The impact of agroforestry combined with water harvesting on soil carbon and nitrogen stocks in Central Chile evaluated using the ICBM/N model. Agric. Ecosyst. Environ. 140, 123–136. doi: 10.1016/j.agee.2010.11.019
Segnini, A., Posadas, A., Quiroz, R., Milori, D. M. B. P., Vaz, C. M. P., and Martin-Neto, L. (2011). Soil carbon stocks and stability across an altitudinal gradient in southern Peru. J. Soil Water Conserv. 66, 213–220. doi: 10.2489/jswc.66.4.213
Sharrow, S. H., and Ismail, S. (2004). Carbon and nitrogen storage in agroforests, tree plantations, and pastures in western Oregon. USA. Agrofor. Syst. 60, 123–130. doi: 10.1023/B:AGFO.0000013267.87896.41
Silva-Parra, A., Trujillo-González, J. M., and Brevik, E. C. (2021). Greenhouse gas balance and mitigation potential of agricultural systems in Colombia: a systematic analysis. Greenh. Gases Sci. Technol. 11, 554–572. doi: 10.1002/ghg.2066
Smith, P. (2008). Land use change and soil organic carbon dynamics. Nutr. Cycl. Agroecosyst. 81, 169–178. doi: 10.1007/s10705-007-9138-y
Smith, P., Bustamante, M., Ahammad, H., Clark, H., Dong, H., Elsiddig, E. A., et al. (2014). “Agriculture, forestry and other land use (AFOLU)” in Climate change 2014: Mitigation of climate change. Contribution of working group III to the fifth assessment report of the intergovernmental panel on climate change (Cambridge: Cambridge University Press).
Smith, P., Martino, D., Cai, Z., Gwary, D., Janzen, H., Kumar, P., et al. (2008). Greenhouse gas mitigation in agriculture. Philos. Trans. R. Soc. B Biol. Sci. 363, 789–813. doi: 10.1098/rstb.2007.2184
Smith, P., Soussana, J. F., Angers, D., Schipper, L., Chenu, C., Rasse, D. P., et al. (2020). How to measure, report and verify soil carbon change to realize the potential of soil carbon sequestration for atmospheric greenhouse gas removal. Glob. Change Biol. 26, 219–241. doi: 10.1111/gcb.14815
Snyder, C. S., Bruulsema, T. W., Jensen, T. L., and Fixen, P. E. (2009). Review of greenhouse gas emissions from crop production systems and fertilizer management effects. Agric. Ecosyst. Environ. 133, 247–266. doi: 10.1016/j.agee.2009.04.021
Sokol, N. W., and Bradford, M. A. (2019). Microbial formation of stable soil carbon is more efficient from belowground than aboveground input. Nat. Geosci. 12, 46–53. doi: 10.1038/s41561-018-0258-6
Souza Filho, W., Nunes, P. A. A., Barro, R. S., Kunrath, T. R., de Almeida, G. M., Genro, T. C. M., et al. (2019). Mitigation of enteric methane emissions through pasture management in integrated crop-livestock systems: trade-offs between animal performance and environmental impacts. J. Clean. Prod. 213, 968–975. doi: 10.1016/j.jclepro.2018.12.245
Stavi, I., and Lal, R. (2013). Agriculture and greenhouse gases, a common tragedy: a review. Agron. Sustain. Dev. 33, 275–289. doi: 10.1007/s13593-012-0110-0
Steinbach, H. S., and Alvarez, R. (2006). Changes in soil organic carbon contents and nitrous oxide emissions after introduction of no-till in Pampean agroecosystems. J. Environ. Qual. 35, 3–13. doi: 10.2134/jeq2005.0050
Steinfeld, J. P., Bianchi, J. J. A., Locatelli, J. L., Rizzo, R., Resende, M. E. B., Ballester, M. V. R., et al. (2023). Increasing complexity of agroforestry systems benefits nutrient cycling and mineral-associated organic carbon storage, in South-Eastern Brazil. Geoderma 440:116726. doi: 10.1016/j.geoderma.2023.116726
Stewart, C. E., Paustian, K., Conant, R. T., Plante, A. F., and Six, J. (2007). Soil carbon saturation: concept, evidence and evaluation. Biogeochemistry 86, 19–31. doi: 10.1007/s10533-007-9140-0
Thevathasan, N. V. (1998). Nitrogen dynamics and other interactions in tree-cereal intercropping systems in southern Ontario. Guelph, ON: University of Guelph.
UNCCD. (2019). Proportion of degraded land over the total land area. Available at: https://data.unccd.int/land-degradation?grouping=UNCCD (Accessed July 15, 2024).
UNEP (1992). Proceedings of the ad-hoc expert group meeting to discuss global soil databases and appraisal of GLASOD/SOTER. Nairobi: UNEP.
van Wesemael, D., Vandaele, L., Ampe, B., Cattrysse, H., Duval, S., Kindermann, M., et al. (2019). Reducing enteric methane emissions from dairy cattle: two ways to supplement 3-nitrooxypropanol. J. Dairy Sci. 102, 1780–1787. doi: 10.3168/jds.2018-14534
Venterea, R. T., Coulter, J. A., and Dolan, M. S. (2016). Evaluation of intensive “4R” strategies for decreasing nitrous oxide emissions and nitrogen surplus in Rainfed corn. J. Environ. Qual. 45, 1186–1195. doi: 10.2134/jeq2016.01.0024
Viglizzo, E. F., Ricard, M. F., Taboada, M. A., and Vázquez-Amábile, G. (2019). Reassessing the role of grazing lands in carbon-balance estimations: Meta-analysis and review. Sci. Total Environ. 661, 531–542. doi: 10.1016/j.scitotenv.2019.01.130
Williams, H., Colombi, T., and Keller, T. (2020). The influence of soil management on soil health: an on-farm study in southern Sweden. Geoderma 360:114010. doi: 10.1016/j.geoderma.2019.114010
Wollenberg, E., Richards, M., Smith, P., Havlík, P., Obersteiner, M., Tubiello, F. N., et al. (2016). Reducing emissions from agriculture to meet the 2 °C target. Glob. Change Biol. 22, 3859–3864. doi: 10.1111/gcb.13340
World Bank. (2023). Data from: Agriculture land (% of land area). Available at: https://data.worldbank.org/indicator/AG.LND.AGRI.K2 (Accessed June 12, 2024).
Keywords: soil C sequestration, climate mitigation, greenhouse gas, agriculture, climate adaptation, soil health, soil organic matter, food systems
Citation: Cerri CEP, Cherubin MR, Villela JM, Locatelli JL, Carvalho ML, Villarreal F, de Castro Mello FF, Ibrahim MA and Lal R (2024) Carbon farming in the living soils of the Americas. Front. Sustain. Food Syst. 8:1481005. doi: 10.3389/fsufs.2024.1481005
Edited by:
Edward Wilczewski, Bydgoszcz University of Science and Technology, PolandReviewed by:
Serkan Ates, Oregon State University, United StatesBorisĐurđević Durdevic, University of Osijek, Croatia
Copyright © 2024 Cerri, Cherubin, Villela, Locatelli, Carvalho, Villarreal, de Castro Mello, Ibrahim and Lal. This is an open-access article distributed under the terms of the Creative Commons Attribution License (CC BY). The use, distribution or reproduction in other forums is permitted, provided the original author(s) and the copyright owner(s) are credited and that the original publication in this journal is cited, in accordance with accepted academic practice. No use, distribution or reproduction is permitted which does not comply with these terms.
*Correspondence: Carlos Eduardo Pellegrino Cerri, Y2VwY2VycmlAdXNwLmJy