- 1Department of Food Science, College of Agriculture and Veterinary Medicine, United Arab Emirates University, Al-Ain, United Arab Emirates
- 2Department of Food Science and Technology, National University of Singapore, Singapore, Singapore
Introduction: The structuring of plant-based meat alternatives is a complex process which is highly dependent on qualitative and quantitative proportion of different ingredients. In the present study, starch, protein, and oil concentrations were optimized for the formulation of meat alternative (MA) using response surface methodology (RSM).
Methods: Protein isolates of mung bean and pea protein, & corn starch were used along with sunflower oil to formulate meat alternatives using heat-induced gelation. The protein functionality of mungbean protein isolate (MBPI) and pea protein isolate (PPI) were analyzed. In addition, the effects of constituent composition on the physicochemical properties of meat alternatives were studied using RSM.
Results: The protein content exhibited an elevation with increased levels of MBPI and PPI in 15:15 ratio. Moisture and hardness were chiefly influenced by oil content, as they displayed a decline with increasing oil levels. The color (L*) was principally affected by starch and oil, where the L* reduced with increasing levels of both variables. Springiness was influenced by the interaction of protein ratio (MBPI:PPI) and starch, as it showed a lowest value at the lowest level of protein and the highest level of starch. Chewiness was influenced by the interaction of hardness and springiness. The microstructure analysis showed dense protein matrix in the meat alternative.
Discussion: Overall, the study shows that starch facilitated the structuring of meat alternative formulated using MBPI and PPI which could be utilized as potential materials for enhanced textural properties of the meat alternatives.
Introduction
Meat alternatives present sustainable options to the existing meat products due to their composition of plant-based ingredients, provision of equivalent protein content, and reduced ecological impact (Nijdam et al., 2012). Interestingly, flavor, texture, emulsion stability, nutrition, and other relevant attributes to the meat alternative can be modified using starch and protein, which are crucial biomacromolecules. Therefore, the incorporation of these biomacromolecules in plant-based food systems has garnered significant attention, due to the resulting outcomes that greatly rely on the ability of these constituents to interact and form intricate structures. Notably, the terms meat analogs, substitutes and alternatives are often used interchangeably with meat alternatives representing a broader category that aims to replace meats in recipes or dishes (Xiong, 2023).
Numerous investigations are conducted on the interplay between plant protein and starch, both of which are prominent constituents in plant-based diets (Donmez et al., 2021). Currently, there is a significant interest in investigating the interaction between proteins and starch, particularly the contribution of protein in the composition of MA - especially in the matrix that contains starch (Zhang et al., 2021). Gelation involves denaturation and network rearrangement of proteins that is most widely induced by thermal treatments (Tang et al., 2024). It is one of the most important properties in popular food products that improves properties and enables water entrapment, addition of probiotics and other functional ingredients (Li and Zhao, 2018). Appropriate thermal exposure of protein networks enables the formation of three-dimensional network structure that affects protein interactions, textural, and functional properties. Gels thus formed have intricate structures and show high water holding capacity, making them useful in formation of meat substitutes. Thermal gelation, additionally, influences the strength and nature of bonds responsible for gel formation including disulfide bridges, hydrogen bonds and hydrophobic interactions (Tang et al., 2023; Tang et al., 2024).
Yang et al. (2019) highlighted that starch and protein interactions during thermal cooking is mainly driven by hydrogen bonding. The authors observed that with the addition of protein, the gelatinization process in starch was inhibited while amylose and amylopectin retrogradation process was strengthened, affecting the physiochemical and nutritional properties of the developed product. The gelation and physical behavior are further influenced by the ratio of starch to protein (Joshi et al., 2014). The inclusion of starches in meat analog and the influence of starch-protein interaction in texture and functional properties of meat alternatives is not extensively investigated. Furthermore, the exclusive use of starch in meat alternative would lead to the absence of rheological, mechanical, and nutritive characteristics that are associated with “meat.” A potential solution for such challenges could involve the appropriate addition of protein isolate and starch to the matrix in desired levels to create a network that could form a meat analogous structure upon heat gelation. The presence of proteins and their interactions with starches significantly affect the structural integrity and characteristics of the matrix. Analyzing the diverse functions of proteins (both endogenous and exogenous) within various starch-based food systems may offer a foundational framework for the formulation and manufacture of enhanced starch-based food items with customized attributes and favorable nutritional properties (Zhang et al., 2021). Seetapan et al. (2023) explored feasibility of mung bean protein isolates to formulate high-moisture extruded meat analogs and observed that pure mung bean isolate was difficult to extrude and had to be textured along with mung bean flour. It thus becomes interesting to explore the role of starch and oil induced modification to improve texturability of mung bean protein isolates. In another study, Le et al. (2024) formulated meat-reduced sausages from mung bean flour and highlighted the improvements in phytochemical profile and improved consumer acceptability. In addition, starch is a hydrocolloid, which is water-soluble or water-dispersible and can bind through cross-linking with protein filaments, thereby improving the textural qualities of meat analogs. Besides, binders and stabilizers such as methylcellulose and gum arabic are examples of hydrocolloids commonly utilized for this purpose, which contribute to the texture, appearance, and shelf life of plant-based meat analogs (Taghian Dinani et al., 2023). Moreover, to achieve improved outcomes, various protein combinations such as wheat gluten blends with isolates of soy and pea proteins (Schreuders et al., 2021) are utilized in meat alternatives. These legume proteins form a viscoelastic gel structure that facilitates the immobilization of particles, fat, and water (Sha and Xiong, 2020). Unlike other plant proteins, legume proteins have relatively high protein content, which typically ranges from 20 to 35% depending on factors such as variety, growing environment, and maturity level. Not only protein, but also the legume seeds are high in a variety of nutrients (Doss et al., 2022). Peas, one of the protein rich edible legumes are characterized by remarkably high concentrations of lysine, threonine, and other essential amino acids. Recent studies have explored the potential use of various plant proteins including mung beans, fava beans, and chickpeas to formulate meat alternatives (Webb et al., 2023).
Among various legumes, mung bean proteins are progressively gaining popularity. These proteins exhibit a substantial protein content ranging from 25 to 28%, while their fat content remains relatively low, ranging from 1 to 2% (Brishti et al., 2021). Mung bean protein contains leucine as the indispensable amino acid. It is worth mentioning that mung bean’s digestible indispensable amino acid score (DIAAS) is rated at 86, whereas pea and soybean proteins achieve scores of 70 and 91, respectively. The majority of proteins present in mung bean possess a globular structure, which imparts exceptional gelling properties to the protein (McClements and Grossmann, 2021a). Moreover, mung bean proteins exhibit comparable emulsion and foam-stabilizing characteristics to those of faba and chickpea proteins. Consequently, they can be utilized to formulate meat analogs that possess a well-balanced amino acid profile and favorable textural attributes (Brishti et al., 2021; Sha and Xiong, 2020). On the other hand, fats and oils are added in plant-based meat analogs to improve mouthfeel, juiciness, flavor release and tenderness of the developed product (Bohrer, 2019). Traditionally, plant based ingredients used for the development of meat analogs are low in lipid content (Kyriakopoulou et al., 2019) and therefore, collective efforts in optimizing the type and nutritional value of oils have been made over the years to improve the consumer acceptance of meat analogue products. Replacing animal-based saturated fats and trans fats with plant-based unsaturated fatty acids in meat analogs makes them more interesting for consumers.
Within the scope of this investigation, we have thoroughly examined the capacity of starch, in conjunction with mung bean and pea protein isolates, to form a matrix capable of generating meat alternative. The ratios of starch, protein, and oil were appropriately adjusted to achieve the desired texture. Subsequently, the resulting meat alternatives underwent comprehensive evaluation with respect to various parameters, including texture, rheology, color, moisture content, and protein composition. The interaction between starch and proteins holds promising potential for the formulation that closely emulate the texture of animal-derived meat, without necessitating energy-consuming process.
Materials and methods
Materials
For the purpose of preparing a meat alternative, protein isolates of mung bean and pea protein of food grade quality were acquired from ET protein in Suzhou, China. Calcium chloride was procured through Sigma-Aldrich Chemicals (Missouri US). Commercially available corn starch, baking powder, gum Arabic, methylcellulose (MC), salt, and sunflower oil were procured from local markets in Al Ain, UAE.
Functional properties of protein isolates
Emulsifying activity
The experimental procedure described by O’Sullivan et al. (2016) for the measurement of emulsifying activity was followed with minor adjustments. Briefly, 300.0 mg of protein isolates were combined with 30.0 mL of deionized water, resulting in a 1% protein equivalent with adjusted pH to 7.0. Next, 10.0 mL of sunflower oil was mixed with the protein solution and homogenized with a high-speed homogenizer (Ultra Turrax, T-25, IKA, Germany) at a speed of 20,500 rpm for a duration of 1 min at ambient temperature, leading to the formation of emulsions. Subsequently, a precise removal of 50.0 μL sample (without any of the foam on top) was done from the lower part of the tube using a small pipette and quickly combined with 0.1% SDS solution in total 5 mL volume. The absorbance of the resulting mixture, serving as an indicator of the emulsifying capability, was recorded at 500 nm using the microplate reader (Thermo Scientific Multiskan SkyHigh, Sweden). Two replicates of each sample were made and each replicate was tested thrice. The emulsifying activity index (EAI) calculation was done using the Equation 1 given by Pearce and Kinsella (1978).
Water holding capacity and oil holding capacity
The prescribed protocol (Sakai et al., 2022) was used to measure the percent water holding capacity and percent oil holding capacity of mung bean and pea protein isolates. First, 1.0 gram of protein (W0) was weighed together with the tube of known weight (W1). The sample solution with a final concentration of 10.0 g/L was prepared by adding water or oil. The solution was shaken at room temperature for 1 h then rested for 30 min and centrifuged at 5000 × g for 20 min. The liquid part was poured out and the tube with the residue was weighed (W2). The WHC and OHC were calculated using the following Equations 2, 3:
Formulation of meat alternatives (MAs)
The method described by Yuliarti et al. (2021) was used for the formulation of MAs. In this study, each recipe of the alternative, weighing 100 g, contained MBPI-PPI, potato starch, baking powder, sunflower oil, calcium chloride, salt, MC, gum Arabic and ice water. The ingredients were mixed in various ratios according to the Box–Behnken design. The effects of three independent variables viz. protein (20–40%); starch (20–40%) and oil (3–7%) content was studied on the moisture and protein content, hardness, springiness and chewiness values of the developed meat alternatives (Table 1). Graphical optimization technique was employed to recommend an optimized condition. Specifically, the protein, starch, and MC were separately mixed in a blender (Black and Decker, FX400BMG) for 3 min. The first mixture was prepared by adding MBPI and PPI, baking powder, calcium chloride solution, salt, starch, and ice water for 3 mins in the blender. Likewise, in the next step, emulsion was made by blending gum Arabic, MC, soybean oil, and ice water. Then, the above two mixtures were mixed and homogenized well for another 3 min to get a stable emulsion. The emulsion was added to the mold having dimensions of 4.0 × 3.0 × 2.5 cm (length × width × height) and steam heated at a temperature of 100°C for 14 min. After this, the meat alternative was frozen at −20°C. Triplicates samples were made for each recipe.
Physicochemical properties of meat alternatives
Total protein content
The total protein was quantified by the Kjeldahl method, as outlined in the AOAC International guidelines. In brief, roughly 1.0 g of meat alternative was subjected to hydrolysis using 15.0 mL of concentrated sulfuric acid (H2SO4), and a copper catalyst tablet, within a heat block (Kjeltec system 2020 digestor, Tecator Inc., Herndon, VA, USA). This hydrolysis process was conducted at a temperature of 420°C for a duration of 2 h. After cooling, H2O was added to the hydrolysates prior to the processes of neutralization and titration. The total protein content was calculated by multiplying the total nitrogen content by a conversion factor of 6.25.
Texture
The method described by Yuliarti et al. (2021) with some modifications was used to analyse the texture profile of MAs using a texture analyzer (CT3, Brookfield Engineering Laboratories, Middleboro, USA). The texture profile analysis (TPA) of the meat alternative was studied using two compression/decompression cycles. The TPA parameters were studied under fixed conditions: pre-test, test and post-test speed of 2 mm/s; target mode (strain); strain/distance (50%); gap time (5 s); trigger type (auto force); trigger force (15 g). The meat alternative was cut into dimensions of 4.0 × 3.0 × 2.5 cm (length × width × height) and kept on the texture analyzer platform. Then, the middle of the meat alternative was pressed to 40% of its initial height twice at a speed of 5.0 mms−1 with a 7 mm cylindrical probe at room temperature. The texture profile analysis was recorded based on the force versus time plots. Three measurements were done for each sample.
Color
The cross-section of the MAs was analysed for the color measurement using colorimeter (Konica Minolta CR-400, Tokyo, Japan). The color assessment was based on the Commission International de l’Eclairage (CIE) system and reported in terms of L*, a*, and b*. Measurements were taken at three different points on the cross-section of each sample.
Moisture content
The moisture content (mc) of all samples was determined through the utilization of the hot air-oven (Baig et al., 2023). The MA sample (3.0 g) was transferred into aluminum pans. These pans were then placed within an oven (Memmert, UN55, Germany) and subjected to a temperature of 103°C for a minimum duration of 16 h, until a constant weight was obtained. Following this, the percent initial moisture content (% mcinitial) was determined subsequent to the cooling process within a desiccator. This calculation was performed utilizing Equation 4.
Viscoelasticity
In accordance with the previously described protocol, the viscoelasticity was measured (Zhu et al., 2021) using a rheometer (HR-2, TA Instruments, Newcastle, USA) equipped with a parallel plate configuration (diameter: 40.0 mm). The measurement was carried out with a plate gap of 1.0 mm at 25°C. The deposition of 2.0 g of the specimen on the lower plate was performed precisely using a spatula. The width of the gap was gradually reduced during the loading process. The frequency sweeps (0 to 16 Hz) at a strain of 1.0% were employed to ascertain the mechanical spectra of the specimens, leading to the acquisition of the storage modulus G′ and the loss modulus G′′.
Microstructure
The microstructure of the meat alternative samples was determined using a Scanning Electron Microscope (SEM) (JEOL JSM-6010LA, Tokyo, Japan) as per previously described method (Yuliarti et al., 2021). Small pieces (2–3 mm) of the MA samples were frozen with liquid nitrogen and kept for 12 h in 2.5% glutaraldehyde in 0.2 M phosphate buffer at a pH of 7.2 for fixation. Then, the samples were washed thrice with double distilled water and kept for 15 h each. The samples were dehydrated in a series of ethanol solutions (50% for 15 min, repeated twice; 70% for 15 min, repeated twice; 80% for 15 min, repeated twice; 90% for 15 min, repeated twice; 100% for 30 min). Finally, the SEM images were captured by putting the samples in the vacuum chamber of the microscope. The images were taken at 100x and 500x magnification, with a voltage of 20 kV.
Statistical analysis
All experiments were conducted in triplicate, and the reported results included the average values along with the standard deviation errors. The SPSS 24.0 software (SPSS INC., Chicago, IL, USA, 2002) was used to analyze one-way ANOVA of collected data. Tukey’s test at a significance level of p < 0.05 was used to compare the mean values. Significant differences among the various MA samples were observed at the same p value. The graphical images were generated by OriginPro version 8.5 (OriginLab, Massachusetts, USA). The RSM data was examined by utilizing the design expert software (Stat Ease Inc., Minneapolis, USA). ANOVA was employed to ascertain the linear regression, quadratic coefficients, and interactions. The estimation coefficients of R2, adjusted R2, and predicted R2 based on the polynomial equations were calculated at a 95% significance level (p < 0.05).
Results and discussion
Functional properties of the proteins
Emulsifying activity index (EAI)
EAI is commonly evaluated in order to measure the quantity of emulsified interfacial area per gram by an emulsifier (Jia et al., 2020). Within the scope of this particular investigation, the EAI of MBPI and PPI at different pH levels (2.0, 4.0, 6.0, 8.0, and 10.0) are presented in Figure 1. MBPI and PPI demonstrated similar magnitudes of EAI as a consequence of pH variations. The minimum value was observed at pH 6.0 for MBPI and pH 4.0 for PPI, while both PPI and MBPI showed maximum values of EAI at pH 10. Specifically, for MBPI, the EAI value of 9.09% was noted at pH 2.0, 8.45% at pH 4.0, which then declined to 5.66% at pH 6.0, before subsequently rising to 7.57% at pH 8.0, and finally reaching 21.89% at pH 10.0 (Figure 1A). On the other hand, for PPI, the EAI value of 9.2% was observed at pH 2.0, which subsequently decreased to 5.5% at pH 4.0, and increased to 8.5% at pH 6.0, 10.2% at pH 8.0, and further rose to 18.3% at pH 10.0 (Figure 1B). The EAI of mung bean protein demonstrates its minimum value at pH 6, which is in close proximity to a state of neutrality. One possible explanation for this phenomenon could be a reduction in the electrical charge of the protein at this particular pH, leading to a diminished capacity to interact with oil and water molecules. Importantly, the mung bean protein’s EAI is also elevated at pH 8, which is slightly basic. This could be ascribed to an augmentation in the protein’s negative charge at this pH, resulting in an intensified repulsion from water molecules and a stronger attraction to oil molecules (Nair and Schreinemachers, 2020).
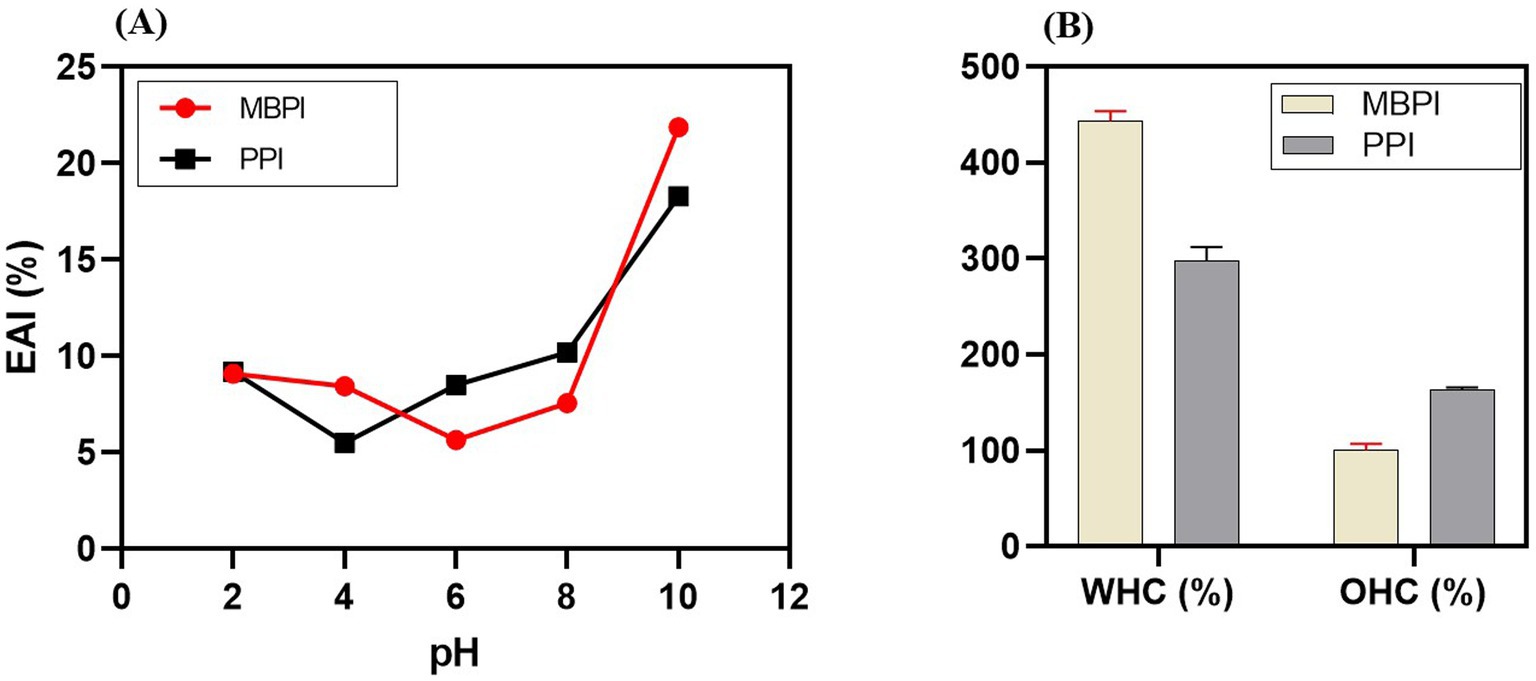
Figure 1. (A) Effect of pH on Emulsifying Activity Index (EAI) of Mung bean protein isolate (MBPI) solutions and Pea protein isolate (PPI) solution and (B) Water Holding Capacity (WHC) and Oil Holding Capacity (OHC) of MBPI and PPI are presented as percentages (%). The data were collected in triplicates (n = 3).
The increase in pH value is typically associated with an augmentation in EAI of PPI, reaching its highest point at pH 8–9. At elevated pH levels, plant proteins exhibit increased solubility, thereby augmenting their capacity to lower surface tension and create stable emulsions (Arteaga et al., 2021). Nonetheless, at excessively high pH levels (over 10), protein denaturation and functional loss may result in a decrease in EAI of PPI (Burger and Zhang, 2019).
Overall, it has been demonstrated that higher EAI is manifested at highly acidic and alkaline pH levels, while inadequate EAI was observed at pH 6.0, thus indicating the pH dependence of both MBPI and PPI on EAI. Previous research conducted by Shevkani et al. (2015) reported similar findings on the EAI of legume proteins (Shevkani et al., 2015). As depicted in Figures 1A,B, the EAI value exhibited a significant decrease as the pH was elevated from 4.0 to 6.0. This can be attributed to the decrease in electric force between proteins because of the closeness of pH to their isoelectric point (PI). In addition, the isoelectric points of MBPI and PPI are 4.6 and 4.3, respectively, as reported by Zhang et al. (2009).
Water and oil holding capacity
The WHC of MBPI and PPI tends to exhibit an upward trend with higher pH levels, temperature, and protein concentration, as these variables augment the solubility and hydration of the protein (Tang et al., 2023). OHC of MBPI and PPI tends to decrease with increasing pH, temperature, and protein concentration, likely due to reduced protein surface area and hydrophobicity (Kiosseoglou and Paraskevopoulou, 2011). The WHC of Mung bean protein (443.36%) is higher than that of pea protein (298.13%), while pea protein has only slightly higher OHC than Mung bean protein. These distinctions may have an impact on the effectiveness and quality of food products that utilize these proteins.
In comparison to Mung bean protein, pea protein exhibits lower WHC but slightly higher OHC, as depicted in Figure 1C, which may have implications for the functional and qualitative properties of food products produced using these proteins. The oil-holding capacity of both proteins was similar to the values between 1.1 and 1.7 g/g, which were reported for proteins from soy, faba, and pumpkin (Ge et al., 2021). Both water and oil can be bound by a large amount of these proteins. These capacities are similar for both types of proteins. The disparities in WHC and OHC (between MBPI and PP) can be ascribed to various factors associated with the protein’s configuration and characteristics. The hydrophobic characteristic of proteins could greatly affect their capacity to interact with water and oil molecules. Proteins with increased hydrophobicity tend to exhibit a stronger inclination toward oil, thus potentially elucidating a higher OHC (Baig et al., 2023). The structural conformation of proteins in three dimensions, encompassing their folding and the exposure of hydrophobic and hydrophilic regions, plays a role in determining their functional attributes. For instance, a more accessible structure could facilitate the entrapment of more water, thereby augmenting WHC. The composition and configuration of amino acids within the protein might impact its functionality. Amino acids for example proline and hydroxyproline, prevalent in collagen, play a role in maintaining moisture levels, while non-polar amino acids help with oil adherence (Sołowiej et al., 2016).
Changes in moisture content
The moisture content ranged between 26.68 and 46.26% with various combinations (Table 2). The MAs demonstrated the maximum moisture of 46.26% when a high level of X1 (40%) of MBPI-PPI (1:1 ratio), a high level of X2 (40%), and a medium level of X3 (5 mL) were utilized. Conversely, the lowest moisture content was observed when all three variables, X1 (30%) of MBPI-PPI (1:1 ratio), X2 (30%), and X3 (5 mL), were set at a medium level. The level of moisture present in the meat alternative plays a significant role in determining its texture, taste, and shelf life. The fitted linear model (Table 3) was very accurate in predicting the response, as indicated by the high R2 value of 0.999. The maximum positive coefficient (5.88) of X2 implies that the quantity of starch has a relatively greater impact on the moisture content compared to the protein (X1) and oil (X3) percentage. The model did not find any significant influence (p > 0.05) from the interactive as well as quadratic effects of ingredient combinations (Supplementary Table S1). The presence of oil percentage (X3) has an adverse effect on the moisture content of the meat alternative. The highest moisture content is observed in run 9 (46.26%), which had the highest level of starch percentage (40%) and a moderate level of oil percentage (5%). On the other hand, run 10 exhibits the lowest percentage of moisture content (26.68%). This particular run was conducted at a moderate level of all independent variables. Notably, the dependent variable experiences a decline as the levels of X3 increase. Moreover, the impact of different ingredients on the moisture is illustrated in Supplementary Figures S1d–f.
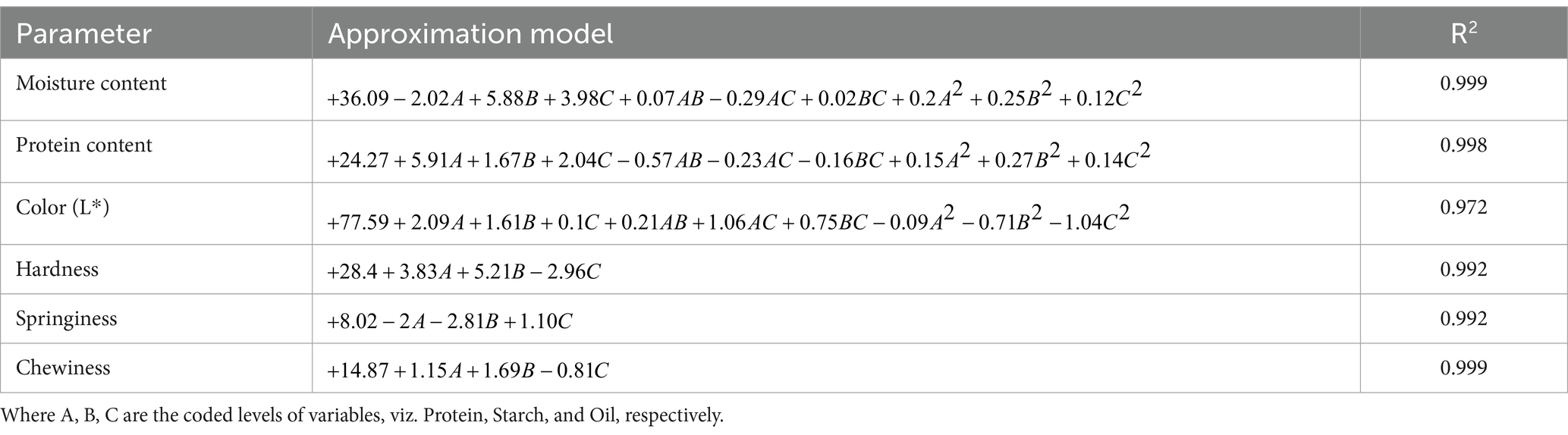
Table 3. Approximation models for prediction of response variables during formulation of meat alternatives.
Protein content (PC)
The protein percentage composition of MAs, in relation to changes in factors X1, X2, and X3, ranged from 16.10 to 32.54% (Table 2). The percentage of protein in the meat alternative is a significant factor that influences its nutritional value, texture, taste, and juiciness. Plant-derived proteins possess the capability to bind water, stabilize emulsions, and form gels (Kyriakopoulou et al., 2021). As expected, protein percentage (X1) had the most significant (p < 0.05) effect on the overall protein content of developed meat alternatives.
The model exhibited a high coefficient of determination (R2 = 0.998) indicating a good adequacy of fit. Elevated levels of protein can enhance the nutritional characteristics of meat alternatives, rendering them more comparable to animal-derived meat in their protein composition. However, the main priority should not only be on the protein quantity; the protein quality, digestibility, and amino acid composition are critical in determining the holistic nutritional impact of the meat alternative (Singh and Sit, 2022). Mung bean protein have a remarkable abundance of essential amino acids, particularly lysine and methionine. However, it displays a comparatively reduced presence of sulfur-associated amino acids including methionine and cysteine. The essential amino acids present in mung bean protein consist of Lysine, Leucine, Isoleucine, Phenylalanine, Threonine, and Tryptophan (Nair and Schreinemachers, 2020). The amino acid makeup of pea protein is quite balanced, showing a notable presence of lysine, arginine, alongside branched-chain amino acids (BCAAs) like leucine, isoleucine, and valine. Key amino acids identified in pea protein comprise Lysine, Arginine, Leucine, Isoleucine, and Valine (Lu et al., 2020). Furthermore, the concentration of protein has the potential to impact the texture, taste, and juiciness of the meat alternative, attributes that hold significance in terms of consumer approval. To illustrate, the combination of various proteins in precise proportions has the ability to alter the water and oil absorption capacity, consequently influencing the cooking efficiency and texture of the end product (Gaber et al., 2023; Wittek et al., 2021). The experimental results indicate that the maximum protein content (%) was observed in run 5 (32.55%), which corresponds to the highest protein ratio (40%) and the lowest starch content (20%). Conversely, the minimum protein content (%) was observed in run 6 (16.10%), which corresponds to the lowest protein ratio (20%) and the highest oil content (7%).
The fitted second-order approximation model (Table 3), illustrates that all three factors demonstrated a positive coefficient, as can be seen from the equation. This finding implies that all the three linear factors had a positive influence on the principal component of MAs specifically the protein concentration, as illustrated in Supplementary Figures S2a–c. This finding implies that all the three linear factors had a positive influence on the principal component of MAs specifically the protein concentration. Consequently, the formulation of MAs in this study utilized an ingredients combination that led to the production of MAs with an adequate principal component–a critical factor in the formulation of MAs.
Color
The color of MAs is crucial due to its substantial influence on consumer perception and acceptance. The determination of the color of MAs is based upon the specific ingredients, additives, and processing methods employed during their production (Godschalk-Broers et al., 2022). Specifically, the L* values, which show the degree of lightness or darkness of the MAs, were used to measure the luminosity function (Table 2). The dependent variable, Color (L*), is primarily affected by the starch percentage (X2) and oil percentage (X3), resulting in a decrease with increase in both variables. Additionally, the protein ratio (X1) exerts a positive impact on this dependent variable, as an elevation in X1 levels corresponds to an increase in Color (L*) levels, possibly due to the inherent color attributes of the mung bean and pea protein isolates. Proteins, particularly those sourced from plant origins such as soy, pea, and wheat, play a pivotal role in the coloration of meat analogs (Tan et al., 2023). They engage with natural colorants and additional components to facilitate the imitation of a meat-like appearance. During the cooking process, proteins participate in the Maillard reaction that imparts to browned foods their characteristic flavor and coloration (Kyriakopoulou et al., 2019). Proteins contribute to the cohesion of other components within the mixture, thereby ensuring that the colorants are uniformly dispersed throughout the product. Furthermore, the texture and moisture content of meat analogs, which are significantly influenced by the proteins employed, also play a crucial role in the sensory perception of color (Sha and Xiong, 2020). The color of the meat alternative may be influenced by various factors, including the type, source, concentration, and purity of the protein (Singh and Sit, 2022). The color stability and development of the meat alternatives can be influenced by the oil content. An increase in oil content can lead to a reduction in color stability, whereas a decrease in oil content can enhance the color stability of the product (Kendler et al., 2021). Consistent texture can significantly affect the distribution and retention of colorants within the product matrix (Kyriakopoulou et al., 2021). Starch interactions with both natural and synthetic colorants are instrumental in attaining a homogeneous color distribution, which is vital for accurately replicating the visual characteristics of traditional meat. Starch also enhances the stability of emulsions in meat substitutes (Dobson et al., 2022). A stable emulsion guarantees that colorants remain uniformly dispersed within the mixture, thereby sustaining a coherent color profile throughout the product (Donmez et al., 2021). Additionally, starches provide thermal resistance of meat substitutes, a property that is critical during the cooking process. This enhancement aids in preserving the coloration achieved during the preparation phase (Ianiţchi et al., 2023). Supplementary Table S1 shows the results of the ANOVA for dependent variables. The table also shows the coefficients of the model, which is significant (p < 0.05) and fits well, as shown by the lack of fit p-values of 0.815. The non-significant lack of fit p-values means that the model can explain the relationship between the dependent variables and the independent variables (X1, X2, and X3). Moreover, the R2 value of the model (0.972) shows that the model accounts for almost 97% of total variations, supporting the model significance (Table 3).
The fitted model explains the linear, quadratic, 2-way association between the independent variables and L* values. The coefficient value (−2.09) of X1 signifies that L* values have indirect relationship with the protein concentration, whereas X3 has a comparatively lesser contribution. This implies that the proteins and other ingredients used to make MAs may have a significant effect on the color parameters of the MAs.
The L* value exhibited an increase when elevated quantities of starch and low quantity of oil was added into the MBPI-PPI blend (15:15), as illustrated in Supplementary Figures S2d–f. A difficulty encountered by certain methodologies in generating alternatives for fat in MAs is the deficiency in the formation of light-scattering structures, resulting in the absence of turbidity or intense color, as well as the requirement for elevated processing temperatures (McClements and Grossmann, 2021b). Animal fat particles are characterized by their whitish appearance, and it is imperative for a fat replacer to effectively imitate this visual attribute. To successfully achieve this emulation, Dreher et al. (2020) implemented an emulsion-based methodology in their investigation. This finding implies that a greater fraction of starch is capable of significantly augmenting the L* value (lightness) of the MAs.
Texture
The understanding of textural attributes is crucial for the development of meat alternatives that exhibit similar characteristics to meat products, such as comparable cutting abilities or mouthfeel attributes (e.g., bite strengths). Elongation tests offer valuable insights into the degree of anisotropic structures present in meat analogs (McClements and Grossmann, 2021b). This comprehensive analysis is presented in Table 2. The texture of meat alternatives is determined by the combination and interaction of protein, oil, and starch, as well as the processing methods employed. Protein plays a crucial role in contributing to hardness, chewiness, and overall mouthfeel. The selection of protein type and its concentration can have an impact on the gelation, water-holding capacity, and elasticity of the final product (Kumar et al., 2022; Singh and Sit, 2022). The juiciness and mouthfeel of meat alternatives can be influenced by oils. Additionally, the introduction of oil may change the mechanical attributes of the protein framework, consequently influencing the toughness and unity of the item. A striking example is a study that uncovered rapeseed oil droplets performing as inert fillers in pea protein matrices, leading to a decrease in gel strength and Young’s modulus, which are indicative of firmness and elasticity in texture (Saavedra Isusi et al., 2023). It is essential to take into account the type of oil used and its interaction with the protein source as critical considerations. Starches are commonly utilized as binders and fillers in meat alternatives, impacting both the moisture content and texture of the product. They can contribute to a certain level of firmness and elasticity, while also influencing water-holding capacity and freeze–thaw stability (Sołowiej et al., 2016). The obtained values for hardness, chewiness, and springiness ranged from 37.66–19.4 mJ, 17.76–12.03 mJ, and 12.95–3.05 mm, respectively (Table 2). The results clearly demonstrate that the highest recorded hardness (mJ) is observed in experiment 8 (37.66), which is associated with a medium amount of oil % (5%), a low amount of protein ratio (20%), and a high amount of starch (40%). Conversely, the lowest recorded hardness (mJ) is observed in experiment 15 (19.4), which is linked to the highest level of protein ratio (X1 = 1), a medium level of oil % (5%), and the lowest level of starch % (20%). Although the lowest level of hardness was noted at elevated levels of protein, moderate levels of oil, and reduced levels of starch, this phenomenon could potentially be attributed to the cumulative impact of oil and starch on water retention, as well as the weakened interaction and crosslinking of proteins, ultimately resulting in lower hardness. The assessment of the coefficient of determination R2 served as a method to validate the resilience of the model. The model accounted for more than 99% of the data variations in hardness, chewiness, and springiness. The linear fitted model (Table 3) gives the formula for hardness based on the coded value of ingredients and shows that hardness increased with increasing the protein concentration and starch concentration, but decreased with increased level of oil concentration. The coefficient of X2 (5.211) was the highest, which means that it had the most influence on the hardness. The hardness was higher when the oil content was lower and vice versa (Figures 2A–C). Figure 2C shows that reducing the oil content significantly increased the hardness. Additionally, the hardness of MAs increased significantly when protein blends, specifically MBPI-PPI, were increased and the oil content was reduced from 7 to 5%, while keeping the starch content constant. These findings are evident in Figures 2A,B. It is worth noting that the decrease in oil content and the increase in starch levels could potentially lead to the formation of breakable MAs, which is advantageous for constructing a fibrous structure. The ramifications of gelatinization and retrogradation extend to the viscosity, water retention, and formation of networks within the starch (Wang et al., 2022). Consequently, these factors can have an effect on the texture and overall quality of meat alternatives (Chen et al., 2022). The measurement of the samples’ springiness (mm) was conducted, and it was determined that the highest value was observed in run 11 (12.95 mm). This particular run exhibited the lowest level of oil % (3%) and moderate levels of protein ratio (30%) and low starch (20%). Conversely, the lowest springiness (mm) was observed in run 8 (3.05 mm). Interestingly, this specific run shared the same levels of independent variables as run 8, except for protein ratio (20%). The highest chewiness (mJ) was recorded in run 8 (17.76 mm). The percentage of protein, starch, and oil effected the chewiness and springiness which is significant (p < 0.05) as demonstrated in Supplementary Table S2. The chewiness (Figures 2G–I) of the MAs increased with the protein and the starch concentrations. On the other hand, the springiness (Figures 2D–F) of the MAs was higher with low levels of protein, starch, and oil. Conversely, low levels of protein, starch, and oil contribute to the springiness (Figures 2D–F) of the MAs.
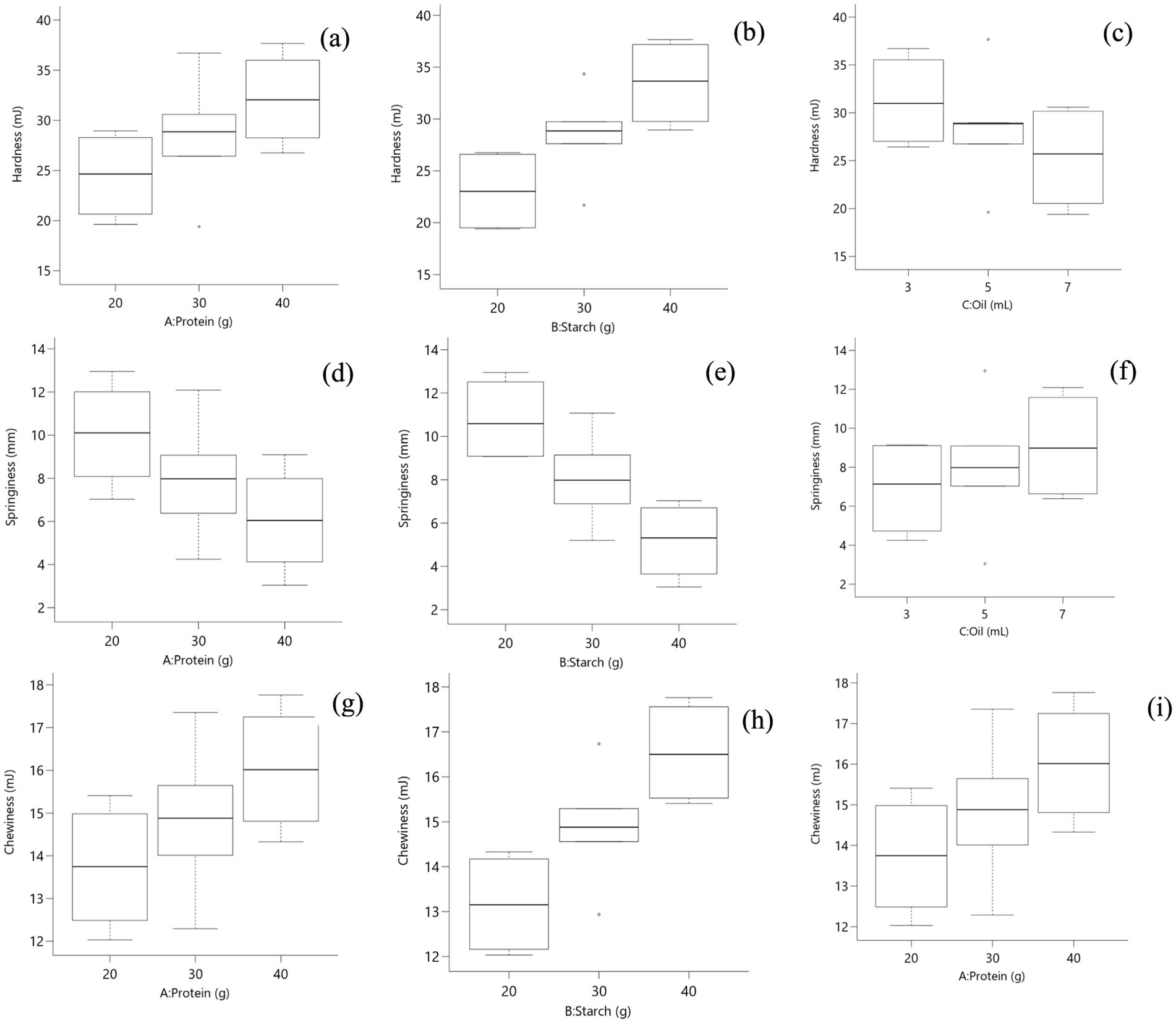
Figure 2. Graphical representations expressing the effects of varying levels of protein, starch and oil on hardness (a-c); springiness (d-f) and chewiness (g-i) of developed meat alternatives respectively.
The fitted models (Table 3) delineate the positive contribution of X1 and X2 to the attribute of chewiness, as evidenced by the presentation of positive coefficients. Furthermore, the coefficient of X3 indicates a negative impact on chewiness. The models elaborate on the negative contribution of X1 and X2 toward the springiness. The starch concentrations effected the chewiness which significantly improve the textural parameters of MAs. The essential characteristic of various components utilized in the creation of MAs plays a crucial role in establishing the final composition of the product (Bakhsh et al., 2021).
Consequently, within this investigation, the quantity of protein and starch could potentially be the primary constituents that influenced the textural characteristics of MAs. This suggests that starch played a role as a particle-filled supportive framework alongside proteins in the development of a fibrous structure.
Consequently, the process variables were optimized using the graphical optimization technique. The optimization process returned an ideal contour area demarcating process variables as shown in overlay plots (Figure 3), that would give best responses in the context of meat alternatives (maximization of protein content, hardness, springiness, chewiness and moisture content within range). It can be observed that the optimum meat alternatives with ideal properties could be formulated using protein content of 33.28%, Starch content of 40% and an oil content of 6.9 mL. The observed moisture content at these experimental levels would be a moisture content of 45.42%, protein content of 29.82%, L* values of 78.06, hardness of 3.2 mJ, springiness of 5.6 mm, and chewiness of 16.16 mJ.
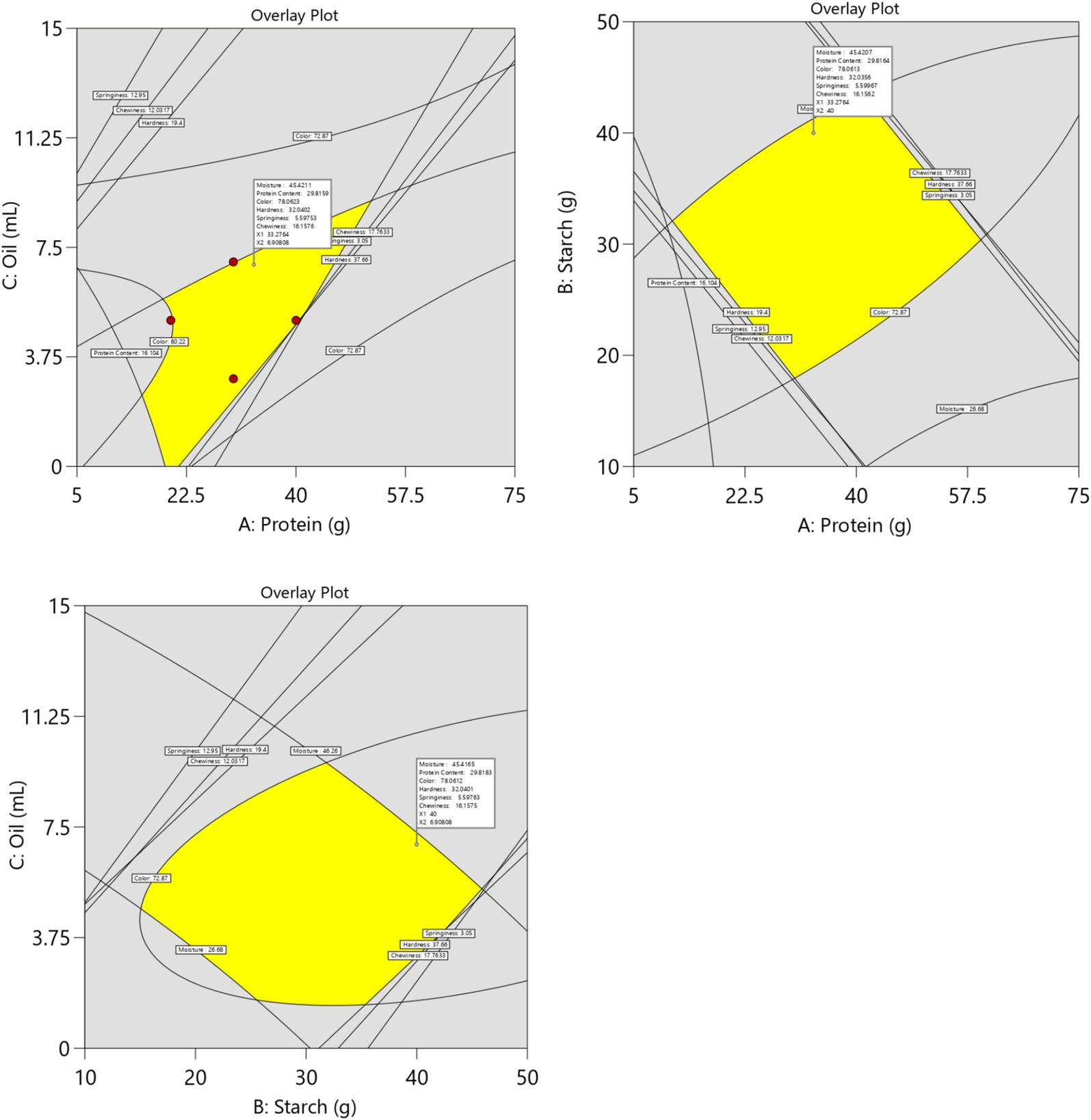
Figure 3. Overlay plot showing contours of graphical optimization for process parameters and the corresponding optimized response values.
Viscoelastic properties
The viscoelasticity of optimized MAs was assessed through frequency sweeps in order to gain additional insights into their viscoelastic properties as a result of changes in frequency (Figures 4A,B). The loss modulus G” was found to be indicative of the viscoelasticity of the network, while the storage modulus G’ exhibited solid-like characteristics similar to elasticity (Yuliarti et al., 2021). Figures 4A,B demonstrated that G’ and G” remained relatively unchanged at lower amplitudes, but at higher amplitudes, there was an intersection of G’ and G,” leading to alterations in the network due to the dominance of G.” The frequency sweep measurements showed that the sample had higher loss and storage moduli as the frequency increased. The elastic nature of the alternative was dominant, as G’ was higher than G” for all the frequencies tested. This study also found that alternatives with more protein and starch had lower viscosity but higher elasticity, which represents the stored energy in the internal network instead of being lost. The protein and starch interaction can affect the starch gelatinization and retrogradation properties, which depend on the amylose and amylopectin ratio in starch (Ianiţchi et al., 2023; Santamaria et al., 2022). The starch-protein interaction influences the viscosity, water retention, and formation of networks within starch, thereby exerting an effect on the texture and quality of meat analogs (Schirmer et al., 2013).
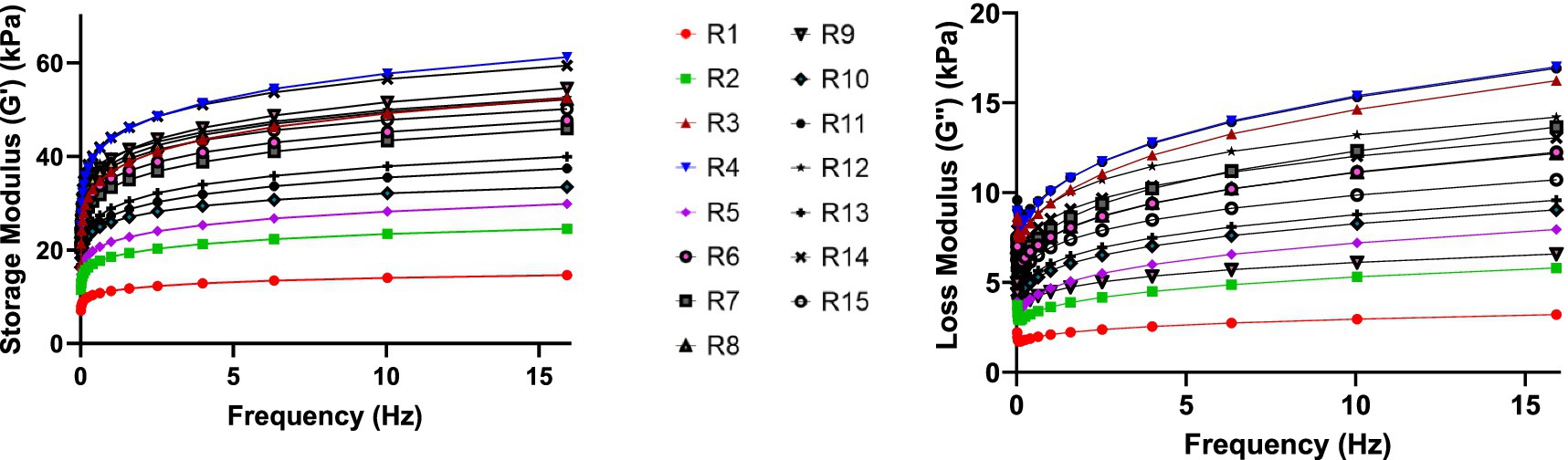
Figure 4. The starch and MBPI + PPI meat alternatives were tested for their viscoelastic properties by changing the frequency from 0 to 16 Hz and keeping the strain at 1%. (A). The elastic behavior of the meat alternatives was shown by the storage modulus G’. The loss modulus G” was shown as a function of the frequency change (0–100 Hz) (B). The runs, R1 to R15 correspond to the Box–Behnken experimental design table (Table 1).
The moduli (G’ and G”) increased when more starch fractions were added to the formulation, which means that the protein matrix had stronger gel strength and formed a better cross-link network. This indicates that starch plays a significant role in reinforcing the meat alternative structure, which can be ascribed to its excellent matrix-forming ability, leading to efficient gel formation and ultimately contributing to the reinforcement of the alternative structure. Similarly, Liu et al. (2021). previously demonstrated that mung bean proteins exhibited superior gelation properties even at lower concentrations compared to other proteins such as soy proteins (Liu et al., 2021). Additionally, The MAs showed elastic behavior because of the gel-forming ability of proteins and starch, which is important for making a tight and elastic network. Our results indicate that the MAs can have better viscoelastic properties by using higher amounts of starch and protein ingredients, which can increase the interactions between proteins and starch. These interactions are necessary for creating the elasticity, strength, and cohesion in the MAs.
Microstructure
As shown in Figure 5, the MAs had a fibrous and porous structure after freeze drying. The transverse section of the meat alternative is depicted in Figure 5A. The SEM images were taken at 100x and 500x magnification (Figures 5B,C) to investigate the impact of ingredient combinations on the fibrous structure of the meat alternative. As shown in Figure 5B, the meat alternative displayed a highly porous and rough structure, likely due to an aggregated network, with some protein networks weakly interconnected. This phenomenon can be attributed to the unfolding and aggregation of protein chains during heating, as well as the interaction between proteins and starch through hydrogen bonding, resulting in the formation of a particle-filled supporting matrix and the development of a three-dimensional network. Previous research has indicated that mixing two proteins can improve the formation of fibrous networks in MAs (Grabowska et al., 2014; Yuliarti et al., 2021). However, the arrangement of the network exhibited a certain degree of laxity and partial compactness, implying that sole reliance on the heat-induced gelation technique proved insufficient in achieving a tightly-knit, well-aligned, and more densely-packed meat alternative. Moreover, the network configuration supports the findings of the viscoelastic nature of the samples, highlighting the advantageous outcomes stemming from the combination of protein and polysaccharide in the formulation of organized, plant-derived MAs.
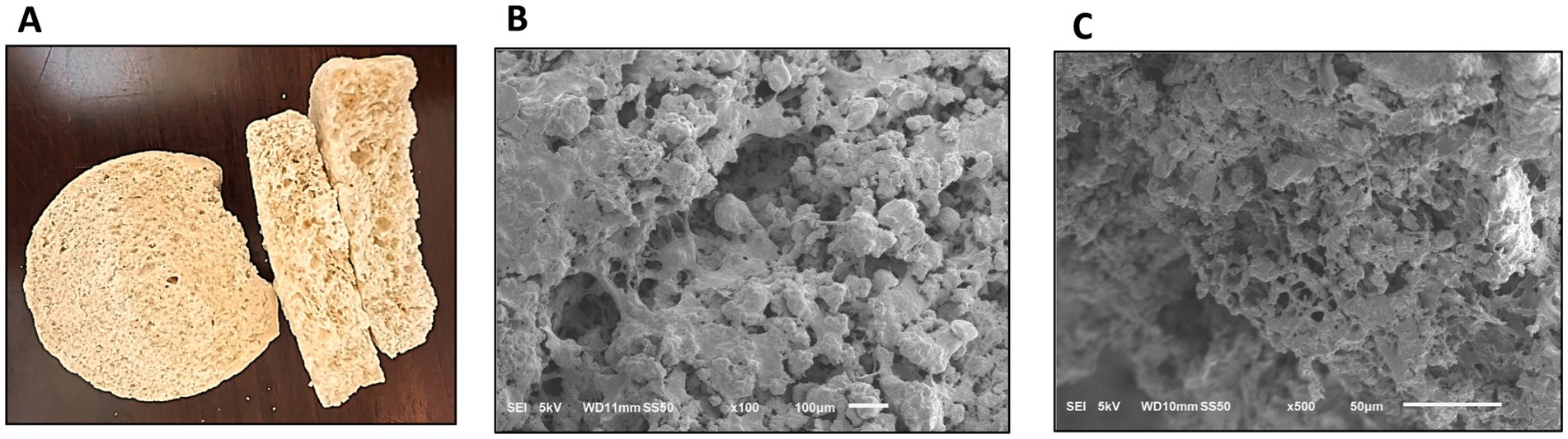
Figure 5. (A) Meat alternative samples formulated with 30% of MBPI-PPI blends in 15:15 ratio, 40% of starch, and 7% of oil (B) micrograph showing porous and fibrous network at 100x, (C) Micrograph of alternatives at 500x.
Conclusion
The application of heat-induced gelation promotes the formation of a starch matrix with MBPI and PPI. The interaction between proteins and starches greatly influences the structure and properties of the matrix. We have shown the possibility of heat-induced gelation in helping the formation of meat alternatives. The texture of the meat alternatives showed a strong dependence on the starch content in the supporting composite. The combination of MBPI, PPI, and starch contributes to the viscoelastic properties of the meat alternatives. The levels of ingredients used have a significant impact on the physicochemical and microstructural qualities of the meat alternatives. However, additional investigation is required for improving heat-induced gelation technique, along with exploring various ingredient combinations, for achieving the desired texture. Proteins and starch are important for creating texture and elasticity. Thus, starch along with proteins could be utilized as potential ingredients for matrix formation in the development of plant-based MAs. The present study demonstrated that the utilization of MBPI-PPI, starch, and oil, can potentially generate MAs via the process of heat-induced gelation.
Data availability statement
The original contributions presented in the study are included in the article/Supplementary material, further inquiries can be directed to the corresponding author.
Author contributions
MB: Formal analysis, Investigation, Methodology, Writing – original draft. HM: Data curation, Writing – review & editing. NS: Writing – review & editing. RA: Data curation, Formal analysis, Writing – review & editing. WZ: Writing – review & editing. SM: Conceptualization, Funding acquisition, Project administration, Supervision, Writing – review & editing.
Funding
The author(s) declare that financial support was received for the research, authorship, and/or publication of this article. The authors are thankful to United Arab Emirates University for funding this project through UAEU-AUA grant (Fund code: 12F002).
Conflict of interest
The authors declare that the research was conducted in the absence of any commercial or financial relationships that could be construed as a potential conflict of interest.
The author(s) declared that they were an editorial board member of Frontiers, at the time of submission. This had no impact on the peer review process and the final decision.
Publisher’s note
All claims expressed in this article are solely those of the authors and do not necessarily represent those of their affiliated organizations, or those of the publisher, the editors and the reviewers. Any product that may be evaluated in this article, or claim that may be made by its manufacturer, is not guaranteed or endorsed by the publisher.
Supplementary material
The Supplementary material for this article can be found online at: https://www.frontiersin.org/articles/10.3389/fsufs.2024.1473663/full#supplementary-material
References
Arteaga, V. G., Kraus, S., Schott, M., Muranyi, I., Schweiggert-Weisz, U., and Eisner, P. (2021). Screening of twelve pea (Pisum sativum L.) cultivars and their isolates focusing on the protein characterization, functionality, and sensory profiles. Food Secur. 10:758. doi: 10.3390/foods10040758
Baig, M. A., Ajayi, F. F., Mostafa, H., Sivapragasam, N., and Maqsood, S. (2023). Mungbean and pumpkin protein isolates as novel ingredients for the development of meat analogs using heat-induced gelation technique. Front Sustain Food Syst 7:1243183. doi: 10.3389/fsufs.2023.1243183
Bakhsh, A., Lee, S. J., Lee, E. Y., Sabikun, N., Hwang, Y. H., and Joo, S. T. (2021). A novel approach for tuning the physicochemical, textural, and sensory characteristics of plant-based meat analogs with different levels of methylcellulose concentration. Food Secur. 10:30560. doi: 10.3390/foods10030560
Brishti, F. H., Chay, S. Y., Muhammad, K., Ismail-Fitry, M. R., Zarei, M., and Saari, N. (2021). Texturized mung bean protein as a sustainable food source: effects of extrusion on its physical, textural and protein quality. Innov. Food Sci. Emerg. Technol. 67:102591. doi: 10.1016/j.ifset.2020.102591
Bohrer, B. M. (2019). An investigation of the formulation and nutritional composition of modern meat analogue products. Food Science and Human Wellness 8, 320–329. doi: 10.1016/j.fshw.2019.11.006
Burger, T. G., and Zhang, Y. (2019). Recent progress in the utilization of pea protein as an emulsifier for food applications. Trends Food Sci. Technol. 86, 25–33. doi: 10.1016/j.tifs.2019.02.007
Chen, Q., Zhang, J., Zhang, Y., Kaplan, D. L., and Wang, Q. (2022). Protein-amylose/amylopectin molecular interactions during high-moisture extruded texturization toward plant-based meat substitutes applications. Food Hydrocoll. 127:107559. doi: 10.1016/j.foodhyd.2022.107559
Dobson, S., Laredo, T., and Marangoni, A. G. (2022). Particle filled protein-starch composites as the basis for plant-based meat analogues. Curr. Res. Food Sci. 5, 892–903. doi: 10.1016/j.crfs.2022.05.006
Donmez, D., Pinho, L., Patel, B., Desam, P., and Campanella, O. H. (2021). Characterization of starch–water interactions and their effects on two key functional properties: starch gelatinization and retrogradation. Curr. Opin. Food Sci. 39, 103–109. doi: 10.1016/j.cofs.2020.12.018
Doss, A., Esther, A., and Rajalakshmi, R. (2022). Influence of UV-B treatment on the accumulation of free phenols and tannins in the legumes of Abrus precatorius L. and Vigna mungo (L.) Hepper. Phytomedi. Plus 2:100189. doi: 10.1016/j.phyplu.2021.100189
Dreher, J., Blach, C., Terjung, N., Gibis, M., and Weiss, J. (2020). Formation and characterization of plant-based emulsified and crosslinked fat crystal networks to mimic animal fat tissue. J. Food Sci. 85, 421–431. doi: 10.1111/1750-3841.14993
Gaber, S. M., Knezevic, D., Saldanha do Carmo, C., Zobel, H., Knutsen, S. H., Sahlstrøm, S., et al. (2023). Meat analogues from pea protein: effect of different oat protein concentrates and post treatment on selected technological properties of high-moisture extrudates. Appl. Sci 13:2354. doi: 10.3390/app132212354
Ge, J., Sun, C. X., Mata, A., Corke, H., Gan, R. Y., and Fang, Y. (2021). Physicochemical and pH-dependent functional properties of proteins isolated from eight traditional Chinese beans. Food Hydrocoll. 112:106288. doi: 10.1016/j.foodhyd.2020.106288
Godschalk-Broers, L., Sala, G., and Scholten, E. (2022). Meat analogues: relating structure to texture and sensory perception. Food Secur. 11:2227. doi: 10.3390/foods11152227
Grabowska, K. J., Tekidou, S., Boom, R. M., and van der Goot, A. J. (2014). Shear structuring as a new method to make anisotropic structures from soy-gluten blends. Food Res. Int. 64, 743–751. doi: 10.1016/j.foodres.2014.08.010
Ianiţchi, D., Pătraşcu, L., Cercel, F., Dragomir, N., Vlad, I., and Maftei, M. (2023). The effect of protein derivatives and starch addition on some quality characteristics of beef emulsions and gels. Agriculture 13:772. doi: 10.3390/agriculture13040772
Jia, C., Cao, D., Ji, S., Lin, W., Zhang, X., and Muhoza, B. (2020). Whey protein isolate conjugated with xylo-oligosaccharides via maillard reaction: characterization, antioxidant capacity, and application for lycopene microencapsulation. LWT 118:108837. doi: 10.1016/j.lwt.2019.108837
Joshi, M., Aldred, P., Panozzo, J. F., Kasapis, S., and Adhikari, B. (2014). Rheological and microstructural characteristics of lentil starch-lentil protein composite pastes and gels. Food Hydrocoll. 35, 226–237. doi: 10.1016/j.foodhyd.2013.05.016
Kendler, C., Duchardt, A., Karbstein, H. P., and Emin, M. A. (2021). Effect of oil content and oil addition point on the extrusion processing of wheat gluten-based meat analogues. Food Secur. 10:697. doi: 10.3390/foods10040697
Kiosseoglou, V., and Paraskevopoulou, A. (2011). Functional and physicochemical properties of pulse proteins, in: pulse foods: processing, quality and nutraceutical applications. Pulse Foods 1, 57–90. doi: 10.1016/B978-0-12-382018-1.00003-4
Kumar, P., Sharma, N., Ahmed, M. A., Verma, A. K., Umaraw, P., Mehta, N., et al. (2022). Technological interventions in improving the functionality of proteins during processing of meat analogs. Front. Nutr. 9:10444024. doi: 10.3389/fnut.2022.1044024
Kyriakopoulou, K., Dekkers, B., and van der Goot, A. J. (2019). “Plant-based meat analogues” in Sustainable meat production and processing. ed. C. M. Galanakis (Amsterdam: Elsevier), 103–126.
Kyriakopoulou, K., Keppler, J. K., and van der Goot, A. J. (2021). Functionality of ingredients and additives in plant-based meat analogues. Food Secur. 10:30600. doi: 10.3390/foods10030600
Le, T. K. Y., Nguyen, K. D., and Hoang, K. A. (2024). Meat-reduced sausage substituted with germinated mung bean flour: proximate composition, physicochemical properties, and sensory acceptability. Int J Food Sci Technol 59, 3391–3401. doi: 10.1111/ijfs.17089
Li, Q., and Zhao, Z. (2018). Interaction between lactoferrin and whey proteins and its influence on the heat-induced gelation of whey proteins. Food Chem 252, 92–98. doi: 10.1016/j.foodchem.2018.01.114
Liu, F. F., Li, Y. Q., Wang, C. Y., Zhao, X. Z., Liang, Y., He, J. X., et al. (2021). Impact of pH on the physicochemical and rheological properties of mung bean (Vigna radiata L.) protein. Process Biochem. 111, 274–284. doi: 10.1016/j.procbio.2021.10.008
Lu, Z. X., He, J. F., Zhang, Y. C., and Bing, D. J. (2020). Composition, physicochemical properties of pea protein and its application in functional foods. Crit. Rev. Food Sci. Nutr. 60, 2593–2605. doi: 10.1080/10408398.2019.1651248
McClements, D. J., and Grossmann, L. (2021a). The science of plant-based foods: constructing next-generation meat, fish, milk, and egg analogs. Compr. Rev. Food Sci. Food Saf. 20, 4049–4100. doi: 10.1111/1541-4337.12771
McClements, D. J., and Grossmann, L. (2021b). A brief review of the science behind the design of healthy and sustainable plant-based foods. NPJ Sci. Food. 5:17. doi: 10.1038/s41538-021-00099-y
Nair, R., and Schreinemachers, P. (2020). Global status and economic importance of Mungbean. Cham: Springer, 1–8.
Nijdam, D., Rood, T., and Westhoek, H. (2012). The price of protein: review of land use and carbon footprints from life cycle assessments of animal food products and their substitutes. Food Policy 37, 760–770. doi: 10.1016/j.foodpol.2012.08.002
O’Sullivan, J., Murray, B., Flynn, C., and Norton, I. (2016). The effect of ultrasound treatment on the structural, physical and emulsifying properties of animal and vegetable proteins. Food Hydrocoll 53, 141–154. doi: 10.1016/j.foodhyd.2015.02.009
Pearce, K. N., and Kinsella, J. E. (1978). Emulsifying properties of proteins: evaluation of a Turbidimetric technique. J. Agric. Food Chem. 26, 716–723. doi: 10.1021/jf60217a041
Sakai, K., Okada, M., and Yamaguchi, S. (2022). Decolorization and detoxication of plant-based proteins using hydrogen peroxide and catalase. Sci Rep 12. doi: 10.1038/s41598-022-26883-8
Saavedra Isusi, G. I., Pietsch, V., Beutler, P., Hoehne, S., and Leister, N. (2023). Influence of rapeseed oil on extruded plant-based meat analogues: assessing mechanical and rheological properties. PRO 11:1871. doi: 10.3390/pr11071871
Santamaria, M., Montes, L., Garzon, R., Moreira, R., and Rosell, C. M. (2022). Unraveling the impact of viscosity and starch type on the in vitro starch digestibility of different gels. Food Funct. 13, 7582–7590. doi: 10.1039/d2fo00697a
Schirmer, M., Höchstötter, A., Jekle, M., Arendt, E., and Becker, T. (2013). Physicochemical and morphological characterization of different starches with variable amylose/amylopectin ratio. Food Hydrocoll. 32, 52–63. doi: 10.1016/j.foodhyd.2012.11.032
Schreuders, F. K. G., Sagis, L. M. C., Bodnár, I., Erni, P., Boom, R. M., and van der Goot, A. J. (2021). Mapping the texture of plant protein blends for meat analogues. Food Hydrocoll. 118:106753. doi: 10.1016/j.foodhyd.2021.106753
Seetapan, N., Raksa, P., Limparyoon, N., Srirajan, S., Makmoon, T., Israkarn, K., et al. (2023). High moisture extrusion of meat analogues using mung bean (Vigna radiata L.) protein and flour blends: investigations on morphology, texture and rheology. Int J Food Sci Technol 58, 1922–1930. doi: 10.1111/ijfs.16334
Sha, L., and Xiong, Y. L. (2020). Plant protein-based alternatives of reconstructed meat: science, technology, and challenges. Trends Food Sci. Technol. 102, 51–61. doi: 10.1016/j.tifs.2020.05.022
Shevkani, K., Singh, N., Kaur, A., and Rana, J. C. (2015). Structural and functional characterization of kidney bean and field pea protein isolates: a comparative study. Food Hydrocoll. 43, 679–689. doi: 10.1016/j.foodhyd.2014.07.024
Singh, A., and Sit, N. (2022). Meat analogues: types, methods of production and their effect on attributes of developed meat analogues. Food Bioprocess Technol. 15, 2664–2682. doi: 10.1007/s11947-022-02859-4
Sołowiej, B., Dylewska, A., Kowalczyk, D., Sujka, M., Tomczyńska-Mleko, M., and Mleko, S. (2016). The effect of pH and modified maize starches on texture, rheological properties and meltability of acid casein processed cheese analogues. Eur. Food Res. Technol. 242, 1577–1585. doi: 10.1007/s00217-016-2658-4
Tang, Q., Roos, Y. H., and Miao, S. (2024). Structure, gelation mechanism of plant proteins versus dairy proteins and evolving modification strategies. Trends Food Sci Technol 147. doi: 10.1016/j.tifs.2024.104464
Taghian Dinani, S., Broekema, N. L., Boom, R., and van der Goot, A. J. (2023). Investigation potential of hydrocolloids in meat analogue preparation. Food Hydrocoll. 135:108199. doi: 10.1016/j.foodhyd.2022.108199
Tan, M., Nawaz, M. A., and Buckow, R. (2023). Functional and food application of plant proteins–a review. Food Rev. Intl. 39, 2428–2456. doi: 10.1080/87559129.2021.1955918
Tang, Q., Roos, Y. H., and Miao, S. (2023). Plant protein versus dairy proteins: a pH-dependency investigation on their structure and functional properties. Food Secur. 12:368. doi: 10.3390/foods12020368
Wang, R. M., Xie, Q. T., Wang, S. Y., Chen, P., He, T. S., and Zhang, B. (2022). The structural, functional and digestive characteristics of acorn starch after combined debranching and heat–moisture treatment and their relationships. Int. J. Food Sci. Technol. 57, 7622–7633. doi: 10.1111/ijfs.16098
Webb, D., Dogan, H., Li, Y., and Alavi, S. (2023). Use of legume flours and fiber for tailoring structure and texture of pea protein-based extruded meat alternatives. J Food Sci 88. doi: 10.1111/1750-3841.16397
Wittek, P., Karbstein, H. P., and Emin, M. A. (2021). Blending proteins in high moisture extrusion to design meat analogues: rheological properties, morphology development and product properties. Food Secur. 10:1509. doi: 10.3390/foods10071509
Xiong, Y. L. (2023). Meat and meat alternatives: where is the gap in scientific knowledge and technology? Ital J Anim Sci 22, 482–496. doi: 10.1080/1828051X.2023.2211988
Yang, C., Zhong, F., Douglas Goff, H., and Li, Y. (2019). Study on starch-protein interactions and their effects on physicochemical and digestible properties of the blends. Food Chem 280, 51–58. doi: 10.1016/j.foodchem.2018.12.028
Yuliarti, O., Kiat Kovis, T. J., and Yi, N. J. (2021). Structuring the meat analogue by using plant-based derived composites. J. Food Eng. 288:110138. doi: 10.1016/j.jfoodeng.2020.110138
Zhang, T., Jiang, B., Mu, W., and Wang, Z. (2009). Emulsifying properties of chickpea protein isolates: Influence of pH and NaCl. Food Hydrocoll 23, 146–152. doi: 10.1016/j.foodhyd.2007.12.005
Zhang, B., Qiao, D., Zhao, S., Lin, Q., Wang, J., and Xie, F. (2021). Starch-based food matrices containing protein: recent understanding of morphology, structure, and properties. Trends Food Sci. Technol. 114, 212–231. doi: 10.1016/j.tifs.2021.05.033
Keywords: meat alternatives, plant proteins, starch, structuring process, sustainable food technology
Citation: Baig MA, Mostafa H, Sivapragasam N, Aslam R, Zhou W and Maqsood S (2024) Investigating the role of starch in the structuring of meat alternatives from mung bean and pea protein isolates via heat-induced gelation. Front. Sustain. Food Syst. 8:1473663. doi: 10.3389/fsufs.2024.1473663
Edited by:
Anwar Ali, Hong Kong Polytechnic University, Hong Kong SAR, ChinaReviewed by:
Khalid Gul, University of Leeds, United KingdomGulden Goksen, Tarsus University, Türkiye
Copyright © 2024 Baig, Mostafa, Sivapragasam, Aslam, Zhou and Maqsood. This is an open-access article distributed under the terms of the Creative Commons Attribution License (CC BY). The use, distribution or reproduction in other forums is permitted, provided the original author(s) and the copyright owner(s) are credited and that the original publication in this journal is cited, in accordance with accepted academic practice. No use, distribution or reproduction is permitted which does not comply with these terms.
*Correspondence: Sajid Maqsood, sajid.m@uaeu.ac.ae