- 1Division of Environment Science, ICAR-IARI, New Delhi, India
- 2Centre for Ecology and Hydrology, Penicuik, Scotland, United Kingdom
Introduction: Climate change significantly impacts food production by influencing crop growth and soil processes. Rising atmospheric CO2 levels and temperatures may affect reactive nitrogen losses from cultivated soils. This study aimed to quantify the effects of nitrification and urease inhibitors on reactive nitrogen losses from wheat soils in the context of elevated CO2 and temperature interactions.
Methods: An experiment was conducted in open top chambers for two consecutive years to quantify the effect of nitrification and urease inhibitors on ammonia (NH3), and nitrous oxide (N2O) emissions in wheat under elevated carbon dioxide (EC), elevated temperature (ET) and their interaction (ECT). The carbon dioxide (CO2) concentration ranged from 552 to 568 ppm in the EC treatment, while the average temperature was 2.1–2.5°C higher in ET treatment than ambient (AMB).
Results and discussion: The N2O–N emission increased under ECT than ambient. Use of neem oil coated urea (NOCU) reduced the N2O–N emission by 10.3%, whereas, Limus coated urea reduced N2O–N emission by 14% as compared to prilled urea treatment under ECT. NH3–N emission from wheat soil also increased under ECT treatment as compared to AMB. Application of N through Limus, reduced NH3–N emission from wheat by 35.7–36.8% when compared with NH3–N emission from prilled urea ECT condition. Elevated temperature reduced grain weight by 7.6%. The grain N content reduced by 10.9% with prilled urea under ECT. The application of NOCU and Limus increased grain N by 6 and 9%, respectively, as compared to urea under ECT interaction. The application of nitrification and urease inhibitors may reduce reactive nitrogen losses and enhance nitrogen use efficiency under future climatic conditions.
Highlights
• N2O–N and NH3–N emission increased while grain N decreased under elevated CO2 and temperature treatment.
• Use of neem oil coated urea (NOCU) reduced the N2O–N emission by 10.3%, whereas, Limus coated urea reduced N2O–N emission by 14% than prilled urea.
• Application of N through Limus, reduced NH3–N emission by 35.7–36.8% than prilled urea under elevated CO2 and temperature condition.
• Nitrification and urease inhibitors, NOCU and LIMUS will reduce the N losses and improve the N use efficiency in wheat under future climatic conditions.
1 Introduction
Carbon dioxide and temperature are important factors for the growth and development of crops. The atmospheric carbon dioxide (CO2) concentration has increased from 350 ppm in 1990s to a record concentration of 417 ppm in 2022 (National Oceanic and Atmospheric Administration, 2023). The sixth assessment report of the Inter-Governmental Panel on Climate Change (IPCC) reported that with an increase in GHG emissions, the earth’s temperature will rise by 3.3–5.7°C by the end of this century (IPCC, 2021). Increased CO2 concentration has a fertilization effect on crops, whereas higher temperatures shorten the crop growth period and may decrease the crop yields (Chakrabarti et al., 2013; Singh S.D. et al., 2013). Global average atmospheric temperatures must stay below the 1.5°C threshold compared to pre-industrial levels to mitigate the risks of severe climate hazards to our ecosystems (IPCC, 2021). Changing climate will not only affect crop yields but will also adversely affect important soil processes linked to soil fertility, thereby threatening food security (Brevik, 2013).
Climate change has the potential to alter the carbon and nitrogen cycle in managed systems causing positive or negative feedbacks (Bardgett and Van Der Putten, 2014). Plant growth might be affected, as changing climate will impact the N cycling rates, influencing the supply of N in the soil as well as plant N demand, thereby, causing a nutrient imbalance (Wang et al., 2019; Bhatia et al., 2021). Although carbon concentration in the plant tissues increases at elevated CO2 levels, the concentration of other elements in the plants including nitrogen may get diluted (Gifford et al., 2000; Yadav et al., 2021). Increased crop growth under elevated CO2 concentration may lead to greater partitioning of photosynthates to the underground plant parts (Daepp et al., 2000) leading to increased belowground C input due to root turnover, and changes in litter quantity, quality and decomposability (Norby et al., 2001; Bhatia et al., 2012), thereby affecting the soil carbon and nitrogen status (Daripa et al., 2014; Maity et al., 2020). Changes in belowground soil carbon and nitrogen under elevated CO2 and temperature impact the N2O emissions from cropped fields (van Groenigen et al., 2013). Increase in CO2 and temperature may accelerate N cycling between plant and soil.
Wheat (Triticum aestivum L.) is an important cereal crop forming the backbone of India’s food security (Gupta et al., 2015), however, its productivity may be significantly affected by the increasing temperatures due to the changing climate (Asseng et al., 2015). Researchers have reported that elevated CO2 and elevated temperatures may also adversely affect the quality of wheat (Myers et al., 2014; Dubey et al., 2020). Increased N fertilizer application may counter the decline in wheat productivity and quality (Chakrabarti et al., 2020). Nitrogen is one of the major nutrients supporting growth and yield of crops (Jiang et al., 2004), and the application of higher rates of N fertilizer has often been suggested as an adaptation option for countering the impacts of climate change (Pathak et al., 2014). Urea is the most preferred fertilizer applied to cropped soils globally (180 Tg), however, the N use efficiency (NUE) is only 10–50% of the applied N (Sutton et al., 2011). However, the increased application of nitrogenous fertilizers for improving crop productivity, may be accompanied by an increased N2O emission, especially under future climate change scenarios (Bhatia et al., 2010). Increased N fertilizer application during wheat cultivation may result in increased nitrous oxide (N2O) emission from the soil. Nitrous oxide is an important greenhouse gas (GHG) with 265 times more global warming potential than CO2 (IPCC, 2021), and N fertilized soils are the largest source of N2O emissions. Half of the global N fertilizer is consumed by the cereal crops of rice, wheat and maize (Ladha et al., 2016). Nitrous oxide is produced in the cropped soil during the microbial processes of nitrification and denitrification of native N and applied N fertilizer (Fu et al., 2020). Nitrification is carried out in two steps, the ammonia-oxidizers limit the first step of nitrification by converting NH4+ into NO2, and then, nitrite-oxidizers convert NO2− into NO3− (Weiske et al., 2001). Another major pathway of N loss from soil is the ammonia (NH3) volatilization which is directly responsible for air pollution and accelerates formation of secondary inorganic aerosols, threatening public health (Xu et al., 2022). Globally, NH3 volatilization comprises around 50% of the total N losses from cultivated land (Pan et al., 2016). The most commonly used inorganic fertilizer urea is rapidly hydrolyzed by urease enzyme to NH3, which gets easily volatilized to the atmosphere (Fu et al., 2020), thereby reducing the nitrogen use efficiency of the applied fertilizer. Beyond economic losses, NH3 emission adversely affect ecosystems, contributing to issues like eutrophication in aquatic systems, acidification of terrestrial environments, and declining biodiversity (Giannadaki et al., 2018). Although NH3 itself is not a greenhouse gas, however its volatilization and subsequent re-deposition results in indirect N2O emissions (IPCC, 2021), indicating positive feedback to climate change (Wang B. et al., 2021).
Management of applied N using enhanced efficiency fertilizers with urease and nitrification inhibitors can reduce both N2O and NH3 emissions (Engel et al., 2017; Thilakarathna et al., 2020). Urease inhibitors slow down the hydrolysis of urea to ammonium, whereas, nitrification inhibitors suppress the activity of the ammonia monooxygenase enzyme, slowing the conversion of ammonium to nitrate, thereby reducing N2O losses and improving the NUE (Fu et al., 2020). Nitrification inhibitors such as nitrapyrin and 3,4-dimethylpyrazole phosphate have been reported to reduce the N2O losses by up to 50% (Lin et al., 2017; Thilakarathna et al., 2020). Urease inhibitor like N-(nbutyl) thiophosphoric triamide (NBPT) showed NH3 mitigation potential up to 70% accompanied with enhanced yield and N use efficiency (Sha et al., 2023). According to Chakraborty et al. (2023), urease inhibitor alone and in combination with nitrification inhibitor was effective in mitigating N2O and NH3 emissions. Nitrification inhibitors also enhance the NUE by reducing the denitrification losses of N due to reduced nitrate availability (Aulakh et al., 2001). Plant-based natural nitrification inhibitors such as Neem oil are known to affect both ammonium oxidizing bacteria and nitrite-oxidizing bacteria by reducing their abundance and/or retarding their activity (Norton and Ouyang, 2019). Neem oil coated urea is an eco-friendly slow-release fertilizer with nitrification inhibition properties, which reduces N2O emissions from cropped soils (Malla et al., 2005; Cowan et al., 2021). Limus is a combination of two urease inhibitors, N-(n-butyl) thiophosphorictriamide (NBPT, 75%) and N-(n-propyl) thiophosphorictriamide (NPPT, 25%) and application of Limus coated urea has been reported to decrease N losses, saving 20% of fertilizer N input and improving the N use efficiency in wheat (Li et al., 2015). A high NUE is valuable as a means of reducing pollution, and it also reduces the amount of fertilizer needed and improves profit for the farmers.
In addition to nitrogen management practices, climatic parameters such as atmospheric CO2 concentration and temperature are key factors controlling N2O emissions from cropped soils (Cai et al., 2012). There are a few reports on the effects of elevated CO2 and elevated temperature on soil properties and greenhouse gas emissions (Bhattacharyya et al., 2013; van Groenigen et al., 2013; Dey et al., 2019; Pramanik et al., 2018), however, the interactive effect of elevated CO2 and temperature on the emission of greenhouse gases has been less evaluated. Process-based ecosystem models applied at regional and continental scales have estimated that net N2O emissions could increase in the future under elevated CO2 and temperatures (Xu et al., 2012; Jiang et al., 2020). However, there are no reports on the impact of enhanced efficiency fertilizers coated with nitrification and urease inhibitors under the elevated CO2 and temperature interactive conditions on N2O emissions.
In a district wise projected climate atlas for India, an increase in the winter (rabi) minimum temperature by 1.5° and 2°C is projected for 18 and 63% of the districts under the RCP 4.5 and RCP 8.5 scenarios, respectively (CSTEP, 2022). Hence, the current investigation focussed on studying the interactive effect of elevated CO2, and temperature increase of 1.5–2.0°C on NH3 and N2O emission, as well as plant and soil N in spring wheat grown in the rabi (winter) season with the application of urea coated with nitrification and urease inhibitors. No studies have been reported on the effect of these inhibitors under future climatic condition. The hypothesis tested was that whether the use of the enhanced efficiency fertilizers can mitigate the reactive N losses while improving the use efficiency of the applied N fertilizer. The objective of this study was to quantify the effects of applying nitrification and urease inhibitors on NH3 and N2O emissions, grain nitrogen content, and nitrogen use efficiency in wheat under elevated atmospheric CO2 concentrations, increased temperatures, and their interactions.
2 Materials and methods
2.1 Experimental details
The experiment was conducted on the farms of the ICAR-Indian agricultural research institute (IARI), New Delhi, India. The experimental site is located at 28°35′N and 77°12′E at an altitude of 228 m above the mean sea level. The region has a semi-arid and subtropical climate with an average annual rainfall of around 750 mm, approximately 80% of which occurs between June and September months. The mean annual maximum and minimum temperatures are 35 and 18°C, respectively. The experimental soil was non saline, mildly alkaline with pH of 7.9. The soil contained 0.5% organic carbon and 205 kg ha−1 of available N (for plant uptake).
The experiment was carried out for 2 years, i.e., 2017–18 and 2018–19 by growing wheat in crates in open top chambers (OTC) under four climate treatments. The climate treatments comprised of ambient (AMB), elevated temperature (ET), elevated CO2 (EC) and elevated CO2 plus elevated temperature (ECT). Each climate treatment had two replicate OTCs in a completely randomized design. In each OTC, wheat was grown with four nitrogen treatments with three replicates of each fertilizer treatment. Two levels of CO2 concentration were maintained inside the OTCs, i.e., ambient and elevated (560 ± 25 ppm). Each OTC (3 m diameter, 2.5 m height) was constructed with an octagonal galvanized steel structure made of galvanized iron pipes. The OTC was covered with polyethylene (120 micron thickness with more than 85% light transmittance), while the top was kept open to maintain near natural conditions. Two temperature levels were created inside the OTC by keeping the top fully open (ambient) and keeping the top partially covered which elevated the temperature by 2.1°C and 2.5°C in the 2 years of the study (Figure 1). On one side of each OTC, an auto closing door allowed access to the inside. To elevate the atmospheric CO2 to the target level of 550 ppm, the OTCs were fumigated with 100% pure commercial CO2 from 30 kg capacity cylinders for (10-h per day 8 am to 6 pm), during the wheat growth period. The CO2 supply in each OTC was regulated using solenoid valves. Pure CO2 was diluted with normal air using an air compressor and then pumped into CO2 circulating pipes (Bhatia et al., 2013). Inside the OTC, the CO2 was distributed using a flexible polyvinyl chloride tube (diameter of 35 mm), located around the sidewalls with several emission holes and was adjusted to be above the canopy level, during the crop growth period. The air from the middle of each OTC was sampled using a PU tubing, and the CO2 concentration was measured using a CO2 analyzer (Fuji, Japan). The target concentration of CO2 was maintained with solenoid valves (Abebe et al., 2016). Air temperature and relative humidity sensors were installed at 1 m above the soil surface, and connected to a data-logger (CR 1000, Campbell Scientific, USA) to monitor the climatic parameters inside the OTCs. To maintain similar conditions of air turbulence, two fans (12 V) were installed inside each OTC at a height of 1 m on opposite walls.
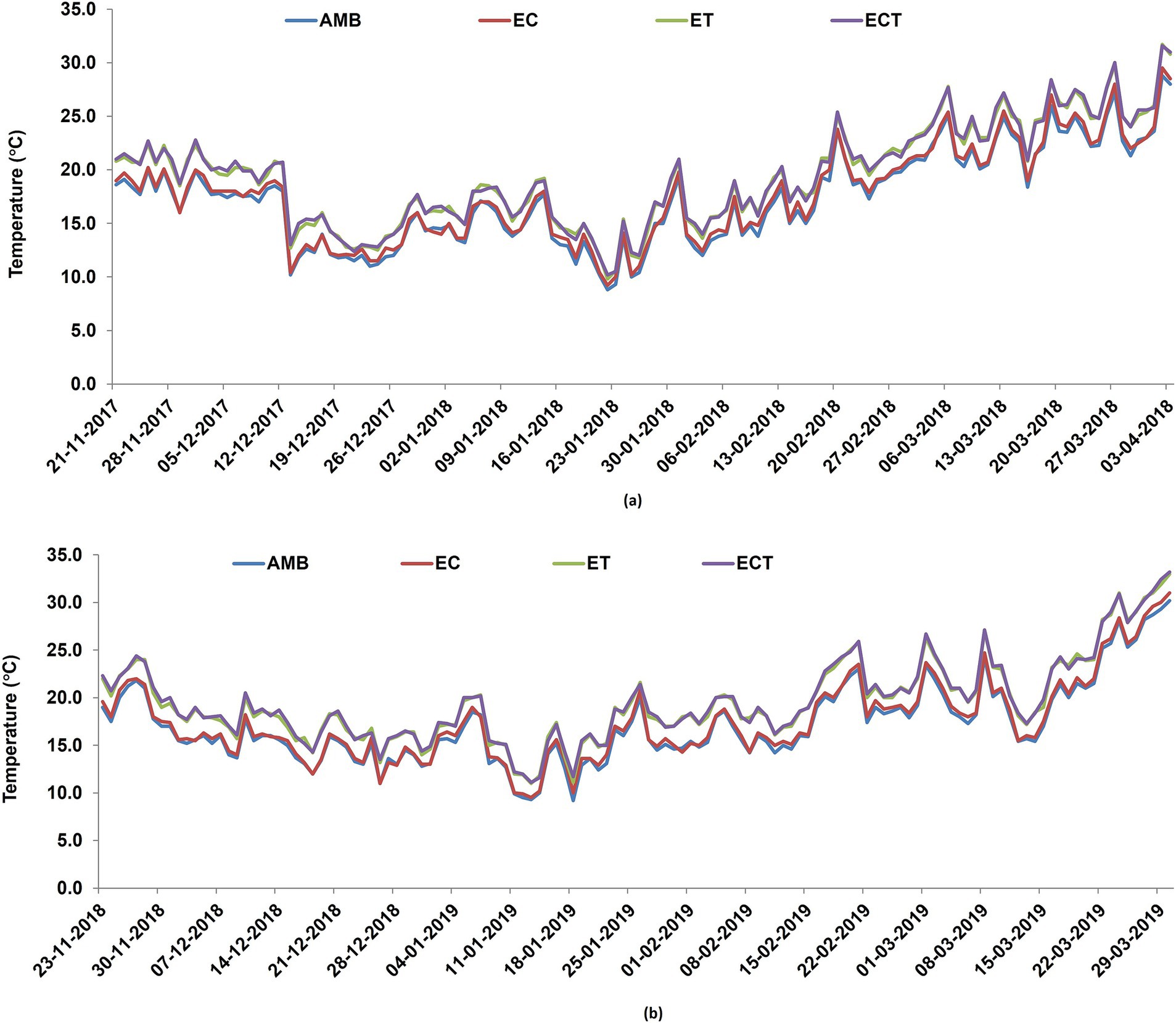
Figure 1. Canopy air temperature during the crop growth period inside the OTCs under ambient conditions, elevated CO2, elevated temperature and elevated CO2 and temperature interaction in (A) first and (B) second year of study.
Wheat variety HD 2967 was grown inside the OTCs during both the years in PVC boxes (0.25 m2 area). The agronomic practices followed in the experiment were similar to those commonly practiced by the farmers in this region. The crates were filled with 50 kg of soil, and wheat seeds were sown in the crates in the second week of November. Phosphorus (60 kg P2O5 ha−1) and Potassium (40 kg K2O ha−1) were applied at the time of wheat sowing. Four nitrogen treatments, i.e., no N (control), N applied through prilled urea, N applied through neem oil (350 ppm) coated over prilled urea (NOCU), and N applied through Limus coated urea (BASF, India) (Table 1) were taken for the experiment. In all the fertilized plots 120 kg N ha−1 (Recommended dose of N) was applied in three splits, i.e., half as basal, and the remaining half in two equal splits at tillering and flowering stages of the crop. Five irrigations were applied to the crop at 0, 21, 45, 65 and 92 days after sowing (DAS). To reduce the effect of environmental heterogeneity within the chambers, plants were randomized within the chambers, on weekly basis throughout the experiment. The light intensity inside and outside the OTCs was measured using a portable light meter (Metravi, 1332), and the temperature and relative humidity were recorded daily at 10.00 and 15.30 h (Singh S. et al., 2013).
2.2 Analysis of gas samples and estimation of global warming potential
Gas samples of N2O were collected from three replicates of each treatment using the closed-chamber technique (Bhatia et al., 2005). Chambers made of 6 mm transparent acrylic sheet with dimension of 15 cm × 15 cm × 30 cm (length × width × height) were used to measure GHG emission. Chambers were placed on aluminum channels that were gently inserted into the soil to a depth of 5 cm. Gas samples from inside the chamber were collected using a 50 mL hypodermic syringe immediately after placing the chamber at 0 min, and then subsequently at 15- and 30-min’ intervals without removing the chambers. The temperature inside each close chamber was recorded during the gas sampling. Collected gas samples were brought to the laboratory and analyzed for N2O using a gas chromatograph fitted with an electron capture detector, within 2 h of sampling using the following Equation 1 (Malyan et al., 2019):
Where, F is the flux of N2O emitted (ug m2 h−1), ρ is the density of air (g m−3), V is the volume of the chamber (m3), and A is the soil surface area covered by the chamber (m2). Δc/Δt was the rate of increase of N2O gas concentration (ug m−2 h−1) inside the chamber during sampling.
Estimation of total N2O emission during the cropping season was performed by successive linear interpolation of average emission on the sampling days assuming that emission followed a linear trend during the periods when no sample was taken (Malyan et al., 2021a). The Global warming potential (GWP) was calculated by multiplying the seasonal N2O emission (g N2O ha−1) with its global warming potential of 265 (IPCC, 2021). GHG intensity was calculated by dividing GWP by the grain yield and expressed as kg CO2 eq. kg yield−1 (Malyan et al., 2021b).
2.3 Measurement of ammonia volatilization
The measurement of ammonia volatilization was carried out using chamber method (Cowan et al., 2021; Bhatia et al., 2023). Transparent static chambers measuring 30 × 15 × 15 cm (length × width × height) were placed on soil base frames inserted in between the rows of wheat plants in each of the treatment replicates in each OTC for 1 h. The ammonia gas volatilized inside the chamber was bubbled through 0.01 N boric acid solution containing mixed indicator (methyl red and bromocresol green) using a suction pump with a flow rate of 3 L min−1 for 2.5 min. The volatilized ammoniacal N was determined by the titration of boric acid solution with 0.001 N sulfuric acid. The volatilized NH3–N was determined by titrating the boric acid solution with 0.001 N H2SO4 until the color changed back to wine red. The calculation of ammonia flux was done using Equation 2,
where N is NH3–N in mg m−2, S is the difference in amount (ml) of 0.001 N sulfuric acid consumed by the N treatment and ambient air, the constant 28.014 is the molecular weight of NH3–N (g mol−1) multiplied by 2 (two moles of NH3 react with one mole of sulfuric acid) and A is the soil area covered by the chambers (m2) and T is the time for which the chamber was placed on the base frame.
2.4 Soil and plant sample collection and analysis
Soil samples were collected at the flowering stage from all the replicates of each treatment combination. Available nitrogen which is the sum of inorganic ammonium and nitrate N, and readily mineralizable organic N in soil was estimated by Subbiah and Asija (1956) method. The microbial biomass carbon (MBC) in soil samples collected at the flowering stage was analyzed as per the method given by Jenkinson and Powlson (1976). Both fumigated and unfumigated soil samples were extracted with 0.5 M potassium sulfate (K2SO4). The extracts were treated and digested under refluxing condition. The cooled samples were then titrated with ferrous ammonium sulfate solution.
Plant samples were collected after harvesting of the crop. The wheat grain was separated from the straw biomass and the samples were dried in an oven at 65 ± 2°C for 72 h and grain and straw weights were recorded. The N concentration in the grain samples was determined as given by Jackson (1973). Grain N uptake (g m−2) was then calculated by multiplying the grain weight by the grain N concentration as per Cowan et al. (2021). Recovery efficiency of N (REN) was calculated by dividing the difference between plant N in fertilized and control treatments by the amount of fertilizer N applied (Ditz, 1988).
2.5 Statistical and correlation analysis
The experiment was a factorial completely randomized design with 16 treatment combinations having six replicates each. Three factorial (carbon dioxide level, temperature and fertilizer) analysis of variance (ANOVA) was performed using SAS (ver. 9.3) statistical package (SAS Institute Inc., CA, USA) to check if the differences in the replicate means of N2O, GWP, yield, plant N, MBC and soil N were statistically significant. When the ANOVA was significant at 5% level of significance, the treatment means were compared using the Turkey’s post-hoc test. The correlation between N2O–N emission and different soil and plant parameters was represented as a scatter plot.
3 Results
3.1 Climatic variables during the crop growth period
During the crop growth period, the mean CO2 concentration inside the OTCs with elevated CO2 (EC) treatment ranged from 558 to 565 ppm during the 2 years. In contrast, in the chamber control ambient treatment, the mean CO2 concentration inside the OTCs was 403–406 ppm (Table 1). Maximum and minimum temperatures were recorded every day inside the different OTCs. The daily temperature data was then averaged for the cropping season. The seasonal mean temperature during the crop growth period was 16.9°C and 16.8°C, respectively, in the first and second year of study. The results showed that seasonal mean temperature inside the partially covered OTCs was higher than that inside the fully open OTCs. The mean temperature in the elevated temperature (ET), and elevated CO2 plus elevated temperature (ECT) treatments was higher than that in the ambient (AMB) treatment by 2.1°C and 2.5°C, respectively, in the first and second years (Figures 1A,B).
Soil temperatures were 0.5–0.8°C higher in the elevated temperature OTCs than the ambient chamber control. The mean daily values of soil temperatures did not differ significantly between ET and ECT treatments, but were significantly higher (p < 0.05) than those of AMB.
3.2 Grain and straw weight of wheat as affected by elevated CO2 and temperature
The grain weight of wheat increased under EC, whereas under ET it was significantly reduced (p ≤ 0.01) as compared with AMB. The temperature rise reduced the mean grain weight by 7.6% as compared to the ambient treatment (Table 2), however, the elevated concentration of CO2 was able to compensate the yield loss in the ECT treatment. There was no significant yield loss in the interaction treatment as compared with AMB. Elevated temperature significantly lowered the grain weight (p < 0.01) in both unfertilized and fertilized treatments as compared to AMB. Grain weight was significantly higher with Limus application than with prilled urea (p < 0.01) under ET and ECT.
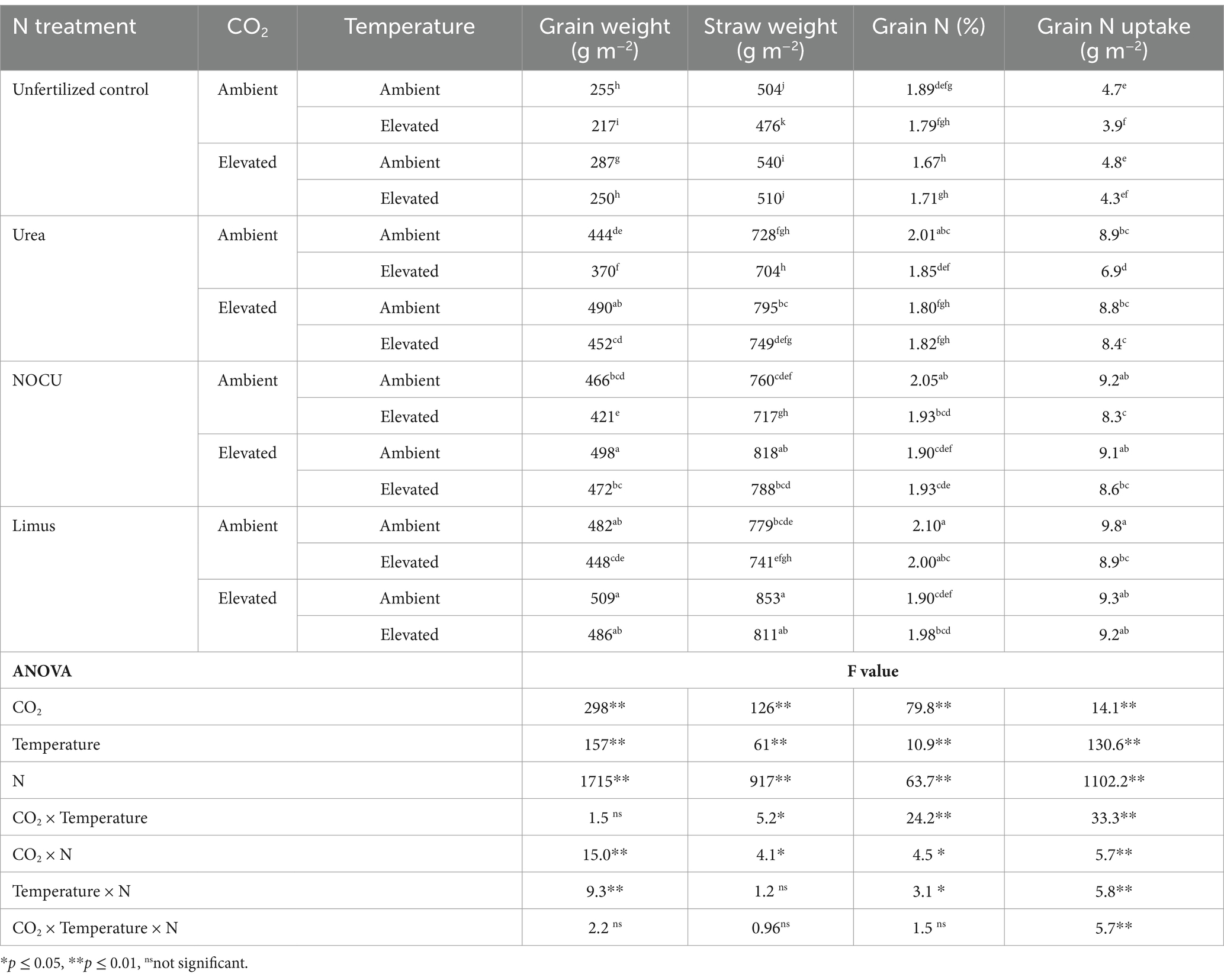
Table 2. Effect of elevated CO2, temperature and N treatments on soil available N, microbial biomass carbon and soil urease activity at flowering stage (mean of 2 years) [Means with at least one letters in common are not statistically significant (p ≤ 0.05)].
The straw weight of wheat was significantly reduced under increased temperature in all N treatments (Table 2), Straw weight increased in EC by 9.2, 7.6 and 9.4% as compared to ambient in prilled urea, NOCU and Limus, respectively. (Table 2). The application of N through Limus further increased the straw weight of wheat as compared to prilled urea under EC and ECT treatments.
3.3 Plant nitrogen under elevated CO2 and temperature
The nitrogen concentration in wheat grains was observed to be higher in the N applied treatments than in the unfertilized control (Table 2). Grain N significantly (p ≤ 0.01) decreased in EC, ET and the interaction (ECT) compared with ambient (AMB) with prilled urea application. Under ECT, it reduced by 10.9% compared to AMB with prilled urea application. When nitrogen was applied through NOCU and Limus the grain N was significantly (p ≤ 0.01) higher than prilled urea under ECT. The application of NOCU and LIMUS increased the grain N by 6 and 9%, respectively, as compared with prilled urea under the ECT interaction.
The mean grain N uptake by wheat crop ranged from 3.9 to 9.8 g m−2 in the different treatments (Table 2). Nitrogen uptake by wheat grains was significantly lower (p ≤ 0.01) under ET in the prilled urea treatment. However, grain N uptake under ECT interaction was at par with the ambient (AMB) treatment. The application of N through Limus led to significantly higher (p ≤ 0.01) grain N uptake in ET and ECT treatments than with prilled urea application. The three-way interaction of elevated CO2, temperature and fertilizer was significant (p ≤ 0.01) for grain N uptake by wheat.
3.4 Soil properties under elevated CO2 and temperature
Soil available N at the flowering stage ranged from 123 to 315 kg ha−1 (Table 3). Soil available N was significantly (p ≤ 0.05) higher under ambient climate in all fertilized treatments. The soil available N decreased significantly (p ≤ 0.05) under elevated CO2 and temperature interaction (ECT) as compared with the ambient (AC) in prilled urea applied treatment. A significant difference (p ≤ 0.01) was observed in the soil available N in the different N treatments under the EC and ET. Significantly higher soil available N (p ≤ 0.01) was observed in Limus and NOCU treatment as compared with prilled urea application under ECT interaction.
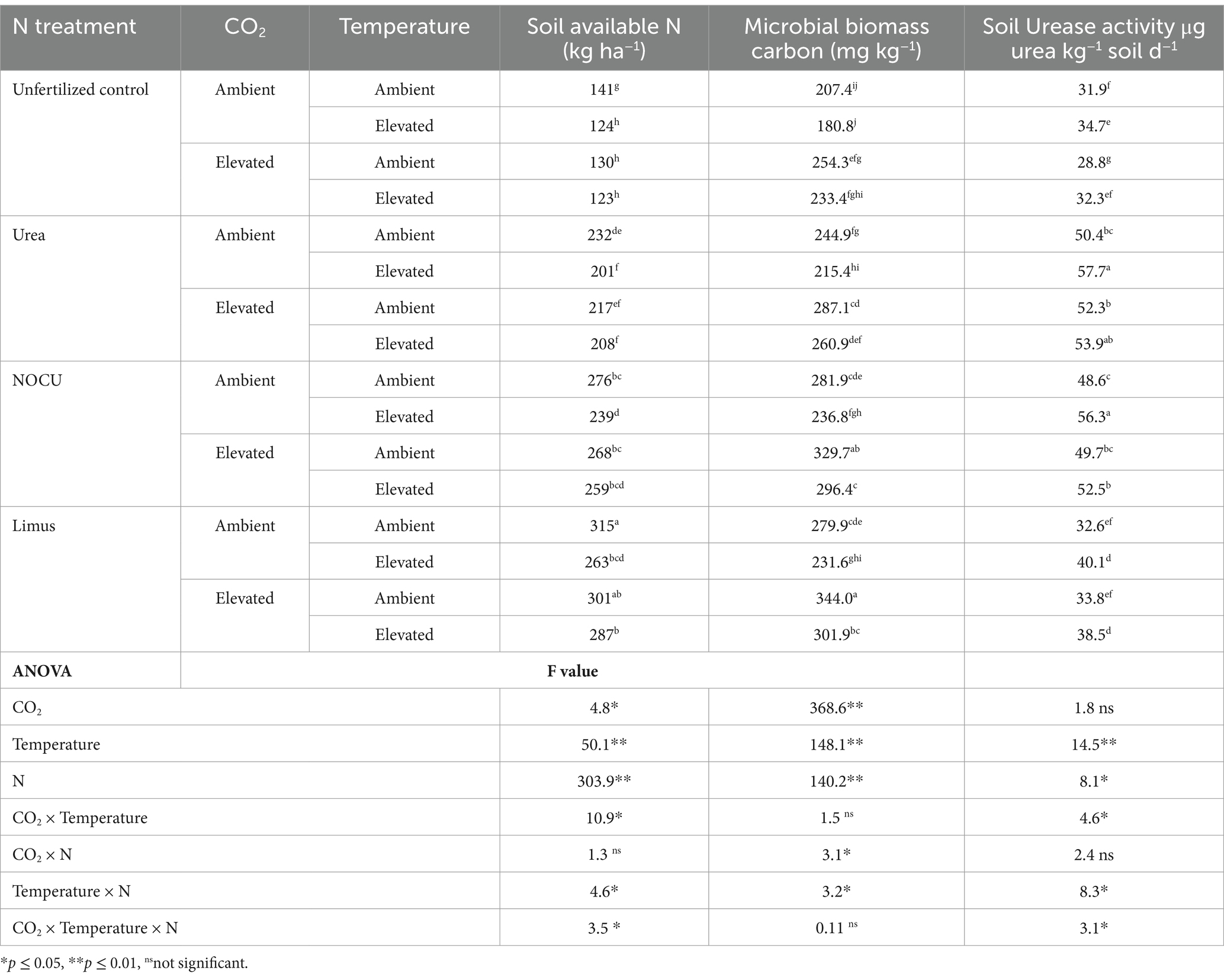
Table 3. Effect of elevated CO2, temperature and N treatments on soil available N (flowering) and microbial biomass carbon (flowering) (mean of 2 years) [Means with at least one letters in common are not statistically significant (p ≤ 0.05)].
Soil MBC significantly increased (p ≤ 0.01) in EC, and decreased (p ≤ 0.01) under ET than under the ambient treatment (Table 3). However, in the ECT interaction, MBC of the soil was higher than that of the ambient in all N treatments. The application of N through NOCU and Limus had significantly (p ≤ 0.01) higher MBC in soil than prilled urea under ECT treatment.
The urease activity was significantly lower (p ≤ 0.05) in the Limus treatment as compared to urea and NOCU, in which the soil urease activity was found to be similar (Table 3). ET significantly increased the activity of soil urease (p ≤ 0.01) in all the fertilizer treatments. No significant change in soil urease was observed under elevated CO2 treatment.
3.5 Nitrous oxide emission under elevated CO2 and temperature
Nitrous oxide (N2O–N) emission in wheat crop ranged from 0.37 to 1.07 kg N ha−1 during first year and from 0.41 to 1.08 kg ha−1 during second year in different treatments (Figures 2A,B). N2O–N significantly increased (p ≤ 0.01) under ET compared with ambient in all the N treatments. No significant difference in N2O–N emission was observed under EC and ECT treatments. The N2O–N emission from soil significantly (p ≤ 0.01) increased in ECT over the ambient (AMB) in all N treatments. With prilled urea application, N2O–N emission increased significantly (p ≤ 0.01) by 11.5% under ECT as compared to ambient. Under ECT, when the N was applied though NOCU and Limus, the N2O–N emission was significantly lower (p ≤ 0.01) than that of prilled urea. It reduced by 10.3% in NOCU and by 14.0% in Limus as compared with prilled urea under ECT.
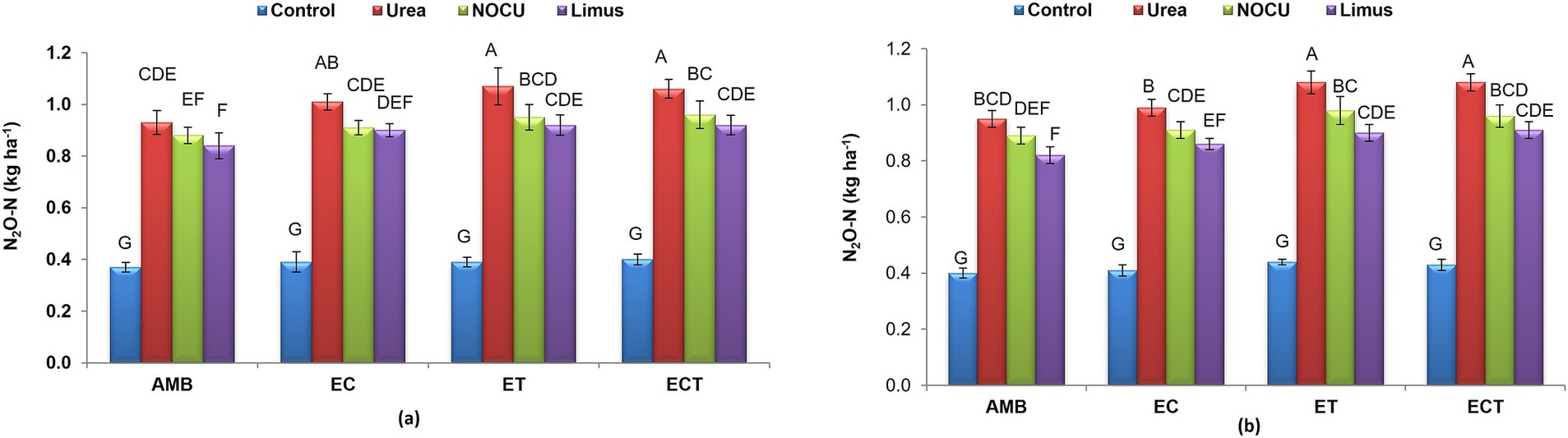
Figure 2. Nitrous oxide (N2O–N) emission in wheat under different treatments in (A) first and (B) second year of study.
3.6 Ammonia volatilization under elevated CO2 and temperature
The cumulative ammonia (NH3–N) volatilization ranged from 0.27 to 17.0 kg ha−1 during first year and from 0.28 to 15.7 kg ha−1 during second year in the different treatments (Figures 3A,B). NH3–N emission was significantly (p ≤ 0.01) higher in Urea and NOCU applied treatments than Limus applied treatment. In control treatment, NH3–N emission was least due to no N application. Elevated temperature and elevated CO2 plus temperature significantly increased NH3–N emission in urea applied treatments. In the first year, emission of NH3–N was highest (17.0 kg ha−1) in NOCU applied followed by urea applied treatment (16.7 kg ha−1) under ET condition. During the second year of study, highest NH3–N emission (15.7 kg ha−1) was observed in NOCU applied treatment under elevated CO2 and temperature condition. Application of N through Limus, reduced NH3–N emission from wheat by 35.7–36.8% than urea applied treatment under ECT condition.
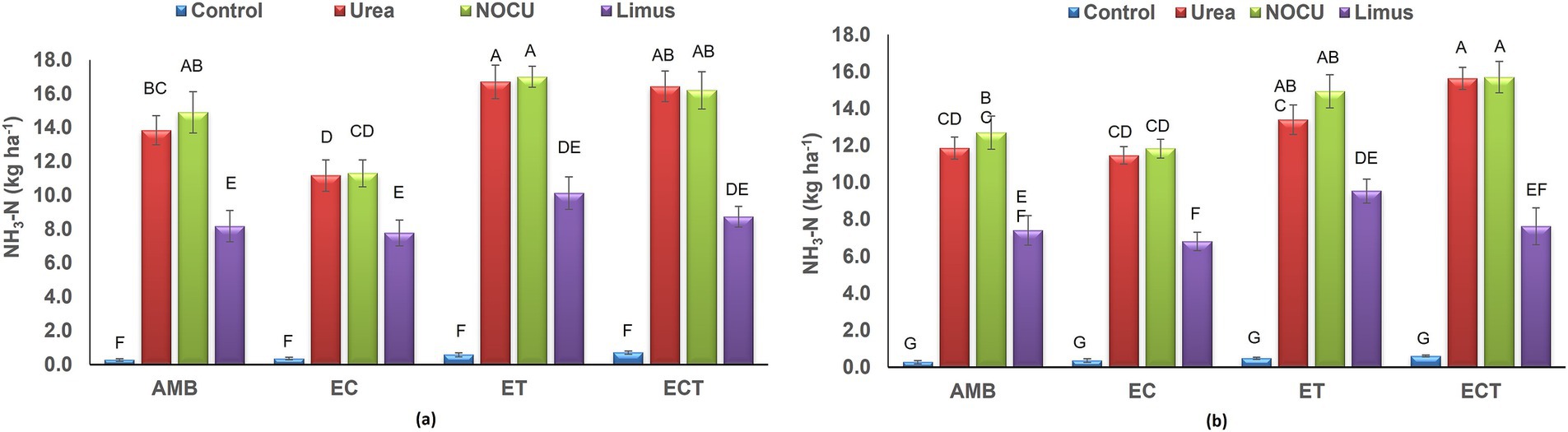
Figure 3. Cumulative ammonia (NH3–N) volatilization in wheat under different treatments in (A) first and (B) second year of study.
3.7 GHG intensity and recovery efficiency under elevated CO2 and temperature
GHG intensity of wheat significantly increased (p ≤ 0.01) under ET and ECT irrespective of N treatments (Table 4). Elevated CO2, temperature and application of N together significantly affected (p ≤ 0.05) the GHG intensity of the crop. Application of N through Limus and NOCU lowered the GHG intensity by 16.7% and by 9.3%, respectively, as compared with prilled urea application under ECT interaction.
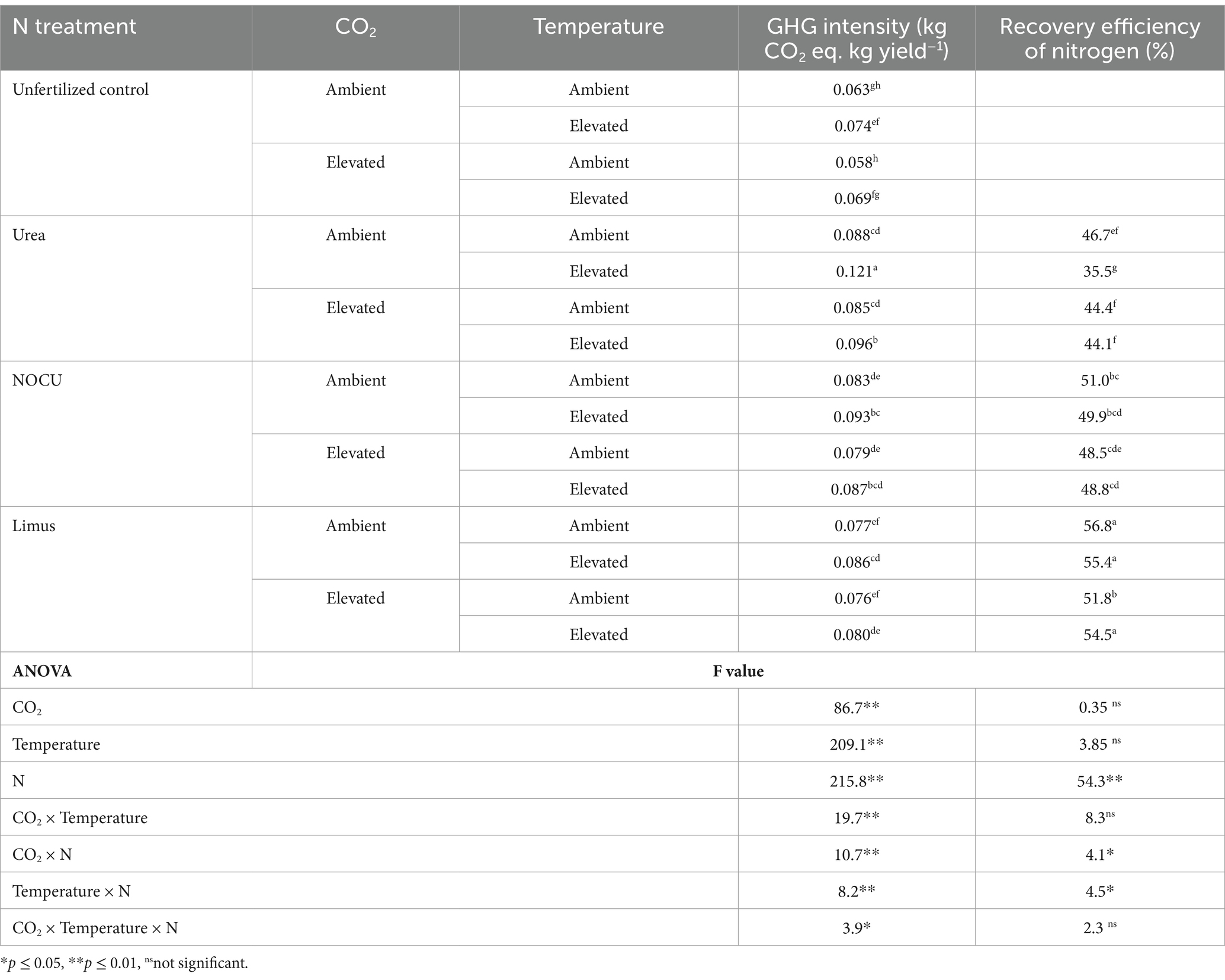
Table 4. Effect of elevated CO2, temperature and N treatments on GHG intensity and recovery efficiency of N (mean of 2 years) [Means with at least one letters in common are not statistically significant (p ≤ 0.05)].
Recovery efficiency of N in wheat ranged from 35.5 to 56.8% in the different treatments (Table 4). Under the ECT interaction treatment, the recovery efficiency of N was 54.5% with Limus, whereas was 44.1% with urea application. The recovery efficiency of N was significantly higher (p ≤ 0.05) with Limus than with prilled urea and NOCU application under EC, ET and ECT interaction treatments.
4 Discussion
4.1 Effect of elevated CO2 and temperature on N2O and NH3 emission
In our study significantly higher N2O was observed under EC, ET and ECT interaction in all the fertilized treatments (Table 3). No significant increase in N2O was observed in the unfertilized treatment under the different climate treatments. The highest emission of N2O–N was observed in the elevated CO2 climate treatments. NH3 emission was also found to increase in ET and ECT treatment than AMB with urea and NOCU application. After fertilizer application, urea granules absorb water and undergo hydrolysis to NH4+(Sommer et al., 2004), and is accompanied by a temporary increase in soil pH leading to increased NH3 volatilization during the first week after N fertilizer application (Mariano et al., 2019). Increasing temperature in ET increased the potential for NH3 loss due to a shift of the NH3/NH4+equilibrium toward NH3 (Fan et al., 2011) and also due to inhibition of nitrification (He et al., 1999), whereas under elevated CO2 plant N assimilation was stimulated, which offset the possible increase in NH3 volatilization, as more soil N was taken up by the plant, thus reducing NH3 loss. Under ECT interaction too higher NH3 loss was observed as compared to AMB, due to increased urease activity. Urease activity of soil was significantly positively correlated with NH3 emission (Figure 4D). Nitrous oxide emission from soil is mainly attributed to the nitrification and denitrification processes both of which are enhanced by the availability of N in soil (Gupta et al., 2016). We observed a significant decrease in the soil available N under both, EC and ECT treatments, probably due to higher N loss as NH3 and N2O. Maity et al. (2020) had also reported a decrease in soil available N under elevated CO2. Higher N2O emission under elevated CO2 may be attributed to an increase in the denitrification of available soil N (Wu et al., 2017). A meta-analysis by van Groenigen et al. (2011) found that higher soil moisture associated with elevated CO2 led to increased denitrification N2O flux. Our study suggests that the increased N2O emissions in the EC treatment were likely due to more denitrification N2O flux under increased soil moisture conditions. The higher soil moisture under EC was a result of reduced stomatal conductance, which lowered transpiration losses and improved plant water-use efficiency (Yadav et al., 2019), thereby promoting soil denitrification N2O production. Elevated CO2 may also increase the labile C input in the soil as a result of the increased belowground plant-C allocation (Daripa et al., 2014) leading to enhanced N2O emission.
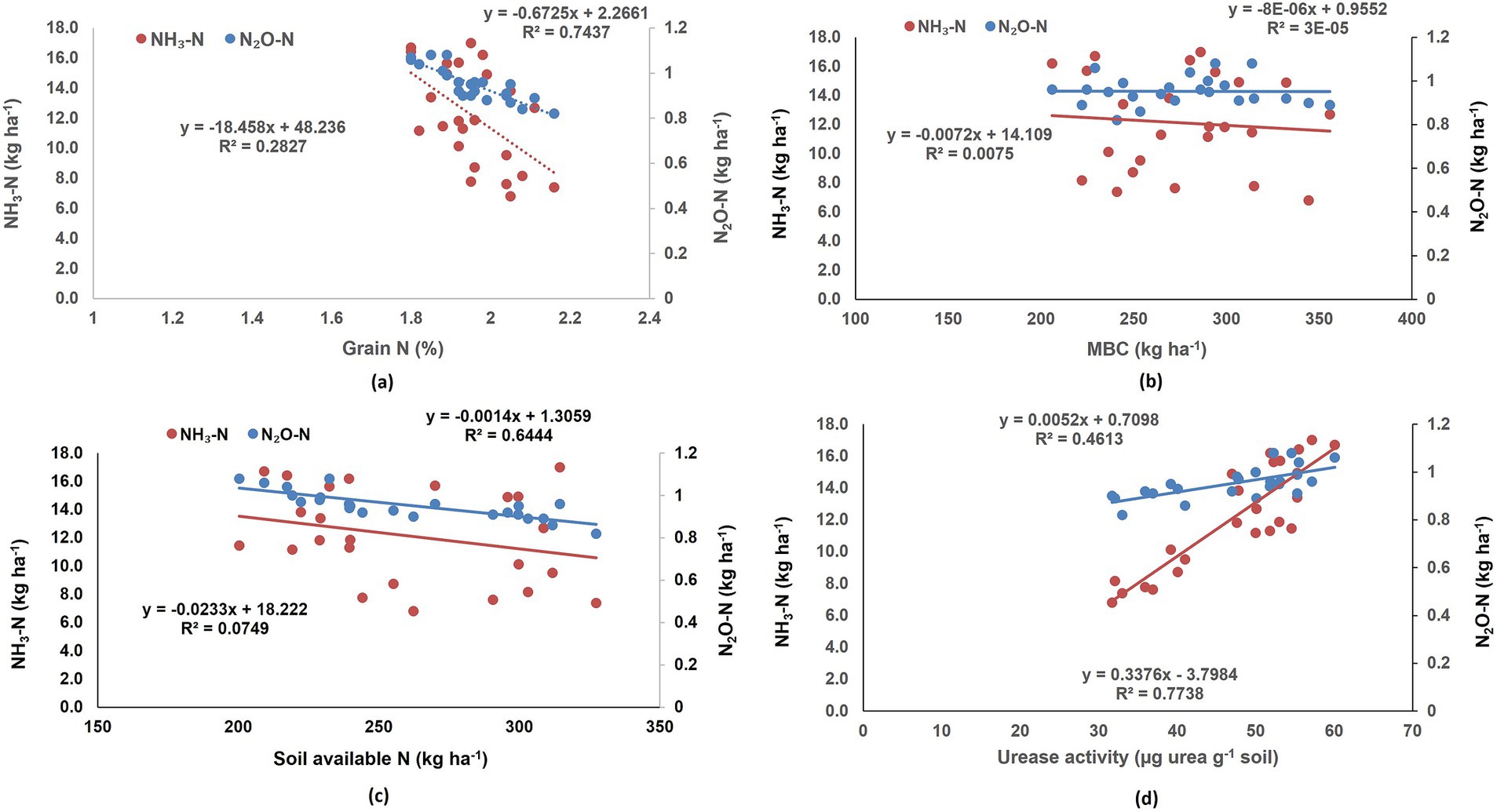
Figure 4. Correlation of NH3–N and N2O–N emission with (A) grain N concentration, (B) soil MBC and (C) soil available N, (D) urease activity of soil in different treatments.
Microbial biomass carbon (MBC) was observed to be higher in Limus and NOCU than prilled urea under the ECT interaction treatment. The release of more root exudates under elevated CO2 might increase the soil labile carbon, and microbial activity compared with the ambient (Maity et al., 2020). In our study the microbial biomass carbon (MBC) was observed to be higher under EC, and under ECT interaction as compared to the ambient. Increased crop growth in Limus and NOCU compared with prilled urea, might have led to the production of more belowground biomass, releasing a greater amount of root exudates, thereby enhancing the soil microbial activity, and increasing the MBC of soil. Bhattacharya et al. (2013), reported that elevated CO2 increased carbon supply due to enhanced root biomass, favored the growth of the denitrifiers resulting in higher N2O fluxes, however, under elevated CO2 plus temperature interaction they did not observe any significant changes in the soil labile carbon, different pools of nitrogen, enzyme activities and microbial populations as compared to elevated CO2 alone. Under EC, significantly higher N2O emissions were observed only in the N fertilized treatments, which shows that N2O production in wheat soil may be limited by the availability of inorganic N. Hence, in the unfertilized plots, N2O emission did not follow the same trend, as the N2O production in soil was limited by the availability of inorganic N. Due to greater plant N demand under elevated CO2 conditions, the availability of soil nitrogen for the nitrifiers and denitrifiers may decrease (Mosier et al., 2002).
The application of fertilizer N increased the emission of N2O. The application of Limus coated urea delayed the urea hydrolysis process (Li et al., 2015; Bhatia et al., 2023), resulting in slower availability of soil ammonium, thereby decreasing the NH3–N emission and further the N2O–N emission by the nitrification pathway. With the delayed availability of ammonium in soil due to the Limus coating, the amount of NH3 emitted was reduced. NBPT which is a constituent of Limus, binds the active sites of urease enzyme and inhibit urea hydrolysis over a period of 7–15 days (Cantarella et al., 2018; Sigurdarson et al., 2018). In China, it was reported to decrease NH3 emission by 33% (Abdo et al., 2020). Nitrification, as opposed to denitrification, is considered be one of the major pathways of N2O production, especially in the presence of ammonium-N from urea hydrolysis under aerobic soil conditions (Inubushi et al., 1996). Significant suppression of N2O emission by the NBPT urease inhibitor has been reported in irrigated potato cultivation (Souza et al., 2021). Neem-based products inhibit nitrification because of numerous active ingredients present in them like nimbidin, azadiractin, nimbolide (Choudhury et al., 2016; Sriraj et al., 2022). Akiyama et al. (2010) did a meta-analysis and observed that application of neem-based products along with urea reduced emission of N2O by 14% than urea. Several researchers have reported that both nitrification and denitrification processes increase with increasing temperature, thereby leading to greater emissions of N2O from soil (Lai et al., 2019; Wang C. et al., 2021). In this study, higher N2O emission was observed under ET and ECT as compared to ambient in all the N fertilized treatments. A meta-analysis of 134 studies by Dai et al. (2020) indicated that elevated temperature decreased soil microbial biomass N and increased N mineralization rates, resulting in enhanced N2O–N production in soil. In our study the soil available N decreased significantly under ET in all the fertilizer treatments, however, under elevated temperature the application of N through NOCU and Limus had higher soil available N than prilled urea. Lower rates of hydrolysis and nitrification in LIMUS and NOCU, respectively, led to lower gaseous N losses, resulting in higher soil available N as compared with prilled urea, under ET treatments. Limus has earlier been reported to reduce gaseous ammonia loss in maize and wheat crops due to slower availability of ammonium in soil (Li et al., 2015; BASF, 2016). Thus, the application of N as NOCU and Limus lowered the N2O emission, and also improved soil available N under ECT interaction as compared with prilled urea. Menéndez et al. (2012) reported a decrease in N2O emissions with the application of 3,4-dimethylpyrazole phosphate (DMPP), a nitrification inhibitor, as temperatures increased from 10 to 20°C. This suggests that nitrification inhibitors could reduce N2O emissions at higher soil temperatures. Soil MBC was found to be negatively corelated with N2O–N emission, however the correlation was not significant. We did not investigate the role of ammonia-oxidizing archaea (AOA) (Figure 4B) and bacteria (AOB), which may be important during the soil nitrification and denitrification processes under elevated temperature.
4.2 Effect of NOCU and LIMUS on plant nitrogen under elevated CO2 and temperature
In NOCU and Limus treatments, both aboveground and belowground biomass of the crop was higher than that of prilled urea in all the climatic treatments. Elevated temperature reduced crop growth and lowered the grain yield of the wheat in all the fertilizer treatments as compared to the ambient. Earlier researchers had reported that rise in temperature reduced crop yields, whereas, elevated CO2 increased the crop yields (Chakrabarti et al., 2012, 2021; Dubey et al., 2020). In our study, elevated CO2 could compensate for the yield loss due to elevated temperature in the ECT interaction treatment. Changes in CO2 and temperature affected the plant N demand by influencing the growth rate of plants. Nitrogen content in wheat grains decreased under the ECT interaction. More grain weight as well as more N losses under the ECT interaction resulted in a lower concentration of N in the grains. Reductions in grain nitrogen and crude protein content due to the dilution effect have been reported in different cereals grown under elevated CO2 (Abebe et al., 2016; Raj et al., 2019; Yadav et al., 2021).
In the NOCU and Limus applied treatments, the N uptake by wheat was more than that with prilled urea, even under ECT interaction. Higher N concentration in grains, and higher grain yield in NOCU and Limus treatments resulted in higher grain N uptake. Higher carbon assimilation by the plants under elevated CO2 concentration led to greater grain weight, resulting in higher grain N uptake and reduced grain N concentration (Ainsworth et al., 2007). However, the decrease in N concentration in wheat grains under elevated CO2 was alleviated by applying N application through NOCU and Limus. Grain N concentration was found to be negatively correlated with NH3–N and N2O–N emission (Figure 4A). Results showing a significant negative correlation between grain N concentration and N2O–N emission proves that less N2O–N emission led to increased soil available N, resulting in more N content in wheat grains (Figure 4C).
4.3 Effect of NOCU and LIMUS on GHG intensity and recovery efficiency
Higher GHG emissions as well as lower yield under ET increased the GHG intensity in wheat crop. The GHG intensity of wheat crop significantly increased under ECT treatment than AMB, due to higher N2O emission. Higher emission of NH3 and N2O lowered the recovery efficiency of N, while the application of N through NOCU and Limus, lowered the NH3–N and N2O–N emission and improved grain N uptake, thereby increasing the N use efficiency. Singh et al. (2019) reported that use of neem coated urea could improve crop yields and nutrient use efficiency by 5–6% with NOCU application. Cowan et al. (2021) had also reported an improvement in N use efficiency and reduction in GHGi in rice cultivation with neem oil coated urea application. Under future climatic conditions, the application of NOCU and Limus coated urea may reduce the NH3–N and N2O–N emission, thereby reducing the GWP and NUE in wheat crop. Recently in a laboratory study Chakraborty et al. (2023) reported that a combination of urease and nitrification inhibitor, was more effective in mitigating NH3 and GHG emission under ambient condition.
4.4 Impact of elevated CO2 and temperature interaction on wheat yield in India
Under the RCP 8.5 warming scenarios of the IPCC, warming in the range of 1.5–2°C is projected for many Indian districts in the major wheat growing states of Madhya Pradesh, Gujarat, Chhattisgarh, Punjab, Haryana, Uttarakhand and Uttar Pradesh (CSTEP, 2022). These states account for approximately 80% of the 107 Million tonnes of wheat produced in India (Agricultural Statistics at a Glance, 2021). Under RCP 4.5 warming scenarios, around 18% of the Indian districts, in the states of Chhattisgarh, Maharashtra, Madhya Pradesh, Gujarat, Uttarakhand, and Telangana, accounting for 21% of the total Indian wheat production may face a temperature increase of 1.5–2°C (CSTEP, 2022). Wheat is highly sensitive to changes in climate as growth and developmental stages of the crop are affected by temperature (Dubey et al., 2019). There are reports that, temperature rise shortens the crop growth duration in wheat, thereby reducing yield of the crop (Bhatia et al., 2010; Nahar et al., 2010; Sanyal et al., 2023).
As per the observations of this study, a temperature increase of 1.5–2.0°C may not have a significant impact on wheat yield if the CO2 levels simultaneously increase up to 560 ppm. However, the grain N content may be reduced by 10% with urea application under the ECT interaction treatment. As wheat is a major source of protein for most Indian population, this may have a significant impact on the country’s nutritional food security. There are reports that wheat grains produced under elevated CO2 concentration have lower nitrogen content and less protein than ambient condition (Chakrabarti et al., 2020; Wroblewitz et al., 2014). Hence, less supply of nitrogen could affect the quality of the crop. The application of urea coated with neem oil or Limus may increase the grain N content of wheat by 6 and 9%, respectively, as compared to prilled urea under the future climatic scenarios. Therefore, these two inhibitors need to be applied to shut down every conceivable escape route of nitrogen from soil (Recio et al., 2020; Zhang et al., 2023), and improve crop yield and NUE under future climatic condition.
5 Conclusion
Under increased levels of atmospheric CO2 and temperature, the emissions of NH3 and N2O could rise, resulting in a decrease in the soil’s nitrogen availability and reduced nitrogen concentration in wheat grains. Applying N through Limus decreased NH3–N emissions from wheat soils under elevated CO2 and temperature levels. Improved nitrogen management, using neem oil-coated or Limus-coated urea, can not only reduce nitrogen losses as N2O and NH3 but also enhance the nitrogen status of both plants and soil under future climatic conditions. This approach will lead to better nitrogen use efficiency of applied fertilizers, decreasing both direct and indirect N2O emissions, while maintaining the N content of wheat grains. This is crucial for ensuring nutritional food security in the Indian subcontinent. This study’s findings should be validated under various climatic and soil conditions to better understand how these inhibitors reduce N2O emissions and enhance plant and soil nitrogen. Our results indicate that coated fertilizers could improve nitrogen management in cultivated systems under future CO2 and temperature scenarios.
Data availability statement
The raw data supporting the conclusions of this article will be made available by the authors without undue reservation.
Author contributions
BC: Formal analysis, Investigation, Writing – original draft. AB: Conceptualization, Formal analysis, Funding acquisition, Writing – review & editing. SS: Visualization, Writing – review & editing. RT: Investigation, Writing – original draft. AS: Data curation, Writing – original draft. AP: Writing – review & editing. VK: Investigation, Writing – review & editing. MS: Writing – review & editing.
Funding
The author(s) declare that financial support was received for the research, authorship, and/or publication of this article. The authors acknowledge the funding support provided by the National Innovations in Climate Resilient Agriculture (IARI-12-115), Indian Council of Agricultural Research, for carrying out this research. Financial support was also provided by the UK Natural Environment Research Council (NERC) as part of the South Asia Nitrogen Hub (SANH) (NE/S009019/1), supported through UKRI Global Challenges Research Fund.
Conflict of interest
The authors declare that the research was conducted in the absence of any commercial or financial relationships that could be construed as a potential conflict of interest.
Publisher’s note
All claims expressed in this article are solely those of the authors and do not necessarily represent those of their affiliated organizations, or those of the publisher, the editors and the reviewers. Any product that may be evaluated in this article, or claim that may be made by its manufacturer, is not guaranteed or endorsed by the publisher.
References
Abdo, A. I., Shi, D., Li, J., Yang, T., and Wang, L. (2020). Ammonia emission from staple crops in China as response to mitigation strategies and agronomic conditions: metanalytic study. J. Clean. Prod. 279:123835. doi: 10.1016/j.jclepro.2020.123835
Abebe, A., Pathak, H., Singh, S. D., Bhatia, A., Harit, R. C., and Kumar, V. (2016). Growth, yield and quality of maize with elevated atmospheric carbon dioxide and temperature in north–west India. Agric. Ecosyst. Environ. 218, 66–72. doi: 10.1016/j.agee.2015.11.014
Agricultural Statistics at a Glance. (2021). Ministry of Agriculture and Farmers’ Welfare, Department of Agriculture, Cooperation and Farmers’ Welfare.
Ainsworth, E. A., Rogers, A., Leakey, A. D. B., Heady, L. E., Gibon, Y., Stitt, M., et al. (2007). Does elevated atmospheric (CO2) alter diurnal C uptake and the balance of C and N metabolites in growing and fully expanded soybean leaves? J. Exp. Bot. 58, 579–591. doi: 10.1093/jxb/erl233
Akiyama, H., Yan, X., and Yagi, K. (2010). Evaluation of effectiveness of enhanced-efficiency fertilizers as mitigation options for N2O and NO emissions from agricultural soils: meta-analysis. Glob. Chang. Biol. 16, 1837–1846. doi: 10.1111/j.1365-2486.2009.02031.x
Asseng, S., Ewert, F., Martre, P., Rötter, R. P., Lobell, D. B., Cammarano, D., et al. (2015). Rising temperatures reduce global wheat production. Nat. Clim. Chang. 5, 143–147. doi: 10.1038/nclimate2470
Aulakh, M. S., Singh, K., and Doran, J. (2001). Effects of 4-amino1,2,4-triazole, dicyandiamide and encapsulated calcium carbide on nitrification inhibition in a subtropical soil under upland and flooded conditions. Biol. Fertil. Soils 33, 258–263. doi: 10.1007/s003740000317
Bardgett, R. D., and Van Der Putten, W. H. (2014). Belowground biodiversity and ecosystem functioning. Nature 515, 505–511. doi: 10.1038/nature13855
BASF (2016). Project completion report on reducing loss of nitrogen and emission of greenhouse gases with urea stabilizer in maize and wheat, submitted by CESCRA. Division of Env. Science, ICAR-IARI, New Delhi: Indian Agricultural Research Institute to BASF India.
Bhatia, A., Cowan, N. J., Drewer, J., Tomer, R., Kumar, V., Sharma, S., et al. (2023). The impact of different fertiliser management options and cultivars on nitrogen use efficiency and yield for rice cropping in the Indo-Gangetic plain: two seasons of methane, nitrous oxide and ammonia emissions. Agric. Ecosyst. Environ. 355:108593. doi: 10.1016/j.agee.2023.108593
Bhatia, A., Mina, U., Kumar, V., Tomer, R., Kumar, A., Chakrabarti, B., et al. (2021). Effect of elevated ozone and carbon dioxide interaction on growth, yield, nutrient content and wilt disease severity in chickpea grown in Northern India, Heliyon :7e06049. doi: 10.1016/j.heliyon.2021.e06049
Bhatia, A., Kumar, V., Kumar, A., Tomer, R., Singh, B., and Singh, S. D. (2013). Effect of elevated ozone and carbon dioxide interaction on growth and yield of maize. Maydica 58, 291–229.
Bhatia, A., Pathak, H., Aggarwal, P. K., and Jain, N. (2010). Trade-off between productivity enhancement and global warming potential of rice and wheat in India. Nutr. Cycl. Agroecosyst. 86, 413–424. doi: 10.1007/s10705-009-9304-5
Bhatia, A., Pathak, H., Jain, N., Singh, P. K., and Singh, A. K. (2005). Global warming potential of manure amended soils under rice-wheat system in the Indo-Gangetic plains. Atmos. Environ. 39, 6976–6984. doi: 10.1016/j.atmosenv.2005.07.052
Bhatia, A., Tomer, R., Kumar, V., Singh, S. D., and Pathak, H. (2012). Impact of tropospheric ozone on crop growth and productivity–a review. J. Sci. Ind. Res. 71, 97–112.
Bhattacharyya, P., Roy, K. S., Neogi, S., Dash, P. K., Nayak, A. K., Mohanty, S., et al. (2013). Impact of elevated CO2 and temperature on soil C and N dynamics in relation to CH4 and N2O emissions from tropical flooded rice (Oryza sativa L.). Science of the Total Environment, 461, 601–611.
Brevik, E. C. (2013). The potential impact of climate change on soil properties and processes and corresponding influence on food security. Agriculture 3, 398–417. doi: 10.3390/agriculture3030398
Cai, Y., Ding, W., and Luo, J. (2012). Spatial variation of nitrous oxide emission between interrow soil and interrow plus row soil in a long-term maize cultivated sandy loam soil. Geoderma 181-182, 2–10. doi: 10.1016/j.geoderma.2012.03.005
Cantarella, H., Otto, R., Soares, J. R., and Silva, A. G. D. B. (2018). Agronomic efficiency of NBPT as a urease inhibitor: a review. J. Adv. Res. 13, 19–27. doi: 10.1016/j.jare.2018.05.008
Chakrabarti, B., Bhatia, A., Pramanik, P., Singh, S. D., Jatav, R. S., Das Saha, N., et al. (2021). Changes in thermal requirements, growth and yield of wheat under the elevated temperature. Indian J. Agric. Sci. 91, 435–439.
Chakrabarti, B., Singh, S. D., Bhatia, A., Kumar, V., and Harit, R. C. (2020). Yield and nitrogen uptake in wheat and chickpea grown under elevated carbon dioxide level. Natl. Acad. Sci. Lett. 43, 109–113. doi: 10.1007/s40009-019-00816-y
Chakrabarti, B., Singh, S. D., Kumar, S. N., Aggarwal, P. K., Pathak, H., and Nagarajan, S. (2012). Low-cost facility for assessing impact of carbon dioxide on crops. Curr. Sci. 102, 1035–1040.
Chakrabarti, B., Singh, S. D., Kumar, V., Harit, R. C., and Misra, S. (2013). Growth and yield response of wheat and chickpea crops under high temperature. Indian J. Plant Physiol. 18, 7–14. doi: 10.1007/s40502-013-0002-6
Chakraborty, R., Purakayastha, T. J., Pendall, E., Dey, S., Jain, N., and Kumar, S. (2023). Nitrification and urease inhibitors mitigate global warming potential and ammonia volatilization from urea in rice-wheat system in India: a field to lab experiment. Sci. Total Environ. 898:165479. doi: 10.1016/j.scitotenv.2023.165479
Choudhury, R., Majumder, M., Roy, D. N., Basumallick, S., and Misra, T. K. (2016). Phytotoxicity of ag nanoparticles prepared by biogenic and chemical methods. Int. Nano Lett. 6, 153–159. doi: 10.1007/s40089-016-0181-z
Cowan, N., Bhatia, A., Drewer, J., Jain, N., Singh, R., Tomer, R., et al. (2021). Experimental comparison of continuous and intermittent flooding of rice in relation to methane, nitrous oxide and ammonia emissions and the implications for nitrogen use efficiency and yield. Agric. Ecosyst. Environ. 319:107571. doi: 10.1016/j.agee.2021.107571
CSTEP. (2022). Climate atlas of India: district-level analysis of historical and projected climate change scenarios. (CSTEP-RR-2022-14). Bengaluru, India: Center for Study of Science, Technology, and Policy.
Daepp, M., Suter, D., Almeida, J. P. F., Isopp, H., Hartwig, U. A., and Frehner, M. (2000). Yield response of Lolium perenneswards to free air CO2 enrichment increased over six years in a high N input system on fertile soil. Glob. Chang. Biol. 6, 805–816. doi: 10.1046/j.1365-2486.2000.00359.x
Dai, Z., Yu, M., Chen, H., Zhao, H., Huang, Y., Su, W., et al. (2020). Elevated temperature shifts soil N cycling from microbial immobilization to enhanced mineralization, nitrification and denitrification across global terrestrial ecosystems. Glob. Chang. Biol. 26, 5267–5276. doi: 10.1111/gcb.15211
Daripa, A., Bhatia, A., Tomer, R., Singh, S. D., Jain, N., and Pathak, H. (2014). Nitrous oxide and carbon dioxide emission from maize (zea mays l.) under fertiliser application and elevated carbon dioxide in Northwest India. Exp. Agric. 50, 625–643. doi: 10.1017/S0014479714000118
Dey, S. K., Chakrabarti, B., Purakayastha, T. J., Prasanna, R., Mittal, R., Singh, S. D., et al. (2019). Interplay of phosphorus doses, cyanobacterial inoculation, and elevated carbon dioxide on yield and phosphorus dynamics in cowpea. Environ. Monit. Assess. 191:223. doi: 10.1007/s10661-019-7378-3
Ditz, K. (1988) in Efficiency of uptake and utilization of fertilizer nitrogen by plants. eds. D. S. Jenkinson and K. A. Smith (London and New York: Elsevier Applied Science), 1–26.Nitrogen efficiency in agricultural soils
Dubey, R., Pathak, H., Chakrabarti, B., Singh, S. D., Gupta, D. K., and Harit, R. C. (2020). Impact of terminal heat stress on wheat yield in India and options for adaptation. Agric. Syst. 181:102826. doi: 10.1016/j.agsy.2020.102826
Dubey, R., Pathak, H., Singh, S. D., Chakravarti, B., Thakur, A. K., and Fagodia, R. K. (2019). Impact of sowing dates on terminal heat tolerance of different wheat (Triticum aestivum L.) cultivars, Natl. Acad. Sci. Lett. 42, 445–449. doi: 10.1007/s40009-019-0786-7
Engel, R., Romero, C., and Jones, C., (2017). Ammonia loss and fertilizer 15N recovery in no-till winter wheat following broadcast urea applications. Crop Soils 50, 30–33. doi: 10.2134/CS2017.50.0406
Fan, X. H., Li, Y. C., and Alva, A. K. (2011). Effects of temperature and soil type on Ammonia volatilization from slow-release nitrogen fertilizers. Commun. Soil Sci. Plant Anal. 42, 1111–1122. doi: 10.1080/00103624.2011.566957
Fu, Q., Abadie, M., Blaud, A., Carswell, A., Misselbrook, T. H., Clark, I. M., et al. (2020). Effects of urease and nitrification inhibitors on soil N, nitrifier abundance and activity in a sandy loam soil. Biol. Fertil. Soils 56, 185–194. doi: 10.1007/s00374-019-01411-5
Giannadaki, D., Giannakis, E., Pozzer, A., and Lelieveld, J. (2018). Estimating health and economic benefits of reductions in air pollution from agriculture. Sci. Total Environ. 622–623, 1304–1316. doi: 10.1016/j.scitotenv.2017.12.064
Gifford, R. M., Barrett, D. J., and Lutze, J. L. (2000). The effects of elevated [CO2] on the C:N and C:P mass ratios of plant tissues. Plant Soil 224, 1–14. doi: 10.1023/A:1004790612630
Gupta, D. K., Bhatia, A., Kumar, A., Chakrabarti, B., Jain, N., and Pathak, H. (2015). Global warming potential of rice (Oryzasativa)–wheat (Triticumaestivum) cropping system of the indo-Gangetic Plains. Indian J. Agric. Sci. 85, 807–816. doi: 10.56093/ijas.v85i6.49243
Gupta, D. K., Bhatia, A., Kumar, A., Das, T. K., Jain, N., Tomer, R., et al. (2016). Mitigation of greenhouse gas emission from rice–wheat system of the Indo-Gangetic plains: through tillage, irrigation and fertilizer management Agric. Ecosyst. Environ. 230, 1–9. doi: 10.1016/j.agee.2016.05.023
He, Z., Kumar, A., Calvert, D., and Banks, D. (1999). Ammonia volatilization from different fertilizer sources and effects of temperature and soil pH. Soil Sci. 164, 750–758. doi: 10.1097/00010694-199910000-00006
Inubushi, K., Naganuma, H., and Kitahara, S. (1996). Contribution of denitrification and autotrophic and heterotrophic nitrification to nitrous oxide production in andosols. Biol. Fertil. Soils. 23, 292–298. doi: 10.1007/BF00335957
IPCC (2021). “Summary for policymakers” in Climate change 2021: The physical science basis. Contribution of working group I to the sixth assessment report of the intergovernmental panel on climate change. eds. V. Masson-Delmotte, P. Zhai, A. Pirani, S. L. Connors, C. Péan, and S. Berger, et al. (Cambridge, United Kingdom and New York, NY, USA: Cambridge University Press), 3–32.
Jenkinson, D. S., and Powlson, D. S. (1976). The effects of biocidal treatments on metabolism in soil-V. A method for measuring soil biomass. Soil Biol. Biochem. 8, 209–213. doi: 10.1016/0038-0717(76)90005-5
Jiang, L., Dai, T., Jiang, D., Cao, W., Gan, X., and Wei, S. (2004). Characterizing physiological N-use efficiency as influenced by nitrogen management in three rice cultivars. Field Crop Res. 88, 239–250. doi: 10.1016/j.fcr.2004.01.023
Jiang, R., Yang, J., Drury, C. F., He, W., Smith, W., Grant, B., et al. (2020). Assessing the impacts of diversified crop rotation systems on yields and nitrous oxide emissions in Canada using the DNDC model. Sci. Total Environ. 759:143433. doi: 10.1016/j.scitotenv.2020.143433
Ladha, J. K., Tirol-Padre, A., Reddy, C. K., Cassman, K. G., Verma, S., Powlson, D. S., et al. (2016). Global nitrogen budgets in cereals: a 50-year assessment for maize, rice and wheat production systems. Sci. Rep. 6:19355. doi: 10.1038/srep19355
Lai, T. V., Farquharson, R., and Denton, M. D. (2019). High soil temperatures alter the rates of nitrification, denitrification and associated N2Oemissions. J. Soils Sediments 19, 2176–2189. doi: 10.1007/s11368-018-02238-7
Li, Q., Yang, A., Wang, Z., Roelcke, M., Chen, X., Zhang, F., et al. (2015). Effect of a new urease inhibitor on ammonia volatilization andnitrogen utilization in wheat in north and Northwest China. Field Crop Res. 175, 96–105. doi: 10.1016/j.fcr.2015.02.005
Lin, S., Hernandez-Ramirez, G., Kryzanowski, L., Wallace, T., Grant, R., Degenhardt, R., et al. (2017). Timing of Slurry Injection and Nitrification Inhibitors Impacts on Nitrous Oxide Emissions and Nitrogen Transformations in a Barley Crop. Soil Sci. Soc. Amer. J. 81,1595–1605.
Maity, P. P., Chakrabarti, B., Purakayastha, T. J., Bhatia, A., Saha Das, N. D., Jatav, R. S., et al. (2020). Do elevated CO2 and temperature affect organic nitrogen fractions and enzyme activities in soil under rice crop? Soil Res. 58, 400–410. doi: 10.1071/SR19270
Malla, G., Bhatia, A., Pathak, H., Prasad, S., Jain, N., and Singh, J., (2005). Mitigating nitrous oxide and methane emissions from soil in rice-wheat system of the Indo-Gangetic plain with nitrification and urease inhibitors. Chemosphere 58, 141–147.
Malyan, S. K., Bhatia, A., Tomer, R., Harit, R. C., Jain, N., Bhowmik, A., et al. (2021a). Mitigation of yield-scaled greenhouse gas emissions from irrigated rice through Azolla, Blue-green algae, and plant growth–promoting bacteria. Environmental Science and Pollution Research, 28, 51425–51439.
Malyan, S. K., Bhatia, A., Fagodiya, R. K., Kumar, S. S., Kumar, A., Gupta, D. K., et al. (2021b). Plummeting global warming potential by chemicals interventions in irrigated rice: A lab to field assessment. Agriculture, Ecosystems & Environment, 319:107545.
Malyan, S. K., Bhatia, A., Kumar, S. S., Fagodiya, R. K., Pugazhendhi, A., and Duc, P. A. (2019). Mitigation of greenhouse gas intensity by supplementing with Azolla and moderating the dose of nitrogen fertilizer. Biocatal. Agric. Biotechnol. 20:101266. doi: 10.1016/j.bcab.2019.101266
Mariano, E., de Sant Ana Filho, C. R., Bortoletto-Santos, R., Bendassolli, J. A., and Trivelin, P. C. O. (2019). Ammonia losses following surface application of enhanced-efficiency nitrogen fertilizers and urea. Atmos. Environ. 203, 242–251. doi: 10.1016/j.atmosenv.2019.02.003
Menéndez, S., Barrena, I., Setien, I., González-Murua, C., and Estavillo, J. M. (2012). Efficiency of nitrification inhibitor DMPP to reduce nitrous oxide emissions under different temperature and moisture conditions. Soil Biol. Biochem. 53, 82–89. doi: 10.1016/j.soilbio.2012.04.026
Mosier, A. R., Morgan, J. A., King, J. Y., LeCain, D., and Milchunas, D. G. (2002). Soil-atmosphere exchange of CH4, CO2, NOx, and N2O in the Colorado shortgrass steppe under elevated CO2. Plant Soil 240, 201–211. doi: 10.1023/A:1015783801324
Myers, S. S., Zanobetti, A., Kloog, I., Huybers, P., Leakey, A. D., Bloom, A. J., et al. (2014). Increasing CO2 threatens human nutrition. Nature 510, 139–142. doi: 10.1038/nature13179
Nahar, K., Ahmad, K., and Fujita, M. (2010). Phenological variation and its relation with yield in several wheat (Triticum aestivum L.) cultivars under normal and late sowing mediated heat stress conditions. Notulae Scientia Biologicae 2, 51–56. doi: 10.15835/nsb234723
National Oceanic and Atmospheric Administration. (2023). State of the Climate in 2022. NOAA Climate.gov. Available at: https://www.climate.gov/news-features/understanding-climate/state-climate-2022
Norby, R. J., Cotrufo, M. F., Ineson, P., O’Neill, E. G., and Canadell, J. G. (2001). Elevated CO2, litter chemistry, and decomposition: a synthesis. Oecologia 127, 153–165. doi: 10.1007/s004420000615
Norton, J., and Ouyang, Y. (2019). Controls and adaptive management of nitrification in agricultural soils. Front. Microbiol. 10:1931. doi: 10.3389/fmicb.2019.01931
Pan, B., Lam, S. K., Mosier, A., Luo, Y., and Chen, D. (2016). Ammonia volatilization from synthetic fertilizers and its mitigation strategies: a global synthesis. Agric. Ecosyst. Environ. 232, 283–289. doi: 10.1016/j.agee.2016.08.019
Pathak, H., Bhatia, A., and Jain, N. (2014). Greenhouse gas emission from Indian agriculture: trends, mitigation and policy needs. New Delhi: Indian Agricultural Research Institute, 39.
Pramanik, P., Chakrabarti, B., Bhatia, A., Singh, S. D., Mridha, N., and Krishnan, P. (2018). Effect of elevated carbon dioxide on soil hydrothermal regimes and growth of maize crop (Zea mays L.) in semi-arid tropics of indo-Gangetic Plains. Environ. Monit. Assess. 190:661. doi: 10.1007/s10661-018-6988-5
Raj, A., Chakrabarti, B., Pathak, H., Singh, S. D., Mina, U., and Purakayastha, T. J. (2019). Growth, yield and nitrogen uptake in rice crop grown under elevated carbon dioxide and different doses of nitrogen fertilizer. Indian J. Exp. Biol. 57, 181–187.
Recio, J., Montoya, M., Gin´es, C., Sanz-Cobena, A., Vallejo, A., Alvarez, J. M., et al. (2020). Joint mitigation of NH3 and N2O emissions by using two synthetic inhibitors in an irrigated cropping soil. Geoderma, 373. doi: 10.1016/j.geoderma.2020.114423
Sanyal, S., Chakrabarti, B., Bhatia, A., Kumar, S. N., Purakayastha, T. J., Kumar, D., et al. (2023). Response of aestivum and durum wheat varieties to elevated CO2 and temperature under OTC condition. J. Agrometeorol. 25, 498–502. doi: 10.54386/jam.v25i4.2366
Sha, Z., Wang, J., Ma, X., Lv, T., Liu, X., and Misselbrook, T. (2023). Ammonia loss potential and mitigation options in a wheat-maize rotation system in the North China plain: a data synthesis and field evaluation. Agric. Ecosyst. Environ. 352:108512. doi: 10.1016/j.agee.2023.108512
Sigurdarson, J. J., Svane, S., and Karring, H. (2018). The molecular processes of urea hydrolysis in relation to ammonia emissions from agriculture. Rev. Environ. Sci. Biotechnol. 17, 1–18.
Singh, S., Bhatia, A., Tomer, R., Kumar, V., Singh, B., and Singh, S. D. (2013). Synergistic action of tropospheric ozone and carbon dioxide on yield and nutritional quality of Indian mustard (Brassica juncea (L.) Czern.). Environ. Monit. Assess. 185, 6517–6529. doi: 10.1007/s10661-012-3043-9
Singh, S. D., Chakrabarti, B., Muralikrishna, K. S., Chaturvedi, A. K., Kumar, V., Mishra, S., et al. (2013). Yield response of important field crops to elevated air temperature and CO2 levels. Indian J. Agric. Sci. 83, 1009–1012.
Singh, A., Jaswal, A., and Singh, M. (2019). Impact of neem coated urea on rice yield and nutrient use efficiency (NUE). Agric. Rev. 40, 70–74.
Sommer, S. G., Schjoerring, J. K., and Denmead, O. T. (2004). Ammonia emission from mineral fertilizers and fertilized crops. Adv. Agron. 82, 557–622. doi: 10.1016/S0065-2113(03)82008-4
Souza, E. F., Rosen, C. J., and Venterea, R. T. (2021). Co-application of DMPSA and NBPT with urea mitigates both nitrous oxide emissions and nitrate leaching during irrigated potato production. Environ. Pollut. 284:117124. doi: 10.1016/j.envpol.2021.117124
Sriraj, P., Toomsan, B., and Butnan, S. (2022). Effects of neem leaf extract on the soil properties, growth, yield, and inorganic nitrogen contents of lettuce. Horticulturae 8:1104. doi: 10.3390/horticulturae8121104
Subbiah, B., and Asija, G. L. (1956). A rapid procedure for estimation of available nitrogen in soils. Curr. Sci. 25, 259–260.
Sutton, M. A., Oenema, O., Erisman, J. W., Leip, A., van Grinsven, H., and Winiwarter, W. (2011). Too much of a good thing? Nature 472, 156–161. doi: 10.1038/472159a
Thilakarathna, S. K., Hernandez-Ramirez, G., Puurveen, D., Kryzanowski, L., Lohstraeter, G., Powers, L. A., et al. (2020). Nitrous oxide emissions and nitrogen use efficiency in wheat: nitrogen fertilization timing and formulation, soil nitrogen, and weather effects. Soil Sci. Soc. Am. J. 84, 1910–1927. doi: 10.1002/saj2.20145
van Groenigen, K. J., Osenberg, C. W., and Hungate, B. A. (2011). Increased soil emissions of potent greenhouse gases under increased atmospheric CO2. Nature 475:214216. doi: 10.1038/nature10176
van Groenigen, K., van Kessel, C., and Hungate, B. (2013). Increased greenhouse-gas intensity of rice production under future atmospheric conditions. Nat. Clim. Chang. 3, 288–291. doi: 10.1038/nclimate1712
Wang, C., Amon, B., Schulz, K., and Mehdi, B. (2021). Nitrous oxide emissions from agricultural soils as well as their representation in simulation models: a review. Agronomy 11, –770. doi: 10.3390/agronomy11040770
Wang, B., Li, R., Wan, Y., Cai, W., Guo, C., Qin, X., et al. (2021). Air warming and CO2 enrichment cause more ammonia volatilization from rice paddies: an OTC field study. Sci. Total Environ. 752:142071. doi: 10.1016/j.scitotenv.2020.142071
Wang, J., Hasegawa, T., Li, L., Lam, S. K., Zhang, X., Liu, X., et al. (2019). Changes in grain protein and amino acids composition of wheat and rice under short-term increased [CO2] and temperature of canopy air in a paddy from East China. New Phytol. 222, 726–734. doi: 10.1111/nph.15661
Weiske, A., Benckiser, G., Herbert, T., and Ottow, J. C. G. (2001). Influence of the nitrification inhibitor 3,4-dimethylpyrazole phosphate (DMPP) in comparison to dicyandiamide (DCD) on nitrous oxide emissions, carbon dioxide fluxes and methane oxidation during 3 years of repeated application in field experiments. Biology and Fertility of Soils 34, 109–117.
Wroblewitz, S., Hiither, L., Manderscheid, R., Weigel, H. J., Wiitzig, H., and Danicke, S. (2014). Effect of rising atmospheric carbon dioxide concentration on the protein composition of cereal grain. J. Agric. Food Chem. 62, 6616–6625. doi: 10.1021/jf501958a
Wu, K. K., Chen, D. M., Tu, C., Qiu, Y. P., Burkey, K. O., and Reberg-Horton, S. C., (2017). CO2-induced alterations in plant nitrate utilization and root exudation stimulate N2O emissions. Soil Biol. Biochem. 106, 9–17.
Xu, X. F., Tian, H. Q., Chen, G. S., Liu, M. L., Ren, W., Lu, C. Q., et al. (2012). Multifactor controls on terrestrial N2O flux over North America from 1979 through 2010. Biogeosciences 9, 1351–1366. doi: 10.5194/bg-9-1351-2012
Xu, W., Zhao, Y., Wen, Z., Chang, Y., Pan, Y., Sun, Y., et al. (2022). Increasing importance of ammonia emission abatement in PM2.5 pollution control. Sci. Bull. 67, 1745–1749. doi: 10.1016/j.scib.2022.07.021
Yadav, A., Bhatia, A., Yadav, S., Kumar, V., and Singh, B. (2019). The effects of elevated CO2 and elevated O3 exposure on plant growth, yield and quality of grains of two wheat cultivars grown in North India. Heliyon 5:e02317. doi: 10.1016/j.heliyon.2019.e02317
Yadav, A., Bhatia, A., Yadav, S., Singh, A., Tomer, R., Harit, R., et al. (2021). Growth, yield and quality of maize under ozone and carbon dioxide interaction in North West India. Aerosol Air Qual. Res. 21:200194. doi: 10.4209/aaqr.2020.05.0194
Keywords: nitrification inhibitor, urease inhibitor, nitrous oxide, ammonia, N use efficiency, future climate
Citation: Chakrabarti B, Bhatia A, Sharma S, Tomer R, Sharma A, Paul A, Kumar V and Sutton MA (2024) Nitrification and urease inhibitors reduce gaseous N losses and improve nitrogen use efficiency in wheat exposed to elevated CO2 and temperature. Front. Sustain. Food Syst. 8:1460994. doi: 10.3389/fsufs.2024.1460994
Edited by:
Rajiv Kumar Srivastava, Texas A and M University, United StatesReviewed by:
Sven Marhan, University of Hohenheim, GermanySubrat Kumar Behera, Orissa University of Agriculture and Technology, India
Copyright © 2024 Chakrabarti, Bhatia, Sharma, Tomer, Sharma, Paul, Kumar and Sutton. This is an open-access article distributed under the terms of the Creative Commons Attribution License (CC BY). The use, distribution or reproduction in other forums is permitted, provided the original author(s) and the copyright owner(s) are credited and that the original publication in this journal is cited, in accordance with accepted academic practice. No use, distribution or reproduction is permitted which does not comply with these terms.
*Correspondence: Arti Bhatia, YXJ0aS5iaGF0aWFAaWNhci5nb3YuaW4=: Mark A. Sutton bXNAY2VoLnVr