- 1Department of Biomedical Engineering and Biotechnology, College of Medicine and Health Sciences, Khalifa University of Science and Technology, Abu Dhabi, United Arab Emirates
- 2Biomedical Engineering and Healthcare Engineering Innovation Center, Khalifa University, Abu Dhabi, United Arab Emirates
- 3Center for Biotechnology, Khalifa University of Science and Technology, Abu Dhabi, United Arab Emirates
- 4Department of Pathophysiology, Faculty of Medicine, University of Belgrade, Belgrade, Serbia
- 5Laboratory for Cellular Physiology, Department of Medical Physiology, Faculty of Medicine, University of Belgrade, Belgrade, Serbia
- 6University of Haifa, Haifa, Israel
- 7Department of Pharmacology, College of Medicine and Health Sciences, Khalifa University of Science and Technology, Abu Dhabi, United Arab Emirates
- 8Creo Medical Limited, Chepstow, United Kingdom
Developing sustainable and effective treatments for chronic venous insufficiency (CVI) is crucial. In this study, we propose an innovative restorative approach utilizing hydrogels derived from the decellularized extracellular matrix (dECM) of cadaveric vascular tissues, adipose-derived stem cells (ADSCs), and gold nanoparticles (AuNPs). This therapeutic method leverages waste valorization by repurposing discarded cadaveric tissues from slaughterhouse livestock. The dECM hydrogels, enriched with ADSCs and AuNPs, offer a biocompatible scaffold that supports cellular differentiation and vascular integrity. Our approach addresses the limitations of current allo-, auto-, and xenograft methods by enhancing integration and functionality while potentially reducing costs through sustainable practices. This study explores functionalized hydrogel formulation solely generated from agri-food waste, gelation mechanisms, and preliminary cost-effectiveness, presenting a promising new avenue for treating early-stage varicose veins that can ultimately be translated to human models using discarded tissues.
Introduction
Superficial venous insufficiency, more commonly known as varicose veins, is bulging, enlarged, and twisted veins that appear under the skin’s surface and are generally found in the legs (Beckman, 2002). Such conditions develop when the venous valves are weakened or damaged, causing blood to pool and enlarge the veins. This condition can result in chronic venous insufficiency (CVI) or varicose vein disease, typically involving lower extremity edema, trophic skin changes, and discomfort secondary to venous hypertension (Patel and Surowiec, 2023).
While primary varicose veins, often referred to as idiopathic varices, are the most common type and are considered congenital, secondary (post-thrombotic) varices are consequences of long-term deep vein damage (Antani and Dattilo, 2023). Superficial venous insufficiency, a common vascular disorder affecting men and women worldwide, though more prevalent in women, encompasses both primary and secondary disorders (DePopas and Brown, 2018). Unfortunately, many healthcare providers overlook this condition due to a limited understanding of primary and secondary venous disorders and the substantial impact these conditions have on individuals, resulting in disease progression (Youn and Lee, 2019). CVI-related disability can lead to a markedly diminished quality of life (QoL) and a loss of productivity. Numerous research outcomes indicate that vascular complications, like CVI, have a notable impact on the QoL index of affected individuals (Wu and Ma, 2021). Symptoms such as pain and swelling restrict daily activities, work, leisure pursuits, social interactions, and sleep. In addition to the physical limitations, such disorders impose a significant emotional burden, leading to uncertainty about the future. Furthermore, emotional difficulties, including anxiety, embarrassment, diminished self-esteem, depression, and social withdrawal related to aesthetic concerns, contribute to the overall emotional challenges (Sritharan et al., 2012), adversely influencing patient relationships (Santiago et al., 2022) and healthcare costs (König et al., 2019).
The prevalence of varicose vein disease varies worldwide, with an incidence rate ranging from 20 to 60% (Shakya et al., 2020). Additional research findings suggest that varicose vein disease in particular geographic areas, such as Iran, could potentially be as high as 73% among individuals employed in high-risk occupations like those in the healthcare sector (Aslam et al., 2022) and armed forces (Alekseev and Belousov, 2020). This elevated incidence can be attributed to prolonged and frequent standing. Overall, such prevalence indicates that varicose veins pose a global challenge, exerting significant pressure on healthcare systems. Regrettably, this burden is anticipated to increase with rising global incidences of diabetes, obesity, pregnancy, and aging (Aslam et al., 2022), which are known to induce inflammation, endothelial dysfunction, and hyperfiltration or leakage, driving the development of these vascular complications.
Several studies have highlighted the significant economic burden and healthcare costs associated with vascular diseases, including CVI. For instance, the annual healthcare burden due to CVI in the United States exceeds $3 billion, accounting for 1–2% of the national budget (Dua et al., 2016). Additionally, as CVD progresses, it significantly diminishes patients’ QoL and can lead to complications like deep vein thrombosis (DVT). A 2019-based report provided insight into the annual incidence of new or recurrent DVT events across different countries. Precisely, the yearly occurrence of these events was estimated at 122,000 in Australia, 263,000 in France, 345,000 in Germany, 253,000 in Italy, 85,000 in Spain, 230,000 in the UK, and 643,000 in the US (Kolluri et al., 2022). These incidence rates ranged from 0.73 to 3.12 per 1,000 individuals annually. Overall, the projected annual direct medical expenditures for patients undergoing conservative management for DVT in these regions were collectively estimated at approximately $10.73 billion (Moon et al., 2023). This figure translates to an average cost of about $5,527 per individual per year (Kolluri et al., 2022).
Primarily caused by valvular incompetence, varicose vein formation can be attributed to leaflet deformation and luminal expansion (Costa et al., 2023), as outlined in Figure 1. Mechanistically, increased venous hydrostatic pressure leads to reflux, enhanced vessel wall tension, and endothelial injury to altered shear stresses, attributing to significant pathophysiological changes in the vein (Piazza, 2014). Specifically, blood reflux and endothelial injury contribute to altered shear stresses, inflammation, and ultimately enhanced matrix metalloproteinase (MMP) over-expression or activity. Such an MMP-based response will drive endothelial cell hyperpolarization, vascular smooth muscle relaxation, and extracellular matrix (ECM) substrate degradation and remodeling that influence wall dilation and leaflet tearing, thinning, and adhesion to the vessel walls (Wang and Khalil, 2018).
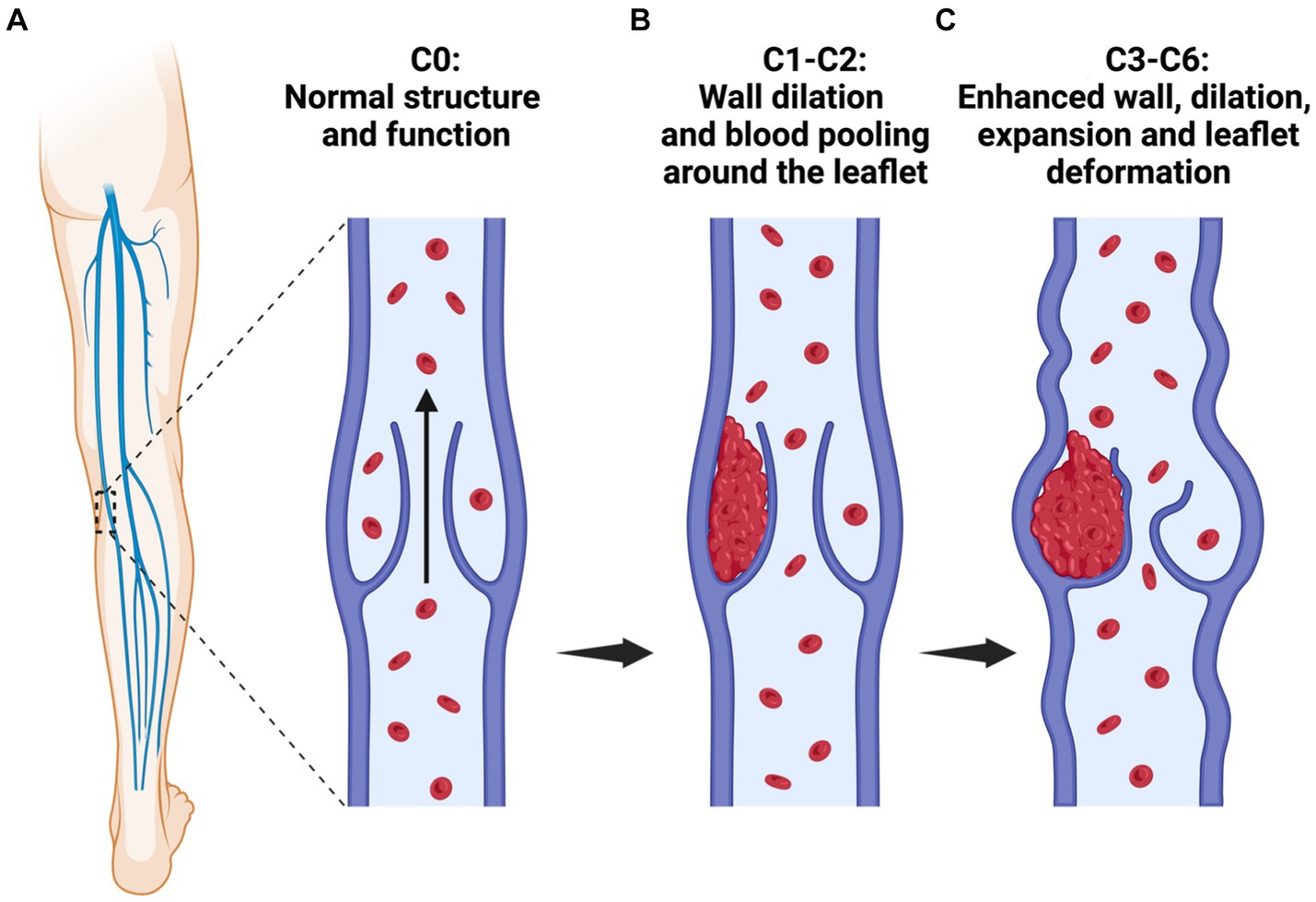
Figure 1. A schematic illustrating the stages of varicose vein disease. Varicose vein formation, classification, and progression: (A) C0, absence of visible venous disease signs; (B) C0-C1: C1, vessel wall dilation, and blood pooling which support leaflet deformation and the manifestation of telangiectasias or reticular veins, and C2, presence of varicose veins; and (C) C3-C6: C3, development of edema and enhanced luminal expansion and leaflet damage. Additional aspects of this disease progression (not included in this schematic) include C4, the occurrence of pigmentation or eczema and the emergence of lipodermatosclerosis or atrophy blanche; C5, a healed ulcer; and C6, an active ulcer. Moreover, early-stage varicose veins are present in stages C1-C2, and stages C3 to C6 are categorized as CVI, indicative of the venous disease’s advanced clinical stage.
Various treatments exist for varicose veins, each with limitations. For instance, conservative measures like compression stockings provide temporary relief without addressing root causes, leading to a recurrence (Raffetto and Khalil, 2008) and disease progression (Kapp et al., 2013). At the same time, minimally invasive procedures, such as endovenous thermal ablation, effectively close veins but can induce DVT, nerve injury, and recurrence, particularly in prominent veins (Kamaraj et al., 2022). Sclerotherapy, on the other hand, while widely used, can provoke inflammation, clots, and allergic reactions and is less effective on larger vessels (Khaki et al., 2018). Given these constraints and a rising prevalence, there is an urgent need for innovative treatments, particularly for early-stage disorders, to enhance patient outcomes and their QoL.
Alternative treatment methods include the use of autografts, allografts, and xenografts. Autografts and allografts rely on harvesting vessels from a patient’s or donor’s arms, leg, chest, and abdomen to replace the damaged vascular compartments (Wang et al., 2022a,b). However, these can cause significant donor site morbidity and can result in multiple post-surgery side effects such as aneurysms, infections, and long-term pain. As a result, there is a need for developing vascular substitutes that are biocompatible, biodegradable, and capable of growth (Singh et al., 2016). Consequently, scientists have created artificial vessel substitutes using polyethylene terephthalate, Goretex®, and Dacron®. Compared to autologous vessels, these synthetic substitutes have an increased risk of thrombosis and limited durability (2 to 5 years). Xenografts have progressed with tissue engineering to reduce immunogenicity and novel immunosuppressants. Human and animal cadavers have been identified as the supply of native vessels for decellularization (Wang et al., 2022a,b).
Decellularized valves have several benefits when used as implants, including preservation of the native tissue’s mechanical properties and original architecture, as well as biocompatibility, which promotes cellular and tissue integration while reducing immunogenicity. On the other hand, hydrogels made from digested decellularized valves offer flexible three-dimensional networks that can be molded into various sizes and forms, and are highly adaptable for different applications by mimicking the ECM environment. Additionally, the sustainable use of waste materials to generate hydrogels from decellularized constructs may offer cost benefits and reduce the risk of immunogenic reactions compared to traditional grafts (Kim et al., 2020).
Such gels can be injected into the body less invasively and engineered to incorporate growth factors, drugs, or cells for regulated release, thereby augmenting the potential for regeneration. Moreover, hydrogels provide temporary structural support and specific biological cues to direct cellular growth until the cells generate their matrix proteins, transforming the scaffold into an alternative valve with characteristics akin to natural valves’ characteristics. Supporting cell adhesion, spreading, and growth in three dimensions is the main objective of hydrogels, necessitating porosity and cytocompatible nature. Because of their crosslinked and hydrophilic architectures, hydrogels are a desirable scaffold material for tissue engineering. They offer a hydrated and porous environment similar to soft tissues and allow for the flow of nutrients, waste products from cells, and oxygen. Another crucial aspect in various cellular behaviors is the proteolytic degradation of the extracellular matrix, which is necessary for cell spreading, migration, and differentiation (Zhang et al., 2015).
Recent studies have highlighted that hydrogels derived from the decellularized extracellular matrix (dECM) of discarded varicose veins provide a tissue-specific microenvironment. This environment effectively directs the differentiation of mesenchymal stem cells into vascular smooth muscle cells, SMCs (Kamaraj et al., 2022), endothelial cells, ECs (Khaki et al., 2018), and vascular interstitial cells, VICs (Ku et al., 2006), or populations with comparable characteristics. Building on the advances in sclerosing solutions and image-guided infusion techniques, this study aimed to analyze how valvular dECM and abdominal adipose tissues could be used to formulate functional hydrogel models from vascular tissues (Figure 2), which can then be infused into the major site of injury to simultaneously facilitate in situ cellular regeneration via ECM restoration and MMP deactivation in early-stage varicose veins (Figure 3). Specifically, abdominal adipose tissues are used for isolating adipose-derived stem cells (ADSCs), which are then differentiated into endothelial cells (ECs), smooth muscle cells (SMCs), and valvular interstitial cells (VICs). The source of these tissues has been specified as human and livestock animal, as illustrated in the model.
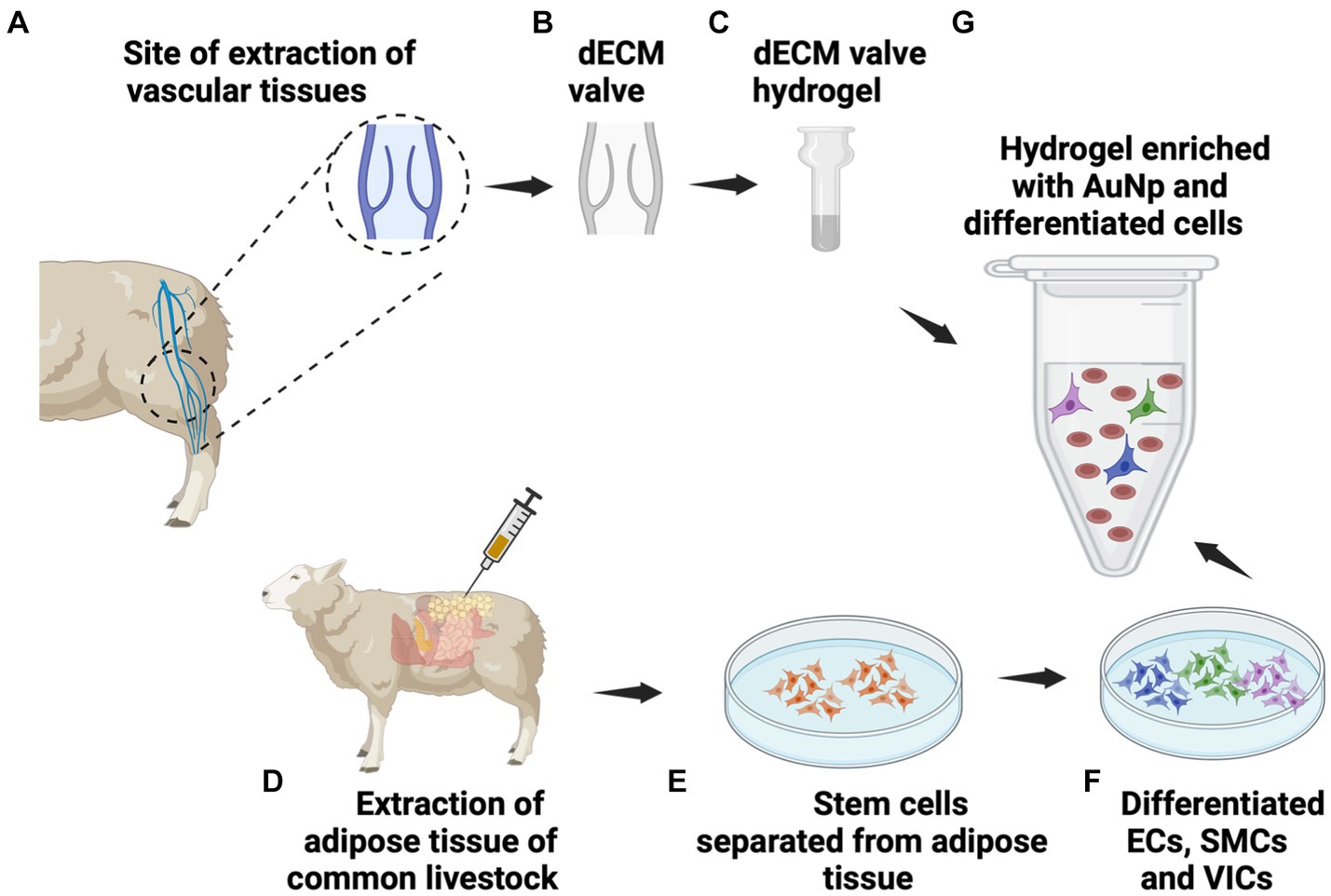
Figure 2. Functional hydrogel formulation. The functional hydrogel can be devised by (A) extracting vascular tissues from cadaveric sources, (B) decellularizing the valves to create dECM, and (C) this dECM can then be lyophilized, ground into powder, digested with pepsin/HCl digested and neutralized to form the hydrogel. Simultaneously, (D) extraction of adipose tissues, (E) provides a source of adipose-derived stem cells (ADSCs) that can be (F) differentiated into ECs, SMCs, and VICs. After that, (G) the tissue-specific hydrogel can be enriched with the various cell types and gold nanoparticles (AuNPs).
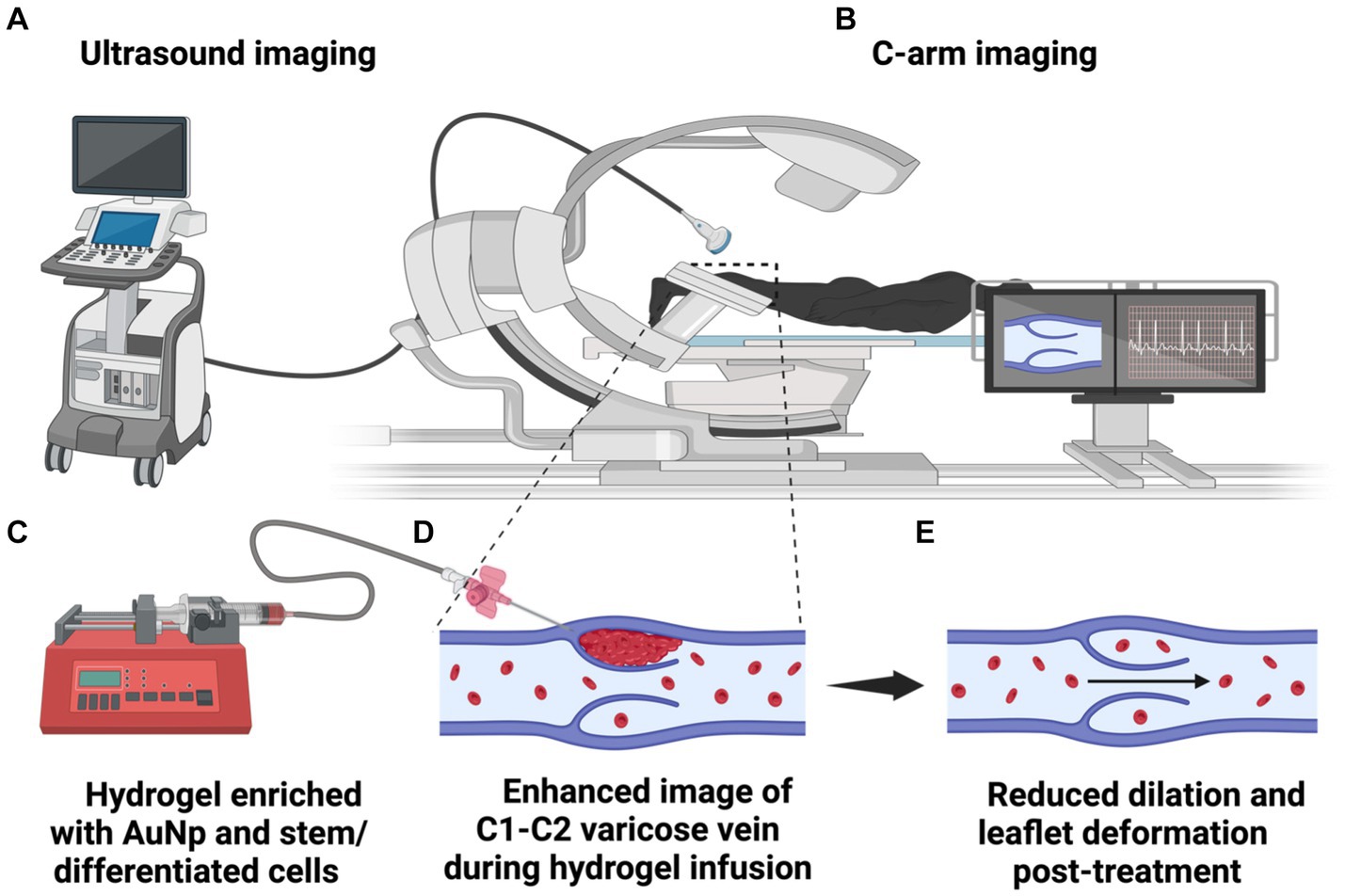
Figure 3. An illustration of image-guided hydrogel infusion for valvular enhancement. The diagram depicts the (A) ultrasound and/or (B) C-arm systems guiding catheter insertion into various valvular regions for hydrogel infusion into the wall and leaflets. (C) The hydrogel, composed of dECM, differentiated cells, and AuNPs, provides a medium matching the native microenvironment that mechanical forces and growth factors can aid valvular restoration and regeneration. (D) Within the infused dECM hydrogel, ECs, SMCs, and VICs contribute to collagen and elastin synthesis, re-endothelialization, and reconstitution of damaged tunics. (E) AuNPs counteract MMP activity, curtailing ECM degradation, vessel wall distension, and the potential propensity to halt disease progression.
This approach not only drives sustainable tissue engineering but also enhances bioeconomic waste management practices by repurposing discarded cadaveric tissues (Zhang et al., 2015; Singh et al., 2016; Kim et al., 2020). It proposes a radical alternative that utilizes tissue engineering to transform agri-food waste from common livestock into viable natural biomaterials (Figure 4). This approach not only effectively addresses the waste reduction challenge but can also be adapted for xenografts without relying on extensive laboratory animal testing, thereby tackling a silent global health crisis sustainably.
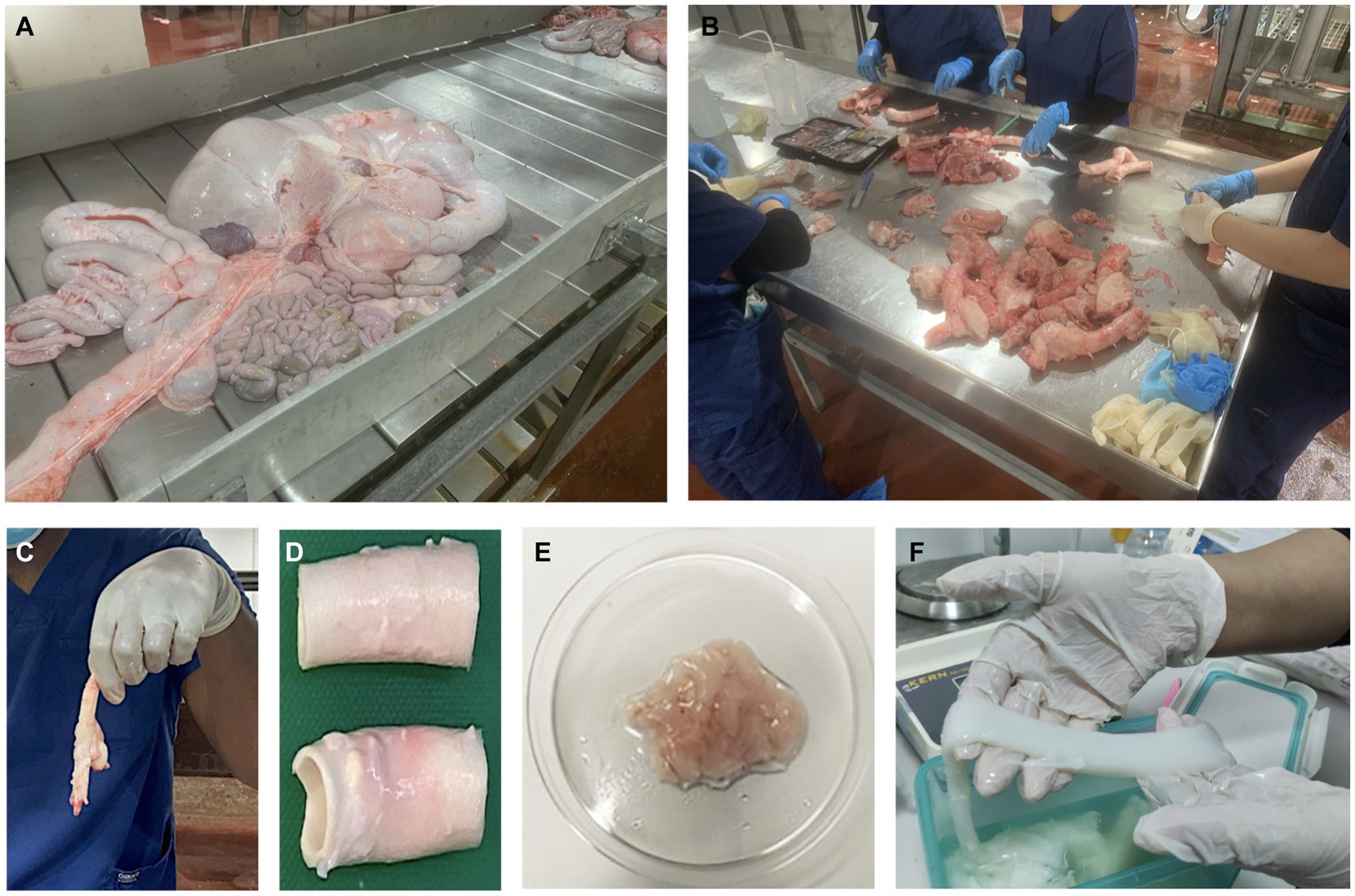
Figure 4. From the slaughterhouse to the lab: extracting natural biomaterials from substantial volumes of common livestock (cows and sheep) during meat processing. (A) Discarded abdominal tissues collected during cow meat processing from which adipose tissues can be extracted. (B) Our expert team shown dissecting tissues to isolate intact vascular substrates. Vascular tissues extracted from the original discarded mass (C) before and (D) after lipectomy to collect perivascular adipose tissue. (E) Extracted adipose tissues after being washed thoroughly with a sterile solution to remove blood and debris, allowing for stem cell extraction. (F) Decellularized tissues that can be used to generate hydrogels.
Methodology
Conceptual analysis
Our model adds to the scholarly literature by questioning the established views on vascular insufficiency. Herein, we propose that the application of a sustainable tissue-engineered hydrogel, synthesized from the dECM of discarded cadaveric vascular tissues as illustrated in Figure 2 and enriched with adipose-derived stem cells (ADSCs), in conjunction with gold nanoparticle (AuNP) metalloproteinase inhibition, as detailed in Figure 3, will result in a notable alleviation of symptoms and delay in the progression of early-stage (C1-C2) varicose vein disease. Additionally, we postulate that this combined intervention will demonstrate additive or synergistic effects, leading to superior outcomes in improved venous function and symptom alleviation, compared to a control group receiving standard care.
Primary clinical outcome measures:
• Quantitative measurement of valve functioning (flow rate, presence or absence of regurgitation using imaging techniques, like ultrasound and venography);
• Assessment of improved venous function through standardized clinical measures; and
• Evaluation of symptom alleviation based on patient-reported outcomes and clinical assessments.
The clinical potential of the proposed therapeutic strategy is evident in its capacity to provide a proficient, personalized, and minimally invasive intervention for early-stage varicose vein disease, developed using sustainable cadaveric models. This potential stems from multifaceted factors, including the early intervention’s capacity to retard disease progression, regenerative attributes, patient-specific tailoring, improvement of the QoL, and reasonable resource utilization with an emphasis on sustainability.
Adipose-derived stem cells (ADSCs)
In our cadaveric models, adipose-derived stem cells (ADSCs) can be harvested from abdominal or perivascular adipose tissue to generate resident cells for valvular leaflets and walls. This approach can then be extended to a minimally invasive procedure that can tailored to the patient. Valvular leaflets, comprising three ECM layers—the elastin-rich lamina ventricularis, proteoglycan-dense lamina spongiosa, and collagen-dense lamina fibrosa (Wang and Khalil, 2018) —along with wall structures containing smooth muscle, endothelial, and fibroblast cells, are considered.
The collection process entails obtaining adipose tissue through biopsy or lipectomy post-slaughter. Following enzymatic digestion, ADSCs can be isolated and hypothetically differentiated into ECs, SMCs, and VICs. Typical phenotypic and genetic expressions will be rigorously monitored throughout this process to ensure precise differentiation (Zhou et al., 2019). ADSCs have the potential to differentiate into fibroblast-like cells, which are crucial connective tissue cells responsible for ECM production and tissue structure maintenance.
As an alternative non-invasive approach, peripheral blood mononuclear cells will be explored. These cells can be reprogrammed into induced pluripotent stem cells (iPSCs) (Murtaza et al., 2024), and ultimately into our target cells (Cai et al., 2024). Specifically, within the spongiosum layer of the valve, the primary valvular cells of interest are specialized fibroblasts and myofibroblasts, pivotal for maintaining the structural and functional integrity of the venous valve.
Hydrogel production
Hydrogels are critical in scaffold technologies and essential to tissue engineering. Their significance lies in their considerable water contents and hydrophilicity, coupled with an ability to mimic the ECM environment. These properties, excellent biocompatibility, and controlled drug-release capabilities make hydrogels invaluable (Luo et al., 2022). Furthermore, they maintain the microenvironment necessary for tissue engineering, promoting cell adhesion and facilitating tissue regeneration. This is mainly due to elements like collagen and fibronectin within these gels, which ensure their biodegradability and allow natural absorption over time (Luo et al., 2022).
Functional hydrogels can be derived by extracting valves from cadaveric or animal donors and decellularizing them to remove any cellular and nuclear matter from native tissue to create the dECM, rich in glycosaminoglycans (GAGs), phosphorylated proteins, collagen, and elastin. This can be done using biological, chemical, or physical techniques to preserve the residual ECM’s composition, biological activity, and mechanical integrity (Wang et al., 2023). Afterward, the tissue is sterilized using either chemical (peracetic acid or ethanol) (Tao et al., 2021), gaseous (ethylene oxide) (de Sousa Iwamoto et al., 2022), or physical (gamma irradiation) treatments (Corridon, 2021; Corridon, 2022; Corridon, 2023). A sterilization process will ensure the resulting structure possesses an aseptic environment with minimal effects on its physical and chemical properties and innate biological activities conducive to the post-transplantation environment. These processes provide additional means of avoiding significant and unwanted physiological events that impede the recellularization and transplantation (Wang et al., 2023). Subsequently, this dECM undergoes lyophilization, transforming it into a powder. The powder is then subjected to digestion with pepsin/HCl, followed by neutralization and non-toxic crosslinking, which can simultaneously be achieved with AuNPs, to create this natural hydrogel, as illustrated in Figure 2 (Shibru et al., 2024) and summarized in the subsequent section.
After gelation, various physicochemical, structural, and thermal techniques can be utilized to characterize the resulting natural hydrogel. These methods are employed to assay the suitability of several critical attributes of the hydrogel, including the density and quality of crosslinking within the fibers or chains, fiber orientation, and overall composition. Additionally, they assess the gel’s strength, the spacing between crosslinked fibers, the proportions of bound and free water within the system, and its capacity for cell loading, growth, division, and migration (Martinez-Garcia et al., 2022).
Previous studies have highlighted the potential for extracting and decellularizing cadaveric tissues to create acellular structures composed of the ECM, in a manner to valorize agri-food waste. These serve as exceptional starting materials for tissue-specific hydrogel formation. Theoretically, this hydrogel can recapitulate the required in vivo milieu for innate cellular function (Sackett et al., 2018). Such a gel, enriched with patient-specific adipose-derived stem cell derivatives of each previously mentioned lineage, leverages the ability to synthesize collagen and elastin (Colazzo et al., 2011), differentiate into multiple cell lineages (Bunnell et al., 2008), and support valvular regeneration through transitions into cells like proteoglycan-secreting vascular smooth muscle cells (Kamaraj et al., 2022) and ECM-secreting fibroblasts with properties phenotypical of vascular interstitial cells (Ku et al., 2006). Endothelial cells can also undergo a mesenchymal transition to become VICs (Rutkovskiy et al., 2017), which populate the three layers of the leaflets and support remodeling/regeneration. Subsequently, the hydrogel can be seeded with patient-specific ECs, SMCs, and VICs, as well as AuNPs obtainable through green/biogenic routes (Menon et al., 2017). AuNPs are renowned for deactivating MMPs, addressing their over-expression or activity, and preventing unwanted ECM degradation (Engin et al., 2017), thus rejuvenating valvular integrity and function, particularly in the early stages of varicose vein disease. In addition to these biological modifiers, dECM-based hydrogels contain several components, namely GAGs, phosphorylated proteins, collagen, and elastin, known to contribute to the sequestration of calcium and phosphate ions. They are crucial in limiting calcification and driving vascular dysfunction.
Due to its unique biological activity and favorable biocompatibility, the dECM is widely utilized in regenerative medicine for scaffolding and additive (Shakeel and Corridon, 2022) manufacturing applications (Zhang et al., 2021). Ideally, to confirm pathogen clearance, viral, bacterial, and prion assessments using PCR- and ELISA-based approaches should be implemented. Additionally, parasitic evaluations employing serological and molecular methods might be conducted, contingent upon the source, to mitigate potential adverse immune responses. This comprehensive testing regimen will also rely on specific decellularization protocols known to produce high-quality dECM and eliminate the abovementioned intra- and inter-species antigens (Sengyoku et al., 2018). Furthermore, the antimicrobial characteristics of AuNPs can be leveraged to alter the permeability of bacterial or fungal cell membranes, thereby eliminating potential pathogens within the infusates (Menon et al., 2017).
Gelation and crosslinking mechanisms
To develop the dECM hydrogels, we initially lyophilize the decellularized extracellular matrix (dECM) derived from cadaveric vascular tissues into a fine powder. This powder is digested using pepsin and hydrochloric acid (HCl) to break down the matrix components into a solubilized form. After digestion, the solution is neutralized to physiological pH, creating a precursor for hydrogel formation. Gold nanoparticles (AuNPs) can then be incorporated as crosslinking agents to enhance the mechanical properties and stability of the hydrogels. The crosslinking mechanism facilitated by AuNPs involves the interaction between the nanoparticles and the matrix proteins, forming a stable network that improves the hydrogel’s structural integrity. This interaction also helps inhibit matrix metalloproteinase (MMP) activity, which is crucial for maintaining the integrity of the ECM and preventing premature hydrogel degradation.
Upon injection into the target tissue, the hydrogel gelation must occur rapidly to ensure it remains in place despite the dynamic environment, such as blood flow in vascular tissues. The rapid gelation is achieved through the physiological conditions (e.g., body temperature and ionic strength) that trigger the transition of the neutralized dECM solution into a gel state. The AuNPs further facilitate this process by promoting quick and effective crosslinking, ensuring the hydrogel forms a stable and cohesive structure immediately upon injection.
dECM-derived hydrogel technologies
Decellularized ECM-based hydrogels, with their unique ability to replicate native ECM properties, present a promising avenue for engineering tissue-specific microenvironments (Kim et al., 2020). Their potential spans various applications, including tissue engineering, disease modeling, and regenerative medicine. The ECM, a complex protein network, provides structural support and influences cellular behavior. Its key components, fibrous proteins, glycoproteins, and bioactive molecules, play crucial roles in cell adhesion, proliferation, migration, and differentiation. The process of decellularization, which removes cellular components while preserving the ECM structure, is facilitated by a combination of treatments:
• Physical methods such as freeze–thaw cycles, sonication, supercritical fluid-based solubilization, and agitation;
• Chemical approaches involving acids, bases, detergents, enzymes like trypsin and DNase, as well as hypertonic or hypotonic solutions; and
• Sterilization techniques, including gamma irradiation, ethylene oxide, or peracetic acid.
Properties of dECM-based bioinks include biocompatibility, where they retain bioactive molecules supporting cell attachment, proliferation, and differentiation, and adjustable mechanical properties for specific tissue types. They offer a native-like environment promoting specific cellular functions. In applications, these bioinks are used in tissue engineering to regenerate cartilage, bone, and cardiovascular tissues and in disease modeling to replicate microenvironments for studying conditions like cancer and fibrosis. dECM-based constructs repair damaged tissues and organs for regenerative medicine applications, facilitating healing and integration. Future research aims to enhance reproducibility, scalability, and functionality, with advancements in 3D bioprinting and multi-material bioinks critical for progress. This proposed sustainable approach can extend their utility (Shibru et al., 2024).
dECM hydrogel synthesis summary
• Tissue collection: extract cadaveric vascular tissues from common livestock animals (e.g., bovine, ovine, or porcine);
• Sterilization: tissue decontamination conducted via chemical, gaseous, or physical treatments;
• Decellularization and scaffold characterization: emersion-based ionic/zwitterionic surfactant treatment coupled with agitation for several days, followed by a rigorous rinsing period with PBS or deionized water to remove cellular debris and residual detergent, and then measure the surface tension of the final wash-out solution and ensure it is roughly that of water, and simultaneously perform histological analysis and DNA quantification assays on the dECM;
• dECM lyophilization: compress the samples to support drying and then subject them to freeze-drying for roughly 24 h;
• Scaffold homogenization and gelation: section decellularized valvular scaffolds into 0.25-inch portions using sterile scissors, grind tissue into a powder, add 1 mg/mL pepsin and 0.01 M HCl, emulsify in a PBS buffer to form a gel, and then neutralize the gel to a pH of 7.4 with 1 M NaOH;
• Cross-linking: perform physical cross-polymerization via 2–3 freeze–thaw cycles with a freezing temperature of −15°C for 20 h and thawing to 5°C for 4 h;
• Hydrogel enrichment with AuNP: add 2–10 nm in varied concentrations (2–40 ug/ml) to the hydrogel and sonicate to disperse to minimize particle aggregation; and
• Hydrogel enrichment with cells and characterization: perform scanning electron microscopy (SEM) to examine surface morphology and structure, then access biomechanical properties (stiffness, elasticity, strength, and deformation behavior), composition (FTIR and mass spectroscopy), and biocompatibility (cell seeding, additional DNA quantification assays, differentiation, cytotoxicity, viability, adhesion, and immune response, and MMP production assays).
Guided infusate delivery
Different imaging techniques, such as Doppler ultrasound and C-arm, are utilized in varicose vein treatment to determine appropriate injection sites. Doppler ultrasound, a non-invasive and radiation-free method, provides real-time visualization of blood flow, aiding in identifying malfunctioning veins. This technique has also been shown to be a suitable method for vein wall thickness measurements compared to histological assessment. Zerrouk et al. (2023) reported duplex ultrasound assessment of the posterior accessory great saphenous vein is possible with the full-thickness wall not only being distinct from surrounding subcutaneous tissues but also easily differentiated into tunica intima, media, and adventitia based on echogenicity. Ongoing improvements in this technology will make the delivery or infusion of hydrogels easier and more accessible.
Conversely, the C-arm, a specialized X-ray device, offers high-resolution images with real-time guidance during procedures, allowing for precise injection of hydrogels into varicose veins. Once the optimal site for injection is determined, a slow-rate infusion pump will administer a uniform delivery of the hydrogel into the spongiosa layer of the valve. A hydrogel will be introduced around the compromised valve to reinforce the vein and, in theory, impede further deformity. Due to the hydrogel’s primary ECM composition, the gel will be strategically positioned in the vein’s media layer to avert clotting. Continuous portable X-ray usage, with contrast, can aid in precise infusion pump placement. Post-procedure, color Doppler ultrasound will oversee valve functionality, providing a way to evaluate blood flow and venous wall integrity. These techniques can help define suitable injection sites. Clinical physical and imaging assessments, with specific emphasis on recognizing early symptoms of venous dysfunction, ideally dysfunctional highlighted by venous segments with valvular reflux, enhanced wall thickness and luminal expansion, and signs of skin ulceration, can also identify areas of potential structural weakness.
The delivery of the hydrogel into the vein wall presents a technical challenge due to the thinness of the venous tissue, typically less than 1 mm. The needle size and the injection technique are critical factors in this process. Administration could be achieved by a 28G needle with an outer diameter of approximately 0.3 mm. While technically challenging, using additional imaging modalities, as discussed above, should facilitate adequate control and placement. Additionally, wall dilation is a crucial aspect of early-stage disease, which can be advantageous for visualization and subsequent needle placement and infusion. Likewise, employing echogenically designed needles allows for enhanced visibility under high-resolution ultrasound, potentially facilitating the precise delivery of the hydrogel even in thin tissues (Hovgesen et al., 2022).
Further investigation into flow rate, pressure, and shear forces will need to be undertaken; however, Aguado et al. (2012) showed that the expected shear forces and even pressure drops through such a needle are not likely to be high enough to significantly disrupt the cell membrane, leading to acute cell death. The authors do note, however, that extensional flow can cause stretching and deformation due to linear velocity. Their proposed solution was to encapsulate cells within a hydrogel carrier to confer mechanical protection by absorbing some of the mechanical forces, distributing the stress more evenly, and reducing the direct force on individual cells. This buffering effect helps maintain cell integrity and viability during and after injection. There is a clear crossover with the intended Infusate described herein, which warrants further investigation. Alternatively, recent developments in microneedle systems for cell delivery provide localized delivery and enhanced cell residence by penetrating the tissue and directly transporting cells to the targets with minimal vascular trauma (Gao et al., 2024). Finally, low-viscosity hydrogel formulations can ensure smooth delivery without applying excessive pressure that could damage the vein wall or the ADSCs. Like other stem cells, ADSCs can be sensitive to mechanical forces, including shear stress, which can influence their behavior and differentiation potential (Zhang et al., 2022). To address this possibility, biochemical pre-conditioning techniques can be applied to enhance cellular resilience to mechanical stresses before their incorporation into the hydrogel (Taheri et al., 2023); after that, in vitro and in vivo assays can be designed to assess ADSCs viability and functionality post-delivery.
Evaluation of the theoretical model
First, it is imperative to ascertain the true nature of cells isolated from adipose tissue and confirm their classification as stem cells. Adipose-derived stem cells should exhibit elevated expression of stem cell-related antigens, such as CD13, CD29, CD44, CD105, and CD166, while demonstrating no expression of hematopoiesis-related antigens CD34 and HLA-DR (Zhu et al., 2008). Consequently, validating the successful differentiation of adipose-derived stem cells is essential to ensure the precise selection of cells for integration into a hydrogel matrix. Given that the predominant cellular population within the valve comprises VICs — mainly quiescent fibroblasts that transition into active fibroblasts within a diseased valve (Mabry et al., 2015) — our objective revolves around encompassing both fibroblasts and interstitial cells, aligning with the diseased varicose valve condition. Thus, we employ immunostaining to discern the presence of active myofibroblasts, with a distinctive marker being alpha-smooth muscle actin commonly found in myofibroblast-like cells (Gould et al., 2012). Moreover, the identification of the valvular interstitial cells is facilitated by detecting the presence of fibroblast-specific protein 1, S100A4 (Witt et al., 2014).
Second, the subsequent step in hydrogelation involves decellularizing discarded human/animal valvular tissues. To ensure the efficacy of decellularization, a battery of tests is imperative post-procedure. DAPI (Delgado et al., 2021) and Hoechst (Corridon, 2021; Corridon et al., 2021; Cai et al., 2022; Corridon, 2022; Corridon, 2023) staining is instrumental in detecting intact nuclear cages or residual DNA in the decellularized material. Likewise, conventional H&E staining is routinely employed to visualize the remaining matrix, while other immunohistochemical staining procedures identify collagen fibers within the matrix (Shupe et al., 2010).
Third, once differentiated cells are integrated into the hydrogel, maintaining the viability of various cellular derivatives becomes paramount. Cell viability is assessed through the calcein AM and ethidium homodimer-1 assay (Garnica-Palafox et al., 2014). Moreover, the metabolic activity of the cells is gauged using the 3-(4,5-dimethylthiazol-2-yl)-2,5-diphenyltetrazolium bromide (MTT) assay, further substantiating cell viability (Kikuchi et al., 2008).
Different techniques can be utilized to assess the success of AuNPs in inhibiting MMP in the hydrogel. For instance, MMP inhibition assays aim to measure the efficacy of AuNPs in curbing the activity of MMPs, vital enzymes for ECM regulation (Hashimoto et al., 2015). Whereas cell viability assays, such as MTT and Alamar Blue, evaluate the health and survival of cells following treatment with MMP inhibitors. Quantitative reverse transcription polymerase chain reaction (RT-qPCR) can be employed to quantify changes in gene expression associated with inflammation and understand the cellular response to inhibition. Lastly, micromorphological analysis via fluorescence and transmission electron microscopy allows the visualization of the structural alterations within cells or tissues caused by MMP inhibition. These techniques can comprehensively explain how MMP inhibition impacts cell behavior and the underlying molecular and structural changes (Hashimoto et al., 2015).
Fourth, from a mechanistic perspective, AuNPs are being extensively researched for their potential in various biomedical applications, notably as inhibitors of MMPs (Passmore et al., 2015). The molecular pathways related to the inhibitory effects of AuNPs on MMPs involve a series of crucial interactions and processes. These include direct binding to the active sites of metzincin proteases due to their small size and high surface area-to-volume ratio, which can lead to adverse alterations in the MMP conformation. Such conformational changes can either block the active site or change its shape, making binding to its natural substrates less effective. In addition, AuNPs can affect MMP synthesis by interfering with the transcription factors or the DNA segments responsible for the expression of MMP genes. These nanoparticles can also alter the reactivity of MMPs. Specifically, MMPs contain thiol (sulfhydryl) groups that are reactive. Gold nanoparticles can interact with these thiol groups, leading to the formation of gold-thiolate complexes. This interaction can inhibit the enzymatic activity of MMPs (Luiz et al., 2022).
The multifaceted impact of AuNPs can drive hydrogel crosslinking, resulting in lower porosity, reduced swelling degree, and slower degradation rates. Compared with their toxic counterparts like glutaraldehyde, this enhances compressive strength, biocompatibility, and cytotoxicity (Tenório et al., 2021). Moreover, AuNPs can inhibit the enzymatic activity of calpain-1. The nanoparticles may bind to the active site or other critical regions of the enzyme, altering its conformation and thereby reducing its ability to interact with MMPs, which are often secreted as inactive proenzymes (pro-MMPs) and require activation to become fully functional. By binding to these regions, AuNPs can inhibit calpain-1, reducing its ability to activate pro-MMPs and consequently decreasing tissue remodeling that contributes to unwanted calcification and valvular incompetence (Wang et al., 2015).
AuNPs can potentially offer several distinct advantages in terms of biocompatibility and versatility related to intracellular and extracellular MMP activation compared to traditional anti-MMP agents (Gonzalez-Avila et al., 2022). Anti-MMP agents comprise multiple molecules that have been investigated for their ability to inhibit the activity of MMPs and provide varied cytotoxic profiles and efficacies that have limited their clinical application (Khan et al., 2020). Nevertheless, both classes of compounds facilitate cation chelation, whereby they bind Zn2+, forming stable complexes, which can also be internalized to enable inhibition with internal and external cellular environments. The sequestration of this cofactor can limit MMP and disintegrin metalloproteinase enzymatic activity while concurrently stabilizing various endogenous MMP inhibitors like tissue inhibitors of metalloproteinases (TIMPs), structure, and function (Murphy, 2011). In comparison, nanoparticle surface coatings or capping agents improve their stability and limit aggregation (Sidhu et al., 2022). For instance, the capping agent polyvinylpyrrolidone (PVP) forms a negative charge on the surface coating that can drive Zn2+ chelation. PVP can also impose its surfactant properties to aid better cellular endocytic uptake (Gergely et al., 2023).
Fifth, the innate components of the dECM-based functional hydrogels can also rely on intrinsic mechanisms to inhibit valvular incompetence. For instance, GAGs, particularly heparin, are known to regulate mineralization by binding to calcium ions, potentially limiting the availability of calcium for the formation of calcium phosphate crystals, which are the primary components of calcific deposits. Likewise, phosphorylated proteins in the ECM can directly bind calcium ions and act as nucleation sites for calcium phosphate. Apart from ionic sequestration, intact ECM structural components like collagen and elastin can provide less favorable substrates for calcification because these fibers offer a physical barrier to nucleation and crystal growth. Moreover, the ECM plays a crucial role in the localization, distribution, and activity of potent matricellular glycoproteins, like osteopontin, which inhibit mineralization (Järveläinen et al., 2009).
Last, post-infusion of the functional hydrogel, vigilant monitoring of the patient is imperative. A comprehensive hemodynamic assessment, encompassing blood flow and pressure gradients, is essential to gauge the evolving valvular function throughout treatment. Histological analyses are crucial to ascertain differentiated cells’ in vivo viability and functionality. Methodologies employing H&E staining, Calcein-AM, and MTT assays are used to evaluate cell viability and metabolic activity. Immunostaining for alpha-smooth muscle actin and S100A4 can also validate valvular interstitial cells and active myofibroblasts within the tissue. Vimentin, a type III intermediate filament protein, is widely used as a marker for VICs because of its robust expression in these cells (Rutkovskiy et al., 2017). Likewise, given the role of VICs in calcific aortic valve disease, osteopontin, a protein associated with bone formation and calcification, is sometimes used as a marker, particularly in diseased states (Passmore et al., 2015). Finally, implementing a post-operative process akin to sclerotherapy proves helpful in monitoring for any signs of adverse reactions, potential clot formation, and impaired mobility using conventional or artificial intelligence approaches can be valuable (Corridon et al., 2022; Davidovic et al., 2022; Pantic et al., 2022; Wang et al., 2022a,b).
Discussion
Devising a novel hydrogel-based therapeutic strategy for treating early-stage (C1-C2) varicose veins requires the examination of potential limitations to the proposed treatment’s efficacy. Primary concerns centered around biocompatibility and potential immune responses to the hydrogel are tied to the challenge of achieving thorough and uniform dECM decellularization, with residual antigens that pose risks to hydrogel performance and elicit undesirable immune responses. The use of AuNPs also introduces challenges due to unclear long-term effects and biocompatibility with vascular tissues, which may pose potential risks of cytotoxicity or unanticipated biochemical interactions. Unfortunately, denatured innate components of the dECM-based functional hydrogels may paradoxically aid the release of sequestered calcium and phosphate ions, facilitating mineralization.
During this experimental phase, the hydrogel’s stability, integration, and function within the venous system are crucial. Concerns include the potential for inconsistent integration and stabilization, which could impede ECM regeneration and result in further vascular injury. Ensuring consistent effectiveness across diverse patient profiles and maintaining process consistency is vital for the broad application and development of the therapy. Given the emphasis of the hypothesis on a synergistic approach to the treatment of early-stage varicose veins, several potential limitations related to immunogenicity, biocompatibility, and stem cell functionality may arise from such a comprehensive intervention.
As a result, it is imperative to address relevant drawbacks that must be overcome. Regarding immunological responses, introducing foreign materials, such as gold nanoparticles and differentiated stem cells, can elicit an immune response in specific individuals, potentially leading to severe immunologic reactions. Furthermore, the inherent variability in individual responses to the treatment necessitates a thorough understanding and management of patient-specific factors influencing outcomes, including underlying health conditions and genetic predispositions.
Biocompatibility is another crucial aspect that must be considered since the successful coexistence of the hydrogel, AuNPs, ECs, SMCs, and VICs within the vessel, aimed at achieving structural reinforcement, is imperative. Determining the optimal concentration of each component and assessing their biocompatibility are crucial considerations. For instance, while AuNPs may offer anti-inflammatory benefits, their potential to inhibit ECM components raises concerns. These particles can also support unwanted protein aggregation, complement activation, and clotting. Balancing these effects is crucial to prevent adverse impacts on the long-term functioning of the hydrogel. Stem cell behavior and fate are also essential aspects, given the intended role of differentiated stem cells is to contribute to tissue repair and facilitate proper functioning. However, ensuring the desired behavior of these cells, without unintended transformations or proliferations, is paramount.
Rigorous scrutiny and control measures are necessary to achieve the therapeutic goals and mitigate potential risks associated with aberrant stem cell behavior. Concerning the infusion process, it is also important to recognize the potential nephrotoxic effects of contrast agents, particularly in high-risk patients with pre-existing renal impairment. In this case, screening assessments can help identify patients who would undergo alternative imaging techniques (Corridon et al., 2006; Neelakanta and Bharti, 2013), including high-resolution and 3D-based non-contrast magnetic resonance imaging, where applicable, given its limited accessibility, substantial costs, and longer procedural times. Likewise, it would be necessary to use the lowest effective volume of contrast agent to achieve sufficient venous visualization and apply adequate pre- and post-procedure hydration to facilitate contrast agent excretion and minimize renal impact. Also, to further address the challenges of identifying appropriate injection sites, we acknowledge the limited resolution in fluoroscopic imaging. Nevertheless, several methods, including digital image magnification, applying image processing techniques that enhance the contrast and sharpness of fluoroscopic images, and combining imaging methods by supplementing fluoroscopy with other modalities, such as ultrasound, help to leverage the real-time guidance of fluoroscopy and the high-resolution ultrasound-based screening.
Addressing these limitations through comprehensive studies and meticulous consideration of various parameters will be instrumental in advancing the understanding of the proposed synergistic approach and optimizing its potential for treating early-stage varicose veins.
It is also essential to balance the implementation costs with the desirable results and health outcomes produced when introducing any treatment. For this process, cost-effectiveness analyses are critical when introducing new interventions, as they provide information on more than the therapeutic effect alone. Such analyses would give insight into the costs of various elements, such as the medical equipment needed, practitioner fees, and clinical overhead. Beneficial outcomes could lower the need for future interventions, improve QoL, and potentially decrease complications leading to costly interventions later on. In a recent network meta-analysis, Kolluri et al. (2020) compared the current state-of-the-art superficial venous therapies for CVI. Their cost–benefit analyses considered the VenaSeal closure system, endovenous laser, radiofrequency, mechanochemical ablation techniques, sclerotherapy, and surgical ligation. The VenaSeal system was best at eliminating postoperative pain, reducing adverse event reoccurrence, and was linked to appreciable QoL ratings.
The VenaSeal system utilizes a proprietary gel-based adhesive that holds the walls of the vein together to facilitate venous ligation and blood flow rerouting to healthy veins. If this proposed hydrogel therapy is effective, it could leverage existing technologies to lessen implementation costs while ultimately impacting the long-term economics of traditional varicose vein treatments. Specifically, similar catheter-infusion interventions can be used to devise the hydrogel infusion process that could prevent the progression of the disease, potentially leading to cost savings by avoiding more invasive future treatments, as well as long-term economic benefits like enhanced QoL.
Future economic analysis, which can be provided by stochastic modeling (Jones et al., 2021) would provide exciting perspectives on real-world insights to patients, payers, and providers. Such a process would entail collecting direct cost and patient outcomes and providing perspective data that can be utilized in a Markov model to simulate patients given various health states. This model can provide insight into the total costs for each therapy and quality-adjusted life years (QALYs) gained by the novel hydrogel therapy compared to existing therapies. Given its novelty and treatment classification, one could also anticipate higher application costs that insurance providers might not cover. Nevertheless, patient insights and feedback can support its adoption (Simone et al., 2020) and reimbursement (Baldie et al., 2018).
Moreover, to assess the approach’s cost-effectiveness, the proposed hydrogel therapy leverages discarded cadaveric tissues from slaughterhouses, which can be an economical source of biomaterials for developing dECM hydrogels (Shibru et al., 2024). By repurposing these waste materials, our approach addresses environmental concerns and reduces the initial costs of sourcing raw materials. However, incorporating adipose-derived stem cells (ADSCs) and gold nanoparticles (AuNPs) introduces additional costs. To evaluate the overall cost-effectiveness, we compared our approach with existing treatments, such as the VenaSeal system, a widely used method for varicose vein therapy. VenaSeal utilizes a medical adhesive to close diseased veins and is cost-effective due to its minimally invasive nature and reduced need for follow-up procedures.
Our proposed hydrogel therapy, although potentially higher in initial costs due to the isolation and differentiation of ADSCs and the inclusion of AuNPs, may offer long-term economic benefits. These benefits include preventing the progression of CVI, reducing the frequency of necessary interventions, and utilizing existing catheter-infusion technologies, which are already in widespread clinical use. Additionally, the sustainable aspect of using repurposed materials contributes to the overall cost savings and environmental benefits. In so doing, we anticipate that the improved integration and functionality of the hydrogels, due to the biocompatible scaffold provided by the dECM and the enhanced mechanical properties conferred by AuNPs, will lead to better patient outcomes. At this juncture, it is important to note Gray et al.’s (2013) previous extraction of silver and gold nanoparticles from biological tissues. Given the previous framework outlined by Jha and Prasad (2016), this novel result can help further leverage waste valorization through green chemistry nanoparticle synthesis, which effectively utilizes goat slaughter waste to synthesize zinc nanoparticles. Overall, these results can, in turn, decrease healthcare costs over time, as successful treatments will reduce the need for additional medical procedures and long-term care associated with CVI complications.
In the future, we propose to assess these impacts quantitatively via a comprehensive life cycle assessment (LCA) to evaluate resource consumption, greenhouse gas emissions, and waste reduction metrics associated with our proposed technology. This LCA will provide empirical data to emphasize the environmental benefits and economic efficiencies achievable through our approach, enhancing the manuscript’s discussion on broader societal implications. Such studies can help address the cost-effectiveness of our proposed technology.
Complementary assessments will also outline the anticipated cost savings compared to traditional treatments for chronic venous insufficiency (CVI), including detailed estimations of treatment costs. This approach will involve comparative analyses with existing therapies, such as the VenaSeal system, considering factors like material costs, procedural expenses, and potential long-term savings from reduced complications and follow-up interventions. By elucidating these financial considerations, we can truly examine the economic viability and affordability of our innovative approach. Thus, as previously stated, the average cost of treatment is currently $5,527 per individual per year (Kolluri et al., 2022), and these proposed studies will better define the cost differential between existing and proposed therapies in the long and short term.
In conclusion, addressing food waste reduction during both consumption and disposal necessitates collaborative ingenuity to enhance the effectiveness of these processes. One innovative approach involves sustainable tissue engineering, exploiting food systems as a source of natural biomaterials. We propose a novel therapeutic strategy utilizing hydrogels derived from the decellularized extracellular matrix (dECM) of discarded cadaveric vascular and adipose tissues from common livestock. This multi-stage strategy includes stem cell differentiation, decellularization, functional hydrogel development, and precise delivery of biological modifiers. Although using livestock waste derivatives to treat patients is not yet common, several preclinical and clinical xenograft models support such waste valorization, with ECM scaffolds frequently used for clinically relevant reconstructive and regenerative investigations (Porzionato et al., 2018; Neishabouri et al., 2022; Khan et al., 2023). Incorporating preliminary results from these tissue sources and implementing rigorous tissue storage and handling protocols will further define the proposed technology’s clinical relevance and applicability (Ali et al., 2024). This approach offers a radical alternative for treating early-stage varicose veins, potentially circumventing current treatments’ limitations, reducing reliance on animal testing, and contributing to waste management in agroecosystems. Ultimately, it positions slaughterhouse-derived tissues as a potentially viable source for clinical translation initiatives following comprehensive tissue screenings, bridging the gap between experimental models and human applications and advancing the ethical use of resources and scientific rigor necessary for clinical adoption.
Ethics statement
The animal study was approved by Animal Research Oversight Committee at Khalifa University of Science and Technology (protocol #H22-036). The study was conducted in accordance with the local legislation and institutional requirements.
Author contributions
PC: Conceptualization, Data curation, Formal analysis, Funding acquisition, Investigation, Methodology, Project administration, Resources, Software, Supervision, Validation, Visualization, Writing – original draft, Writing – review & editing. ZA: Writing – original draft, Writing – review & editing. MS: Writing – original draft, Writing – review & editing. JP: Writing – original draft, Writing – review & editing. IP: Writing – original draft, Writing – review & editing. SP: Writing – original draft, Writing – review & editing.
Funding
The author(s) declare financial support was received for the research, authorship, and/or publication of this article. This work was supported by funds granted to PC from Khalifa University of Science and Technology, grant numbers FSU-2020-25, RC2-2018-022 (HEIC), ESIG-2023-005, 8434000571 and the Center for Biotechnology and the College of Medicine and Health Sciences. The project was also funded by support granted to PC from the Abu Dhabi Automated Slaughterhouse, Municipality of the City of Abu Dhabi, and Creo Medical, Ltd, Chepstow, Monmouthshire, UK. IP and JP received funding from the Ministry of Science, Technological Development and Innovation of the Republic of Serbia grant 451-03-66/2024-03/200110, and the Science Fund of the Republic of Serbia, grant SensoFracTW, No. 7739645.
Acknowledgments
We want to thank Maja Corridon for proofreading the article. We also gratefully acknowledge Yelyzaveta Vitalii Borodynia’s input regarding the initial development of the conceptual analysis. Additionally, we thank Ms. Shouq Ali Alzaabi, Mahra Ahmed Alazeezi, Hamda Alhammadi, and Ghaya Alkamali for their help with the vascular lipectomy process. Igor Pantic and Jovana Paunovic are grateful to the Ministry of Science, Technological Development and Innovation of the Republic of Serbia, project 451-03-66/2024-03/200110.
Conflict of interest
PC and SP would like to declare support from Creo Medical, Ltd., a medical device company headquartered in Chepstow, Monmouthshire, UK.
The remaining authors declare that the research was conducted in the absence of any commercial or financial relationships that could be construed as a potential conflict of interest.
The author(s) declared that they were an editorial board member of Frontiers, at the time of submission. This had no impact on the peer review process and the final decision.
Publisher’s note
All claims expressed in this article are solely those of the authors and do not necessarily represent those of their affiliated organizations, or those of the publisher, the editors and the reviewers. Any product that may be evaluated in this article, or claim that may be made by its manufacturer, is not guaranteed or endorsed by the publisher.
References
Aguado, B. A., Mulyasasmita, W., Su, J., Lampe, K. J., and Heilshorn, S. C. (2012). Improving viability of stem cells during syringe needle flow through the design of hydrogel cell carriers. Tissue Eng. Part A 18, 806–815. doi: 10.1089/ten.tea.2011.0391
Alekseev, P. E., and Belousov, D. A. (2020). Hygienic diagnostics of risk factors for varicose disease in military service. RMMA Rep 39, 17–18. doi: 10.17816/rmmar43191
Ali, Z. M., Wang, X., Shibru, M. G., Alhosani, M., Alfadhli, N., Alnuaimi, A., et al. (2024). A sustainable approach to derive sheep corneal scaffolds from stored slaughterhouse waste. Regen. med. 10, 1–13. doi: 10.1080/17460751.2024.2357499
Antani, M. R., and Dattilo, J. B. (2023). Varicose veins. Treasure Island, FL: StatPearls Publishing LLC.
Aslam, M. R., Muhammad Asif, H., Ahmad, K., Jabbar, S., Hayee, A., Sagheer, M. S., et al. (2022). Global impact and contributing factors in varicose vein disease development. SAGE Open Med 10. doi: 10.1177/20503121221118992
Baldie, D. J., Guthrie, B., Entwistle, V., and Kroll, T. (2018). Exploring the impact and use of patients' feedback about their care experiences in general practice settings-a realist synthesis. Fam. Pract. 35, 13–21. doi: 10.1093/fampra/cmx067
Beckman, J. A. (2002). Diseases of the veins. Circulation 106, 2170–2172. doi: 10.1161/01.CIR.0000036740.75461.80
Bunnell, B. A., Estes, B. T., Guilak, F., and Gimble, J. M. (2008). Differentiation of adipose stem cells. Methods Mol. Biol. 456, 155–171. doi: 10.1007/978-1-59745-245-8_12
Cai, Z., Zhu, M., Xu, L., Wang, Y., Xu, Y., Yim, W. Y., et al. (2024). Directed differentiation of human induced pluripotent stem cells to heart valve cells. Circulation 149, 1435–1456. doi: 10.1161/CIRCULATIONAHA.123.065143
Cai, N., Lai, A. C., Liao, K., Corridon, P. R., Graves, D. J., and Chan, V. (2022). Recent Advances in Fluorescence Recovery after Photobleaching for Decoupling Transport and Kinetics of Biomacromolecules in Cellular Physiology. Polymers (Basel), 14. doi: 10.3390/polym14091913
Colazzo, F., Sarathchandra, P., Smolenski, R. T., Chester, A. H., Tseng, Y. T., Czernuszka, J. T., et al. (2011). Extracellular matrix production by adipose-derived stem cells: implications for heart valve tissue engineering. Biomaterials 32, 119–127. doi: 10.1016/j.biomaterials.2010.09.003
Corridon, P. R. (2021). In vitro investigation of the impact of pulsatile blood flow on the vascular architecture of decellularized porcine kidneys. Sci. Rep. 11:16965. doi: 10.1038/s41598-021-95924-5
Corridon, P. R., Karam, S. H., Khraibi, A. A., Khan, A. A., and Alhashmi, M. A. (2021). Intravital imaging of real-time endogenous actin dysregulation in proximal and distal tubules at the onset of severe ischemia-reperfusion injury. Scientific Reports, 11:8280. doi: 10.1038/s41598-021-87807-6
Corridon, P. R. (2022). Intravital microscopy datasets examining key nephron segments of transplanted decellularized kidneys. Sci Data 9:561. doi: 10.1038/s41597-022-01685-9
Corridon, P. R. (2023). Capturing effects of blood flow on the transplanted decellularized nephron with intravital microscopy. Sci. Rep. 13:5289. doi: 10.1038/s41598-023-31747-w
Corridon, P., Ascázubi, R., Krest, C., and Wilke, I. (2006). Time-domain terahertz spectroscopy of artificial skin (Vol. 6080). SPIE.
Corridon, P. R., Wang, X., Shakeel, A., and Chan, V. (2022). Digital technologies: advancing individualized treatments through gene and Cell therapies, pharmacogenetics, and disease detection and Diagnostics. Biomedicines 10:2445. doi: 10.3390/biomedicines10102445
Costa, D., Andreucci, M., Ielapi, N., Serraino, G. F., Mastroroberto, P., Bracale, U. M., et al. (2023). Molecular determinants of chronic venous disease: A comprehensive review. Int. J. Mol. Sci. 24:1928. doi: 10.3390/ijms24031928
Davidovic, L. M., Cumic, J., Dugalic, S., Vicentic, S., Sevarac, Z., Petroianu, G., et al. (2022). Gray-level co-occurrence matrix analysis for the detection of discrete, ethanol-induced, structural changes in cell nuclei: an artificial intelligence approach. Microsc. Microanal. 28, 265–271. doi: 10.1017/S1431927621013878
de Sousa Iwamoto, L. A., Duailibi, M. T., Iwamoto, G. Y., de Oliveira, D. C., and Duailibi, S. E. (2022). Evaluation of ethylene oxide, gamma radiation, dry heat and autoclave sterilization processes on extracellular matrix of biomaterial dental scaffolds. Sci. Rep. 12:4299. doi: 10.1038/s41598-022-08258-1
Delgado, A. L. J., Carreira, A. C. O., de Carvalho, H. J. C., da Palma, R. K., Sasahara, T. H. C., de Carvalho, C. M. F., et al. (2021). Development of a new decellularization protocol for the whole porcine heart. J. Clin. Transl. Res. 7, 563–574
DePopas, E., and Brown, M. (2018). Varicose veins and lower extremity venous insufficiency. Semin. Intervent. Radiol. 35, 056–061. doi: 10.1055/s-0038-1636522
Dua, A., Desai, S. S., and Heller, J. A. (2016). The impact of race on advanced chronic venous insufficiency. Ann. Vasc. Surg. 34, 152–156. doi: 10.1016/j.avsg.2016.01.020
Engin, A. B., Nikitovic, D., Neagu, M., Henrich-Noack, P., Docea, A. O., Shtilman, M. I., et al. (2017). Mechanistic understanding of nanoparticles’ interactions with extracellular matrix: the cell and immune system. Part. Fibre Toxicol. 14:22. doi: 10.1186/s12989-017-0199-z
Gao, Z., Sheng, T., Zhang, W., Feng, H., Yu, J., Gu, Z., et al. (2024). Microneedle-mediated cell therapy. Adv. Sci. 11:2304124. doi: 10.1002/advs.202304124
Garnica-Palafox, I. M., Sanchez-Arevalo, F. M., Velasquillo, C., Garcia-Carvajal, Z. Y., Garcia-Lopez, J., Ortega-Sanchez, C., et al. (2014). Mechanical and structural response of a hybrid hydrogel based on chitosan and poly(vinyl alcohol) cross-linked with epichlorohydrin for potential use in tissue engineering. J. Biomater. Sci. Polym. Ed. 25, 32–50. doi: 10.1080/09205063.2013.833441
Gergely, L. P., Yüceel, Ç., İşci, Ü., Spadin, F. S., Schneider, L., Spingler, B., et al. (2023). Comparing PVP and polymeric micellar formulations of a PEGylated photosensitizing Phthalocyanine by NMR and optical techniques. Mol. Pharm. 20, 4165–4183. doi: 10.1021/acs.molpharmaceut.3c00306
Gonzalez-Avila, G., Sommer, B., García-Hernandez, A. A., Ramos, C., and Flores-Soto, E. (2022). Nanotechnology and matrix metalloproteinases in Cancer diagnosis and treatment. Front. Mol. Biosci. 9:918789. doi: 10.3389/fmolb.2022.918789
Gould, S. T., Darling, N. J., and Anseth, K. S. (2012). Small peptide functionalized thiol-ene hydrogels as culture substrates for understanding valvular interstitial cell activation and de novo tissue deposition. Acta Biomater. 8, 3201–3209. doi: 10.1016/j.actbio.2012.05.009
Gray, E., Coleman, J., Bednar, A., Kennedy, A., Ranville, J., and Higgins, C. (2013). Extraction and analysis of silver and gold nanoparticles from biological tissues using single particle inductively coupled plasma mass spectrometry. Environ. Sci. Technol. 47, 14315–14323. doi: 10.1021/es403558c
Hashimoto, M., Sasaki, J. I., Yamaguchi, S., Kawai, K., Kawakami, H., Iwasaki, Y., et al. (2015). Gold nanoparticles inhibit matrix metalloproteases without cytotoxicity. J. Dent. Res. 94, 1085–1091. doi: 10.1177/0022034515589282
Hovgesen, C. H., Wilhjelm, J. E., Vilmann, P., and Kalaitzakis, E. (2022). Echogenic surface enhancements for improving needle visualization in ultrasound. J. Ultrasound Med. 41, 311–325. doi: 10.1002/jum.15713
Järveläinen, H., Sainio, A., Koulu, M., Wight, T. N., and Penttinen, R. (2009). Extracellular matrix molecules: potential targets in pharmacotherapy. Pharmacol. Rev. 61, 198–223. doi: 10.1124/pr.109.001289
Jha, A., and Prasad, K. (2016). Synthesis of ZnO nanoparticles from goat slaughter waste for environmental protection. Int. J. Curr. Eng. Technol. 6, 147–151. doi: 10.14741/Ijcet/22774106/6.612016.26
Jones, S., White, N., Holt, T., and Graves, N. (2021). Cost-effectiveness analysis of hydrogel spacer for rectal toxicity reduction in prostate external beam radiotherapy. J. Med. Imaging Radiat. Oncol. 65, 931–939. doi: 10.1111/1754-9485.13311
Kamaraj, M., Giri, P., Mahapatra, S., Pati, F., and Rath, S. N. (2022). Bioengineering strategies for 3D bioprinting of tubular construct using tissue-specific decellularized extracellular matrix. Int. J. Biol. Macromol. 223, 1405–1419. doi: 10.1016/j.ijbiomac.2022.11.064
Kapp, S., Miller, C., and Donohue, L. (2013). The clinical effectiveness of two compression stocking treatments on venous leg ulcer recurrence: a randomized controlled trial. Int. J. Low Extrem. Wounds 12, 189–198. doi: 10.1177/1534734613502034
Khaki, M., Salmanian, A. H., Abtahi, H., Ganji, A., and Mosayebi, G. (2018). Mesenchymal stem cells differentiate to endothelial cells using recombinant vascular endothelial growth factor -A. Rep. Biochem. Mol. Biol. 6, 144–150
Khan, R. L., Khraibi, A. A., Dumee, L. F., and Corridon, P. R. (2023). From waste to wealth: repurposing slaughterhouse waste for xenotransplantation. Front. Bioeng. Biotechnol. 11:1091554. doi: 10.3389/fbioe.2023.1091554
Khan, Y. H., Uttra, A. M., Qasim, S., Mallhi, T. H., Alotaibi, N. H., Rasheed, M., et al. (2020). Potential role of phytochemicals against matrix metalloproteinase induced breast Cancer; an explanatory review. Front. Chem. 8:592152. doi: 10.3389/fchem.2020.592152
Kikuchi, T., Kubota, S., Asaumi, K., Kawaki, H., Nishida, T., Kawata, K., et al. (2008). Promotion of bone regeneration by CCN2 incorporated into gelatin hydrogel. Tissue Eng. Part A 14, 1089–1098. doi: 10.1089/ten.tea.2007.0167
Kim, B. S., Das, S., Jang, J., and Cho, D.-W. (2020). Decellularized extracellular matrix-based bioinks for engineering tissue- and organ-specific microenvironments. Chem. Rev. 120, 10608–10661. doi: 10.1021/acs.chemrev.9b00808
Kolluri, R., Chung, J., Kim, S., Nath, N., Bhalla, B. B., Jain, T., et al. (2020). Network meta-analysis to compare VenaSeal with other superficial venous therapies for chronic venous insufficiency. J. Vasc. Surg. Venous Lymphat. Disord. 8, 472–481.e3. doi: 10.1016/j.jvsv.2019.12.061
Kolluri, R., Lugli, M., Villalba, L., Varcoe, R., Maleti, O., Gallardo, F., et al. (2022). An estimate of the economic burden of venous leg ulcers associated with deep venous disease. Vasc. Med. 27, 63–72. doi: 10.1177/1358863X211028298
König, H., König, H. H., and Konnopka, A. (2019). The excess costs of depression: a systematic review and meta-analysis. Epidemiol. Psychiatr. Sci. 29:e30. doi: 10.1017/S2045796019000180
Ku, C.-H., Johnson, P. H., Batten, P., Sarathchandra, P., Chambers, R. C., Taylor, P. M., et al. (2006). Collagen synthesis by mesenchymal stem cells and aortic valve interstitial cells in response to mechanical stretch. Cardiovasc. Res. 71, 548–556. doi: 10.1016/j.cardiores.2006.03.022
Luiz, G. N. D. A., Hayley, T., Yekta, E., Sameeksha, C., Daniel, Y., Sean, G., et al. (2022). Matrix metalloproteinases: from molecular mechanisms to physiology, pathophysiology, and pharmacology. Pharmacol. Rev. 74:714.
Luo, T., Tan, B., Zhu, L., Wang, Y., and Liao, J. (2022). A review on the Design of Hydrogels with Different Stiffness and Their Effects on tissue repair. Front. Bioeng. Biotechnol. 10:817391. doi: 10.3389/fbioe.2022.817391
Mabry, K. M., Lawrence, R. L., and Anseth, K. S. (2015). Dynamic stiffening of poly(ethylene glycol)-based hydrogels to direct valvular interstitial cell phenotype in a three-dimensional environment. Biomaterials 49, 47–56. doi: 10.1016/j.biomaterials.2015.01.047
Martinez-Garcia, F. D., Fischer, T., Hayn, A., Mierke, C. T., Burgess, J. K., and Harmsen, M. C. (2022). A Beginner's guide to the characterization of hydrogel microarchitecture for cellular applications. Gels 8:535. doi: 10.3390/gels8090535
Menon, S., R S,, and V K, S. (2017). A review on biogenic synthesis of gold nanoparticles, characterization, and its applications. Resour. Efficient Technol. 3, 516–527. doi: 10.1016/j.reffit.2017.08.002
Moon, T., O’Donnell, T. F., Weycker, D., and Iafrati, M. (2023). Impact of lymphedema in the management of venous leg ulcers. Phlebology 38, 613–621. doi: 10.1177/02683555231197597
Murphy, G. (2011). Tissue inhibitors of metalloproteinases. Genome Biol. 12:233. doi: 10.1186/gb-2011-12-11-233
Murtaza, Z. F., Abou Fares, A., AlMuhairi, F., Paunovic, J., Valjarevic, S., Pantic, I. V., et al. (2024). A bioengineered model for reinnervating the decellularized extracellular matrix of corneal scaffolds. Med. Hypotheses 185:111315. doi: 10.1016/j.mehy.2024.111315
Neelakanta, P. S., and Bharti, S. (2013). “De Novo Radio Frequency Ablation Therapy: Application of Unexplored Electromagnetic Spectral Resources of Mm-Wave/THz Band in Clinical Ablation Procedures – A Review”. Journal of Advances in Medicine and Medical Research 3, 1701–1730. doi: 10.9734/BJMMR/2013/4368
Neishabouri, A., Soltani Khaboushan, A., Daghigh, F., Kajbafzadeh, A. M., and Majidi Zolbin, M. (2022). Decellularization in tissue engineering and regenerative medicine: evaluation, modification, and application methods. Front. Bioeng. Biotechnol. 10:805299. doi: 10.3389/fbioe.2022.805299
Pantic, I. V., Shakeel, A., Petroianu, G. A., and Corridon, P. R. (2022). Analysis of vascular architecture and parenchymal damage generated by reduced blood perfusion in Decellularized porcine kidneys using a Gray level co-occurrence matrix. Front. Cardiovasc. Med. 9:797283. doi: 10.3389/fcvm.2022.797283
Passmore, M., Nataatmadja, M., Fung, Y. L., Pearse, B., Gabriel, S., Tesar, P., et al. (2015). Osteopontin alters endothelial and valvular interstitial cell behaviour in calcific aortic valve stenosis through HMGB1 regulation. Eur. J. Cardiothorac. Surg. 48, e20–e29. doi: 10.1093/ejcts/ezv244
Patel, S. K., and Surowiec, S. M. (2023). Venous insufficiency. Treasure Island, FL: StatPearls Publishing LLC.
Porzionato, A., Stocco, E., Barbon, S., Grandi, F., Macchi, V., and Caro, R. (2018). Tissue-engineered grafts from human Decellularized extracellular matrices: A systematic review and future perspectives. Int. J. Mol. Sci. 19:4117. doi: 10.3390/ijms19124117
Raffetto, J. D., and Khalil, R. A. (2008). Mechanisms of varicose vein formation: valve dysfunction and wall dilation. Phlebology 23, 85–98. doi: 10.1258/phleb.2007.007027
Rutkovskiy, A., Malashicheva, A., Sullivan, G., Bogdanova, M., Kostareva, A., Stensløkken, K. O., et al. (2017). Valve interstitial cells: the key to understanding the pathophysiology of heart valve calcification. J. Am. Heart Assoc. 6:e006339. doi: 10.1161/JAHA.117.006339
Sackett, S. D., Tremmel, D. M., Ma, F., Feeney, A. K., Maguire, R. M., Brown, M. E., et al. (2018). Extracellular matrix scaffold and hydrogel derived from decellularized and delipidized human pancreas. Sci. Rep. 8:10452. doi: 10.1038/s41598-018-28857-1
Santiago, F. R., Ulloa, J., Régnier, C., Peudon, T., Braund, E., Fradet-Aubignat, C., et al. (2022). The impact of lower limb chronic venous disease on quality of life: patient and physician perspectives. J. Comp. Effect. Res. 11, 789–803. doi: 10.2217/cer-2022-0054
Sengyoku, H., Tsuchiya, T., Obata, T., Doi, R., Hashimoto, Y., Ishii, M., et al. (2018). Sodium hydroxide based non-detergent decellularizing solution for rat lung. Organogenesis 14, 94–106. doi: 10.1080/15476278.2018.1462432
Shakeel, A., and Corridon, P. R. (2022). Mitigating challenges and expanding the future of vascular tissue engineering-are we there yet? Front Physiol, 13:1079421. doi: 10.3389/fphys.2022.1079421
Shakya, R., Karmacharya, R. M., Shrestha, R., and Shrestha, A. (2020). Varicose veins and its risk factors among nurses at Dhulikhel hospital: a cross sectional study. BMC Nurs. 19:8. doi: 10.1186/s12912-020-0401-8
Shibru, M. G., Ali, Z. M., Almansoori, A. S., Paunovic, J., Pantic, I. V., and Corridon, P. R. (2024). Slaughterhouse waste: a unique and sustainable source for dECM-based bioinks. Regen. Med. 19, 113–118. doi: 10.2217/rme-2023-0194
Shupe, T., Williams, M., Brown, A., Willenberg, B., and Petersen, B. E. (2010). Method for the decellularization of intact rat liver. Organogenesis 6, 134–136. doi: 10.4161/org.6.2.11546
Sidhu, A. K., Verma, N., and Kaushal, P. (2022). Role of biogenic capping agents in the synthesis of metallic nanoparticles and evaluation of their therapeutic potential. Front. Nanotechnol. 3:801620. doi: 10.3389/fnano.2021.801620
Simone, B., Ana Maria, S., and Fernando Tobal, B. (2020). Using patient feedback to drive quality improvement in hospitals: a qualitative study. BMJ Open 10:e037641. doi: 10.1136/bmjopen-2020-037641
Singh, R., Singh, D., and Singh, A. (2016). Radiation sterilization of tissue allografts: A review. World J. Radiol. 8, 355–369. doi: 10.4329/wjr.v8.i4.355
Sritharan, K., Lane, T. R., and Davies, A. H. (2012). The burden of depression in patients with symptomatic varicose veins. Eur. J. Vasc. Endovasc. Surg. 43, 480–484. doi: 10.1016/j.ejvs.2012.01.008
Taheri, S., Ghazali, H. S., Ghazali, Z. S., Bhattacharyya, A., and Noh, I. (2023). Progress in biomechanical stimuli on the cell-encapsulated hydrogels for cartilage tissue regeneration. Biomater. Res. 27:22. doi: 10.1186/s40824-023-00358-x
Tao, M., Ao, T., Mao, X., Yan, X., Javed, R., Hou, W., et al. (2021). Sterilization and disinfection methods for decellularized matrix materials: review, consideration and proposal. Bioact. Mater. 6, 2927–2945. doi: 10.1016/j.bioactmat.2021.02.010
Tenório, F. S., do Amaral Montanheiro, T. L., Santos, A. M. I. D., Silva, M. D. S., Lemes, A. P., and Tada, D. B. (2021). Chitosan hydrogel covalently crosslinked by gold nanoparticle: eliminating the use of toxic crosslinkers. J. Appl. Polym. Sci. 138:49819. doi: 10.1002/app.49819
Wang, X., Chan, V., and Corridon, P. R. (2022b). Decellularized blood vessel development: current state-of-the-art and future directions. Front. Bioeng. Biotechnol. 10:951644. doi: 10.3389/fbioe.2022.951644
Wang, X., Chan, V., and Corridon, P. R. (2022a). Acellular tissue-engineered vascular grafts from polymers: methods, achievements, characterization, and challenges. Polymers 14:4825. doi: 10.3390/polym14224825
Wang, X., Elbahrawi, R. T., Abdukadir, A. M., Ali, Z. M., Chan, V., and Corridon, P. R. (2023). A proposed model of xeno-keratoplasty using 3D printing and decellularization. Front. Pharmacol. 14:1193606. doi: 10.3389/fphar.2023.1193606
Wang, X., and Khalil, R. A. (2018). Matrix metalloproteinases, vascular remodeling, and vascular disease. Adv. Pharmacol. 81, 241–330. doi: 10.1016/bs.apha.2017.08.002
Wang, M., Kim, S. H., Monticone, R. E., and Lakatta, E. G. (2015). Matrix metalloproteinases promote arterial remodeling in aging, hypertension, and atherosclerosis. Hypertension 65, 698–703. doi: 10.1161/HYPERTENSIONAHA.114.03618
Witt, W., Büttner, P., Jannasch, A., Matschke, K., and Waldow, T. (2014). Reversal of myofibroblastic activation by polyunsaturated fatty acids in valvular interstitial cells from aortic valves. Role of RhoA/G-actin/MRTF signalling. J. Mol. Cell. Cardiol. 74, 127–138. doi: 10.1016/j.yjmcc.2014.05.008
Wu, Z., and Ma, Y. (2021). A narrative review of the quality of life scales specific for chronic venous diseases. Medicine 100:e25921. doi: 10.1097/MD.0000000000025921
Youn, Y. J., and Lee, J. (2019). Chronic venous insufficiency and varicose veins of the lower extremities. Korean J. Intern. Med. 34, 269–283. doi: 10.3904/kjim.2018.230
Zerrouk, S., Casoni, P., Cervi, E., Amirat, R., Bousquet, G., Leboeuf, C., et al. (2023). Ultrasound assessment is linked to histological vein wall thickness in chronic venous disease. Phlebology 38, 270–280. doi: 10.1177/02683555231160896
Zhang, W., Du, A., Liu, S., Lv, M., and Chen, S. (2021). Research progress in decellularized extracellular matrix-derived hydrogels. Regen. Ther. 18, 88–96. doi: 10.1016/j.reth.2021.04.002
Zhang, X., Xu, B., Puperi, D. S., Wu, Y., West, J. L., and Grande-Allen, K. J. (2015). Application of hydrogels in heart valve tissue engineering. J. Long Term Eff. Med. Implants 25, 105–134. doi: 10.1615/JLongTermEffMedImplants.2015011817
Zhang, X., Zhang, S., and Wang, T. (2022). How the mechanical microenvironment of stem cell growth affects their differentiation: a review. Stem Cell Res. Ther. 13:415. doi: 10.1186/s13287-022-03070-0
Zhou, Z. Q., Chen, Y., Chai, M., Tao, R., Lei, Y. H., Jia, Y. Q., et al. (2019). Adipose extracellular matrix promotes skin wound healing by inducing the differentiation of adipose-derived stem cells into fibroblasts. Int. J. Mol. Med. 43, 890–900. doi: 10.3892/ijmm.2018.4006
Keywords: venous insufficiency, decellularized extracellular matrix (dECM), dECM hydrogel, adipose-derived stem cells, valvular interstitial cells (VICs), gold nanoparticles (AuNPs)
Citation: Corridon PR, Ali ZM, Shibru MG, Paunovic J, Pantic IV and Preston SH (2024) Sustainable varicose vein therapy using functionalized hydrogels derived solely from livestock waste. Front. Sustain. Food Syst. 8:1434977. doi: 10.3389/fsufs.2024.1434977
Edited by:
Christian Bux, University of Foggia, ItalyReviewed by:
Paola Sanjuan Alberte, Associação do Instituto Superior Técnico de Investigação e Desenvolvimento (IST-ID), PortugalDong-Woo Cho, Pohang University of Science and Technology, Republic of Korea
Copyright © 2024 Corridon, Ali, Shibru, Paunovic, Pantic and Preston. This is an open-access article distributed under the terms of the Creative Commons Attribution License (CC BY). The use, distribution or reproduction in other forums is permitted, provided the original author(s) and the copyright owner(s) are credited and that the original publication in this journal is cited, in accordance with accepted academic practice. No use, distribution or reproduction is permitted which does not comply with these terms.
*Correspondence: Peter R. Corridon, peter.corridon@ku.ac.ae