- 1Key Laboratory of Modern Agricultural Equipment and Technology (Jiangsu University), Ministry of Education, School of Agricultural Engineering, Jiangsu University, Zhenjiang, China
- 2College of Agricultural Equipment Engineering, Henan University of Science and Technology, Luoyang, China
Rapid assessment and prevention of diseases caused by foodborne pathogens is one of the existing food safety regulatory issues faced by various countries, and it has received wide attention from all sectors of society. When the content of foodborne pathogens in food is higher than the limit standard and spreads in a certain way, it can cause disease outbreaks, which seriously threaten human health or life safety. Developing a novel method to detect foodborne pathogens accurately and rapidly is significant. Because of the limitations of complex steps, time-consuming, low sensitivity or poor selectivity of commonly used methods, a photoelectrochemical (PEC) biosensor based on electrochemistry is developed. Its advantages include a low background signal, fast response and simple operation. It also has broad application prospects for sensing, which has attracted wide attention. However, an organized summary of the latest PEC biosensors for foodborne pathogen sensing has not been reported. Therefore, this review introduces the recent advances in foodborne pathogen detection using PEC biosensors as follows: (i) the construction of PEC biosensors, (ii) the research status of PEC biosensors for the detection of foodborne pathogens and (iii) the direction of future development in this field. Hopefully, the study will provide some insight into developing more mature bio-sensing strategies to meet the practical needs of foodborne pathogen surveillance.
1 Introduction
Foodborne pathogens are microorganisms that enter humans or animals via ingestion and cause infectious or toxic diseases (Schirone et al., 2019). Foodborne diseases are an ongoing and worldwide public health problem, seriously endangering human life. According to a report by the World Health Organization in June 2021, up to 600 million cases of foodborne diseases are reported every year. About one in 10 people get sick from eating contaminated food, and the situation of foodborne diseases is more severe in developing countries than in developed countries (Todd, 2020). Food safety has become a hot topic of common concern across the whole of society (Scharff et al., 2016). Although the government is highly concerned about this issue, foodborne illnesses remain stubbornly high. The Centers for Disease Control and Prevention (CDC) estimates that one in six people in the United States are affected each year, and that up to 3,000 die from eating contaminated food (Scallan et al., 2011).
Common foodborne pathogens include Listeria monocytogenes (Taylor et al., 2019; Vongkamjan et al., 2017; Capita et al., 2019), Staphylococcus aureus (S. aureus) (Kümmel et al., 2016; Li et al., 2022; Argudín et al., 2012), Vibrio parahaemolyticus (Miles et al., 1997; Sun et al., 2016; Li LingZhi et al., 2019), Salmonella (Paniel and Noguer, 2019; Pye et al., 2023; Han et al., 2017) and Escherichia coli (E. coli) (Leng et al., 2022; Munekata et al., 2020; Lima et al., 2017) (Table 1). These pathogens have different tolerance to the external environment, and the foods they contaminate also differ (Guldimann and Johler, 2018). The prevention of foodborne diseases is mainly achieved by washing food, and keeping raw from cooked foods separate (Todd, 2020; Palomino-Camargo et al., 2018). Nevertheless, pathogenic bacteria can still enter processed foods at the packaging, transportation and marketing stages, making it challenging to eliminate contamination through these means. As a result, there is a need to focus on methods for rapid and early detection of pathogenic bacteria to significantly reduce the incidence of foodborne diseases.
Currently, traditional detection methods for foodborne pathogens include microbial and immunological detection (Liu et al., 2023; Xu et al., 2021). These methods can be used for the qualitative and quantitative detection of foodborne pathogens with reliable results. However, they are labor-intensive and time-consuming (Hao and Wang, 2016). In recent years, many optical biosensors have been used for the detection of foodborne pathogens (Xu et al., 2021, 2023), such as fluorescent (FL) biosensors (Liu et al., 2021), colorimetric (CL) biosensors (Zhu S. et al., 2021) and surface-enhanced Raman scatting (SERS) biosensors (Chen et al., 2017). FL biosensors are highly sensitive, but the instrument is very expensive (Liu et al., 2022). CL biosensors enable rapid detection, while their sensitivity is easily limited by color changes (Acunzo et al., 2022). SERS biosensors are simple to operate, but SERS probes are easily affected by the environment, resulting in signal fluctuations (Zhu et al., 2023). Compared with optical biosensors, electrochemical (EC) biosensors have the advantages of high sensitivity and low detection limits, and they have been increasingly used to detect foodborne pathogens (Wang J. et al., 2024; Yu et al., 2021; Wang B. et al., 2023). In particular, photoelectrochemical (PEC) biosensors developed based on EC biosensors have been widely explored in pathogen detection due to their advantages of high sensitivity, simple apparatus, quick detection and low cost (Ding et al., 2021; Zhang Z. et al., 2021). Some reviews have focused on the progress in PEC bio-sensing technologies and their applications for detecting various analyzes, such as metal ions, tumor markers, nucleic acids and antibiotics (Shi et al., 2022; Zhao et al., 2016; Bettazzi and Palchetti, 2018; Dong et al., 2022). Although PEC biosensors have been successfully used for the detection of foodborne pathogens (Wang X. et al., 2024; Ma et al., 2023; Yang G. et al., 2020; Yang H. et al., 2020; Jiang et al., 2024; Zhao et al., 2024; Chen et al., 2024), as far as is known, there has been no review of PEC biosensors for monitoring foodborne pathogens. Accordingly, this paper reviews the application of PEC biosensors in detecting foodborne pathogens. As shown in Figure 1, this review introduces the principle of the PEC biosensor. It summarizes the research progress of the PEC biosensor in the detection of foodborne pathogens in recent years, including the design of photoactive materials (such as improving the photoelectric conversion efficiency of photoactive materials and broadening the spectral absorption of photoactive materials) and optimization of sensing strategies (such as interface regulation, signal amplification and dual-signal output). Meanwhile, the development prospects and challenges of the PEC biosensor in detecting foodborne pathogens have been discussed in detail. Hopefully, this review provides broad applications and beneficial insights for scientists in diverse fields, including food safety monitoring, drug detection, medical diagnosis and environmental monitoring.
2 Brief overview of PEC biosensors
2.1 The principle of photocurrent generation
The PEC method is an emerging analytical technique based on EC, which interfaces with many disciplines, such as optics, electrochemistry, surface science and solid-state physics (Zhao et al., 2014; Sun et al., 2022; Zhao et al., 2015). It is generally believed that photoactive materials can absorb photons with sufficient energy to produce electron–hole pairs. The created carriers are then transferred to the electrodes, which generate an electrical signal in the electrolyte and convert energy through the redox reaction involved. These unique properties of photochemistry have resulted in its widespread use in energy harvesting and environmental pollution mitigation (Lv et al., 2022; Dong et al., 2020).
Notably, the PEC sensor is an innovative and promising technology that integrates PEC processes and sensing. Its underlying principle is that when the surface of the photoactive materials (semiconductor materials, organic photoelectric materials, noble metals with various nanostructures, etc.) is irradiated such that the energy of the exciting light is greater than or equal to the band gap width of photoactive material, photo induced electron transfer reaction will occur in the photoactive material (Ge et al., 2019). The separation of electron–hole pairs occurs when electrons transition from the valence band (VB) to the conduction band (CB). This makes the excited photogenerated electrons unstable, and some will be transferred to the electrode surface or electrolyte. In contrast, others recombine the photogenerated holes of the VB. If the photogenerated electrons at the CB are transferred to the electrode surface, an anode photocurrent is generated. A cathode photocurrent is generated if the photogenerated electrons at the CB are transferred to the electrolyte and consumed by the electron acceptors in the electrolyte.
The generally accepted PEC mechanism consists of four successive steps: (i) the absorption of photons, (ii) the separation of electron–hole pairs, (iii) the migration and recombination of electron–hole pairs and (iv) the utilization of electron–hole pairs. This process involves the generation of electrical signals at the interface and active participation in redox reactions (Sivula and van de Krol, 2016; Zang et al., 2017). The cumulative effect of these processes determines both the photoelectric conversion efficiency and the strength of the output signal (Figure 2A). When the concentration of the target changes, the electrical signal will change accordingly, so the target can be quantitatively detected through the change in the PEC signal. Current PEC sensors are basically amperometric biosensors (Wang et al., 2018). The presence of the analyte can cause a change in the photoactive material or electrolyte environment, which can affect one or more of the above processes. This results in a change in the PEC signal and facilitates the quantitative analysis process. Therefore, the detection signal can be processed by a variety of methods, which provide an effective way to detect different targets (Shu and Tang, 2019).
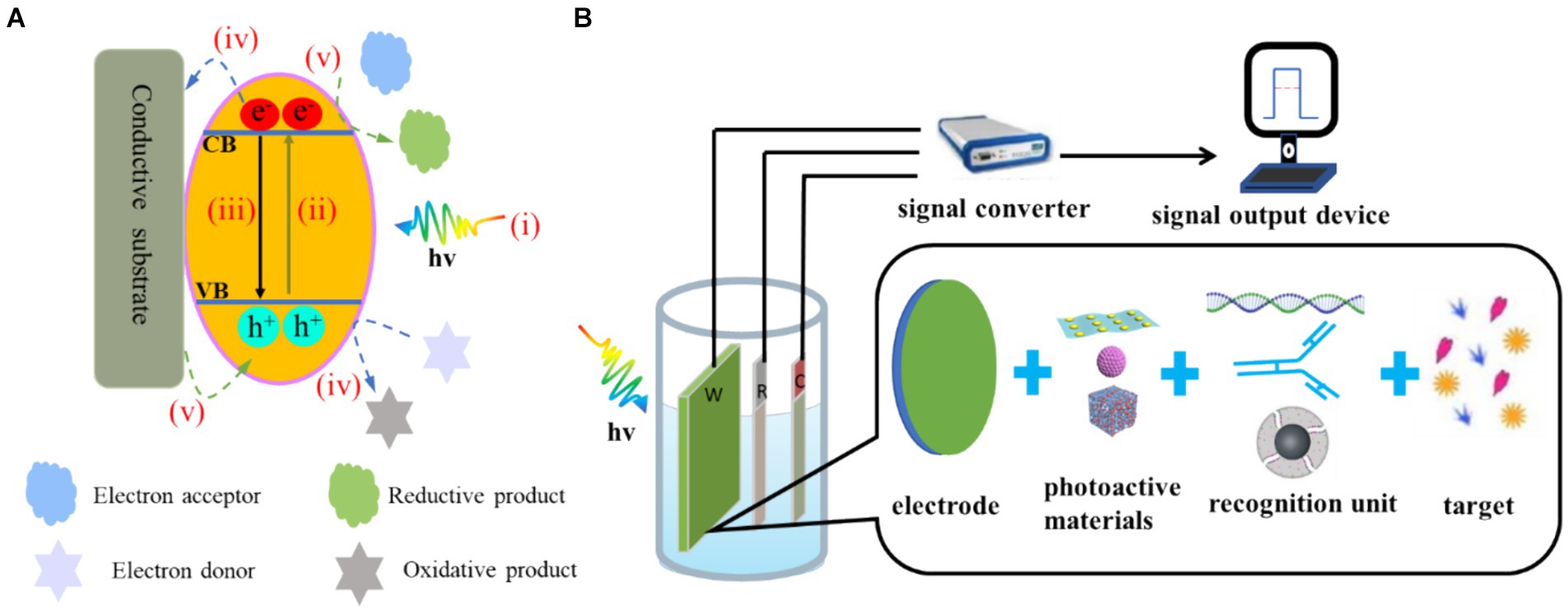
Figure 2. (A) Electron–hole generation and transfer as well as possible recombination pathways on the photoelectrode: (i) photon absorption, (ii) electron transfer, (iii) electron–hole recombination. (iv) For n-type semiconductors, the electrons in the CB flow to the electrode and the photogenic holes in the VB oxidise the reducing substance in the electrolyte to produce the anode photocurrent. (v) For p-type semiconductors, the electrons of the electrode flow to the photogenerated holes in the VB and the photogenerated electrons in the CB reduce the oxidising substances in the electrolyte, resulting in the cathode photocurrent. (B) Composition of PEC detection system.
2.2 Composition and characteristics of the PEC biosensor
The PEC detection system is shown in Figure 2B. This system consists of three parts: an excitation light source, a test device (three-electrode system and electrochemical workstation) and a signal output device (Shu and Tang, 2019). Compared with EC biosensors, PEC biosensors have two key elements: (i) photoactive materials (Dini et al., 2016; Yan et al., 2021) and (ii) bio-recognition elements (Mitsubayashi et al., 2022; Cesewski and Johnson, 2020). Photoactive materials have a photoelectric conversion effect. Bio-recognition elements include enzymes, antibodies and nucleic acids.
In PEC detection, light is used as the excitation source to excite the photoactive substance, and the electrical signal generated by photoexcitation is the detection signal. Because the excitation signal and detection signal of the PEC process belong to different energy forms, the background of the sensing signal is lower than that of the traditional electrochemical method, and the high signal-to-noise ratio is conducive to further reducing the detection limit (Jiang et al., 2021). Due to the uniqueness of the bio-recognition elements, a specific biological reaction occurs with the target. The electrochemical workstation and signal output device are easy to integrate and miniaturise, so the technology has the numerous benefits of simple operation, fast response and portable equipment (Fu et al., 2020; Tao et al., 2023).
3 Construction of PEC biosensors for sensitive detection of foodborne pathogens
Establishing a rapid and sensitive PEC analytical method is one of the important means of ensuring global food safety and minimizing public health problems. Currently, most studies on the PEC detection of foodborne pathogens mainly focus on improving the performance of photoactive materials and optimizing sensing strategies, as shown in Table 2.
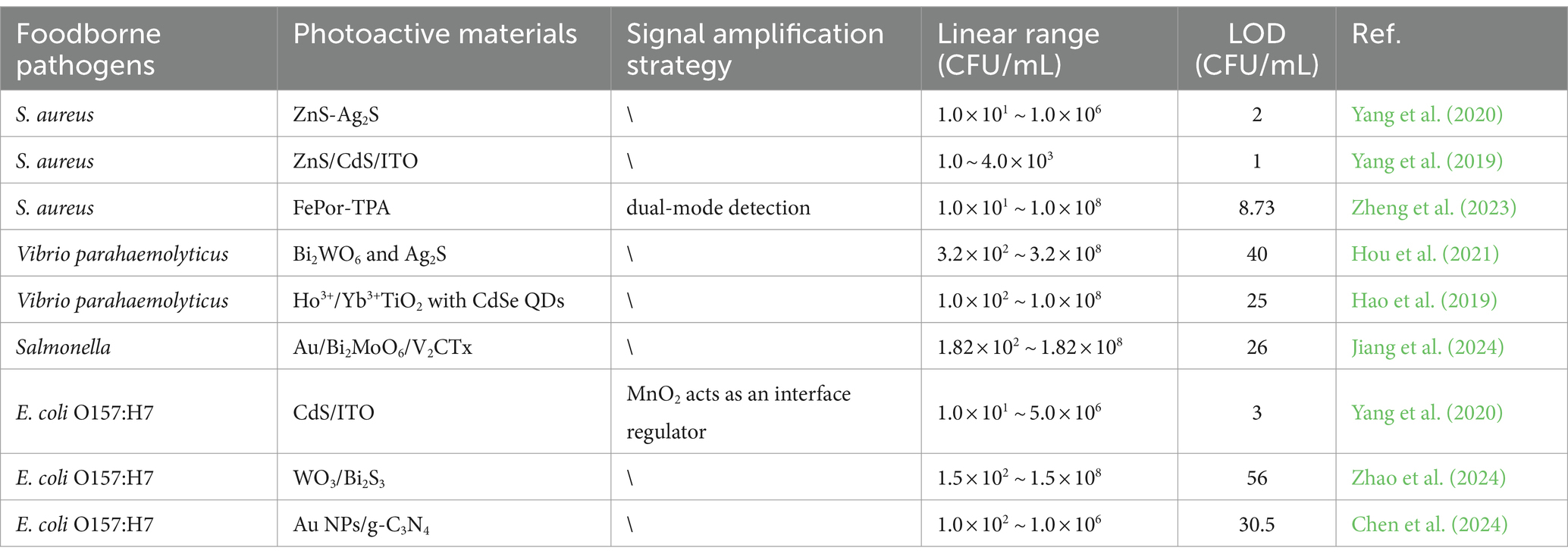
Table 2. Characterization of PEC analytical properties based on different photoactive materials or signal amplification strategy for the determination of foodborne pathogens.
3.1 Improving the performance of photoactive materials
The critical part of a PEC biosensor is the photoelectric conversion unit. Therefore, selecting the photoactive material is a key factor in the sensing performance of a PEC sensor. To date, different types of photoactive materials with distinct energy levels have been effectively utilized to develop PEC biosensors. For example, inorganic semiconductor materials (metal oxides and metal sulphides, etc.) (Wang et al., 2018; Zhu et al., 2019; Shu et al., 2018), organic small molecules, organic polymers and other common organic semiconductor materials (Zhu et al., 2015; Haddour et al., 2004; Zhou et al., 2019), graphite-phase carbon nitride, metal–organic frameworks, perovskites, quantum dots (QDs) and other new photoactive materials (Yang X. et al., 2019; Zhang G. et al., 2018; Li et al., 2019; Shi et al., 2018) have attracted extensive research interest due to both their optical and electrical-related properties. However, due to the shortcomings of a single photoactive material, such as a wide band gap, poor photon absorption ability or toxicity, it is challenging to meet the requirements for actual analysis and testing. Thus, it is necessary to improve the performance of photoactive materials to obtain high photoelectric conversion efficiency by speeding up electron transfer and broadening spectral absorption.
3.1.1 Improving the photoelectric conversion efficiency of photoactive materials
As mentioned above, to address these shortcomings of a single photoactive material, various strategies have been employed to improve the photoelectric conversion efficiency of individual semiconductors, such as doping heteroatoms or forming semiconductor heterojunctions (Sheng et al., 2020; Li et al., 2024). Element doping in the photoactive material effectively regulates the semiconductor energy band, reducing the band gap energy and inhibiting photogenerated electron–hole pair recombination (Raut et al., 2017). For example, Hou et al. (2021) combined rare earth-doped Bi2WO6 and Ag2S as photoactive materials. The doped rare-earth ions in the Bi2WO6 matrix can effectively inhibit the recombination of photogenerated electron–hole pairs and improve the photocurrent response of Bi2WO6, thereby developing a PEC aptasensor for the detection of Vibrio parahaemolyticus (Figure 3A). The constructed PEC aptasensor exhibited excellent specificity, stability and reproducibility. By co-doping TiO2 with Ho3+ and Yb3+, Hao et al. (2019) broadened the spectral response range of TiO2 to the infrared region, improved the photocurrent response of TiO2 and realized the sensitive detection of Vibrio parahaemolyticus. At the same time, building a heterostructure with two semiconductors is considered one of the most popular methods for obtaining photoelectrodes with the desired properties (Low et al., 2017). Band structure and energy level matching are the main considerations in constructing semiconductor–semiconductor heterojunctions (Long et al., 2021). Zhao et al. (2024) modified Bi2S3 through continuous ion layer adsorption and reaction on the WO3 electrode surface to form heterojunction, reduced electron–hole pair complexation and realized rapid detection of E. coli. Yang et al. (2019) synthesized ZnS/CdS heterojunction nanoparticles by electrodeposition on an ITO electrode in one pot, achieving the sensitive detection of S. aureus. The linear range of PEC detection for S. aureus is 1.0 ~ 4.0 × 103 CFU/mL under ideal circumstances. The detection of S. aureus in the milk and juice samples was effectively accomplished using the proposed method (Figure 3B). These results indicate that this is an effective strategy to enhance the photocurrent signal by element doping or forming a heterojunction. In addition, it is expected to further improve the performance of PEC biosensors by introducing new doping elements (such as lanthanide) (Arumugam et al., 2022) or constructing new heterojunctions (such as S-type heterojunctions) (Xiao et al., 2023).
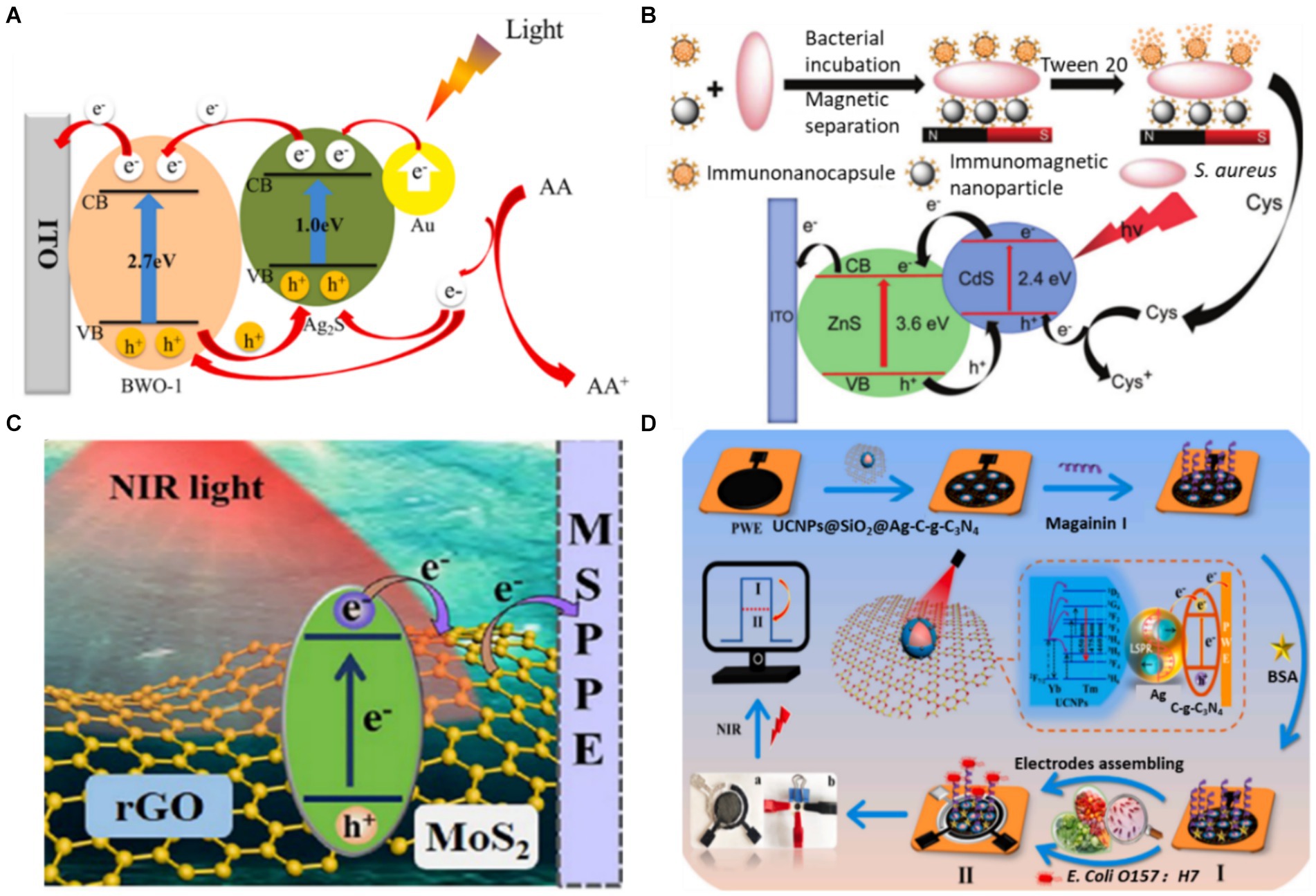
Figure 3. (A) Schematic diagram of photogenic electron transfer mechanism of aptamer sensor (Hou et al., 2021). (B) Illustration of the PEC detection of S. aureus (Yang H. et al., 2019). (C) Schematic of the PEC process on a GMS-modified electrode (Ge et al., 2022). (D) PEC response of UCNPs@SiO2@Ag/C-g-C3N4 under NIR light (980 nm) and the fabrication process of a PEC sensor for the detection of E. coli (Yin et al., 2022). Ascorbic acid (AA), cysteine (Cys), rGO-MoS2 sheets (GMS), up-converting nanophosphors (UCNPs), bovine serum albumin (BSA).
3.1.2 Widening the optical absorption range of photoactive materials
Up to now, plenty of efforts have been devoted to investigating photosensitive materials. Most current PEC sensors are excited by ultraviolet–visible (UV–Vis) light (Chen et al., 2020), which hampers their future applications in bioanalysis (e.g., in vivo detection) due to the intrinsic limitation of short penetration of UV/Vis light. The efficient utilization of sunlight, especially wavelengths in the near-infrared (NIR) region, is particularly important for PEC biosensors, as NIR light accounts for the largest proportion of sunlight, at nearly 50% (Hsieh et al., 2020). In contrast to UV light, NIR light exhibits minimal phototoxicity and is highly compatible with living organisms. This advantageous biocompatibility makes NIR light an ideal choice for PEC biosensors that involve the detection of biological macromolecules (Lv et al., 2020). NIR PEC sensing materials have mainly been divided into direct NIR photoactive materials and indirect NIR photoactive materials (Yang et al., 2021). Direct NIR photoactive materials were constructed using narrow-bandgap photoactive materials, and indirect NIR photoactive materials were constructed using up-conversion nanomaterials.
The semiconductor bandgap directly affects the light efficiency of a photocatalysis reaction. A semiconductor with a wider bandgap can absorb higher-energy photons. As the semiconductor absorbs short wavelengths of light with high photonic energy, it can take advantage of only the smaller range of the solar spectrum concentrated in the UV region. Conversely, a semiconductor with a narrower bandgap can absorb lower-energy photons. Narrow-bandgap semiconductors absorb long wavelengths of light, thus utilising the solar spectrum from the Vis region to the NIR region (Feng et al., 2022). Therefore, controlling the narrow bandgap of semiconductors benefits the absorption of NIR light. Ge et al. (2022) reported an NIR-response PEC immunosensor for the ‘on’ analysis of E. coli O157:H7 under 980 nm light irradiation (as shown in Figure 3C). The form of narrow-band gap MoS2 is regulated by rGO to promote carrier generation and migration. On the sensing platform, E. coli O157:H7 was detected in concentrations ranging from 5.0 ~ 5.0 × 106 CFU/mL, and the LOD was only 2.0 CFU/mL. Subsequently, this research group combined NIR-responsive materials with polar-flipping strategies in their follow-up work, expanding the scope of application of NIR-responsive PEC materials (Ge et al., 2023).
The construction of the indirect NIR PEC sensor is based on the inverse Stokes effect of the up-conversion material, which converts incident light with long wavelengths into emitted light with shorter wavelengths (Sakamoto et al., 2022). Due to its long luminous life, strong optical stability and large anti-Stokes displacement, the up-conversion material can be introduced from FL to the PEC sensing field as a light source converter (Wang G. et al., 2023). Combining it with appropriate PEC active materials can establish an indirect NIR PEC sensing platform. Yin et al. (2022) established an NIR PEC sensing platform for the ultra-sensitive detection of E. coli O157:H7 by assembling a flexible conducting paper electrode with core-shell up-converting nanophosphors (UCNPs)@SiO2. As shown in Figure 3D, the presence of Ag nanoparticles significantly boosted the up-conversion luminescence of the UCNPs, promoting the separation and transport of photoelectrons through local surface plasmon resonance effects. The NIR PEC sensor successfully detected E. coli O157:H7 with a detection limit as low as 2.0 CFU/mL. It was effectively utilized to determine the presence of E. coli O157:H7 in contaminated pork, cabbage and milk samples. In the past two decades, the PEC analysis has received extensive attention and has made significant progress. However, research on NIR PEC sensing is still in its initial stage. Future research could focus on developing new NIR light-responsive materials and miniaturized photoelectrodes and further applying them to foodborne pathogen analysis.
3.2 Optimising the sensing strategy
In addition to preparing photoactive materials, the design of the sensing strategy is another key aspect that determines the performance of a constructed PEC sensor. At present, in the construction of various PEC biosensors, sensing strategies can be divided into three categories: (i) interface regulation, (ii) signal amplification strategy and (iii) signal output mode.
3.2.1 Interface regulation
The interface interaction in the PEC analysis was directly correlated with the performance of the PEC system. Precisely regulating the interface holds significant importance in achieving accurate detection, mainly through the control of the sensor recognition interface and the photoelectric conversion interface of the sensor (Kuang et al., 2017). For the recognition interface of a sensor, selecting a suitable immobilisation technique is crucial in the preparation of a sensor (Naresh and Lee, 2021). Bio-recognition elements in conventional PEC biosensors are mainly fixed to the electrode surface by attaching reagents. However, this approach may increase non-specific adsorption, leading to reduced sensitivity and limiting the ability to detect targets (Li et al., 2018). This adverse effect can be avoided by modifying the components (e.g., adding groups) or enhancing specific adsorption (e.g., designing photoactive materials with abundant active sites). Hao et al. (2019) detected Vibrio parahaemolyticus by sensitizing Ho3+/Yb3+ TiO2 with CdSe QDs. The antibody was immobilized by a coupling reaction between the -COOH group of the CdSe QDs and the -NH2 group of the antibody of Vibrio parahaemolyticus (Figure 4A). Chemical bonds resulted in orderly and firm binding of the antibody. The detection limit of the constructed PEC immunosensor was as low as 25 CFU/mL, and the detection range was as wide as 1.0 × 102 ~ 1.0 × 108 CFU/mL. Generally, the PEC sensing interface is affected by interfacial charge recombination and interfacial reaction kinetics (Xin et al., 2022). Therefore, designing photosensitive materials with different component interfaces in close contact and abundant active sites may be an effective method to accelerate charge transfer. Given the homogeneously distributed pores, good biocompatibility and rich functionality, covalent–organic frameworks (COFs) are widely utilized as platforms for fabricating biosensors to sensitively analyze various analytes. Huo et al. (2023) established a novel Cu2O-constrained photoactive COF network as a sensitive and selective platform for the PEC detection of E. coli (Figure 4B). The COF synthesized using 1,3,5-tris(4-aminophenyl)-benzene (TAPB) and 1,3,5-triformylphloroglucinol (Tp) as building blocks acted as the scaffold for encapsulating Cu2O nanoparticles (denoted as Cu2O@TAPB-Tp-COF). Given the high photoelectric conversion efficiency, large porous structure, rich functionality and encapsulation ability towards metal nanoparticles of Cu2O@TAPB-Tp-COF, the manufactured PEC aptasensor exhibited an ultralow detection limit of 2.5 CFU/mL within a range of 1.0 × 101 to 1.0 × 104 CFU/mL toward E. coli. In PEC sensing, the recognition and photoelectric conversion interfaces play a crucial role in charge recombination and transfer dynamics. Therefore, it is necessary to explore electrode interfaces with more active sites and more effective biological stationary technology.
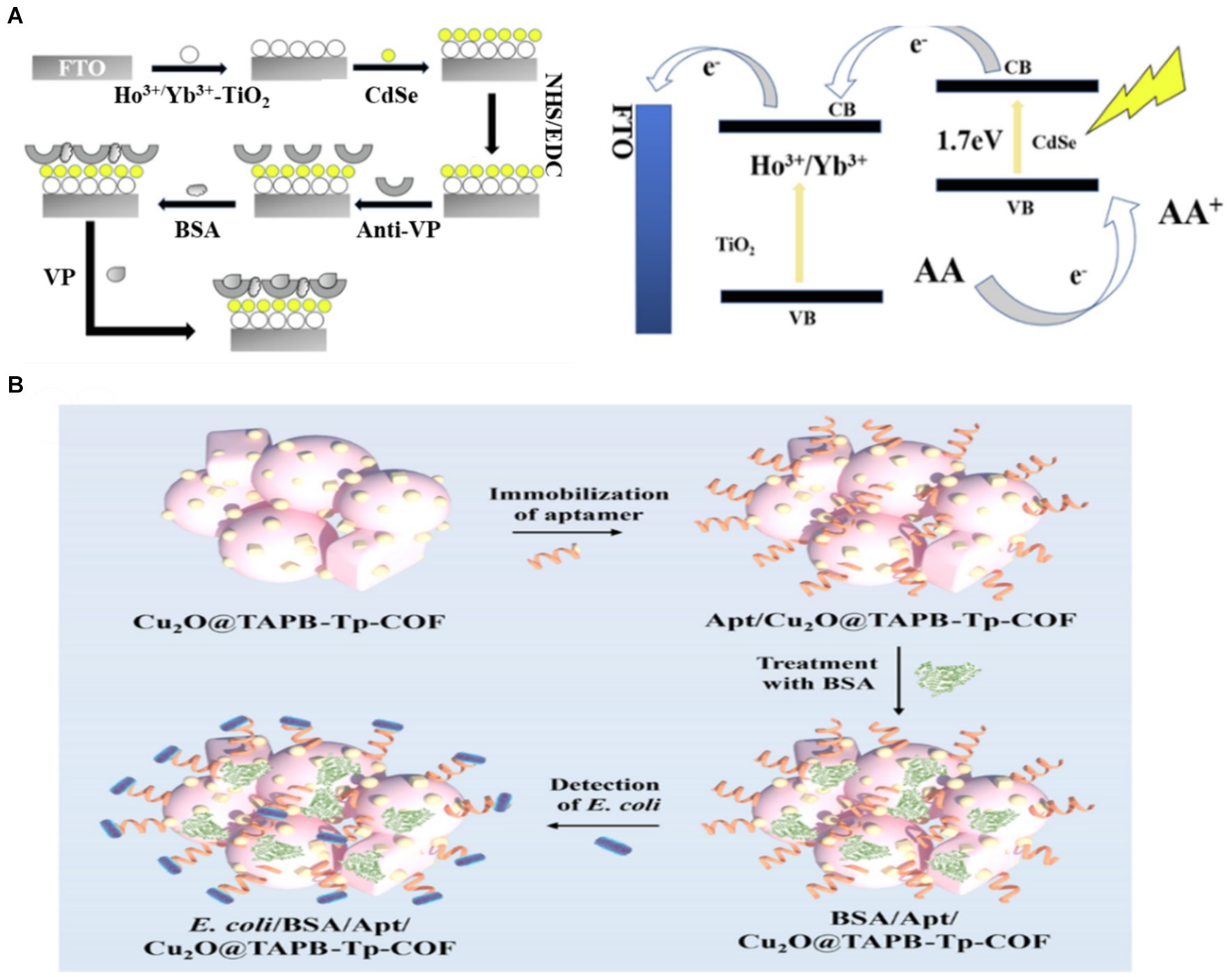
Figure 4. (A) Schematic illustration of the proposed PEC biosensor fabrication procedure and electron-transfer mechanism of CdSe QDs–sensitised Ho3+/Yb3+-TiO2 PEC sensor in AA electrolyte (Hao et al., 2019). (B) fabrication of the Cu2O@TAPB-Tp-COF-based PEC aptasensor for the detection of E. coli (Huo et al., 2023). 1,3,5-tris(4-aminophenyl)-benzene (TAPB), 1,3,5-triformylphloroglucinol (Tp), covalent–organic framework (COF).
3.2.2 Signal amplification
For PEC detection, quantitative detection is usually achieved by photoelectron transfer at the electrode/electrolyte interface and photocurrent response after interface changes (Shu and Tang, 2019). However, due to the influence of substrate in food, it is challenging to achieve sensitive detection of trace objects. Signal amplification strategies are essential in developing a highly sensitive PEC immunoassay system. Moreover, gaining a profound understanding of PEC signal amplification strategies will significantly enhance the development of advanced sensors. Standard signal amplification strategies include enzyme-mediated catalytic precipitation (Song et al., 2023) and DNA amplification (Yuan et al., 2020).
Enzyme-mediated catalytic precipitation is a simple and effective method of producing insoluble products on the electrode surface, resulting in changes in the photocurrent signal (Zhuang et al., 2015). However, natural enzymes have some problems, such as poor stability, high cost and harsh storage conditions. To overcome these shortcomings, nanozyme, a nanomaterial with enzyme-like properties, has emerged as the most promising alternative due to its good stability and low cost (Chi et al., 2024; Wang et al., 2020). Luo et al. (2022) used a single layer of Cu-C3N4 nano-enzyme as the signal amplifier for the highly selective and ultra-sensitive detection of S. aureus through the steric hindrance effect. The Cu–C3N4@Apt nanozyme acted as a peroxidase to catalyze the oxidation of 4-chloro-1-naphthol (4-CN) to produce an insoluble precipitate on the electrode surface and resulted in a significant decrease in photocurrent. Based on the signal amplification of Cu-C3N4@Apt nanozyme, a PEC sensor was successfully constructed to detect S. aureus (Figures 5A–C). The sensor had a linear range of 1.0 × 101 ~ 1.0 × 108 CFU/mL with LOD as low as 3.4 CFU/mL. Additionally, the ability of the sensor to detect S. aureus that had been added to orange juice and milk was also verified, and the recovery rate was 91% ~ 113%. Enzyme-mediated catalytic precipitation has been employed as an effective and simple signal amplification strategy owing to its inhibition effect on interfacial mass and electron transfer. However, most enzyme-mediated catalytic precipitation reactions belong to the signal-off mode, resulting in limited signal variation.
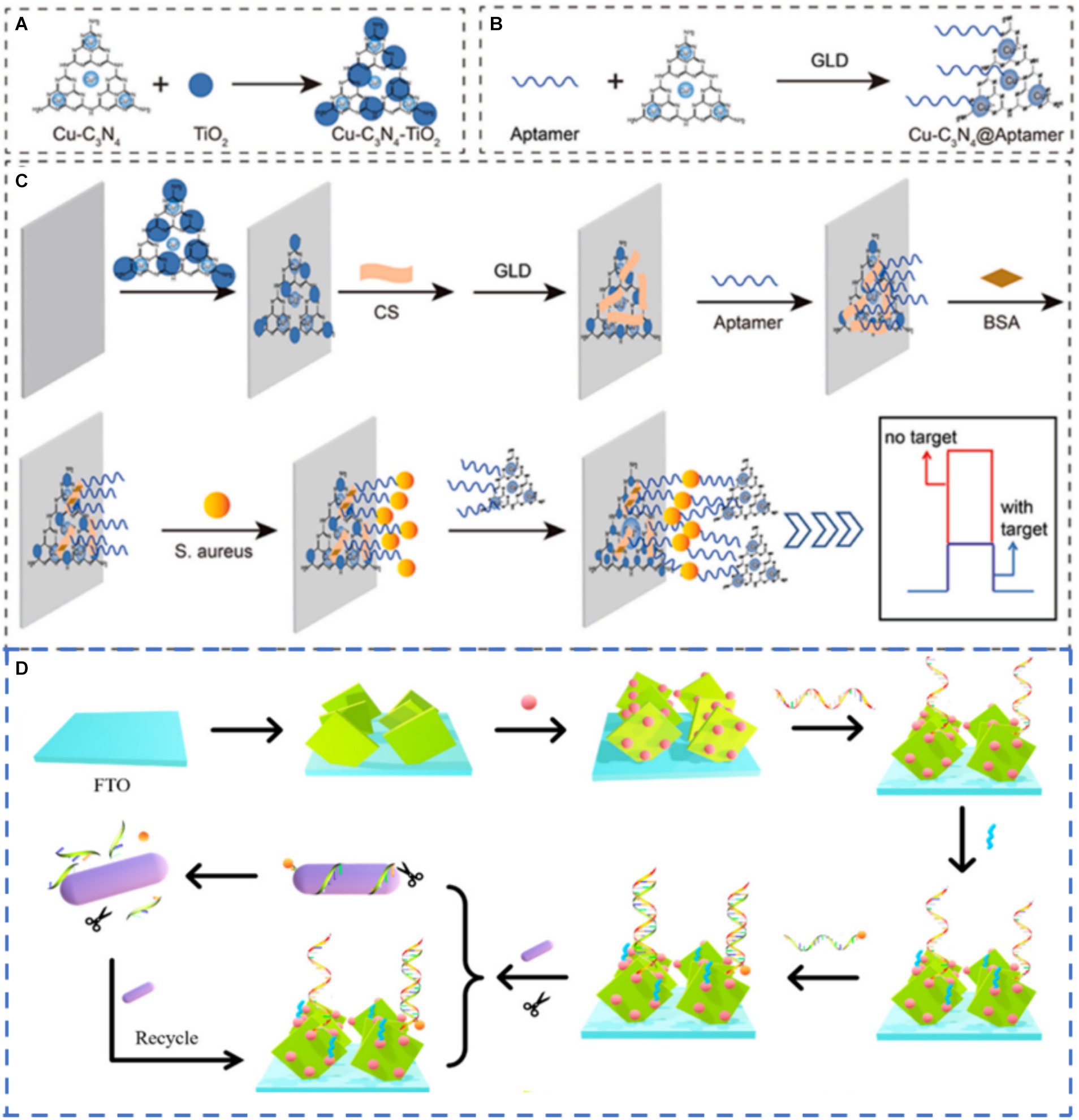
Figure 5. Schematic diagram showing (A) synthesis of Cu–C3N4–TiO2. (B) preparation of Cu–C3N4@Apt. (C) construction of PEC sensor and detection of S. aureus (Luo et al., 2022) and (D) working mechanism of the sensors (Zhu L. et al., 2021). Glutaraldehyde (GLD), F-doped Tin Oxide (FTO).
To detect trace or even trace objects, a DNA amplification strategy was introduced into the construction process of the PEC biosensor. Currently, the DNA amplification strategy applied in the PEC biosensor mainly includes hybridization chain reaction (HCR) (Zong et al., 2024) and rolling circle amplification (RCA) with nuclease-assisted recycling amplification (Zhang K. et al., 2018). Generally, these strategies amplify photocurrent signals by expanding DNA fragments, thus enhancing the number of sensitisers and mimetic enzymes. Zhu et al. (2021) constructed a PEC aptasensor using exonuclease I–assisted amplification and the initial identification of aptamers. As shown in Figure 5D, WO3 was used as the photoactive material. In contrast, the sensitization effect of CdTe QDs and the shear impact of exonuclease I were used to amplify the signal, achieving highly sensitive detection. The LOD was 45 CFU/mL in the concentration range of 1.3 × 101 ~ 1.3 × 107 CFU/mL. This construction strategy provides a novel approach for the identification of Listeria monocytogenes. The DNA amplification strategy can effectively improve the sensitivity of the PEC biosensor, but several deficiencies still hinder its further development, such as its complicated operation and high cost.
3.2.3 Dual-mode detection platform
The PEC method has unique advantages and characteristics (Zhao et al., 2015). However, with the increasing demand for sensitivity and accuracy in detecting foodborne pathogens, single-mode biosensors are susceptible to various factors, such as different manual operation, unstable experimental environments and differences in substrate morphology and loads from batch to batch. Therefore, a new output signal is introduced, and dual-mode biosensors are developed. This can simultaneously introduce dual-channel detection into the recognition system, allowing multiple response signals to be output under the same or different test conditions. In this case, each signal was independent and free from interference. Therefore, the experimental results can be mutually verified, further improving the accuracy. In the case of a difference in the sensitivity of the two detection modes, the detection range of the analytes can be broadened, thus realizing the primary screening and sensitive detection of the samples (Zhang J. et al., 2021). Zhang’s team built a CL and PEC dual-mode biosensor for S. aureus assay based on Porphyrin-Based Porous Organic Polymer (FePor-TPA) (Zheng et al., 2023). As shown in Figure 6A, on the one hand, the 2D FePor-TPA thin film shows a sensitive photocurrent response and outstanding catalase catalytic activity, which can decompose H2O2 to O2. The produced O2 as an electron donor further improved the photoelectric signal of the 2D FePor-TPA thin film. On the other hand, under acidic conditions caused by gluconic acid, FePor-TPA showed excellent peroxidase activity, which can oxidize TMB (Tetramethylbenzidine) to a chromogenic product. Then, a CL-detecting platform was realized. During the detection process, more targets generated more H2O2 and gluconic acid. As a result, a ‘signal-on’ and dual-mode detection platform with high sensitivity and selectivity was constructed for detecting S. aureus. Liu et al. (2023) combined PEC and SERS to construct a dual-mode biosensor. A photoactive heterostructure was formed by combining C3N4 and MXene via simple electrostatic self-assembly, as they possess well-matched band-edge energy levels. Subsequently, in situ growth of gold nanoparticles on the formed surface resulted in better PEC performance and SERS activity because of the synergistic effects of surface plasmon resonance and the Schottky barrier (as shown in Figure 6B). Experimental results revealed that the effective combination of PEC and SERS was achieved for amplification detection of S. aureus with a detection range of 5.0 ~ 1.0 × 108 CFU/mL (PEC) and 1.0 × 101 ~ 1.0 × 108 CFU/mL (SERS) and a detection limit of 0.70 CFU/mL (PEC) and 1.35 CFU/mL (SERS), respectively (Figure 6C). Dual-mode biosensors were beneficial to compensate for the shortcomings of single-mode biosensors, such as large assay consumption, less information acquisition and poor results accuracy. The construction of more types of dual-mode biosensors by combining PEC with other analytical methods (FL, EC, etc.) has received increasing attention.
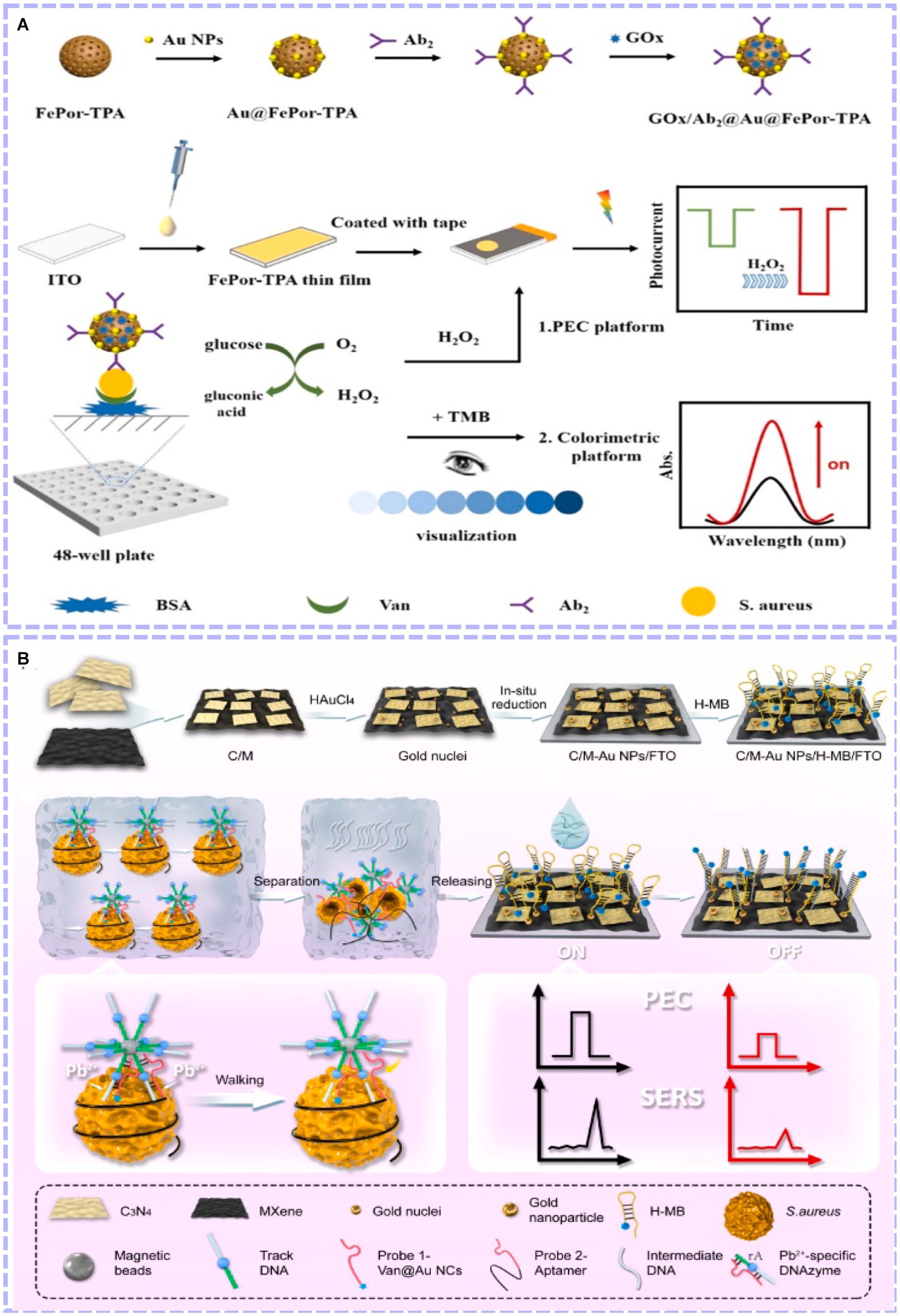
Figure 6. (A) Principle of the constructed dual-mode immunosensor for S. aureus detection and performance of the biosensor (Zheng et al., 2023). (B) Schematic illustration of the dual-mode sensing platform and performance of the biosensor (Liu et al., 2023). Porphyrin-based porous organic polymer (FePor-TPA), vancomycin (Van), antibody (Ab).
4 Conclusion and perspective
Over the past few decades, methods for detecting foodborne pathogens have developed rapidly. With the continuous exploration of photoactive materials and sensing strategies, PEC biosensors with strong specificity and high sensitivity have gradually attracted increasing attention. This review summarizes the progress of the PEC biosensor in detecting foodborne pathogens in recent years. The main focus is on the design of photoactive materials (improving the photoelectric properties of materials and expanding the spectral absorption range of materials) and the construction of sensing strategies (realizing the effective fixation of target objects and signal amplification). The PEC biosensor has reached very low detection limits for some of the major foodborne pathogens in food, such as S. aureus and E. coli. Moreover, the capability and accuracy of actual sample analysis and its application in detecting foodborne pathogens have been demonstrated. Compared to the results of traditional techniques, the PEC biosensor exhibits good accuracy, confirming the effectiveness of this method for real sample detection. Importantly, this method will provide an ideal alternative for the rapid, ultra-sensitive and selective detection of foodborne pathogens in food.
Compared with traditional approaches to electrochemical and spectral analysis, PEC sensing technology is still in the initial stage of development due to its short history. The following strategies may provide new possibilities for the detection of foodborne pathogens.
4.1 Dual-photoelectrode self-powered PEC biosensors
Traditional PEC sensors are mostly single-photoelectrodes, which have certain limitations. For example, the photoanode may react with reducing substances in the sample, and the photocathode photoelectric response is poor. To solve these problems, developing dual-photoelectrode self-powered sensors has become an attractive strategy that eliminates the effect of reducing substances and enables sensitive detection.
4.2 High-throughput detection
It is common to perform PEC sensing on a single interface for single analyte detection, which limits detection efficiency and is not suitable for rapidly screening mass samples. Selective and sensitive quantification of multiple analytes in a system can significantly reduce the cost of detection and improve the efficiency of analysis. It is highly desired in practical application. Designing sensing arrays coupled with the light-addressing strategy is an effective method. For this strategy, the concentration of different targets can be easily detected in turns by merely moving the light source from one sensing area to another. At the same time, a higher throughput can be conveniently obtained by merely enlarging the size of the substrate electrode and increasing the number of separated sensing zones.
4.3 Portable commercialization
The ultimate goal of all developed PEC bio-sensing devices is to achieve commercialization. However, most current efforts are being conducted in laboratory settings, with limited exploration in real-world settings. Portable PEC analyzers have great potential for environmental monitoring and have attracted widespread attention. Developing split-type PEC biosensors and integrating PEC bio-sensing with arrays, microfluidics and chips would contribute to high-throughput and automation. Additionally, PEC sensors are used in combination with mobile phones to enable intelligent detection. In summary, with the rapid development of nanotechnology and biotechnology in recent years, PEC analysis will play a more critical role in the field of food safety in the future.
Author contributions
XD: Conceptualization, Funding acquisition, Investigation, Project administration, Supervision, Writing – original draft, Writing – review & editing. AH: Writing – original draft, Writing – review & editing, Conceptualization, Investigation. LH: Writing – review & editing. CC: Writing – review & editing. TY: Funding acquisition, Supervision, Writing – original draft, Writing – review & editing.
Funding
The author(s) declare that financial support was received for the research, authorship, and/or publication of this article. This work was supported by the National Natural Science Foundation of China (No. 22074055 and 62201230), Natural Science Foundation of Jiangsu Province (No. BK20220546), the Postdoctoral Science Foundation of China (No. 2021 M691314), the Fund of Guangdong Provincial Key Laboratory of Food Quality and Safety, China (No. 2021KF001) and the Priority Academic Program Development of Jiangsu Higher Education Institutions (No. PAPD-2023-87). Dong also thanks the support from the Innovation/Entrepreneurship Program of Jiangsu Province (No. JSSCBS20210935).
Conflict of interest
The authors declare that the research was conducted in the absence of any commercial or financial relationships that could be construed as a potential conflict of interest.
Publisher’s note
All claims expressed in this article are solely those of the authors and do not necessarily represent those of their affiliated organizations, or those of the publisher, the editors and the reviewers. Any product that may be evaluated in this article, or claim that may be made by its manufacturer, is not guaranteed or endorsed by the publisher.
References
Acunzo, A., Scardapane, E., De Luca, M., Marra, D., Velotta, R., and Minopoli, A. (2022). Plasmonic nanomaterials for colorimetric biosensing: a review. Chemosensors 10:136. doi: 10.3390/chemosensors10040136
Argudín, M., Mendoza, M., González-Hevia, M., Bances, M., Guerra, B., and Rodicio, M. (2012). Genotypes, exotoxin gene content, and antimicrobial resistance of Staphylococcus aureus strains recovered from foods and food handlers. Appl. Environ. Microbiol. 78, 2930–2935. doi: 10.1128/AEM.07487-11
Arumugam, G. M., Karunakaran, S. K., Galian, R. E., and Pérez-Prieto, J. (2022). Recent Progress in lanthanide-doped inorganic perovskite nanocrystals and Nanoheterostructures: a future vision of bioimaging. Nano 12:2130. doi: 10.3390/nano12132130
Bettazzi, F., and Palchetti, I. (2018). Photoelectrochemical genosensors for the determination of nucleic acid cancer biomarkers. Curr. Opin. Electrochem. 12, 51–59. doi: 10.1016/j.coelec.2018.07.001
Capita, R., Felices-Mercado, A., García-Fernández, C., and Alonso-Calleja, C. (2019). Characterization of Listeria monocytogenes originating from the Spanish meat-processing chain. Food Secur. 8:542. doi: 10.3390/foods8110542
Cesewski, E., and Johnson, B. N. (2020). Electrochemical biosensors for pathogen detection. Biosens. Bioelectron. 159:112214. doi: 10.1016/j.bios.2020.112214
Chen, A., Huang, A., and Ju, P. (2024). Photoelectrochemical pathogenic bacteria sensing platform integrated with sterilization function based on the g-C3N4 nanosheets decorated with AuNPs and poly (3-thiophene boronic acid). Microchem. J. 196:109680. doi: 10.1016/j.microc.2023.109680
Chen, J., Park, B., Huang, Y. W., Zhao, Y., and Kwon, Y. (2017). Label-free SERS detection of Salmonella Typhimurium on DNA aptamer modified ag NR substrates. J. Food Meas. Charact. 11, 1773–1779. doi: 10.1007/s11694-017-9558-6
Chen, Y., Yin, H., Li, F., Zhou, J., Wang, L., Wang, J., et al. (2020). Polydopamine-sensitized WS2/black-TiO2 heterojunction for histone acetyltransferase detection with enhanced visible-light-driven photoelectrochemical activity. Chem. Eng. J. 393:124707. doi: 10.1016/j.cej.2020.124707
Chi, J., Ju, P., Bi, F., Wang, S., Jiang, T., Wen, S., et al. (2024). MXene@ MnIn2S4-gated organic Photoelectrochemical transistors with Nanozyme-mediated multiple quenching effects for ultrasensitive detection of Okadaic acid, advanced functional materials. Early View. 2407201. doi: 10.1002/adfm.202407201
Ding, L., Wei, J., Qiu, Y., Wang, Y., Wen, Z., Qian, J., et al. (2021). One-step hydrothermal synthesis of telluride molybdenum/reduced graphene oxide with Schottky barrier for fabricating label-free photoelectrochemical profenofos aptasensor. Chem. Eng. J. 407:127213. doi: 10.1016/j.cej.2020.127213
Dini, D., Calvete, M. J. F., and Hanack, M. (2016). Nonlinear optical materials for the smart filtering of optical radiation. Chem. Rev. 116, 13043–13233. doi: 10.1021/acs.chemrev.6b00033
Dong, X., Liu, D., Meng, X., and You, T. (2022). Research progress on photoelectrochemical sensors for contamination analysis in agricultural fields. Anal. Sci. 38, 459–481. doi: 10.2116/analsci.21SAR09
Dong, G., Wang, H., Yan, Z., Zhang, J., Ji, X., Lin, M., et al. (2020). Cadmium sulfide nanoparticles-assisted intimate coupling of microbial and photoelectrochemical processes: mechanisms and environmental applications. Sci. Total Environ. 740:140080. doi: 10.1016/j.scitotenv.2020.140080
Feng, X., Lin, L., Duan, R., Qiu, J., and Zhou, S. (2022). Transition metal ion activated near-infrared luminescent materials. Prog. Mater. Sci. 129:100973. doi: 10.1016/j.pmatsci.2022.100973
Fu, Y., Zou, K., Liu, M., Zhang, X., Du, C., and Chen, J. (2020). Highly selective and sensitive Photoelectrochemical sensing platform for VEGF165 assay based on the switching of photocurrent polarity of CdS QDs by porous Cu2O-CuO flower. Anal. Chem. 92, 1189–1196. doi: 10.1021/acs.analchem.9b04319
Ge, R., Lin, X., Dai, H., Wei, J., Jiao, T., Chen, Q., et al. (2022). Photoelectrochemical sensors with near-infrared-responsive reduced graphene oxide and MoS2 for quantification of Escherichia Coli O157:H7. ACS Appl. Mater. Interfaces 14, 41649–41658. doi: 10.1021/acsami.2c13292
Ge, L., Liu, Q., Hao, N., and Kun, W. (2019). Recent developments of photoelectrochemical biosensors for food analysis. J. Mater. Chem. B 7, 7283–7300. doi: 10.1039/C9TB01644A
Ge, R., Zhang, S.-M., Dai, H.-J., Wei, J., Jiao, T.-H., Chen, Q.-M., et al. (2023). G-Quadruplex/hemin-mediated polarity-switchable and photocurrent-amplified system for Escherichia coli O157:H7 detection. J. Agric. Food Chem. 71, 16807–16814. doi: 10.1021/acs.jafc.3c06052
Guldimann, C., and Johler, S. (2018). An introduction to current trends in foodborne pathogens and diseases. Curr. Clin. Microbiol. Rep. 5, 83–87. doi: 10.1007/s40588-018-0093-y
Haddour, N., Cosnier, S., and Gondran, C. (2004). Electrogeneration of a biotinylated poly(pyrrole–ruthenium(ii)) film for the construction of photoelectrochemical immunosensor. Chem. Commun. 21, 2472–2473. doi: 10.1039/B410727F
Han, H., Wei, X., Wei, Y., Zhang, X., Li, X., Jiang, J., et al. (2017). Isolation, characterization, and bioinformatic analyses of lytic Salmonella Enteritidis phages and tests of their antibacterial activity in food. Curr. Microbiol. 74, 175–183. doi: 10.1007/s00284-016-1169-7
Hao, H., Hao, S., Hou, H., Zhang, G., Hou, Y., Zhang, Z., et al. (2019). A novel label-free photoelectrochemical immunosensor based on CdSe quantum dots sensitized Ho3+/Yb3+-TiO2 for the detection of Vibrio parahaemolyticus. Methods 168, 94–101. doi: 10.1016/j.ymeth.2019.06.005
Hao, N., and Wang, K. (2016). Recent development of electrochemiluminescence sensors for food analysis. Anal. Bioanal. Chem. 408, 7035–7048. doi: 10.1007/s00216-016-9548-2
Hou, Y., Zhu, L., Hao, H., Zhang, Z., Ding, C., Zhang, G., et al. (2021). A novel photoelectrochemical aptamer sensor based on rare-earth doped Bi2WO6 and Ag2S for the rapid detection of Vibrio parahaemolyticus. Microchem. J. 165:106132. doi: 10.1016/j.microc.2021.106132
Hsieh, P.-Y., Wu, J.-Y., Chang, T.-F. M., Chen, C.-Y., Sone, M., and Hsu, Y.-J. (2020). Near infrared-driven photoelectrochemical water splitting: review and future prospects. Arab. J. Chem. 13, 8372–8387. doi: 10.1016/j.arabjc.2020.05.025
Huo, E., Shahab, S., Dang, H., Jia, Q., and Wang, M. (2023). Triazine-based covalent–organic framework embedded with cuprous oxide as the bioplatform for photoelectrochemical aptasensing Escherichia coli. Microchim. Acta 190:407. doi: 10.1007/s00604-023-05987-6
Jiang, S., Wang, Y., Cheng, H., Zhang, G., Hou, H., Bi, G., et al. (2024). Multifunctional photoelectrochemical platform based on layer-by-layer assembly of au/Bi2MoO6/V2CTx for simultaneous detection and inactivation of Salmonella enteritidis. J. Photochem. Photobiol. A Chem. 451:115535. doi: 10.1016/j.jphotochem.2024.115535
Jiang, J., Xia, J., Zang, Y., and Diao, G. (2021). Electrochemistry/Photoelectrochemistry-based Immunosensing and Aptasensing of carcinoembryonic antigen. Sensors 21:7742. doi: 10.3390/s21227742
Kuang, Y., Yamada, T., and Domen, K. (2017). Surface and Interface engineering for Photoelectrochemical water oxidation. Joule 1, 290–305. doi: 10.1016/j.joule.2017.08.004
Kümmel, J., Stessl, B., Gonano, M., Walcher, G., Bereuter, O., Fricker, M., et al. (2016). Staphylococcus aureus entrance into the dairy chain: tracking S. aureus from dairy cow to cheese. Front. Microbiol. 7:1603. doi: 10.3389/fmicb.2016.01603
Leng, W., Wu, X., Xiong, Z., Shi, T., Sun, Q., Yuan, L., Gao, R., et al. (2022). Study on antibacterial properties of mucus extract of snakehead (Channa argus) against Escherichia coli and its application in chilled fish fillets preservation. Lwt-Food Sci. Technol. 167:113840. doi: 10.1016/j.lwt.2022.113840
Li LingZhi, L. L., Meng HongMei, M. H., Dan, G. D. G., Li Yang, L. Y., and MengDie, J. M. J. (2019). Molecular mechanisms of Vibrio parahaemolyticus pathogenesis. Microbiol. Res. 222, 43–51. doi: 10.1016/j.micres.2019.03.003
Li, J., Li, C., Shi, C., Aliakbarlu, J., Cui, H., and Lin, L. (2022). Antibacterial mechanisms of clove essential oil against Staphylococcus aureus and its application in pork. Int. J. Food Microbiol. 380:109864. doi: 10.1016/j.ijfoodmicro.2022.109864
Li, M., Xiong, C., Zheng, Y., Liang, W., Yuan, R., and Chai, Y. (2018). Ultrasensitive Photoelectrochemical biosensor based on DNA tetrahedron as Nanocarrier for efficient immobilization of CdTe QDs-methylene blue as signal probe with near-zero background noise. Anal. Chem. 90, 8211–8216. doi: 10.1021/acs.analchem.8b01641
Li, X., Yuan, Y., Pan, X., Zhang, L., and Gong, J. (2019). Boosted photoelectrochemical immunosensing of metronidazole in tablet using coral-like g-C3N4 nanoarchitectures. Biosens. Bioelectron. 123, 7–13. doi: 10.1016/j.bios.2018.09.084
Li, F., Zhu, G., Jiang, J., Yang, L., Deng, F., and Arramel, X. (2024). A review of updated S-scheme heterojunction photocatalysts. J. Mater. Sci. Technol. 177, 142–180. doi: 10.1016/j.jmst.2023.08.038
Lima, C. M., Souza, I. E. G. L., dos Santos Alves, T., Leite, C. C., Evangelista-Barreto, N. S., and de Castro Almeida, R. C. (2017). Antimicrobial resistance in diarrheagenic Escherichia coli from ready-to-eat foods. J. Food Sci. Technol. 54, 3612–3619. doi: 10.1007/s13197-017-2820-4
Liu, R., Ali, S., Haruna, S. A., Ouyang, Q., Li, H., and Chen, Q. (2022). Development of a fluorescence sensing platform for specific and sensitive detection of pathogenic bacteria in food samples. Food Control 131:108419. doi: 10.1016/j.foodcont.2021.108419
Liu, R., Ali, S., Haruna, D., Zhang, Y., Lu, P., and Chen, Q. (2023). A Sensitive Nucleic Acid Detection Platform for Foodborne Pathogens Based on CRISPR-Cas13a System Combined with Polymerase Chain Reaction. Food Anal. Methods 16, 356–366. doi: 10.1007/s12161-022-02419-8
Liu, R., Zhang, Y., Ali, S., Haruna, S. A., He, P., Li, H., et al. (2021). Development of a fluorescence aptasensor for rapid and sensitive detection of Listeria monocytogenes in food. Food Control 122:107808. doi: 10.1016/j.foodcont.2020.107808
Liu, F., Zhao, J., Liu, X., Zhen, X., Feng, Q., Gu, Y., et al. (2023). PEC-SERS dual-mode detection of foodborne pathogens based on binding-induced DNA Walker and C3N4/MXene-au NPs accelerator. Anal. Chem. 95, 14297–14307. doi: 10.1021/acs.analchem.3c02529
Long, D., Tu, Y., Chai, Y., and Yuan, R. (2021). Photoelectrochemical assay based on SnO2/BiOBr p–n heterojunction for ultrasensitive DNA detection. Anal. Chem. 93, 12995–13000. doi: 10.1021/acs.analchem.1c02745
Low, J., Yu, J., Jaroniec, M., Wageh, S., and Al-Ghamdi, A. A. (2017). Heterojunction Photocatalysts. Adv. Mater. 29:1601694. doi: 10.1002/adma.201601694
Luo, S., Liu, F., Gu, S., Chen, K., Yang, G., Gu, Y., et al. (2022). Nanozyme-mediated signal amplification for ultrasensitive photoelectrochemical sensing of Staphylococcus aureus based on cu-C3N4-TiO2 heterostructure. Biosens. Bioelectron. 216:114593. doi: 10.1016/j.bios.2022.114593
Lv, J., Xie, J., Mohamed, A. G. A., Zhang, X., and Wang, Y. (2022). Photoelectrochemical energy storage materials: design principles and functional devices towards direct solar to electrochemical energy storage. Chem. Soc. Rev. 51, 1511–1528. doi: 10.1039/D1CS00859E
Lv, S., Zhang, K., Zhu, L., and Tang, D. (2020). ZIF-8-assisted NaYF4:Yb,tm@ZnO converter with exonuclease III-powered DNA Walker for near-infrared light responsive biosensor. Anal. Chem. 92, 1470–1476. doi: 10.1021/acs.analchem.9b04710
Ma, L., Chen, K., Dang, H., and Wang, M. (2023). Establishment of a photoactive heterojunction of triazine covalent-organic polymer and copper (II) selenide: Photoelectrochemical aptasensing strategy for efficiently detecting Salmonella typhimurium. Microchem. J. 193:109162. doi: 10.1016/j.microc.2023.109162
Miles, D. W., Ross, T., Olley, J., and McMeekin, T. A. (1997). Development and evaluation of a predictive model for the effect of temperature and water activity on the growth rate of Vibrio parahaemolyticus. Int. J. Food Microbiol. 38, 133–142. doi: 10.1016/S0168-1605(97)00100-1
Mitsubayashi, K., Toma, K., Iitani, K., and Arakawa, T. (2022). Gas-phase biosensors: a review. Sensors Actuators B Chem. 367:132053. doi: 10.1016/j.snb.2022.132053
Munekata, P. E., Pateiro, M., Rodríguez-Lázaro, D., Domínguez, R., Zhong, J., and Lorenzo, J. M. (2020). The role of essential oils against pathogenic Escherichia coli in food products. Microorganisms 8:924. doi: 10.3390/microorganisms8060924
Naresh, V., and Lee, N. (2021). A review on biosensors and recent development of nanostructured materials-enabled biosensors. Sensors 21:1109. doi: 10.3390/s21041109
Palomino-Camargo, C., Gonzalez-Munoz, Y., Perez-Sira, E., and Aguilar, V. H. (2018). Delphi methodology in food safety management and foodborne disease prevention. Rev. Peru Med. Exp. Salud Publica 35, 483–490. doi: 10.17843/rpmesp.2018.353.3086
Paniel, N., and Noguer, T. (2019). Detection of Salmonella in food matrices, from conventional methods to recent aptamer-sensing technologies. Food Secur. 8:371. doi: 10.3390/foods8090371
Pye, H. V., Thilliez, G., Acton, L., Kolenda, R., Al-Khanaq, H., Grove, S., et al. (2023). Strain and serovar variants of Salmonella enterica exhibit diverse tolerance to food chain-related stress. Food Microbiol. 112:104237. doi: 10.1016/j.fm.2023.104237
Raut, V. S., Lokhande, C. D., and Killedar, V. V. (2017). Photoelectrochemical studies on electrodeposited indium doped CdSe thin films using aqueous bath. J. Electroanal. Chem. 788, 137–143. doi: 10.1016/j.jelechem.2017.02.010
Sakamoto, D., Shiratani, M., and Seo, H. (2022). Near-infrared light harvesting of upconverting Y2O3:Er3+ nanoparticles and their photovoltaic application. Electrochim. Acta 436:141407. doi: 10.1016/j.electacta.2022.141407
Scallan, E., Hoekstra, R. M., Angulo, F. J., Tauxe, R. V., Widdowson, M. A., Roy, S. L., et al. (2011). Foodborne illness acquired in the United States—major pathogens. Emerg. Infect. Dis. 17, 7–15. doi: 10.3201/eid1701.P11101
Scharff, R. L., Besser, J., Sharp, D. J., Jones, T. F., Peter, G. S., and Hedberg, C. W. (2016). An economic evaluation of PulseNet: a network for foodborne disease surveillance. Am. J. Prev. Med. 50, S66–S73. doi: 10.1016/j.amepre.2015.09.018
Schirone, M., Visciano, P., Tofalo, R., and Suzzi, G. (2019). Editorial: foodborne pathogens: hygiene and safety. Front. Microbiol. 10:1974. doi: 10.3389/fmicb.2019.01974
Sheng, P., Yao, L., Yang, P., Yang, D., Lu, C., Cao, K., et al. (2020). The origin of enhanced photoelectrochemical activity in metal-ion-doped ZnO/CdS quantum dots. J. Alloys Compd. 822:153700. doi: 10.1016/j.jallcom.2020.153700
Shi, J., Chen, Z., Zhao, C., Shen, M., Li, H., Zhang, S., et al. (2022). Photoelectrochemical biosensing platforms for tumor marker detection. Coord. Chem. Rev. 469:214675. doi: 10.1016/j.ccr.2022.214675
Shi, X.-M., Mei, L.-P., Zhang, N., Zhao, W.-W., Xu, J.-J., and Chen, H.-Y. (2018). A polymer dots-based Photoelectrochemical pH sensor: simplicity, high sensitivity, and broad-range pH measurement. Anal. Chem. 90, 8300–8303. doi: 10.1021/acs.analchem.8b02291
Shu, J., Qiu, Z., Lv, S., Zhang, K., and Tang, D. (2018). Plasmonic enhancement coupling with defect-engineered TiO2–x: a mode for sensitive Photoelectrochemical biosensing. Anal. Chem. 90, 2425–2429. doi: 10.1021/acs.analchem.7b05296
Shu, J., and Tang, D. (2019). Recent advances in Photoelectrochemical sensing: from engineered photoactive materials to sensing devices and detection modes. Anal. Chem. 92, 363–377. doi: 10.1021/acs.analchem.9b04199
Sivula, K., and van de Krol, R. (2016). Semiconducting materials for photoelectrochemical energy conversion. Nat. Rev. Mater. 1:15010. doi: 10.1038/natrevmats.2015.10
Song, P., Wang, M.-L., Duan, Y.-X., Wang, A.-J., Xue, Y., Mei, L.-P., et al. (2023). Bifunctional photoelectrochemical aptasensor based on heterostructured Ag3PO4/ag/TiO2 nanorod array for determination of two tumor markers. Microchim. Acta 190:85. doi: 10.1007/s00604-023-05654-w
Sun, J., He, Y., He, S., Liu, D., Lu, K., Yao, W., et al. (2022). A self-powered photoelectrochemical cathodic molecular imprinting sensor based on au@TiO2 nanorods photoanode and Cu2O photocathode for sensitive detection of sarcosine. Biosens. Bioelectron. 204:114056. doi: 10.1016/j.bios.2022.114056
Sun, Y., Zhang, X., Wang, G., Lin, S., Zeng, X., Wang, Y., et al. (2016). PI3K-AKT signaling pathway is involved in hypoxia/thermal-induced immunosuppression of small abalone Haliotis diversicolor. Fish Shellfish Immunol. 59, 492–508. doi: 10.1016/j.fsi.2016.11.011
Tao, Q., Tang, N., Jiang, Y., Chen, B., Liu, Y., Xiong, X., et al. (2023). Double bipolar electrode electrochemiluminescence color switch for food-borne pathogens detection. Biosens. Bioelectron. 237:115452. doi: 10.1016/j.bios.2023.115452
Taylor, B. J., Quinn, A. R., and Kataoka, A. (2019). Listeria monocytogenes in low-moisture foods and ingredients. Food Control 103, 153–160. doi: 10.1016/j.foodcont.2019.04.011
Todd, E. (2020). Food-borne disease prevention and risk assessment. Int. J. Environ. Res. Public Health 17:129. doi: 10.3390/ijerph17145129
Vongkamjan, K., Benjakul, S., Vu, H. T. K., and Vuddhakul, V. (2017). Longitudinal monitoring of Listeria monocytogenes and Listeria phages in seafood processing environments in Thailand. Food Microbiol. 66, 11–19. doi: 10.1016/j.fm.2017.03.014
Wang, X., Chen, H., Zhang, J., Zhou, H., Meng, X., Wang, N., et al. (2024). Photoelectrochemical sensor for the detection of Escherichia coli O157:H7 based on TPA-NO2 and dual-functional polythiophene films. Food Chem. 441:138299. doi: 10.1016/j.foodchem.2023.138299
Wang, J., Cui, X., Liang, L., Li, J., Pang, B., and Li, J. (2024). Advances in DNA-based electrochemical biosensors for the detection of foodborne pathogenic bacteria. Talanta 275:126072. doi: 10.1016/j.talanta.2024.126072
Wang, G., Li, L., Zheng, H., Li, Q., Huang, J., Zhang, L., et al. (2023). Bifunctional strategy toward constructing perovskite/Upconversion lab-on-paper Photoelectrochemical device for sensitive detection of malathion. ACS Nano 17, 13418–13429. doi: 10.1021/acsnano.3c01692
Wang, B., Wang, H., Lu, X., Zheng, X., and Yang, Z. (2023). Recent advances in electrochemical biosensors for the detection of foodborne pathogens: current perspective and challenges. Food Secur. 12:2795. doi: 10.3390/foods12142795
Wang, G.-L., Yuan, F., Gu, T., Dong, Y., Wang, Q., and Zhao, W.-W. (2018). Enzyme-initiated Quinone-chitosan conjugation chemistry: toward a general in situ strategy for high-throughput Photoelectrochemical enzymatic bioanalysis. Anal. Chem. 90, 1492–1497. doi: 10.1021/acs.analchem.7b04625
Wang, Z., Zhang, R., Yan, X., and Fan, K. (2020). Structure and activity of nanozymes: inspirations for de novo design of nanozymes. Mater. Today 41, 81–119. doi: 10.1016/j.mattod.2020.08.020
Xiao, M., Zhu, M., Yuan, R., and Yuan, Y. (2023). Dual-sensitized heterojunction PDA/ZnO@ MoS2 QDs combined with multilocus domino-like DNA cascade reaction for ultrasensitive photoelectrochemical biosensor. Biosens. Bioelectron. 227:115151. doi: 10.1016/j.bios.2023.115151
Xin, C., Cheng, Y., Wang, J., Sun, Q., Liu, E., Hu, X., et al. (2022). Hole storage interfacial regulation of Sb2Se3 photocathode with significantly enhanced Photoelectrochemical performance. Langmuir 39, 627–637. doi: 10.1021/acs.langmuir.2c02999
Xu, L., Bai, X., and Bhunia, A. K. (2021). Current state of development of biosensors and their application in foodborne pathogen detection. J. Food Prot. 84, 1213–1227. doi: 10.4315/JFP-20-464
Xu, Y., Hassan, M. M., Sharma, A. S., Li, H., and Chen, Q. (2023). Recent advancement in nano-optical strategies for detection of pathogenic bacteria and their metabolites in food safety. Crit. Rev. Food Sci. Nutr. 63, 486–504. doi: 10.1080/10408398.2021.1950117
Yan, K., Karthick Kannan, P., Doonyapisut, D., Wu, K., Chung, C.-H., and Zhang, J. (2021). Advanced functional electroactive and photoactive materials for monitoring the environmental pollutants. Adv. Funct. Mater. 31:2008227. doi: 10.1002/adfm.202008227
Yang, H., Chen, H., Cao, L., Wang, H., Deng, W., Tan, Y., et al. (2020). An immunosensor for sensitive photoelectrochemical detection of Staphylococcus aureus using ZnS–Ag2S/polydopamine as photoelectric material and Cu2O as peroxidase mimic tag. Talanta 212:120797. doi: 10.1016/j.talanta.2020.120797
Yang, X., Gao, Y., Ji, Z., Zhu, L.-B., Yang, C., Zhao, Y., et al. (2019). Dual functional molecular imprinted polymer-modified Organometal Lead halide perovskite: synthesis and application for Photoelectrochemical sensing of salicylic acid. Anal. Chem. 91, 9356–9360. doi: 10.1021/acs.analchem.9b01739
Yang, Y., Tan, H., Cheng, B., Fan, J., Yu, J., and Ho, W. (2021). Near-infrared-responsive Photocatalysts. Small Methods 5:e2001042. doi: 10.1002/smtd.202001042
Yang, G., Wang, H., Dong, Y., Li, Z., and Wang, G. L. (2020). High-throughput photoelectrochemical determination of E. coli O157: H7 by modulation of the anodic photoelectrochemistry of CdS quantum dots via reversible deposition of MnO2. Microchim. Acta 187, 1–9. doi: 10.1007/s00604-019-3968-6
Yang, H., Zhao, X., Wang, H., Deng, W., Tan, Y., Ma, M., et al. (2019). Sensitive photoelectrochemical immunoassay of Staphylococcus aureus based on one-pot electrodeposited ZnS/CdS heterojunction nanoparticles. Analyst 145, 165–171. doi: 10.1039/c9an02020a
Yin, M., Liu, C., Ge, R., Fang, Y., Wei, J., Chen, X., et al. (2022). Paper-supported near-infrared-light-triggered photoelectrochemical platform for monitoring Escherichia coli O157:H7 based on silver nanoparticles-sensitized-upconversion nanophosphors. Biosens. Bioelectron. 203:114022. doi: 10.1016/j.bios.2022.114022
Yu, H., Guo, W., Lu, X., Xu, H., Yang, Q., Tan, J., et al. (2021). Reduced graphene oxide nanocomposite based electrochemical biosensors for monitoring foodborne pathogenic bacteria: a review. Food Control 127:108117. doi: 10.1016/j.foodcont.2021.108117
Yuan, Y., Hu, T., Zhong, X., Zhu, M., Chai, Y., and Yuan, R. (2020). Highly sensitive Photoelectrochemical biosensor based on quantum dots sensitizing Bi2Te3 Nanosheets and DNA-amplifying strategies. ACS Appl. Mater. Interfaces 12, 22624–22629. doi: 10.1021/acsami.0c04536
Zang, Y., Lei, J., and Ju, H. (2017). Principles and applications of photoelectrochemical sensing strategies based on biofunctionalized nanostructures. Biosens. Bioelectron. 96, 8–16. doi: 10.1016/j.bios.2017.04.030
Zhang, J., Gao, Y., Zhang, X., Feng, Q., Zhan, C., Song, J., et al. (2021). "Dual signal-on" Split-type Aptasensor for TNF-alpha: integrating MQDs/ZIF-8@ZnO NR arrays with MB-liposome-mediated signal amplification. Anal. Chem. 93, 7242–7249. doi: 10.1021/acs.analchem.1c00415
Zhang, Z., Liu, Q., Zhang, M., You, F., Hao, N., Ding, C., et al. (2021). Simultaneous detection of enrofloxacin and ciprofloxacin in milk using a bias potentials controlling-based photoelectrochemical aptasensor. J. Hazard. Mater. 416:125988. doi: 10.1016/j.jhazmat.2021.125988
Zhang, K., Lv, S., Lu, M., and Tang, D. (2018). Photoelectrochemical biosensing of disease marker on p-type cu-doped Zn0. 3Cd0. 7S based on RCA and exonuclease III amplification. Biosens. Bioelectron. 117, 590–596. doi: 10.1016/j.bios.2018.07.001
Zhang, G., Shan, D., Dong, H., Cosnier, S., Al-Ghanim, K. A., Ahmad, Z., et al. (2018). DNA-mediated nanoscale metal–organic frameworks for ultrasensitive Photoelectrochemical enzyme-free immunoassay. Anal. Chem. 90, 12284–12291. doi: 10.1021/acs.analchem.8b03762
Zhao, W.-W., Xiong, M., Li, X.-R., Xu, J.-J., and Chen, H.-Y. (2014). Photoelectrochemical bioanalysis: a mini review. Electrochem. Commun. 38, 40–43. doi: 10.1016/j.elecom.2013.10.035
Zhao, W.-W., Xu, J.-J., and Chen, H.-Y. (2015). Photoelectrochemical bioanalysis: the state of the art. Chem. Soc. Rev. 44, 729–741. doi: 10.1039/C4CS00228H
Zhao, W. W., Xu, J. J., and Chen, H. Y. (2016). Photoelectrochemical detection of metal ions. Analyst 141, 4262–4271. doi: 10.1039/C6AN01123C
Zhao, Y., Zhu, L., Jiang, S., Zhang, G., Hou, H., Bi, G., et al. (2024). A novel photoelectrochemical phage sensor based on WO3/Bi2S3 for Escherichia coli detection. Colloids Surf. A Physicochem. Eng. Asp. 686:133392. doi: 10.1016/j.colsurfa.2024.133392
Zheng, Z., Ma, L., Li, B., and Zhang, X. (2023). Dual-modal biosensor for Staphylococcus aureus detection based on a porphyrin-based porous organic polymer FePor-TPA with excellent peroxidase-like Catalase-like, and Photoelectrochemical Properties. Anal. Chem. 95, 13855–13863. doi: 10.1021/acs.analchem.3c01950
Zhou, Y., Shi, Y., Wang, F.-B., and Xia, X.-H. (2019). Oriented self-assembled monolayer of Zn(II)-Tetraphenylporphyrin on TiO2 electrode for Photoelectrochemical analysis. Anal. Chem. 91, 2759–2767. doi: 10.1021/acs.analchem.8b04478
Zhu, A., Ali, S., Jiao, T., Wang, Z., Ouyang, Q., and Chen, Q. (2023). Advances in surface-enhanced Raman spectroscopy technology for detection of foodborne pathogens. Compr. Rev. Food Sci. Food Saf. 22, 1466–1494. doi: 10.1111/1541-4337.13118
Zhu, L., Hao, H., Ding, C., Gan, H., Jiang, S., Zhang, G., et al. (2021). A novel Photoelectrochemical aptamer sensor based on CdTe quantum dots enhancement and exonuclease I-assisted signal amplification for Listeria monocytogenes detection. Food Secur. 10:2896. doi: 10.3390/foods10122896
Zhu, S., Tang, Y., Shi, B., Zou, W., Wang, X., Wang, C., et al. (2021). Oligonucleotide-mediated the oxidase-mimicking activity of Mn3O4 nanoparticles as a novel colorimetric aptasensor for ultrasensitive and selective detection of Staphylococcus aureus in food. Sensors Actuators B Chem. 349:130809. doi: 10.1016/j.snb.2021.130809
Zhu, P., Wang, P., Kan, L., Sun, G., Zhang, Y., and Yu, J. (2015). An enhanced photoelectrochemical immunosensing platform: supramolecular donor–acceptor arrays by assembly of porphyrin and C60. Biosens. Bioelectron. 68, 604–610. doi: 10.1016/j.bios.2015.01.050
Zhu, Y.-C., Xu, Y.-T., Xue, Y., Fan, G.-C., Zhang, P.-K., Zhao, W.-W., et al. (2019). Three-dimensional CdS@carbon Fiber networks: innovative synthesis and application as a general platform for Photoelectrochemical bioanalysis. Anal. Chem. 91, 6419–6423. doi: 10.1021/acs.analchem.9b01186
Zhuang, J., Lai, W., Xu, M., Zhou, Q., and Tang, D. (2015). Plasmonic AuNP/g-C3N4 nanohybrid-based photoelectrochemical sensing platform for ultrasensitive monitoring of polynucleotide kinase activity accompanying DNAzyme-catalyzed precipitation amplification. ACS Appl. Mater. Interfaces 7, 8330–8338. doi: 10.1021/acsami.5b01923
Zong, C., Kong, L., Li, C., Xv, H., Lv, M., Chen, X., et al. (2024). Light-harvesting iridium (III) complex-sensitized NiO photocathode for photoelectrochemical bioanalysis. Microchim. Acta 191:223. doi: 10.1007/s00604-024-06321-4
Glossary
Keywords: foodborne pathogens, photoelectrochemical, biosensors, detection, food safety
Citation: Dong X, Huang A, He L, Cai C and You T (2024) Recent advances in foodborne pathogen detection using photoelectrochemical biosensors: from photoactive material to sensing strategy. Front. Sustain. Food Syst. 8:1432555. doi: 10.3389/fsufs.2024.1432555
Edited by:
Arun K. Bhunia, Purdue University, United StatesReviewed by:
Andrew G. Gehring, Agricultural Research Service (USDA), United StatesQingli Dong, University of Shanghai for Science and Technology, China
Copyright © 2024 Dong, Huang, He, Cai and You. This is an open-access article distributed under the terms of the Creative Commons Attribution License (CC BY). The use, distribution or reproduction in other forums is permitted, provided the original author(s) and the copyright owner(s) are credited and that the original publication in this journal is cited, in accordance with accepted academic practice. No use, distribution or reproduction is permitted which does not comply with these terms.
*Correspondence: Tianyan You, eW91dHlAdWpzLmVkdS5jbg==