- 1Department of Biological and Chemical Engineering, Aarhus University, Aarhus, Denmark
- 2Department of Biological and Chemical Engineering, Aarhus University, Tjele, Denmark
Introduction: Anaerobic digestion of manure, together with other biowastes, produces biogas that can substitute fossil energy and thereby reduce CO2 emissions and post- digestion greenhouse gas emissions. The final digestate of the process is an organic fertilizer rich in plant nutrients and recalcitrant organic constituents. The digestate characteristics and quality depend on several parameters, such as input feedstocks and operational conditions of the biogas plants. In Denmark, the rapid expansion of the biogas sector in recent years has resulted in a great variety of feedstocks used in the plants. The first generation of biogas plants mainly treated manure, industrial wastes, and energy crops with short retention times, while the new generation of biogas plants are co-digesting manure with higher amounts of lignocellulosic feedstocks and operating with longer retention times. This study evaluated whether this shift in feedstock composition could impact the fertilizer quality and post-digestion greenhouse gases and ammonia emissions during storage and application of digestate.
Methods: Digestate samples from 2015 to 2023 were collected and analyzed for composition and residual methane yields. The efficiencies of solid–liquid separation applied to several digestate samples from the new generation of biogas plants were investigated and the nutrients contents of the liquid and solid fractions of digestate were evaluated.
Results and discussion: The most evident change caused by the feedstock transition was an average increase of 52% in the total solids content of digestate, which can negatively impact ammonia emissions during digestate application. In contrast, similar average residual methane yields on a fresh matter basis of approximately 5 L/kg indicated comparable risks of methane emissions during storage. The liquid fraction of industrially separated digestate presented, on average, nutrient concentrations similar to those of unseparated digestate, while the solid fraction presented similar K, lower total ammoniacal nitrogen, and higher organic N and P contents than unseparated digestate on a fresh matter basis. The average residual methane yield of the industrially separated solid fraction of digestate was 101 L/kg volatile solids, while the average calorific value was 21 MJ/kg volatile solids, indicating its potential for additional energy generation.
1 Introduction
Anaerobic digestion (AD) is a common method of treating organic matter to produce energy in the form of methane rich biogas. However, the de-gassed digestate can be considered as a valuable secondary resource that is commonly used as a fertilizer (Teglia et al., 2011). The fertilizer value of digestates is usually associated with the NPK concentrations (Teglia et al., 2011), and sometimes more specifically to the ammonium nitrogen content (Alburquerque et al., 2012b). However, high concentrations of heavy metals may restrict their use (Alburquerque et al., 2012b), and concerns associated to ammonia and greenhouse gases emissions exist.
Digestate can be utilized in its raw form or separated into solid and liquid fractions for easier transport and application and for partitioning of the nutrients (Teglia et al., 2011). Normally, the liquid fraction of digestate (LFD) is valued by its ammonium and K concentrations, while the solid fraction (SFD) is attractive due to its organic matter and P contents (Drosg et al., 2015). The characterization of other nutrients, heavy metals, and plant-derived polymers and their partitioning into SFD and LFD are seldom reported in the literature. The LFD can be used for direct fertilization or fertilization combined with irrigation (Alburquerque et al., 2012b). The SFD is often considered as a soil amendment (Kovačić et al., 2022) or it can be used for additional energy generation through, for instance, prolonged AD by SFD recirculation to the digester, combustion, hydrothermal liquefaction, or pyrolysis (Monlau et al., 2015). The produced biochar from pyrolysis is suitable for use as a soil amendment (Kovačić et al., 2022) and can be a method for carbon capture and sequestration (Wu et al., 2023).
AD of agricultural wastes such as manures results in relatively low biogas yields (Orlando and Velazquez-Marti, 2020), so the use of energy crops such as maize silage to boost gas production became popular in many countries, with maize silage constituting 70% of the fresh matter input to German biogas plants (Daniel-Gromke et al., 2018). This has led to concerns regarding competition with food production and overall sustainability of the process (Bartoli et al., 2019). As a result of these concerns, the Danish government has progressively reduced the proportion of energy crops added to manure-based biogas plants in recent years, to a maximum of 4% of the fresh mass added from August 2024 to a complete restriction of maize silage from 2025 (KEFM, 2023). To replace energy crops and increasingly expensive and limited industrial wastes, Danish biogas plants have focused on the utilization of agricultural byproducts such as cereal straws (Larsen et al., 2023), grasses, and deep litter manures (Møller et al., 2022). Due to the poorer degradability of these substrates compared to energy crops, higher loads have been applied, along with longer hydraulic retention times (HRTs). This transition in AD feedstock may lead to changes in the digestate quality, affecting fertilizing and amendment properties, and associated ammonia and greenhouse gases emissions during digestate storage and after field application.
This study examines digestates from several biogas plants in Denmark from 2015 to 2016 and from 2020 to 2023. The two periods compare the digestate compositional changes with a reduced portion of energy crops and increased portion of straws and deep litter in the latter period, when compared to the earlier period. Samples of LFD and SFD from the latter period were also examined, and the partition of digestate components into these fractions were evaluated. The work examines physical and chemical parameters including nutrients and heavy metals contents and fiber composition. Residual methane potentials were determined to assess the risks of methane emissions during storage and the potential of methane recovery by prolonged AD. Calorific values were estimated to evaluate the utilization of digestate for pyrolysis or combustion.
2 Materials and methods
2.1 Experimental set-up
Digestate samples were collected from the final digester or post-storage tank of a selection of Danish biogas plants. Samples were divided into Group 1 and Group 2. Group 1 consisted of samples collected between 2015 and 2016 and comprised samples from biogas plants treating mainly cattle and pig manure, industrial waste, energy crops, and some portions of deep litter. Group 2 contained samples collected between 2020 and 2023 from biogas plants treating cattle and pig manure, industrial waste, smaller amounts of energy crops, and increased portions of deep litter and straw. Within Group 2, ten of the collected digestate samples were separated into solid and liquid fractions in the lab on the same day as they were collected. Another 12 samples of solid and liquid digestate separated at the respective biogas plants were obtained. The main feedstock employed, the digestion temperature, and the HRTs are presented in Table 1. Digestate samples and their fractions were analyzed on the same day of collection or after storage at −18°C. They were characterized in terms of pH, conductivity, total solids (TS), volatile solids (VS), volatile fatty acids (VFA), total ammoniacal nitrogen (TAN), total Kjeldahl nitrogen (TKN), crude protein and lipids contents, fiber (cellulose, hemicellulose, and lignin) and elemental compositions (C, N, H, and S), calorific values, contents of macro-nutrients (Ca, Mg, K, and P), and micro-nutrients, and heavy metals (Na, Fe, Mn, Zn, Cu, Cr, Al, Ni, and Mo). Mass balances determined the efficiency of the separation methods. AD batch tests were employed to determine the residual methane potentials of the samples and started on the same day of sample collection, except for industrially separated SFD samples, which were previously frozen. Part of the unseparated digestate samples from Group 2 (samples A1-A10 and B1-B19) were characterized in terms of pH, TS, VS, VFA, TAN, fiber and elemental compositions, and methane yields in a previous study (Romio et al., 2023).
2.2 Solid–liquid separation
In the lab-scale separation, 3 kg of each digestate sample was separated with a 1 mm mesh sieve of 20 cm diameter, initially by manual screening, followed by applying a pressure of 5.3 kPa for 5 min. The weights of the solid and liquid fractions were recorded. The industrial separation was conducted with screw presses for eight samples and decanter centrifuges for four samples (Table 1).
2.3 Anaerobic digestion tests
The AD batch tests were conducted in triplicates to determine the residual methane yields of digestate and its fractions and lasted between 84 and 148 days. The procedures of the AD tests and methods of determination of volumes of biogas and methane have been described elsewhere (Romio et al., 2023). An amount of 300 g digestate was employed for samples A1-A10 and lab-separated LFD samples A1L-A10L, while 200 g of digestate was employed for the remaining unseparated and industrially separated LFD samples. The samples were digested at the same temperatures as the digesters/storage tanks from which they were collected (Table 1).
Lab-separated SFD samples A1S-A10S were not included in the AD tests, and their methane productions were estimated through the methane productions of the respective unseparated and LFD samples (Section 2.5). Industrially separated SFD samples were digested with an inoculum, collected from the biogas plant at Aarhus University (Viborg, Denmark) 14 days before the start of the tests, filtered through a 1 mm mesh sieve, and incubated at 51°C for the partial deployment of its residual organic matter. 200 g of inoculum was employed in the AD tests, containing a VS content of 1.30%. The mass of the SFD samples added corresponded to the mass yielding an inoculum to substrate VS ratio of 2:1. AD tests were performed at 51°C.
2.4 Analytical methods
The determination of pH, TS, VS, TAN, and VFA contents, the elemental analysis, and the fiber characterization were performed as described by Romio et al. (2023). The fiber analysis fractionated the sample into neutral detergent soluble fraction (NDS), neutral detergent fiber (NDF), acid detergent fraction (ADF), and acid detergent lignin (ADL), as proposed by Van Soest et al. (1991). The hemicellulose content was considered to be the difference between NDF and ADF, the cellulose content as the difference between ADF and ADL, and the lignin content corresponded to the ADL fraction.
The conductivity was determined with an EcoSense EC300 conductivity meter (YSI, USA). The TKN was determined following the procedure described by APHA (2017), utilizing the digestion apparatus Tecator Kjeltec 2,400 (Foss Analytics, Denmark), the distillation system Vapodest 45 s (Gerhardt Analytical Systems, Germany), and the titration unit TitroLine easy (SI Analytics, Germany). The protein content was assumed to correspond to the difference between TKN and TAN multiplied by 6.25 (Hattingh et al., 1967). The lipids content was determined as described by Jensen (2008). The contents of nutrients and heavy metals were determined using optical emission spectrometry with inductively coupled plasma (ICP-OES, 4300 Optima, Perkin-Elmer, United States).
2.5 Calculations and data analysis
2.5.1 Calorific value
The theoretical calorific value was calculated through Eqs. 1, 2 (Annamalai et al., 1987):
where the calorific value is given in kJ/kg, and xC, xH, xS, xN, xo, and xash represent the contents of C, H, S, N (from elemental analysis), O, and ash (from VS determination) in % on a TS basis, respectively.
2.5.2 Separation efficiency
The enrichment of any analyzed component x into the solid fraction by the solid–liquid separation was evaluated by its relative separation index (RSI), calculated through Eqs. 3, 4 (Hjorth et al., 2011):
where SIx is the separation index of component x, RSIx is the relative separation index of species x, mUD represents the initial mass of unseparated digestate, mSFD represents the mass of digestate recovered as SFD, and Cx,UD and Cx,SFD represent the contents of x in the unseparated digestate and SFD (on a fresh matter basis).
The RSI considers the amount of mass recovered in the SFD; therefore, it can determine if a component was selectively transferred to the solid fraction. An RSI value equal to 1 represents a complete recovery of a component in the SFD (occurring when = ), while an RSI value close to 0 means that the given component was not selectively recovered and simply followed the overall mass fractionation into SFD and LFD (occurring when = ). Negative values of the RSI indicate the removal of a component from the SFD to the LFD (occurring when < ).
In the case of lab-scale separation, mUD, and mSFD were determined experimentally. However, when considering the industrial separation, mass balances were required to estimate to determine the RSI. A general mass balance was combined with a mass balance of the TS, resulting in Eq. 5:
where CTS,UD, CTS,LFD, and CTS,SFD represent the contents of TS in the unseparated digestate, LFD, and SFD.
The TS content was chosen to be included in the mass balance due to the reliability of its determination method, allowing the use of greater amounts of sample compared to other analyses and, therefore, minimizing the impacts of sample heterogeneity. The calculations were performed for the subsets of ten samples with the unseparated digestate, SFD, and LFD that were collected on the same day (Table 1). Two of these samples underwent separation with decanter centrifuges while the remaining were separated with screw presses. Notice that the subsets of samples B9, B9L and B30S, which underwent separation with decanter centrifuges, were not included in the calculations, since samples B9S, B30, and B30L were missing.
The RSI of any component x can, however, be severely impacted by inconsistencies in mass balances caused by sampling or analysis issues. Therefore, an error threshold of ±20% was assumed, and the RSI was only calculated when mass balances errors (E) did not surpass this limit (Eq. 6).
where mLFD corresponds to the mass of digestate recovered as LFD and Cx,LFD represents the content of any component x in the LFD (on a fresh matter basis).
2.5.3 Methane production
The methane production during the batch AD tests was modeled with the first-order kinetic model (Eq. 7):
where B(t) is the methane yield at time t (on a fresh matter or VS basis), B0 is the ultimate methane yield (on a fresh matter or VS basis), and k is the apparent hydrolysis rate.
The first-order kinetic model was used for all samples except for the industrially separated SFD samples, for which the Modified-Gompertz model was employed due to a lag-phase in gas production (Eq. 8):
where μmax is the maximum methane production rate, and λ is the lag-phase duration.
The kinetic parameters of both models were determined through the least squares method using the Solver function of Microsoft Excel.
For the lab-separated SFD samples, the methane production was estimated based on Eq. 9:
where BUD, BLFD, and BSFD are the methane yields of the unseparated digestate, LFD, and SFD, respectively. In this equation, the methane yields are given on a fresh matter basis, and the conversion of BSFD to a VS basis can be obtained by multiplying BSFD by the VS content of the SFD sample.
2.5.4 Statistical analysis
Statistical analysis was performed with the software JMP Pro 16.0.0. The Tukey`s HSD test was used to determine whether sample characteristics significantly differed among groups of samples. The Pearson correlation factor (r) was used to describe the linear relationships among the averaged ultimate methane yields, calorific values, digestate characteristics, and operational conditions of origin digesters. The significance of r was determined through a t-test. Tukey`s HSD and t-tests considered a significance level of 0.05. Significant differences and correlations were represented by the probability value (p) < 0.05.
3 Results
3.1 Digestate characterization
The distributions of digestate characteristics on a fresh matter basis are displayed in Figures 1–4. Table 2 shows the averages of digestate characteristics on fresh matter and TS basis. Results for the parameters below the quantification limits were not included. For the characterization of every analyzed sample and for specific averages of samples that underwent separation and the resulting fractions, see Supplementary material.
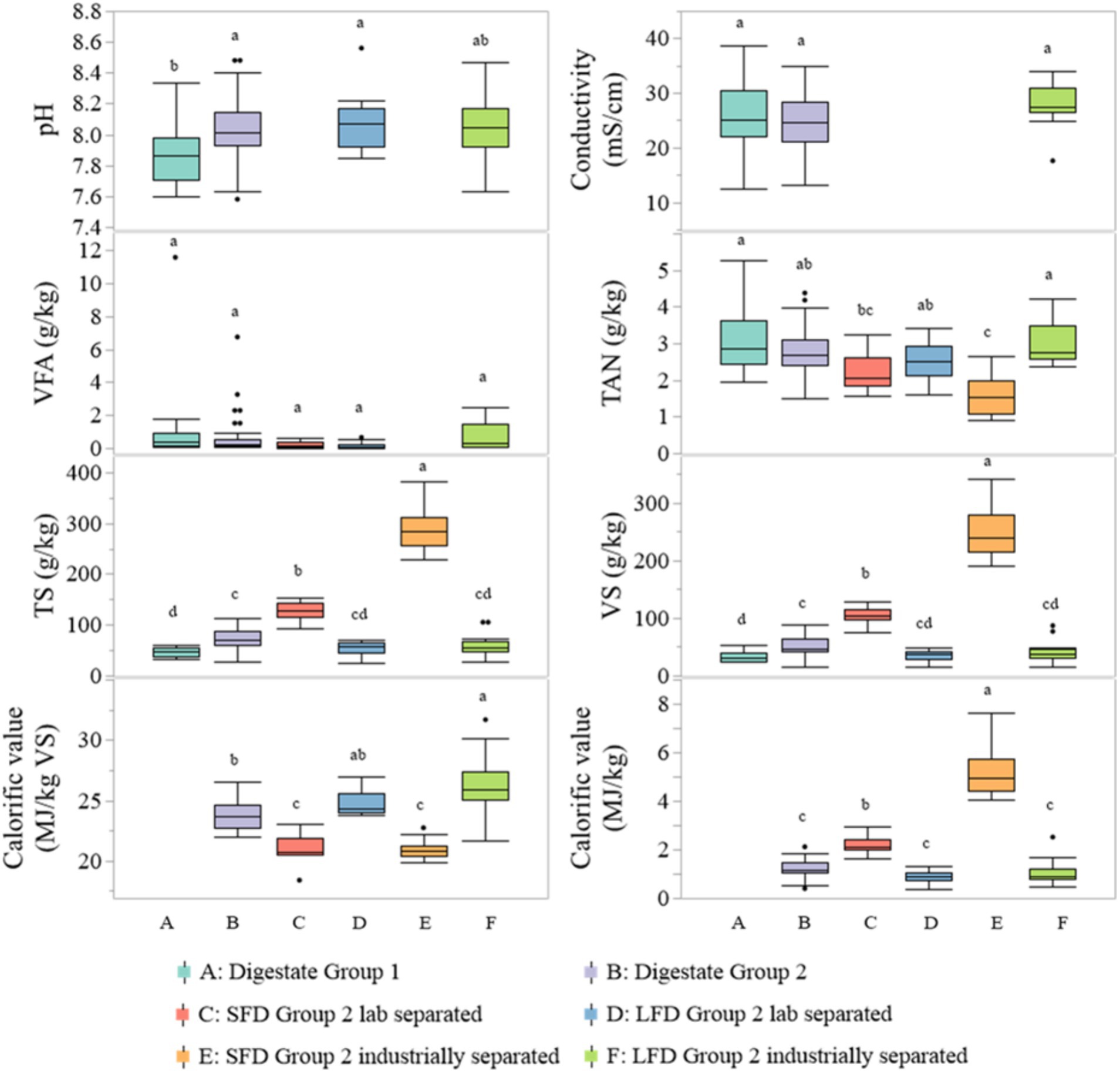
Figure 1. Digestate characterization: distribution of pH, conductivity, VFA, TAN, TS, VS, and calorific values. Letter indices above the boxplots originate from the Tukey’s HSD test, comparing means at a significance level of differences set at p < 0.05, where different letters represent significant differences among the groups of samples. VFA, volatile fatty acids; TAN, total ammoniacal nitrogen; TS, total solids; VS, volatile solids; SFD, solid fraction of digestate; LFD, liquid fraction of digestate.
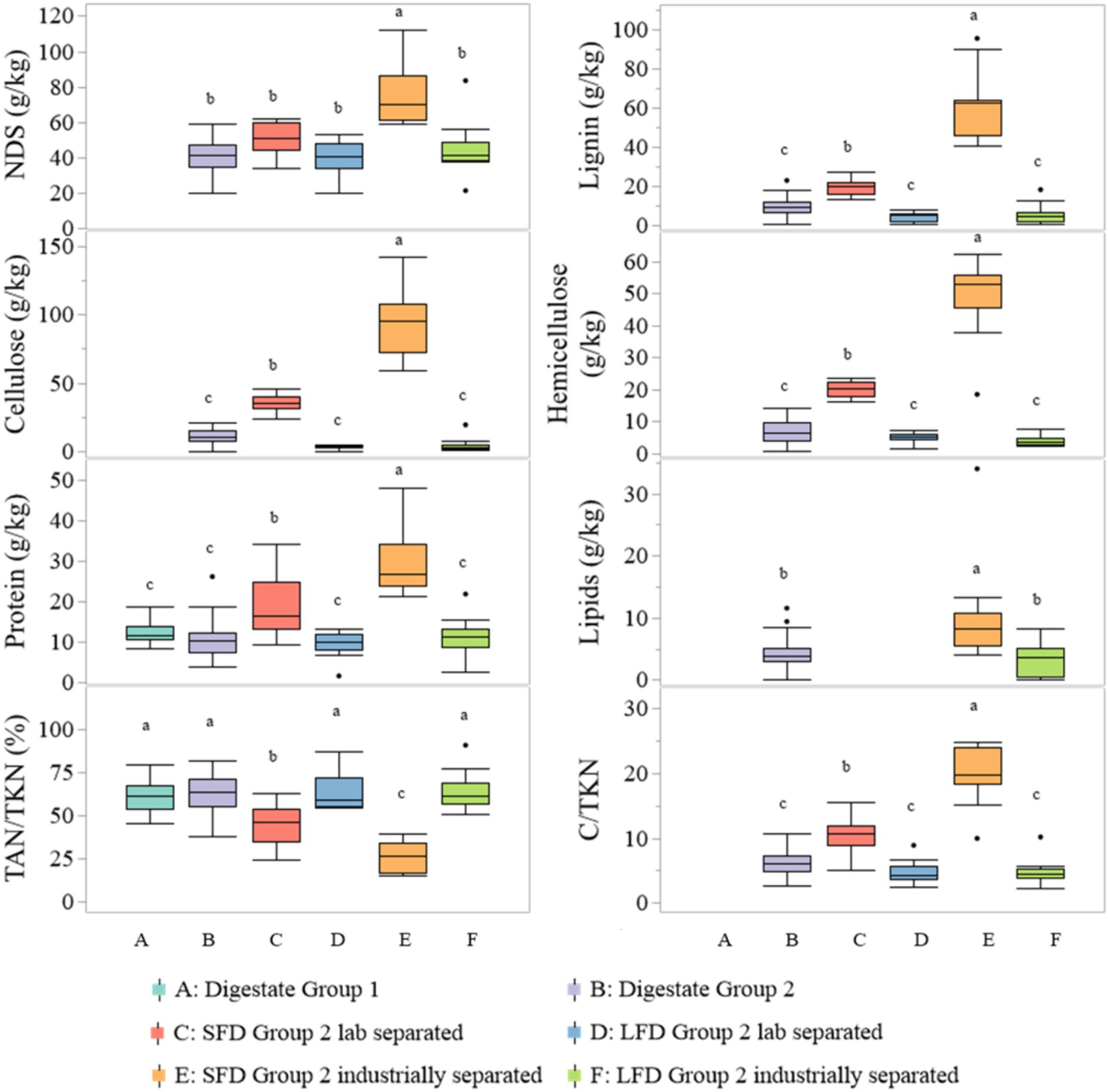
Figure 2. Digestate characterization: distribution of NDS, lignin, cellulose, hemicellulose, protein, lipids, and TAN/TKN and C/TKN ratios. Letter indices above the boxplots originate from the Tukey’s HSD test, comparing means at a significance level of differences set at p < 0.05, where different letters represent significant differences among the groups of samples. VFA, volatile fatty acids; TAN, total ammoniacal nitrogen; TS, total solids; VS, volatile solids; NDS, neutral detergent soluble fraction; TKN, total Kjeldahl nitrogen; SFD, solid fraction of digestate; LFD, liquid fraction of digestate.
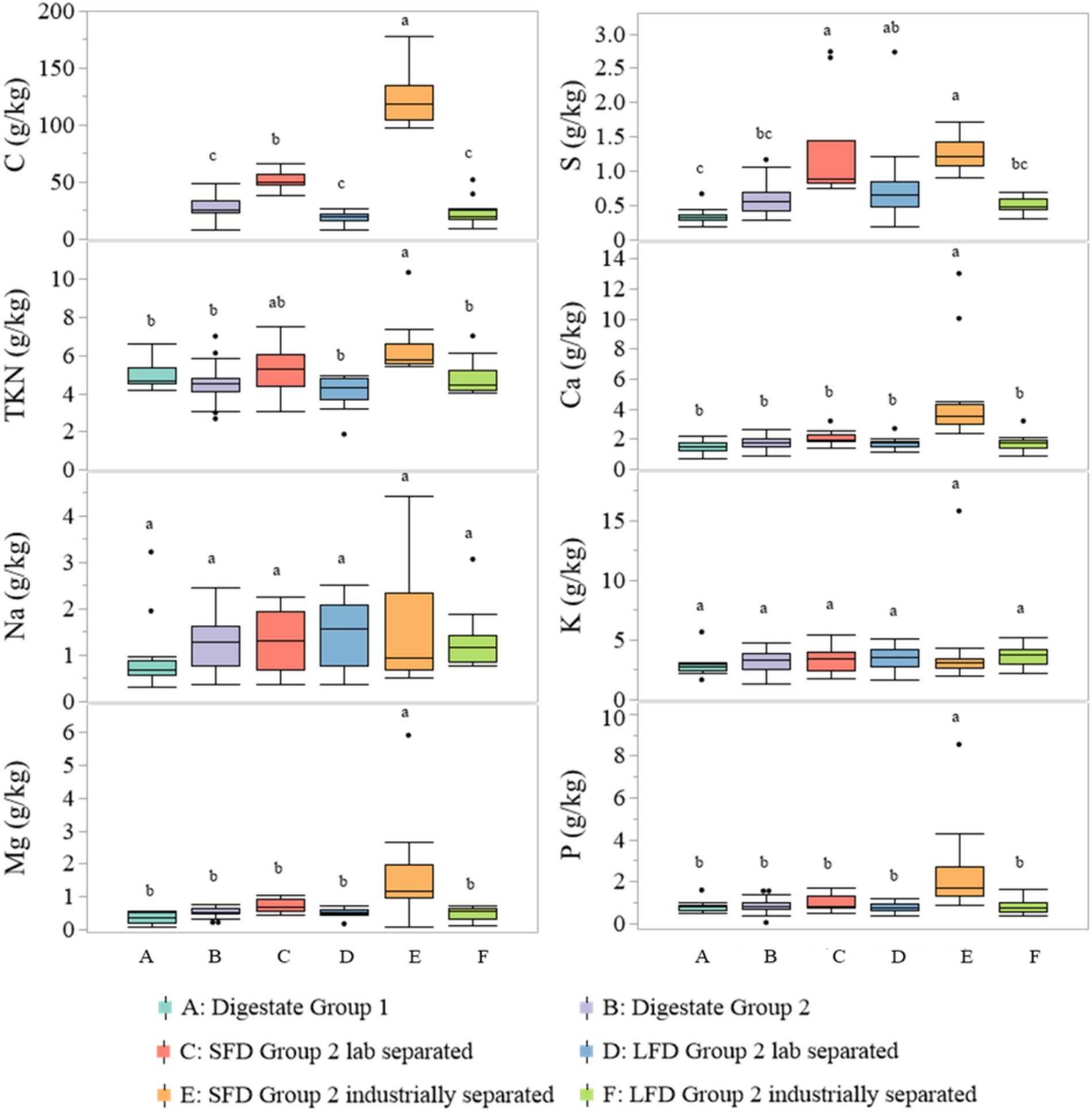
Figure 3. Digestate characterization: distribution of nutrients. Letter indices above the boxplots originate from the Tukey’s HSD test, comparing means at a significance level of differences set at p < 0.05, where different letters represent significant differences among the groups of samples. TKN, total Kjeldahl nitrogen; SFD, solid fraction of digestate; LFD, liquid fraction of digestate.
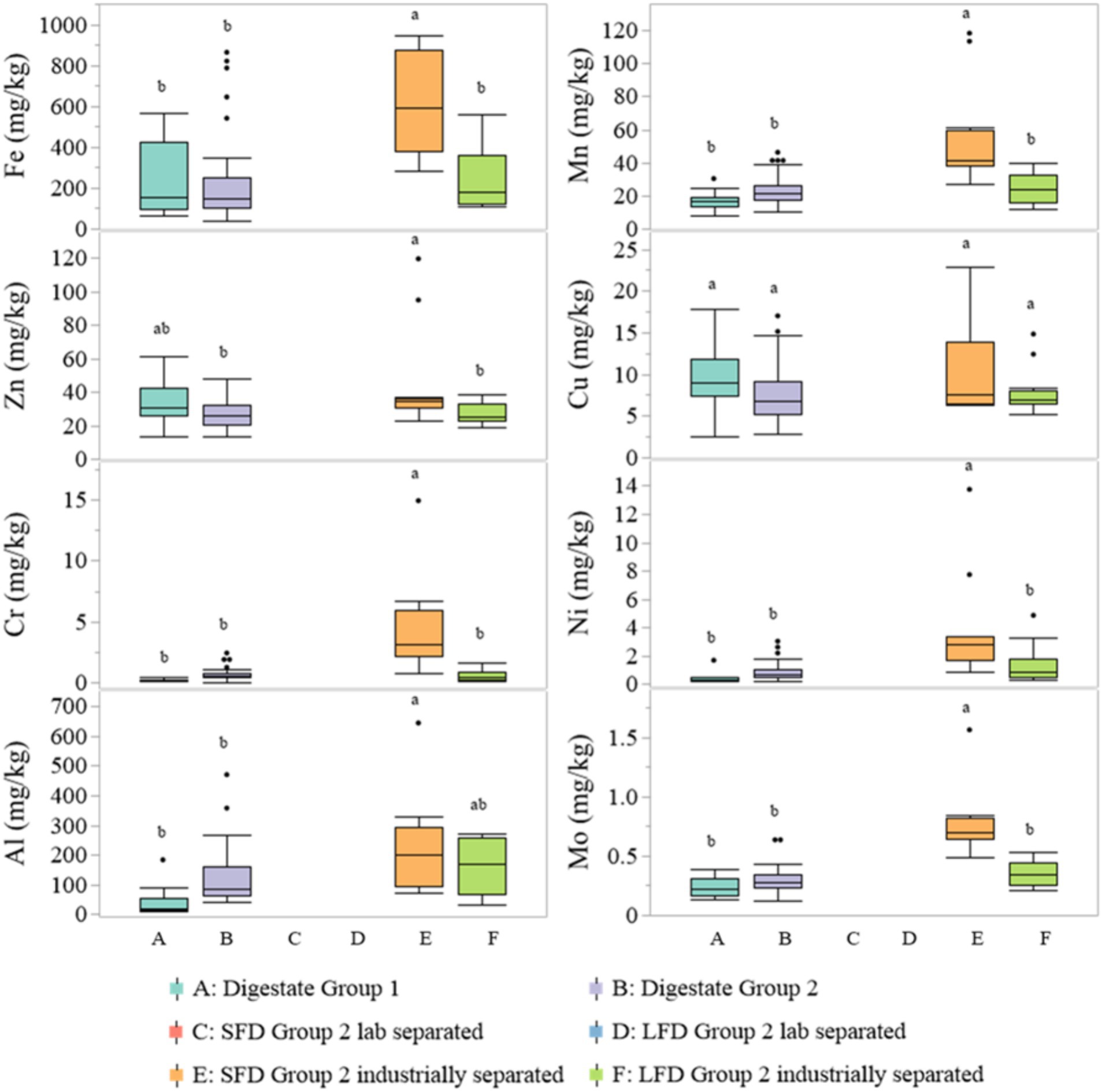
Figure 4. Digestate characterization: distribution of micro-nutrients and heavy metals. Letter indices above the boxplots originate from the Tukey’s HSD test, comparing means at a significance level of differences set at p < 0.05, where different letters represent significant differences among the groups of samples. SFD, solid fraction of digestate; LFD, liquid fraction of digestate.
The pH of digestate samples belonging to Group 2 was, on average, 8.04, significantly (p < 0.05) higher than the average pH of digestate samples from Group 1, which was 7.87 (Figure 1). The same applies to the TS content, which was, on average, 71.03 g/kg for samples from Group 2 against 46.85 g/kg for digestates from Group 1 (Figure 1). The VS content followed the same trend on a fresh matter basis (Figure 1). However, no significant differences (p > 0.05) were found for the VS content on a TS basis, resulting in average VS contents of 705.50 and 709.42 g/kg TS for digestate samples from Group 1 and Group 2, respectively (Table 2). No significant differences (p > 0.05) were observed for the conductivities, VFA and TAN concentrations on a fresh matter basis, and TAN/TKN ratios between samples from Group 1 and Group 2 (Figures 1, 2). VFA contents were, on average, 1.25 and 0.62 g/kg for digestates from Group 1 and Group 2, respectively. However, a few outliers were present with high VFA concentrations. TAN contents were, on average, 3.01 and 2.80 g/kg for digestates in Group 1 and Group 2, and the average TAN/TKN ratios were 60.08 and 63.05%. The TAN (Figure 1), protein (Figure 2), TKN, K, P (Figure 3), Fe, Zn, and Cu (Figure 4) contents did not differ (p > 0.05) in samples from Group 1 and Group 2 when considered on a fresh matter basis. However, when their contents on a TS basis were evaluated, significantly (p < 0.05) higher contents in samples from Group 1 were encountered (Table 2). No significant differences (p > 0.05) were observed between the two groups when considering the contents of Na, Mg, S, Ca (Figure 3), Mn, Cr, Ni, Al, and Mo (Figure 4), neither on a fresh matter nor on a TS basis.
Within Group 2, the LFD samples did not differentiate (p > 0.05) from the unseparated digestate when characteristics on a fresh matter basis were compared (Figures 1–4). The same occurred for the ratios of TAN/TKN and C/TKN. When characteristics were evaluated on a TS basis, lower (p < 0.05) VS, lignin, cellulose, and C contents were obtained for lab or industrially separated LFD. In contrast, the contents of NDS, S, TKN, Ca, K, and Mo were higher (p < 0.05) (Table 2). On a fresh matter basis, the VFA (Figure 1), Na, K (Figure 3), and Cu (Figure 4) contents of SFD and unseparated digestate samples were similar (p > 0.05), while TAN contents were lower (p < 0.05) in industrially separated digestate (Figure 1). TAN/TKN ratios were lower, while C/TKN ratios were higher in SFD samples (p < 0.05) compared to unseparated digestate (Figure 2). C/TKN were, on average, 10.38 and 19.99 for lab and industrially separated SFD. The remaining components were higher in lab or industrially separated SFD samples than in unseparated digestates (p < 0.05) (Figures 1–4). When considering the concentrations on a TS basis, lab or industrially separated SFD samples displayed higher (p < 0.05) VS, cellulose, hemicellulose, lignin, C, and calorific values contents and lower (p < 0.05) TAN, NDS, TKN, S, K, Ca, Na, Mo, Al, Cu, Mn, and Zn concentrations than unseparated digestate samples (Table 2).
The average calorific value of unseparated digestates from Group 2 was 1.21 MJ/kg. The calorific values of lab and industrially separated SFD were higher (p < 0.05), on average, 2.22 and 5.28 MJ/kg, respectively (Table 2). On a TS basis, the average calorific value of the unseparated digestate samples was 16.88 MJ/kg TS. Only the industrially separated SFD achieved a significantly higher (p < 0.05) average calorific value, resulting in 18.33 MJ/kg TS (Table 2). When calculated on a VS basis, the average calorific value of unseparated digestate achieved a content of 23.83 MJ/kg VS. In this case, the calorific values of both SFD groups were lower while the calorific value of the industrially separated LFD was higher (Figure 1).
3.2 Separation efficiency
Figure 5 presents the TS contents of the unseparated digestate and the resulting LFD after separation by different methods, i.e., lab-scale and industrial separation by screw press and decanter centrifuge. Linear curves represented well the relationships between the TS contents of the samples. According to the curve slopes, the decanter centrifuges were the most efficient at reducing the TS contents of the LFD, followed by the lab-scale separation. Average reductions in TS contents from the unseparated digestate were 32.35, 26.82, and 18.69% for decanter centrifuge, lab-scale separation, and screw press, with ranges of 28–39, 19–39, and 2–32%, respectively. It should be noticed, however, that only three samples (B8, B9, and B18) were separated with decanter centrifuges, and their initial TS contents were some of the lowest. Contrarily, screw presses were employed for the separation of some of the samples with the highest TS contents.
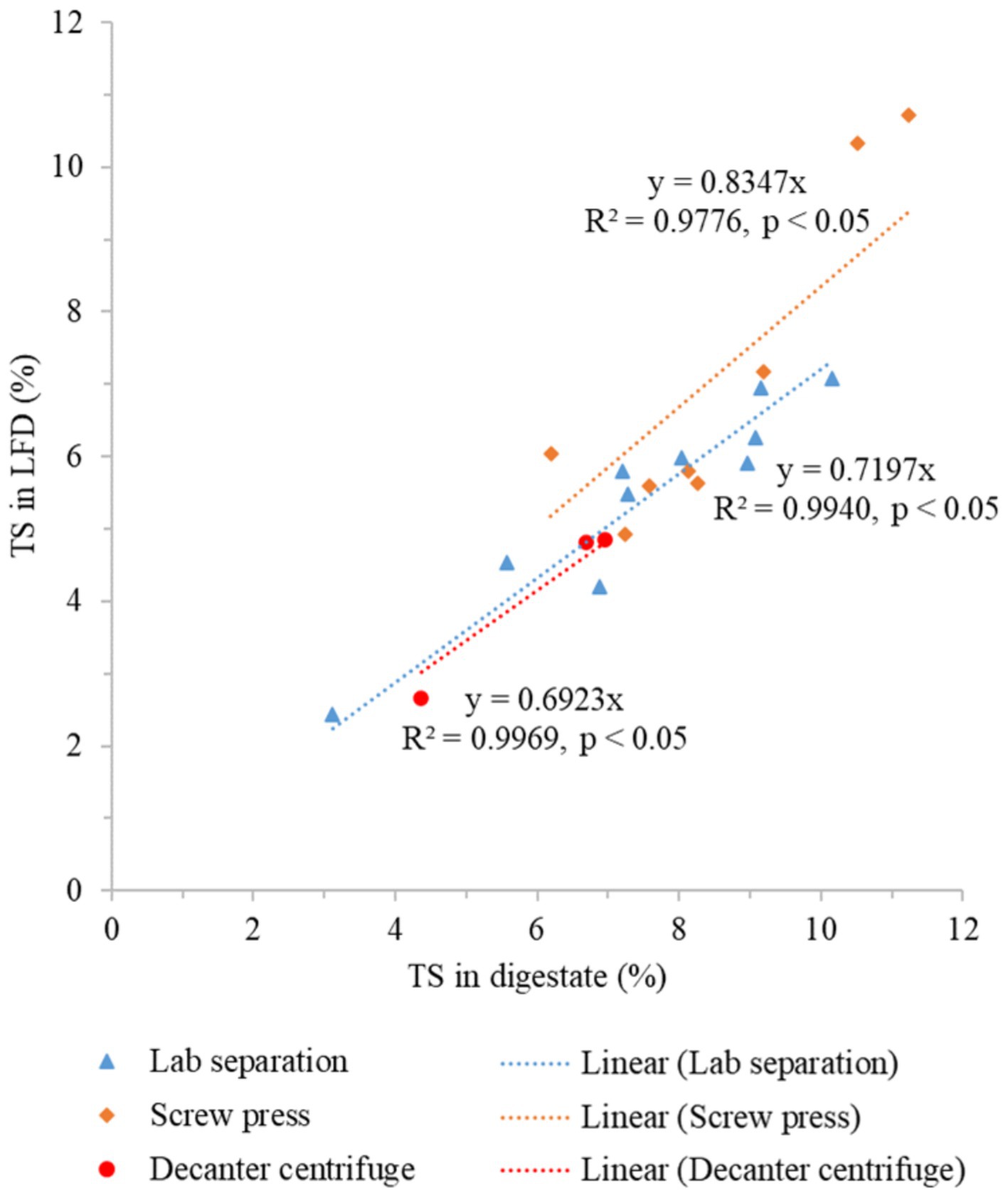
Figure 5. Total solids reduction in digestate separation. TS, total solids; LFD, liquid fraction of digestate.
The distributions of digestate components fractioned into solid and liquid fractions and the RSI of lab-scale and industrially separated samples are presented in Figure 6. The averages of every component mass fraction in the SFD (corresponding to the SIs) and in the LFD and the RSIs are displayed in Table 3. Values presented in Figure 6 and Table 3 only considered groups of samples in which mass balance errors were not greater than 20%. The mass balances for all groups of samples, including the excluded ones, can be found in the Supplementary material, along with the absolute values of digestate fractionation into SFD and LFD, the SIs, and RSIs for all samples.
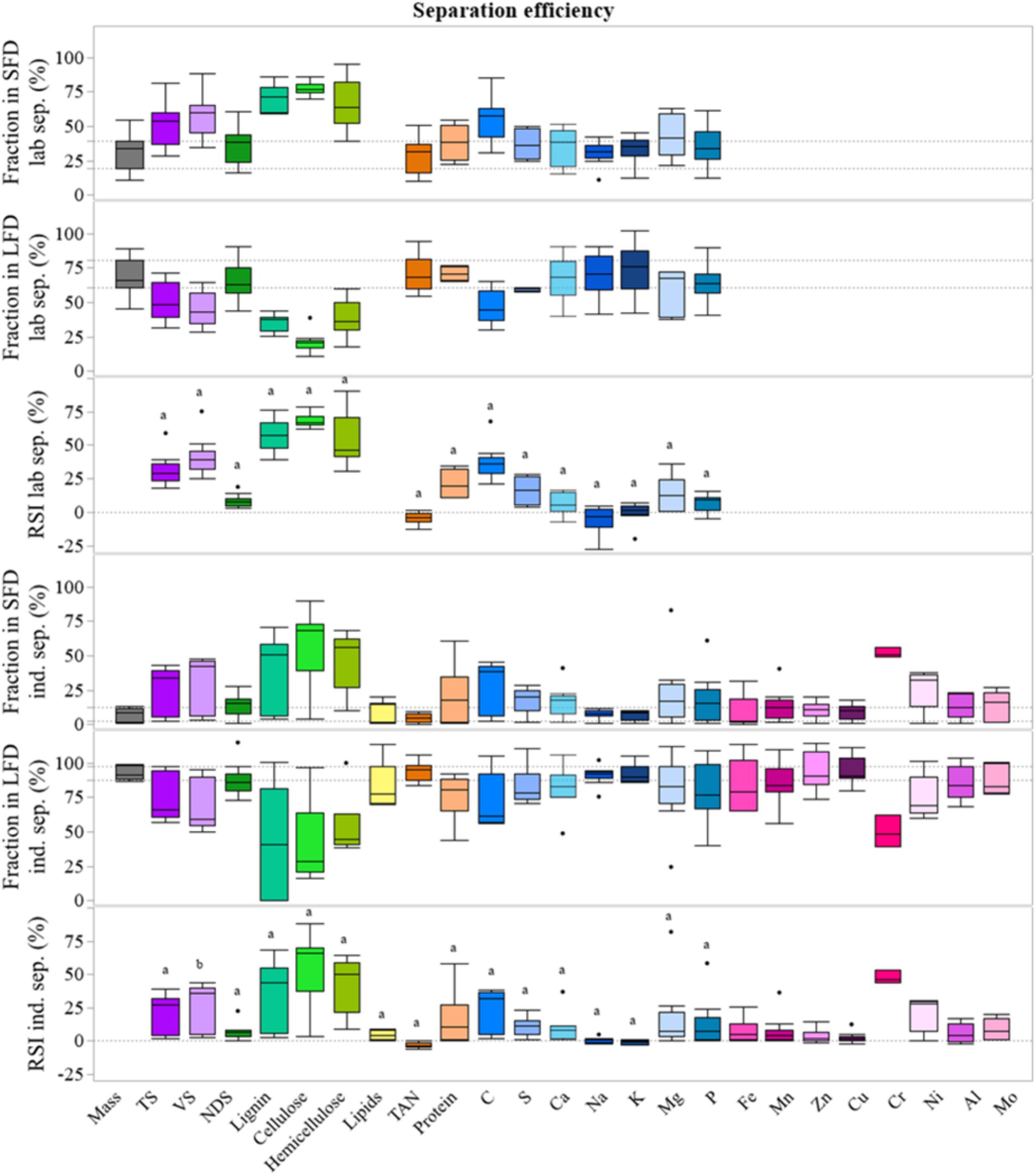
Figure 6. Digestate characterization: separation efficiency. Letter indices above the boxplots originate from the Tukey’s HSD test, comparing means at a significance level of differences set at p < 0.05, where different letters represent significant differences (comparisons are made between the RSI of each component obtained through lab sep. and ind. sep.). SFD, solid fraction of digestate; LFD, liquid fraction of digestate; RSI, relative separation index; lab sep., lab-scale separation; ind. sep., industrial separation; TS, total solids; VS, volatile solids; NDS, neutral detergent soluble fraction; TAN, total ammoniacal nitrogen.
On average, 31.31% of the digestate mass was retained in the SFD when separated in lab-scale (Table 3), resulting in an average TS content in the SFD of 128.02 g/kg and approximately equal fractions of TS in the LFD and SFD. The average TS of the SFD obtained through industrial separation was 286.97 g/kg and was significantly higher (p < 0.05) than that obtained with lab-scale separation (Table 2). In this case, the estimated mass retained in the SFD was, on average, only 7.24%, resulting in most of the TS (73.16%), remaining in the LFD (Table 3). The resulting RSIs of the TS were 31.59 and 21.65% for lab-scale and industrial separation, respectively (Table 3).
Except for VS, the RSIs of all components separated by both lab-scale and industrial methods did not differ significantly (p > 0.05) by the scale of separation (Figure 6). In the case of VS, the highest RSI was obtained when employing the lab-scale separation, with an average of 41.80% (Table 3). The separation methods also selectively highly retained lignin, cellulose, hemicellulose, and Cr in the SFD (Figure 6), with average RSIs between 36.07 and 68.60% (Table 3). Some moderate RSIs were obtained for protein, C, S, Mg, and Ni (10.67–37.56%) (Table 3). Lower RSIs were achieved by lipids, NDS, Ca, P, Fe, Mn, Zn, Cu, Al, and Mo (2.49–13.90%) (Table 3). The average RSIs of TAN, Na, and K were negative (Table 3), indicating that they were preferably transferred to the LFD.
The results of industrial separation in Figure 6 do not discriminate between separation by screw presses or decanter centrifuges. However, the outliers in the RSIs of NDS, Ca, Mg, P, Mn, and Cu correspond to sample B18, which was separated with a decanter centrifuge.
3.3 Residual methane yields
The experimental methane yields obtained in the batch tests and by mass balance for lab-scale separated SFD and the kinetic parameters calculated with models are presented in Table 4. The first-order kinetic model represented well the methane production of all groups of samples with a minimum r2 of 0.939, except for the SFD samples separated industrially. For the latter group, the Modified-Gompertz model was utilized, resulting in a minimum r2 of 0.962. The digestion of these samples was accompanied by long lag-phases, averaging 15.47 days.
The average ultimate methane yields of digestate samples belonging to Group 1 were significantly higher (p < 0.05) than those achieved by samples from Group 2 (152.04 mL/g VS against 99.40 mL/g VS) (Table 4). On a fresh matter basis, the difference was not significant (p > 0.05), with average ultimate methane yields of 4.91 and 4.95 mL/g obtained for Group 1 and Group 2. Within Group 2, the methane yields of the digestate fractions (LFD and SFD) were not significantly different from those of unseparated digestate on a VS basis (Table 4). On a fresh matter basis, significant differences (p < 0.05) were observed only for the SFD, where average ultimate methane yields were 8.94 and 24.88 mL/g in samples separated in the lab and industrially.
When considering only the samples utilized in the lab-scale separation experiments, the average ultimate methane yields of the unseparated digestate, SFD, and LFD were 86.46, 86.86, and 80.95 mL/g VS (or 4.62, 8.94, and 2.73 mL/g). When samples separated industrially were evaluated, the average methane yields were 112.79, 104.49, and 98.40 mL/g VS (or 5.98, 26.87, and 3.81 mL/g) for unseparated digestate, SFD, and LFD. In the first case, approximately 60% of the methane potential would be captured in the SFD against 40% in the LFD. However, the industrial separation of digestate would result in most of the methane potential held in the LFD (59%).
3.4 Correlations among digestate characteristics, operational conditions, methane yields, and calorific values
In Table 5, the linear correlations factors are presented. Equations describing significant linear relationships are shown in the Supplementary material. Correlation factors were not consistent within all groups of samples. Significant (p < 0.05) correlations included negative relationships between the ultimate methane yield in Group 2 and the temperature (r = −0.31) and the natural logarithm of the HRT (r = −0.59). When evaluating the digestate composition, the ultimate methane yield correlated positively with the protein content (r = 0.54) in Group 1, positively with the hemicellulose content in the SFD (r = 0.71 for both lab and industrially separated SFD), and negatively with the lignin content in industrially separated SFD and LFD (r = −0.87 and r = −0.69, respectively). The calorific value did not correlate with the operational conditions of the digesters. It presented a negative relationship with the protein content in industrially separated SFD (r = −0.67). Negative correlations were also observed for the NDS content in Group 2 and industrially separated SFD (r = −0.50 and r = −0.82). Positive correlation factors were found for the cellulose content in Group 2 and industrially separated SFD (r = 0.43 and r = 0.64) and for lignin and lipids contents in Group 2 (r = 0.57 and r = 0.44).
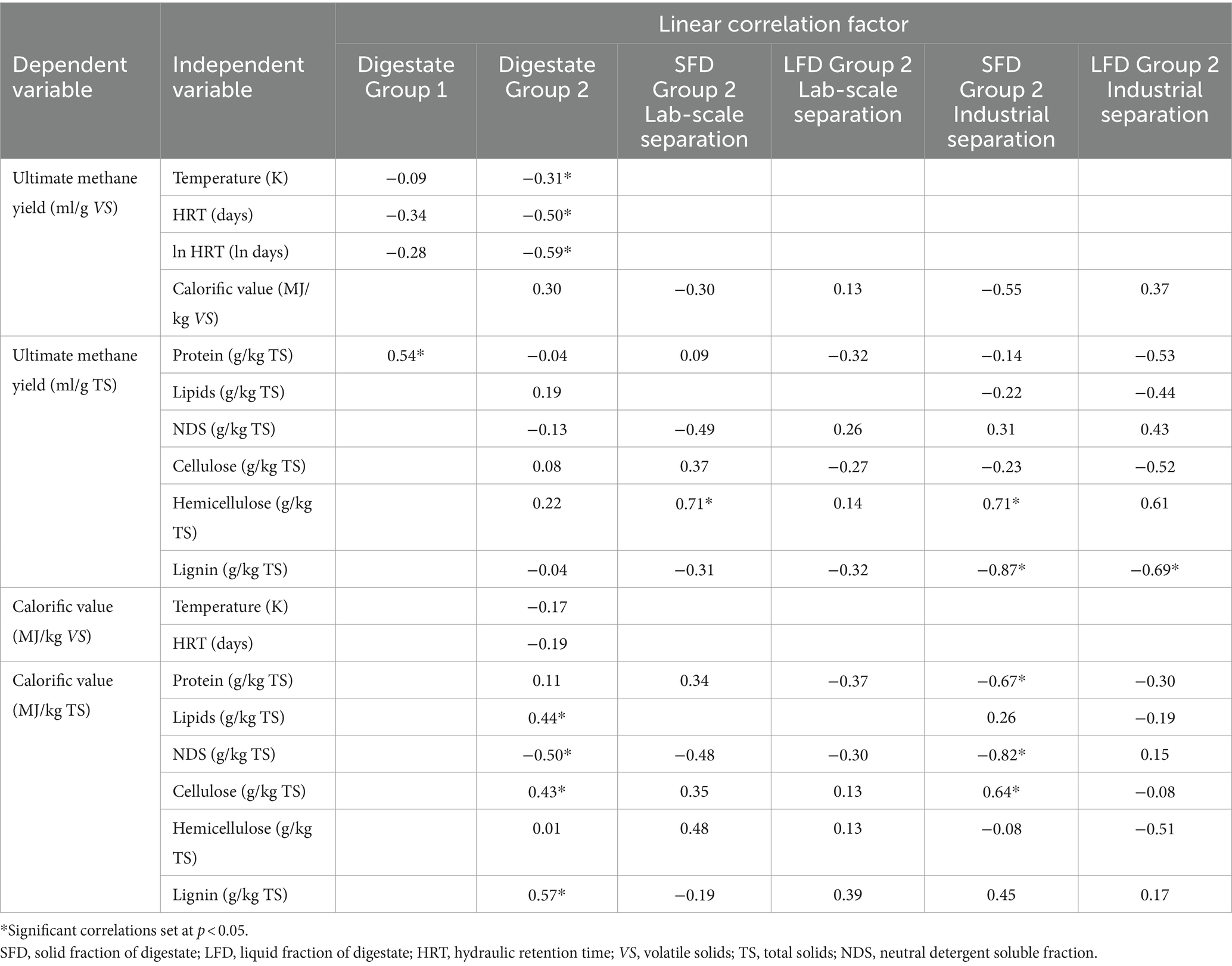
Table 5. Relationships among ultimate methane yields, calorific values, solids composition, and operational conditions.
4 Discussion
4.1 Digestate characterization and its nutrient contents
4.1.1 Nutrient contents of unseparated digestate
Digestate characteristics of samples from Group 1 and Group 2 were, in general, in agreement with values previously reported for agricultural digestate (Schievano et al., 2008; Möller and Müller, 2012; Alburquerque et al., 2012a,b; Monlau et al., 2015; Sambusiti et al., 2015; Tambone et al., 2017; Akyol et al., 2019; Guilayn et al., 2019; Finzi et al., 2021; Ekstrand et al., 2022). Digestate characteristics vary widely in literature, depending on feedstock composition and operational parameters of digesters.
In Denmark all agricultural digestate is currently applied as a fertilizer without solid–liquid separation. Digestate has generally been considered as a good alternative to manure since digestate often presents higher ratios of TAN/TKN (Crolla et al., 2013) and other nutrients in more available forms for plant uptake (Möller and Müller, 2012). Furthermore, digestate contains less pathogens, less odorous compounds, and less easily degradable C than manure (Crolla et al., 2013). The reduction in the contents of readily degradable C during AD results in less availability of this component for denitrification, potentially reducing N2O emissions from digestate when compared to manure (Crolla et al., 2013). Additionally, the loss of N through soil microbial immobilization, stimulated by high C contents and C/TKN ratios, is reduced (Alburquerque et al., 2012b). Despite the benefits, the increase in pH and TAN content during AD and the associated potential increase in ammonia emissions due to a shift in the NH4+/NH3 equilibrium towards ammonia formation are well known (Crolla et al., 2013). Nonetheless, digestate is still widely applied as a soil fertilizer. In the past, when the use of a higher share of manure with minimal amounts of co-substrates in biogas plants was predominant, it was expected that AD would reduce the viscosity of manure due to TS reduction during the process, allowing it to infiltrate faster into the soil, therefore balancing off the effects of higher pH and TAN content on ammonia emissions. However, substantial changes in the substrates fed to anaerobic digesters in Denmark may challenge the fertilizer value of digestate and its acceptance by farmers, as it is discussed in Section 4.1.2.
Besides N, digestate offers appreciable amounts of the other primary nutrients, P and K. However, it is known that the excessive application of nutrients can lead to pollution, for instance, due to the leaching of N and P to water bodies and the consequent stimulation of eutrophication (Teglia et al., 2011). Therefore, in Denmark, the amount of N supplied in digestate must currently not exceed 170 kg/ha/year, in agreement with the EU Nitrates Directive, while P addition is limited to 30 kg/ha/year from 2025 (Sommer and Knudsen, 2021). Depending on the N and P concentrations in each digestate and crop requirements, either of these nutrients should be supplemented by commercial fertilizers. In Group 1 and Group 2, four out of 13 and 26 out of 46 samples would have their application limited by P. Secondary essential nutrients, such as S, Ca, and Mg, are also present in digestate, along with other elements required by plants in smaller amounts, such as Fe, Na, Cu, Zn, Mo, and Mn (Crolla et al., 2013). The source of nutrients in the sampled digestates was restricted to the feedstock material treated by biogas plants since no supplementation is performed in Danish digesters to boost microbial activity in AD. However, Cu and Zn are commonly added to animal diets to prevent pig diseases. Results in this study showed some high variability in the concentrations of all nutrients, especially Fe, Na, Zn, and Cu, evidenced by the high standard deviations compared to the concentrations averages in Table 2. Differences in nutrient composition in digestate can lead to different supplementation requirements to accommodate the needs of different plants (Coelho et al., 2018). Solid–liquid separation of digestate can result in the concentration of different nutrients in the different streams, easing the adjustment of nutrients during land application to the needs of the plants. Further processing, for instance, ammonia stripping to recover TAN as (NH4)2SO4 and struvite precipitation to recover N and P, may be relevant (Barampouti et al., 2020).
Among the mentioned nutrients, Zn and Cu can also have detrimental effects on plants and the environment, as they are heavy metals (Teglia et al., 2011). Zn and Cu, along with the other analyzed heavy metals Ni and Cr, were below the limits set for waste in Danish legislation on a TS or P basis (FLFM, 2018), except for one sample, which surpassed the limit for Ni on a TS basis. In general, digestate does not need to comply with the waste legislation in Denmark since digestate is regarded as manure with no heavy metals restrictions. However, biogas plants cannot treat any waste products that exceed the limits. When the biomass is anaerobically digested, the TS based heavy metal contents are almost doubled since approximately 50% of the TS are converted into biogas. Therefore, when waste is treated, it can be more appropriate to use the heavy metals contents in relation to P, which is conserved in the AD process. Therefore, it can be inferred that the materials fed to the digester were within the limits set for waste.
High concentrations of Na+ and other free ions may also negatively affect crop growth by impacting the biological activity in soil (Teglia et al., 2011) and causing osmotic stress, affecting the uptake of essential nutrients (Machado and Serralheiro, 2017). Conductivity can be an indicator of salinity since it indicates the concentration of free ions (Coelho et al., 2018). While Na concentrations differed notably within each group of samples, the conductivities presented low variability, indicating overall similar salinities of the samples and, possibly, similar risks of harmful effects.
Despite part of the C fed to digesters being recovered as biogas, substantial contents of C remained in digestate. A fraction of it was present as VFA, which are easily degradable and can lead to odorous (Crolla et al., 2013) and greenhouse gas emissions during digestate storage and application. Most digestates presented low concentrations of VFA, but some samples contained high VFA levels, achieving values up to 11.55 g/kg. Soluble organic compounds present in NDS, hemicellulose, lipids, and proteins may also be degraded during storage and after incorporation into the soil. At the same time, cellulose and, especially, lignin are supposed to be more stable forms of C, partially contributing to C sequestration into the soil.
4.1.2 Effects of feedstock shift on characteristics of unseparated digestate
The shift in feedstock in Danish biogas plants seen in recent years was accompanied by important changes in digestate quality. Although Group 2 focused on biogas plants that employed large shares of lignocellulosic substrates, such as straw and deep litter, and do not represent a national average, it still is a good representative of future digestates (Pedersen and Hafner, 2023). The most evident change in digestate quality was the increase of average TS content (approximately 52%) seen in samples from Group 2 despite the longer HRTs. The higher TS can make digestate handling and field application difficult and potentially increase ammonia emissions following broadcast or band application by reducing digestate infiltration into the soil and prolonging its exposure to the atmosphere (Sommer and Hutchings, 2001). Additionally, samples in Group 2 presented an increase (p < 0.05) in average pH of 0.17 units while the average TAN contents remained unaffected (p > 0.05). This may further increase ammonia emissions by the shift in NH4+/NH3 equilibrium towards ammonia formation (Sommer and Hutchings, 2001). Modeling predictions showed that field application by trailing hose of samples from Group 2 would cause higher ammonia emissions than undigested cattle and pig slurry (Pedersen and Hafner, 2023). For some samples, losses of 60% of the applied TAN were estimated (Pedersen and Hafner, 2023). Evidently, experimental trials are required to endorse these predictions. The potential loss of TAN reduces the attractivity of digestate for the farmers, requiring the implementation of ammonia emissions mitigation strategies. Digestate soil injection can reduce ammonia emissions by lowering the emitting surface area (Pedersen and Hafner, 2023); however, the method is energy demanding and can damage crops (Hansen et al., 2003). Additionally, injection may stimulate N2O emissions by lowering the O2 supply due to a more concentrated placement of the slurry (Thomsen et al., 2010; Petersen and Sommer, 2011). Therefore, alternative treatments to reduce digestate TS and pH may become crucial. Feedstock physical, chemical, and biological pre-treatments (Carrere et al., 2016) may result in a reduced TS content in digestate due to enhanced organic matter degradation during AD but can be limited by high costs. Solid–liquid separation of digestate appears as an obvious option to reduce TS contents; however, as seen in Section 4.1.5, enhancements of the currently applied technologies are required. Acidification with H2SO4, commonly applied to animal slurry (Fangueiro et al., 2015), can be challenging on digestate due to its high buffer capacity, requiring large amounts of acid (Møller et al., 2022), which increases costs and risks of overfertilization with S (Pedersen and Hafner, 2023). A recently explored technology to reduce digestate pH is plasma treatment, which fixes N from the atmosphere in the digestate as NO3− and NO2−, forming the acids HNO3 and HNO2 (Nyangau et al., 2024). However, the treatment is costly and the risks of increased N2O emissions should be assessed.
Regarding the TS composition of digestate, significant differences (p < 0.05) were observed between Group 1 and Group 2. Samples from Group 2 showed average lower TAN, TKN, protein, K, P, Fe, Zn, and Cu contents, indicating the lower contents of these compounds in the substrates substituting energy crops and other materials. Compositional differences between straws and energy crops can be considerable. For example, Garcia-Ruiz et al. (2019) found average wheat straw C/N ratios between 82.4–152.1 depending on variety, soil and management techniques, which were much higher than typical values for cropped silages of 30.8 for maize silage and 19.4 for grass silage (Bahar et al., 2005). These differences can directly affect the digestate quality.
4.1.3 Nutrient contents of the liquid fraction of digestate
In general, pH, conductivities, TS contents, TS/VS, TAN/TKN, and C/TKN ratios, and concentrations of some nutrients and heavy metals in the LFD obtained with screw presses were in line with those in the literature (Tambone et al., 2017; Guilayn et al., 2019; Mazzini et al., 2020; Akhiar et al., 2021; Finzi et al., 2021; Cathcart et al., 2023), except for Cu and Zn, whose concentrations were higher than those reported. TS contents of the LFD originating from the separation with decanter centrifuges were higher than those reported by Akhiar et al. (2021) and Cathcart et al. (2023), resulting in also higher concentrations of other components on a fresh matter basis. Data regarding the lignocellulosic composition and lipids contents in LFD are still scarce in the literature.
Within Group 2, LFD samples presented lower TS contents than unseparated digestate, which were brought to levels closer to those presented by samples from Group 1. However, differences in TS contents were not statistically significant (p > 0.05). Some samples of LFD still presented very high TS contents, achieving values of up to 107.12%. Considering the similar levels of pH and TAN in the LFD as those of unseparated digestate (p > 0.05), the application of LFD with a more efficient solid–liquid separation, achieving higher reduction in TS, could contribute to counterbalance the potential increase in ammonia emissions during digestate soil application in unseparated digestate from Group 2 due to its increased TS content compared to Group 1. Lower TS contents in the LFD could also ease digestate handling and soil application since the material may become more pumpable, and blockages of application equipment may be avoided. Pedersen et al. (2022) estimated the ammonia emissions in unseparated digestate and LFD based on literature data concerning emission factors and digestate separation efficiency. The authors showed that a clear reduction in emissions could be obtained by utilizing LFD as a soil fertilizer instead of the unseparated digestate when trailing hoses and trailing shoes were employed, even when including the emissions from the SFD during storage and application. However, estimates were based on few data available in the literature, with even fewer being representative of Danish digestates containing high TS contents. Additionally, the authors reviewed that, in some cases, emissions factors were higher for the liquid fraction of cattle and pig slurry compared to those of raw slurry. The reasons were not clear, but a reduction in TS may reduce the mass transfer resistance, which impedes ammonia volatilization (Pedersen et al., 2022), or increase the exposed surface area of the material due to more spreading out after application (Pedersen and Hafner, 2023). Therefore, field experiments are crucial to determine whether attainable TS reductions with the currently utilized separation methods are sufficient to deliver significant reductions in ammonia emissions from the new Danish digestates or if such a technique could have undesirable consequences as those observed for some samples of separated animal slurry. The assessment of emissions of other environmentally harmful gases, such as CH4 and N2O, during storage of LFD and SFD and their comparison to the emissions associated with the unseparated digestate, is also important.
Regarding other characteristics, results showed comparable concentrations to unseparated digestate on a fresh matter basis (p > 0.05), even though the concentrations of some components in the SFD were significantly higher (p < 0.05). This may be explained by the low mass of digestate retained as SFD when industrial separators were employed and the low differences in concentrations between LFD and SFD obtained in lab-scale separation. Comparable concentrations in LFD and unseparated digestate have also been reported in literature, for instance for TKN, P (Finzi et al., 2021; Cathcart et al., 2023), and TAN (Finzi et al., 2021) when separations were performed with screw presses and for K when separations were conducted with either screw presses or decanter centrifuges (Finzi et al., 2021; Cathcart et al., 2023). However, when decanter centrifuges were utilized, Cathcart et al. (2023) observed clear reductions in concentrations of TKN and P in the LFD. The reduction in contents of VS, lignin, cellulose, and C contents and the increase in NDS, S, TKN, Ca, K, and Mo on a TS basis (p < 0.05) in the industrially separated LFD compared to unseparated digestate samples indicate that the first group of components may be associated with larger particles, while the former may be associated with smaller ones. Contents of measured heavy metals were within legislated recommendations for waste, except for Ni, which surpassed the limit for two LFD samples, but only on a TS basis.
4.1.4 Nutrient contents of the solid fraction of digestate
In most cases, TS contents, VS/TS and C/TKN ratios, and concentrations of some nutrients and heavy metals in industrially separated SFD were comparable to those reported for SFD obtained by screw presses (Thygesen et al., 2014; Sambusiti et al., 2015; Tambone et al., 2017; Guilayn et al., 2019; Brémond et al., 2020; Mazzini et al., 2020; Akhiar et al., 2021; Brémond et al., 2021; Finzi et al., 2021; Cathcart et al., 2023) and decanter centrifuges (Kratzeisen et al., 2010; Thygesen et al., 2014; Akhiar et al., 2021; Cathcart et al., 2023), except for C contents, which were higher than those presented in the literature. TAN contents and TAN/TKN ratios in the SFD samples obtained after separation with decanter centrifuges were lower than those in the literature, while data from SFD originated from screw presses were similar to reported values. As in the case of LFD, data about lignocellulosic composition and lipids contents are still limited.
In comparison to the unseparated digestate from Group 2, the concentrations of VFA, Na, K, and Cu remained constant in industrially separated SFD on a fresh matter basis (p > 0.05), while TAN contents and TAN/TKN contents decreased (p < 0.05). C/TKN ratio and the contents of other parameters increased (p < 0.05). Finzi et al. (2021) and Cathcart et al. (2023) also reported higher TKN and P and similar K contents in the SFD compared to the unseparated digestate. Finzi et al. (2021) additionally observed similar TAN contents in SFD, while Cathcart et al. (2023) described higher C contents in this fraction. On a TS basis, lower contents of NDS, TKN (likely driven by TAN), S, Ca, K, and Mo and higher contents of VS, cellulose, lignin, and C were found in the industrially separated SFD compared to unseparated digestate (p < 0.05). The opposite trends were noticed for LFD. Hence, these observations corroborate the idea that the first group of compounds may be associated with smaller particles, while the second should be part of larger ones.
The differences in nutrients concentrations in SFD compared to unseparated digestate, especially the higher P contents, make SFD an attractive fertilizer to be applied to soils lacking P. Considering the legislation limits for application of N and P, 11 out of 12 of the industrially separated SFD would have their soil application limited by P concentrations. Soils lacking P, however, are usually located in areas far from agricultural biogas plants, where intensive farming is present. The higher TS contents of SFD could reduce the transportation costs of digestate to these locations. The contents of heavy metals were lower than the national limits for waste, except for Ni, where the concentration on a TS basis was higher than allowed for one SFD sample.
Although the SFD has fertilizer properties, it has mainly been proposed as a soil amendment due to its high organic matter content, which is more stable than the organic matter of undigested organic wastes. Soil amendments improve soil properties, such as water retention, permeability, water infiltration, aeration, or structure, providing a better environment for roots (Teglia et al., 2011). Surface-applied SFD should be incorporated into the soil to avoid odor emissions and loss of nutrients through runoff or volatilization (Crolla et al., 2013). Given the higher C/TKN ratios in SFD than in unseparated digestate, the risks of N immobilization may be increased. Most industrially separated SFD samples presented C/TKN ratios higher than 18, often assumed as a threshold for immobilization stimulation (Teglia et al., 2011). However, immobilization risks do not rely solely on C/TKN ratios but also depend on the stability of the organic carbon in soil amendments (Teglia et al., 2011). Although the concentrations of recalcitrant fractions, such as cellulose and lignin, increased in the SFD, the attained methane yields demonstrate that the organic matter in SFD was not completely stabilized.
A process capable of enhancing organic matter stability before soil application is composting, in which part of the organic matter is degraded under controlled aerobic conditions (Akyol et al., 2019). A fraction of the organic substances is transformed into humic compounds, ensuring stability after soil application. Additionally, the increase in temperature during the process can eliminate pathogens that may have remained after AD (Lu et al., 2021). However, composting of SFD faces some challenges. For instance, the C/TKN ratios of the SFD obtained in the present study were lower than those reported as optimal for initiating composting (25–35) (Akyol et al., 2019). The high cellulose and lignin contents may also prolong the duration of composting of SFD due to their recalcitrance (Lu et al., 2021). Finally, the TS contents of SFD were, with few exceptions, also lower than the recommended range of 35–60%, which is optimal for microbial activity and allows proper air transport through the solids pile during the composting process (Akyol et al., 2019). A potential solution for these issues includes adding dry materials with high C contents to the SFD to adjust C/TKN ratios and TS contents for more favorable composting conditions (Akyol et al., 2019). This strategy may also reduce methane and N2O emissions by minimizing the formation of anaerobic zones due to facilitated aeration (Li et al., 2020). However, ammonia emissions may increase as aeration boosts microbial activity, leading to increased temperature in the SFD pile (Li et al., 2020).
4.1.5 Efficiency of solid–liquid separation
Decanter centrifuges were employed to separate digestate in the lowest range of TS contents containing fewer fibers and coarse particles, while screw presses were applied to digestates with more TS and fibrous materials. Decanter centrifuges are generally more efficient at retaining smaller particles than screw presses (Finzi et al., 2021), which explains the higher reductions in TS contents observed in LFD originating from these separators. However, the TS reductions in the LFD obtained with decanter centrifuges were lower than those reported in the literature, which is, on average, approximately 50%, as reviewed by Pedersen et al. (2022). Screw presses were very inefficient for three samples, with TS reductions of less than 5%. The TS reductions in the remaining samples separated by screw presses were in line with those in the literature (Pedersen et al., 2022). In general, both separator types resulted in low amounts of digestate mass recovered as SFD, on average, only 7.24%. It is unclear why the separation efficiencies were, in many cases, lower than those in previous studies. The differences might have been caused by changes in substrate composition and operation of anaerobic digesters. The increasing amounts of lignocellulosic substrates fed to anaerobic digesters have forced biogas plants to implement maceration as a pre-treatment of certain recalcitrant substrates, such as straw. The process reduces the sizes of particles, which, to some extent, are recovered in the LFD. At the same time, HRTs have been prolonged, also leading to smaller particles in digestate. The separation with screw presses may be improved by utilizing smaller screen sizes with 0.5 mm, since 1 mm screen size is currently used. Furthermore, the separation process could be optimized by the utilization of precipitating agents, polymers, and flocculants and by integrating sequential separation steps, such as screw presses and decanter centrifuges followed by microfiltration (Barampouti et al., 2020).
The major part of the digestate mass and its components remained mainly within the industrially separated LFD, except cellulose, hemicellulose, lignin, and Cr, which were retained mainly in the SFD. Accordingly, Guilayn et al. (2019) have reported that most of the TS, VS, organic N, TAN, P, K, S, Ca, and Mg remained in the LFD, while Tambone et al. (2017) observed the same trend for TS, TKN (likely driven by TAN), and P. Excluding the three mentioned samples with very inefficient separation, the SIs of industrially separated digestate were comparable to those reported in the literature for fresh mass, TS, VS, organic N (or protein), TAN, P, K, and C (Tambone et al., 2017; Guilayn et al., 2019; Finzi et al., 2021; Cathcart et al., 2023). The RSIs of TS, K, and TAN of digestate separated by screw press were similar to those reported by Finzi et al. (2021). Data on the RSIs of components resulting from the separation of agricultural digestate with a decanter centrifuge are limited in the literature. However, Cathcart et al. (2023) showed that the SIs of TS, C, TKN, P, and K were higher for decanter centrifuges than screw presses. Likewise, in the present study, the separation with decanter centrifuges resulted in higher RSIs for P (see Supplementary material). Literature data concerning the RSIs of other components in agricultural digestate have not been found for comparison. The high RSIs obtained for cellulose, hemicellulose, and lignin are linked to their presence mainly in large fibrous particles. Likewise, Cr presented high RSIs. While the reason for this is unclear, Zhu and Guo (2014) showed that Cr had high stability in digestate, being present mainly in residual form and being unlikely to be released to the digestate solution. The separation of the remaining analyzed components resulted, in general, in lower RSIs, suggesting that they were, to a larger degree than cellulose, hemicellulose, lignin, and Cr, also associated with smaller particles (as an original part of them or adsorbed) or partially solubilized (Möller and Müller, 2012; Zhu and Guo, 2014). The negative RSIs obtained for TAN, K, and Na can be explained by their high solubility in water (Baryga et al., 2021; Finzi et al., 2021).
Industrially separated SFD was obtained with different equipment types likely operating with different capacities and at different conditions, such as different applied pressures in screw presses and bowl speeds in decanter centrifuges (Cathcart et al., 2023). Therefore, the lab-scale separation was performed to evaluate RSIs by applying the same separation method to several digestate samples. This type of separation was not efficient, with larger mass proportions retained in the sieve, but with lower TS contents in SFD and smaller differences in components concentrations between LFD and SFD when compared to industrial separation. Nevertheless, the lab-scale separation resulted in average RSIs comparable to those obtained with industrial separation.
4.2 Energetic potential of digestate
Significant amounts of digestible fractions (protein, lipids, cellulose, hemicellulose, part of NDS) were still present in digestate, representing a potential for additional methane recovery in biogas plants. Methane yields of unseparated digestate on a VS basis were within the range of those presented in a previous publication, including some samples belonging to Group 2 (Romio et al., 2023). The average methane yield on a VS basis of samples from Group 1 was approximately 50% higher than that from Group 2 (p < 0.05). The average longer HRT and the more recalcitrant nature of substrates applied to digesters in Group 2 explain this difference. However, the higher TS contents (p < 0.05) of digestates from Group 2 resulted in similar (p > 0.05) average methane yields on a fresh matter basis for both groups. This observation indicates comparable risks of methane emissions during digestate storage, unless the different TS contents affect crust formation, which may also influence gaseous emissions (Wood et al., 2012). The negative relationships (p < 0.05) between the residual methane yield on a VS basis and the temperature and logarithm of HRT found by Romio et al. (2023) were maintained but slightly weakened by the addition of data from new samples in Group 2. Higher temperatures should enhance organic matter degradation, resulting in lower residual methane yields in the digestate. Extending the HRT by expanding digestion volume capacity can be economically feasible for several biogas plants, as previously demonstrated (Romio et al., 2023). Besides enhancing the revenues of biogas plants, this strategy also has the potential to reduce methane emissions during digestate storage prior to field application.
Digestate separation resulted in similar (p > 0.05) methane yields on a VS basis in both SFD and LFD, indicating comparable overall recalcitrance of these fractions despite the different compositions. Protein and hemicellulose contents positively affected the methane yields in some groups of samples, while lignin contents had a negative impact. Protein and hemicellulose are digestible fractions, while lignin is hardly degradable under anaerobic conditions and contributes to increasing the resistance of holocellulose to degradation (Schievano et al., 2008).
Methane yields of the SFD agreed with values found in the literature (Thygesen et al., 2014; Maynaud et al., 2017; Romio et al., 2021). The methane production of LFD started rapidly, while long lag-phases were observed in the digestion of industrially separated SFD. The reasons behind this behavior are unclear, but the larger particles and the reduction in soluble organic compounds in SFD may have had an influence. Moreover, it should be noted that SFD samples had been frozen before digestion, while LFD samples were utilized immediately after collection. The inactivation of AD microbes during the freezing period may have contributed to the longer start-up phase. The same batch assay run included samples of raw cattle manure and wheat straw (both from an unrelated study), and these all showed good biogas production with no significant lag-phase (data not shown). Therefore, low inoculum activity should not have been a reason for the occurrence of the long lag-phases.
On a fresh matter basis, the average methane yield of the industrially separated SFD was approximately seven times higher than that of LFD. The average methane yield of the SFD on a fresh matter basis achieved 46–100% and 126–222% of the yields of samples of maize and grass analyzed by Triolo et al. (2011). However, only 8% of the methane yield of straw was achieved by the SFD (Triolo et al., 2011). The results indicate that SFD has the potential to be reutilized in AD and be a valuable substitute for some expensive, low-quality, or scarce feedstock or simply be included as additional material to the substrate mix in a system where it is recirculated to the digester (Brémond et al., 2021). Likely, only a portion of the SFD should be used to avoid the accumulation of inorganic matter and inhibitors in the digester, such as heavy metals (Brémond et al., 2021), and to avoid an excessive increase in the TS content in the digester, which could difficult mixing and mass and heat transfer. As shown by Dinuccio et al. (2013), the recirculation of the totality of the SFD in continuous reactors operating with a HRT of 40 days resulted in an increase of 16% in the TS content and maintained the volumetric methane production 4% higher than that of control reactors without recirculation for 120 days. After this period, the methane production of the two sets of reactors leveled out, possibly due to the accumulation of recalcitrant compounds in the reactors to which SFD was recirculated. The economic viability of the SFD recirculation would depend on the resulting methane yields, prices of substituted substrates, and costs of digestate solid–liquid separation. Methane production associated with SFD recirculation could be enhanced by applying post-treatment methods to the SFD to open up its structure, increasing its methane yield or accelerating the hydrolysis rate. Several studies evaluated the effects of post-treatments, such as thermal, mechanical, chemical, and biological methods, in the SFD. Varying results were obtained in batch tests, from reductions in methane yields to increases of up to 300%, as reviewed by Romio et al. (2021). In a continuous test, Jagadabhi et al. (2008) showed that recirculating untreated or alkali treated SFD to the digester was unjustified in a system operating with a HRT of 20 days and with a ratio between the mass load of SFD and fresh feedstock of 10%. Nevertheless, studies evaluating continuous tests mimicking the recirculation of the SFD to the digester at different rates with or without SFD post-treatment are still lacking in the literature.
Although industrially separated SFD presents higher methane yields on a fresh matter basis than LFD, most of the methane potential, about 2/3, is held in the LFD due to its higher recovered mass in separation. This means that, although reduced, risks of methane emissions during LFD storage remain, as observed by Gioelli et al. (2011); hence, storage of LFD in covered tanks with methane capture is recommended. The emissions from the SFD should also be considered for an overall assessment of the effects of separation in methane emissions. Emissions from the SFD will depend on storage type (in covered or uncovered tanks or piles) and utilization, i.e., recirculated to the digester, as a soil amendment, composting, or for another purpose.
Alternative uses of SFD include bioethanol production, hydrothermal carbonization with the production of hydrochar, pyrolysis with the production of biochar, syngas, and bio-oil, and combustion, as reviewed by Monlau et al. (2015) and Peng et al. (2020). While bioethanol, hydrochar, syngas, and bio-oil can be further processed for energy generation, biochar has been considered a soil amendment, with a significant role in sequestering carbon in soil (Monlau et al., 2015). Regarding combustion, calorific values were similar to the experimental ones obtained by Czekała (2021) and Kratzeisen et al. (2010) for SFD and comparable to those of wood. These authors suggested that the waste heat in plants owning combined heat and power units could be utilized for SFD drying to a moisture content of about 10–20% and pelletizing could be applied to produce a homogeneous and transportable material. Cathcart et al. (2021) and Kratzeisen et al. (2010) estimated positive energy balances for the combustion of SFD, considering the energy demand for separation, drying, and pelletizing. According to Cathcart et al. (2021), the highest costs of the process would derive from the investment in a belt dryer (corresponding to 61 and 78% of the total costs when separating digestate with a decanting centrifuge and a screw press), as mechanical aid is likely required in the drying process. The profitability of the process would highly depend on the amount of SFD being produced. Kratzeisen et al. (2010) showed that the emissions of flue gas during combustion of SFD pellets were in agreement with German limits set for biofuels and suggested that the ash residue could be a valuable fertilizer since nutrients such as P, K, and minerals are conserved. However, the contents of the heavy metals Ni and Cr were above the safe limits for land application.
5 Conclusion
The transition to higher shares of lignocellulosic feedstock seen in Danish biogas plants in recent years has caused an average increase of 52% in the TS content of digestate and an average pH increase of 0.17 units. These changes may impact ammonia emissions during digestate soil application. Solid–liquid separation and the utilization of the LFD as a fertilizer may alleviate the increased risks of ammonia emissions by reducing TS contents and accelerating digestate soil infiltration rate. However, currently employed separation technologies have not proved highly efficient at reducing TS contents in the LFD, indicating that new approaches are necessary. The SFD was shown to be an attractive fertilizer to be applied to P deprived areas or a valuable source for additional energy recovery through its recirculation to the digester (with average methane yield of 101 mL/g VS) or combustion (with average calorific value of 21 MJ/kg VS).
Data availability statement
The original contributions presented in the study are included in the article/Supplementary material, further inquiries can be directed to the corresponding author.
Author contributions
CR: Conceptualization, Data curation, Formal analysis, Investigation, Methodology, Writing – original draft. AW: Writing – review & editing. HM: Conceptualization, Funding acquisition, Supervision, Writing – review & editing.
Funding
The author(s) declare that financial support was received for the research, authorship, and/or publication of this article. This work was funded by the Ministry of Food, Agriculture, and Fisheries of Denmark through a project with the title Methods for reducing ammonia loss and increased methane yield from biogas slurry (MAG) (journal nr.: 34009-21-1829) and the FertiHood project (journal nr: 34009-20-1707) as part of Organic RDD6, coordinated by the International Centre for Research in Organic Food Systems (ICROFS).
Acknowledgments
The authors acknowledge the biogas plants that provided samples for the conduction of this study.
Conflict of interest
The authors declare that the research was conducted in the absence of any commercial or financial relationships that could be construed as a potential conflict of interest.
Publisher’s note
All claims expressed in this article are solely those of the authors and do not necessarily represent those of their affiliated organizations, or those of the publisher, the editors and the reviewers. Any product that may be evaluated in this article, or claim that may be made by its manufacturer, is not guaranteed or endorsed by the publisher.
Supplementary material
The Supplementary material for this article can be found online at: https://www.frontiersin.org/articles/10.3389/fsufs.2024.1415508/full#supplementary-material
References
Akhiar, A., Guilayn, F., Torrijos, M., Battimelli, A., Shamsuddin, A. H., and Carrère, H. (2021). Correlations between the composition of liquid fraction of full-scale digestates and process conditions. Energ 14:971. doi: 10.3390/en14040971
Akyol, Ç., Ince, O., and Ince, B. (2019). Crop-based composting of lignocellulosic digestates: focus on bacterial and fungal diversity. Bioresour. Technol. 288:121549. doi: 10.1016/j.biortech.2019.121549
Alburquerque, J. A., de la Fuente, C., and Bernal, M. P. (2012a). Chemical properties of anaerobic digestates affecting C and N dynamics in amended soils. Agric. Ecosyst. Environ. 160, 15–22. doi: 10.1016/j.agee.2011.03.007
Alburquerque, J. A., de la Fuente, C., Ferrer-Costa, A., Carrasco, L., Cegarra, J., Abad, M., et al. (2012b). Assessment of the fertiliser potential of digestates from farm and agroindustrial residues. Biomass Bioenergy 40, 181–189. doi: 10.1016/j.biombioe.2012.02.018
Annamalai, K., Sweeten, J. M., and Ramalingam, S. C. (1987). Technical notes: estimation of gross heating values of biomass fuels. Trans. ASAE 30, 1205–1208. doi: 10.13031/2013.30545
APHA (2017). Standard methods for the examination of water and wastewater. 23rd Edn. Washington, DC: American public health association.
Bahar, B., Monahan, F. J., Moloney, A. P., O’Kiely, P., Scrimgeour, C. M., and Schmidt, O. (2005). Alteration of the carbon and nitrogen stable isotope composition of beef by substitution of grass silage with maize silage. Rapid Commun. Mass Spectrom. 19, 1937–1942. doi: 10.1002/rcm.2007
Barampouti, E. M., Mai, S., Malamis, D., Moustakas, K., and Loizidou, M. (2020). Exploring technological alternatives of nutrient recovery from digestate as a secondary resource. Renew. Sust. Energ. Rev. 134:110379. doi: 10.1016/j.rser.2020.110379
Bartoli, A., Hamelin, L., Rozakis, S., Borzecka, M., and Brandao, M. (2019). Coupling economic and GHG emission accounting models to evaluate the sustainability of biogas policies. Renew. Sustain. Energ. Rev. 106, 133–148. doi: 10.1016/j.rser.2019.02.031
Baryga, A., Połeć, B., and Klasa, A. (2021). The effects of soil application of digestate enriched with P, K, mg and B on yield and processing value of sugar beets. Ferment 7:241. doi: 10.3390/fermentation7040241
Brémond, U., Bertrandias, A., de Buyer, R., Latrille, E., Jimenez, J., Escudié, R., et al. (2021). Recirculation of solid digestate to enhance energy efficiency of biogas plants: strategies, conditions and impacts. Energy Convers. Manag. 231:113759. doi: 10.1016/j.enconman.2020.113759
Brémond, U., Bertrandias, A., Loisel, D., Jimenez, J., Steyer, J. P., Bernet, N., et al. (2020). Assessment of fungal and thermo-alkaline post-treatments of solid digestate in a recirculation scheme to increase flexibility in feedstocks supply management of biogas plants. Renew. Energy 149, 641–651. doi: 10.1016/j.renene.2019.12.062
Carrere, H., Antonopoulou, G., Affes, R., Passos, F., Battimelli, A., Lyberatos, G., et al. (2016). Review of feedstock pretreatment strategies for improved anaerobic digestion: from lab-scale research to full-scale application. Bioresour. Technol. 199, 386–397. doi: 10.1016/j.biortech.2015.09.007
Cathcart, A., Smyth, B. M., Lyons, G., Murray, S. T., Rooney, D., and Johnston, C. R. (2021). An economic analysis of anaerobic digestate fuel pellet production: can digestate fuel pellets add value to existing operations? Clean. Eng. Technol. 3:100098. doi: 10.1016/j.clet.2021.100098
Cathcart, A., Smyth, B. M., Lyons, G., Murray, S. T., Rooney, D., and Johnston, C. R. (2023). Optimising mechanical separation of anaerobic digestate for total solids and nutrient removal. J. Environ. Manag. 345:118449. doi: 10.1016/j.jenvman.2023.118449
Coelho, J. J., Prieto, M. L., Dowling, S., Hennessy, A., Casey, I., Woodcock, T., et al. (2018). Physical-chemical traits, phytotoxicity and pathogen detection in liquid anaerobic digestates. Waste Manag. 78, 8–15. doi: 10.1016/j.wasman.2018.05.017
Crolla, A., Kinsley, C., and Pattey, E. (2013). “Land application of digestate” in The biogas handbook. eds. A. F. Wellinger, J. Murphy, and D. Baxter (Oxford: Woodhead Publishing), 302–325.
Czekała, W. (2021). Solid fraction of digestate from biogas plant as a material for pellets production. Energ 14:5034. doi: 10.3390/en14165034
Daniel-Gromke, J., Rensberg, N., Denysenko, V., Stinner, W., Schmalfuß, T., Scheftelowitz, M., et al. (2018). Current developments in production and utilization of biogas and biomethane in Germany. Chem. Ing. Tech. 90, 17–35. doi: 10.1002/cite.201700077
Dinuccio, E., Gioelli, F. S., Cuk, D., Rollè, L., and Balsari, P. (2013). The use of co-digested solid fraction as feedstock for biogas plants. J. Agric. Eng. 44, 153–159. doi: 10.4081/jae.2013.208
Drosg, B., Fuchs, W., Al Seadi, T., Madsen, M., and Linke, B. (2015). Nutrient recovery by biogas digestate processing. Dublin: IEA Bioenergy.
Ekstrand, E. M., Björn, A., Karlsson, A., Schnürer, A., Kanders, L., Yekta, S. S., et al. (2022). Identifying targets for increased biogas production through chemical and organic matter characterization of digestate from full-scale biogas plants: what remains and why? Biotechnol. Biofuels Bioprod. 15, 1–22. doi: 10.1186/s13068-022-02103-3
Fangueiro, D., Hjorth, M., and Gioelli, F. (2015). Acidification of animal slurry–a review. J. Environ. Manag. 149, 46–56. doi: 10.1016/j.jenvman.2014.10.001
Finzi, A., Guido, V., Riva, E., Ferrari, O., Quilez, D., Herrero, E., et al. (2021). Performance and sizing of filtration equipment to replace mineral fertilizer with digestate in drip and sprinkler fertigation. J. Clean. Prod. 317:128431. doi: 10.1016/j.jclepro.2021.128431
FLFM (Bekendtgørelse om anvendelse af affald til jordbrugsformål. BEK nr. (Gældende). (2018). Available at: https://www.retsinformation.dk/eli/lta/2018/1001
Garcia-Ruiz, R., Carranza-Gallego, G., Aguilera, E., De Molina, M. G., and Guzman, G. I. (2019). C and N mineralisation of straw of traditional and modern wheat varieties in soils of contrasting fertility. Nutr. Cycl. Agroecosyst. 113, 167–179. doi: 10.1007/s10705-019-09973-4
Gioelli, F., Dinuccio, E., and Balsari, P. (2011). Residual biogas potential from the storage tanks of non-separated digestate and digested liquid fraction. Bioresour. Technol. 102, 10248–10251. doi: 10.1016/j.biortech.2011.08.076
Guilayn, F., Jimenez, J., Rouez, M., Crest, M., and Patureau, D. (2019). Digestate mechanical separation: efficiency profiles based on anaerobic digestion feedstock and equipment choice. Bioresour. Technol. 274, 180–189. doi: 10.1016/j.biortech.2018.11.090
Hansen, M. N., Sommer, S. G., and Madsen, N. P. (2003). Reduction of ammonia emission by shallow slurry injection: injection efficiency and additional energy demand. J. Environ. Qual. 32, 1099–1104. doi: 10.2134/jeq2003.1099
Hattingh, W. H. J., Thiel, P. G., and Siebert, M. L. (1967). Determination of protein content of anaerobic digesting sludge. Wat. Res. 1, 185–189. doi: 10.1016/0043-1354(67)90008-5
Hjorth, M., Christensen, K. V., Christensen, M. L., and Sommer, S. G. (2011). “Solid–liquid separation of animal slurry in theory and practice” in Sustainable Agriculture Volume 2 eds. E. Hamelin, M. Navarrete, and P. Debaeke (Dordrecht: Springer), 953–986.
Jagadabhi, P. S., Lehtomäki, A., and Rintala, J. (2008). Co-digestion of grass silage and cow manure in a cstr by re-circulation of alkali treated solids of the digestate. Environ. Technol. 29, 1085–1093. doi: 10.1080/09593330802180385
Jensen, S. K. (2008). Improved Bligh and dyer extraction procedure. Lipid Technol. 20, 280–281. doi: 10.1002/lite.200800074
KEFM. (2023). Ny aftale understøtter produktion af dansk biogas. Available at: https://kefm.dk/aktuelt/nyheder/2023/apr/ny-aftale-understoetter-produktion-af-dansk-biogas#:~:text=juni%202021%20blev%20der%20aftalt,4%20procent%20som%20tidligere%20aftalt
Kovačić, Đ., Lončarić, Z., Jović, J., Samac, D., Popović, B., and Tišma, M. (2022). Digestate management and processing practices: a review. Appl. Sci. 12:9216. doi: 10.3390/app12189216
Kratzeisen, M., Starcevic, N., Martinov, M., Maurer, C., and Müller, J. (2010). Applicability of biogas digestate as solid fuel. Fuel 89, 2544–2548. doi: 10.1016/j.fuel.2010.02.008
Larsen, S. U., Ma, N., Nielsen, S. V., Ward, A. J., and Møller, H. B. (2023). The impact of water content and additives on ensiling and methane yield of cereal straw. Bioresour. Technol. Report. 24:101672. doi: 10.1016/j.biteb.2023.101672
Li, Y., Han, Y., Zhang, Y., Fang, Y., Li, S., Li, G., et al. (2020). Factors affecting gaseous emissions, maturity, and energy efficiency in composting of livestock manure digestate. Sci. Total Environ. 731:139157. doi: 10.1016/j.scitotenv.2020.139157
Lu, M., Shi, X., Feng, Q., Li, X., Lian, S., Zhang, M., et al. (2021). Effects of humic acid modified oyster shell addition on lignocellulose degradation and nitrogen transformation during digestate composting. Bioresour. Technol. 329:124834. doi: 10.1016/j.biortech.2021.124834
Machado, R. M. A., and Serralheiro, R. P. (2017). Soil salinity: effect on vegetable crop growth. Management practices to prevent and mitigate soil salinization. Horticulture 3:30. doi: 10.3390/horticulturae3020030
Maynaud, G., Druilhe, C., Daumoin, M., Jimenez, J., Patureau, D., Torrijos, M., et al. (2017). Characterisation of the biodegradability of post-treated digestates via the chemical accessibility and complexity of organic matter. Bioresour. Technol. 231, 65–74. doi: 10.1016/j.biortech.2017.01.057
Mazzini, S., Borgonovo, G., Scaglioni, L., Bedussi, F., D'imporzano, G., Tambone, F., et al. (2020). Phosphorus speciation during anaerobic digestion and subsequent solid/liquid separation. Sci. Total Environ. 734:139284. doi: 10.1016/j.scitotenv.2020.139284
Möller, K., and Müller, T. (2012). Effects of anaerobic digestion on digestate nutrient availability and crop growth: a review. Eng. Life Sci. 12, 242–257. doi: 10.1002/elsc.201100085
Møller, H. B., Sørensen, P., Olesen, J., Petersen, S. O., Nyord, T., and Sommer, S. G. (2022). Agricultural biogas production—climate and environmental impacts. Sustain. For. 14:1849. doi: 10.3390/su14031849
Monlau, F., Sambusiti, C., Ficara, E., Aboulkas, A., Barakat, A., and Carrère, H. (2015). New opportunities for agricultural digestate valorization: current situation and perspectives. Energy Environ. Sci. 8, 2600–2621. doi: 10.1039/C5EE01633A
Nyangau, J. O., Sørensen, P., and Møller, H. B. (2024). Effects of plasma treatment of digestates on pH, nitrification and nitrogen turnover during storage and after soil application. Environ. Technol. Innov. 34:103578. doi: 10.1016/j.eti.2024.103578
Orlando, M. Q., and Velazquez-Marti, B. (2020). Pretreatment of animal manure biomass to improve biogas production: a review. Energ 13:3573. doi: 10.3390/en13143573
Pedersen, J., and Hafner, S. D. (2023). Ammonia emissions after field application of anaerobically digested animal slurry: literature review and perspectives. Agric. Ecosyst. Environ. 357:108697. doi: 10.1016/j.agee.2023.108697
Pedersen, J., Hafner, S. D., and Adamsen, A. P. S. (2022). Effectiveness of mechanical separation for reducing ammonia loss from field-applied slurry: assessment through literature review and model calculations. J. Environ. Manag. 323:116196. doi: 10.1016/j.jenvman.2022.116196
Peng, W., Lü, F., Hao, L., Zhang, H., Shao, L., and He, P. (2020). Digestate management for high-solid anaerobic digestion of organic wastes: a review. Bioresour. Technol. 297:122485. doi: 10.1016/j.biortech.2019.122485
Petersen, S. O., and Sommer, S. G. (2011). Ammonia and nitrous oxide interactions: roles of manure organic matter management. Animal Feed Sci. Technol. 166-167, 503–513. doi: 10.1016/j.anifeedsci.2011.04.077
Romio, C., Kofoed, M. V. W., and Møller, H. B. (2021). Digestate post-treatment strategies for additional biogas recovery: a review. Sustain. For. 13:9295. doi: 10.3390/su13169295
Romio, C., Kofoed, M. V. W., and Moller, H. B. (2023). Exploring increased hydraulic retention time as a cost-efficient way of valorizing residual biogas potential. Bioresour. Technol. 387:129646. doi: 10.1016/j.biortech.2023.129646
Sambusiti, C., Monlau, F., Ficara, E., Musatti, A., Rollini, M., Barakat, A., et al. (2015). Comparison of various post-treatments for recovering methane from agricultural digestate. Fuel Process. Technol. 137, 359–365. doi: 10.1016/j.fuproc.2015.04.028
Schievano, A., Pognani, M., D’Imporzano, G., and Adani, F. (2008). Predicting anaerobic biogasification potential of ingestates and digestates of a full-scale biogas plant using chemical and biological parameters. Bioresour. Technol. 99, 8112–8117. doi: 10.1016/j.biortech.2008.03.030
Sommer, S. G., and Hutchings, N. J. (2001). Ammonia emission from field applied manure and its reduction. Eur. J. Agron. 15, 1–15. doi: 10.1016/S1161-0301(01)00112-5
Sommer, S. G., and Knudsen, L. (2021). Impact of Danish livestock and manure management regulations on nitrogen pollution, crop production, and economy. Front. Sustain. 2:658231. doi: 10.3389/frsus.2021.658231
Tambone, F., Orzi, V., D'Imporzano, G., and Adani, F. (2017). Solid and liquid fractionation of digestate: mass balance, chemical characterization, and agronomic and environmental value. Bioresour. Technol. 243, 1251–1256. doi: 10.1016/j.biortech.2017.07.130
Teglia, C., Tremier, A., and Martel, J. L. (2011). Characterization of solid digestates: part 1, review of existing indicators to assess solid digestates agricultural use. Waste Biomass Valoriz. 2, 43–58. doi: 10.1007/s12649-010-9051-5
Thomsen, I. K., Pedersen, A. R., Nyord, T., and Petersen, S. O. (2010). Effects of slurry pre-treatment and application technique on short-term N2O emissions as determined by a new non-linear approach. Agric. Ecosyst. Environ. 136, 227–235. doi: 10.1016/j.agee.2009.12.001
Thygesen, O., Sommer, S. G., Shin, S. G., and Triolo, J. M. (2014). Residual biochemical methane potential (BMP) of concentrated digestate from full-scale biogas plants. Fuel 132, 44–46. doi: 10.1016/j.fuel.2014.04.062
Triolo, J. M., Sommer, S. G., Møller, H. B., Weisbjerg, M. R., and Jiang, X. Y. (2011). A new algorithm to characterize biodegradability of biomass during anaerobic digestion: influence of lignin concentration on methane production potential. Bioresour. Technol. 102, 9395–9402. doi: 10.1016/j.biortech.2011.07.026
Van Soest, P. J., Robertson, J. B., and Lewis, B. A. (1991). Methods for dietary fiber, neutral detergent fiber, and nonstarch polysaccharides in relation to animal nutrition. J. Dairy Sci. 74, 3583–3597. doi: 10.3168/jds.S0022-0302(91)78551-2
Wood, J. D., Gordon, R. J., Wagner-Riddle, C., Dunfield, K. E., and Madani, A. (2012). Relationships between dairy slurry total solids, gas emissions, and surface crusts. J. Environ. Qual. 41, 694–704. doi: 10.2134/jeq2011.0333
Wu, B., Lin, R., Bose, A., Huerta, J. D., Kang, X., Deng, C., et al. (2023). Economic and environmental viability of biofuel production from organic wastes: a pathway towards competitive carbon neutrality. Energy 285:129322. doi: 10.1016/j.energy.2023.129322
Keywords: residual methane potential, calorific value, screw press, decanter centrifuge, straw
Citation: Romio C, Ward AJ and Møller HB (2024) Characterization and valorization of biogas digestate and derived organic fertilizer products from separation processes. Front. Sustain. Food Syst. 8:1415508. doi: 10.3389/fsufs.2024.1415508
Edited by:
Tom Misselbrook, Rothamsted Research, United KingdomReviewed by:
Arturo F. Chica, University of Cordoba, SpainElio Dinuccio, University of Turin, Italy
Copyright © 2024 Romio, Ward and Møller. This is an open-access article distributed under the terms of the Creative Commons Attribution License (CC BY). The use, distribution or reproduction in other forums is permitted, provided the original author(s) and the copyright owner(s) are credited and that the original publication in this journal is cited, in accordance with accepted academic practice. No use, distribution or reproduction is permitted which does not comply with these terms.
*Correspondence: Cristiane Romio, YW5lLnJvbWlvQGJjZS5hdS5kaw==