- 1Terrestrial Ecosystem Management Laboratory, Graduate School of Global Environmental Studies, Kyoto University, Kyoto, Japan
- 2International Rice Research Institute-South Asia Regional Center, Varanasi, Uttar Pradesh, India
- 3International Maize and Wheat Improvement Centre (CIMMYT), NASC Complex, New Delhi, India
- 4International Crops Research Institute for the Semi-arid Tropics, Hyderabad, Telangana, India
- 5Borlaug Institute of South Asia (BISA), International Maize and Wheat Improvement Center, Pusa, Bihar, India
The rice-wheat cropping system (RWCS) provides the world’s population with staple foods, and it is crucial to maintain global food demand and security. Food systems are a complex ecosystem and sustain many feedback mechanisms. Crop residue management is one of those feedback mechanisms that was assessed under conservation agriculture, and a decomposition study was analyzed for the rice-wheat cropping system using rice, wheat, and maize crop residue for decomposition rate and nutrients release under agricultural practices (zero till, raised beds). Different zero tillage techniques in Samastipur demonstrated an accelerated decomposition trend, which was especially noticeable in the straw from wheat and rice. At the same time, permanent bed systems showed a relatively larger residue mass, especially in the case of wheat and maize straw. Permanent bed systems (wheat-maize system), particularly those using wheat straw, held the largest amounts of residue mass when the total residue mass throughout the sites was taken into account. Samastipur showed higher nutrient release for all the rice residue in wheat, rice residue in maize, and wheat residue in rice except maize residue in rice as compared to the Karnal sites. Decomposition kinetics, modeled via a first-order exponential decay function, showed high correlations (R2: 0.941 to 0.996) across treatment methods. The research underscores the significant effect of agroecological factors on residue decomposition and nutrient release, irrespective of residue type, highlighting the importance of tailored residue management practices for enhanced nutrient cycling and sustainability. These findings contribute to the optimization of residue management strategies in RWCS, promoting sustainable agriculture practices in the face of climate change and increasing food security demands.
Highlights
• The study found zero-tillage in Samastipur accelerates wheat and rice straw decomposition, releasing more nutrient compared to Karnal, highlighting agroecological effects on residue breakdown.
• Samastipur showed 36% higher nitrogen, phosphorus, and potassium release from rice residues than Karnal, emphasizing conservation agriculture’s role in improving soil fertility.
• Highlighting the need for supportive policies, the research underscores conservation agriculture’s effectiveness in small landholding areas, recommending government assistance to ease farmers’ transition and promote sustainable practices.
• Conservation agriculture led to a 55% reduction in residue mass, demonstrating significance improvements in nutrient dynamics and residue management for sustainability.
• The study advocates for adaptive, region-specific conservation agriculture practices in the Indo-Gangetic Plains to address soil health, productivity and sustainability challenges.
1 Introduction
Amidst climate change and increasing uncertainty, maintaining global food security has become increasingly vital. The rice-wheat cropping system (RWCS) is central to this effort, as it significantly contributes to the supply of staple foods needed to support a rapidly increasing global population, despite the decline in agricultural land (Lalik et al., 2014; Banjara et al., 2021a). However, the combined impact of climate change and population growth intensifies the challenges, creating a more complex scenario for sustaining agricultural productivity and ensuring global food security. This has to be done in the same or even reduced crop area due to an increased load for shelter, production industries, railways-roadways, and other needs to maintain the grain production supply and other foods. Highly populous RWCS countries such as India, Pakistan, Bangladesh, and China are expected to bear more pressure. The RWCS is a highly efficient, long-established, and advocated food system and the world’s most technologically advanced system. Nearly 13.5 million hectares (MHA) of land are under agricultural activities throughout Asia, and 57% of this area alone is in South Asia (Ladha et al., 2009). Additionally, more than 85% of the RW cropping in South Asia is being done in the nutrient-rich and highly fertile lands of Indo-Gangetic and Bramhaputra Plains (IGP; Banjara et al., 2021b; Gathala et al., 2011). In India, rice-wheat cropping systems are predominantly established and occupy a 9.2 mha area, contributing significantly to the country’s food security and economy (Jat H. S. et al., 2020) and supports more than 80% of nation’s total cereal production and about 50% of the calories consumed (Bijay-Singh et al., 2008). The RW cropping in the IGP of northwestern India is largely mechanized, and more than 90% of both cereals are harvested by following the residue management in the field (Bijay-Singh et al., 2008; Mishra et al., 2022). Through RWCS, on average, 55 million tons of crop residue are produced per year, of which more than 22 million tons of agricultural residue are contributed by rice only (Gupta et al., 2020) which has become the main threat for the long-term sustainability of RWCS in north-western India (Sharma and Dhaliwal, 2021). Managing rice straw has always been difficult for the farmers and local authorities. However, the wheat straw produced is taken out of the fields after they have been harvested and used as animal feed. Further, due to the poor economic worth of the rice straw and other problems including a labor shortage (Chaudhary et al., 2019), interference with the field preparation and seeding of succeeding crops, and the limited window period for managing rice straw before the sowing of wheat, more than 80% of rice straw is burned in the field (Singh and Sidhu, 2014; Jat M. L. et al., 2020; Babu et al., 2023).
Surface retention of rice residue utilizing the Happy Seeder technology is also one of the interventions for in-situ rice residue management that farmers in north-western India frequently use for increasing soil organic matter and nutrient supplying capacity (Singh et al., 2020a). Another practice employed is incorporating rice residue in the soil after rotavator or mold board plowing. Residue incorporation has been recommended 15–20 days prior to wheat seeding to improve soil health and crop yield (Bijay-Singh et al., 2008). Incorporation, however, requires multiple tillage operations, which increases the cost of cultivation and can delay the sowing of wheat due to the short window period. The area of zero-tilled wheat after complete or partial burning of rice residues in the IGP has expanded exponentially since the late 1990s because of large cost savings through reduced use of fuel and labor (Gupta and Sayre, 2007). However, direct drilling of wheat into rice residues was not possible due to (1) impediments to cultivation in the loose residue and (2) non-uniform sowing depth resulting from frequent lifting of the drill to clear blockages.
Compared with incorporation, mulched rice residue is less likely to result in N immobilization and can also provide non-N benefits such as conservation of soil, water, and weed suppression. Nutrient release is the first process in a series of reactions that transforms residue organic forms into soil mineral forms. The rates of decomposition and nutrient release are influenced by environmental factors (i.e., air temperature, precipitation, and soil characteristics) and the biochemical composition of the residue (Kaleeem Abbasi et al., 2015; Khalil et al., 2005; Yadvinder-Singh et al., 2010). Rice residues are rich in recalcitrant components such as silica (12–16%) and lignin (6–7%), which slow down their decomposition and cause temporary nitrogen immobilization when incorporated into the soil. This immobilization can lead to nitrogen deficiency in the following wheat crop (Yadvinder-Singh et al., 2010). The immobilization phase typically lasts for several weeks, during which nitrogen is locked up, limiting its availability to plants. However, after this period, the process shifts to nitrogen mineralization, eventually releasing nitrogen back into the soil for plant uptake (Yadvinder-Singh et al., 2010). This delayed release of nitrogen is critical for understanding nutrient management in rice-wheat cropping systems. Soil microenvironments for biological and chemical processes differ in surface placement than in incorporated residues, thereby influencing the nature and extent of organic matter dynamics and nutrient cycling (Beare, 1997; Cookson et al., 1998). Gupta and Ladha, 2010; Elrys et al., 2021; used degree-days (DGD) to account for the effect of temperature and to predict C and N mineralization. The advantage of this approach is that it allows comparisons of field and laboratory experiments done at different locations and years. In addition, because cumulative DGD is commonly used to predict crop development, it is a good tool to match soil and crop processes and analyze the synchrony between residue N release and crop N uptake. Although the effects of placement on the decomposition of different residues other than rice are known, information is lacking in the rice-wheat system, the world’s most important cropping system. There is a need to study decomposition and nutrient dynamics as a function of DGD in different rice-wheat soils. This information would help in developing an accurate composition of integrated nutrient management. Therefore, the present study hypothesizes that there is some positive impact of decomposition and placement of residue on NPK nutrient dynamics of soil. So, the objective of the study was to (1) predict the decomposition and release of N, P, and K from rice and wheat residue and (2) analyze the effects of placement and soil type on residue decomposition and nutrient dynamics over a rice-wheat cropping cycle.
2 Materials and methods
An experiment was conducted for two years (2016–17 and 2017–18) at two sites of IGP, i.e., Taraori, Karnal, India (Lat. 29°48’ N and Long. 76°55′ E) and BISA farm, Samastipur, India (Lat. 25°57’ N and Long. 85°40′ E) in an ongoing field experiment (Figure 1). In both the sites, the trial was initiated in 2012 (June in Taraori, Karnal and November in BISA farm, Samastipur) on well level (0–1% slope) having loam (46.7% sand, 34.4% silt and 18.9% clay) and silty loam (49.6% sand, 44.9% silt and 5.48% clay) soil texture, respectively. The Karnal soil to a depth of 0–11 cm was slightly alkaline (pH 8.02) with electrical conductivity (EC) of 13.1 mSm−1, bulk density of 1.56 Mg m−3, 9.14 cmolc kg−1 cation exchange capacity (CEC), 5.87 g kg−1 soil organic carbon and 5.30 g kg−1 soil inorganic carbon. Likewise, Samastipur soil for a depth of 0–12 cm was also slightly alkaline with pH 8.27, EC 12.9 mSm−1, bulk density of 1.68 Mg m−3, 11.2 cmolc kg−1 cation exchange capacity (CEC), 7.86 g kg−1 organic carbon and 28.5 g kg−1 soil inorganic carbon. In Karnal, the climate is semiarid with hot, dry to wet summers (May–October) and cool, dry winters (November–April) having 24°C average annual temperature and 700 mm mean annual rainfall of which 50–90% is received normally during southwest monsoon (July to September) (Figure 2). Whereas, in Samastipur, the climate is characterized by semi-arid to sub-tropical with hot and humid summers and cold winters with average annual temperature and rainfall of 25.5°C and 1,245 mm, respectively 50–70% of which is received between July to September (Table 1).
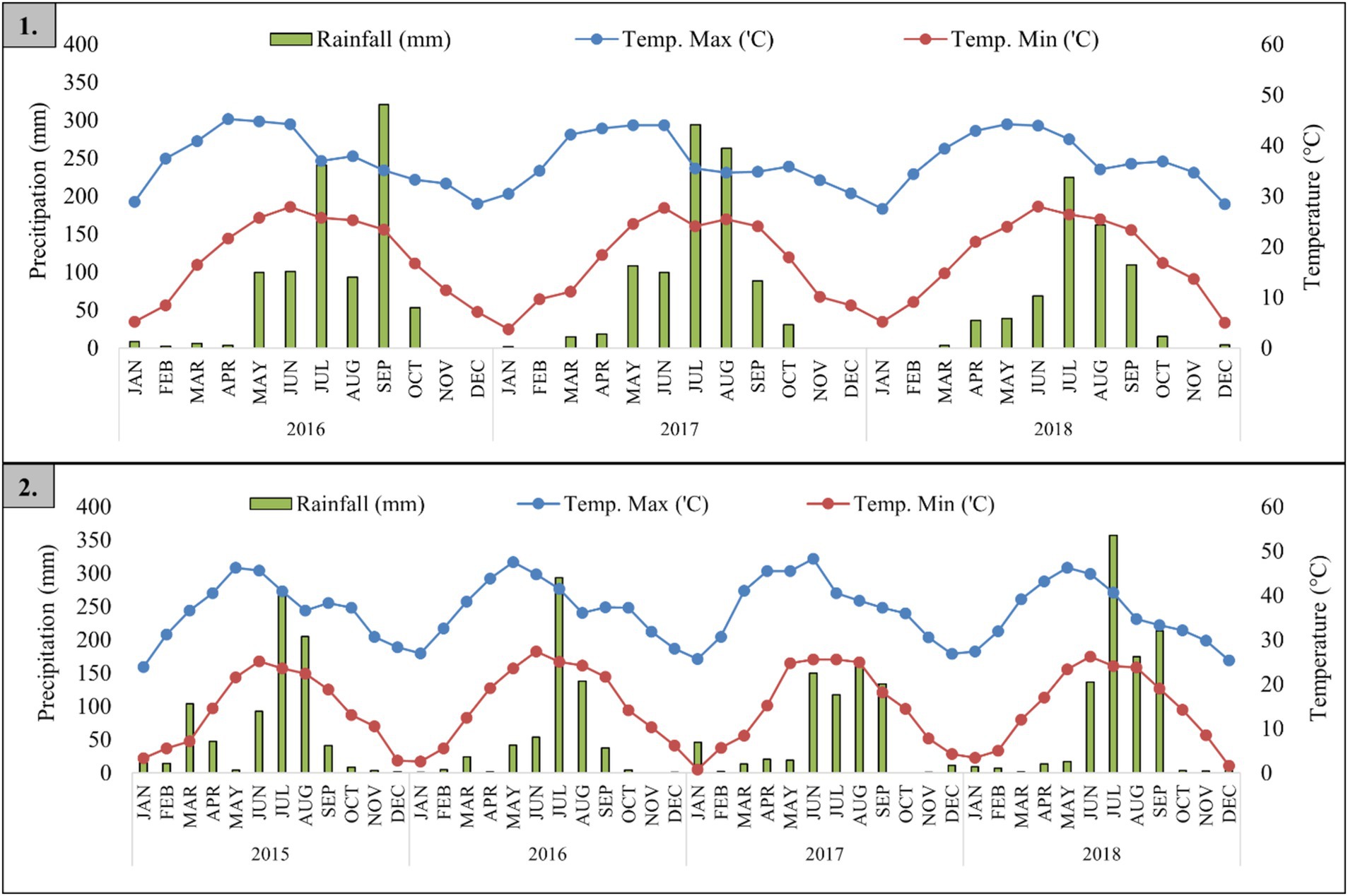
Figure 2. Under the experiment period, the average maximum and minimum temperature with rainfall (mm) of the experimental sites (1-Bisa, 2-Karnal).
2.1 Crop establishment
The experiment was conducted in summer (June in Taraori, Karnal) and winter (November in BISA farm, Samastipur) 2016 and 2017 respectively, after the harvest of rice and wheat as the first crop. After harvesting rice from Karnal and wheat from BISA farm, respective residues were removed. Rice, Maize and Wheat residue were applied at the rate of 6 t ha−1, 10 t ha−1 and 3 t ha−1, respectively, at Karnal while in Samastipur rice, maize and wheat residues were applied to the field at the rate of 6 t ha−1, 8 t ha−1 and 1 t ha−1, respectively. The main reason for applying higher rates of maize and wheat resiude in Samastipur is mainly due to relatively higher rainfall and temperature to prevent nutrient leaching and higher residue application maintain soil organic carbon matter. Rice was sown between 15th to 30th June, 2016 while wheat was sown during 10th-15th November, 2016 after harvesting wheat and rice, respectively, in both the places. In both the locations, Rice plots fertilized with 120 kg N + 60 kg P + 40 kg K ha−1, Wheat plots fertilized with 120 kg N + 60 kg P + 40 kg K ha−1 and Maize plots fertilized with 150 kg N + 60 kg P + 40 kg K ha−1 under conservation tillage treatments. During the study period, rice and wheat received irrigation during the critical growth stages of the crop. Experimental plot size was 4 m wide by 10 m long. For rice, the initial two years used hybrid PHB-71, followed by the long-duration variety Rajendra Mashuri from 2008. Zero-till direct-seeded rice was planted at a seed rate of 25 kg/ha with 20 cm row spacing. For wheat, variety PBW-343 was used initially, followed by HD-2733. Zero-till wheat was planted at 100 kg/ha with 20 cm row spacing. Maize-wheat system on permanent beds were used. In permanent bed wheat, two rows (30 cm apart) were drilled at 75 kg/ha. Beds were reshaped during the wheat cycle, combining seeding and reshaping in one operation.
Weed management varied by treatment. Glyphosate was applied pre-planting in permanent beds and zero-tillage plots, while pre-emergence pendimethalin and post-emergence ethoxisulfuron and bispyribac sodium were used in rice. Wheat required post-emergence herbicides like 2,4-D, metsulfuron methyl, carfentrazone, fenoxaprop, sulfosulfuron, and clodinafop, depending on weed intensity.
Water management practices included 6–8 irrigations for rice and 3–4 irrigations for wheat, with applications adjusted according to rainfall. The study underscores the effectiveness of integrated management for improving productivity and resource efficiency in rice-wheat systems.
2.2 Residue decomposition and nutrient release
The decomposition kinetics of residues and the subsequent release of nitrogen (N), phosphorus (P), and potassium (K) was done using a nylon mesh bag method. Nylon mesh bags containing rice, wheat and maize residues were sampled 6 times (0, 30, 60, 90, 120 and 150 days in 2015–2016 and 2016–2017) in both the locations.
Fifteen grams of residue (cut into 1 cm size) containing total N 0.48%, total P 1.31%, total K 1.21%, total C 37.8% for Rice, total N 0.48%, total P 1.69%, total K 1.43%, total C 44.7% for Maize and total N 0.62%, total P 0.86%, total K 1.41%, total C 47.0% for Wheat was used for study under Karnal condition while residue having total N 0.61%, total P 1.41%, total K 1.34%, total C 26.8% for Rice, total N 0.56%, total P 1.53%, total K 1.44%, total C 42.3% for Maize and total N 0.34%, total P 0.91%, total K 1.44%, total C 38.4% for Wheat under Samastipur condition were placed in each nylon bag (10 × 15 cm, 1 mm mesh). So, regarding macronutrients, Maize has the highest percentage of total K and C, while Wheat has the highest percentage of total N. Likewise, the data suggests that Wheat has the highest percentage of cellulose (41–44%) while Maize has the highest percentage of lignin (56–57%) and polyphenol (5–6%) at both locations. Rice has the lowest lignin percentage (12–14%) among the three residues (Table 2). The C:N ratio, which is a measure of the availability of nitrogen in the residues, is highest in Wheat at both locations, indicating that Wheat residues may have lower nitrogen availability compared to residues from other crops. Six sealed nylon mesh bags were positioned on the soil surface, after wheat and rice sowing, respectively. To track their placement, each bag was marked with a nylon thread attached to a wooden stick. At each of the six sampling points (as indicated in Table 3), one nylon mesh bag was randomly retrieved from each plot. On each sampling date, the residue remaining in the bag was carefully extracted, lightly shaken over a 1 mm sieve to eliminate most of the soil, and then thoroughly rinsed with distilled water. Samples were then oven dried at 60°C for 48 h, followed by weighing and grinding them to pass through a < 1 mm sieve. Decomposition was assessed by measuring the loss in mass of the rice and wheat residue within each bag. Total nitrogen (N) content in the residues was determined using the Kjeldahl method, while for phosphorus (P) and potassium (K) determination, the residue underwent wet digestion with a mixture of HNO3, H2SO4, and HClO4 in a 10:4:1 ratio. Phosphorus content was measured colorimetrically through the molybdate yellow color method using a spectrophotometer, following the method, and potassium content was determined using flame photometry as described. Total N, P, and K in the residue were calculated by multiplying the percentages of N, P, and K by the final weights at each sampling period. Changes in N, P, and K content of the decomposing rice residue represented the amount that had either mineralized or immobilized during the study period.

Table 2. The initial biochemical composition of different plant residues was used in the study at Karnal and Samastipur.
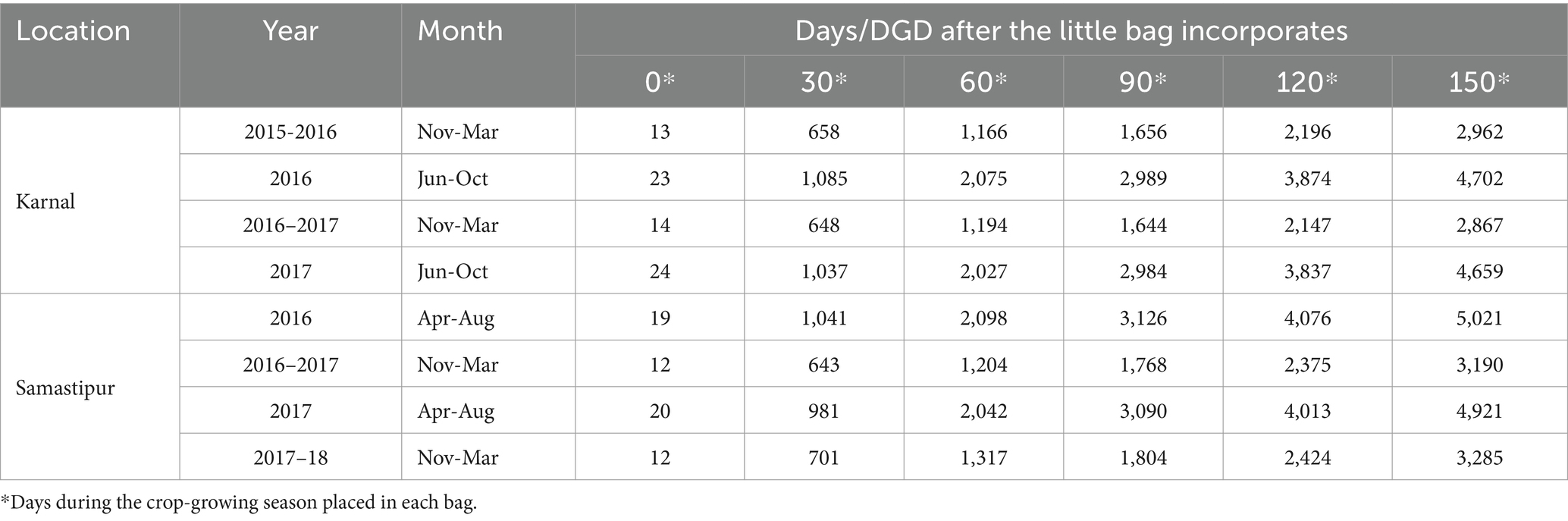
Table 3. Decomposition time (days) and equivalent degree days (DGD) were used for the 2 years of the study in different locations (Karnal and Samastipur).
2.3 Calculations and statistical analysis
Over a two-year period, data on residue decomposition (expressed as % mass remaining) and the concentrations of nitrogen (N), phosphorus (P), and potassium (K) in both locations were analyzed using a first-order exponential single-pool decay model.
2.3.1 Residue decomposition
The remaining residue at time t (Yt) is calculated using the first-order exponential decay model:
where:
Yt = % mass remaining at time t (in degree-days, DGD).
Y0 = % mass remaining at t = 0.
k = decomposition rate constant.
To account for variations over the two years, the data for N, P, and K concentrations were converted into relative concentrations (%). The effect of soil type on P changes was negligible, allowing for a combined analysis.
2.3.2 Degree-days (DGD)
DGD = (Average daily temperature) × (Number of days).
Nutrient release from rice, wheat, and maize residue was determined by solving the exponential mass loss functions (Figure 3) to determine the amount of residue remaining at a given time and exponential functions describing nutrient concentration changes with time (DGD) as affected by different crop establishment methods.
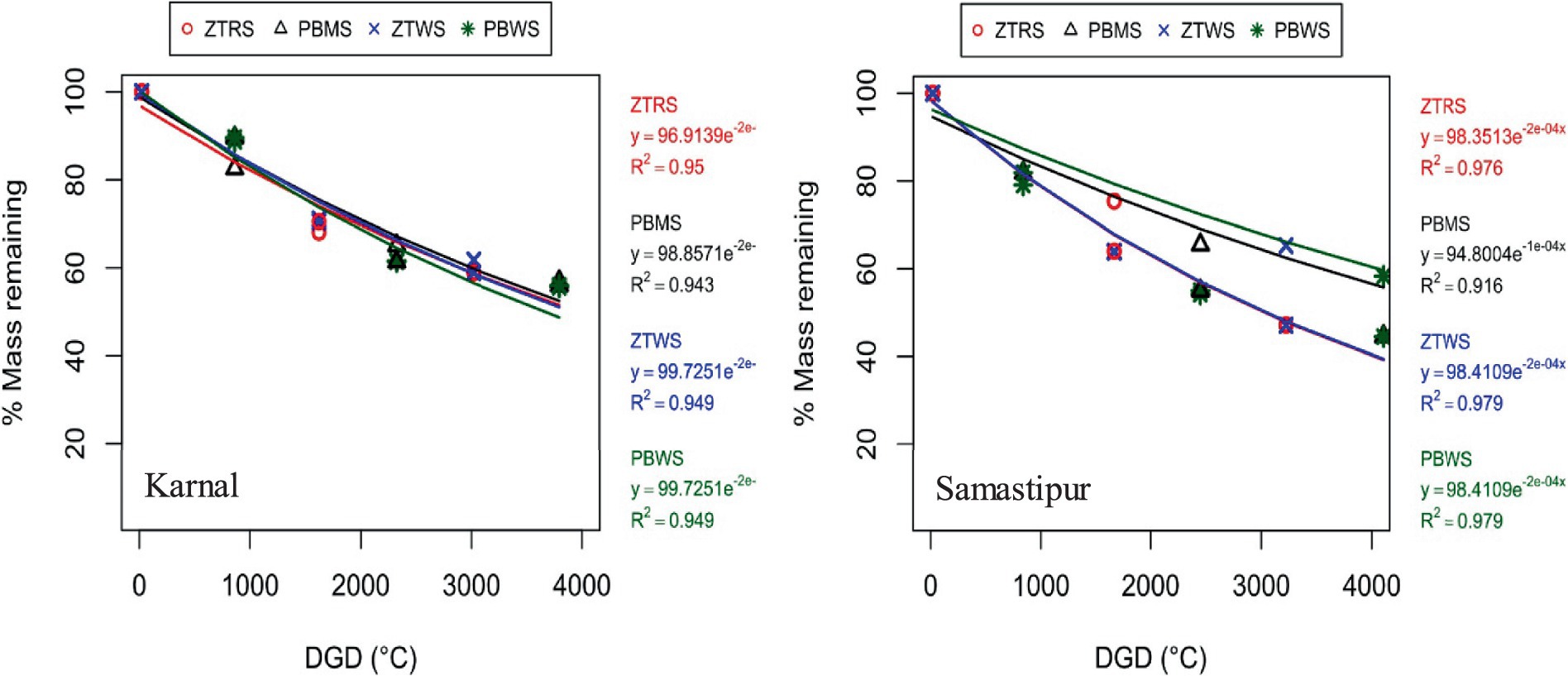
Figure 3. The mass remaining of different residues throughout the decomposition cycle as a function of degree-days (DGD) affected by crop establishment methods. (a) rice residue in wheat, (b) rice residue in maize, (c) maize residue in rice, and (d) wheat residue in rice.
2.3.3 Nutrient release
Nutrient release is calculated using exponential mass loss functions and nutrient concentration changes over time (DGD).
Initial nutrient content (kg ha−1) = % initial mean concentration × 8,000 (assuming an 8,000 Kg ha−1 load) (for N, P, and K, let these be Nt, Pt, and Kt respectively).
2.3.4 Total biomass remaining
Biomass remaining at any given time (Mg ha−1) = Initial mass × % mass remaining (denote as x).
2.3.5 Nutrient concentration
Nutrient concentration in residue after reconversion to actual concentration = a (%).
2.3.6 Total nutrient in residue
Total nutrient remaining (kg ha−1) = a × 1,000 × x.
2.3.7 Nutrient released
Nutrient released (kg) = Initial nutrient content − Nutrient remaining.
For nitrogen: N released (kg) = Nt − (a × x).
2.3.8 N released (% of initial N)
N released (%) = (Nt − (a × x))/Nt.
Similar calculations were carried out for P and K releases. Nutrient release was considered in three growth stages (maximum tillering, boot stage and maturity) of rice, wheat and maize, respectively. The decomposition (% mass remaining), % N, % P, and % K data were collected using a split-plot design, with the crop establishment method serving as the main plot and time as the sub-plot treatment. Statistical analysis was done using variance analysis (ANOVA) and the general linear model in SPSS version 26. To evaluate the significant differences among treatment means, least significant difference tests (LSD) were employed at a significance level of p < 0.05.
3 Results and discussion
3.1 Crop residue decomposition
The changes in residue mass over the decomposition cycle, influenced by crop establishment methods and locations, revealed intriguing patterns, as shown in Figure 3. Initially, at Karnal, residue mass retention across placement techniques appeared uniform. However, in Samastipur, distinct zero tillage methods showed an accelerated decomposition trend, particularly in wheat and rice straw. In contrast, permanent bed systems retained comparatively higher residue mass, notably with wheat and maize straw. Initial carbon degradation displayed swift decline that was seen more pronounced in zero till wheat straw, followed by gradual decreases in zero till rice straw and permanent bed wheat straw. Further, nitrogen degradation kinetics was observed with rapid decomposition in both the practices, zero till rice straw and permanent bed maize straw, as compared to slower rate in zero till wheat straw. Potassium degradation exhibited rapid rates in zero till rice and wheat straw, while permanent bed wheat straw showed slower kinetics (Table 4). Phosphorus retention post-decomposition was showed varied trend. Permanent bed maize straw retaining the highest proportion of P and zero till rice straw exhibiting the lowest concentration. In case of Karnal, during initial sampling days (30 days), rice, wheat and maize residue decomposed were 17.5, 9 and 13%, respectively, of the initial amount. On the 60th sampling day in Karnal, the decomposition of wheat and maize was completed almost 3 times (32 and 32% of initial, respectively), while that of rice residue decomposition was 1.5 times the residue decomposed (28.8% of initial). The trend of decomposition of residues increased till the 150th sampling day. However, the increase in the decomposition of residues was not as fast as it had been 30 to 60 days before the sampling. On the 150th sampling day, the rice, wheat, and maize residues were decomposed to 43.8, 46, and 44.6% of initial, respectively. Almost half of the applied rice, wheat, and maize residues were decomposed within 150 days in the Karnal field. An increase in the residue decomposition with sampling days was also observed in Samastipur. During the earlier stage of sampling (30 days), the amount of rice, wheat, and maize residue decomposition was 17.5, 19, and 21% of the initial, respectively. Similarly, residue decomposition increased at an increasing rate till 150 sampling days (Table 5) in Samastipur as well. The amount of rice, wheat, and maize residue decomposed at 150 sampling days was 55, 48.7, and 41% of the initial amount, respectively. While comparing both the locations, the amount of rice and wheat residue decomposed in Samastipur was greater than in Karnal, while the amount of wheat residue that decomposed was higher in Karnal than at Samastipur (Figure 3).
The initial rapid decay phase likely corresponds to the breakdown of easily degradable components such as carbohydrates and proteins. Subsequent slower decomposition stages may stem from recalcitrant fractions less susceptible to microbial degradation. These observations align with previous studies employing similar exponential decay models, reflecting the consensus regarding crop residue decomposition dynamics. Additionally, application of crop residues coupled with minimum soil disturbance and inclusion of mungbean improved the formation of aggregates resulting in the dominance of macro aggregates compared to micro aggregates through validation of such changes requires long-term experiments. In CT systems, tillage causes breakage of aggregates that may expose the OM to microbial attack, leading to rapid decomposition (Jat et al., 2018). Findings of this study are supported by the values reported by previous studies.
Apart from safeguarding soil, residue also serves as a source of nutrients and carbon for the soil dynamics, supporting nutrient release patterns of nitrogen (N), phosphorus (P), and potassium (K) from rice, wheat and maize residue that was either placed through zero till or permanent bed, as shown in Table 4.
3.2 Nitrogen (N)
Residue type and its placement method strongly influenced N-release behavior during both districts’ decomposition cycles. In Karnal and Samastipur, the total N release from rice straw at the end of 30 days was 5.43 kg ha−1 and 5.97 kg ha-1, respectively. By 90 days, the N release from Karnal and Samastipur sites increased to 12 kg ha−1 and 14.9 kg ha−1, respectively (Figure 4; Table 5). On the 150th day, the total amount of N release from rice straw applied at Karnal and Samastipur was 13.4 kg ha−1 and 18.3 kg ha−1, respectively.
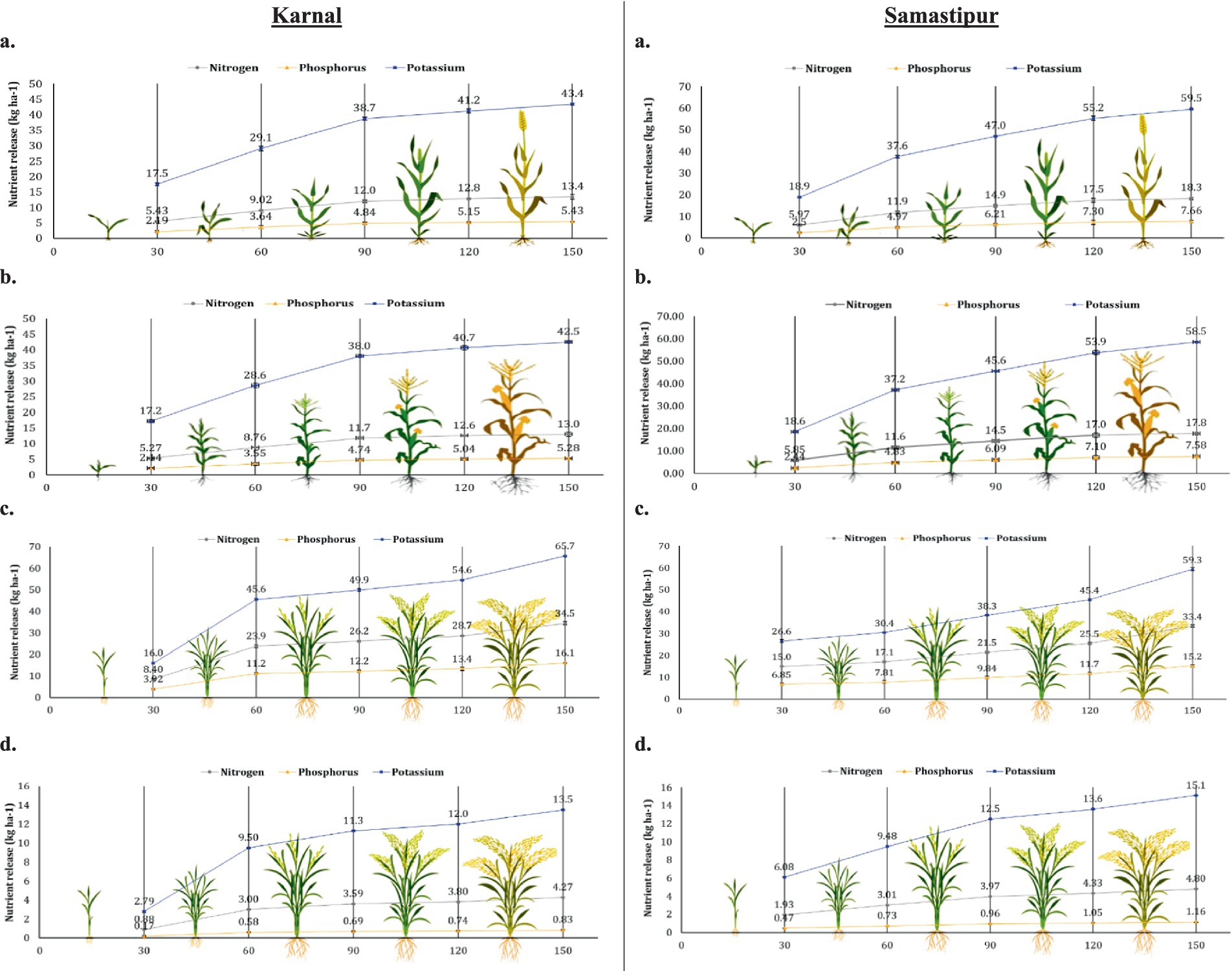
Figure 4. Variation in soil nutritional profile (NPK) in different crop residue and cropping system combinations under residue degradation study.
Similarly, the N release from the wheat and maize residues in the Karnal site (30 days) was 0.88 kg ha-1 and 8.40 kg ha-1, respectively, while the values for the Samastipur site were 1.93 kg ha−1 and 15 kg ha−1, respectively (Table 5). The amount of N released in 90 days increased to 3.59 kg ha−1 and 26.2 kg ha−1 from wheat and maize residues, respectively, for the Karnal, and 3.97 kg ha−1 and 21.5 kg ha−1 for wheat and maize residues for the Samastipur, respectively. By 150th days, residues of wheat and maize released 4.27 kg ha−1 and 34.5 kg ha−1 of N in Karnal, and 4.80 kg ha−1 and 33.4 kg ha−1 in wheat and maize residues, respectively at Samastipur site (Table 5).
Since N application after tillering and panicle initiation in rice, while in wheat after maximum tiller stage has less effect on the grain yield. The additional amount of N released after the boot stage may not be absorbed by growing rice or wheat and remains unutilized. Residue N release, however, was negligible during the initial (30 days) and could not supplement the crop’s nitrogen requirement initially.
Irrespective of the type or location of crop residue, a consistent increase in total nitrogen (N) release was observed as decomposition progressed, primarily due to residue bulk reduction. This phenomenon aligns with findings of prior studies, including Dubeux et al. (2006), Hamadi et al. (2000), Liu et al. (2011), and Ngwira et al. (2014), which reported enhanced N release over time from plant residues with varying carbon-to-nitrogen (C: N) ratios. An essential factor for the rise in N release was identified as the upsurge in carbon discharge, likely driven by increased microbial activity and its interaction with residue decomposition dynamics, as suggested by Gupta and Ladha (2010). This complex mechanism further underscores the significance of residue quality, its volume, and the timing of N release, which critically affects the residue’s utility as a nitrogen source in agriculture, backed by the findings of Clément et al. (1995) and Mishra et al. (2022). Despite the potential for N recycling through soil microbial biomass and the risk of N losses, the inherent N content in residues from crops like rice, wheat, and maize is insubstantial for significantly reducing N fertilizer requirements in the short term for these crops (Fagodiya et al., 2024). Optimizing the timing of residue incorporation and N fertilizer application appears vital for aligning residue decomposition with crop N uptake, necessitating further investigation to refine management practices for sustainable crop production without excessive N fertilizer dependence (Jat M. L. et al., 2020).
3.3 Phosphorus (P)
At the Karnal and Samastipur sites, phosphorus (P) released from rice straw reached 2.19 kg ha−1 and 2.50 kg ha−1, respectively, after 30 days. By 90 days, P release increased to 4.84 kg ha−1 in Karnal and 6.21 kg ha−1 in Samastipur. At 150 days, total P release was 5.43 kg ha−1 in Karnal and 7.66 kg ha−1 in Samastipur.
For wheat and maize residues in Karnal, P release was 0.17 kg ha−1 and 3.92 kg ha−1, respectively, after 30 days, while in Samastipur, it was 0.47 kg ha−1 and 6.85 kg ha−1. By 90 days, P release increased to 0.69 kg ha−1 and 12.2 kg ha−1 in Karnal, and 0.96 kg ha−1 and 9.84 kg ha−1 in Samastipur. At 150 days, P release from wheat and maize residues in Karnal reached 3.92 kg ha−1 and 16.1 kg ha−1, and in Samastipur, 1.16 kg ha−1 and 15.2 kg ha−1 (Table 5). Change in phosphorus trend can be visualized in the Figure 4.
Phosphorus release from rice and wheat straw was consistently higher in Samastipur compared to Karnal. For maize straw, initial P release was higher in Samastipur, but after 60 days, Karnal exhibited greater P release until the end of the study.
Phosphorus is crucial for plant cells, aiding in cell division and developing the plant’s growing tip. Its presence is essential for the growth of seedlings and young plants. However, the amount of nutrients released from the rice, wheat, and maize residues within 30 days was not noted as significant for maintaining the crop’s proper root growth and development. The release of P from rice, wheat, and maize residue increased with an increase in sampling days, but the amount released was not sufficient for the nutrition requirement of the existing crop. Similarly, McLaughlin et al. (1988) and Gupta and Ladha (2010) observed that while crop residue P may not notably benefit subsequent crops in the short term, it does contribute to the accumulation of organic P forms over time. This finding was also well documented by Fagodiya et al. (2024) in 15 years long term study. Recycling crop residues can enhance soil P availability for wheat and other crops (Jat M. L. et al., 2020). It has also been noticed that crop residue burning results in nutrients losses (Jat M. L. et al., 2020). So, a continuous CA practice can support the soil health and mitigate the negative impact of climate (Mishra et al., 2022; Fagodiya et al., 2024).
3.4 Potassium (K)
The dynamics of potassium (K) release from various crop residues were investigated for the Karnal and Samastipur regions. After 30 days, the release of K from rice straw was measured at 17.5 kg ha−1 in Karnal and 18.9 kg ha−1 in Samastipur. By the 90-day mark, these values increased to 29.1 kg ha−1 in Karnal and 37.6 kg ha−1 in Samastipur. After the study period (150 days), the total K release from rice straw in Karnal and Samastipur was recorded as 43.4 kg ha−1 and 55.2 kg ha−1, respectively.
By 90 days, N release increased to 11.3 kg ha−1 and 49.9 kg ha−1 for wheat and maize residues in Karnal, and 12.5 kg ha−1 and 38.3 kg ha−1 for wheat and maize residues in Samastipur. Ultimately, at the end of the 150 days, N release from wheat and maize residues was observed at 13.5 kg ha−1 and 65.7 kg ha−1 in Karnal, and 15.1 kg ha−1 and 59.3 kg ha−1 in Samastipur, respectively (Table 4). Ultimate differences in the potassium over time can be compared in Figure 4.
Potassium (K) release from rice and wheat straw was higher in Samastipur than in Karnal, with both sites showing an increasing trend in residue decomposition throughout the sampling period. For maize residue, initial decomposition was higher in Samastipur, but from 60 days onward, it was higher in Karnal. Potassium release was faster compared to other nutrients, likely due to its presence in the cell fluid as a non-structural component (Christensen, 1985), with similar findings by Rezig et al. (2014) and Mubarak et al. (2002). Unlike N and P, K release from rice, wheat, and maize residues was sufficient to support the growth of the succeeding crop. While conservation agriculture (CA) offers significant economic, agronomic, and environmental benefits in South Asia, including improved soil quality, the adoption of residue management remains slow (Sapkota et al., 2015; Fagodiya et al., 2024). Hence, adoption of the conservational practices (Jat et al., 2018), reusing crop residue (Devi et al., 2017) can be an energy saving and sustainable options under the existing circumstances for improving the soil quality and reducing the greenhouse gas emissions.
4 Conclusion
The study revealed that crop residue decomposition rates and nutrient release patterns differ significantly depending on the crop establishment method and location, particularly between Karnal and Samastipur. Zero tillage systems promoted faster residue decomposition, especially in rice and wheat straw, while slower rates were observed in permanent bed systems, notably in wheat and maize straw. Nitrogen release from residues initially occurred at a slower pace, accelerating later, particularly in maize residues, which proved insufficient to meet early crop nutrient demands. Phosphorus and potassium releases followed different patterns, with potassium being released more quickly and in amounts sufficient to support subsequent crop growth. These differences can largely be attributed to climatic factors, notably temperature variations modeled through degree-days. While crop residues contribute valuable nutrients and improve soil health, optimizing their timing and release for maximum benefit is crucial for sustainable agriculture. Continuous cropping systems, such as conservation agriculture, show potential for enhancing nutrient cycling and soil fertility but need further refinement in management practices. The findings reinforce the importance of residue management in sustainable farming and align with previous research on residue decomposition and nutrient dynamics. The future research should focus on optimizing residue management practices under conservation agriculture to enhance nutrient synchronization with crop uptake. This will help reduce fertilizer dependency, improve soil health, and promote sustainable agricultural practices in South Asia, considering site-specific decomposition dynamics and nutrient release pattern.
Data availability statement
The original contributions presented in the study are included in the article/supplementary material, further inquiries can be directed to the corresponding author.
Author contributions
AM: Investigation, Methodology, Writing – original draft, Writing – review & editing. HS: Supervision, Writing – original draft, Writing – review & editing. HJ: Writing – original draft, Writing – review & editing. MJ: Resources, Writing – original draft, Writing – review & editing. RJ: Resources, Writing – review & editing. SF: Conceptualization, Funding acquisition, Investigation, Methodology, Project administration, Resources, Supervision, Writing – original draft, Writing – review & editing.
Funding
The author(s) declare that financial support was received for the research, authorship, and/or publication of this article. This project work was support from Kyoto University, CGIAR Research Programs on Climate Change, Agriculture and Food Security (CCAFS), and Wheat Agri-Food Systems and Indian Council of Agricultural Research (ICAR).
Acknowledgments
We appreciate the MEXT Scholarship for providing funds for a Doctoral degree program for the first author at Kyoto University, Kyoto, Japan. The AM wishes to acknowledge the funding support from Prof. Shinya Funakawa, Dean, Graduate School of Global Environmental Studies, Kyoto University, Kyoto, Japan, and Kyoto University Foundation, Kyoto, Japan. We appreciate the support from the farmers, key communicators, and local officials who contributed their knowledge to this study and helped build rapport with the community. We also acknowledge the support of CIMMYT Academy and Borlaug Institute for South Asia (BISA) for providing an essential platform in various climate-smart villages of Haryana and Bihar, India and scientific contributions to the study. The authors also thank Deepak Bijarniya and Love K. Singh of CIMMYT India for their support while visiting the sites and collecting data.
Conflict of interest
The authors declare that the research was conducted in the absence of any commercial or financial relationships that could be construed as a potential conflict of interest.
Publisher’s note
All claims expressed in this article are solely those of the authors and do not necessarily represent those of their affiliated organizations, or those of the publisher, the editors and the reviewers. Any product that may be evaluated in this article, or claim that may be made by its manufacturer, is not guaranteed or endorsed by the publisher.
References
Babu, S., das, A., Singh, R., Mohapatra, K. P., Kumar, S., Rathore, S. S., et al. (2023). Designing an energy efficient, economically feasible, and environmentally robust integrated farming system model for sustainable food production in the Indian Himalayas. Sustain. Food Technol. 1, 126–142. doi: 10.1039/D2FB00016D
Banjara, T. R., Bohra, J. S., Kumar, S., Ram, A., and Pal, V. (2021a). Diversification of rice–wheat cropping system improves growth, productivity, and energetics of rice in the indo-Gangetic Plains of India. Agric. Res. 10, 1–10. doi: 10.1007/s40003-020-00533-9
Banjara, T. R., Bohra, J. S., Kumar, S., Singh, T., Shori, A., and Prajapat, K. (2021b). Sustainable alternative crop rotations to the irrigated rice-wheat cropping system of indo-Gangetic Plains of India. Arch. Agron. Soil Sci. 67, 1–18. doi: 10.1080/03650340.2021.1912324
Beare, M. H. (1997). “Fungal and bacterial pathways of organic matter decomposition and nitrogen mineralization in arable in arable soils” in Soil ecology in sustainable agricultural systems. eds. L. Brussard and R. Frrera-Cerrato (Boca Raton: Lewis Publishers), 37–70.
Bijay-Singh,, Shan, Y. H., Johnson-Beebout, S. E., Yadvinder-Singh,, Buresh, R. J., and Donald, L. S. (2008). Crop residue management for lowland rice-based cropping systems in Asia. Adv. Agron. 98, 117–199. doi: 10.1016/S0065-2113(08)00203-4
Chaudhary, A., Chhokar, R. S., Yadav, D. B., Sindhu, V. K., Ram, H., Rawal, S., et al. (2019). In-situ paddy straw management practices for higher resource use effciency and crop productivity in Indo-Gangetic Plains (IGP) of Indian. J. Cereal Res. 11, 172–198.
Christensen, B. T. (1985). Wheat and barley straw decomposition under field conditions: effect of soil type and plant cover on weight loss, nitrogen and potassium content. Soil Biol. Biochem. 17, 691–697. doi: 10.1016/0038-0717(85)90047-1
Clément, A., Chalifour, F. P., and Ladha, J. K. (1995). Crop residue effects on nitrogen mineralization, microbial biomass, and rice yield in submerged soils. Soil Sci. Soc. Am. J. 59, 1595–1603. doi: 10.2136/sssaj1995.03615995005900060013x
Cookson, W. R., Beare, M. H., and Wilson, P. E. (1998). Effects of prior crop residue management on microbial properties and crop residue decomposition. Appl. Soil Ecol. 7, 179–188. doi: 10.1016/S0929-1393(97)00032-2
Devi, S., Gupta, C., Jat, S. L., and Parmar, M. S. (2017). Crop residue recycling for economic and environmental sustainability: the case of India. Open Agric. 2, 486–494. doi: 10.1515/opag-2017-0053
Dubeux, J. C. B., Sollenberger, L. E., Interrante, S. M., Vendramini, J. M. B., and Stewart, R. L. (2006). Litter decomposition and mineralization in Bahia grass pastures managed at different intensities. Crop Sci. 46, 1305–1310. doi: 10.2135/cropsci2005.08-0263
Elrys, A. S., Ali, A., Zhang, H., Cheng, Y., Zhang, J., Cai, Z. C., et al. (2021). (2021) patterns and drivers of global gross nitrogen mineralization in soils. Glob. Chang. Biol. 27, 5950–5962. doi: 10.1111/gcb.15851
Fagodiya, R. K., Sharma, G., Verma, K., Rai, A. K., Prajapat, K., Singh, R., et al. (2024). Computation of soil quality index after fifteen years of long-term tillage and residue management experiment (LT&RE) under rice wheat system. Agric. Syst. 219:104039. doi: 10.1016/j.agsy.2024.104039
Gathala, M. K., Ladha, J. K., Kumar, V., Saharawat, Y. S., Kumar, V., Sharma, P. K., et al. (2011). Tillage and crop establishment affects sustainability of south Asian rice-wheat system. Agron. J. 103, 961–971. doi: 10.2134/agronj2010.0394
Gupta, R. K., Hussain, A., Yadvinder-Singh,, Sooch, S. S., Kang, J. S., Sharma, S., et al. (2020). Rice straw biochar improves soil fertility, growth, and yield of rice-wheat system on a sandy loam soil. Exp. Agric. 56, 118–131. doi: 10.1017/S0014479719000218
Gupta, R. K., and Ladha, J. K. (2010). Placement effects on rice residue decomposition and nutrient dynamics on two soil types during wheat cropping in rice–wheat system in North-Western India. Nutr. Cycl. Agroecosyst. 88, 471–480.
Gupta, R., and Sayre, K. (2007). Paper presented at international workshop on increasing wheat yield potential, CIMMYT, Obregon, Mexico, 20–24 March 2006 conservation agriculture in South Asia. J. Agric. Sci. 145, 207–214. doi: 10.1017/S0021859607006910
Hamadi, Z., Steinberger, Y., Kutiel, P., Lavee, H., and Barness, G. (2000). Decomposition of Avena sterilis litter under arid conditions. J. Arid Environ. 46, 281–293. doi: 10.1006/jare.2000.0672
Jat, M. L., Jat, H., Agarwal, T., Bijarniya, D., Kakraliya, S. K., Choudhary, K. M., et al. (2020). A compendium of key climate smart agriculture practices in intensive cereal based systems of South Asia. New Delhi: International Maize and Wheat Improvement Center (CIMMYT), 42.
Jat, H. S., Jat, R. D., Nanwal, R. K., Lohan, S. K., Yadav, A. K., Poonia, T., et al. (2020). Energy use efficiency of crop residue management for sustainable energy and agriculture conservation in NW India. Renew. Energy 155, 1372–1382. doi: 10.1016/j.renene.2020.04.046
Jat, A. L., Rathore, B. S., Desai, A. G., and Shah, S. K. (2018). Production potential, water productivity and economic feasibility of Indian mustard (Brassica juncea) under deficit and adequate irrigation scheduling with hydrogel. Indian J. Agric. Sci. 88, 212–215. doi: 10.56093/ijas.v88i2.79170
Kaleeem Abbasi, M., Mahmood Tahir, M., Sabir, N., and Khurshid, M. (2015). Impact of the addition of different plant residues on nitrogen mineralization–immobilization turnover and carbon content of a soil incubated under laboratory conditions. Solid Earth 6, 197–205. doi: 10.5194/se-6-197-2015
Khalil, M. I., Hossain, M. B., and Schmidhalter, U. (2005). Carbon and nitrogen mineralization in different upland soils of the subtropics treated with organic materials. Soil Biol. Biochem. 37, 1507–1518. doi: 10.1016/j.soilbio.2005.01.014
Ladha, J. K., Kumar, V., Alam, M. M., Sharma, S., Gathala, M. K., Chandna, P., et al. (2009). “Integrating crop and resource management technologies for enhanced productivity, profitability and sustainability of the rice–wheat system in South Asia” in Integrated crop and resource Management in the Rice– Wheat System of South Asia. eds. J. K. Ladha, Y. Singh, O. Erenstein, and B. Hardy (Los Banos: International Rice Research Institute), 69–108.
Lalik, R., Sharma, S., Idris, M., Singh, A. K., Singh, S. S., Bhatt, B. P., et al. (2014). Integration of conservation agriculture with best management practices for improving system performance of the rice–wheat rotation in the eastern indo-Gangetic Plains of India. Agric. Ecosyst. Environ. 195, 68–82. doi: 10.1016/j.agee.2014.06.001
Liu, K., Sollenberger, L. E., Silveira, M. L., Vendramini, J. M. B., and Newman, Y. C. (2011). Grazing intensity and nitrogen fertilization affect litter responses in ‘Tifton 85’ Bermudagrass pastures: II. Decomposition and nitrogen mineralization. Agron. J. 103, 163–168. doi: 10.2134/agronj2010.0320
McLaughlin, M. J., Alston, A. M., and Martin, J. K. (1988). Phosphorus cycling in wheat pasture rotations. I. The source of phosphorus taken up by wheat. Soil Res. 26, 323–331. doi: 10.1071/SR9880323
Mishra, A. K., Shinjo, H., Jat, H. S., Jat, M. L., Jat, R. K., Funakawa, S., et al. (2022). Farmers’ perspectives as determinants for adoption of conservation agriculture practices in indo-Gangetic Plains of India. Resour. Conserv. Recycl. Adv. 15:200105. doi: 10.1016/j.rcradv.2022.200105
Mubarak, A. R., Rosenani, A. B., Anuar, A. R., and Zauyah, S. (2002). Decomposition and nutrient release of maize Stover and groundnut haulm under tropical field conditions of Malaysia. Commun. Soil Sci. Plant Anal. 33, 609–622. doi: 10.1081/CSS-120002767
Ngwira, A., Johnsen, F. H., Aune, J. B., Mekuria, M., and Thierfelder, C. (2014). Adoption and extent of conservation agriculture practices among smallholder farmers in Malawi. J. Soil Water Conserv. 69, 107–119. doi: 10.2489/jswc.69.2.107
Rezig, F. A., Elhadi, E. A., and Abdalla, M. R. (2014). Decomposition and nutrient release pattern of wheat (Triticum aestivum) residues under different treatments in desert field conditions of Sudan. Int. J. Recycl. Org. Waste Agric. 3, 1–9. doi: 10.1007/s40093-014-0069-8
Sapkota, T. B., Jat, M. L., Aryal, J. P., Jat, R. K., and Khatri-Chhetri, A. (2015). Climate change adaptation, greenhouse gas mitigation and economic profitability of conservation agriculture: some examples from cereal systems of indo-Gangetic plains. J. Integr. Agric. 14, 1524–1533. doi: 10.1016/S2095-3119(15)61093-0
Sharma, S., and Dhaliwal, S. S. (2021). Conservation agriculture-based practices enhanced micronutrients transformation in earthworm cast soil under rice-wheat cropping system. Ecol. Eng. 163:106195. doi: 10.1016/j.ecoleng.2021.106195
Singh, Y., and Sidhu, H. S. (2014). Management of cereal crop residues for sustainable rice-wheat production system in the indo-Gangetic plains of India. Proc. Indian Natl. Sci. Acad. 80, 95–114. doi: 10.16943/ptinsa/2014/v80i1/55089
Singh, P., Singh, G., and Sodhi, G. S. (2020). Energy and carbon footprints of wheat establishment following different rice residue management strategies Vis-` a-Vis conventional tillage coupled with rice residue burning in North-Western India. Energy 200:117554. doi: 10.1016/j.energy.2020.117554
Yadvinder-Singh,, Gupta, R. K., Jagmohan-Singh,, Gurpreet-Singh,, Gobinder-Singh,, and Ladha, J. K. (2010). Placement effects on rice residue decomposition and nutrient dynamics on two soil types during wheat cropping in rice–wheat system in northwestern India. Nutr. Cycl. Agroecosyst. 88, 471–480. doi: 10.1007/s10705-010-9370-8
Keywords: crop residue, decomposition, resource conservation practices, residue recycling, permanent raised bed
Citation: Mishra AK, Shinjo H, Jat HS, Jat ML, Jat RK and Funakawa S (2024) Assessing the impact of rice-wheat-maize residue decomposition rate and nutrient dynamics of residue and soil using different placement method in the IGP of India. Front. Sustain. Food Syst. 8:1409370. doi: 10.3389/fsufs.2024.1409370
Edited by:
Roberto Valdivia, Oregon State University, United StatesReviewed by:
Subash Nataraja Pillai, Indian Agricultural Research Institute (ICAR), IndiaDakshina Murthy Kadiyala, Acharya N. G. Ranga Agricultural University, India
Copyright © 2024 Mishra, Shinjo, Jat, Jat, Jat and Funakawa. This is an open-access article distributed under the terms of the Creative Commons Attribution License (CC BY). The use, distribution or reproduction in other forums is permitted, provided the original author(s) and the copyright owner(s) are credited and that the original publication in this journal is cited, in accordance with accepted academic practice. No use, distribution or reproduction is permitted which does not comply with these terms.
*Correspondence: Ajay Kumar Mishra, YWttOGNlc3RAZ21haWwuY29t