- 1Department of Food Biotechnology and Food Process Engineering, Technische Universität Berlin, Berlin, Germany
- 2School of Business and Economics, Freie Universität Berlin, Berlin, Germany
Introduction: Cultivated meat has received growing attention since claims were made that cultivated meat can be produced more ethically and sustainably than the current meat production. However, there are still major challenges in the development of cell lines, scaffolding, growth media, and bioprocess, which need to be overcome to reach industrial production levels. Numerous technological innovations have been proposed to overcome these challenges but they have rarely been evaluated with regard to their social sustainability. Consequently, it remains unclear if and how cultivated meat would contribute towards creating inclusive food systems.
Methods: To bring more clarity, the study identifies different technological solutions that are used or developed for production of cultivated meat and identifies the positive and negative claims about the expected contributions of these technological solutions to social inclusion in food systems, using evidence from the literature review and 11 expert interviews. An innovation radar for cultivated meat is proposed to visualise the variety of technological innovations and the claims about their expected contributions to social sustainability.
Results: The technologies in the areas cell line development, scaffolding, growth media, and bioprocess are expected to have an impact on inclusion in consumption of cultivated meat. Some cell innovations are expected to raise cognitive barriers due to complex technologies that might be difficult for the consumer to understand. Cultural barriers are expected to be raised by cell innovation entailing genetic engineering and medium innovation using FBS or animal components, which is considered to harm animals. Further, regulatory barriers are expected in the EU if genetic modification is used in the production process, which concerns the areas cells, media, and scaffolding. The innovations for scaffolds are expected to mainly lower cost and cultural barriers since most technologies are already used in the food industry. Bioprocess innovations promise to lower cost barriers, however it must be considered, that most of the collected data for innovations in the bioprocess domain are based on assumptions.
Discussion: The study concludes that at this point in time, the most socially sustainable approach to cultivated meat production is not obvious. Under the current technological state of the art, it is not thinkable that production and consumption of cultivated meat could be socially inclusive. As it remains poorly understood if technologies for cultivated meat production could raise or lower barriers to inclusive consumption and production, further research is needed.
1 Introduction
The current agricultural and food systems are characterized by interlinkages of environmental, social and health problems (IPCC, 2023). Agricultural and food systems produce around 31% of human-caused greenhouse gasses, of which conventional meat production accounts for a large proportion. The livestock sector is the largest anthropogenic source of methane emissions and therefore has a high impact on climate change (Godfray et al., 2018; Scherer et al., 2023). Further, the production of meat is very inefficient due to a poor feed to food conversion rate. Thus, to produce 1 kg of boneless meat around 2.8 kg of feed is needed. Ruminants have a worse feed to food conversion rate and lead to the highest emissions per unit of energy produced compared to non-ruminant mammals and poultry (Mottet et al., 2017; Godfray et al., 2018; Scherer et al., 2023). 560 million ha of the arable land is used for livestock feed, which represents 40% of the world’s arable land (Mottet et al., 2017). Meat production acquires a third of the total fresh water used for agriculture (Hoekstra and Mekonnen, 2012; Godfray et al., 2018). In addition to the environmental issues, there are also food safety issues related to the meat production. Foodborne illnesses caused by Salmonella, Campylobacter, Listeria monocytogenes and E. Coli found in meat lead to consumer health problems (Sofos, 2008; Painter et al., 2013; Jairath et al., 2021). Additionally, it has been shown that the use of antibiotics in the livestock production contributes to antibiotic resistant bacteria (Mathew et al., 2007). However, the demand for meat is predicted to increase worldwide, driven by global wealth and population growth (Steinfeld, 2006; Godfray et al., 2018). Satisfying this increased demand will present a challenge, as it is predicted to rise by over 60%, representing 464 million tons, until 2050 (Revell, 2015; Jairath et al., 2021). Due to the above mentioned problems meat alternatives are needed (Ben-Arye and Levenberg, 2019). Many stakeholders call for a broad transition to more inclusive, healthier, climate-smart, and resilient food systems (United Nations, 2020; Food Systems Summit Dialogs, 2021). The meat alternatives present possible transition pathways. Nevertheless, the comprehension of how a transition to inclusive food system can be achieved is presently limited (Weckowska et al., 2022).
One alternative for conventional meat is cultivated meat, also known as “cultured meat,” “artificial meat,” “synthetic meat,” “laboratory meat” or “in vitro meat” (Smetana et al., 2023). The aim for cultivated meat is to replicate conventional meat in taste and texture by growing cells, such as adipose and muscle stem cells, in vitro (Scherer et al., 2023). This approach originates from regenerative tissue engineering and biotechnology (Enrione et al., 2017; Jairath et al., 2021; Scherer et al., 2023). Cultivated meat is produced through cell isolation from an animal’s biopsy or necropsy. These cells can then be expanded in bioreactors and manufactured into meat (Newton and Blaustein-Rejto, 2021; Seah et al., 2022). Cultivated meat promises to reduce the above-mentioned hazards (Enrione et al., 2017; Fernandes et al., 2020). Selling of cultivated meat is allowed in Singapore and the USA (Chodkowska et al., 2022).
It is still unclear how cultivated meat would contribute to creating socially inclusive food systems. Various approaches to production of cultivated meat are currently developed but their social, environmental, and economic impacts are yet to be fully explored. Some studies report environmental impacts (Tuomisto and de Mattos, 2011; Tuomisto et al., 2014; Smetana et al., 2015; Mattick et al., 2015a,b; Lynch and Pierrehumbert, 2019; Post et al., 2020; Tuomisto et al., 2022) and debate social impacts, but the evidence remains scattered and limited. There is a need to recognize that it is not enough to speak about impacts of cultivated meat as the impacts of different technological solutions for production of cultivated meat may vary. The understanding of impacts of innovative solutions is urgently needed to enable scientists, firms, investors, funders, and policy makers to dedicate their efforts toward supporting, developing, and diffusing solutions that have a potential to create inclusive systems for production and consumption of alternative proteins.
To address this gap, this study aims (1) to identify the technologies for production of cultivated meat, using a literature review focused on four areas: cell line development, growth media, scaffolding and bioprocessing and (2) to identify the criteria for assessing the potential impacts of identified technologies on social inclusion in food systems and (3) to provide an overview of the positive and negative claims made in the literature and by the interviewees about the potential of the identified technologies for production of cultivated meat to contribute toward creating inclusive food systems. The results of this study are derived from a review of primary and secondary sources, including scientific literature, Good Food Institute (GFI) databases and expert interviews (see Section 2). The results are presented in the format of an innovation radar for cultivated meat.
2 Materials and methods
2.1 Identifying impact categories
A set of the social impact categories was derived from the literature on social inclusion, social exclusion, and social cohesion (Percy-Smith, 2000; Berger-Schmitt, 2002; Labonté et al., 2011; Giambona and Vassallo, 2014; Miranti and Yu, 2015; Vrooman et al., 2015) before April 2022. Social exclusion and inclusion is a multidimensional concept as people can be excluded from “normal” participation in economic activity, political activities, social activities or from possession of individual qualities such as health (Percy-Smith, 2000) or from food. Moreover, it is important to consider who experiences the exclusion (Percy-Smith, 2000). In the context of agri-food transformations, two important groups of actors are food producers and consumers. Finally, we also specified the reasons for social exclusion - that is, various barriers that could hinder participation in consumption or production of cultivated meat. The above insights were captured in a multidimensional coding scheme (see Table 1), which was subsequently used in the analysis of the literature on cultivated meat and interview transcripts.
2.2 Identifying technologies
To identify different technological solutions that are used or developed for production of cultivated meat, the GFI database was reviewed and a literature review was conducted.
The Web of Science (WoS) was searched with the search string: “cultured meat*” OR “clean meat*” OR “lab-grown meat*” OR “lab grown meat*” OR “animal-free meat*” OR “animal free meat*” OR “in vitro meat*” OR “in-vitro meat*” OR “synthetic meat*” OR “cultivated meat*” OR “slaughter-free meat*” OR “slaughter free meat*” OR “cell-based meat*” OR “cell based meat*” OR “artificial meat*” OR “Frankenmeat*“OR “lab meat*” OR “craft meat*” OR “cruelty-free meat*” OR “cruelty free meat*” OR “shmeat*” OR “test tube meat*” OR “unnatural meat*” OR “cellular meat*” OR ((“meat* substitute*” OR “meat* alternative*” OR “meatless meat*” OR “synthetic meat*” OR “meat replace*” OR “imitation meat*” OR “simulated meat*” OR “meat analog* OR “meat analog* OR “meat-free meat*” OR “meat free meat*” OR “man-made meat*” OR “manmade meat*” OR “meat like meat*” OR” meat-like meat*” OR “mock meat*” OR “imitation meat*” OR “fake meat*” OR “faux meat*”) AND (cultured OR cultivated OR “in-vitro” OR “in vitro”)). The searches were conducted from April 2022 to May 2023. The search yielded 610 articles. The subset of 241 articles classified by WoS as ‘Food Science Technology’ was screened by a researcher with technical expertise to identify articles related to different technologies for production of cultivated meat. The search was updated for specific technological areas in January 2024. The articles referring to various approaches for production of cultured meat were downloaded for full text review.
Early in the review it became apparent that there are four broad technological areas in the production of cultivated meat, namely: growth media, cell line development, scaffolding, and bioprocess (Stephens et al., 2018; Bhat et al., 2019; Chen L. et al., 2022; Ye et al., 2022). These four categories were subsequently used for categorization of all technologies identified in the literature. In scientific articles, text passages describing a technological solution were identified and coded in an inductive way to capture the key characteristics of each technology. This process was executed in Citavi and resulted in a ‘knowledge structure’ of which a section is presented in Figure 1.
2.3 Identifying claims about the impacts of technological innovation: mixed methods
2.3.1 Literature review
To identify the positive and negative claims about the contributions of technological solutions to social inclusion in food systems, the selected literature in ‘Food Science Technology’ (see Section 2.2) was further analyzed by a researcher with technical expertise. The hybrid process of inductive and deductive thematic analysis (Fereday and Muir-Cochrane, 2006) was performed with assistance of Citavi. The deductive coding scheme (see Section 2.1) was used to sensitize the researcher to claims about social impacts. The emerging insights were regularly discussed with a social scientist to ensure the consistent use of the coding scheme. A few additional categories emerged from the data in an inductive way, related mostly to specific reasons for social exclusion. The review took place between April 2022 and May 2023. It identified some claims about social effects of technologies but also revealed technologies of which social impacts were not discussed in the literature.
As the above search strategy (see Section 2.2) was aiming at the maximum variety of technological solutions for production of cultivated meat, there was a possibility that some articles focused on impacts were overlooked. To address this shortcoming, another search strategy was performed in the Web of Science database. First, a search string which aimed to identify papers focused on inclusion was used: (“cultured meat” OR “cultivated meat” OR “cell-cultured meat” OR “in-vitro meat” AND inclus*). Only one document was identified. The search string was then broadened to social impacts: (“cultured meat” OR “cultivated meat” OR “cell-cultured meat” OR “in-vitro meat”) AND “social impact*.” Only two documents were identified. Subsequently, the search string was broadened to capture papers that address various impacts: (“cultured meat” OR “cultivated meat” OR “cell-cultured meat” OR “in-vitro meat”) AND ((social OR political OR environmental OR health OR economic) AND impact). The searches were executed between December 2022 and May 2023 and yielded 133 publications, which include the search terms in title, abstract or keywords. All abstracts were reviewed, and 98 articles were selected for a full text analysis. The papers were analyzed by researchers with technical or social science expertise, as appropriate. Most papers referred to environmental impacts and to cultivated meat in general, rather than to specific production technologies.
2.3.2 Expert interviews
Given the limited literature about social impacts of various technological solutions, 11 expert interviews were conducted between December 2022 and May 2023. Experts are believed to understand the “causal mechanisms” or to be the mechanics behind the “causal mechanisms” and hence expert interviews are suitable to gain insights into how “x and y interact” (von Soest, 2023). This provides insights into how technological choices and social impacts are related to each other.
Researchers and entrepreneurs with expertise relevant to production of cultivated meat were identified using the aforementioned secondary sources and were invited to an interview. The selected experts have either extensive expertise in this field or have expertise on technologies for which the social impacts could not be identified in the literature (see the interviewee list in Table 2). In advance of the interview, experts were provided with information about the project, the data management policy and an interview consent form. The interviews were conducted online by two researchers with expertise in social and technical sciences and lasted 1–2 h. One interview took place in person. Experts were asked how different technological solutions relate to food affordability, food acceptability, health, and social relations. The interview recordings were transcribed using an automatic transcription software and were verified manually. The anonymized interview transcripts were numbered I1 to I11, uploaded to Citavi, analyzed by a social scientist and consulted with the scientist with technical expertise. As in the case of literature analysis, the hybrid process of inductive and deductive thematic analysis (Fereday and Muir-Cochrane, 2006) was performed to analyze the interview transcripts and identify positive and negative claims about the contributions of technologies to social inclusion.
2.4 Visualization of results: innovation radar
To present the findings in an accessible way, they are visualized in form of an innovation radar. Innovation radars are used to generate information about a range of technologies and their impacts, typically with a focus on technologies relevant to a specific firm or industry (Rohrbeck et al., 2006; Golovatchev et al., 2010; Boe-Lillegraven and Monterde, 2015). Unlike commercial radars, the radar presented here is based on qualitative analysis of the secondary sources and interview data and it illustrates the positive and negative claims about the expected effects of various cultivated meat production technologies on social inclusion in food systems. Given the exploratory and qualitative nature of this study, it is possible that both positive and negative claims are identified for the same technology.
3 Results
The results are structured according to the four technological areas which are identified in the literature: cell line development, growth media, scaffolding, and bioprocess (Stephens et al., 2018; Bhat et al., 2019; Chen L. et al., 2022; Ye et al., 2022). Each area includes numerous technological solutions, which could have different social implications. The literature review revealed that there is very little evidence about the potential impacts of cultivated meat production on social inclusion. In the next sections the technologies identified in each area and the claims about their expected impacts, identified in the literature and by expert interviews, are presented. An overview of the four technological areas and their connected technologies is provided in Figure 2.
3.1 Cells
3.1.1 Overview of cells used in production of cultivated meat
Meat is composed of muscle fibers, connective, adipose, vascular and nervous tissue (Listrat et al., 2016). For cultivated meat, skeletal muscle cells, fat cells and cells of the connective tissue are considered (Post et al., 2020; Jara et al., 2023). Muscle cells make up the largest proportion and are rich in proteins. The fat cells serve as flavor carriers while the connective tissue cells contribute to the tenderness and texture (Weston et al., 2002; Li et al., 2020a; Jara et al., 2023). Additional requirements for cell selection are the capacity for replication and for the development into a specific cell type, also called differentiation (Post et al., 2020). The long-term capacity of replication allows a continuous production process which enables to lower the overall costs of cultivated meat production (Specht et al., 2018). The following paragraphs will therefore focus on different approaches to sourcing cells and cell innovations for production of cultivated meat.
3.1.1.1 Primary cell lines
Cells directly isolated from a biopsy and cultivated in vitro are referred to as primary cells. It is regarded as a cell line when it is further successfully subcultured (Schaeffer, 1990). Primary cells are usually not viable for a long period of time (Gstraunthaler and Lindl, 2013). Due to limited replication times recurring biopsies are needed (Pasitka et al., 2023). The development of a cell line can be divided into three phases. Phase one represents the primary cell culture. In phase two the maximum replication rate is reached, it is possible that spontaneous cell changes occur, which leads to a continuous cell line, which is indefinitely viable. If no spontaneous cell changes occur, the cell line enters phase three where the cell division decreases, and the cell culture dies (Gstraunthaler and Lindl, 2013).
3.1.1.2 Immortalized cells
To avoid recurring biopsies, primary cells need to be immortalized allowing indefinitely viability of the cells. Immortalization can be induced by inactivation proteins that regulate the cell cycle or excessive cell growth or by forcing the expression of another protein that maintains cell viability (Maqsood et al., 2013). Those procedures are based on genetic engineering (Gstraunthaler and Lindl, 2013).
3.1.1.3 Adult stem cells
Adult stem cells allow 30 to 50 divisions before they stop dividing (Roobrouck et al., 2008; Jara et al., 2023). Due to their limited proliferation capacity this would require frequent reseeding of the cells in the production process. The proliferation capacity can be influenced by the age of the donor animal wherefore the quality might vary dependent on the animal (Jara et al., 2023). In their natural environment they can differentiate into a certain tissue type and replace dead cells. An example for adult stem cells are myosatellite cells, which are muscle stem cells. Since 1 out of 106 cells in tissue is an adult stem cell, they are very rare. Furthermore, they are hard to identify and isolate. The variation in quality is dependent on the donor animal and requires more research to determine the ideal animal source (Jara et al., 2023).
3.1.1.4 Embryonic stem cells
Embryonic stem cells are sourced from early-stage in vitro cultivated blastocysts and allow unlimited expansion. ESCs can differentiate in different cell types and tissues. Stable ESCs have been recently successfully established for cows, pigs and chicken (Intarapat and Stern, 2013; Bogliotti et al., 2018; Pain et al., 2018; Choi et al., 2019; Post et al., 2020). ESCs are suitable for establishing a cell bank for cultured meat production purposes, since it is possible to use one vial of cells for several production batches. Zehorai et al. (2023) isolated bovine ESCs for cultivated meat production and showed that the cells could maintain their pluripotency and were genetically stable, which makes them suitable for cultivated meat.
Induced pluripotent stem cells (iPSCs) have an unlimited replicative capacity and are therefore considered for cultivation for cultured meat (Pasitka et al., 2023). Furthermore, they can differentiate into any cell type (Gstraunthaler and Lindl, 2013; Post et al., 2020). They are obtained by reprogramming of cells (Rackham et al., 2016). The advantage of iPSCs is that they can be obtained from differentiated cells. iPSCs exist for livestock species such as pig and cattle (Ezashi et al., 2009; Wu et al., 2009; Su et al., 2021). However, the production of iPSCs is expensive and the proliferation and differentiation of those cells need to be strictly monitored.
3.1.2 Expected impacts of cells used in production of cultivated meat
Technological solutions for sourcing and optimizing cells for cultivated meat production are expected to have various impacts, which with the use of the coding framework (see Table 1) can be categorized as positive or negative claims about the barriers to social inclusion. The overview of impact claims is provided in Table 3 and is displayed on the innovation radar (see Figure 3).
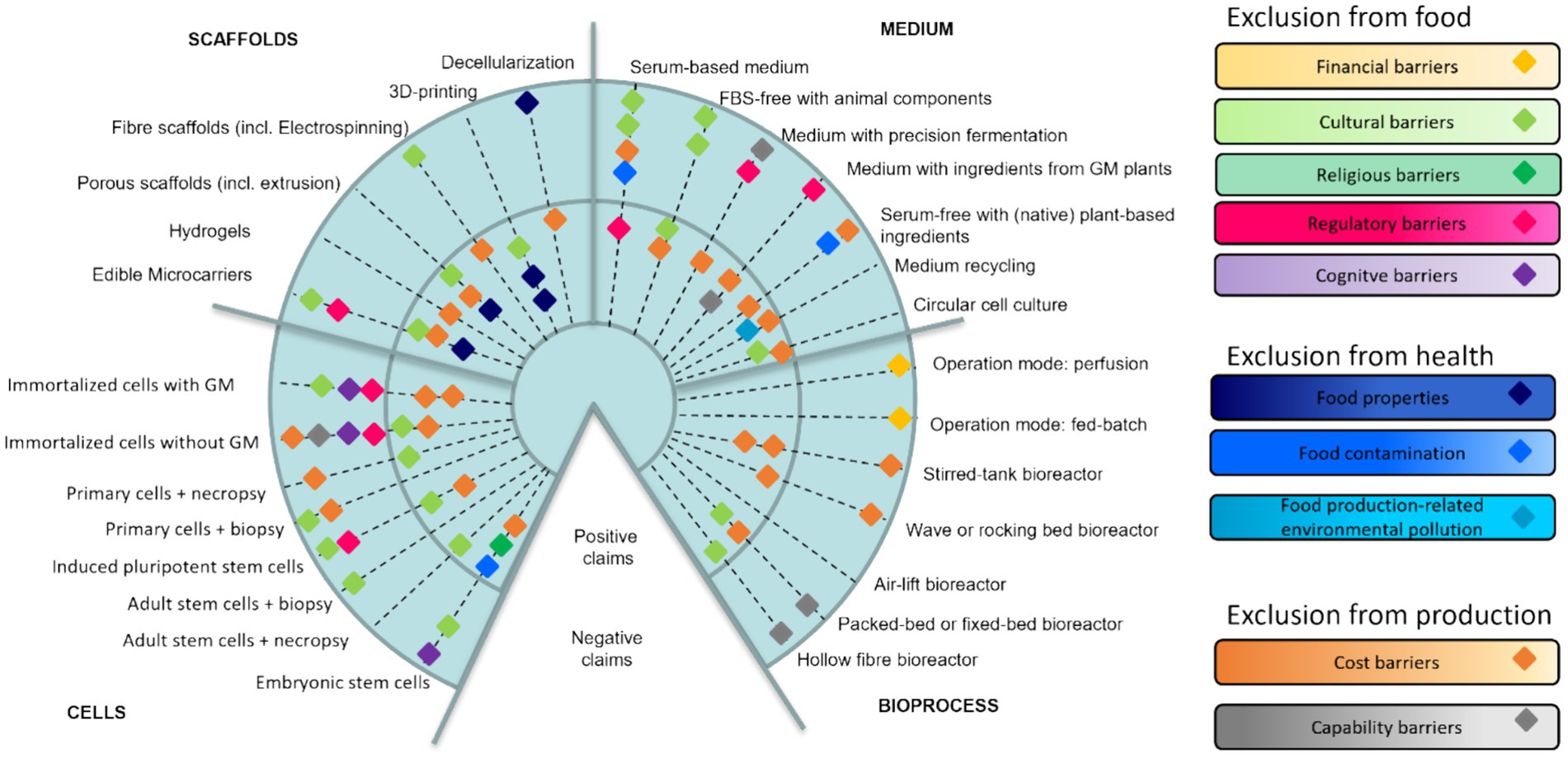
Figure 3. Innovation Radar for cultivated meat: production technologies and positive/negative claims about their contributions to inclusion in consumption and production.
Some people could be excluded from consumption when ESCs are used because they would find it difficult to understand the production (I4) (cognitive barrier is expected = negative claim) or consider it unethical (Zehorai et al., 2023) (cultural barrier is expected = negative claim). However, those following kosher diets would be included in consumption as ESCs are expected to be compatible with Jewish dietary laws (I4) (lack of religious barrier is expected = positive claim). Further, ESCs could improve inclusion in production as the cost barrier would be lowered given the reduced need for biopsies taken from animals and reduced costs for cell isolation and analytics. Positive claims about inclusion in health are exemplified by expected increases in food safety and security (Zehorai et al., 2023) (food contamination not expected = positive claim).
The use of adult stem cells harvested by biopsies could raise concerns about animal welfare (I2) - for some it would create a cultural barrier to consumption (negative claim). But biopsies could be replaced by necropsies (Gstraunthaler and Lindl, 2013), which could lower such cultural barriers (positive claim).
An alternative to stem cells are primary cells but as they require frequent biopsies, they are expected to raise not only concerns about animal welfare but also production costs (I4), that is cultural barriers to consumption and cost barriers to production (negative claims). Should the primary cells be harvested by necropsies, experts raise concerns about production costs and additionally see the need for new safety procedures to manage the risk of contamination (I3) (expected extra costs to production = negative claim). However, as mentioned above, necropsies allow cells isolation without hurting animals and could be positively perceived by consumers (I3) (no cultural barrier to consumption = positive claim).
Primary cells can be immortalized without genetic modification (GM), leading to an enhanced replication which lowers the production costs (Zehorai et al., 2023) (positive claim). Those cells can be used continuously without harming animals (I2) - they create no cultural barriers to consumption (positive claim). However, the immortalization process without GM is more expensive, longer, and less reliable compared to immortalization using GM (I2), resulting in increased production costs compared to immortalization with GM (negative claim about cost barrier). The techniques used for immortalization can be misunderstood or not allowed (Soice and Johnston, 2021) (negative claims about cognitive and regulatory barriers to consumption). Further, only a few firms in the world can immortalize cells without GM (I2) (expected capability barrier = negative claim).
Primary cells can also be immortalized through GM. The cells are affordable for producers and researchers (I2) and offer a good replication capacity further lowering the production costs (Zehorai et al., 2023) (positive claims about the cost barriers). However, the use of GM can be seen as unacceptable to consumers (I2, I4) (expected cultural barriers = negative claim) and the technologies used for immortalization can be misunderstood by them (Gaskell et al., 2000; Hubalek et al., 2022) (expected cognitive barriers = negative claim). It is further unclear if selling in the EU would be approved for cultured meat if GM is used (I2, I4) (expected regulatory barriers = negative claim).
iPSCs come without the need for frequent biopsies, have neither the donor-to-donor variability that primary cells suffer from (Hubalek et al., 2022), nor the ethical issues connected to embryonic stem cells (Rosselló et al., 2013). These are positive claims about the lack of cultural barriers to consumption and lower costs barriers to production. However, the regulatory approval and public acceptance for iPSCs cells is seen as uncertain, e.g., in the EU, as their stem cell-like properties are induced with GM methods (I3, I2, I4), (Gaskell et al., 2000; Soice and Johnston, 2021; Su et al., 2021). Such claims about cultural barriers and regulatory barriers are negative.
3.2 Scaffolding
3.2.1 Scaffolds used in cultivated meat production
The natural microenvironment of the cells is often mimicked by the scaffold. Its microstructure ensures the efficient transport of oxygen, nutrients, and waste products to and from the cells (O'Brien, 2011). The production techniques can be divided into bottom-up and top-down approaches. For bottom-up approaches smaller building blocks such as tubes, sheets, spheres or other microstructures seeded with cells are built together to reconstitute the meat cut. Whereas for top-down approaches, a prefabricated scaffold is used to seed cells, which grow inside the 3D-Structure and mature into tissue (Nichol and Khademhosseini, 2009; Bomkamp et al., 2022).
The following paragraphs will present the current scaffolding innovations. Until now, the main consideration was the structure and the scale up of scaffolds but social impacts have not been considered so far. Currently, six innovative approaches exist for scaffolding: hydrogels, microcarriers, porous scaffolds, fiber scaffolds, 3D-printing and decellularization (Bomkamp et al., 2022; Chen Y. P. et al., 2022).
3.2.1.1 Hydrogel
Hydrogels can be versatilely used as a soft scaffold, in or for microporous scaffolds or as a component of a bioink (Enrione et al., 2017; Ben-Arye and Levenberg, 2019; Simsa et al., 2019; Furuhashi et al., 2021; Bomkamp et al., 2022; Lee et al., 2022). Hydrogels mimic the natural environment of the cells in the tissue and are cell compatible. The cells must be provided with nutrients wherefore diffusion of nutrients throughout the hydrogel must be possible. During cell culturing the cells should degrade the hydrogel and produce their own extracellular matrix.
3.2.1.2 Microcarriers
Mass production of cells is mostly realized using microcarriers, which allow anchorage dependent cells to attach, grow and differentiate while being suspended in a bioreactor (Bomkamp et al., 2022; Zernov et al., 2022). For cultivated meat purposes microcarriers are used that are food grade, avoiding cost intensive detaching of the cells from the microcarrier post cultivation and increasing the cell yield (Bodiou et al., 2020; Zernov et al., 2022; Yen et al., 2023).
3.2.1.3 Porous scaffolds (includes extrusion)
Porous scaffolds remain in the final product and have a sponge-like structure. Interconnectivity of pores is beneficial for supplying nutrients and oxygen to the cells and removing waste products from the cell. Porous scaffolds offer mechanical stability to the cells and aim to mimic their natural environment (Bomkamp et al., 2022; Singh et al., 2023). One method to produce porous scaffolds is through extrusion. The process originates from the food industry and uses plant proteins and polysaccharides (Bomkamp et al., 2022).
3.2.1.4 Fiber scaffolds (electrospinning)
Fiber scaffolds are typically produced through electrospinning or rotary jet spinning but can also be cultivated by some fungi (Bomkamp et al., 2022). Food grade materials, such as collagen, gelatin, whey protein, chitosan, zein, cellulose, starch, soy isolate, egg albumen, and pullulan have shown to be suitable for electrospinning (Law et al., 2017; D'Odorico et al., 2018; Kumar, 2019). The scalability of electrospinning for the food industry is however limited due to low production rates (MacQueen et al., 2019; Levi et al., 2022). MacQueen et al. (2019) overcame this limitation by developing a fiber production called immersion rotary jet spinning (iRJS). This allowed to improve the production by two to four-fold. The porosity of gelatin spun scaffolds lay between 20 and 60%, which depends on the fiber composition, and ensures the transport of oxygen, nutrients and waste (Bomkamp et al., 2022).
3.2.1.5 3D-printing
3D-printing allows the fabrication of complex scaffolds by depositing ink layer-by-layer based on a 3D-model (Barbosa et al., 2023). Those 3D-models aim to mimic the natural environment of the cells (Ramesh et al., 2021). The most common method is 3D extrusion-based bioprinting. It is applicable to a broad field, low in cost and simple to handle. The cells are embedded in the ink used for printing, which further allows their exact deposition (Cui et al., 2020; Levi et al., 2022). The limitations of extrusion printing are slow printing times and low cell viability between 40 and 80% (Levi et al., 2022). The bioinks used for the process must fulfill requirements to be suitable for printing but also for cultivated meat production. The ink must flow during the printing process but hold its shape right after deposition to prevent spreading and obtain shape fidelity. Furthermore, the ink should contain food grade ingredients only (Schwab et al., 2020; Ianovici et al., 2022). A remaining challenge for 3D-printing is the scalability of the process for industrial production (Dong et al., 2023).
3.2.1.6 Decellularization
Decellularized scaffolds originate from tissue engineering. The production procedure consists of removing cells from a tissue, which preserves the natural surrounding structure of the cells including the extra cellular matrix. Furthermore, the vasculature is kept, which allows the perfusion of the scaffold. Tissue engineering uses predominantly organs or other tissues derived from animals or humans. For cultivated meat, plants and fungi are used to produce decellularized scaffolds. A wide variation of vegetables has been tested to produce decellularized scaffolds. Additional post processing of the scaffolds needs to occur to make it compatible for cell attachment and support cell growth (Levi et al., 2022; Singh et al., 2023).
3.2.2 Expected impacts of scaffolds used in production of cultivated meat
Literature was analyzed and interviews were conducted to understand if scaffolding technologies are expected to make the production and consumption of cultivated meat inclusive. The results are summarized in Table 4 and used for the creation of the innovation radar in Figure 3.
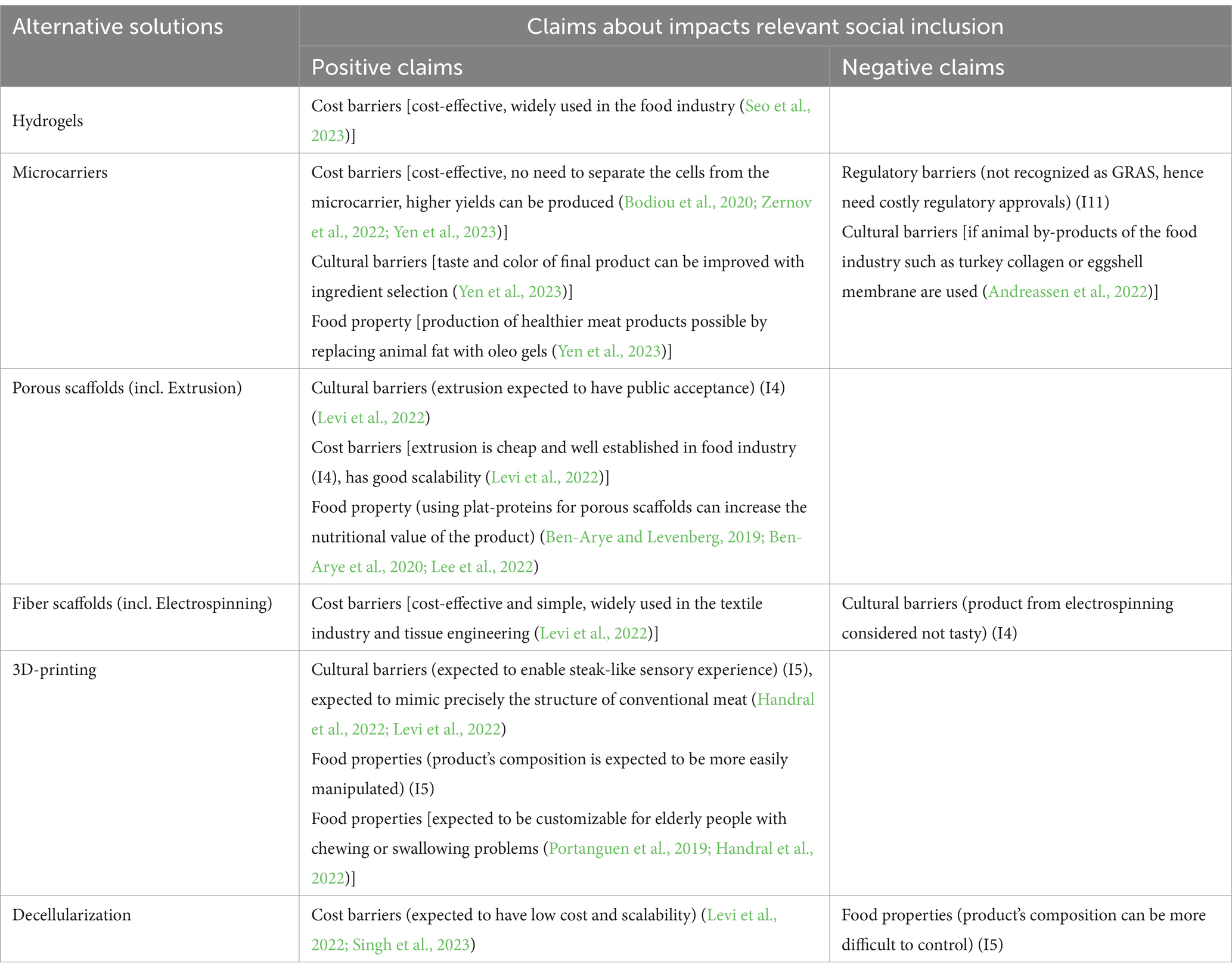
Table 4. Solutions for production of scaffoldings for cultivated meat and claims about their expected impacts.
Scaffolds can influence the organoleptic properties by creating structures that resemble the structure of conventional meat. Furthermore, scaffolds may have an impact on costs and on scalability of the process by enabling to suspend cells that need to attach to a surface in a bioreactor (Bomkamp et al., 2022).
Microcarriers that are food grade and remain in the final product, lower the production costs (positive claim), since the cells do not need to be separated from the scaffold, which results in higher cell yields (Bodiou et al., 2020; Zernov et al., 2022; Yen et al., 2023). It is possible to enhance the nutritional value of the final product when using microcarriers, for example, by encapsulating nutraceuticals (Yen et al., 2023) (enhancing food properties = positive claim). Additionally, the color of the final product can be influenced (Chen L. et al., 2022; Chen Y. P. et al., 2022; Yen et al., 2023) leading to greater consumer acceptance, (expected low cultural barriers = positive claim). Yen et al. (2023) incorporated oleo gels into microcarriers, which had similar properties to beef fat with enhanced nutritional values (enhanced food properties = a positive claim). Others produced microcarriers out of by-products of the food industry, such as turkey collagen and eggshell membrane (Andreassen et al., 2022). Those components are low in cost, have a high nutritional value and are biocompatible for the cells (enhanced food properties = positive claim). However, they are not vegetarian/vegan, thus there could be cultural barrier for some consumers (negative claim). One main advantage of microcarriers is that they are simple to control and monitor compared to the other scaffolds, which can result in enhanced quality, consistency and cost reduction (Moslemy et al., 2023) (expected lower production cost = positive claim).
When using hydrogels for scaffolding some of the ingredients, such as alginate, are already widely used in the food industry and therefore represent and affordable solution for cultivated meat producers (Seo et al., 2023) (expected no cost barriers = positive claim).
For porous scaffolds plant proteins represent a suitable scaffolding ingredient due to their affordability, high nutritional value, and compatibility with cells (Ben-Arye and Levenberg, 2019; Ben-Arye et al., 2020; Lee et al., 2022). These positive claims refer to low cost barriers for producers and food properties that are conducive to consumer health. Using extrusion to produce porous scaffolds allows for the use of side streams, for example from the oil industry, increasing the sustainability of the process (Ben-Arye et al., 2020). Even though further steps need to be taken to increase the functionality of extruded scaffolds, this technology represents a promising approach due to its scalability, affordability, and consumer acceptance (I4) (Levi et al., 2022). It is thus associated with reduction of cost barriers for producers and lowering of cultural barriers for consumers (positive claims).
Electrospinning is a cost-effective and simple process and already widely used in the textile industry and tissue engineering (Levi et al., 2022), (expected low cost barriers for producers = positive claim). However, products made by electro-spinning are not considered tasty (I4) and therefore the technology is associated with cultural barriers to consumption (negative claim).
3D-bioprinted cultivated meat could lead to more consumer acceptance due to the possibility to precisely mimic the structure of conventional meat (Handral et al., 2022; Levi et al., 2022) (expected low cultural barriers = positive claim). Furthermore, it could offer appealing food by bringing it into desired shapes for elderly people who might have chewing or swallowing problems (Portanguen et al., 2019; Handral et al., 2022) (food properties enhancing inclusion in consumption = positive claim). Including antioxidants as a scaffolding component during 3D-bioprinting allows to enhance the nutritional value of the final product (Dutta et al., 2022) (food properties conducive to consumer health = positive claim).
Decellularized scaffolds are low in cost, sustainable, contain the vascularization and have the potential to be scaled up in a bioreactor (Levi et al., 2022; Singh et al., 2023). These positive claims refer to lowering the cost barriers for producers. However, the composition of the scaffold might not be as easy to enhance compared to the other scaffolds. The nutritional value of the final product would be influenced directly by the growth of the cells (I5) (no possibility to enhance food properties = negative claim).
3.3 Media
3.3.1 Media used for scaffolds in cultivated meat production
Cell culture media is the major cost driver when considering large scale cultivated meat production (Post et al., 2020; Pajčin et al., 2022). It is estimated to make up to 99% of the total cultivated meat production cost (Stout et al., 2021; Yang et al., 2023). Therefore, it is important to ensure that a medium enables efficient proliferation and differentiation of cells while maintaining food safety of the final product (Guan et al., 2021). The media contains nutrients and oxygen for the cell culture and influences the characteristics of the final cultivated product (Post et al., 2020). Efficiency of the cell culture is impacted by the composition of the medium and each cell type has its unique requirements. Therefore, adjusting the medium based on the specific needs of the cell type is crucial. Finding appropriate concentrations of growth factors and proteins is essential to prevent inefficient nutrient uptake and excessive buildup of metabolic compounds (Hubalek et al., 2022).
3.3.1.1 Serum-based medium
The medium contains fetal bovine serum (FBS), which contains 200–400 different proteins along with thousands of small molecule metabolites in undefined concentration and is hence suitable for different cell types (Post et al., 2020). It supports cell attachment and proliferation (Gstraunthaler and Lindl, 2013).
3.3.1.2 Medium with plant components
There are ongoing efforts to replace the serum in the culture media. It has been shown that cell viability of muscle cells can be improved by using glucose and amino acids extracted from algae. Nevertheless, the use of FBS is still necessary to maintain and increase cell viability (Okamoto et al., 2020, 2022).
3.3.1.3 Chemically defined medium
In chemically defined media the composition of each component is clearly defined. It can be mixed with pacificated hormones and growth factors (Gstraunthaler and Lindl, 2013). Serum-free and chemically defined media for proliferation and differentiation of bovine and porcine muscle cells were recently developed (Kolkmann et al., 2022; Messmer et al., 2022; Guan et al., 2023). However, more research needs to be done for media optimization. The use of stochastic methods combined with mathematical networks was proposed to analyze and predict the effect of the components (Kolkmann et al., 2022).
3.3.1.4 Precision fermentation
FBS can be replaced by albumin, which is a protein present in blood plasma (Harris and Hoeger, 2020; Stout et al., 2021). Albumin can be recombinantly produced by (typically genetically engineered) bacteria, yeast, animal or plant cells. However, the production is very cost intensive and up to now not suitable for cultivated meat production (Stout et al., 2021; Hubalek et al., 2022). A price calculation of Hubalek et al., 2022 showed that the price for albumin would need to be reduced by factor 100. While primary cells need albumin for proliferation, iPSCs can proliferate without it (Hubalek et al., 2022). Stout et al. (2021) suggested a serum-free medium containing B8 medium based on glucose, amino acids, vitamins, salts, fatty acids and recombinant human albumin, which was expressed in rice. This medium showed to be effective for the expansion of primary bovine satellite cells and showed comparable short time growth rates of the cells as cells grown with 20% FBS. A passaging protocol further showed that the cells were able to retain their ability to form muscle tissue when using this medium. The estimated costs of this medium was between 46 and 74 $/L depending on the growth factor concentration, which is 75–85% cheaper than serum-containing media. However, the major cost driver of this medium remained the recombinant proteins, wherefore more research is needed to decrease the costs further (Stout et al., 2021).
3.3.1.5 Medium recycling
Metabolic waste produced by the cells during cell cultivation, such as ammonium and lactate have an impact on the cell productivity and growth. This makes it crucial to replace the medium frequently. Therefore, the medium is often exchanged due to metabolic build ups and not due to a lack in sufficient nutrient concentration, which has an impact on the cost effectiveness of the medium (Haraguchi and Shimizu, 2021; Haraguchi et al., 2022; Hubalek et al., 2022). Early exchange of the medium will also remove growth factors or proteins secreted by the cells that are needed for cell signaling. Therefore, the cells constantly need to replace those proteins which can additionally cause changes in the morphology or have an impact on the cell interaction (Hubalek et al., 2022). Further, the wasted medium contains nitrogen and phosphorus nutrients, which could lead to eutrophication of water bodies (Mattick et al., 2015b). Therefore, medium recycling is a crucial step for the sustainable production of cultured meat (Haraguchi and Shimizu, 2021). Different feeding regimes were tested on bovine adipose derived fat cells by exchanging 80, 65 or 50% of the medium. It was shown that 80% medium exchange led to the most economical result, since the cell growth decreased when exchanging less medium (Hanga et al., 2020). Hubalek et al. (2022) suggested three different strategies to either recycle the medium or extend its life span. One strategy would be to reduce the accumulation of metabolic waste in the medium as such or to dilute the metabolic waste. Another way would be to remove the metabolic waste directly by an ion exchange and revalorize this for example using ammonia as a plant fertilizer or reintroducing lactate later in the cultivation process to induce myogenic differentiation (Hubalek et al., 2022). By removing the harmful metabolic compounds, the valuable compounds left in the media could be reintroduced into the bioreactor and supplemented with substrates. Another strategy would be the circular use of conditioned medium by growing animal cells and microalgae simultaneously. This strategy was proposed by Haraguchi and Shimizu (2021) and will be explained in more detail in the following paragraph (Hubalek et al., 2022).
3.3.1.6 Circular cell culture
Haraguchi et al. (2022) suggested a method, where mammalian cells grow using microalgae culture waste medium. Once the nutrients are used by mammalian cells, the medium can be reused for microalgae, creating a circular usage pattern. The waste medium of the microalgae is supplemented with growth factor produced by liver cells. Amino acids and glucose are extracted from microalgae. During muscle cell cultivation, the glucose concentration in the medium decreased after two cycles while the total proteinogenic amino acid concentration remained almost stable. Growth factors obtained from the condition liver cells medium were added frequently to the medium, since inactivation of those could occur after multiple cycles. This system allows growth of muscle cells in a serum-free environment. Further, waste medium produced by muscle cells can be used as a culture media for microalgae. Ammonia produced by muscle cells during cell cultivation is a valuable component for microalgae to produce proteinogenic amino acids, which can then again be used for muscle cell cultivation. It was shown that microalgae effectively metabolized ammonia in the medium up to 90% without metabolizing glucose or other amino acids left in the medium. This shows that microalgae can be used to effectively reduce metabolic products produced by mammalian cells which would otherwise be harmful for mammalian cell culture. This system allows to decrease culture medium costs by eliminating the need for FBS in the medium and increasing the efficiency of the medium due to recycling of culture medium by microalgae. Furthermore, reusing waste medium of mammalian cell cultures will decrease the environmental impact and the resource energy use associated with medium production (Haraguchi et al., 2022).
3.3.2 Expected impacts of media used in production of cultivated meat
As for the other technological areas, literature was analyzed and interviews were conducted to understand if various approaches to production of cultured medium are expected to help make the production and consumption of cultivated meat inclusive. The results are summarized in Table 5 and further used for the creation of the radar in Figure 3.
The serum-based media using FBS are expected to have a high batch to batch variation and be more expensive than serum-free media (I11) (Gstraunthaler and Lindl, 2013; Post et al., 2020). These claims relate to production cost and are negative. Moreover, the serum-based media are expected to be seen by consumers as unethical (I4, I6, I11) (Gstraunthaler and Lindl, 2013; Post et al., 2020) and incompatible with vegetarian diets due to animal welfare concerns (I4, I5, I6) (expected cultural barriers to consumption = negative claims). Including FBS or other animal components in the medium poses a food contamination risk (Gstraunthaler and Lindl, 2013; Post et al., 2020) and large-scale decontamination process is yet to be developed (I3) (enhanced risk for consumer health = negative claim). However, it is expected to be easier to get regulatory approval for serum-based media compared to the other alternatives (I4) (expected low regulatory barriers = positive claim).
The expectations about media using animal components other than FBS, such as blood from slaughtered animals, are mixed. Some experts expect costs to be lower than in case of chemically defined medium and the acceptance to be high among meat eaters (I3) (lower cost for producers and low cultural barrier for consumers = positive claims). Others expect the same ethical concerns as for FBS-based medium would affect the general public acceptance but also stop vegans and vegetarians from consumption of cultivated meat (I3, I5) (expectation of cultural barriers to consumption = negative claims). These positive and negative claims about cultural barriers concern people with different eating habits.
Similarly, in the case of media with plant components, some experts expect high batch-to-batch variations (increased costs = negative claim) and possible exposure to high concentrations of pesticides or herbicides to pose problems (risk of food contamination = negative claim) (Su et al., 2021; Messmer et al., 2022). However, media with plant components are expected to be cheaper than chemically defined medium (I3) (lower costs barrier for producers = positive claim).
Should GM plants be used for production of medium, experts expect that crop farmers could participate in the emerging value chains for cultivated meat by doing what they already know for example producing crops but with higher added value (I2) (no capability barrier for producers = positive claim). The use of GM crops for medium production is also expected to allow scale-up and lower the production costs (I2) (lowering cost for producers = positive claim). However, one expert noted that regrettably it is unclear if sales of cultivated meat would be allowed in the EU when the GM crops are used in culture medium (I2) (risk of regulatory barriers to consumption = negative claim).
Medium with ingredients obtained by precision fermentation is expected to be 75–85% cheaper compared to serum-containing medium when produced at scale (Stout et al., 2021) (expected lower cost for producers = positive claim). However, the scale-up is currently not possible (I2) (Stout et al., 2021; Hubalek et al., 2022) (capability barrier for producers = negative claim). Moreover, it is unclear if sales of cultivated meat would be allowed in the EU when GM microorganisms are used to produce components of the culture medium used in the production of cultivated meat (I2, I4) (regulatory barriers to consumption = negative claim).
Medium recycling is expected to mitigate the problem of eutrophication of water bodies, which would arise if wasted media, containing nitrogen and phosphorus, is released into water (Mattick et al., 2015b) and could put public health at risk (risk of water pollution is reduced = positive claim). Furthermore, the recycling would lead to more effective use of the media lowering the production cost (Haraguchi and Shimizu, 2021; Haraguchi et al., 2022; Hubalek et al., 2022) (lower cost barrier for producers = positive claim). In circular cell culture the use of the medium would be more effective and the use of FBS not necessary (Haraguchi et al., 2022) further lowering cost barrier for producers and cultural barrier for consumers (positive claims).
3.4 Bioreactors
3.4.1 Bioreactors used in cultivated meat production
Bioreactors are needed to scale-up the production of cultivated meat, which represents further challenges. The scaling effects on animal muscle cells is not yet fully known and the use of different bioreactor types needs yet to be explored (Li et al., 2020b). Only a few proof of concept publications using microcarriers and/or spinner flasks for the cultivation of meat at a bigger scale exist (Verbruggen et al., 2018; Hanga et al., 2021; Zhang et al., 2021).
The choice of bioreactors depends on the cell type during proliferation and maturation phase, the scaffold and the product characteristics that need to be achieved (Specht et al., 2018; Allan et al., 2019). Even though the bioprocess design for cultivated meat can rely on the expertise of other industries, the cultivation of mammalian anchorage dependent cells continues to face difficulties for large scale production (Allan et al., 2019). The cells used for meat cultivation have lower shear limits compared to prokaryotes used in fermentation processes, wherefore the stirring and agitation rate in the process plays a crucial role to preserve the viability of the cells (Hu et al., 2011; Pajčin et al., 2022). Further the surface area to volume ratio of the scaffold used in the cultivation process has an impact on the size of the bioreactor. Additionally, the choice of the scaffold has an influence on the bioreactor seeding efficiency, the passaging requirements, downstream processing, the bioreactor fluid dynamics, the mass transfer, and the costs (Allan et al., 2019).
Operation modes to cultivate cells in a bioreactor are batch, fed-batch, or perfusion. The batch process allows no additional feeding throughout the process and is the simplest method. However, only limited control of the growth rate of the cells are possible. Fed-batch is the most frequently used operation mode, here the nutrients are added in a controlled manner to the bioprocess. It allows good control of cell growth and thus results in higher productivity (Meyer et al., 2017). The perfusion mode allows the cultivation of higher cell densities. Here the medium is continuously cycled through a porous structure containing attached cells. This allows to provide nutrients and oxygens to the cells and to discard metabolic compounds. The perfusion is limited by the cell growth in the structure (Humbird, 2021).
Cell proliferation and differentiation are the two key stages that need to be considered for bioprocess design (Pajčin et al., 2022; Roy et al., 2023). For proliferation purposes the cells can be grown on microcarriers in dynamically mixed bioreactors such as stirred-tank bioreactors, air-lift reactors or rocking bed reactors. Differentiation of cells is supported by static bioreactors including scaffolds such as packed-bed bioreactors or hollow fiber bioreactors (Zidarič et al., 2020). The conditions present in those bioreactors mimic the in vivo conditions of the cells and supports differentiation (Specht et al., 2018; Pajčin et al., 2022). Finally, the bioreactor choice influences the structure of the final product since some bioreactors support two-dimensional growth of cells, while others support three-dimensional growth of cells (Pajčin et al., 2022). Bioreactors for unstructured cultivated meat products are stirred-tank bioreactors, wave or rocking bed bioreactors and air-lift bioreactors, while the packed- or fixed-bed and the hollow fiber bioreactors allow the cultivation of more complex structures (Specht et al., 2018; Pajčin et al., 2022; Roy et al., 2023).
Stirred-tank bioreactors are often used for mammalian cell cultivation. Stirrers in those bioreactors are used to ensure a homogeneous distribution of the nutrients, oxygen, and cells. This can lead to high shear stresses acting on the cells, which has an impact on their viability (Marks, 2003). Since most cells are anchorage dependent, they can either be grown on microcarriers or in aggregates. Microcarriers provide a large surface to grow on and are suitable for bovine muscle cells (Verbruggen et al., 2018; Bodiou et al., 2020). Different cell types such as modified iPSCs, ESCs from mice and human and mice muscle cells can be grown in aggregates (Fok and Zandstra, 2005; Abbasalizadeh et al., 2012; Chen et al., 2012; Aguanno et al., 2019; Post et al., 2020). However, mice muscle cells showed the characteristics of dormant cells when grown in aggregates, which make them unsuitable for cell expansion (Post et al., 2020). More research is needed for cell aggregates in the cultured meat domain to investigate the relevancy of this process (Djisalov et al., 2021).
Wave or rocking bioreactors are a low-shear-alternative compared to the stirred-tank bioreactor and are as well commonly used for cell cultivation (Cantarero Rivera and Chen, 2022). The cells are suspended in aggregates or on microcarriers in a bag or container, which is mounted to a rocking platform (Cantarero Rivera and Chen, 2022). Both bioreactor types, stirred-tank and wave or rocking, can be used as single-use bioreactors, which has multiple advantages such as easier sterilization, lower contamination risk, lower energy and sensor cost and allows to shorten the downtimes between batches. Further, single-use bioreactors allow to lower the water and energy usage, reducing the environmental impact compared to traditional reusable stainless-steel bioreactors (Chen L. et al., 2022).
The mixing in air-lift bioreactors is carried out without mixing units but through integrated air streams or the stream of other gases and special components that force a circulating flow. This is advantageous because it can lead to less inhomogeneity, less shear stress and lower power requirement due to less energy dissipation compared to stirred-tank bioreactors (Merchuk, 1990).
Packed-bed or fixed-bed bioreactors consist of two vessels: one contains the porous or fibrous scaffold for the cells to attach to while the other contains the culture medium. Both vessels are connected to each other through a circulating loop. The medium is pumped from the medium vessel through the scaffold containing the cells and brought back to the medium vessel. Operation modes are batch, fed-batch or perfusion mode. At a larger scale a radial flow is needed to overcome potential oxygen limitations (Pörtner et al., 2007).
As mentioned before hollow fiber bioreactors are considered for the differentiation phase of cells. The bioreactor consists of semipermeable hollow fibers, which allow the artificial circulation of nutrients and oxygen. Cellulose and polyethersulfone are most commonly used for the hollow fibers (Pajčin et al., 2022). Tuomisto et al. (2022) recently calculated the life cycle assessment of a bioprocess design using hollow fiber bioreactors. The bioreactor used for research consisted of polystyrene fibers that were used as scaffolds and further allowed the proliferation and differentiation of the cells. It was possible to produce a cell slurry that could later be incorporated in a product. However, the production of whole-cut meat involves other design challenges, which were not addressed in the work (Tuomisto et al., 2022). If cells must be detached from the fibers it can cause problems, since the reagent might not be able to penetrate all cell layers (Kirsch et al., 2023).
3.4.2 Expected impacts of bioreactors used in production of cultivated meat
Due to high media and cell costs, scaling of the cultivated meat production process is very expensive leading to lacking data of scaled bioprocess designs (Li et al., 2020b). Therefore, the data present for bioprocess design is based on assumptions, small-scale experiments or simulations. As explained above, the choice of the bioreactor will influence the structure/texture of the final product, which either raises or lowers cultural barrier for consumers (Specht et al., 2018; Pajčin et al., 2022; Roy et al., 2023). Further, the operation mode of the bioprocess is directly related to the capital costs (Humbird, 2021), hence it relates to the cost barrier for producers. More data is needed to specifically determine the impact of the different innovations possible in the field. The claims about impacts of technologies on inclusion in production or consumption are analyzed below and summarized in Table 6, the results are further used for the creation of the radar shown in Figure 3.
Humbird (2021) estimated the costs for cultured meat bioprocesses running in fed-batch and perfusion. He assumes that achieving a price of cultured meat at $50 per kilogram in supermarkets, which relates to the price for premium cut meat, might result in the replacement of traditional meat with cell-cultured alternatives. Running the bioprocess in fed-batch could potentially allow to meet the 50$ price mark if low-cost hydrolysates could be used in the media. However, perfusion exceeds the price mark due to the increased capital cost and capital-dependent costs. These claims refer to financial barrier for consumers. In both cases the price would be equal or higher to premium cut meat, which is not affordable for many (hence these claims are negative).
The advantage of stirred-tank bioreactors is a good scalability of the cultivation process (Moritz et al., 2015; Post et al., 2020). Additionally, stirred-tank bioreactors allow for a better quality control compared to hollow fiber and fixed-bed bioreactors which lead to lower production costs (Bodiou et al., 2020) (positive claim). Hanga et al. (2020) demonstrated the cultivation of bovine adipose cells on microcarriers in a 3 L-single-use stirred-tank bioreactor. This approach was specially developed for the cultivation of meat. A negative aspect of stirred-tank bioreactors is the need for higher media volume in comparison to other bioreactors (Allan et al., 2019; Bellani et al., 2020; Thyden et al., 2022), which raises the production costs (negative claim).
The scale up of wave or rocking bioreactors is limited since the bags can only be operated at 50% of their capacity to guarantee homogeneous mixing (Cantarero Rivera and Chen, 2022). Further, to be used in the cultivated meat production, the single-use bioreactors need to be more affordable (Chen Y. P. et al., 2022). The limited scale-up and high costs of single-use bioreactors constitute cost barriers for producers (negative claim). However, compared to fixed-bed bioreactors and hollow fiber bioreactors, the use of microcarriers in stirred-tank and wave bioreactors is advantageous since it allows to control and monitor the culturing process. This results in a more consistent quality and reduces the costs (Bodiou et al., 2020) (expectation of lower cost barrier = positive claim).
Packed- or fixed-bed bioreactors allow the cultivation of more complex, structured cultured meat products (Pajčin et al., 2022). Such products are expected to be more acceptable to consumers (lower cultural barriers = positive claim). The reduction of the cultivation medium and the operational costs might be possible due to the large surface area of the scaffold which allows for a high cell concentration and therefore circumvents the need for frequent passaging. This lowers cost barriers for producers (positive claim). Meuwly et al. (2004) analyzed the effect of the perfusion rate in the fixed-bed bioreactor while cultivating Chinese Hamster Ovary cells. A reduction of 25% media perfusion on a pilot scale allowed to maintain the productivity while reducing the costs for media, material, and human resources (Meuwly et al., 2004). However, high cell densities also lead to concentration gradients in nutrients and oxygen, which impacts the cellular behavior (Kumar and Starly, 2015). As mentioned above controlling and monitoring the culturing process is more complex compared to stirred-tank and wave or rocking bioreactors (Bodiou et al., 2020). This complexity creates a barrier for producers who lack advanced controlling and monitoring capabilities (negative claim about capability barrier).
Hollow fiber bioreactors may allow for the cultivation of a continuous piece of tissue allowing to grow more complex structures (Specht et al., 2018; Roy et al., 2023), hence meeting the cultural expectations of consumers (positive claim about cultural barriers). However, the bioreactors allowing to produce more complex meat structures such as the packed-bed or the hollow fiber bioreactor allow less quality control compared to the wave or stirred-tank bioreactor (Bodiou et al., 2020) and require higher capabilities from producers (capability barrier = negative claim).
4 Discussion and conclusion
As it is still unclear if and how cultivated meat would contribute toward creating inclusive food systems, this study used the secondary and primary data to create an overview of technologies for production of cultivated meat and the claims about their expected contributions toward inclusion in production and consumption.
Very different technological approaches are being developed for the cultivated meat production in the four areas identified as current technological bottlenecks: cells, medium, scaffolds and bioprocess (Chen L. et al., 2022). Most technologies are at the early stages of development and there is little knowledge about their future impacts. Based on expert opinions, each technological innovation-in-the-making is expected to have some impacts and contributions toward inclusive consumption and/or production. Figure 3 gives an overview of the claims presented in Section 3 in the format of an innovation radar for cultivated meat. The technologies are listed around the rim of the radar and are divided into the sections cells, scaffolds, medium and bioprocess. The positive and negative claims about the technology’s contributions toward social inclusion are displayed as diamonds. The claims relate to barriers and risks and each is assigned a different color. The positive claims are displayed in the inner circular section of the radar. The negative claims are displayed in the outer circular section of the radar. The claims presented in this format have been discussed in the sections 3.1.2, 3.2.2, 3.3.2, and 3.4.2.
The findings displayed on the radar bring us to three important conclusions. First, most technological solutions are associated with positive and negative expectations and currently it is not possible that one specific approach to cells, scaffolds, medium and bioprocess is going to be much better for enabling social inclusion than others. Second, most technological solutions for sourcing and optimizing cells and for producing culture medium are expected to have some exclusionary effects, suggesting that currently it is not thinkable that production and consumption of cultivated meat could be socially inclusive. Third, while there is some understanding of how the technologies could change the costs of production and if they would align or misalign with cultural values and norms, it is poorly understood how most technologies for cultivated meat production relate to other barriers to inclusive consumption and production.
The current study has some limitations. First, the study identifies a range of technologies for production of cultivated meat and expert claims about their impacts but neither of these lists is exhaustive. It is likely that relevant impacts have not been identified and some technologies were missed given the limited number of interviews. Second, the study is exploratory and not evaluative – it assesses neither the extent to which the impacts are considered to be possible or likely by the experts, nor does it assess the magnitude of these impacts. Our study shows that the different technological solutions are expected to have contributions toward inclusive consumption and production, but the impacts need to be assessed in future studies.
The following implications for research and development funding policies can be drawn from the study. First, public funders and private investors should be aware that there are many different approaches for cultivated meat production, which are expected to have different contributions toward inclusive consumption and production. Financial support for research and development should come with a requirement to thoroughly assess the impacts of emerging processes for production of cultivated meat. To this end, support for inter- and transdisciplinary collaborative research assessing the economic, environmental, and social impacts of technological solutions for cultivated meat production is advised. Second, the policy makers should recognize that there is a possibility of a ‘directionality failure’ (Weber and Rohracher, 2012) within the field of cultivated meat – that is a possibility that the less sustainable approaches to production of cultivated meat will be developed, diffused, and institutionalized while more sustainable approaches will be abandoned. To avoid such failure, there is a need for (1) support for a variety of approaches for cultivated meat production, (2) coordinated efforts of actors in the public and private sectors to generate missing knowledge about impacts of various approaches for production of cultivated meat, (3) system-level monitoring of emerging approaches and their impacts, (4) readiness to abandon technological directions when it becomes apparent that their impacts are undesirable or less desirable than those of other protein sources. Lastly, the regulatory framework for cultivated meat production and sales needs to be clarified in the EU.
In conclusion, this article identifies the possible impacts on social inclusion that the currently developed different technological approaches for production of cultivated meat could have in the future. It visualizes the findings as the innovation radar for cultivated meat. The study identifies the gaps in the understanding of the contributions of cultivated meat production toward inclusive food systems and makes recommendations for future research and development and funding policies.
Data availability statement
The raw data supporting the conclusions of this article will be made available by the authors, without undue reservation.
Author contributions
LW: Conceptualization, Data curation, Investigation, Project administration, Validation, Visualization, Writing – original draft, Writing – review & editing, Formal analysis, Funding acquisition, Methodology. DW: Conceptualization, Formal analysis, Investigation, Methodology, Project administration, Supervision, Validation, Visualization, Writing – original draft, Writing – review & editing. CD: Funding acquisition, Project administration, Supervision, Writing – review & editing. CR: Funding acquisition, Project administration, Supervision, Writing – review & editing.
Funding
The author(s) declare that financial support was received for the research, authorship, and/or publication of this article. The work presented here was funded under the program Grand Challenges 1: Social Cohesion, Exploratory Projects (IFST Project, Grant. No. 111_MC_SocCoh_5) by the Berlin University Alliance (BUA), funded by the Federal Ministry of Education and Research (BMBF) and the State of Berlin under the Excellence Strategy of the Federal Government and the States. We acknowledge also support of the FEI (Grant No. 01IF22232N), supported within the program for promoting the Industrial Collective Research (IGF) of the German Ministry of Economics and Climate Action (BMWK), based on a resolution of the German Parliament. We acknowledge support by the Open Access Publication Fund of the Technische Universität Berlin.
Acknowledgments
The authors thank all participants of the interviews for supporting this study with primary data for scientific evaluation.
Conflict of interest
The authors declare that the research was conducted in the absence of any commercial or financial relationships that could be construed as a potential conflict of interest.
Publisher’s note
All claims expressed in this article are solely those of the authors and do not necessarily represent those of their affiliated organizations, or those of the publisher, the editors and the reviewers. Any product that may be evaluated in this article, or claim that may be made by its manufacturer, is not guaranteed or endorsed by the publisher.
References
Abbasalizadeh, S., Larijani, M. R., Samadian, A., and Baharvand, H. (2012). Bioprocess development for mass production of size-controlled human pluripotent stem cell aggregates in stirred suspension bioreactor. Tissue Eng. Part C Methods 18, 831–851. doi: 10.1089/ten.tec.2012.0161
Aguanno, S., Petrelli, C., Di Siena, S., de Angelis, L., Pellegrini, M., and Naro, F. (2019). A three-dimensional culture model of reversibly quiescent myogenic cells. Stem Cells Int. 2019:7548160. doi: 10.1155/2019/7548160
Allan, S. J., de Bank, P. A., and Ellis, M. J. (2019). Bioprocess design considerations for cultured meat production with a focus on the expansion bioreactor. Front. Sustain. Food Syst. 3:44. doi: 10.3389/fsufs.2019.00044
Andreassen, R. C., Rønning, S. B., Solberg, N. T., Grønlien, K. G., Kristoffersen, K. A., Høst, V., et al. (2022). Production of food-grade microcarriers based on by-products from the food industry to facilitate the expansion of bovine skeletal muscle satellite cells for cultured meat production. Biomaterials 286:121602. doi: 10.1016/j.biomaterials.2022.121602
Barbosa, W., Correia, P., Vieira, J., Leal, I., Rodrigues, L., Nery, T., et al. (2023). Trends and technological challenges of 3D bioprinting in cultured meat: technological prospection. Appl. Sci. 13:12158. doi: 10.3390/app132212158
Bellani, C. F., Ajeian, J., Duffy, L., Miotto, M., Groenewegen, L., and Connon, C. J. (2020). Scale-up Technologies for the Manufacture of adherent cells. Front. Nutr. 7:575146. doi: 10.3389/fnut.2020.575146
Ben-Arye, T., and Levenberg, S. (2019). Tissue engineering for clean meat production. Front. Sustain. Food Syst. 3:46. doi: 10.3389/fsufs.2019.00046
Ben-Arye, T., Shandalov, Y., Ben-Shaul, S., Landau, S., Zagury, Y., Ianovici, I., et al. (2020). Textured soy protein scaffolds enable the generation of three-dimensional bovine skeletal muscle tissue for cell-based meat. Nat. Food 1, 210–220. doi: 10.1038/s43016-020-0046-5
Berger-Schmitt, R. (2002). Considering social cohesion in quality of life assessments: concept and measurement. Soc. Indic. Res. 11, 403–428. doi: 10.1007/0-306-47513-8_18
Bhat, Z. F., Morton, J. D., Mason, S. L., Bekhit, A. E.-D. A., and Bhat, H. F. (2019). Technological, regulatory, and ethical aspects of in vitro meat: a future slaughter-free harvest. Compr. Rev. Food Sci. Food Saf. 18, 1192–1208. doi: 10.1111/1541-4337.12473
Bodiou, V., Moutsatsou, P., and Post, M. J. (2020). Microcarriers for upscaling cultured meat production. Front. Nutr. 7:10. doi: 10.3389/fnut.2020.00010
Boe-Lillegraven, S., and Monterde, S. (2015). Exploring the cognitive value of technology foresight: the case of the Cisco Technology radar. Technol. Forecast. Soc. Chang. 101, 62–82. doi: 10.1016/j.techfore.2014.07.014
Bogliotti, Y. S., Wu, J., Vilarino, M., Okamura, D., Soto, D. A., Zhong, C., et al. (2018). Efficient derivation of stable primed pluripotent embryonic stem cells from bovine blastocysts. Proc. Natl. Acad. Sci. USA 115, 2090–2095. doi: 10.1073/pnas.1716161115
Bomkamp, C., Skaalure, S. C., Fernando, G. F., Ben-Arye, T., Swartz, E. W., and Specht, E. A. (2022). Scaffolding biomaterials for 3D cultivated meat: prospects and challenges. Adv. Sci. (Weinh) 9:e2102908. doi: 10.1002/advs.202102908
Cantarero Rivera, F. J., and Chen, J. (2022). Computational fluid dynamics modeling of cell cultures in bioreactors and its potential for cultivated meat production—a mini-review. Future Foods 6:100195. doi: 10.1016/j.fufo.2022.100195
Chen, V. C., Couture, S. M., Ye, J., Lin, Z., Hua, G., Huang, H.-I. P., et al. (2012). Scalable GMP compliant suspension culture system for human ES cells. Stem Cell Res. 8, 388–402. doi: 10.1016/j.scr.2012.02.001
Chen, Y. P., Feng, X., Blank, I., and Liu, Y. (2022). Strategies to improve meat-like properties of meat analogs meeting consumers' expectations. Biomaterials 287:121648. doi: 10.1016/j.biomaterials.2022.121648
Chen, L., Guttieres, D., Koenigsberg, A., Barone, P. W., Sinskey, A. J., and Springs, S. L. (2022). Large-scale cultured meat production: trends, challenges and promising biomanufacturing technologies. Biomaterials 280:121274. doi: 10.1016/j.biomaterials.2021.121274
Chodkowska, K. A., Wódz, K., and Wojciechowski, J. (2022). Sustainable future protein foods: the challenges and the future of cultivated meat. Foods 11:4008. doi: 10.3390/foods11244008
Choi, K.-H., Lee, D.-K., Kim, S. W., Woo, S.-H., Kim, D.-Y., and Lee, C.-K. (2019). Chemically defined media can maintain pig pluripotency network in vitro. Stem Cell Rep. 13, 221–234. doi: 10.1016/j.stemcr.2019.05.028
Cui, X., Li, J., Hartanto, Y., Durham, M., Tang, J., Zhang, H., et al. (2020). Advances in extrusion 3D bioprinting: a focus on multicomponent hydrogel-based bioinks. Adv. Healthc. Mater. 9:e1901648. doi: 10.1002/adhm.201901648
Djisalov, M., Knežić, T., Podunavac, I., Živojević, K., Radonic, V., Knežević, N. Ž., et al. (2021). Cultivating Multidisciplinarity: manufacturing and sensing challenges in cultured meat production. Biology (Basel) 10:204. doi: 10.3390/biology10030204
D'Odorico, P., Davis, K. F., Rosa, L., Carr, J. A., Chiarelli, D., Dell'Angelo, J., et al. (2018). The global food-energy-water Nexus. Rev. Geophys. 56, 456–531. doi: 10.1029/2017RG000591
Dong, H., Wang, P., Yang, Z., and Xu, X. (2023). 3D printing based on meat materials: challenges and opportunities. Curr. Res. Food Sci. 6:100423. doi: 10.1016/j.crfs.2022.100423
Dutta, S. D., Ganguly, K., Jeong, M.-S., Patel, D. K., Patil, T. V., Cho, S.-J., et al. (2022). Bioengineered lab-grown meat-like constructs through 3D bioprinting of Antioxidative protein hydrolysates. ACS Appl. Mater. Interfaces 14, 34513–34526. doi: 10.1021/acsami.2c10620
Enrione, J., Blaker, J. J., Brown, D. I., Weinstein-Oppenheimer, C. R., Pepczynska, M., Olguín, Y., et al. (2017). Edible scaffolds based on non-mammalian biopolymers for myoblast growth. Materials (Basel) 10:1404. doi: 10.3390/ma10121404
Ezashi, T., Telugu, B. P. V. L., Alexenko, A. P., Sachdev, S., Sinha, S., and Roberts, R. M. (2009). Derivation of induced pluripotent stem cells from pig somatic cells. Proc. Natl. Acad. Sci. USA 106, 10993–10998. doi: 10.1073/pnas.0905284106
Fereday, J., and Muir-Cochrane, E. (2006). Demonstrating rigor using thematic analysis: a hybrid approach of inductive and deductive coding and theme development.
Fernandes, A. M., de Souza Teixeira, O., Palma Revillion, J. P., and de Souza, Â. R. L. (2020). Conceptual evolution and scientific approaches about synthetic meat. J. Food Sci. Technol. 57, 1991–1999. doi: 10.1007/s13197-019-04155-0
Fok, E. Y. L., and Zandstra, P. W. (2005). Shear-controlled single-step mouse embryonic stem cell expansion and embryoid body-based differentiation. Stem Cells 23, 1333–1342. doi: 10.1634/stemcells.2005-0112
Food Systems Summit Dialogs (2021). Meat Sector in Transition: creating an inclusive approach to systemic change. Available at: https://summitdialogues.org/dialogue/16769/ (accessed September 02, 2024).
Furuhashi, M., Morimoto, Y., Shima, A., Nakamura, F., Ishikawa, H., and Takeuchi, S. (2021). Formation of contractile 3D bovine muscle tissue for construction of millimetre-thick cultured steak. NPJ Sci. Food 5:6. doi: 10.1038/s41538-021-00090-7
Gaskell, G., Allum, N., Bauer, M., Durant, J., Allansdottir, A., Bonfadelli, H., et al. (2000). Biotechnology and the European public. Nat. Biotechnol. 18, 935–938. doi: 10.1038/79403
Giambona, F., and Vassallo, E. (2014). Composite Indicator of social inclusion for European countries. Soc. Indic. Res. 116, 269–293. doi: 10.1007/s11205-013-0274-2
Godfray, H. C. J., Aveyard, P., Garnett, T., Hall, J. W., Key, T. J., Lorimer, J., et al. (2018). Meat consumption, health, and the environment. Science 361, 1–8. doi: 10.1126/science.aam5324
Golovatchev, J., Budde, O., and Kellmereit, D. (2010). Technology and innovation radars: effective instruments for the development of a sustainable innovation strategy and successful procut launches. Int. J. Innov. Technol. Manag. 7, 229–236. doi: 10.1142/S0219877010002008
Gstraunthaler, G., and Lindl, T. (2013). Zell- und Gewebekultur: Allgemeine Grundlagen und spezielle Anwendungen. Berlin, Heidelberg: Springer Spektrum.
Guan, X., Lei, Q., Yan, Q., Li, X., Zhou, J., Du, G., et al. (2021). Trends and ideas in technology, regulation and public acceptance of cultured meat. Future Foods 3:100032. doi: 10.1016/j.fufo.2021.100032
Guan, X., Yan, Q., Ma, Z., and Zhou, J. (2023). Production of mature myotubes in vitro improves the texture and protein quality of cultured pork. Food Funct. 14, 3576–3587. doi: 10.1039/D3FO00445G
Handral, H. K., Hua Tay, S., Wan Chan, W., and Choudhury, D. (2022). 3D printing of cultured meat products. Crit. Rev. Food Sci. Nutr. 62, 272–281. doi: 10.1080/10408398.2020.1815172
Hanga, M. P., Ali, J., Moutsatsou, P., de La Raga, F. A., Hewitt, C. J., Nienow, A., et al. (2020). Bioprocess development for scalable production of cultivated meat. Biotechnol. Bioeng. 117, 3029–3039. doi: 10.1002/bit.27469
Hanga, M. P., de La Raga, F. A., Moutsatsou, P., Hewitt, C. J., Nienow, A. W., and Wall, I. (2021). Scale-up of an intensified bioprocess for the expansion of bovine adipose-derived stem cells (bASCs) in stirred tank bioreactors. Biotechnol. Bioeng. 118, 3175–3186. doi: 10.1002/bit.27842
Haraguchi, Y., Okamoto, Y., and Shimizu, T. (2022). A circular cell culture system using microalgae and mammalian myoblasts for the production of sustainable cultured meat. Arch. Microbiol. 204:615. doi: 10.1007/s00203-022-03234-9
Haraguchi, Y., and Shimizu, T. (2021). Microalgal culture in animal cell waste medium for sustainable 'cultured food' production. Arch. Microbiol. 203, 5525–5532. doi: 10.1007/s00203-021-02509-x
Harris, J. R., and Hoeger, U. (2020). Vertebrate and invertebrate respiratory proteins, lipoproteins and other body fluid proteins. Switzerland: Springer Nature.
Hoekstra, A. Y., and Mekonnen, M. M. (2012). The water footprint of humanity. Proc. Natl. Acad. Sci. USA 109, 3232–3237. doi: 10.1073/pnas.1109936109
Hu, W., Berdugo, C., and Chalmers, J. J. (2011). The potential of hydrodynamic damage to animal cells of industrial relevance: current understanding. Cytotechnology 63, 445–460. doi: 10.1007/s10616-011-9368-3
Hubalek, S., Post, M. J., and Moutsatsou, P. (2022). Towards resource-efficient and cost-efficient cultured meat. Curr. Opin. Food Sci. 47:100885. doi: 10.1016/j.cofs.2022.100885
Humbird, D. (2021). Scale-up economics for cultured meat. Biotechnol. Bioeng. 118, 3239–3250. doi: 10.1002/bit.27848
Ianovici, I., Zagury, Y., Redenski, I., Lavon, N., and Levenberg, S. (2022). 3D-printable plant protein-enriched scaffolds for cultivated meat development. Biomaterials 284:121487. doi: 10.1016/j.biomaterials.2022.121487
Intarapat, S., and Stern, C. D. (2013). Chick stem cells: current progress and future prospects. Stem Cell Res. 11, 1378–1392. doi: 10.1016/j.scr.2013.09.005
IPCC (2023). Climate change 2022: Mitigation of climate change: Working group III contribution to the sixth assessment report of the intergovernmental panel on climate change. Cambridge: Cambridge University Press.
Jairath, G., Mal, G., Gopinath, D., and Singh, B. (2021). A holistic approach to access the viability of cultured meat: a review. Trends Food Sci. Technol. 110, 700–710. doi: 10.1016/j.tifs.2021.02.024
Jara, T. C., Park, K., Vahmani, P., van Eenennaam, A. L., Smith, L. R., and Denicol, A. C. (2023). Stem cell-based strategies and challenges for production of cultivated meat. Nat. Food 4, 841–853. doi: 10.1038/s43016-023-00857-z
Kirsch, M., Morales-Dalmau, J., and Lavrentieva, A. (2023). Cultivated meat manufacturing: technology, trends, and challenges. Eng. Life Sci. 23:e2300227. doi: 10.1002/elsc.202300227
Kolkmann, A. M., van Essen, A., Post, M. J., and Moutsatsou, P. (2022). Development of a chemically defined medium for in vitro expansion of primary bovine satellite cells. Front. Bioeng. Biotechnol. 10:895289. doi: 10.3389/fbioe.2022.895289
Kumar, A. (2019). Beyond technical smartness: rethinking the development and implementation of sociotechnical smart grids in India. Energy Res. Soc. Sci. 49, 158–168. doi: 10.1016/j.erss.2018.10.026
Kumar, A., and Starly, B. (2015). Large scale industrialized cell expansion: producing the critical raw material for biofabrication processes. Biofabrication 7:44103. doi: 10.1088/1758-5090/7/4/044103
Labonté, R., Hadi, A., and Kauffmann, X. (2011). Indicators of social exclusion and inclusion: a critical and comparative analysis of the literature. PHIRN Working Papers 2.
Law, J. X., Liau, L. L., Saim, A., Yang, Y., and Idrus, R. (2017). Electrospun collagen nanofibers and their applications in skin tissue engineering. Tissue Eng. Regen. Med. 14, 699–718. doi: 10.1007/s13770-017-0075-9
Lee, M., Park, S., Choi, B., Kim, J., Choi, W., Jeong, I., et al. (2022). Tailoring a gelatin/agar matrix for the synergistic effect with cells to produce high-quality cultured meat. ACS Appl. Mater. Interfaces 14, 38235–38245. doi: 10.1021/acsami.2c10988
Levi, S., Yen, F.-C., Baruch, L., and Machluf, M. (2022). Scaffolding technologies for the engineering of cultured meat: towards a safe, sustainable, and scalable production. Trends Food Sci. Technol. 126, 13–25. doi: 10.1016/j.tifs.2022.05.011
Li, X., Fu, X., Yang, G., and Du, M. (2020a). Review: enhancing intramuscular fat development via targeting fibro-adipogenic progenitor cells in meat animals. Animal 14, 312–321. doi: 10.1017/S175173111900209X
Li, X., Zhang, G., Zhao, X., Zhou, J., Du, G., and Chen, J. (2020b). A conceptual air-lift reactor design for large scale animal cell cultivation in the context of in vitro meat production. Chem. Eng. Sci. 211:115269. doi: 10.1016/j.ces.2019.115269
Listrat, A., Lebret, B., Louveau, I., Astruc, T., Bonnet, M., Lefaucheur, L., et al. (2016). How muscle structure and composition influence meat and flesh quality. Sci. World J. 2016:3182746. doi: 10.1155/2016/3182746
Lynch, J., and Pierrehumbert, R. (2019). Climate impacts of cultured meat and beef cattle. Front. Sustain. Food Syst. 3:5. doi: 10.3389/fsufs.2019.00005
MacQueen, L. A., Alver, C. G., Chantre, C. O., Ahn, S., Cera, L., Gonzalez, G. M., et al. (2019). Muscle tissue engineering in fibrous gelatin: implications for meat analogs. NPJ Sci. Food 3:20. doi: 10.1038/s41538-019-0054-8
Maqsood, M. I., Matin, M. M., Bahrami, A. R., and Ghasroldasht, M. M. (2013). Immortality of cell lines: challenges and advantages of establishment. Cell Biol. Int. 37, 1038–1045. doi: 10.1002/cbin.10137
Marks, D. M. (2003). Equipment design considerations for large scale cell culture. Cytotechnology 42, 21–33. doi: 10.1023/A:1026103405618
Mathew, A. G., Cissell, R., and Liamthong, S. (2007). Antibiotic resistance in bacteria associated with food animals: a United States perspective of livestock production. Foodborne Pathog. Dis. 4, 115–133. doi: 10.1089/fpd.2006.0066
Mattick, C. S., Landis, A. E., and Allenby, B. R. (2015a). A case for systemic environmental analysis of cultured meat. J. Integr. Agric. 14, 249–254. doi: 10.1016/S2095-3119(14)60885-6
Mattick, C. S., Landis, A. E., Allenby, B. R., and Genovese, N. J. (2015b). Anticipatory life cycle analysis of in vitro biomass cultivation for cultured meat production in the United States. Environ. Sci. Technol. 49, 11941–11949. doi: 10.1021/acs.est.5b01614
Merchuk, J. C. (1990). Why use air-lift bioreactors? Trends Biotechnol. 8, 66–71. doi: 10.1016/0167-7799(90)90138-N
Messmer, T., Klevernic, I., Furquim, C., Ovchinnikova, E., Dogan, A., Cruz, H., et al. (2022). A serum-free media formulation for cultured meat production supports bovine satellite cell differentiation in the absence of serum starvation. Nat. Food 3, 74–85. doi: 10.1038/s43016-021-00419-1
Meuwly, F., von Stockar, U., and Kadouri, A. (2004). Optimization of the medium perfusion rate in a packed-bed bioreactor charged with CHO cells. Cytotechnology 46, 37–47. doi: 10.1007/s10616-005-2105-z
Meyer, H.-P., Minas, W., and Schmidhalter, D. (2017). “Industrial-scale fermentation” in Industrial biotechnology. eds. C. Wittmann and J. C. Liao (Weinheim: Wiley), 1–53.
Miranti, R., and Yu, P. (2015). Why social exclusion persists among older people in Australia. Soc. Inclus. 3, 112–126. doi: 10.17645/si.v3i4.214
Moritz, M. S. M., Verbruggen, S. E. L., and Post, M. J. (2015). Alternatives for large-scale production of cultured beef: a review. J. Integr. Agric. 14, 208–216. doi: 10.1016/S2095-3119(14)60889-3
Moslemy, N., Sharifi, E., Asadi-Eydivand, M., and Abolfathi, N. (2023). Review in edible materials for sustainable cultured meat: scaffolds and microcarriers production. Int. J. Food Sci. Tech. 58, 6182–6191. doi: 10.1111/ijfs.16703
Mottet, A., de Haan, C., Falcucci, A., Tempio, G., Opio, C., and Gerber, P. (2017). Livestock: on our plates or eating at our table? A new analysis of the feed/food debate. Glob. Food Sec. 14, 1–8. doi: 10.1016/j.gfs.2017.01.001
Newton, P., and Blaustein-Rejto, D. (2021). Social and economic opportunities and challenges of plant-based and cultured meat for rural producers in the US. Front. Sustain. Food Syst. 5:270. doi: 10.3389/fsufs.2021.624270
Nichol, J. W., and Khademhosseini, A. (2009). Modular tissue engineering: engineering biological tissues from the bottom up. Soft Matter 5, 1312–1319. doi: 10.1039/B814285H
O'Brien, F. J. (2011). Biomaterials & scaffolds for tissue engineering. Mater. Today 14, 88–95. doi: 10.1016/S1369-7021(11)70058-X
Okamoto, Y., Haraguchi, Y., Sawamura, N., Asahi, T., and Shimizu, T. (2020). Mammalian cell cultivation using nutrients extracted from microalgae. Biotechnol. Prog. 36:e2941. doi: 10.1002/btpr.2941
Okamoto, Y., Haraguchi, Y., Yoshida, A., Takahashi, H., Yamanaka, K., Sawamura, N., et al. (2022). Proliferation and differentiation of primary bovine myoblasts using Chlorella vulgaris extract for sustainable production of cultured meat. Biotechnol. Prog. 38:e3239. doi: 10.1002/btpr.3239
Pain, B., Kress, C., and Rival-Gervier, S. (2018). Pluripotency in avian species. Int. J. Dev. Biol. 62, 245–255. doi: 10.1387/ijdb.170322bp
Painter, J. A., Hoekstra, R. M., Ayers, T., Tauxe, R. V., Braden, C. R., Angulo, F. J., et al. (2013). Attribution of foodborne illnesses, hospitalizations, and deaths to food commodities by using outbreak data, United States, 1998-2008. Emerg. Infect. Dis. 19, 407–415. doi: 10.3201/eid1903.111866
Pajčin, I., Knežić, T., Savic Azoulay, I., Vlajkov, V., Djisalov, M., Janjušević, L., et al. (2022). Bioengineering outlook on cultivated meat production. Micromachines (Basel) 13:402. doi: 10.3390/mi13030402
Pasitka, L., Cohen, M., Ehrlich, A., Gildor, B., Reuveni, E., Ayyash, M., et al. (2023). Spontaneous immortalization of chicken fibroblasts generates stable, high-yield cell lines for serum-free production of cultured meat. Nat. Food 4, 35–50. doi: 10.1038/s43016-022-00658-w
Percy-Smith, J. (2000). Policy responses to social exclusion. Buckingham, Philadelphia PA: Open University Press.
Portanguen, S., Tournayre, P., Sicard, J., Astruc, T., and Mirade, P.-S. (2019). Toward the design of functional foods and biobased products by 3D printing: a review. Trends Food Sci. Technol. 86, 188–198. doi: 10.1016/j.tifs.2019.02.023
Pörtner, R., Platas, O. B., Fassnacht, D., Nehring, D., Czermak, P., and Märkl, H. (2007). Fixed bed reactors for the cultivation of mammalian cells: design, performance and scale-up. TOBIOTJ 1, 41–46. doi: 10.2174/1874070700701010041
Post, M. J., Levenberg, S., Kaplan, D. L., Genovese, N., Fu, J., Bryant, C. J., et al. (2020). Scientific, sustainability and regulatory challenges of cultured meat. Nat. Food 1, 403–415. doi: 10.1038/s43016-020-0112-z
Rackham, O. J. L., Firas, J., Fang, H., Oates, M. E., Holmes, M. L., Knaupp, A. S., et al. (2016). A predictive computational framework for direct reprogramming between human cell types. Nat. Genet. 48, 331–335. doi: 10.1038/ng.3487
Ramesh, S., Harrysson, O. L., Rao, P. K., Tamayol, A., Cormier, D. R., Zhang, Y., et al. (2021). Extrusion bioprinting: recent progress, challenges, and future opportunities. Bioprinting 21:e00116. doi: 10.1016/j.bprint.2020.e00116
Revell, B. (2015). Meat and Milk consumption 2050: the potential for demand-side solutions to greenhouse gas emissions reduction. EuroChoices 14, 4–11. doi: 10.1111/1746-692X.12103
Rohrbeck, R., Heuer, J., and Arnold, H. (2006). “The technology radar - an instrument of technology intelligence and innovation strategy,” In 2006 IEEE International Conference on Management of Innovation and Technology (IEEE), 978–983.
Roobrouck, V. D., Ulloa-Montoya, F., and Verfaillie, C. M. (2008). Self-renewal and differentiation capacity of young and aged stem cells. Exp. Cell Res. 314, 1937–1944. doi: 10.1016/j.yexcr.2008.03.006
Rosselló, R. A., Chen, C.-C., Dai, R., Howard, J. T., Hochgeschwender, U., and Jarvis, E. D. (2013). Mammalian genes induce partially reprogrammed pluripotent stem cells in non-mammalian vertebrate and invertebrate species. eLife 2:e00036. doi: 10.7554/eLife.00036
Roy, N. K., Panda, S., and Dey, G. (2023). Engineering a sustainable protein revolution: recent advances in cultured meat production. Food Bioeng. 2, 301–316. doi: 10.1002/fbe2.12066
Schaeffer, W. I. (1990). Terminology associated with cell, tissue, and organ culture, molecular biology, and molecular genetics. Tissue culture association terminology committee. In Vitro Cell. Dev. Biol. 26, 97–101. doi: 10.1007/BF02624162
Scherer, L., Rueda, O., and Smetana, S. (2023). “Environmental impacts of meat and meat replacements” in Meat and meat replacements: Chapter 14 - environmental impacts of meat an meat replacements (Cambridge, United States and Kidlington, United Kingdom: Woodhead Publishing, Elsevier), 365–397.
Schwab, A., Levato, R., D'Este, M., Piluso, S., Eglin, D., and Malda, J. (2020). Printability and shape Fidelity of bioinks in 3D bioprinting. Chem. Rev. 120, 11028–11055. doi: 10.1021/acs.chemrev.0c00084
Seah, J. S. H., Singh, S., Tan, L. P., and Choudhury, D. (2022). Scaffolds for the manufacture of cultured meat. Crit. Rev. Biotechnol. 42, 311–323. doi: 10.1080/07388551.2021.1931803
Seo, J. W., Jung, W. K., Park, Y. H., and Bae, H. (2023). Development of cultivable alginate fibers for an ideal cell-cultivated meat scaffold and production of hybrid cultured meat. Carbohydr. Polym. 321:121287. doi: 10.1016/j.carbpol.2023.121287
Simsa, R., Yuen, J., Stout, A., Rubio, N., Fogelstrand, P., and Kaplan, D. L. (2019). Extracellular Heme proteins influence bovine Myosatellite cell proliferation and the color of cell-based meat. Food Secur. 8:521. doi: 10.3390/foods8100521
Singh, A., Kumar, V., Singh, S. K., Gupta, J., Kumar, M., Sarma, D. K., et al. (2023). Recent advances in bioengineered scaffold for in vitro meat production. Cell Tissue Res. 391, 235–247. doi: 10.1007/s00441-022-03718-6
Smetana, S., Mathys, A., Knoch, A., and Heinz, V. (2015). Meat alternatives: life cycle assessment of most known meat substitutes. Int. J. Life Cycle Assess. 20, 1254–1267. doi: 10.1007/s11367-015-0931-6
Smetana, S., Ristic, D., Pleissner, D., Tuomisto, H. L., Parniakov, O., and Heinz, V. (2023). Meat substitutes: resource demands and environmental footprints. Resour. Conserv. Recycl. 190:106831. doi: 10.1016/j.resconrec.2022.106831
Sofos, J. N. (2008). Challenges to meat safety in the 21st century. Meat Sci. 78, 3–13. doi: 10.1016/j.meatsci.2007.07.027
Soice, E., and Johnston, J. (2021). Immortalizing cells for human consumption. Int. J. Mol. Sci. 22:11660. doi: 10.3390/ijms222111660
Specht, E. A., Welch, D. R., Rees Clayton, E. M., and Lagally, C. D. (2018). Opportunities for applying biomedical production and manufacturing methods to the development of the clean meat industry. Biochem. Eng. J. 132, 161–168. doi: 10.1016/j.bej.2018.01.015
Steinfeld, H. (2006). Livestock's long shadow: Environmental issues and options. Rome: Food and Agriculture Organization of the United Nations.
Stephens, N., Di Silvio, L., Dunsford, I., Ellis, M., Glencross, A., and Sexton, A. (2018). Bringing cultured meat to market: technical, socio-political, and regulatory challenges in cellular agriculture. Trends Food Sci. Technol. 78, 155–166. doi: 10.1016/j.tifs.2018.04.010
Stout, A. J., Mirliani, A. B., White, E. C., Yuen, J. S., and Kaplan, D. L. (2021). Simple and effective serum-free medium for sustained expansion of bovine satellite cells for cell cultured meat.
Su, Y., Wang, L., Fan, Z., Liu, Y., Zhu, J., Kaback, D., et al. (2021). Establishment of bovine-induced pluripotent stem cells. Int. J. Mol. Sci. 22:10489. doi: 10.3390/ijms221910489
Thyden, R., Perreault, L. R., Jones, J. D., Notman, H., Varieur, B. M., Patmanidis, A. A., et al. (2022). An edible, Decellularized plant derived cell carrier for lab grown meat. Appl. Sci. 12:5155. doi: 10.3390/app12105155
Tuomisto, H. L., Allan, S. J., and Ellis, M. J. (2022). Prospective life cycle assessment of a bioprocess design for cultured meat production in hollow fiber bioreactors. Sci. Total Environ. 851:158051. doi: 10.1016/j.scitotenv.2022.158051
Tuomisto, H. L., and de Mattos, M. J. T. (2011). Environmental impacts of cultured meat production. Environ. Sci. Technol. 45, 6117–6123. doi: 10.1021/es200130u
Tuomisto, H. L., Ellis, M. J., and Haastrup, P. (2014). Environmental impacts of cultured meat: alternative production scenarios. Proceedings of the 9th International Conference on Life Cycle Assesment in the Agri-Food Sector.
Verbruggen, S., Luining, D., van Essen, A., and Post, M. J. (2018). Bovine myoblast cell production in a microcarriers-based system. Cytotechnology 70, 503–512. doi: 10.1007/s10616-017-0101-8
von Soest, C. (2023). Why do we speak to experts? Reviving the strength of the expert interview method. Perspect. Polit. 21, 277–287. doi: 10.1017/S1537592722001116
Vrooman, J. C., Hoff, S. J. M., and Guiaux, M. (2015). Descendants of hardship: prevalence, drivers and scarring effects of social exclusion in childhood. Soc. Inclus. 3, 76–97. doi: 10.17645/si.v3i4.129
Weber, K. M., and Rohracher, H. (2012). Legitimizing research, technology and innovation policies for transformative change: combining insights from innovation systems and multi-level perspective in a comprehensive ‘failures’ framework. Res. Policy 41, 1037–1047. doi: 10.1016/j.respol.2011.10.015
Weckowska, D., Barnickel, C., Dreher, C., and Feindt, P. (2022). “2022. Towards a framework for inclusive food systems transitions,” 13th International Sustainability Transitions Conference, Online., 2022.
Weston, A. R., Rogers, R. W., and Althen, T. G. (2002). Review: the role of collagen in meat tenderness. Profess. Anim. Sci. 18, 107–111. doi: 10.15232/S1080-7446(15)31497-2
Wu, Z., Chen, J., Ren, J., Bao, L., Liao, J., Cui, C., et al. (2009). Generation of pig induced pluripotent stem cells with a drug-inducible system. J. Mol. Cell Biol. 1, 46–54. doi: 10.1093/jmcb/mjp003
Yang, M., Wang, Q., Zhu, Y., Sheng, K., Xiang, N., and Zhang, X. (2023). Cell culture medium cycling in cultured meat: Key factors and potential strategies. Trends Food Sci. Technol. 138, 564–576. doi: 10.1016/j.tifs.2023.06.031
Yao, T., and Asayama, Y. (2017). Animal-cell culture media: history, characteristics, and current issues. Reprod. Med. Biol. 16, 99–117. doi: 10.1002/rmb2.12024
Ye, Y., Zhou, J., Guan, X., and Sun, X. (2022). Commercialization of cultured meat products: current status, challenges, and strategic prospects. Fut. Foods 6:100177. doi: 10.1016/j.fufo.2022.100177
Yen, F.-C., Glusac, J., Levi, S., Zernov, A., Baruch, L., Davidovich-Pinhas, M., et al. (2023). Cultured meat platform developed through the structuring of edible microcarrier-derived microtissues with oleogel-based fat substitute. Nat. Commun. 14:2942. doi: 10.1038/s41467-023-38593-4
Zehorai, E., Maor-Shoshani, A., Molotski, N., Dorojkin, A., Marelly, N., Dvash, T., et al. (2023). From fertilised oocyte to cultivated meat - harnessing bovine embryonic stem cells in the cultivated meat industry. Reprod. Fertil. Dev. 36, 124–132. doi: 10.1071/RD23169
Zernov, A., Baruch, L., and Machluf, M. (2022). Chitosan-collagen hydrogel microparticles as edible cell microcarriers for cultured meat. Food Hydrocoll. 129:107632. doi: 10.1016/j.foodhyd.2022.107632
Zhang, J., Li, X., Liu, H., Zhou, J., Chen, J., and Du, G. (2021). Hydrodynamics and mass transfer in spinner flasks: implications for large scale cultured meat production. Biochem. Eng. J. 167:107864. doi: 10.1016/j.bej.2020.107864
Keywords: cultured meat, cultivated meat, scaffolding, cells, cell culture media, bioprocess, innovation radar, social inclusion
Citation: Woelken L, Weckowska DM, Dreher C and Rauh C (2024) Toward an innovation radar for cultivated meat: exploring process technologies for cultivated meat and claims about their social impacts. Front. Sustain. Food Syst. 8:1390720. doi: 10.3389/fsufs.2024.1390720
Edited by:
Chunhong Yuan, Iwate University, JapanReviewed by:
M. Maria Leena, SRM Institute of Science and Technology, IndiaSabine Bornkessel, Osnabrück University of Applied Sciences, Germany
Copyright © 2024 Woelken, Weckowska, Dreher and Rauh. This is an open-access article distributed under the terms of the Creative Commons Attribution License (CC BY). The use, distribution or reproduction in other forums is permitted, provided the original author(s) and the copyright owner(s) are credited and that the original publication in this journal is cited, in accordance with accepted academic practice. No use, distribution or reproduction is permitted which does not comply with these terms.
*Correspondence: Lisa Woelken, ZnJhbmtlQHR1LWJlcmxpbi5kZQ==; Cornelia Rauh, Y29ybmVsaWEucmF1aEB0dS1iZXJsaW4uZGU=