- 1International Livestock Research Institute, Nairobi, Kenya
- 2Mekelle University, Mekelle, Ethiopia
- 3Roslin Institute at the Royal (Dick) School of Veterinary Studies, University of Edinburgh, Edinburgh, United Kingdom
- 4Centre for Tropical Livestock Genetics and Health (CTLGH), The Roslin Institute at the Royal (Dick) School of Veterinary Studies, University of Edinburgh, Edinburgh, United Kingdom
- 5Centre for Tropical Livestock Genetics and Health (CTLGH), International Livestock Research Institute, Nairobi, Kenya
- 6Paul G. Allen School for Global Health, Washington State University, Washington, WA, United States
- 7Global Animal Health Tanzania, Arusha, Tanzania
- 8Livestock Climate Solutions, Bergen op Zoom, The Hague, Netherlands
- 9Environmental Defense Fund, New York City, NY, United States
- 10Nelson Mandela African Institution of Science and Technology, Arusha, Tanzania
- 11Edinburgh Napier University, Edinburgh, United Kingdom
- 12New Zealand Agricultural Greenhouse Gas Research Centre, Palmerston North, New Zealand
This study investigates the environmental and food security implications of livestock abortions and calf mortality in Tanzanian dairy systems and Kenyan beef systems by utilizing data from previously published studies. The environmental impact of livestock abortion is assessed in Tanzanian dairy systems, examining indigenous and exotic breeds of cattle and goats in Northern Tanzania. Calf mortality’s impact is evaluated in Kenyan beef systems, involving local cattle breeds in western Kenya. Greenhouse gas (GHG) emission intensity (EI) is estimated for both countries. The GHG emissions in Tanzania consider enteric fermentation, manure management, and feed production in different cattle and goat groups, as well as total milk production. In Kenya, enteric methane (CH4) EI related to calf mortality is assessed by estimating lifetime enteric CH4 emissions and total carcass production from dams and their offspring. The EI is compared between the observed scenario (16% calf mortality) and alternative scenarios (8, 4, and 0% calf mortality). A life cycle assessment using the Global Livestock Environmental Assessment Model-interactive (GLEAM-i) examines GHG sources and potential tradeoffs. Estimates are made for milk and carcass losses due to abortions and calf mortality, scaled to represent the entire country. Abortion increases milk EI by 4–18% in Tanzania, while Kenya’s EI ranges from 25.9 to 27.6 kg CO2 eq per kg carcass weight. Animal protein loss due to abortions is equivalent to the potential annual animal protein requirements of approximately 649 thousand people in Tanzania, while a 16% calf mortality rate in Kenya is equivalent to per capita consumption of 4.5 million people. The findings highlight the significant impact of abortions and calf mortality on GHG emissions and animal protein availability, emphasizing the potential for reduced emissions and improved food security through mitigation efforts. The contribution of emissions from enteric fermentation and manure management is significant across both countries, underscoring the importance of a systems perspective in evaluating the environmental impact of livestock production. This study provides insights into the environmental and food security implications of livestock abortions and calf mortality in Tanzania and Kenya, emphasizing the need for targeted interventions in sustainable livestock production.
1 Introduction
In low- and middle-income countries (LMIC), population growth, increased incomes, and urbanization have intensified the demand for animal-source foods (ASF; Latino et al., 2020). As such livestock production is currently one of the fastest-growing sectors within the agriculture industry in developing countries (Schneider and Tarawali, 2021), with projections indicating a three-fold increase in meat and a two-fold increase in milk demand across Africa between 2015 and 2050 (FAO, 2018). Meeting this escalating demand for animal-source foods in an environmentally sustainable manner poses a formidable challenge for the agriculture industry (Henchion et al., 2021).
A significant environmental challenge arises from the greenhouse gas (GHG) emissions associated with livestock farming as the global livestock sector is estimated to account for approximately 12% of all anthropogenic GHG emissions, with cattle meat and milk alone contributing 62% of these emissions (FAO, 2023). Identifying and implementing strategies that reduce emission intensity (EI = emission per unit of ASF; Durojaye et al., 2020) is crucial to fulfilling the demand for ASF without exacerbating GHG emissions (Skuce et al., 2016).
Improving livestock health presents a promising and cost-effective approach to increasing production while reducing GHG EI (Skuce et al., 2016). The World Organization for Animal Health (WOAH) estimates that approximately 20% of global livestock production is lost annually due to animal diseases (World Organization for Animal Health, 2014). These losses are due to mortality, decreased production efficiency, and compromised output quality or quantity (Skuce et al., 2016; Özkan et al., 2022). Abortions and calf mortality significantly contribute to these losses (Gulliksen et al., 2009; Keshavarzi et al., 2017; Parvez et al., 2020). Implementing control measures to address abortion rates and calf mortality potentially reduces GHG EI (Skuce et al., 2016; Samsonstuen et al., 2020).
Understanding the effects of improving livestock health, specifically by reducing abortions and calf mortality, on production and GHG emissions is particularly relevant in sub-Saharan Africa (SSA), where livestock farming is the major contributor to agricultural GHG emissions (Leitner et al., 2020). Methane (CH4) emissions from enteric fermentation and manure management across SSA are estimated to contribute to approximately 21% of anthropogenic GHG emissions, while nitrous oxide (N2O) from applied, deposited, and managed manure account for approximately 11% of emissions (Graham et al., 2022). The demand for livestock products in SSA is expected to increase several-fold by 2050 (Herrero et al., 2014) potentially contributing to an increase in livestock GHG emissions without mitigation efforts. Moreover, EI for milk and meat tend to be higher in SSA compared to other regions (FAO, 2023), due to productivity factors such as the relatively low milk and carcass yield in these systems, which in turn are influenced by livestock health issues including abortions and calf mortality (Skuce et al., 2016).
There are studies in non-African countries (Skuce et al., 2016; MacLeod and Moran, 2017) that demonstrated that addressing abortions and calf mortality in livestock herds reduces the EI of meat and milk production. However, there is a paucity of evidence in SSA that explore the relationship between animal health (specifically abortions and calf mortality), and GHG emissions. Consequently, there is a need for research to provide reliable quantitative estimates of the mitigation potential associated with improved animal health, particularly about abortions and calf mortality. This research paper aims to address this gap by quantifying the impact on the environmental footprint and food security resulting from livestock abortions and calf mortality in SSA livestock systems, using existing data that were collected from previous studies in Tanzania and Kenya.
2 Materials and methods
2.1 Study design
The data analyzed in this study was compiled from previously conducted studies in Tanzania on livestock abortion and calf mortality in Kenya that utilized a combination of cross-sectional and longitudinal approaches to collect the necessary data. The studies focused on examining two distinct livestock systems: dairy systems in Tanzania and beef systems in Kenya.
2.2 Data on livestock abortion
The data on livestock abortions was collected in northern Tanzania between October 2017 and September 2019. This region is known for its diverse range of agroecological systems and livestock management practices, including pastoralists, agro-pastoralists, and smallholder farmers (de Glanville et al., 2020). The studies by Thomas et al. (2022), Lankester et al. (2024) and Semango et al. (2024) collected data from 13 wards randomly selected from the Arusha, Kilimanjaro, and Manyara regions in northern Tanzania. For a visual representation of the study area, refer to Figure 1 adopted from Thomas et al. (2022).
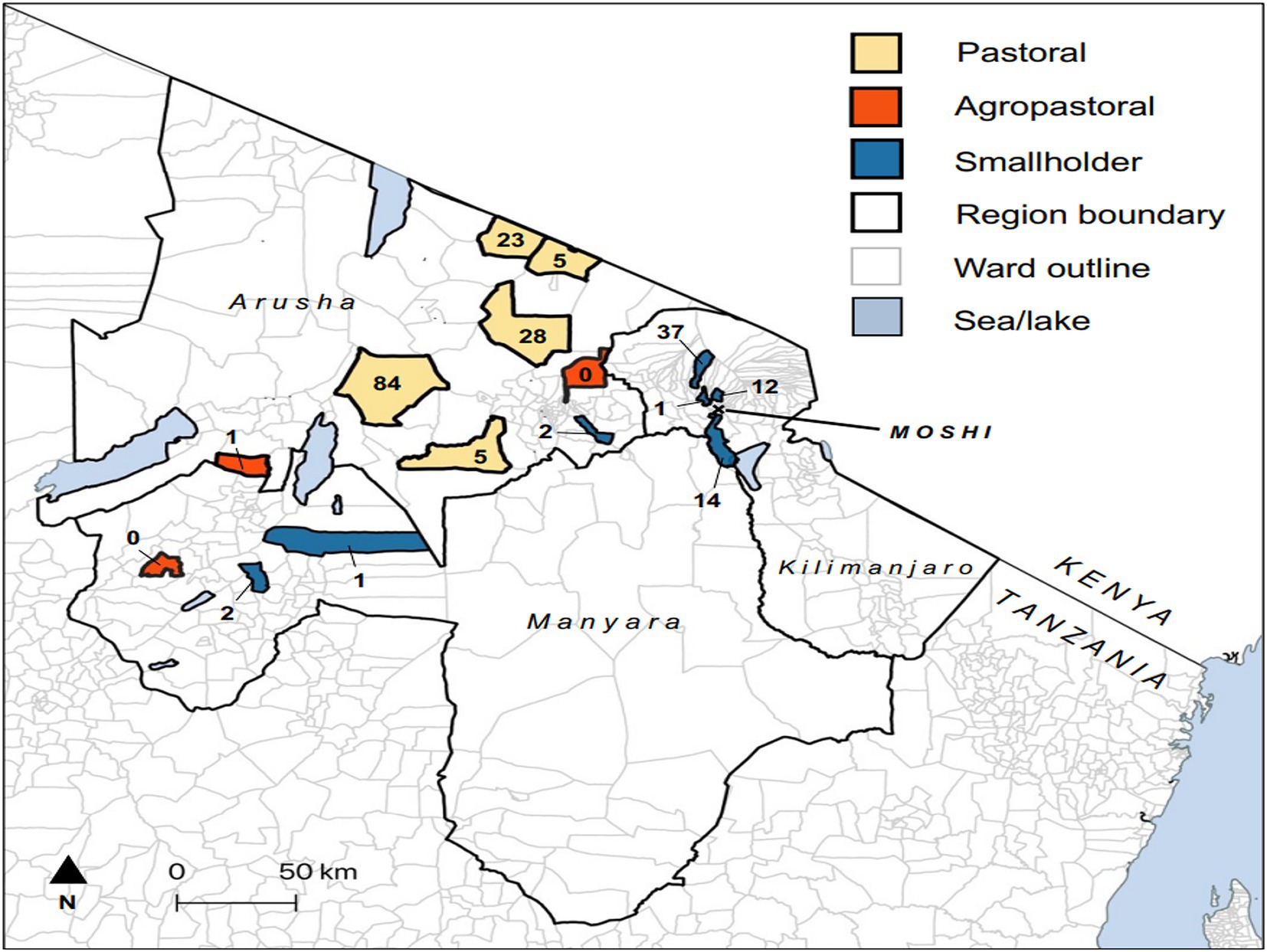
Figure 1. Map of the study area in Tanzania. Source: Thomas et al. (2022). Licensed under Creative Commons Attribution 4.0 (http://creativecommons.org/licenses/by/4.0/).
Detailed information on the data collection methods employed can be found in Thomas et al. (2022) and Lankester et al. (2024). In summary, farmers were instructed to report any abortion events either directly to the project field team or to their local livestock field officers via phone calls. Upon receiving a phone call, an investigation was initiated to gather detailed information about the dam that experienced the abortion, along with other demographic data. The collected data included the dam’s abortion history, previous abortions in the herd, herd management practices, herd composition, and the history of new animals introduced to the herd, among other factors.
The field team engaged with the farmers within 3 days of the abortion and followed up 28 days later to monitor the condition of the dam and collect information on milk yield after abortion. The timing of the abortion during pregnancy was determined through a combination of the farmers’ estimation and examination of the aborted fetus by qualified veterinarians. The survey covered both indigenous and exotic breeds of dairy cattle and goats, with the breeds identified based on farmers’ perceptions rather than genetic testing. Input parameters for estimating GHG emissions were derived from Semango et al. (2024), supplemented by additional information primarily sourced from national studies whenever available.
2.2.1 Estimation of greenhouse gas emission intensity from abortion data
To estimate the impact of abortions on milk production and subsequently, on daily GHG emissions and GHG EI, we calculated emissions for one calving or kidding interval and compared it between two groups: animals experiencing abortions (AB) and animals not experiencing abortions (NAB) for indigenous and exotic breeds. The calving or kidding interval refers to the duration between two consecutive calving or kidding events and was considered to be approximately 16 months for cows and 9 months for small ruminants (Asimwe and Kifaro, 2007; Chenyambuga et al., 2010). For this study, abortion was defined as any loss of pregnancy in animals that were confirmed pregnant (Deresa et al., 2020).
The estimation considered CH4 emissions from enteric fermentation, CH4 and N2O from manure management, N2O from managed soils by manure and urine deposited on pasture, and N2O from inorganic fertilizer application to produce crop residue following the guidelines provided by the Intergovernmental Panel on Climate Change (IPCC, 2019) for low productivity systems in Africa. It also included estimation of CO2 emissions from inorganic fertilizer production. Input parameters for the estimation of GHG emissions were derived from the study, and additional information was primarily sourced from national studies whenever available as demonstrated in Table 1. In situations where national data were not accessible, the study employed default values for low-productivity systems in Africa from the IPCC (2019) guidelines.
2.2.1.1 Methane emissions from enteric fermentation
To estimate the gross energy intake (MJ day−1), the study considered the energy content of the diet (as outlined in Table 1) and the daily energy requirements of the cows and goats according to IPCC (2019) guidelines. These requirements encompass maintenance, milk production, and activity and pregnancy. The energy requirements for maintenance remained consistent for animals, irrespective of whether they experienced abortion or not. However, the energy requirement for milk production varied depending on the level of milk yield. Similarly, the energy requirements varied between the animals that aborted and those that did not abort. In line with the IPCC (2019) guidelines, a CH4 conversion factor (Ym) of 7.0% for cattle and 5.5% for goats was utilized.
2.2.1.2 Methane and nitrous oxide emissions from manure management
The estimation of CH4 and N2O emissions originating from manure management utilized the guidelines provided by IPCC (2019).The direct emissions of N2O and CH4 from manure in stables, storage facilities, and on pasture were calculated using Equation 10.25 and Equation 10.22, respectively. These equations provide the necessary framework to estimate the direct emissions of N2O and CH4 from manure in different settings.
In addition to direct emissions, the study also considered the indirect emissions of N2O. These indirect emissions encompass N2O derived from the volatilization of ammonia (NH3) and nitrogen oxides (NOx), as well as from the leaching of nitrate (NO3) from manure. The estimation of these indirect emissions was based on Equation 10.27 and Equation 10.29 (IPCC, 2019, p. 10.77–78), which provide the necessary calculations to estimate N2O emissions resulting from these processes.
2.2.1.3 Emissions from manure deposited on pasture and feed production
The Tier 1 methodology outlined in the IPCC (2019) guidelines was employed to determine the N2O resulting from both direct and indirect sources, and N2O from manure deposited on pasture and applied for crop production. The direct emissions of N2O were estimated using Equation 11.1, as specified in the guidelines. Similarly, the indirect emissions of N2O from manure applied and deposited were computed using Equations 11.9 and 11.10, which are relevant equations provided in the IPCC guidelines.
The CO2 and N2O emissions linked to crop residue relied on the composition of the diet. To determine the crop residue composition in the diet, we referenced a report by (Baltussen et al., 2020), which showed that 10% of the diet consisted of crop residue (wheat straw) and 90% was pasture. Since farming activities in this context are mostly manual or animal-powered, we assumed no emissions from energy usage during crop residue production.
To estimate the emissions associated with the production of wheat straw, including both N2O from the inorganic fertilizer applied on croplands and CO2 during the production of the inorganic fertilizer, we followed an indirect approach. Initially, the daily intake of wheat straw (in kg) was estimated based on its composition in the animals’ diet, which was determined to be 10%. We then estimated the land area (in hectares) needed to produce the estimated amount of wheat straw using data from (Tanzania Agricultural Research Institute, 2023), assuming 1.6 tons of wheat per hectare, and a harvest index (HI) of 0.8. The HI is the ratio of wheat crop yield to the combined yield of wheat straw and wheat crop (Agegnehu et al., 2012).
To determine the amount of inorganic fertilizer needed per land area, we consulted a report by Mussei et al. (2001), which recommended the use of 41 kg per ha (18.9 kg N per ha) of urea and 57 kg/ha (10.3 kg N per ha) of diamine phosphate. The nitrogen (N) content required to produce the estimated amount of crop residue was then computed. Once the fertilizer quantity was determined, the N2O emissions from fertilizer application were estimated using equations 11.2, 11.9, and 11.1 from the IPCC (2019) guidelines. These equations provided us with the direct and indirect N2O emissions associated with the application of inorganic fertilizer.
Additionally, we calculated the CO2 emissions resulting from the production of the inorganic fertilizer. This estimation was based on the quantity of nitrogen (kg N) used in wheat crop residue production. We multiplied this quantity by the emission factor per kilogram of nitrogen in inorganic fertilizer, computed from the emission factor of 1.26 kg CO2 per kg fertilizer (GREET®, 2017). The emissions from pesticides were not accounted for due to a lack of data on pesticide use specific to the context being analyzed.
2.2.1.4 Emission intensity
Emissions of GHGs were estimated for animals with abortions and without abortions for indigenous and exotic animals and were expressed as kg CO2 equivalents (CO2 eq) per kg fat-and-protein-corrected milk (FPCM), which is calculated as milk production standardized to fat and protein content of the respective animal (International Dairy Federation, 2015). Emissions from different sources were summed based on their equivalent factor: 1 for CO2, 27 for CH4, and 273 for N2O (100-year time horizon; Forster et al., 2021). Emissions intensities were expressed to a functional unit of 1 kg of fat and protein-corrected milk (FPCM).
Where “kg FPCM” represents the fat-protein-corrected milk yield in kilograms; “Milk kg” refers to the weight of the milk produced, measured in kilograms; 0.1226 represents the estimated conversion factor or weightage given to the fat content in determining the FPCM value; “Fat %” represents the percentage of fat in the milk; 0.0776 represents the estimated conversion factor or weightage given to the protein content in determining the FPCM value; and “Protein %” represents the percentage of protein in the milk; 0.2534 is a constant value that represents the contribution of factors other than fat and protein to the FPCM value.
2.2.2 Milk loss associated with abortion
The milk loss resulting from abortion was determined by calculating the average daily milk yield difference between animals that aborted and those that had a live birth. To estimate the total milk loss associated with abortion, the average daily milk yield difference reported by Semango et al. (2024) is multiplied by the lactation period within a specific calving or kidding interval.
2.2.3 Carcass loss associated with abortion
The study estimated the potential carcass loss or yield that could have been obtained from the aborted fetus if it had reached maturity. The calculation considered 25% calf mortality, 9% adult cattle mortality, 20% kid mortality, and 8% adult goat mortality derived from Baltussen et al. (2020). This estimation was made by multiplying the average slaughter weight by the dressing percentage (shown in Table 2) and then further multiplying the result by the observed number of abortions. Assuming an equal male-to-female ratio for the aborted fetuses, the average carcass weight was assumed to be the average slaughter weight of both female and male cattle. To convert the carcass into protein, a meat yield of 85.0% (Mummed and Webb, 2019) and meat crude protein (CP) content of 21.0% (on a wet basis; Muchenje et al., 2008) were considered.
2.2.4 Impact of milk and meat loss caused by abortion at the national level
To obtain a comprehensive understanding of the impact of milk and carcass loss associated with abortions, we extended our estimates to a national livestock population of the category of livestock studied as well as animal protein consumption levels. By extrapolating these estimates, we aimed to provide insights into the magnitude of the losses experienced by the entire human population, allowing us to conclude on a broader scale. At the national level, the abortion numbers were estimated as follows: 102,147 for indigenous cattle, 11,759 for exotic cattle, 558,022 for indigenous goats, and 6,475 for exotic goats (Semango et al., 2024). We assumed a daily per capita protein consumption of 10 g and an annual per capita meat and milk protein consumption of 3.65 kg, which were derived from data obtained from the Tanzania Bureau of Statistics in 2019.
2.3 Data on calf mortality
The data on calf mortality was collected in western Kenya between 2007 and 2009 as part of the Infectious Diseases of East Africa Livestock (IDEAL) project.1 The study area (Figure 2) covered Busia, Bugoma, Kakamega, and Siaya counties. It considered 20 sub-locations within each district, representing the smallest administrative units in Kenya with available cattle data.
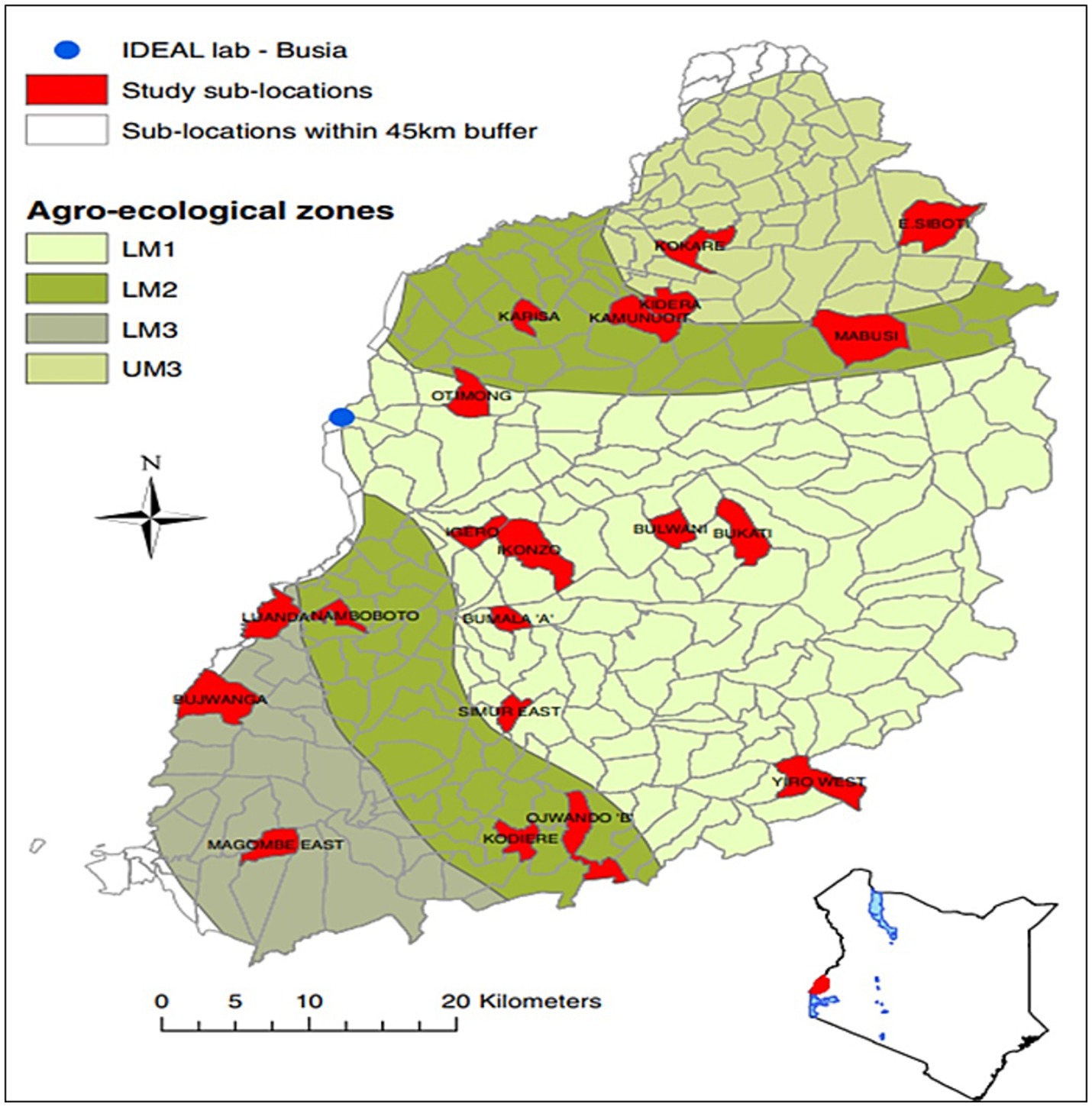
Figure 2. Map of the study area in Kenya. Source: de Clare Bronsvoort et al. (2013). Licensed under Creative Commons Attribution (http://creativecommons.org/licenses/by/2.0).
Detailed information on the data collection methods employed can be found in (de Clare Bronsvoort et al., 2013). In summary, the study targeted indigenous African Shorthorn Zebu calves and their causes of death. Within each of the 20 selected sub-locations, 28 calves were randomly chosen to achieve a minimum sample size of 500 calves. The selection criteria included age (3–7 days), natural birth, and non-zero-grazing conditions. Recruitment took place over a 5-week cycle, visiting 4 sub-locations per week, spanning 3 years. A reporting pathway was established from farmers to the IDEAL Office through sub-location chiefs and sub-chiefs. At each visit, calves were subjected to weight measurements, blood sampling, and fecal samples to screen for pathogens. The IDEAL staff attended dead calves to get clinical history, conduct a post-mortem examination and collect samples for further analysis to determine the cause of death. Information on the dams of the recruited calves had also been collected, including parity and heart girth.
Several useful variables, including the average weaning age of 340 days, a male-to-female calf ratio of 52:48, a calf mortality rate of 16%, and a calving interval of 1.3 years were retrieved from the study or the database. These variables were included in the enteric methane, EI, and carcass loss estimation. These data were supplemented by additional information primarily sourced from national studies whenever available. These included variables such as dressing percentage, mature live weight, age at attaining mature weight, average age at first calving, and calving interval (Table 3).
2.3.1 Estimation of enteric methane emission intensity from the calf mortality data
The study aimed to estimate EI from calf mortality in meat production. The methodology incorporated primary data collected through cross-sectional and longitudinal studies and supplemented with relevant information obtained from literature sources (Table 3). In cases where country-specific data was unavailable, default values for low-production systems in Africa from the IPCC (2019) were utilized. The calves were finished at 24 months at 288 kg for females and 294 kg for males as shown in Table 3 above, and the cattle are finished on pasture.
To provide a comprehensive assessment of enteric emissions and EI, we employed the “animal life and production loss (ALPL)” approach. This approach considers both the enteric emissions and production losses associated with the entire lifespan of the animals, before their slaughter. After removing outliers based on live weight, the model started with 523 cows (dams) over 10 years, encompassing 5 parities, which represents the lifespan of short horn zebu beef dams reared under pasture conditions (Rege et al., 2001). It was assumed that these cows were either slaughtered or sold for meat purposes after completing 5 parities. The newborn calves from each calving were raised until they reached finishing age. The quantity of beef carcass produced (measured in kilograms) was calculated based on the number of cattle slaughtered (dams, finished bulls, and finished heifers), their respective slaughter weights, and the dressing percentage (Table 3).
2.3.1.1 Methane emission from enteric fermentation
The estimation of enteric methane emission was conducted according to IPCC (2019) as described for the abortion data. The following steps were followed to estimate the enteric emission using the ‘ALPL’ approach.
1. Total enteric CH4 emissions were estimated for the 523 dams at each development stage until the time of slaughter (five parities or four lactations). These stages include birth to weaning, weaning to mating, first gestation, and four calving intervals. The emissions from all these stages were summed to determine the total lifetime emissions of individual dams.
2. The total enteric emissions were calculated for surviving male and female calves that were maintained until slaughter (finishing). Emissions were calculated from birth to finishing. This value was then multiplied by five to account for the calves produced during the dam’s lifetime. All calves that survived the first year were assumed to survive until finishing age.
3. For each male and female dead calf, enteric emissions from birth to death were estimated. This value was also multiplied by five to account for the five parities in the dam’s lifetime, assuming a constant calf mortality rate for all parties.
4. The total lifetime enteric emissions of dams and calves were obtained by summing the emissions estimated in steps 1–3.
2.3.1.2 Emission intensity
The enteric CH4 EI (kg CO2 eq per kg carcass) was estimated by dividing the total lifetime enteric CH4 emissions by the total carcass weight. The total carcass weight (kg) produced by dams, finishing male calves, and finishing female calves was estimated, taking into account the slaughter weight and dressing percentage. The total lifetime enteric emissions of dams and calves were converted to kg CO2 eq according to Forster et al. (2021) as described in the abortion data.
The above approach to estimating the enteric CH4 EI in meat production was applied to four scenarios (one business-as-usual (BAU) and three alternatives) as shown in Table 4. The baseline scenario (BAU) was based on the observed calf mortality rate of 16%. The alternative scenarios were based on hypothetical calf mortality rates of 8, 4, and 0% for scenarios 1, 2, and 3, respectively. For all scenarios, we assumed a similar herd size, herd structure (number of dams, male-to-female calf ratio), male and female slaughter weights, and dressing percentage.
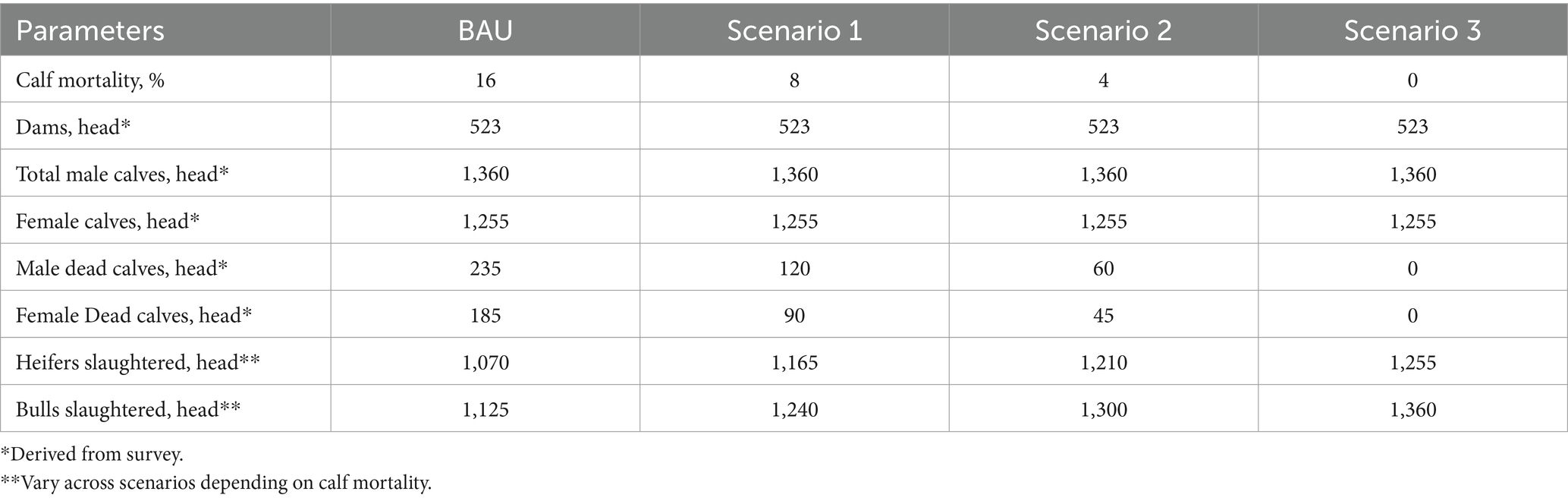
Table 4. Animal performance and inputs for scenarios used to estimate Greenhouse gas emission intensities from beef cattle operations in the lifetime of the cow.
2.3.2 Carcass loss from calf mortality data
To estimate the carcass and protein loss due to calf mortality, an analysis was made considering different calf mortality scenarios. The quantity of carcass lost was calculated by multiplying the number of dead calves with their mature weight and dressing percentage (as shown in Table 3). To convert the carcass into protein, a meat yield of 85.0% (Mummed and Webb, 2019) and meat CP content of 21.0% (on a wet basis; Muchenje et al., 2008) were considered.
2.3.3 Impact of meat loss caused by calf mortality at the national level
To obtain a comprehensive understanding of the impact of carcass/meat loss associated with calf mortality, we extended our estimates to a national livestock population of the category of livestock studied as well as animal protein consumption levels. The population of cows, which is 4,070,464, utilized for extrapolating the loss to the national level, was obtained from the State Department of Livestock (2024). This way, the magnitude of the losses experienced by the entire population is shown. We considered a per capita crude protein consumption of 5.65 g per year, as reported by Groot et al. (2023).
2.4 GLEAM-i assessment
Using the Global Livestock Environmental Assessment Model-interactive (GLEAM-i), we carried out an additional assessment to look at the tradeoffs in terms of sources of different GHGs. This is made to ascertain the share of individual GHGs in the total emissions in both cases. The computations were carried out over 1 year for the datasets from both countries.
3 Results
3.1 Greenhouse gas emission intensity of milk in Tanzania
A comparison was conducted to assess the EI of milk in indigenous and exotic cows, considering cases with and without abortion (Table 5). For indigenous cattle with no abortion (IC-NAB), the EI of milk was 3.3 kg CO2 eq per kg FPCM. This value increased by 4% for IC-AB. In the case of exotic cattle with no abortion (EC-NAB), the EI was lower at 1.16 kg CO2 eq per kg FPCM, while it increased by 15% in exotic cattle with abortion (EC-AB).
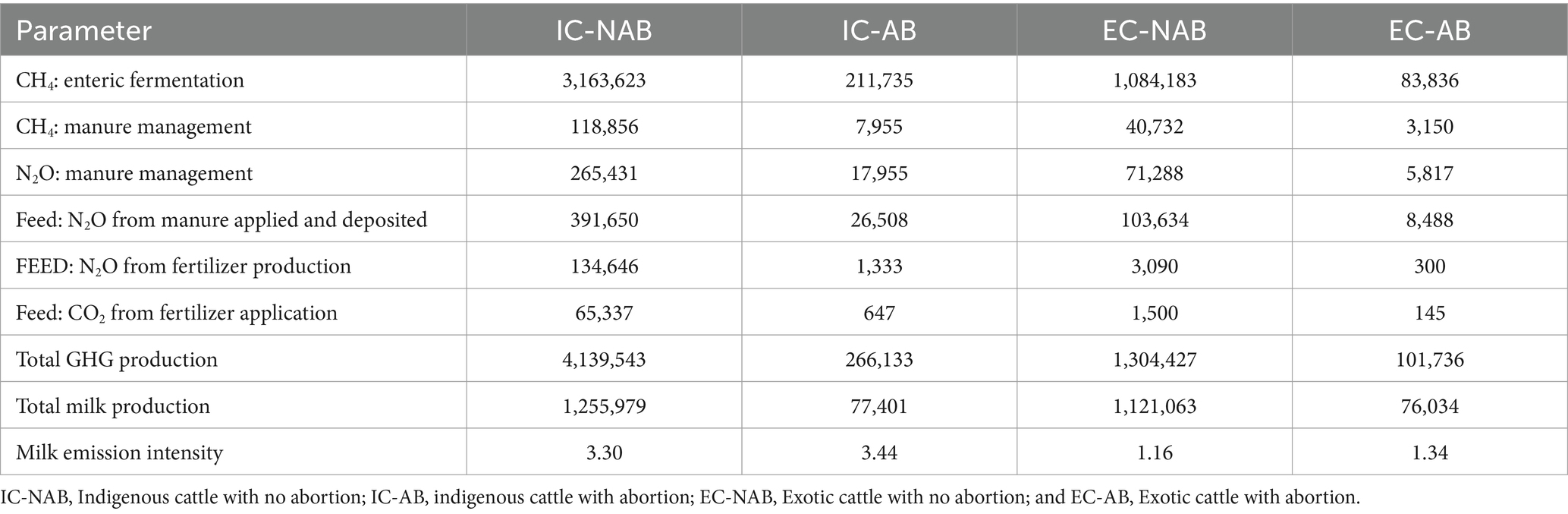
Table 5. Comparison of emissions and emission intensity of milk (kg CO2 eq per kg FPCM) between indigenous and exotic cattle without abortion and with abortion.
Table 6 presents a comparison of the EI of milk between indigenous and exotic goats with and without abortion. The EI is highest for IG-AB at 5.2 kg CO2 eq per kg FPCM, followed by IG-NAB at 4.86 kg CO2 eq per kg FPCM. EG-NAB has a lower EI at 1.9 kg CO2 eq per kg FPCM, and the EI increased by 18% for EG-AB.
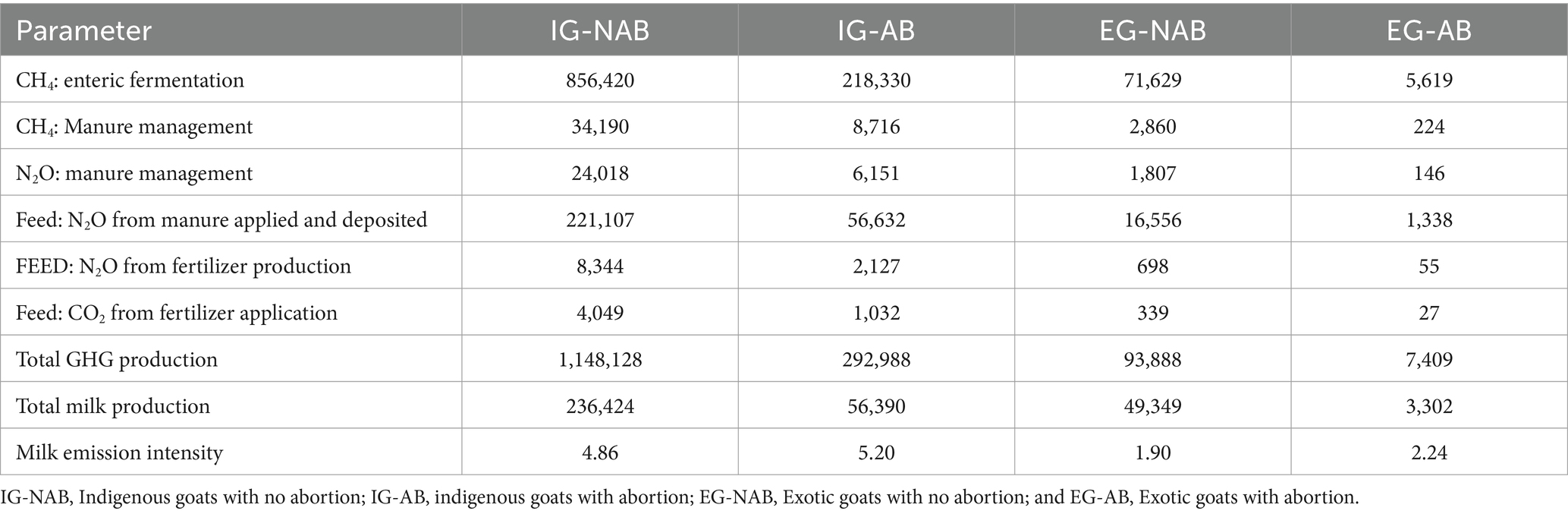
Table 6. Comparison of emissions and emission intensity of milk (kg CO2 eq per kg FPCM) between indigenous and exotic goats with and without abortion.
3.2 Carcass and milk loss due to abortion in Tanzania
Table 7 provides the results of milk loss associated with abortion. For indigenous cattle, the milk loss per day is 0.33 kg, resulting in a milk loss of 94.8 kg per lactation period of 285 days. At the national level, the estimated milk loss associated with abortion in indigenous cattle is 9,687 metric tons per year. For exotic cattle, the milk loss per day is 6.73 kg, leading to a milk loss of 1,918.5 kg per lactation period of 285 days. The estimated milk loss at the national level for exotic cattle is 22,560 metric tons per year. In the case of indigenous goats, the milk loss per day is 0.03 kg, resulting in a milk loss of 4.2 kg per lactation period of 150 days. The estimated milk loss at the national level for indigenous goats is 2,342 metric tons per year. For exotic goats, the milk loss per day is 0.42 kg leading to a milk loss of 69.3 kg per lactation period of 150 days. The estimated milk loss at the national level for exotic goats is 448 metric tons per year. The total milk loss associated with abortion, considering all animal categories, is 35,038 metric tons per year. This is equivalent to a loss of 1,230 metric tons of milk protein.
Table 8 provides the results of carcass loss associated with abortion. The table includes the slaughter weight for each animal category and the estimated carcass loss at the national level. For indigenous and exotic cattle, the estimated carcass losses at the national level are 3,993 and 739 metric tons, respectively. In the case of indigenous and exotic goats, the estimated carcass losses are 1,620 and 18 metric tons, respectively. The total estimated carcass loss associated with abortion, considering all animal categories, is 6,373 metric tons. This is equivalent to 1,137 metric tons of meat protein lost over a year.
Table 9 reveals that animal protein loss associated with abortion accounts for the potential animal protein requirements of approximately 649 thousand people in Tanzania per year. This assumes the current per capita consumption of animal protein of 3.65 kg per capita per year. These results demonstrate the significant impact of preventing milk and meat losses on protein availability and potential access to meat and milk protein.
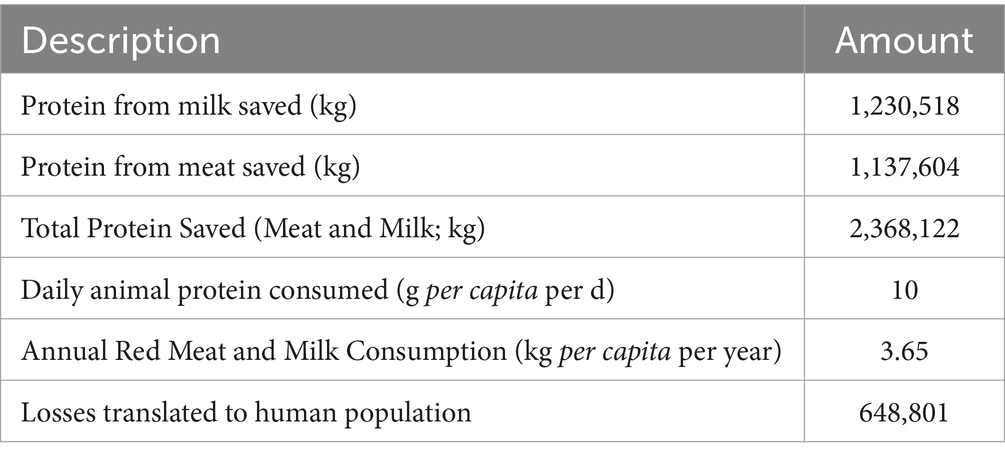
Table 9. Summary of protein loss and consumption data in Tanzania associated with livestock abortions.
3.3 Enteric methane emission intensity of meat production in Kenya
The total lifetime CH4 emission for the BAU scenario was approximately 12 million kg CO2 eq (Table 10). Scenarios (1–3) resulted in increased total emissions with increasing calf survival, compared with BAU.
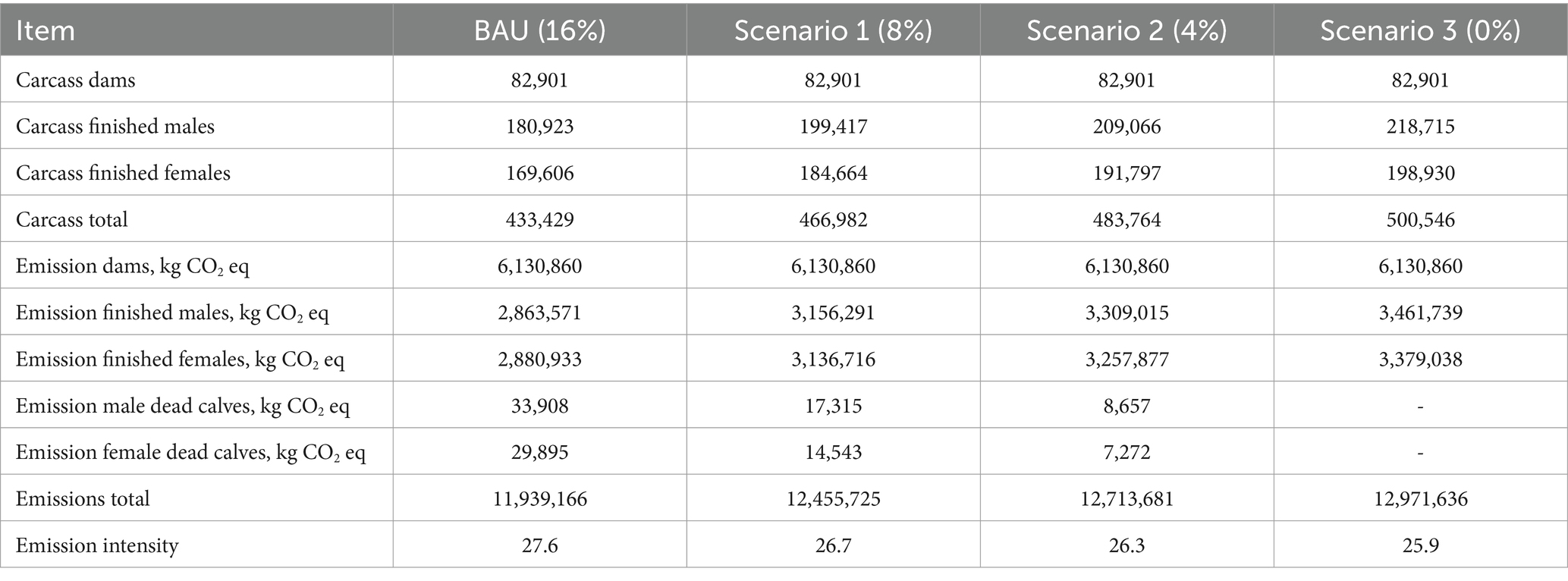
Table 10. Total carcass production (kg), total enteric methane emissions (kg CO2 eq), and enteric methane emission intensity (kg CO2 eq per kg carcass).
Emission intensities for BAU and alternative scenarios ranged from 25.9–27.6 kg CO2 eq per kg carcass weight carcass (Table 10). Reducing calf mortality from 16 to 8%, 4, and 0% resulted in a reduction of EI by 3.2, 4.6 and 5.9%, respectively.
3.4 Loss of animal protein due to calf mortality in Kenya
The results presented in Table 11 illustrate the estimated impact of protein loss associated with calf mortality in Kenya. The animal protein loss with a 16% calf mortality rate is translated to losses equivalent to annual per capita consumption by 4.5 million people, assuming a beef CP consumption of 2,064 g per capita per year. When the calf mortality rate decreases to 8%, it is translated to losses equivalent to per capita consumption by 2.2 million people, indicating a significant improvement in protein availability for the population. With a calf mortality rate of 4%, it is translated to losses equivalent to the annual per capita consumption of 1.1 million people. This reflects a consistent decrease in losses translated to per capita consumption across the population.
3.5 Emission trade-offs using GLEAM-i
In Tanzania, the total GHG emissions were lower in the group that experienced abortion compared to the group that did not, for both cattle and goats. Among the various emission sources, enteric fermentation stood out as the largest contributor, accounting for approximately 87% of the total GHG emissions. The second most significant contributor was manure emissions, with N2O and CH4 being responsible for about 34–35 and 3% of the emissions, respectively. A similar trend was observed in Kenya for all scenarios of calf mortality, except that the N2O emissions from manure were slightly lower, accounting for 30% of the total emissions.
4 Discussion
The impact of breed and abortion on milk EI was investigated in the study, with noteworthy findings. Using this methodology, exotic breeds were found to have lower estimated EI in milk production compared to indigenous breeds. This can be attributed to factors such as their higher milk production potential and greater feed conversion efficiency if better diets are fed to these breeds, both of which lead to lower CH4 production per unit of milk, as indicated by FAO and NZAGRC (2017). The study placed particular emphasis on the significant role of mean milk yield in influencing EI. It demonstrated that an increase in milk yield per cow resulted in a decrease in EI. These findings aligns with a separate study conducted by Ndung'u et al. (2022) in Kenya, which highlighted that the average milk yield per animal, rather than milk production per farm, was the primary factor influencing EI. Consistent with the findings from the abortion data, Ndung'u et al. (2022) found that an increase in milk yield was associated with a reduction in EI.
The findings demonstrated that abortion increased EI in both indigenous and exotic animals. The rise in EI is associated with the reduction in milk yield resulting from abortion. Previous studies have indicated that abortion leads to marked reductions in total and daily milk yield (Gädicke et al., 2010; El-Tarabany, 2015). The increase in EI is more pronounced in exotic breeds, with a 15% increase in cows and an 18% increase in goats, compared to a 6% increase in cows and a 7% increase in goats for indigenous breeds. This increase in EI is a result of the comparatively larger decrease in total milk production due to abortion, with a 30% decrease in cows and a 26% decrease in goats for exotic breeds, as opposed to a 10% decrease in cows and an 8% decrease in goats for indigenous breeds. It is worth highlighting that there are relatively fewer exotics within the local study livestock population, so we do have to be more careful generalizing the estimates. Despite this, the higher decrease in milk yield observed in exotic breeds is consistent with the findings of Keshavarzi et al. (2020), who reported a reduction of milk yield by 19% in Holstein cows as a result of abortion. Indigenous breeds often have lower milk production potential compared to exotic breeds (Gebreyohanes et al., 2021). When abortion occurs in indigenous breeds, the absolute decrease in milk yield may be comparatively less pronounced due to their lower baseline milk production. For instance, in the case of cows, the absolute decrease in daily milk yield may be from 2.5 to 2.2 liters, while in goats, it may be decreased from 0.25 to 0.23 liters. These smaller absolute decreases can be attributed to the fact that indigenous breeds typically have lower initial levels of milk production compared to higher-yielding exotic breeds.
As increased levels of milk production are associated with increases in GHG emissions it may seem logical to assume that abortion which leads to lower milk production would result in reduced emissions (Skuce et al., 2016; Keshavarzi et al., 2020). However, this idea may give the impression that abortion will also lead to lower EI of products, but this holds true if we assume that abortion results in reduced feed consumption (De Vries, 2006). These resources contribute to the overall emissions of the system. Consequently, the EI of milk produced in cases with abortion can be increased due to the lower milk yield associated with the abortion.
The timing of abortion during the gestation period also significantly impacts the emission profile. According to Keshavarzi et al. (2020), the stage of pregnancy when abortion occurs has a profound effect on production performance. For instance, early abortions where the ongoing lactation remains uninterrupted and the nutritional requirements to support the developing fetus are relatively small (Rhind, 2004), have a smaller environmental footprint compared to late-term abortions. Therefore, generalizing the observed EI reduction associated with abortion without considering timing can be misleading.
In the Tier 2 methodology for estimating enteric CH4 emissions, the Ym, which represents the percentage of feed energy converted to CH4, does not currently account for the effects of abortions. Özkan et al. (2022) suggest that the health status of animals can influence their energy requirements. Considering this finding, it becomes necessary to conduct further research to investigate the potential impact of abortions on feed intake. By exploring this aspect, we can enhance the accuracy of estimating enteric CH4 emissions and better understand the relationship between reproductive health and methane production in animals.
The implications of reduced calf mortality on enteric CH4 EI in beef production were also examined. The findings from the analysis using the IDEAL study data in Kenya demonstrated a decrease in enteric CH4 EI with lower calf mortality rates, highlighting the importance of addressing this issue. One of the primary drivers behind the observed reduction in EI is the reduction of “unproductive emissions” (Gerber et al., 2013; FAO and NZAGRC, 2017). This can be due to when calves die, the emissions associated with their conception, growth in the dam, and upbringing remain, while their potential meat production (carcass weight) is lost. As a result, scenarios with higher calf mortality rates exhibit a higher EI. Previous research by Samsonstuen et al. (2020) supports this finding, as they reported that reduced calf mortality from 3.6 to 0% reduced the enteric CH4 EI by 3.7%. Moreover, Samsonstuen et al. (2020) revealed a reduction in EI by 11.2% by implementing a combination of strategies aimed at reducing calf mortality by 10.8%, alongside other mitigation measures, such as improving female fertility, in Norwegian beef cattle herds. These findings provide compelling evidence for the role of reducing “unproductive emissions” in reducing enteric CH4 EI in beef production.
It is essential to recognize that the timing of calf mortality plays a pivotal role in determining the magnitude of “unproductive emissions” and, consequently, the EI. In the pre-ruminant phase, when calves primarily consume milk, enteric CH4 emissions are assumed to be negligible according to the IPCC (2019). As a result, mortality during this phase may have a relatively smaller direct impact on enteric CH4 emissions compared to post-weaning mortality. The direct impact on enteric CH4 emissions is expected to be more pronounced after the calves have transitioned to a solid feed-based diet and actively ferment feed in the rumen. These considerations should be taken into account when implementing strategies to reduce calf mortality and mitigate EI.
Reducing calf mortality can be achieved by enhancing dam and calf health. In the IDEAL dataset, more than 80% of diagnosed calf deaths in the study were associated with infectious diseases (de Clare Bronsvoort et al., 2013). Hence, calf health can be improved through various management practices, such as good hygienic practices, vaccination, and enhanced veterinary services such as diagnostics, to minimize the risk of infections (Murray et al., 2016) and improve disease control and treatment outcomes. Reducing calf mortality often comes with additional costs, necessitating careful consideration of the economic feasibility for individual producers. Implementing improved management strategies can be costly. Therefore, conducting not only studies on calf deaths but also cost–benefit analyses, as suggested by Nganga et al. (2020), is crucial to determine the optimal strategies and investment levels for different contexts.
Livestock production plays a crucial role in food security and nutritional well-being, particularly in LMICs (Idamokoro, 2023). However, the challenges of abortion and calf mortality can significantly hinder the full potential of this sector (Skuce et al., 2016). The study conducted in Tanzania and Kenya sheds light on the extensive consequences of these issues, particularly in terms of protein availability and overall food security.
Previous studies demonstrated the impact of abortion on animal protein losses (Kardjadj, 2018; Keshavarzi et al., 2020). The present study also depicted the protein losses associated with livestock abortions. The implications for food security are evident (Alemayehu et al., 2021), as the results revealed that preventing abortions could provide the potential annual animal protein requirements of approximately 649 thousand people in Tanzania. These findings emphasize the critical role that interventions targeting abortions can play in addressing dietary deficiencies (African Union Commission, 2015).
The study in Kenya revealed how calf mortality affects protein access at the national level. A 16% calf mortality rate is translated to protein loss equivalent to annual per capita consumption by 4.5 million people. These findings are consistent with previous research by Prachurja (2023), which highlighted the economic losses for farmers and the broader nutritional concerns associated with calf mortality. Therefore, the scenarios with lower calf mortality rates (8, 4, and 0%) result in a significant decrease in losses translated to per capita consumption across the population. This highlights the importance of implementing strategies to improve calf survival. Policymakers and stakeholders in agriculture and livestock management must take note of these findings and invest in targeted interventions to safeguard both the livelihoods of farmers and the protein security of vulnerable populations. In addition to the potential benefit from increased protein supply and its general contribution to food security, abortion, and calf mortality can contribute to other Sustainable Development Goals (SDGs). Reducing food loss and waste is one of the targets of the SDGs.2 The Food and Agricultural Organization demonstrated how big the contribution of mortalities during breeding to global estimates of food loss was estimated to be (Gustafsson et al., 2013).
The findings from the GLEAM-i assessment open up research opportunities for exploring innovative technologies and practices to reduce emissions from enteric fermentation and manure management. Continued research and development in these areas will contribute to sustainable livestock production and the overall goal of mitigating climate change. The findings highlight the importance of considering the systems perspective when evaluating the environmental impact of livestock production.
5 Limitations
The study faces several limitations primarily due to the reliance on datasets initially intended to assess livestock abortions and calf mortality, which were later adapted to evaluate GHG emissions. This repurposing presents challenges in managing data heterogeneity, ensuring consistency, and addressing generalizability. Differences in the data sources make it difficult to achieve uniform analysis, especially when combining multiple datasets with varying collection methods and variables. To address missing data, IPCC default values or country-specific estimates from the literature were often used, which may limit methodological transparency and the accuracy of findings. These limitations could affect the reliability of the results and pose challenges for updating data sources and refining the analysis in future studies.
6 Conclusion
In conclusion, addressing abortions and calf mortality presents opportunities to reduce GHG EI and increase food security. The present study focused in Tanzania and Kenya, and it is essential to conduct further research to assess the generalizability of these findings to other contexts and livestock populations. Furthermore, a more detailed roadmap for potential interventions is needed, including cost–benefit analyses of specific strategies tailored to regional contexts and animal breeds. Such analyses can pave the way for the effective implementation of interventions aimed at preventing abortions and reducing calf mortality, thereby enhancing food security and protein availability in livestock production.
Data availability statement
The raw data supporting the conclusions of this article will be made available by the authors, without undue reservation.
Author contributions
EG: Conceptualization, Formal analysis, Methodology, Writing – original draft. BB: Conceptualization, Funding acquisition, Methodology, Writing – review & editing. EC: Conceptualization, Funding acquisition, Methodology, Writing – review & editing. FL: Methodology, Writing – review & editing. ŞÖ: Conceptualization, Formal analysis, Methodology, Writing – review & editing. PR: Conceptualization, Funding acquisition, Methodology, Writing – review & editing. GS: Conceptualization, Methodology, Writing – review & editing. NW: Conceptualization, Methodology, Writing – review & editing. AW: Conceptualization, Writing – review & editing. CA: Conceptualization, Formal analysis, Funding acquisition, Methodology, Writing – review & editing.
Funding
The author(s) declare that financial support was received for the research, authorship, and/or publication of this article. Funding for this work was obtained from the following sources: Environmental Defense Fund, with support from the Wellcome Trust (226478/Z/22/Z). Bill & Melinda Gates Foundation: UK aid from the UK Foreign, Commonwealth and Development Office (Grant Agreement INV-040641) through the Centre for Tropical Livestock Genetics and Health (CTLGH), established jointly by the University of Edinburgh, SRUC (Scotland’s Rural College), and the International Livestock Research Institute. Mitigate+: Research for Low Emissions Food Systems and Livestock and Climate CGIAR research initiatives supported by contributors to the CGIAR Trust Fund. In addition, Barend Brons Voort received BBSRC core funding through the Institute Strategic Program Grant (BBS/E/RL/230002D).
Acknowledgments
The authors would like to thank all funders who support this research through their contributions to the CGIAR Trust Fund: www.cgiar.org/funders. The findings and conclusions presented in this work are solely those of the authors and do not necessarily reflect the positions or policies of the funders mentioned above, including the Bill & Melinda Gates Foundation and the UK Government. ChatGPT-4 (OpenAI) was used for editorial purposes, assisting in refining language and structure within this manuscript.
Conflict of interest
The authors declare that the research was conducted in the absence of any commercial or financial relationships that could be construed as a potential conflict of interest.
The author(s) declared that they were an editorial board member of Frontiers, at the time of submission. This had no impact on the peer review process and the final decision.
Publisher’s note
All claims expressed in this article are solely those of the authors and do not necessarily represent those of their affiliated organizations, or those of the publisher, the editors and the reviewers. Any product that may be evaluated in this article, or claim that may be made by its manufacturer, is not guaranteed or endorsed by the publisher.
Footnotes
References
African Union Commission (2015). The livestock development strategy for Africa (LiDeSA) 2015–2035: The roadmap to a successful livestock sector. Nairobi, Kenya: African Union- Interafrican Bureau for Animal Resources (AU-IBAR).
Agegnehu, G., Tsigie, A., and Tesfaye, A. (2012). Evaluation of crop residue retention, compost and inorganic fertilizer application on barley productivity and soil chemical properties in the central Ethiopian highlands. Eth. J. Agric. Sci. 22, 45–61.
Alemayehu, G., Mamo, G., Alemu, B., Desta, H., Tadesse, B., Benti, T., et al. (2021). Causes and flock level risk factors of sheep and goat abortion in three agroecology zones in Ethiopia. Front. Vet. Sci. 8:615310. doi: 10.3389/fvets.2021.615310
Asimwe, L., and Kifaro, G. (2007). Effect of breed, season, year and parity on reproductive performance of dairy cattle under smallholder production system in Bukoba district, Tanzania. Livest. Res. Rural. Dev. 19, 1–9.
AU-IBAR (2019). Kenya livestock breeds catalogue. Ministry of Agriculture, Livestock, Fisheries and Cooperatives State Department of Livestock: AU-IBAR.
Baltussen, W., van Berkum, S., Dijkxhoorn, Y., Helmes, R., Ozkan-Gulzari, S., Vellinga, T. V., et al. (2020). Traditional livestock systems in Tanzania: An application of the TEEB framework. UNEP TEEB. Available at: http://teebweb.org/wp-content/uploads/2020/09/TEEBAgriFood-Tanzania-livestock-LC-final.pdf
Chenyambuga, S., Komwihangilo, D., and Ndemanisho, E. (2010). "Development of management practices for sustainable improvement of indigenous goats in Tanzania", in Second RUFORUM biennial regional conference on “building capacity for food security in Africa”, Entebbe, Uganda, 20–24 September 2010. pp. 1139–1145.
de Clare Bronsvoort, B. M., Thumbi, S. M., Poole, E. J., Kiara, H., Auguet, O. T., Handel, I. G., et al. (2013). Design and descriptive epidemiology of the infectious diseases of east African livestock (IDEAL) project, a longitudinal calf cohort study in western Kenya. BMC Vet. Res. 9, 1–22. doi: 10.1186/1746-6148-9-171
de Glanville, W. A., Davis, A., Allan, K. J., Buza, J., Claxton, J. R., Crump, J. A., et al. (2020). Classification and characterisation of livestock production systems in northern Tanzania. PLoS One 15:e0229478. doi: 10.1371/journal.pone.0229478
De Vries, A. (2006). Economic value of pregnancy in dairy cattle. J. Dairy Sci. 89, 3876–3885. doi: 10.3168/jds.S0022-0302(06)72430-4
Deresa, B., Tulu, D., and Deressa, F. B. (2020). Epidemiological investigation of cattle abortion and its association with brucellosis in Jimma zone, Ethiopia. Vet. Med. (Auckl.) 11, 87–98. doi: 10.2147/VMRR.S266350
Durojaye, O., Laseinde, T., and Oluwafemi, I. (2020). “A descriptive review of carbon footprint” in Human systems engineering and design II. IHSED 2019. Advances in intelligent systems and computing. eds. T. Ahram, W. Karwowski, S. Pickl, and R. Taiar (Cham: Springer International Publishing), 960–968.
El-Tarabany, M. S. (2015). Impact of stillbirth and abortion on the subsequent fertility and productivity of Holstein, Brown Swiss and their crosses in subtropics. Trop. Anim. Health Prod. 47, 1351–1356. doi: 10.1007/s11250-015-0870-z
FAO (2023). Pathways towards lower emissions: a global assessment of the greenhouse gas emissions and mitigation options from livestock agrifood systems. Rome: FAO.
FAO and NZAGRC (2017). Supporting low emissions development in the Ethiopian dairy cattle sector: reducing enteric methane for food security and livelihoods.: Food and Agricultural Organization & New Zealand Agricultural Greenhouse Gas Research Centre
Forster, P., Storelvmo, T., Armour, K., Collins, W., Dufresne, J.-L., Frame, D., et al. (2021). “The Earth's energy budget, climate feedbacks, and climate sensitivity. In climate change 2021: the physical science basis” in Contribution of working group I to the sixth assessment report of the intergovernmental panel on climate change. eds. V. Masson-Delmotte, P. Zhai, A. Pirani, S. L. Connors, C. Péan, and S. Berger, et al. (Cambridge, United Kingdom and New York, NY, USA: Cambridge University Press), 923–1054.
Gädicke, P., Vidal, R., and Monti, G. (2010). Economic effect of bovine abortion syndrome in commercial dairy herds in southern Chile. Prev. Vet. Med. 97, 9–19. doi: 10.1016/j.prevetmed.2010.07.008
Gebreyohanes, G., Yilma, Z., Moyo, S., and Okeyo Mwai, A. (2021). Dairy industry development in Ethiopia: Current status, major challenges and potential interventions for improvement. Nairobi, Kenya: International Livestock Research Institute.
Gerber, P. J., Henderson, B., and Makkar, H. P. (2013). Mitigation of greenhouse gas emissions in livestock production: A review of technical options for non-CO2 emissions. Rome, Italy: Food and Agriculture Organization of the United Nations (FAO).
Goopy, J. P., Onyango, A. A., Dickhoefer, U., and Butterbach-Bahl, K. (2018). A new approach for improving emission factors for enteric methane emissions of cattle in smallholder systems of East Africa–Results for Nyando, Western Kenya. Agric. Syst. 161, 72–80. doi: 10.1016/j.agsy.2017.12.004
Graham, M. W., Butterbach-Bahl, K., du Doit, C. L., Korir, D., Leitner, S., Merbold, L., et al. (2022). Research progress on greenhouse gas emissions from livestock in sub-Saharan Africa falls short of national inventory ambitions. Front. Soil Sci. 2:927452. doi: 10.3389/fsoil.2022.927452
GREET® (2017). Available at: https://greet.anl.gov/greet/versions.html (Accessed January 20, 2024).
Groot, J., Broeze, J., and Castelein, R. (2023). Food and nutrition security in Kibera (Nairobi, Kenya) with a focus on protein and amino acids : Wageningen Food & Biobased Research.
Gulliksen, S., Lie, K., Løken, T., and Østerås, O. (2009). Calf mortality in Norwegian dairy herds. J. Dairy Sci. 92, 2782–2795. doi: 10.3168/jds.2008-1807
Gustafsson, J., Cederberg, C., Sonesson, U., and Emanuelsson, A. (2013). The methodology of the FAO study: Global food losses and food waste-extent, causes and prevention”-FAO, 2011. SIK Institutet för livsmedel och bioteknik.
Henchion, M., Moloney, A., Hyland, J., Zimmermann, J., and McCarthy, S. (2021). Trends for meat, milk and egg consumption for the next decades and the role played by livestock systems in the global production of proteins. Animal 15:100287. doi: 10.1016/j.animal.2021.100287
Herrero, M., Havlik, P., McIntire, J., Palazzo, A., and Valin, H. (2014). African livestock futures: Realizing the potential of livestock for food security, poverty reduction and the environment in sub-Saharan Africa. Geneva, Switzerland: Office of the Special Representative of the UN Secretary General for Food Security and Nutrition and the United Nations System Influenza Coordination (UNSIC).
Idamokoro, E. M. (2023). The relevance of livestock husbandry in the context of food security: a bibliometric outlook of research studies from 1938 to 2020. Front. Sustain. Food Syst. 7:1204221. doi: 10.3389/fsufs.2023.1204221
International Dairy Federation (2015). A common carbon footprint approach for dairy: The IDF guide to standard lifecycle assessment methodology for the dairy sector. Bull. Int. Dairy Fed. Available at: http://www.fil-idf.org/EventsCalendar.htm. (Accessed November 4, 2023).
IPCC (2019). "Chapter 10: emissions from livestock and manure management," in 2019 refinement to the 2006 IPCC Guidelines for National Greenhouse Gas Inventories.Volume 4: Agriculture, Forestry and Other land Use. IPCC. 10.11–10.209.
Jackson, M., Chenyambuga, S., Ndemanisho, E., and Komwihangilo, D. (2012). Production performance of Toggenburg dairy goats in semi-arid and sub-humid areas of Tanzania. Live.s Res. Rural. Dev. 26:30.
Kardjadj, M. (2018). The epidemiology of cattle abortion in Algeria. Trop. Anim. Health Prod. 50, 445–448. doi: 10.1007/s11250-017-1430-5
Keshavarzi, H., Sadeghi-Sefidmazgi, A., Ghorbani, G. R., Kowsar, R., Razmkabir, M., and Amer, P. (2020). Effect of abortion on milk production, health, and reproductive performance of Holstein dairy cattle. Anim. Reprod. Sci. 217:106458. doi: 10.1016/j.anireprosci.2020.106458
Keshavarzi, H., Sadeghi-Sefidmazgi, A., Kristensen, A. R., and Stygar, A. H. (2017). Abortion studies in Iranian dairy herds: I. Risk factors for abortion. Livest. Sci. 195, 45–52. doi: 10.1016/j.livsci.2016.11.004
Lankester, F., Kibona, T.J., Allan, K.J., de Glanville, W., Buza, J.J., Katzer, F., et al. (2024). The value of livestock abortion surveillance in Tanzania: identifying disease priorities and informing interventions. bioRxiv doi: 10.1101/2024.01.07.574517 [Preprint].
Latino, L. R., Pica-Ciamarra, U., and Wisser, D. (2020). Africa: the livestock revolution urbanizes. Glob. Food Secur. 26:100399. doi: 10.1016/j.gfs.2020.100399
Leitner, S., Ring, D., Wanyama, G., Korir, D., Pelster, D., Goopy, J., et al. (2020). "Nitrous oxide and methane emissions from cattle manure heaps in Kenya", in EGU General Assembly Conference Abstracts. p. 21299.
MacLeod, M., and Moran, D. (2017). Integrating livestock health measures into marginal abatement cost curves. Rev. Sci. Tech.-Off. Int. Épizooties 36, 97–104. doi: 10.20506/rst.36.1.2613
Mruttu, H., Ndomba, C., Nandonde, S., and Brook, K. N. (2016). Animal genetics strategy and vision for Tanzania:Tanzania livestock master plan background paper. Nairobi, Kenya: Tanzania Ministry of Agriculture, Livestock and Fisheries and ILRI.
Msalya, G. M., Urassa, F., and Kifaro, G. (2021). Quality of milk from Norwegian dairy goats bred and raised in Mgeta division, Morogoro region, Tanzania, Tanz. J. Agric. Sci. 20, 54–62.
Muchenje, V., Dzama, K., Chimonyo, M., Raats, J., and Strydom, P. (2008). Meat quality of Nguni, Bonsmara and Aberdeen Angus steers raised on natural pasture in the eastern cape, South Africa. Meat Sci. 79, 20–28. doi: 10.1016/j.meatsci.2007.07.026
Mummed, Y., and Webb, E. C. (2019). Carcass weight, meat yield and meat cuts from Arado, Boran, Barka, Raya cattle breeds in Ethiopia. J. Agric. Sci. 11, 45–51. doi: 10.5539/jas.v11n18p45
Murray, C., Fick, L., Pajor, E., Barkema, H., Jelinski, M., and Windeyer, M. (2016). Calf management practices and associations with herd-level morbidity and mortality on beef cow-calf operations. Animal 10, 468–477. doi: 10.1017/S1751731115002062
Mussei, A., Mwanga, J., Mwangi, W., Verkuijl, H., Mongi, R., and Elanga, A. (2001). Adoption of improved wheat technologies by small-scale farmers in Mbeya District, Southern Highlands, Tanzania. Mexico,D.F: International Maize and Wheat Improvement Center (CIMMYT) and the United Republic of Tanzania.
Ndung'u, P. W., Takahashi, T., Du Toit, C. J. L., Robertson-Dean, M., Butterbach-Bahl, K., McAuliffe, G., et al. (2022). Farm-level emission intensities of smallholder cattle (Bos indicus; B. indicus–B. taurus crosses) production systems in highlands and semi-arid regions. Animal 16:100445. doi: 10.1016/j.animal.2021.100445
Nganga, S., Smith, G., Mwungu, C., Alemayehu, S., Girvetz, E., and Hyman, E. (2020). Cost-benefit analysis of improved livestock management practices in the Oromia lowlands of Ethiopia. Washington, DC: Crown Agents USA and Abt Associates, with the International Center for Tropical Agriculture (CIAT), USAID.
Özkan, Ş., Teillard, F., Lindsay, B., Montgomery, H., Rota, A., Gerber, P., et al. (2022). The role of animal health in national climate commitments. Rome, Italy: Food and Agricultural Organization (FAO).
Parvez, M., Faruque, M., and Khatun, R. (2020). Prevalence of abortion, calf mortality and proportion of cattle population in commercial dairy farms of Bangladesh. Res J. Vet. Pract. 8, 51–55. doi: 10.17582/journal.rjvp/2020/8.4.51.55
Prachurja, R.A. (2023). Calf mortality and its possible causes in chattogram district of Bangladesh. DVM thesis, Chattogram Veterinary and Animal Sciences University.
Rege, J. E. O., Kahi, A., Okomo-Adhiambo, M., Mwacharo, J., and Hanotte, O. (2001). Zebu cattle of Kenya: Uses, performance, farmer preferences, measures of genetic diversity and options for improved use. Kenya: International Livestock Research Institute.
Rhind, S. (2004). Effects of maternal nutrition on fetal and neonatal reproductive development and function. Anim. Reprod. Sci. 82, 169–181. doi: 10.1016/j.anireprosci.2004.04.003
Samsonstuen, S., Åby, B. A., Crosson, P., Beauchemin, K. A., and Aass, L. (2020). Mitigation of greenhouse gas emissions from beef cattle production systems. Acta Agric. Scand. A Anim. Sci. 69, 220–232. doi: 10.1080/09064702.2020.1806349
Schneider, F., and Tarawali, S. (2021). Sustainable development goals and livestock systems. Rev. Sci. Tech. Off. Int. Epiz. 40, 585–595. doi: 10.20506/rst.40.2.3247
Semango, G., Yoder, J., Kibona, T., Claxton, J. R., Buza, J., Mmbaga, B. T., et al. (2024). Economic burden of livestock abortions in northern Tanzania. J. Agric. Appl. Econ. 56, 195–215. doi: 10.1017/aae.2024.6
Shija, D. S., Mtenga, L. A., Kimambo, A. E., Laswai, G. H., Mushi, D. E., Mgheni, D. M., et al. (2013). Preliminary evaluation of slaughter value and carcass composition of indigenous sheep and goats from traditional production system in Tanzania. Asian-Aust. J. Anim. Sci. 26, 143–150. doi: 10.5713/ajas.2012.12431
Shirima, E. J., Nsiima, L. M., Mwilawa, A. J., Temu, J., Michael, S., and Mlau, D. D. S. (2016). Evaluation of slaughter and carcass characteristics from indigenous beef cattle in six abattoirs of Tanzania. J. Sci. Res. Rep. 10, 1–8. doi: 10.9734/JSRR/2016/22397
Skuce, P., Bartley, D., Zadoks, R., and Macleod, M. (2016). Livestock health and greenhouse gas emissions. Edinburgh, UK: Scotland's center of expertise connecting climate change research and policy.
State Department of Livestock (2024). Inventory of GHG Emissions from Non-dairy Cattle, Sheep & Goats, and Camel in Kenya [Manuscript in preparation]. Nairobi: State Department of Livestock.
Tanzania Agricultural Research Institute (2023). Wheat [Online]. Available at: https://www.tari.go.tz/index.php/tari-uyole/programmes/collaborative-research-/wheat (Accessed November 20, 2023).
Thomas, K. M., Kibona, T., Claxton, J. R., de Glanville, W. A., Lankester, F., Amani, N., et al. (2022). Prospective cohort study reveals unexpected aetiologies of livestock abortion in northern Tanzania. Sci. Rep. 12:11669. doi: 10.1038/s41598-022-15517-8
World Organization for Animal Health (2014). Impact of diseases on meat and milk [online]. Available at: https://rr-africa.woah.org/en/news/impact-of-diseases-on-meat-and-milk/ (Accessed December 5, 2023).
Keywords: abortion, calf mortality, GHG, methane, emission intensity, animal protein, food security, animal health
Citation: Gurmu EB, Bronsvoort B, Cook EAJ, Lankester F, Özkan Ş, Rosenstein PK, Semango G, Wheelhouse N, Wilkes A and Arndt C (2024) Environmental and food security implications of livestock abortions and calf mortality: a case study in Kenya and Tanzania. Front. Sustain. Food Syst. 8:1390047. doi: 10.3389/fsufs.2024.1390047
Edited by:
Jan Verhagen, Wageningen University and Research, NetherlandsReviewed by:
Kolawole Odubote, Zambian Open University, ZambiaBridget Bwalya, University of Zambia, Zambia
Copyright © 2024 Gurmu, Bronsvoort, Cook, Lankester, Özkan, Rosenstein, Semango, Wheelhouse, Wilkes and Arndt. This is an open-access article distributed under the terms of the Creative Commons Attribution License (CC BY). The use, distribution or reproduction in other forums is permitted, provided the original author(s) and the copyright owner(s) are credited and that the original publication in this journal is cited, in accordance with accepted academic practice. No use, distribution or reproduction is permitted which does not comply with these terms.
*Correspondence: Claudia Arndt, Y2xhdWRpYS5hcm5kdEBjZ2lhci5vcmc=