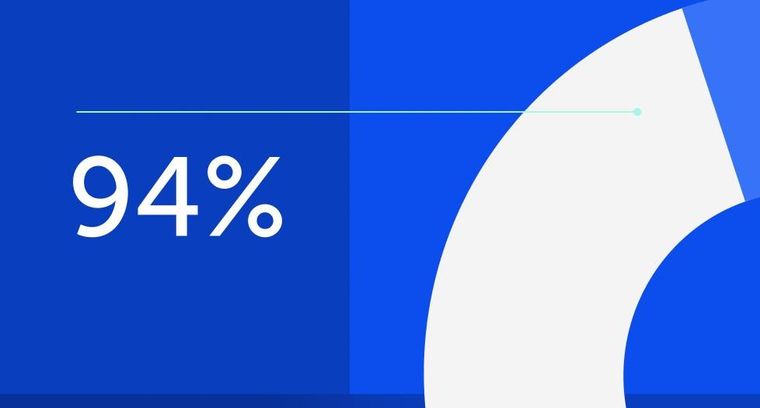
94% of researchers rate our articles as excellent or good
Learn more about the work of our research integrity team to safeguard the quality of each article we publish.
Find out more
ORIGINAL RESEARCH article
Front. Sustain. Food Syst., 04 April 2024
Sec. Crop Biology and Sustainability
Volume 8 - 2024 | https://doi.org/10.3389/fsufs.2024.1373183
This article is part of the Research TopicAbiotic Stresses in Field Crops: Response, Impacts and Management under Climate Change ScenarioView all 15 articles
Crop adaptation to waterlogging stress necessitates alterations in their morpho-physiological and biochemical characteristics. Cowpeas, which serve as a dual-purpose legume crop (food and fodder), are sensitive to waterlogging stress, especially when exposed to extended periods of water stagnation during the early growth stage. In this study, we subjected five distinct and superior cowpea varieties to 10 days of waterlogging stress at the early seedling stage (V2, 15 days post emergence for 10 days) under controlled conditions. The aim was to comprehend the response of these varieties and identify the ideal trait for screening a large collection of cowpea genetic resources for waterlogging tolerance. We measured and analyzed changes in morpho-physiological and root parameters to gain a deeper understanding of the mechanism underlying waterlogging tolerance. The treatment (waterlogging and control), genotype, and their interactions had a significant impact on the most studied traits (p < 0.05). The results indicated a significant reduction in morpho-physiological parameters such as plant height, leaf area, leaf number, Normalized Difference Vegetation Index (NDVI), chlorophyll content, and chlorophyll fluorescence parameters under stress treatment than control conditions. However, root parameters like the number of adventitious roots (AR) and their length (ARL) significantly increased under waterlogging stress in tolerant cowpea varieties like DC15 and PL4. Correlation and PCA analyses further revealed a positive and significant association between cowpeas’ waterlogging tolerance and AR formation and its AR length. Therefore, the current study reveals that swift development of AR and ARL may serve as potential traits conferring waterlogging tolerance in cowpeas. Using suitable mapping populations, these traits could reveal genomic regions associated with waterlogging tolerance in cowpeas. The tolerant varieties and key traits identified in this study could be beneficial in breeding programs aimed at enhancing waterlogging tolerance in cowpeas.
Excess soil moisture due to heavy and high-frequency precipitation and poor soil drainage constraints negatively affects more than 16% of global arable land (Zhang et al., 2015; Olorunwa et al., 2022a). Episodes of flooding or waterlogging have become more frequent in recent years, posing a severe threat to global food security (Ward et al., 2020). Furthermore, NASA climate simulation models predict that by 2030, there will be a 30% increase in heavy rainfall and flood events due to the impact of climate change, making the situation worse. Crops, except rice, are susceptible to waterlogging stress, showing significant yield reduction when exposed to prolonged water stagnation (Zhang et al., 2015). Cowpea is a dual-purpose legume crop sensitive to waterlogging stress, with 10–52% yield loss reported when waterlogging occurs during the vegetative period. If it is during the reproductive stage, more than 52% yield reduction was observed. This sensitivity and yield loss vary depending on the variety grown, soil type, and crop growth stage (Olorunwa et al., 2022a,b).
Oxygen deprivation in the root zone from waterlogging is the main factor influencing plants’ growth and development. Lack of oxygen results in reduced ATPs and leads to modification in physiological and biochemical parameters and the final yield of the crops (Olorunwa et al., 2022a,b). During waterlogging conditions, a decrease in leaf area, reduced shoot growth, reduced leaf nitrogen content, and reduction in net photosynthesis rates, transpiration, and stomatal conductance are reported in cowpeas (Zhang et al., 2015; Olorunwa et al., 2022a,b, 2023).
The negative impact of waterlogging stress on crops can be managed by soil application or foliar spray of nutrients, providing drainage, and sowing on raised beds (Pang et al., 2007). However, developing and deploying inherent waterlogging tolerant cultivars is the most economical and plausible approach. Nevertheless, the low heritability of the trait and complexity in phenotyping are the main bottlenecks in the genetic improvement of cowpea cultivars for waterlogging tolerance (Olorunwa et al., 2022a,b, 2023).
Deeper insight into the underlying mechanism of waterlogging tolerance in cowpeas is essential to identify relevant trait(s) that can improve waterlogging tolerance. Plants have evolved various tolerance mechanisms and developed adaptive trait(s) for waterlogging stress. For instance, rice survives and reproduces by the mechanism of shoot elongation and formation of aerenchyma (Steffens et al., 2010), whereas lysigenous aerenchyma formation confers tolerance to waterlogging in barley (Barrett-Lennard, 2003). Similarly, the formation of aerenchyma cells, lenticels, and adventitious roots in the tolerant genotypes was associated with waterlogging tolerance in pigeonpea (Hingane et al., 2015). Therefore, the main objective of the investigation is to identify key trait(s) contributing to waterlogging tolerance in cowpeas. Those traits can be targeted in future cowpea breeding programs to improve waterlogging tolerance.
The experiment was conducted under controlled conditions at the Green House (®Allice Biotechnology) of ICAR-National Institute of Abiotic Stress Management, Pune, India, from June to December 2022. The treatments were arranged in a factorial completely randomized design with five replications. Five popular cowpea varieties, viz., RC101, DC15, PL3, PL4, and GC3, were selected for the study. The selection was based on popularity among farmers and cultivation in large areas. The average daily temperature of 28.1 ± 4.6°C and relative humidity of 68.2 ± 10.8% were maintained in the greenhouse conditions using a water-cooling pad system throughout the experiment.
The plants were grown in plastic pots of 20 cm diameter and height of 20 cm with three perforations at the base. Pots were filled with a mixture of black soil and farmyard manure (FYM) at the proportion of 50:1 (V/V). Nutrients (nitrogen, phosphorus, and potassium) were applied as per the recommended dose of 10: 20: 10 kg ha−1, respectively. The amount of NPK added to each pot was calculated on a soil weight basis and blended adequately into the soil. After filling, each pot was weighed to 13 kg soil to ensure the same amount of soil mixture and constant moisture in each pot. Ten pots were used for each genotype (Five pots were used for imposing waterlogging stress treatment, and five were kept as a control). Filled pots were sown with six seeds per pot at 17–20 mm depth, and after seven days, the seedlings were thinned, and two healthy plants per pot were retained.
At the V2 leaf stage (15 days after emergence, 15 DAE), one set of uniformly emerged seedlings was subjected to waterlogging and the other set was retained as control for ten days (Olorunwa et al., 2022a). Waterlogging stress was achieved by placing the pots in a water-filled cement tank (8 × 5 × 1 m). The water level was maintained at least 20 mm above the soil surface throughout the stress. At the same time, five pots of each variety were kept at optimum soil moisture below field capacity as a control (<80% of FC). After ten days of stress treatment, excess water in the pots was drained and allowed to recover for five days.
Leaf area was measured using a leaf area meter (LI-3100C®Licor), Normalized Difference Vegetation Index (NDVI) was recorded during the recovery period (5 days post completion of stress treatment) using a hand-held device (GreenSeeker®, Trimble, United States) from 1.0 m above the soil surface of the experimental pot by following the method of Verhulst and Govaerts (2010), canopy temperature was measured by Infrared thermal Camera (Vario CAM hr. inspect 575, Jenoptic, Germany) with spatial resolution of 768 × 576 pixels. Chlorophyll fluorescence in leaves was measured during recovery (5 days post-completion of stress treatment) to study changes in maximum PSII efficiency in response to waterlogging. Leaves were adapted to dark for 30 min before measurement, and lights in the chlorophyll fluorescence imaging chamber were turned off to avoid any effect of light on maximum PSII efficiency. The temperature in the imaging chamber was set around 25 ± 1°C. The leaf images were captured at given time points by chlorophyll fluorescence measuring system (FC 1000-H/GFP, Handy Fluor Cam, P.S.I., Brno, Czech Republic) as described in Nedbal et al. (2000). Fluorescence was detected by a high-sensitivity charge-coupled device (CCD) camera. It was driven by the FluorCam software package (FluorCam 7). First, the minimum fluorescence level (F0) of dark-adapted leaves was determined using non-actinic measuring flashes provided by super-bright light emitting diodes (LEDs) followed by a saturation pulse of light radiation [2,500 μmol (photon) m−2 s−1] to obtain the maximum fluorescence (Fm). The maximum photochemical efficiency of PSII (Fv/Fm) was calculated according to Krause and Weis (1991).
where the difference between Fm and F0 represents the variable fluorescence (Fv).
For assessing response to waterlogging stress by image analysis, four colors were set manually for taking fluorescent images: blue (corresponding to Fv/Fm; 0.8), yellow, green, and red (related to Fv/Fm; 0.1). Photosynthetic pigments such as Chlorophyll a, b, and carotenoids in the leaf sample of cowpea (both stress and control sample) were estimated following the protocol of Lichtenthaler and Wellburn (1983).
Parameters such as root length (cm), shoot length (cm), root fresh weight (g/plant), root dry weight (g/plant), shoot fresh biomass (g/plant), and shoot dry biomass (g/plant), number of roots in each plant, nodules per plant, number of adventitious roots per plant and adventitious root length were measured destructively at the end of the stress treatment in each set manually.
Growth and yield attributes such as plant height (cm), number of branches per plant, number of leaves per plant, pod length (cm), the weight of 5 pods/plant (g), test weight (g), number of pods per plant and grain yield per plant (g) were measured in both the control and waterlogged plants in each replication after harvest of the crop.
The Analysis of variance and significant treatment differences (LSD) was carried out using SAS (version 9.4; SAS Institute, Cary, NC). The Spearman correlation coefficient was determined to assess the relationship between different parameters. Furthermore, Principal component analysis (PCA), biplot analysis, and box plots were drawn using R v 4.3.1. software.
The Analysis of variance revealed a significant difference between control and stress treatment and their interaction for all the root traits, physiological parameters, and yield and its attributing traits (p < 0.05). Results presented in Table 1 imply considerable genetic variability among cowpea varieties, as evidenced by significant genotype and genotype x treatment interaction.
Table 1. Analysis of variance in response to waterlogging stress (WL) and varieties (V) in cowpea for different physiological, root, growth and yield-attributing traits.
Waterlogging stress significantly (p < 0.05, Tables 1, 2) influenced the physiological parameters of all cowpea varieties. Waterlogging reduced the leaf area (31.89%), Normalized Difference Vegetation Index value (51.89%), chlorophyll a (33.08%), chlorophyll b (34.54%) and total chlorophyll content (32.44%) and maximum quantum efficiency (Qmax) by 4.93%. In contrast, waterlogging led to an increase in the canopy temperature by 3.38°C, ground fluorescence (F0), maximum fluorescence (Fm), and variable chlorophyll fluorescence (Fv) by 40.71, 15.75 and 9.76%, respectively, compared to plants under control conditions.
The physiological performance of the varieties was also found to be significant under both treatments. Among all the varieties, DC15 was found to perform better for leaf area (45.24 cm2 plant−1), chlorophyll a, b and total chlorophyll (1.60, 0.66 and 2.18, respectively), carotenoids (2.35), maximum quantum efficiency (0.80) and lower canopy temperature (28.94°C) followed by variety PL4. Meanwhile, RC101 performed the lowest in terms of physiological parameters (Table 2).
The interaction of waterlogging and cowpea varieties was also found to be significant. All the varieties performed better under control than stress conditions for most of the studied physiological traits. Among those, the performance of DC15 was significantly superior with higher leaf area (58.21 cm2 plant−1), Normalized Difference Vegetation Index I (0.80), total chlorophyll (2.72) and maximum quantum efficiency (0.82). Similarly, the same variety has performed better under stress with higher Normalized Difference Vegetation Index (0.44), total chlorophyll (1.63), carotenoids (1.63) and lower canopy temperature (30.96°C) followed by variety PL4. Maximum quantum efficiency was high in the variety PL4 variety, followed by DC15. Nevertheless, higher leaf area (35.43 cm2 plant−1), ground fluorescence (155.72), maximum fluorescence (649.12) and variable chlorophyll fluorescence were observed in the GC3 variety.
Waterlogging stress significantly (p < 0.05) affected the root morphological traits of cowpea varieties (Tables 1, 3). In response to waterlogging, there was a reduction in the root length (27.90%), the number of roots per plant (7.44%), and root nodules per plant (79.73%), while an increase in the root fresh weight (383.46%) and root dry weight (118.18%) due to the formation of adventitious roots under stress compared to plants grown under non-stress conditions. Irrespective of waterlogging stress, the performance of cowpea varieties differed regarding root morphological traits; among them, the DC15 variety was found to be more promising with significantly distinct root morphological parameters such as root length (11.80 cm), number of roots (20.88 plant−1), root nodules (7.66 plant−1), root fresh weight (1.32 g plant−1) and adventitious roots followed by GC3 and PL4 varieties. Root dry weight (0.26 g plant−1) recorded in the PL4 variety was more than that of DC15 or GC3.
The interaction between waterlogging stress and varieties was also significant (Tables 1, 3). All the varieties recorded better root growth under control than waterlogging stress. The minimum reduction in the root length (3.66%), root dry weight (19.04%) and number of roots (7.15%) was observed in the DC15 variety under stress. Meanwhile, the maximum reduction in root morphological parameters is recorded in RC101 and PL3 varieties compared to their performance under control conditions. Among all the varieties, the DC15 variety has registered higher root length (11.58 cm), root fresh weight (1.60 g plant−1), number of roots per plant (20.11), number of nodules per plant (4) and adventitious roots per plant (38.33) followed by PL4 and GC3 varieties.
The effect of waterlogging on plant growth and yield attributes was significant (p < 0.05, Table 1), as shown in Table 4. Under waterlogging stress, there was a reduction in shoot length (5.77%), plant height (15.43%), number of branches per plant (21.34%), number of leaves per plant (65.74%), pods per plant (64.70%), pod length (23.93%), pod weight (42.40%), test weight (17.53%) and yield (60.81%) compared to control condition.
Evaluation of cowpea varieties showed that DC15 performed better in terms of growth (plant height, 108.13 cm; number of branches, 5.50 and leaves per plant, 54.66) and yield attributes (pods per plant, 54.66; pod weight, 10.43 g; test weight, 10.26 g and grain yield, 48.16 g) followed by PL4. Meanwhile, the performance of the RC101 variety (in terms of growth and yield) was less than all other four cowpea varieties.
Compared to the other varieties, the performance of DC15 was better under both control and stress conditions with higher growth and yield parameters. This variety has had better growth attributes such as plant height (116.16, 100.11 cm), number of branches (6.33, 4.66), and leaves per plant (26.78, 11.66) as well as yield attributes such as number of pods (25.57 and 17.59), pod weight (13.12 and 7.12 g) and test weight (12.42, 10.70 g), respectively under control and stress conditions.
The higher grain yield was recorded by DC 15, followed by PL4, under both control and stress conditions. The same varieties have recorded a minimum decrease in grain yield, i.e., 23.68 and 18.88% under stress conditions relative to their yield under control, respectively. Meanwhile, GC3, PL3, and RC 101 recorded 44.56, 64.86, and 65.09% yield reductions, respectively, under stress, compared to control.
Correlation studies were conducted to determine the association between different parameters and grain yield under control and stress conditions, and results were presented in the form of a correlogram (Figures 1, 2). Results revealed that traits viz., plant height (PH), number of branches per plant (NBP), no. of leaves per plant (LPP), Normalized Difference Vegetation Index (NDVI), shoot length (SL), number of roots (NR), number of pods per plant (NPP) and initial fluorescence/ground fluorescence (F0) are negatively associated with grain yield under control conditions. In contrast, all the other traits such as leaf area (LA), canopy temperature (CT), chlorophyll a (Chla), chlorophyll b (Chlb), total chlorophyll (TotalChl), carotenoids (Car), root length (RL), root fresh weight (RFW), shoot fresh weight (SFW), root dry weight (RDW), shoot dry weight (SDW), nodules, pod length (PL), pod weight (PW), maximum fluorescence (Fm), variable fluorescence (Fv) and Qmax were positively associated with grain yield under control conditions (Figure 1). Similarly, under stress conditions, traits such as plant height, number of branches per plant, no. of leaves per plant, root length, Qmax, test weight, no. of pods per plant, and adventitious roots had a strong significant positive association with grain yield (r = 0.5 to1). Traits viz., shoot fresh weight, shoot dry weight, adventitious root length, and pod length had a moderate positive association with grain yield under stress (r = 0.25 to 0.5). In contrast, traits such as canopy temperature, chlorophyll a, b total chlorophyll, shoot length, no. of roots and nodules per plant had weak to moderate positive correlation with grain yield under stress (r = 0 to 0.25) (Figure 2).
Figure 1. Correlation coefficient between grain yield with, root, shoot, growth and physiological parameters under control conditions RL, Root length (cm); SL, Shoot Length (cm); RFW, Root Fresh Weight (g/plant); SFW, Shoot Fresh Weight (g/plant); RDW, Root Dry Weight (g/plant); SDW, Shoot Dry Weight (g/plant); NR, No. of Roots/plant; NN, Root Nodules/plant; AR, Adventitious Roots/plant; ARL, Adventitious Root Length (cm); LA, Leaf Area; NDVI, Normalized Difference in Vegetative Index; CT, Canopy Temperature; Chla, Chlorophyll a (mg/mL); Chlb, Chlorophyll b (mg/mL); TChl, Total Chlorophyll (mg/mL); Car, Carotenoids (mg/mL); F0, ground fluorescence; Fm, maximum chlorophyll fluorescence; Fv, Variable chlorophyll fluorescence; QYmax, maximum quantum efficiency; PH, Plant Height (cm); NBP, No. of Brancher per plant; LPP, Leaves per plant; NPP, Pods per plant; PL, Pod Length (cm); PW, Pod Weight (g/5pods); TW, 100 seed weight (g); GY, Grain Yield (g/plant).
Figure 2. Correlation coefficient between grain yield with, root, shoot, growth and physiological parameters under waterlogging stress conditions RL, Root length (cm); SL, Shoot Length (cm); RFW, Root Fresh Weight (g/plant); SFW, Shoot Fresh Weight (g/plant); RDW, Root Dry Weight (g/plant); SDW, Shoot Dry Weight (g/plant); NR, No. of Roots/plant; NN, Root Nodules/plant; AR, Adventitious Roots/plant; ARL, Adventitious Root Length (cm); LA, Leaf Area; NDVI, Normalized Difference in Vegetative Index; CT, Canopy Temperature; Chla, Chlorophyll a (mg/mL); Chlb, Chlorophyll b (mg/mL); TChl, Total Chlorophyll (mg/mL); Car, Carotenoids (mg/mL); F0, ground fluorescence; Fm, maximum chlorophyll fluorescence; Fv, Variable chlorophyll fluorescence; QYmax, maximum quantum efficiency; PH, Plant Height (cm); NBP, No. of Brancher per plant; LPP, Leaves per plant; NPP, Pods per plant; PL, Pod Length (cm); PW, Pod Weight (g/5pods); TW, 100 seed weight.
To assess how cowpea varieties responded to waterlogging, Principal Component Analysis (PCA) was employed to determine the morpho-physiological traits that most effectively explained their response (Figures 3A,B). This helped identify which genotypes are tolerant and sensitive to waterlogging. The analysis revealed four principal components (PCs), with PC1 (42.95%) and PC2 (28.88%) accounting for 71.83% of the total variation (Figures 3A,B). Among the different variables, AR, Qmax, F0, Fm, Fv, and GY showed the highest variation in PC1, while in PC2, variables such as Chla, Chlb, TotalChl, Car, and NR contributed significantly compared to other variables. Furthermore, the biplot analysis revealed that traits such as Chla, b, total chlorophyll content, no. of roots, number of branches per plant, plant height, no. of pods per plant, shoot dry weight, pod length, nodules per plant, shoot length, adventitious roots, root length, test weight, Qmax, root dry weight, grain yield and adventitious root length are in the same direction as PC1. Hence, they are positively correlated with PC1. Further, cowpea varieties DC15 and PL4 are on the positive side of PC1, suggesting their tolerance nature toward waterlogging. On the other hand, leaf area, maximum florescence, variable florescence, and root fresh weight are in the opposite direction of PC1, which implies a negative association. Further, varieties PL3, GC3 and RC101 were situated in the opposite direction of PC, indicating the sensitive nature of these varieties (Figure 4). Further, the length of the vector indicated the significance of the traits. In the present study, adventitious roots, no. of pods per plant, no. of roots, and total chlorophyll content had higher contributions to the waterlogging tolerance of the DC15 variety. Similarly, root dry weight, Qmax, test weight, adventitious root length, and pod weight significantly contributed to the PL4 variety.
Figure 3. (A) Scree plot depicting percentage of variance explained for various growth, physiology, root, and yield parameters of cowpea genotypes under water- logging stress. 1 to 4 number indicates component numbers. (B) Contribution of each variable to total variability in PC1 and PC2 and correlation between them.
Figure 4. Biplot depicting the interrelationship between various growth, physiology, root, and yield parameters of cowpea genotypes under waterlogging stress.
In response to waterlogging stress, a series of modifications in morpho-physiological, root, growth and development-related parameters were observed in cowpeas, which eventually hindered the potential yield of cowpea genotypes (Olorunwa et al., 2022a,b, 2023). Additionally, projections have suggested that climate change factors intensify this situation, resulting in declined cowpea productivity, which could worsen world food security (Ray et al., 2019). Therefore, it is imperative to find an appropriate solution to this problem. A possible approach to managing waterlogging stress includes agronomic interventions (sowing on raised beds), growth regulators, chemical applications/spay, and the genetic improvement of cowpea cultivars resilient to waterlogging. However, among the approaches mentioned above, developing and deploying waterlogging tolerant cowpeas would be the most ideal, economical, and eco-friendly approach (Olorunwa et al., 2022a,b).
However, due to the poor heritability and extremely unpredictable waterlogging conditions, little success has been achieved in breeding cowpea genotypes for waterlogging tolerance (Olorunwa et al., 2022a,b). Breeders mostly screened genotypes that were tolerant to waterlogging through field-based trials rather than laboratory-based physiological features (Khabaz-Saberi et al., 2005). Direct selection for waterlogging tolerance in the field may not be efficient due to the intricacy of waterlogging tolerance and variation in field conditions. Deeper insight into the underlying mechanisms of waterlogging tolerance allows plant breeders to target specific physiological trait (s) and pyramid different tolerance-related traits to generate cowpea pre-breeding material with enhanced tolerance to waterlogging. Therefore, identifying physiological characteristics associated with waterlogging tolerance in cowpeas is essential to accomplish this task. Therefore, we have studied morpho-physiological, root responses to waterlogging stress under controlled situations, as it avoids variation arising due to field heterogeneity.
The rate of oxygen diffusion in water is 104 times slower than that of air (Armstrong, 1980). Therefore, roots under waterlogging conditions lack or have minimal oxygen uptake. As a result of oxygen deprivation, ATP production is affected severely, causing an energy crisis in the waterlogged plants (Colmer and Voesenek, 2009). Plants have developed many adoptive strategies to withstand O2 deficiency conditions, including aerenchyma formation, adventitious root (AR) development, and regulation of shoot elongation (Suralta and Yamauchi, 2008). Different crop plants have different survival mechanisms; for instance, rice, maize, and barley can form aerenchyma tissue in response to waterlogging (Steffens et al., 2010; Zhang et al., 2015). The primary roots of cowpeas under waterlogged conditions decay or deteriorate quickly due to lack of oxygen, leading to an energy crisis. A fundamental response in cowpeas is developing adventitious roots to replace the damaged original root system under flooded conditions (AR). The development of AR has been observed in many species, including rice (Mhimdi and Pérez-Pérez, 2020), maize (Mano and Omori, 2013), grain legumes such as mungbean and Blackgram (Kyu et al., 2021). The AR develops as a part of the existing root system; however, they primarily differ in terms of emergence, i.e., from the base of stem, nodes, mesocotyl and hypocotyls and have more aerenchyma than the primary root system (Della Rovere et al., 2013).
Consequently, under anoxygenic conditions, ARs support and enhance gas diffusion and water and nutrient uptake in plants along and across the roots via air-filled space known as aerenchyma (Steffens and Rasmussen, 2016). Therefore, plants that can quickly form AR under waterlogged conditions are expected to be a better adaptation trait for waterlogging environments (Yang et al., 2023). In the current study, no tested varieties developed AR under control conditions (Figures 5, 6). Contrasting to this, all the varieties formed AR under waterlogging conditions. However, oxygen transport efficiency depends on AR formation and the number of AR formed during waterlogging stress. In the present experiment, the number of AR varied significantly among the varieties; this signifies the variation in waterlogging tolerance among varieties.
Figure 5. Variation in the number and length of adventitious roots of different cowpea varieties under waterlogging stress.
Figure 6. Variation in root and shoot parameters of cowpea varieties under control and waterlogging stress (picture is taken immediately after 10th day of waterlogging treatment).
Further, tolerant varieties had significantly higher and longer AR than sensitive varieties. Akin to our results, Thomas et al. (2005) reported that faster AR formation is associated with the recovery of N metabolism in the roots of legumes. Further, the formation of AR enhances the internal oxygen transport from shoot to waterlogged roots, increasing the oxygen concentration in the root zone (Shimamura et al., 2010; Teakle et al., 2011).
Waterlogging significantly influenced the growth and development of cowpea varieties. Ten days of waterlogging treatment on cowpeas induced significant changes in morphological parameters, including decreased plant height, leaf area, number of leaves, and biomass accumulation to varying degrees in different cowpea varieties. In the present study, waterlogging for ten days significantly decreased plant height, number of branches per plant, and number of leaves per plant in all the tested cowpea varieties compared to the control. Similar modifications and decreases in plant height and number of leaves were reported in cowpea (Olorunwa et al., 2022a,b), decrease in plant height of soybean (Dhungana et al., 2019), mung (Kumar et al., 2013), and pigeonpea (Basavaraj et al., 2023) under waterlogging stress at vegetative stage, this supports the present results. As a result of waterlogging, a decrease in plant height and resultant stunted growth was observed in all the tested varieties; this is akin to observations of the previous reports in cowpea (Umaharan et al., 1997; Olorunwa et al., 2022a,b). Although we have not measured ethylene production in the present investigation, the decrease in plant height under waterlogging is mainly due to increased production of ethylene compound, 1-aminocyclopropane-1-carboxylic acid (ACC), production of which enhanced under waterlogging conditions (Pan et al., 2019). Further, increased ACC levels might have inhibited the abscisic acid biosynthesis, thereby inhibiting shoot elongation and overall plant growth (Ntukamazina et al., 2017; Ploschuk et al., 2018; Olorunwa et al., 2022a).
Additionally, plants under waterlogging stress during the vegetative stage respond in various ways to sustain their growth and development by utilizing their morphological characteristics, particularly those associated with their leaves, which are the site of photosynthesis. The onset of leaf senescence and a notable decrease in leaf area and leaf number are typical signs of waterlogging stress, which worsens depending on the severity of waterlogging, particularly in legumes (Hingane et al., 2015; Olorunwa et al., 2022a,b; Basavaraj et al., 2023). A similar trend was observed in the present study: a decrease in leaf area and number of leaves per plant in stress treatment compared to control plants of all tested cowpea varieties. This decrease in leaf area is mainly due to decreased photosynthesis (Pn) caused by stomata closure under waterlogging stress (Takele and McDavid, 1994; Olorunwa et al., 2022a). Changes in photosynthetic capacity are mainly due to modifications in enzyme carboxylation, reduction in chlorophyll content and reduced leaf area. Further, tolerant varieties such as DC15 and PL4 in the present study could maintain higher leaf area and leaf number under waterlogging conditions, resulting in optimum photosynthesis, growth, development, and economic yield. Kumutha et al. (2009) made similar observations on leaf area in waterlogging tolerant pigeonpea genotypes with higher leaf area than sensitive genotypes under waterlogging stress.
Waterlogging stress significantly affected the morpho-physiological functions of cowpea varieties, notably altered shoot morphology and physiology, which had an adverse impact on carbon fixation and stomatal conductance (Ploschuk et al., 2018). Since the waterlogging condition has 10,000 times less oxygen diffusion, the stomata and cell walls find it difficult to exchange the CO2 needed for the plant’s fundamental functions (Voesenek and Bailey-Serres, 2015). Further, reduced CO2 availability under waterlogging/flooding causes a reduction in heterotrophic energy production in mitochondria, therefore decreasing photosynthesis. In addition, waterlogging stress caused a decline in the photosynthetic capacity of tested cowpea varieties, inhibiting the electron transport rate and leading to photo-inhibition in PSII. In the present study, we observed a decrease in chlorophyll fluorescence parameters such as F0, Fv, Fm and Fv/Fm, which are signs of damaged PSII. Alternatively, a reduction of photosynthetic pigments (Chlorophyll and carotenoids) contributed to a decline in photosynthesis in the sensitive cowpea varieties in the present study. Under waterlogging, the drop in Fv/Fm indicates that the light energy absorbed by PSII was utilized to reduce PSII’s potential vigor change and the efficiency of the principal electron acceptor (Rao et al., 2021). These alterations represent the plants’ ability to withstand a range of environmental stressors, such as hypoxia and anoxia (Zhu et al., 2016).
Additionally, cowpea genotypes under waterlogging in the early phases of vegetative growth had lower Fv, Fm, F0 and Fv/Fm, suggesting that cowpeas are susceptible to waterlogging stress. These results aligned with those of Ploschuk et al. (2018), who reported that field peas are sensitive to waterlogging because of impaired PSII. Further, a decrease in quantum efficiency of PSII (Fv/Fm) was observed under stress treatment compared to optimum conditions and tolerant cowpea varieties such as DC15 and PL4 had higher PSII values under stress compared to sensitive RC101 cowpea variety, indicating that tolerant cowpea genotypes can prevent photodamage during waterlogging stress more effectively than susceptible genotypes. According to Zhu et al. (2016), the xanthophyll cycle’s capacity to shield the photosynthetic machinery from photo-inhibitory damage under waterlogging is responsible for this waterlogging tolerance. Previously, Olorunwa et al. (2022a,b) also reported similar results.
Waterlogging tolerance is a complex quantitative trait involving plants’ morphological, physiological, anatomical, and molecular characteristics (Yamauchi et al., 2018; Zeng et al., 2020; Basavaraj et al., 2023). More than one criterion is required to reflect plants’ waterlogging tolerance precisely. Therefore, correlation and principal component analysis were used to evaluate cowpea varieties in the present study. Association analysis indicated that grain yield in the present study is significantly positively associated with forming adventitious roots (root length, root number). Therefore, adventitious root traits provide selection criteria for screening waterlogging-tolerant cowpea genotypes. Thus, these findings illustrated the significance of root characteristics in selecting genotypes tolerant of waterlogging stress. Further, significant differences for various morpho-physiological and root traits were observed among the tested cowpea varieties, suggesting inherent genetic variation. These results are consistent with Olorunwa et al. (2022a,b) studies in cowpeas and beans (Velasco et al., 2019).
The present study highlighted the significance of morpho-physiological and root parameters tolerant to waterlogging, and the study revealed the quantitative complex nature of tolerance traits among the cowpea varieties. There is a significant positive association between adventitious root number and root length with grain yield under waterlogging stress, suggesting that while screening cowpea genotypes, tolerance to waterlogging, priority should be given to root traits, especially AR and ARL. Cowpea varieties like PL4 and DC15 could aid in developing novel genotypes of cowpeas that are tolerant to waterlogging. These tolerant genotypes can be crossed with them to transfer waterlogging tolerance genes into high-yielding commercial cowpea varieties without compromising their natural performance under waterlogging stress. However, before crossing programs, these findings must be validated under field conditions to evaluate their growth, development, and yield performance under waterlogging conditions to guarantee sustainable cowpea production.
The raw data supporting the conclusions of this article will be made available by the authors, without undue reservation.
PB: Conceptualization, Data curation, Formal analysis, Funding acquisition, Investigation, Methodology, Project administration, Resources, Software, Supervision, Validation, Visualization, Writing – original draft, Writing – review & editing. KJ: Visualization, Writing – review & editing. RB: Formal analysis, Software, Writing – review & editing. VG: Writing – review & editing. AG: Resources, Validation, Writing – review & editing. SS: Formal analysis, Resources, Validation, Writing – review & editing. KT: Software, Writing – review & editing. DP: Resources, Writing – review & editing. KB: Investigation, Methodology, Supervision, Writing – review & editing. JR: Formal analysis, Supervision, Writing – review & editing. CH: Methodology, Writing – review & editing. HH: Data curation, Formal analysis, Writing – review & editing. KS: Funding acquisition, Supervision, Writing – review & editing. MP: Writing – review & editing.
The author(s) declare financial support was received for the research, authorship, and/or publication of this article. The research was carried out under the ICAR-NICRA project “Phenotyping of Pulses for Enhanced Tolerance to Drought and Heat (OXX01737).”
The authors thank ICAR-NICRA for funding the project “Phenotyping of Pulses for Enhanced Tolerance to Drought and Heat” and ICAR-NIASM for the logistic support.
The authors declare that the research was conducted without any commercial or financial relationships that could be construed as a potential conflict of interest.
All claims expressed in this article are solely those of the authors and do not necessarily represent those of their affiliated organizations, or those of the publisher, the editors and the reviewers. Any product that may be evaluated in this article, or claim that may be made by its manufacturer, is not guaranteed or endorsed by the publisher.
Armstrong, W. (1980). Aeration in higher plants. Adv. Bot. Res. 7, 225–332. doi: 10.1016/S0065-2296(08)60089-0
Barrett-Lennard, E. G. (2003). The interaction between waterlogging and salinity in higher plants: causes, consequences and implications. Plant Soil 253, 35–54. doi: 10.1023/A:1024574622669
Basavaraj, P. S., Rane, J., Boraiah, K. M., Gangashetty, P., and Harisha, C. B. (2023). Genetic analysis of the tolerance to transient waterlogging stress in pigeonpea (Cajanus cajan L. Millspaugh). Indian J. Genet. Plant Breed. 83, 316–325. doi: 10.31742/ISGPB.83.3.3
Colmer, T. D., and Voesenek, L. A. C. J. (2009). Flooding tolerance: suites of plant traits in variable environments. Funct. Plant Biol. 36, 665–681. doi: 10.1071/FP09144
Della Rovere, F., Fattorini, L., D’angeli, S., Veloccia, A., Falasca, G., and Altamura, M. (2013). Auxin and cytokinin control formation of the quiescent Centre in the adventitious root apex of Arabidopsis. Ann. Bot. 112, 1395–1407. doi: 10.1093/aob/mct215
Dhungana, S. K., Kim, H. S., Kang, B. K., Seo, J. H., Kim, H. T., Shin, S. O., et al. (2019). Evaluation of flooding tolerance of soybean (Glycine max L. Merr.) in greenhouse under upland and paddy soil conditions. J. Crop. Sci. Biotechnol. 22, 283–290. doi: 10.1007/s12892-019-0106-0
Hingane, A., Saxena, K., Patil, S., Sultana, R., Srikanth, S., Mallikarjuna, N., et al. (2015). Mechanism of water-logging tolerance in pigeonpea. Indian J. Genet. Plant Breed. 75, 208–214. doi: 10.5958/0975-6906.2015.00032.2
Khabaz-Saberi, H., Setter, T. L., and Waters, I. (2005). Waterlogging induces high to toxic concentrations of iron, aluminum, and manganese in wheat varieties on acidic soil. J. Plant Nutr. 29, 899–911. doi: 10.1080/01904160600649161
Krause, G. H., and Weis, E. (1991). Chlorophyll fluorescence and photosynthesis—the basics. Annu rev plant Physiol. Plant Mol. Biol. 42, 313–349. doi: 10.1146/annurev.pp.42.060191.001525
Kumar, P., Pal, M., Joshi, R., and Sairam, R. K. (2013). Yield, growth and physiological responses of mung bean [Vigna radiata (L.) Wilczek] genotypes to waterlogging at vegetative stage. Physiol. Mol. Biol. Plants 19, 209–220. doi: 10.1007/s12298-012-0153-3
Kumutha, D., Ezhilmathi, K., Sairam, R. K., Srivastava, G. C., Deshmukh, P. S., and Meena, C. R. (2009). Waterlogging induced oxidative stress and antioxidant activity in pigeonpea genotypes. Biol. Plant. 53, 75–84. doi: 10.1007/s10535-009-0011-5
Kyu, K. L., Malik, A. I., Colmer, T. D., Siddique, K. H. M., and Erskine, W. (2021). Response of mungbean (cvs. Celera II-AU and jade-AU) and blackgram (cv. Onyx-AU) to transient waterlogging. Front. Plant Sci. 12:709102. doi: 10.3389/fpls.2021.709102
Lichtenthaler, H. K., and Wellburn, A. R. (1983). Determinations of total carotenoids and chlorophylls a and b of leaf extracts in different solvents. Biochem. Soc. Trans. 11:592.
Mano, Y., and Omori, F. (2013). Relationship between constitutive root aerenchyma formation and flooding tolerance in Zea nicaraguensis. Plant Soil 370, 447–460. doi: 10.1007/s11104-013-1641-0
Mhimdi, M., and Pérez-Pérez, J. M. (2020). Understanding of adventitious root formation: what can we learn from comparative genetics? Front. Plant Sci. 11:582020. doi: 10.3389/fpls.2020.582020
Nedbal, L., Soukupová, J., Kaftan, D., Whitmarsh, J., and Trtílek, M. (2000). Kinetic imaging of chlorophyll fluorescence using modulated light. Photosynth. Res. 66, 3–12. doi: 10.1023/A:1010729821876
Ntukamazina, N., Onwonga, R. N., Sommer, R., Mukankusi, C. M., Mburu, J., and Rubyogo, J. C. (2017). Effect of excessive and minimal soil moisture stress on agronomic performance of bush and climbing bean (Phaseolus vulgaris L.). Cogent Food Agric. 3:1373414. doi: 10.1080/23311932.2017.1373414
Olorunwa, O. J., Adhikari, B., Brazel, S., Bheemanahalli, R., Barickman, T. C., and Reddy, K. R. (2023). Waterlogging stress reduces cowpea (Vigna unguiculata L.) genotypes growth, seed yield, and quality at different growth stages: implications for developing tolerant cultivars under field conditions. Agric. Water Manag. 284:108336. doi: 10.1016/j.agwat.2023.108336
Olorunwa, O. J., Adhikari, B., Brazel, S., Shi, A., Popescu, S. C., Popescu, G. V., et al. (2022b). Growth and photosynthetic responses of cowpea genotypes under waterlogging at the reproductive stage. Plan. Theory 11:2315. doi: 10.3390/plants11172315
Olorunwa, O. J., Adhikari, B., Shi, A., and Barickman, T. C. (2022a). Screening of cowpea (Vigna unguiculata (L.) Walp.) genotypes for waterlogging tolerance using morpho-physiological traits at early growth stage. Plant Sci. 315:111136. doi: 10.1016/j.plantsci.2021.111136
Pan, R. W., Jiang, Q., Wang, L., Xu, S., Shabala, W. Y., and Zhang, L. (2019). Differential response of growth and photosynthesis in diverse cotton genotypes under hypoxia stress. Photo-Dermatology 57, 772–779. doi: 10.32615/ps.2019.087
Pang, J., Ross, J., Zhou, M., Mendham, N., and Shabala, S. (2007). Amelioration of detrimental effects of waterlogging by foliar nutrient sprays in barley. Funct. Plant Biol. 34, 221–227. doi: 10.1071/fp06158
Ploschuk, R. A., Miralles, D. J., Colmer, T. D., Ploschuk, E. L., and Striker, G. G. (2018). Waterlogging of winter crops at early and late stages: impacts on leaf physiology, growth and yield. Front. Plant Sci. 9:425885. doi: 10.3389/fpls.2018.01863
Rao, L., Li, S., and Cui, X. (2021). Leaf morphology and chlorophyll fluorescence characteristics of mulberry seedlings under waterlogging stress. Sci. Rep. 11:13379. doi: 10.1038/s41598-021-92782-z
Ray, D. K., West, P. C., Clark, M., Gerber, J. S., Prishchepov, A. V., and Chatterjee, S. (2019). Climate change has likely already affected global food production. PLoS One 14:e0217148. doi: 10.1371/journal.pone.0217148
Shimamura, S., Yamamoto, R., Nakamura, T., Shimada, S., and Komatsu, S. (2010). Stem hypertrophic lenticels and secondary aerenchyma enable oxygen transport to roots of soybean in flooded soil. Ann. Bot. 106, 277–284. doi: 10.1093/aob/mcq123
Steffens, B., Geske, T., and Sauter, M. (2010). Aerenchyma formation in the rice stem and its promotion by H2O2. New Phytol. 190, 369–378. doi: 10.1111/j.1469-8137.2010.03496.x
Steffens, B., and Rasmussen, A. (2016). The physiology of adventitious roots. Plant Physiol. 170, 603–617. doi: 10.1104/pp.15.01360
Suralta, R. R., and Yamauchi, A. (2008). Root growth, aerenchyma development, and oxygen transport in rice genotypes subjected to drought and waterlogging. Environ. Experiment. Bot. 64, 75–82. doi: 10.1016/j.envexpbot.2008.01.0
Takele, A., and McDavid, C. R. (1994). Effects of short-term waterlogging on cultivars of cowpea (Vigna uniguiculata (L.) Walp.). Trop. Agric. 71, 275–280.
Teakle, N. L., Armstrong, J., Barrett-Lennard, E. G., and Colmer, T. D. (2011). Aerenchymatous phellem in hypocotyl and roots enables O2 transport in Melilotus siculus. New Phytol. 190, 340–350. doi: 10.1111/j.1469-8137.2011.03655.x
Thomas, A. L., Guerreiro, S. M. C., and Sodek, L. (2005). Aerenchyma formation and recovery from hypoxia of the flooded root system of nodulated soybean. Ann. Bot. 96, 1191–1198. doi: 10.1093/aob/mci272
Umaharan, P., Ariyanayagam, R. P., and Haque, S. Q. (1997). Effect of short-term waterlogging applied at various growth phases on growth, development and yield in Vigna unguiculata. J. Agric. Sci. 128, 189–198. doi: 10.1017/S0021859696004121
Velasco, N. F., Ligarreto, G. A., Díaz, H. R., and Fonseca, L. P. M. (2019). Photosynthetic responses and tolerance to root-zone hypoxia stress of five bean cultivars (Phaseolus vulgaris L.). South Afr. J. Bot. 123, 200–207. doi: 10.1016/j.sajb.2019.02.010
Verhulst, N., and Govaerts, B. (2010). The normalized difference vegetation index (NDVI) GreenSeekerTM handheld sensor: toward the integrated evaluation of crop management. Part A: concepts and case studies. Mexico: CIMMYT.
Voesenek, L. A. C. J., and Bailey-Serres, J. (2015). Flood adaptive traits and processes: an overview. New Phytol. 206, 57–73. doi: 10.1111/nph.13209
Ward, P. J., De Ruiter, M. C., Mård, J., Schröter, K., Van Loon, A., Veldkamp, T., et al. (2020). The need to integrate flood and drought disaster risk reduction strategies. Water Security. 11:100070. doi: 10.1016/j.wasec.2020.100070
Yamauchi, T., Colmer, T. D., Pedersen, O., and Nakazono, M. (2018). Regulation of root traits for internal aeration and tolerance to soil waterlogging-flooding stress. Plant Physiol. 176, 1118–1130. doi: 10.1104/pp.17.01157
Yang, L., Li, N., Liu, Y., Miao, P., Liu, J., and Wang, Z. (2023). Updates and prospects: morphological, physiological, and molecular regulation in crop response to waterlogging stress. Agronomy 13:2599. doi: 10.3390/agronomy13102599
Zeng, R., Chen, L., Wang, X., Cao, J., Li, X., Xu, X., et al. (2020). Effect of waterlogging stress on dry matter accumulation, photosynthesis characteristics, yield, and yield components in three different ecotypes of peanut (Arachis hypogaea L.). Agronomy 10:1244. doi: 10.3390/agronomy10091244
Zhang, X., Shabala, S., Koutoulis, A., Shabala, L., Johnson, P., Hayes, D., et al. (2015). Waterlogging tolerance in barley is associated with faster aerenchyma formation in adventitious roots. Plant Soil 394, 355–372. doi: 10.1007/s11104-015-2536-z
Keywords: legumes, aerial roots, lateral roots, waterlogging, flood, abiotic stress, climate change
Citation: Basavaraj PS, Jangid KK, Babar R, Gangana Gowdra VM, Gangurde A, Shinde S, Tripathi K, Patil D, Boraiah KM, Rane J, Harisha CB, Halli H, Sammi Reddy K and Prabhakar M (2024) Adventitious root formation confers waterlogging tolerance in cowpea (Vigna unguiculata (L.) Walp.). Front. Sustain. Food Syst. 8:1373183. doi: 10.3389/fsufs.2024.1373183
Received: 19 January 2024; Accepted: 20 March 2024;
Published: 04 April 2024.
Edited by:
Pushp Sheel Shukla, Centre for Cellular and Molecular Platforms, IndiaReviewed by:
Kapil Gupta, Siddharth University Kapilvastu, IndiaCopyright © 2024 Basavaraj, Jangid, Babar, Gangana Gowdra, Gangurde, Shinde, Tripathi, Patil, Boraiah, Rane, Harisha, Halli, Sammi Reddy and Prabhakar. This is an open-access article distributed under the terms of the Creative Commons Attribution License (CC BY). The use, distribution or reproduction in other forums is permitted, provided the original author(s) and the copyright owner(s) are credited and that the original publication in this journal is cited, in accordance with accepted academic practice. No use, distribution or reproduction is permitted which does not comply with these terms.
*Correspondence: P. S. Basavaraj, YmFzc3VwdGxAZ21haWwuY29t
Disclaimer: All claims expressed in this article are solely those of the authors and do not necessarily represent those of their affiliated organizations, or those of the publisher, the editors and the reviewers. Any product that may be evaluated in this article or claim that may be made by its manufacturer is not guaranteed or endorsed by the publisher.
Research integrity at Frontiers
Learn more about the work of our research integrity team to safeguard the quality of each article we publish.