- 1College of Food Science and Engineering, Dalian Ocean University, Dalian, China
- 2Faculty of Agriculture, Iwate University, Iwate, Japan
- 3Agri-Innovation Center, Iwate University, Iwate, Japan
The annual changes of the scallop’s adductor muscle in Ca2+-ATPase activity, chymotryptic digestion, endogenous fluorescence spectra, sulfhydryl content, and surface hydrophobicity were studied. Ca2+-ATPase activity peaked in February and reached its minimum in August for males and in July for females. However, no significant differences were observed between males and females throughout the year. The inactivation rate of Ca2+-ATPase activity increased by 17- to 36-fold when the temperature rose from 38°C to 45°C. The ratio of free myosin to bound myosin was approximately 5:4 at 0.5 M KCl and changed to 5:1 at 1.0 M, as determined by modeling. Chymotryptic digestion demonstrated that scallop myosin could be cleaved into S-1/rod portions at 0.1 M and HMM/LMM at 0.5 M KCl. Furthermore, no significant seasonal variations were observed in chymotryptic digestion patterns, endogenous fluorescence, surface hydrophobicity, or sulfhydryl content. In conclusion, the structure and thermal stability of both male and female scallops remained stable throughout the year, making them suitable for processing and preservation.
1 Introduction
Yesso scallops (Mizuhopecten yessoensis) are one of the most economically important aquaculture species worldwide. As a cold-water marine bivalve mollusk, it has been widely cultivated in the northeast area of Japan and Liaoning province in China. The world production of scallops was 1.7 million tons in 2020 (FAO, 2022). The main edible part, the adductor muscle, which is composed of fast (striated) and slow (catch) muscles, has high nutritional value and health-beneficial substances such as peptides, vitamins, and minerals (Odeleye et al., 2019). With their distinctive flavor and delicious taste, scallops ranked as the third largest species in production output among aquaculture mollusks (Palma and Viegas, 2020).
The adductor muscle of the scallop is mainly composed of myofibrillar proteins, of which myosin contains 40% (Craig and Padrón, 2004). Scallop myofibrillar proteins hugely influence the textural properties of muscle, such as water-holding, elasticity, and functional properties (solubility, gelation, emulsification, foaming, etc.) (Hayakawa and Nakai, 2006; Wilding et al., 2008; Zheng et al., 2012). It was reported that heat treatment changed secondary structures and water distribution in proteins, resulting in aggregation, increased hydrophobicity, and cross-linking between myofibrils and muscle fibers. All these contributed to distinguished changes in shear force, hardness, springiness, cohesiveness, and chewiness (Wu et al., 2022a,b). As one of the most functional proteins in myofibrillar protein, myosin is critical for storing and processing muscle-based products such as dried products, ready-to-eat products (surimi products), and canned products (Hayashi and Konno, 2006). Myosin comprises two unique structures: a globular double-head structure called subfragment-1 (S-1) and a long flexible coiled tail called a rod (Harrington and Rodgers, 1984). The head contains ATPase and F-actin binding sites, and the tail region assembles to form filaments under low salt conditions. Satoh et al. compared the Ca2+-ATPase changes of scallop myosin in the presence of Ca2+ or EDTA. The results revealed that Ca2+ unaffected the thermostability of myosin at 0.1 M KCl, while monomeric myosin content and salt solubility decreased more quickly than Ca2+-ATPase, suggesting a faster denaturation of the rod portion than S-1. Contrarily, when heated at 0.5 M KCl, a slower denaturation of the rod portion was observed (Satoh et al., 2012). In scallop adductor muscle, Ca2+ is directly bound to one of the myosin light chains known as the regulatory light chain, which causes muscle contraction in the myosin-linked Ca2+ regulation system (Szent-Gyorgyi et al., 1973; Konno et al., 1981).
It is generally agreed that the thermal stability of fish myosin is deeply dependent on the inhabiting water temperature (Johnston et al., 1973; Nakaya et al., 1995). Hashimoto et al. (1982) studied the thermostabilities of several species of marine fish myofibrils. The results showed that the myosin of cold-water fish was easily denatured upon heating compared to warm-water fish because of its unstable myosin at the molecular level. Yesso scallops are cold-water filter-feeding shellfish with strict requirements on their living environment and are less resistant to stress. Despite the previous investigation, the seasonal-specific thermostability and structural property remained poorly understood.
Therefore, this study explored the thermal stability of scallop myofibrillar proteins at low and high salt concentrations throughout the year for the first time, using the Ca2+-ATPase denaturation profile as an indicator. Meanwhile, the differences in the structural properties among seasons were also investigated initially using chymotryptic digestion, endogenous fluorescence, surface hydrophobicity, and sulfhydryl content. Our findings will provide helpful information and a theoretical basis for storing and effectively utilizing scallop muscle.
2 Materials and methods
2.1 Sample preparation
The 30 male and 30 female live Yesso scallops (Mizuhopecten yessoensis) with an average length of 9.78 ± 0.40 cm and weight of 100.56 ± 11.09 g were bought from a Dalian market between January and December 2019. After transportation to the laboratory using ice bag cooling, the live scallops were placed in oxygenated circulating seawater for 12 h. The scallop adductor muscle was chopped and mixed with 10% sorbitol, left for 20 min at 4°C, and then stored in the freezer at −80°C.
2.2 Preparation of myofibrillar proteins
Myofibrillar proteins were prepared according to the method of our previous study (Jiang et al., 2020) with slight modifications. The addition of EDTA, which chelates divalent metal ions, could reduce the consumption of ATP during homogenization, decrease the binding between myosin and actin, and result in better salt solubility of myofibrils. Consequently, 5 mM EDTA was incorporated into the process of preparing myofibrillar proteins. Two grams of the chopped scallop adductor muscle were washed three times with 10-fold volumes of 0.1 M KCl, 5 mM EDTA, and 20 mM Tris–HCl (pH 7.5) and centrifuged at 4°C, 5000× g for 3 min. The washed muscle was homogenized at 10000 rpm for 30 s repeated three times, followed by centrifuging at 4°C, 5000 × g for 5 min. The pellet was collected and re-suspended in 10-fold volumes of 0.1 M KCl, 20 mM Tris–HCl (pH 7.5), repeated three times. Finally, the suspension was filtered through two layers of gauze. The filtrate was used as myofibrillar proteins.
2.3 Analysis of Ca2+-ATPase activity
Ca2+-ATPase activity was measured at 25°C in a medium of 0.5 M KCl, 5 mM CaCl2, 25 mM Tris–HCl (pH 7.0), 2.0 mg/mL myofibrillar proteins, and 1 mM ATP (Zheng et al., 2012). Liberated inorganic phosphate was measured with an absorbance of 640 nm. The ATPase activity was determined by the following equation:
where ρ is the protein concentration (mg/ml), t represents the reaction time (min), and A is the absorbance at 640 nm.
2.4 Determination of thermal inactivation profile
2.4.1 Ca2+-ATPase inactivation rate (KD)
Scallop myofibrillar proteins were incubated in January, April, July, and October at 38°C and 45°C, with a salt concentration of 0.1 M, 0.5 M, and 1.0 M KCl, for durations ranging from 0 to 90 min. Ca2+-ATPase activity was measured, as described above. The thermal inactivation rate (kD) as described by Hashimoto and Arai (1978) was determined using the following equation:
where C0 and Ct are the relative remaining Ca2+-ATPase activity before and after incubation, and t is the incubation time in seconds.
2.4.2 Inactivation rate simulation curve
The calculated thermal inactivation rate (km) was simulated according to the following equation using the actual kD at 38°C of female scallop myofibrillar proteins in January.
K1 is equivalent to KD at 0.1 M KCl, representing the binding state of myosin in actomyosin. K2 is equivalent to KD of the first 2 min at 1.0 M KCl, representing the released myosin monomer from actomyosin. α is the estimated percentage of myosin in binding form; 1-α is the percentage of myosin in monomer form. Calculated Km could be defined as the inactivation rate at different ratios of the bound and free form of myosin, represented by the inactivation rate at different salt concentrations.
2.5 Determination of structural properties
2.5.1 Chymotryptic digestion
Scallop myofibrillar proteins (1 mg/mL) in January, April, July, and October, respectively, were digested by chymotrypsin at 0.1 M KCl, 20 mM Tris–HCl (pH 7.5), and 1 mM EDTA or 0.5 M KCl, 20 mM Tris–HCl (pH 7.5), and 1 mM CaCl2, at 20°C for 60 min. The ratio of TLCK-treated α-chymotrypsin to scallop myofibrillar proteins was 1/500 (w/w). The digestion was stopped by 2 mM phenylmethylsulfonyl fluoride.
2.5.2 Endogenous fluorescence
Scallop myofibrillar proteins (0.5 mg/mL) in January, April, July, and October were scanned using a spectrofluorometer (RF-1501, Shimadzu, Kyoto, Japan) with an excitation wavelength of 297 nm. The spectral scan range was 250–400 nm with a slit width of 5.0 nm.
2.5.3 Surface hydrophobicity (S0)
Surface hydrophobicity of scallop myofibrillar proteins in January, April, July, and October was determined using an ANS probe according to the method of Alizadeh-Pasdar and Li-Chan (Alizadeh-Pasdar and Li-Chan, 2000) with some modifications due to differences in reagent suppliers. Myofibrillar protein was prepared with the concentrations of 0.05, 0.1, 0.15, and 0.2 mg/mL, mixed with 8 mM ANS, and set at room temperature for 15 min. Fluorescence intensities were immediately measured using a spectrofluorometer (RF-1501, Shimadzu, Kyoto, Japan) with excitation and emission wavelengths of 390 nm and 470 nm, respectively. S0 was calculated from the initial slope of the net relative fluorescence intensity versus the myofibrillar protein concentration.
2.5.4 Determination of total sulfhydryl content
Total sulfhydryl content was determined according to the method of Benjakul et al. (1997) with some modifications. The ratio of the protein-to-reaction solution was modified to 1:4 rather than 1:9. Due to sample variations, we did not achieve satisfactory results using a 1:9 ratio. Therefore, the ratio was modified to 1:4. Scallop myofibrillar proteins (1 mg/mL, 0.5 mL) in January, April, July, and October were mixed with 2 mL 8 M urea, 10 mM EDTA, 2% SDS, and 0.2 M Tris–HCl (pH 7.5) at 4°C and 50 μL 0.1 M sodium phosphate buffer (pH 7.2) containing 10 mM DTNB at 4°C. The mixture was incubated at 40°C for 25 min before measuring absorbance at 412 nm. The total thiol content was calculated using the following equation:
where A is the absorbance value at 412 nm; B is the protein concentration (mg/mL); C is the absorbance factor of 13,600; and D is the dilution factor.
2.6 Electrophoretic analysis
SDS-PAGE was performed following the method of Porzio and Pearson (Porzio and Pearson, 1977) using 7.5% or 12.5% polyacrylamide gels containing 0.1% SDS. The gels were stained with 0.1% Coomassie Brilliant Blue R-250 and then decolorized in a solution of 45% methanol and 9% acetic acid.
2.7 Data analysis
All the measurements were repeated three times. The experimental data were expressed as mean ± standard deviation, and statistical analysis of variance and graphing was carried out using GraphPad Prism 8.0 software. One-way ANOVA was performed on the differences between groups using SPSS 17.0 software, and the significance level was set at a p-value of <0.05.
3 Results and discussion
3.1 Annual variation in Ca2+-ATPase activity
Ca2+-ATPase activity serves as a sensitive indicator, frequently employed for the analysis of the denaturation of myofibrillar proteins, especially myosin (Yin et al., 2019). Annual variations in Ca2+-ATPase activity of male and female scallop myofibrillar proteins are shown in Figure 1. Ca2+-ATPase activity was high around February to March, reaching a maximum of 0.516 and 0.526 μm/(min·mg) for males and females, respectively. As the environmental temperature rose, Ca2+-ATPase activity began to decline. The lowest activity was observed in August at 0.354 μm/(min·mg) for males and 0.320 μm/(min·mg) for females in July. The annual trend of the changes in Ca2+-ATPase activity displayed high activity in autumn and winter when the temperature was low. In contrast, at midsummer around July and August, the Ca2+-ATPase activity displayed the lowest value. Fluctuations in water temperature within fish and shellfish habitats have been reported to be correlated with Ca2+-ATPase activity. In instances where the water temperature is not optimal, organisms experience temperature stress, resulting in a reduction in Ca2+-ATPase activity (Ranjan et al., 2014). Scallops, as a species of cold-water shellfish, have lower Ca2+-ATPase activity during the summer seasons because of stress caused by unsuitable water temperatures. In addition, Ca2+-ATPase activity in scallops was much higher than in most fish, such as grass carp, silver carp, mackerel, and walleye (Benjakul et al., 2003). Compared to terrestrial animals, the nutrient composition of aquatic animals such as fish and shellfish was more easily affected by seasonal changes, habitat, reproductive cycles, and males and females, whereas there were no significant differences between males and females (p > 0.05).
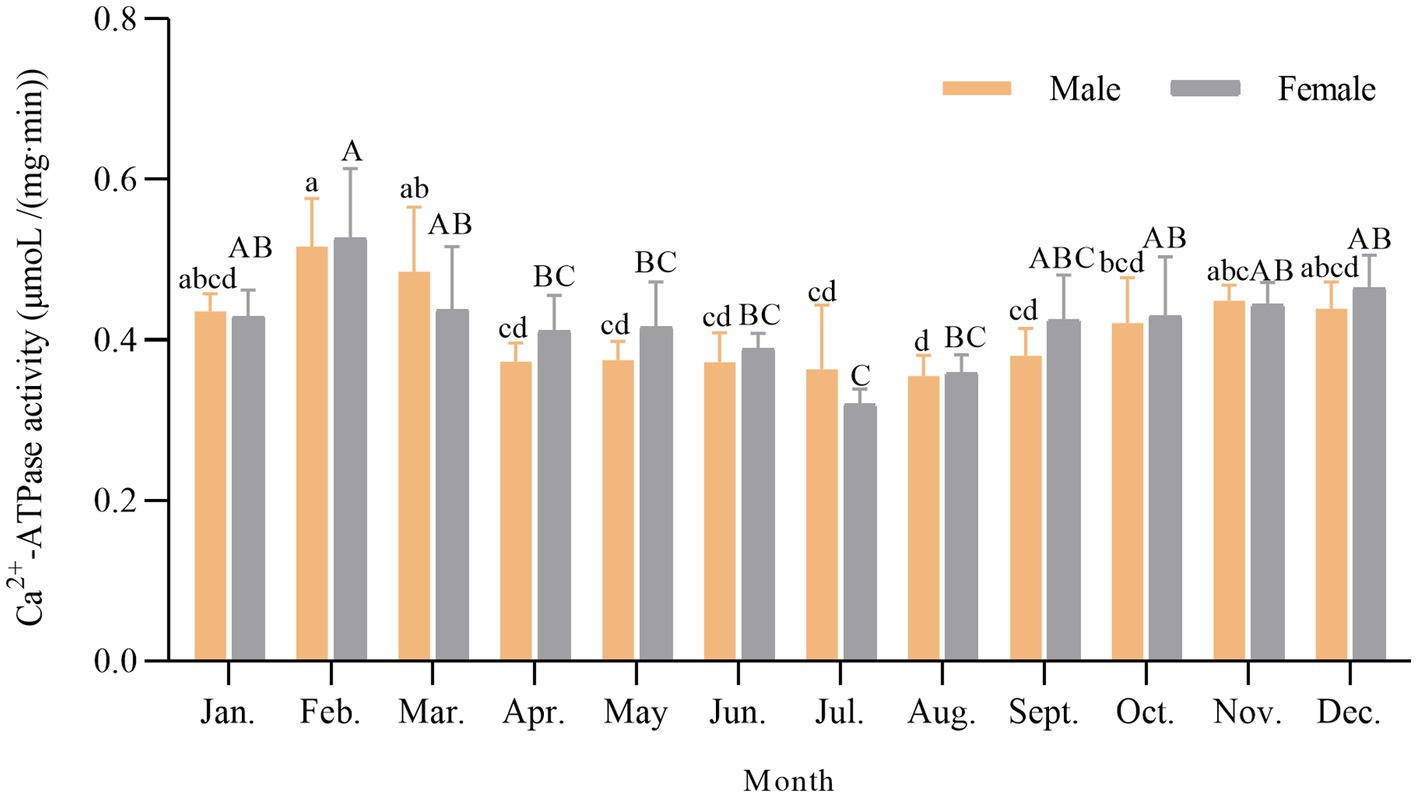
Figure 1. Annual variation of Ca2+-ATPase activity in scallop myofibrillar proteins. Different lowercase letters indicate a significant difference (p < 0.05) in males among different months. Different capital letters indicate a significant difference (p < 0.05) in females among different months.
3.2 Seasonal thermal inactivation profile of scallop myofibrillar proteins
The seasonal differences in the thermal inactivation profile of scallop myofibrillar proteins were investigated by incubating at 38°C (a relatively low temperature) and 45°C (a relatively high temperature) for 0 to 90 min at 0.1 M KCl. The quality of animal protein-based products is determined by the stability of the myofibrillar proteins (Wang et al., 2023). The scallops in January, April, July, and October were used in the following study because of the different representative Ca2+-ATPase as referred to in 3.1. As shown in Figure 2A, KD at 38°C was 7.299 × 10−5/s in January, 1.023 × 10−4/s in April, 5.270 × 10−5/s in July, and 4.792 × 10−5/s in October, respectively. KD at 45°C was 1.670 × 10−3 to 1.750 × 10−3/s among all four seasons, which was accelerated by 17- to 36-fold. No significant seasonal difference was observed among these 4 months (p > 0.05). In contrast to scallops, certain fish species such as silver carp and grass carp exhibited significant seasonal differences in their myosin, with the inactivation rate during winter being 4 to 20 times faster than that during summer (Yuan et al., 2006). The reasons could be attributed to a combination of several factors, including temperature changes, sunlight intensity, and dissolved oxygen content in different seasons, which affected the expression of the genes encoding myosin to a certain extent, resulting in seasonal differences in myosin structure and protein properties (Vornanen, 1994; Imai et al., 1997). However, the seasonal differences in the thermal inactivation rate of scallop myofibrillar proteins were insignificant. It was speculated that the seasonal variation in seawater temperature had less drastic changes, resulting in less structural change and single gene expression of myosin. Nevertheless, the expression patterns of scallop myosin heavy chains in different seasons need to be further studied. The thermal inactivation rate of scallop myofibrillar proteins was relatively slower than other cold-water species, such as Alaska Pollock and rainbow trout, but similar to that found in summer-type myosin of silver carp (Hashimoto et al., 1982; Yuan et al., 2005). When the salt concentration was at 0.1 M KCl, myosin bounded tightly to actin through the S-1 portion. They formed a cross-bridge structure in a post-rigor state, under which actin strongly protected myosin from thermal denaturation (Zhao et al., 2021). The high thermal stability of scallop myofibrillar proteins indicated a greater protective effect from actin compared to fish actin. The differential thermal stability of myofibrillar proteins affected the heating temperature required for gel formation and stability during storage (Yuan et al., 2005; Zhang et al., 2023). The high stability of scallop myofibrillar proteins implied advantages for processing and storage.
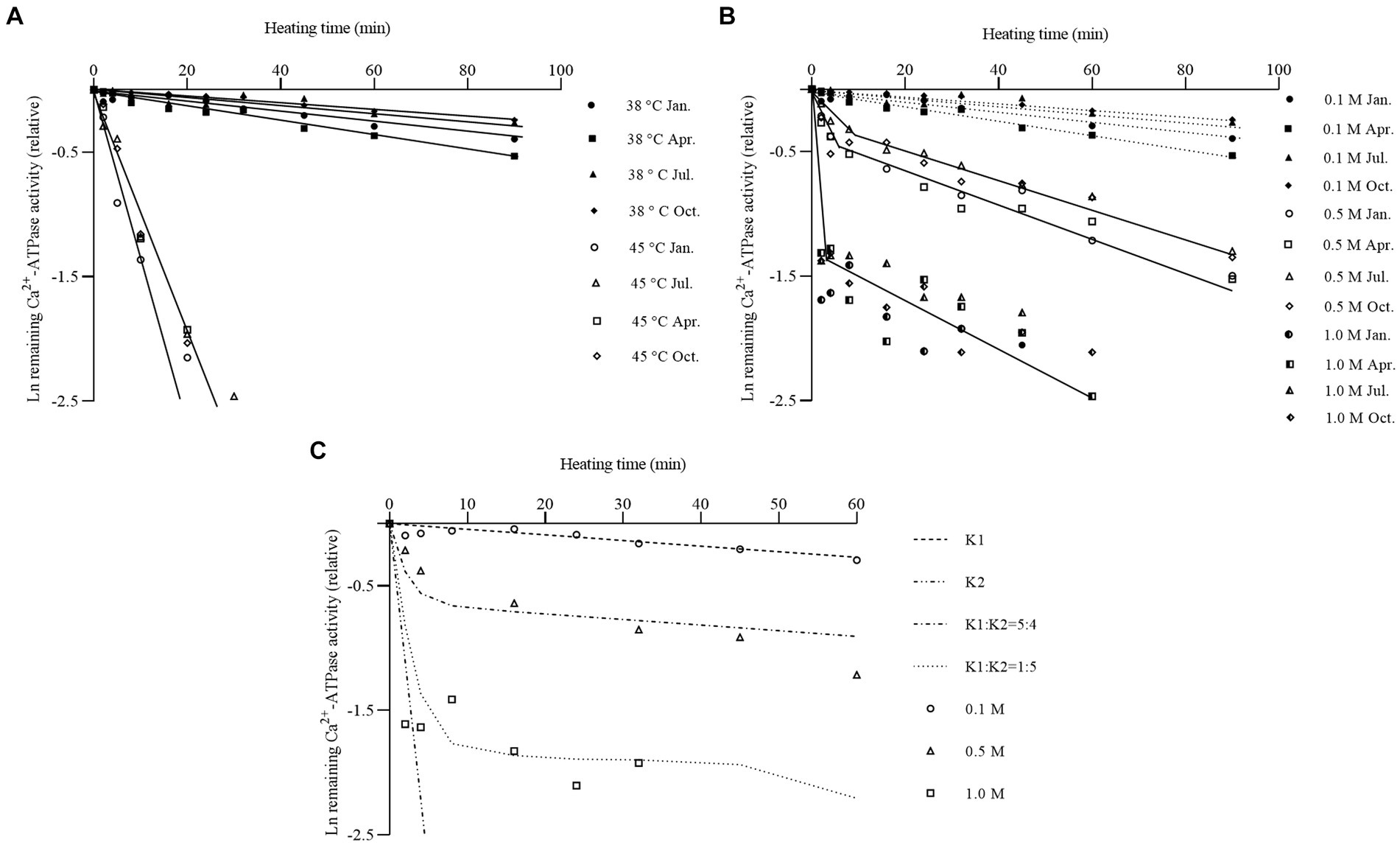
Figure 2. Changes of thermal inactivation profile of scallop myofibrillar proteins in different seasons. (A) Comparison of thermal inactivation profile of scallop myofibrillar proteins at 38°C and 45°C at 0.1 M KCl. Scallop myofibrillar protein in January (circles), April (squares), July (triangles), and October (diamonds) was heated from 0 to 90 min at 38°C (closed symbol) and 45°C (open symbol), respectively. (B) Comparison of thermal inactivation profile of scallop myofibrillar proteins at various salt concentrations at 38°C. Scallop myofibrillar proteins were heated at 38°C in the presence of 0.1 M (closed symbol), 0.5 M (open symbol), and 1.0 M KCl (semi-closed symbol) at January (circles), April (squares), July (triangles), and October (diamonds). (C) Simulated thermal inactivation profile of scallop myofibrillar protein at different salt concentrations. The experimental result at 0.1 M (circles), 0.5 M (triangles), and 1.0 M KCl (squares) was represented by dots. The dashed line represents the simulated inactivation profile. K1 means the bound state of myosin, and K2 means the free state of myosin.
Salt concentration could weaken myosin binding to the thin filaments and dissolve myosin into monomers (Yuan et al., 2011). To investigate the effect of actin bound, the Ca2+-ATPase was measured at 0.5 M and 1.0 M KCl, respectively, after incubating scallop myofibrillar proteins at 38°C from 0 to 60 min. The results are displayed in Figure 2B, with the data of the inactivation rate at 0.1 M shown as a dotted line for comparison. An inflection point could be observed at 0.5 M and 1.0 M KCl, whereas, at 0.1 M KCl, the inactivation rate fitted well with the first-order reaction. KD of the initial speed was approximately 1.75 × 10−3/s to 2.17 × 10−3/s at 0.5 M KCl in four seasons, approximately 23- to 40-fold faster than at 0.1 M KCl. After the salt concentration increased to 1.0 M KCl, the thermal inactivation rate was highly rapid, reaching 0.01/s at the initial speed. The maximum difference was 140- to 200-fold from 0.1 M to 1.0 M KCl. At 0.1 M KCl, myosin exhibited better thermal stability with a slower inactivation rate because of the protective effect of actin. This state could be defined as the stable bound state of myosin. After the salt concentration was raised to 0.5 M KCl, the inactivation rate of myosin showed an obvious inflection point, with a fastened inactivation rate in the initial period and a slow inactivation rate in the latter part. In this condition, myosin bounded weaker to actin. The existence of free myosin probably fastened the inactivation rate in the initial period. The slower inactivation rate in the latter part came from myosin, which was still bound to actin. Some studies also mentioned this result on rabbits, fish, and echinoderms, in which the Ca2+-ATPase activity displayed a rapid drop phase and a slow decreasing phase, corresponding to the thermal inactivation of free myosin and remaining actomyosin (Kawakami et al., 1971; Yuan et al., 2006; Zhao et al., 2021). Nevertheless, the seasonal differences in Ca2+-ATPase inactivation rates could not be detected (p > 0.05).
To simulate inactivation curves at different salt concentrations, the inactivation profile of myosin in winter female scallops (January) was plotted as shown in Figure 2C. KD at 0.1 M KCl was used as the inactivation rate of bound myosin (K1), and KD before 180 s at 1.0 M KCl was used as the inactivation rate of free myosin (K2). The simulated inactivation curves fitted well with the real data. At 0.5 M KCl, the ratio of free myosin to bounded myosin was approximately 5:4. When the salt concentration was increased to 1.0 M KCl, a large amount of myosin monomer appeared, and bound myosin detached from actin into myosin monomers, with a ratio of approximately 1:5.
3.3 Seasonal chymotryptic digestibility patterns of scallop myofibrillar proteins
Chymotryptic digestion is usually used to characterize the molecular structural properties of myosin and the denaturation degree of actin (Zheng et al., 2012; Wang et al., 2022). In general, myosin could be cleavage into subfragment-1 (S-1) and rod at 0.1 M KCl with 1 mM EDTA and heavy meromyosin (HMM) and light meromyosin (LMM) at 0.5 M KCl with 1 mM CaCl2 (Weeds and Pope, 1977). The chymotryptic digestion patterns of scallop myofibrillar proteins in four seasons are shown in Figure 3. Three major protein bands could be observed. Myosin heavy chain (MHC) and actin bands were identified based on their similarities to their counterparts in fish myofibrillar protein. The band between MHC and actin was identified as paramyosin, which could be found in various mollusks (Sonobe et al., 2016; Zhao et al., 2021). There was practically no difference in the patterns obtained from the four seasons. Yuan et al. reported that winter silver carp myosin could be cleavaged into a slightly larger molecular weight of S-1 and a smaller molecular weight of rod compared to summer myosin (Yuan et al., 2011). However, there were no significant differences in chymotryptic digestion patterns observed in scallops, suggesting that the chymotryptic digestion pattern was the same in four seasons, consistent with the Ca2+ATPase inactivation rate. Therefore, scallop myosin had high thermostability and slight fluctuation annually, suitable for preservation and processing.
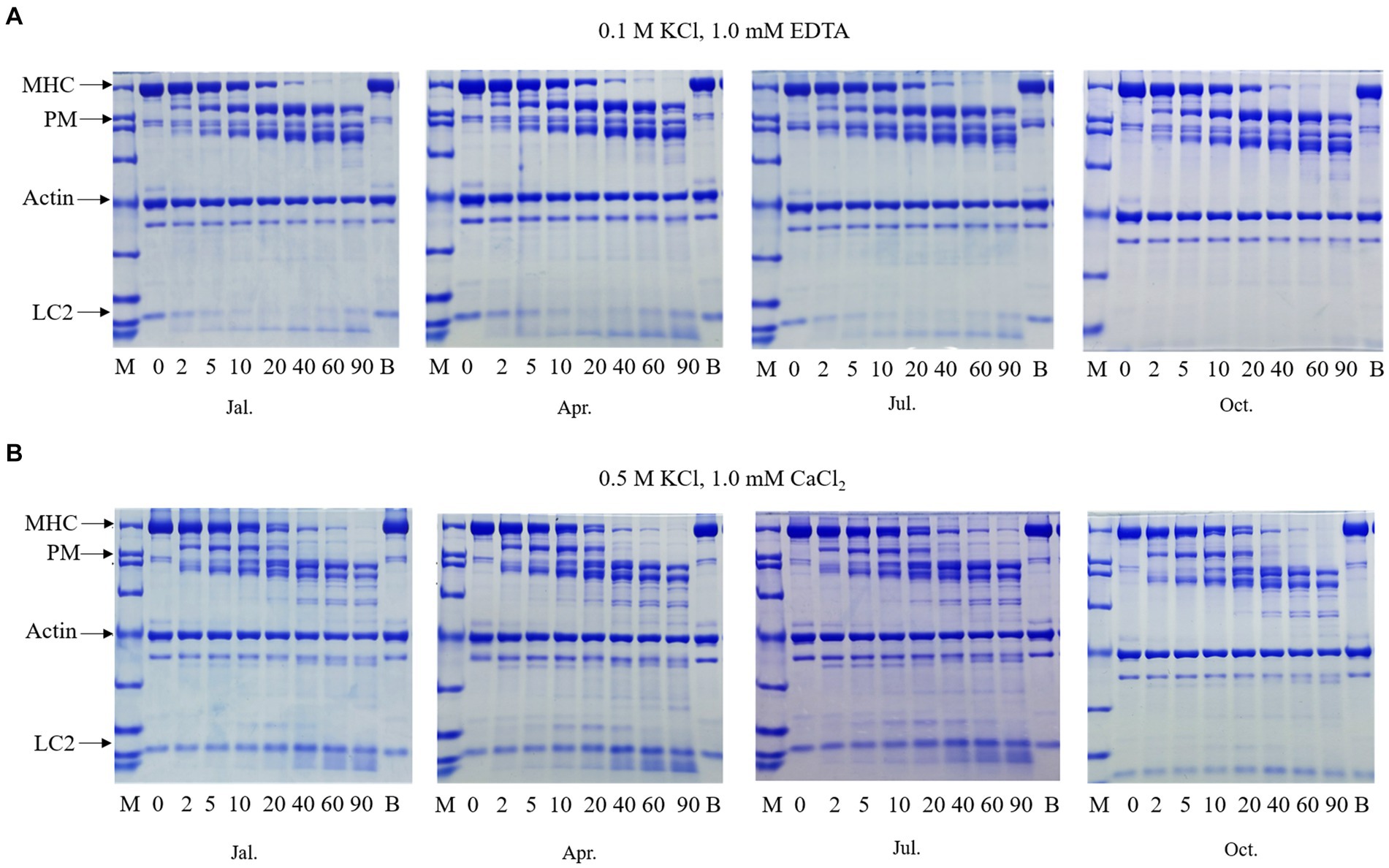
Figure 3. Chymotryptic digestion patterns of scallop myofibrillar protein in different seasons at 0.1 M KCl (A) and 0.5M KCl (B). Scallop myofibrillar proteins from January, April, July, and October were digested at 0.1 M KCl, 1.0 mM EDTA (A), or 0.5 M KCl, 1.0 mM CaCl2 (B) at 20 °C for 60 mins. MHC means myosin heavy chain. PM represents paramyosin. LC 2 means Ca2+-binding light chain. The α-chymotrypsin ratio to protein was 1/500 (w/w).
When digested in a 0.1 M KCl solution, which closely resembles physiological ionic strength, myosin was observed to form fibrils bound to actin and undergo cleavage into S-1 and rod fragments (Figure 3A). After 80% of MHC was digested within 20 min, paramyosin degradation commenced. Myosin probably exhibited a protective effect on paramyosin, which appeared to be enveloped by a layer of myosin. It was reported that high ionic strength could affect the rod portion of myosin, dissociating myosin from the thick filament into myosin monomer (Shen and Swartz, 2010). After adjusting the salt concentration to 0.5 M KCl and adding Ca2+, the degraded rate of MHC was accelerated hugely, as shown in Figure 3B. There were almost no patterns of protein bands at HMM molecular weight at 40 min. These results suggested that the second cleavage site (HMM/LMM junction) contained several cleavable points, similar to the result of sea cucumber (Zhao et al., 2021). Apart from this, paramyosin was digested entirely into small fragments at 2 min due to the lack of myosin protection, earlier than the condition of 0.1 M. Actin band in both 0.1 and 0.5 M KCl exhibited no changes, suggesting its higher thermal and structural stability. Apart from major bands such as myosin, paramyosin, and actin, when comparing SDS-PAGE digested at 0.1 M and 0.5 M KCl, it was observed that one of the myosin light chains, LC2, located at the bottom of the patterns, was nearly completely digested under the conditions of 0.1 M KCl at the 10-min digestion, whereas at the conditions of 0.5 M KCl, the amount of the band during digestion for 10 min remained almost unchanged from the initial stage. This suggested that LC2 is a Ca2+-binding light chain, consistent with previous research (Konno et al., 1981). Hence, when scallops were digested at 0.1 M in the presence of EDTA, which can chelate calcium ions, the degradation of the Ca2+-binding light chain by chymotrypsin played a crucial role in cleaving the myosin heavy chain at the S-1/rod junction. This phenomenon was also observed in other fish species such as yellowtail kingfish and silver carp (Zheng et al., 2012; Liu et al., 2022).
3.4 Seasonal structural properties of scallop myofibrillar proteins
The endogenous fluorescence, surface hydrophobicity (S0), and total sulfhydryl content of myosin were investigated in four seasons to reveal the structural difference. Myosin consists of two spherical heads and a double helix tail (Sharp and Offer, 1992), which contains a large number of protein endogenous chromogenic groups, mainly including tryptophan, tyrosine, and phenylalanine. By measuring the relative intensity of endogenous fluorescence of myosin, it was possible to determine whether there were seasonal differences in the tertiary structure of the scallop myosin molecule. The results are shown in Figure 4. Myosin had a maximum absorption peak at 303 nm for tyrosine fluorescence, and its maximum absorption wavelength and peak did not differ among the four seasons, whether in 0.1 M or 0.5 M KCl solutions. However, at 0.5 M KCl, the peak intensity of myosin absorption at 303 nm was stronger compared to that at 0.1 M KCl. This might be attributed to the fact that at 0.5 M KCl, myofibrils were in a dissolved state, resulting in a greater presence of myosin monomers and thus a stronger absorption peak intensity. Shen and Swartz also demonstrated that high salt concentrations can induce the dissociation of myosin from the thick filament (Shen and Swartz, 2010).
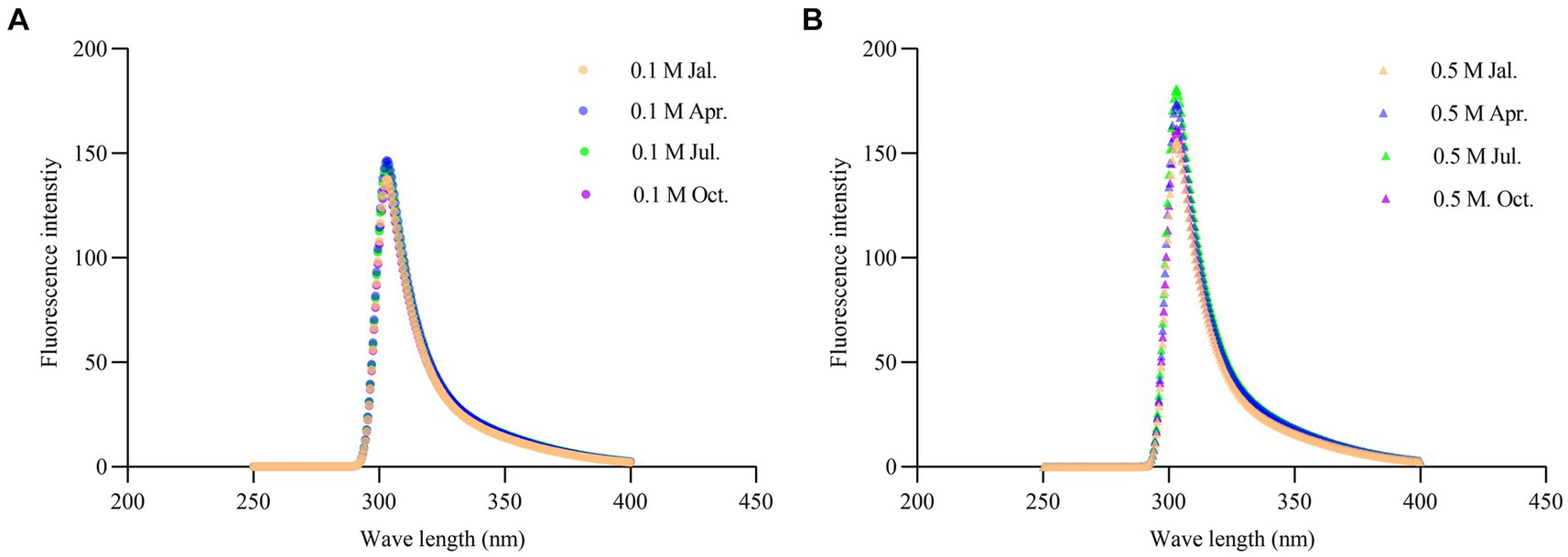
Figure 4. Changes of endogenous fluorescence spectra of scallop myofibrillar protein in different seasons at 0.1 M KCl (A) and 0.5 M KCl (B).
Hydrophobic interactions mainly maintain the tertiary structure of proteins. Measuring the surface hydrophobicity (S0) of proteins could monitor the conformational changes of proteins from the native structures (Yongsawatdigul et al., 2005). Researchers have found that proteins with a higher So were unfolded to a greater extent (Jia et al., 2016; He et al., 2019). ANS fluorescent probes are susceptible to electrostatic forces that create strong interactions with hydrophobic groups of protein molecules, resulting in a significant increase in So. Therefore, it was commonly used to determine the S0 of proteins (Alizadeh-Pasdar and Li-Chan, 2000; Poowakanjana and Park, 2013). The result is shown in Figure 5. S0 of scallop myofibrillar proteins in January, April, July, and October was compared at low (0.1 M KCl) and high (0.5 M KCl) salt concentrations. Scallop myofibrillar proteins treated with 0.5 M KCl had a higher S0 than that with 0.1 M KCl (p < 0.05), which was probably due to the dissociation of myosin by high ionic strength. The increase of myosin monomer enhanced the interaction between myosin and the fluorescent probe, resulting in a higher So. Furthermore, there was no significant difference in the fluorescence intensity of myosin among four seasons (p > 0.05), suggesting that the bound and free states of myosin in scallop myofibrillar proteins did not show significant seasonal differences in their proportions and structures.
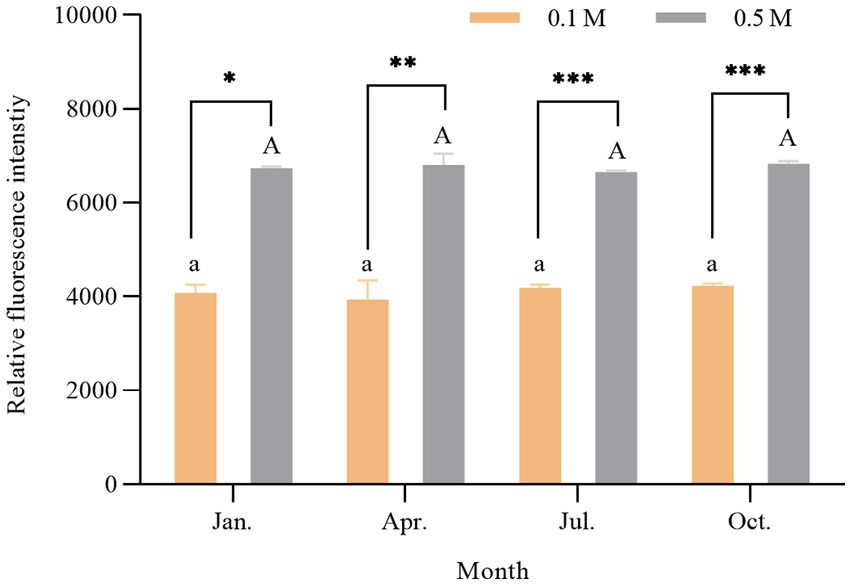
Figure 5. Change of surface hydrophobicity of scallop myofibrillar protein in different seasons. Different lowercase letters indicate a significant difference (p < 0.05) at 0.1 M among different months. Different capital letters indicate a significant difference (p < 0.05) at 0.5 M among different months. * represents p < 0.05; **represents p < 0.01; *** represents p < 0.001.
Sulfhydryl groups are essential functional groups of proteins. There were approximately 42 sulfhydryl groups in myosin, partly located in the head of myosin (approximately 24–26) and partially encapsulated in the tail of myosin (Hamm, 1960). The disulfide covalent bonds, formed from the oxidized sulfhydryl groups, were closely related to the molecular conformation and structural stability of proteins and played an important role in the gel formation process of proteins (Thawornchinsombut and Park, 2007). Figure 6 shows the comparison of the total sulfhydryl content of myosin in scallop myofibrillar proteins in four seasons at 0.1 M KCl (low ionic strength) and 0.5 M KCl (high ionic strength). There was no significant seasonal difference in the protein sulfhydryl content between the low and high salt conditions treatments. The above results of endogenous fluorescence, surface hydrophobicity (S0), and total sulfhydryl content indicated that there were no significant seasonal differences in the structure of myosin.
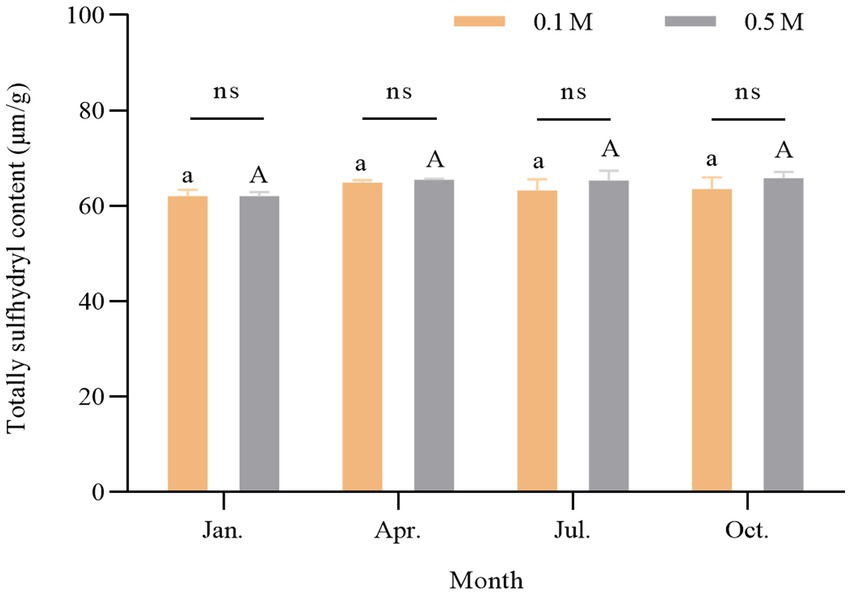
Figure 6. Change of sulfhydryl content of scallop myofibrillar protein in different seasons. Different lowercase letters indicate a significant difference (p < 0.05) at 0.1 M among different months. Different capital letters indicate a significant difference (p < 0.05) at 0.5 M among different months.
4 Conclusion
No significant seasonal differences in the structure of myosin in terms of chymotryptic digestion pattern, endogenous fluorescence, surface hydrophobicity, and sulfhydryl content were observed, suggesting less variation in thermal and structural properties and suitability for processing and preservation throughout the year. Ca2+-ATPase activity of scallop myofibrillar proteins exhibited a temperature-dependent annual change, reaching a maximum of 0.516 and 0.526 μm/(min·mg) in winter (February) for males and females and a minimum in the summer of August at 0.354 for males and 0.320 μm/(min·mg) in July for females. However, no significant annual difference was observed between males and females (p > 0.05). Ca2+-ATPase activity inactivation rate at 38°C was 7.299 × 10−5/s in January, 1.023 × 10−4/s in April, 5.270 × 10−5/s in July, and 4.792 × 10−5/s in October. The rate was accelerated by 17- to 36-fold when heating at 45°C. Actin in scallop myofibrillar protein had a high protective effect on myosin, as evidenced by the high thermal stability at low salinity. Model calculations estimated that approximately 50% of bound myosin was present at 0.5 M KCl. The result of chymotryptic digestion indicated that scallop myosin could be cleavaged into S-1/rod portion by chymotrypsin at 0.1 M and HMM and LMM at 0.5 M KCl. Paramyosin, which was rich in various mollusks, was found to be protected by myosin filaments due to the latter degrading followed by myosin heavy chain. The next study needed to focus on the seasonal variation of Ca2+-ATPase activity and explore further possibilities such as protein modifications.
Data availability statement
The original contributions presented in the study are included in the article/supplementary material, further inquiries can be directed to the corresponding authors.
Ethics statement
The animal study was approved by the Iwate University Institutional Review Board. The study was conducted in accordance with the local legislation and institutional requirements.
Author contributions
YT: Data curation, Writing – original draft, Methodology. MJ: Writing – original draft, Data curation. ZW: Writing – original draft, Methodology, Writing – review & editing. CY: Writing – review & editing.
Funding
The author(s) declare that financial support was received for the research, authorship, and/or publication of this article. This study was supported by the Scientific Research Fund of Liaoning Provincial Education Department of China under Grant No. LJKZ0714 and Key Laboratory of Environment Controlled Aquaculture (Dalian Ocean University), Ministry of Education (Grant No. 202323).
Conflict of interest
The authors declare that the research was conducted in the absence of any commercial or financial relationships that could be construed as a potential conflict of interest.
Publisher’s note
All claims expressed in this article are solely those of the authors and do not necessarily represent those of their affiliated organizations, or those of the publisher, the editors and the reviewers. Any product that may be evaluated in this article, or claim that may be made by its manufacturer, is not guaranteed or endorsed by the publisher.
References
Alizadeh-Pasdar, N., and Li-Chan, E. C. (2000). Comparison of protein surface hydrophobicity measured at various pH values using three different fluorescent probes. J. Agric. Food Chem. 48, 328–334. doi: 10.1021/jf990393p
Benjakul, S., Seymour, T. A., Morrissey, M. T., and An, H. (1997). Physicochemical changes in Pacific whiting muscle proteins during iced storage. J. Food Sci. 62, 729–733. doi: 10.1111/j.1365-2621.1997.tb15445.x
Benjakul, S., Visessanguan, W., Thongkaew, C., and Tanaka, M. (2003). Comparative study on physicochemical changes of muscle proteins from some tropical fish during frozen storage. Food Res. Int. 36, 787–795. doi: 10.1016/S0963-9969(03)00073-5
Craig, R., and Padrón, R. (2004). Chapter 7 molecular structure of the sarcomere. In Myology (3rd edition). eds. A. C. Engel and C. Franzini-Armstrong. (McGraw-Hill), 129–166.
FAO (2022). The state of world fisheries and aquaculture 2022. Towards Blue Transformation. Rome: FAO.
Harrington, W. F., and Rodgers, M. E. (1984). Myosin. Annu. Rev. Biochem. 53, 35–73. doi: 10.1146/annurev.bi.53.070184.000343
Hashimoto, A., and Arai, K.-I. (1978). The effect of pH and temperature of the stability of Myofibrillar ca-ATPase from some fish species. Nippon Suisan Gakkaishi 44, 1389–1393. doi: 10.2331/suisan.44.1389
Hashimoto, A., Kobayashi, A., and Arai, K.-I. (1982). Thermostabilty of fish Myofibrillar ca-ATPase and adaption to Enviromental temperature. Nippon Suisan Gakkaishi 48, 671–684. doi: 10.2331/suisan.48.671
Hayakawa, S., and Nakai, S. (2006). Relationships of hydrophobicity and net charge to the solubility of Milk and soy proteins. J. Food Sci. 50, 486–491. doi: 10.1111/j.1365-2621.1985.tb13433.x
Hayashi, K., and Konno, K. (2006). Stabilization of myosin by ionic compounds as affected by F-actin. Fish. Sci. 72, 1306–1312. doi: 10.1111/j.1444-2906.2006.01294.x
He, Y., Park, J. W., and Yin, T. (2019). Biochemical and gelling properties of silver carp surimi as affected by harvesting season and chopping time. FNP J. Food Process. Preserv. 43:43. doi: 10.1111/jfpp.14247
Imai, J., Hirayama, Y., Kikuchi, K., Kakinuma, M., and Watabe, S. (1997). cDNA cloning of myosin heavy chain isoforms from carp fast skeletal muscle and their gene expression associated with temperature acclimation. J. Exp. Biol. 200, 27–34. doi: 10.1242/jeb.200.1.27
Jia, D., Huang, Q., and Xiong, S. (2016). Chemical interactions and gel properties of black carp actomyosin affected by MTGase and their relationships. Food Chem. 196, 1180–1187. doi: 10.1016/j.foodchem.2015.10.030
Jiang, M., Tian, Y., Yan, L. I., Wang, X., Yuan, C., and Liu, J. (2020). Microstructure change and Myofibrillar protein stability of Patinopecten yessoensis striated adductor muscle during chilling storage. Food Sci. 41, 175–181. doi: 10.7506/spkx1002-6630-20191016-159
Johnston, I. A., Frearson, N., and Goldspink, G. (1973). The effects of environmental temperature on the properties of myofibrillar adenosine triphosphatase from various species of fish. Biochem. J. 133, 735–738. doi: 10.1042/bj1330735
Kawakami, H., Morita, J., Takahashi, K., and Yasui, T. (1971). Thermal denaturation of myosin, heavy meromyosin and subfragment 1. J. Biochem. 70, 635–648. doi: 10.1093/oxfordjournals.jbchem.a129679
Konno, K., Arai, K., Yoshida, M., and Watanabe, S. (1981). Calcium regulation in squid mantle and scallop adductor muscles. J. Biochem. 89, 581–589. doi: 10.1093/oxfordjournals.jbchem.a133234
Liu, Y., Wang, Z., Huang, Y., Konno, K., Tong, T., Yu, C., et al. (2022). Characteristic stable structure of the myosin rod in dark muscle of yellowtail kingfish (Seriola aureovittata). Int. J. Food Sci. Technol. 57, 7917–7928. doi: 10.1111/ijfs.16146
Nakaya, M., Watabe, S., and Ooi, T. (1995). Differences in the thermal stability of acclimation temperature-associated types of carp myosin and its rod on differential scanning calorimetry. Biochemistry 34, 3114–3120. doi: 10.1021/bi00009a043
Odeleye, T., White, W. L., and Lu, J. (2019). Extraction techniques and potential health benefits of bioactive compounds from marine molluscs: a review. Food Funct. 10, 2278–2289. doi: 10.1039/C9FO00172G
Palma, M., and Viegas, I. (2020). “Aquaculture: farming our food in water” in In: Life below water. eds. W. Leal Filho, A. M. Azul, L. Brandli, A. Lange Salvia, and T. Wall (Cham: Springer International Publishing), 1–9.
Poowakanjana, S., and Park, J. W. (2013). Biochemical characterisation of Alaska Pollock, Pacific whiting, and threadfin bream surimi as affected by comminution conditions. Food Chem. 138, 200–207. doi: 10.1016/j.foodchem.2012.09.109
Porzio, M. A., and Pearson, A. M. (1977). Improved resolution of myofibrillar proteins with sodium dodecyl sulfate-polyacrylamide gel electrophoresis. Biochim. Biophys. Acta 490, 27–34. doi: 10.1016/0005-2795(77)90102-7
Ranjan, R., Priyanka, B. S., and Thakur, M. S. (2014). ATPase inhibitor based luciferase assay for prolonged and enhanced ATP pool measurement as an efficient fish freshness indicator. Anal. Bioanal. Chem. 406, 4541–4549. doi: 10.1007/s00216-014-7840-6
Satoh, A., Kinoshita, Y., and Konno, K. (2012). Myosin denaturation in heated myofibrils of scallop adductor muscle. Fish. Sci. 79, 149–155. doi: 10.1007/s12562-012-0565-6
Sharp, A., and Offer, G. (1992). The mechanism of formation of gels from myosin molecules. J. Sci. Food Agric. 58, 63–73. doi: 10.1002/jsfa.2740580112
Shen, Q. W., and Swartz, D. R. (2010). Influence of salt and pyrophosphate on bovine fast and slow myosin S1 dissociation from actin. Meat Sci. 84, 364–370. doi: 10.1016/j.meatsci.2009.09.003
Sonobe, H., Obinata, T., Minokawa, T., Haruta, T., Kawamura, Y., Wakatsuki, S., et al. (2016). Characterization of paramyosin and thin filaments in the smooth muscle of acorn worm, a member of hemichordates. J. Biochem. 160, 369–379. doi: 10.1093/jb/mvw047
Szent-Gyorgyi, A. G., Szentkiralyi, E. M., and Kendrick-Jonas, J. (1973). The light chains of scallop myosin as regulatory subunits. J. Mol. Biol. 74, 179–203. doi: 10.1016/0022-2836(73)90106-X
Thawornchinsombut, S., and Park, J. W. (2007). Effect of nacl on gelation characteristics of acid- and alkali-treated pacific whiting fish protein isolates. J. Food Biochem. 31, 427–455. doi: 10.1111/j.1745-4514.2007.00121.x
Vornanen, M. (1994). Seasonal and temperature-induced changes in myosin heavy chain composition of crucian carp hearts. Am. J. Phys. 267, R1567–R1573. doi: 10.1152/ajpregu.1994.267.6.R1567
Wang, M., Li, Y., Ma, C., Zhang, Z., Guo, L., Huang, M., et al. (2023). Stability of native/thermally denatured myofibrillar protein particles: improvement with decreasing pH. Food Hydrocoll. 140:108628. doi: 10.1016/j.foodhyd.2023.108628
Wang, Z., Niu, Y., Zhao, S., Tian, Y., Yu, K., Yamashita, T., et al. (2022). Thermal stability of actin of silver carp (Hypophthalmichthys molitrix) harvested in summer and winter as affected by myosin complexation. J. Food Process. Preserv. 46:e17003. doi: 10.1111/jfpp.17003
Weeds, A. G., and Pope, B. (1977). Studies on the chymotryptic digestion of myosin. Effects of divalent cations on proteolytic susceptibility. J. Mol. Biol. 111, 129–157. doi: 10.1016/S0022-2836(77)80119-8
Wilding, P., Lillford, P. J., and Regenstein, J. M. (2008). Functional properties of proteins in foods. J. Chem. Technol. Biotechnol. Biotechnol. 34, 182–189. doi: 10.1002/jctb.280340307
Wu, Z. X., Fan, Y. C., Guo, C., Liu, Y. X., Li, D. Y., Jiang, P. F., et al. (2022a). Effects of boiling processing on texture of scallop adductor muscle and its mechanism. Food Secur. 11:1947. doi: 10.3390/foods11131947
Wu, Z. X., Li, D. Y., Shen, M., Wang, Z. Y., Wang, Z. W., Liu, Y. X., et al. (2022b). Effect of different sous-vide cooking conditions on textural properties, protein physiochemical properties and microstructure of scallop (Argopecten irradians) adductor muscle. Food Chem. 394:133470. doi: 10.1016/j.foodchem.2022.133470
Yin, T., He, Y., Liu, L., Shi, L., Xiong, S., You, J., et al. (2019). Structural and biochemical properties of silver carp surimi as affected by comminution method. Food Chem. 287, 85–92. doi: 10.1016/j.foodchem.2019.02.066
Yongsawatdigul, J., Carvajal-Rondanelli, P., and Lanier, T. (2005). Surimi gelation chemistry, in Surimi and Surimi Seafood. 2nd Edn. Ed. J. W. Park. (CRC Press), 435–489.
Yuan, C., Fukuda, Y., Kaneniwa, M., Chen, S., Cheng, Y., Wang, X., et al. (2005). Comparison of gel-forming properties of silver carp (Hypophthalmichthys molitrix) surimi prepared in different seasons. J. Food Sci. 70, C326–C331. doi: 10.1111/j.1365-2621.2005.tb09961.x
Yuan, C., Kaneniwa, M., Wang, X., Chen, S., Cheng, Y., Qu, Y., et al. (2006). Seasonal expression of 2 types of myosin with different Thermostability in silver carp muscle (Hypophthalmichthys molitrix). J. Food Sci. 71, C39–C43. doi: 10.1111/j.1365-2621.2006.tb12386.x
Yuan, C., Wang, X., Chen, S., Qu, Y., and Konno, K. (2011). Structural stability of myosin rod from silver carp as affected by season. J. Food Sci. 76, C686–C693. doi: 10.1111/j.1750-3841.2011.02175.x
Zhang, Y., Bai, G., Wang, J., Wang, Y., Jin, G., Teng, W., et al. (2023). Myofibrillar protein denaturation/oxidation in freezing-thawing impair the heat-induced gelation: mechanisms and control technologies. Trends Food Sci. Technol. 138, 655–670. doi: 10.1016/j.tifs.2023.06.035
Zhao, M., Konno, K., Zhang, N., Liu, Y., Zhou, D., Yu, C., et al. (2021). Characteristic thermal denaturation profile of myosin in the longitudinal retractor muscle of sea cucumber (Stichoupus japonicas). Food Chem. 357:129606. doi: 10.1016/j.foodchem.2021.129606
Keywords: scallop, Ca2+-ATPase, myosin, chymotryptic digestion, endogenous fluorescence spectra, sulfhydryl content, surface hydrophobicity
Citation: Tian Y, Jiang M, Wang Z and Yuan C (2024) Annual variation in thermal and structural properties of yesso scallops (Mizuhopecten yessoensis) adductor muscle. Front. Sustain. Food Syst. 8:1357410. doi: 10.3389/fsufs.2024.1357410
Edited by:
Monica Trif, Centre for Innovative Process Engineering, GermanyReviewed by:
Maryam El Bakali, Abdelmalek Essaadi University, MoroccoPramod K. Prabhakar, National Institute of Food Technology Entrepreneurship and Management, India
Copyright © 2024 Tian, Jiang, Wang and Yuan. This is an open-access article distributed under the terms of the Creative Commons Attribution License (CC BY). The use, distribution or reproduction in other forums is permitted, provided the original author(s) and the copyright owner(s) are credited and that the original publication in this journal is cited, in accordance with accepted academic practice. No use, distribution or reproduction is permitted which does not comply with these terms.
*Correspondence: Zhuolin Wang, emh1b2xpbjZAaXdhdGUtdS5hYy5qcA==