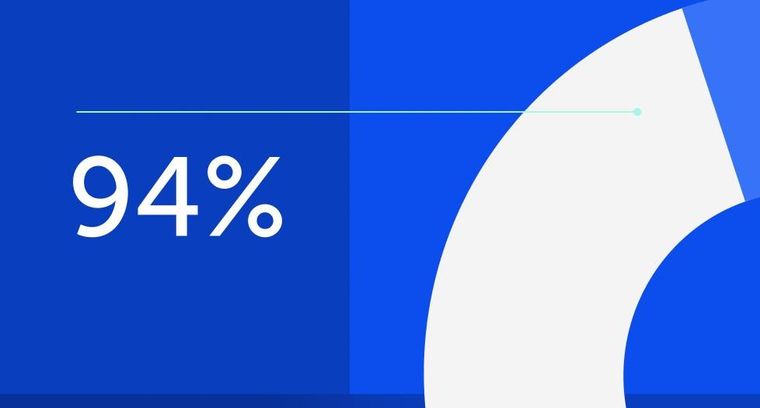
94% of researchers rate our articles as excellent or good
Learn more about the work of our research integrity team to safeguard the quality of each article we publish.
Find out more
ORIGINAL RESEARCH article
Front. Sustain. Food Syst., 26 July 2024
Sec. Agroecology and Ecosystem Services
Volume 8 - 2024 | https://doi.org/10.3389/fsufs.2024.1356579
This article is part of the Research TopicOptimizing Natural Features and BMPs in Agroecosystems Through a One-Health ApproachView all 5 articles
Global pesticide use has resulted in widespread environmental degradation, persistent contamination of surface and ground waters, bioaccumulation of these contaminants in food webs, and unintended impacts on non-target species (e.g., fish kills). Mixtures of multiple pesticides are commonly found in the environment, yet these chemicals are rarely studied in combination. Insecticides are of particular concern as these chemicals are designed to target terrestrial insect pests but also impact aquatic macroinvertebrates. In the following study, we explore the regional context of surface water concentrations of four insecticides in 10 study watersheds over a 20-year period (2002 to 2022) in Prince Edward Island, a region of Canada with continuous agricultural activity since the 1720s. These agroecosystems have been subject to generations of restoration, conservation, and more recently, implementation of various beneficial or best management practices (BMPs). The changing climate significantly adds to the complexity of monitoring these systems as the regional rate of change is exceptionally high (e.g., a 0.70°C to 1.14°C increase in air temperature and 5–8% decrease in precipitation in the last 3 decades). The results of this study highlight that efforts in this area would benefit from a more collaborative, transdisciplinary approach that integrates local, regional, national, and global perspectives while respecting the needs of growers, consumers, and the immense natural capital in the aquatic ecosystems draining these landscapes - such as Atlantic salmon, that can draw both anglers and ecotourists alike. A pivot toward a One-Health Framework is a logical next step for the province as, at present, efforts to integrate observational and monitoring efforts are already conducted by multiple federal, provincial, Indigenous rightsholders, as well as non-government stakeholders. Finally, in recognition that financial resources for these efforts are finite, we would recommend the implementation of a standardized, seasonal water quality sampling scheme that includes flow-weighted sampling and automated samplers to better capture and predict rapidly changing conditions in the region in response to climate change.
Pesticide use is primarily associated with agricultural activity, but both conventional pesticides (natural and synthetic chemicals) and unconventional (e.g., biopesticides and devices) are widely used in other sectors (Tilman et al., 2001; FAO, 2018; IPBES, 2018; Schäfer, 2019). Over the last century, considerable intensification of croplands and agro-forestry has occurred (Tilman et al., 2001). Global use of pesticides (and fertilizers) has greatly increased since the Second World War (Schäfer, 2019). Correspondingly, crop yields have also sharply increased globally in response to increased pesticide use (IPBES, 2018). This intensification also coincides with an increase of pesticides detected in surface waters. For instance, in a survey of surface waters conducted over a 10-year period by the United States Geological Survey, researchers found that 95% of surface water samples contained one or more pesticides (Gilliom, 2007). Half of the waterways surveyed contained five or more pesticides (Gilliom, 2007). Evidence from Canada is patchier but similarly suggests that pesticides are detected in surface waters in as much as 40% of the samples measured (Environment Canada, 2011). In Canada, extensive agricultural activity occurs in Prince Edward Island (PEI), with an estimated 42.5% of total land use on the island attributed to agriculture and approximately 18.3% is used solely for the cultivation of an estimated 100 varieties of potato (PEI, 2016, 2020; Danielescu et al., 2024). Sales of insecticides in the province has tripled increasing from, on average 59,038 ± 7,137 kg of active ingredient (a.i.) between 1993 and 2008, to an average of 177,075 ± 13,136 kg a.i. between 2014 and 2016.1 This is similar to agricultural insecticide sales nationally that have, on average increased 5.21 ± 2.94% year-over-year since 2008.2
Numerous efforts have been made to synthesize habitat, water quality, and ultimately the health of agriculturally impacted watersheds both nationally (e.g., Gagnon et al., 2016; Health Canada, 2021a,b, among others) and internationally (Brock et al., 2000; Gilliom, 2007; Damalas and Eleftherohorinos, 2011; US EPA, 2015; Xu et al., 2016; FAO, 2018; US EPA, 2023). However, even at regional scales, efforts to collaborate across agencies and disciplines tend to be plagued by differences in data collection, and proprietary data control over certain types of information. For instance, pesticide sales and usage data are rarely made public in Canada and when this type of information is released it is at national or regional scales that “protect personal information, confidentiality and information of business value” (Health Canada, 2024). In contrast, Prince Edward Island has continued to make their data more accessible and much of this can be found online.3 This greater data accessibility in the province is likely due to several highly publicized fish kills on the island (see Supplementary Table S1).
Pesticide exposure, notably to known, or “suspected pesticides” in the aquatic environment has been reported as the probable cause of 51 fish kills (ntotal = 61) that have occurred in 27 different PEI watersheds since 1960 (see Xing et al., 2012; PEI, 2016). Pesticides are used to control pests and can be toxic to other organisms, including fish, birds, and beneficial insects among other organisms (e.g., Tudi et al., 2021). Due to the extent of agriculture in the province, and the known potential for negative effects of pesticide exposure to non-target aquatic organisms such as fish, residents of PEI are concerned about the health and integrity of freshwater ecosystems, and particularly of the drinking water supply on the island (PEI, 2016, 2020). In addition to pesticides, other agricultural stressors (e.g., habitat degradation, nutrient enrichment associated with fertilizer use, and increased water withdrawal rates) have also been shown to contribute to changes in non-target aquatic communities (Gormley et al., 2005; Alexander et al., 2007; Davidson and Knapp, 2007; Relyea, 2009; Alexander et al., 2013; Goulson, 2013; Olaya-Arenas et al., 2020; Rossi et al., 2020). In freshwater ecosystems, benthic (bottom-dwelling) macroinvertebrates tend to predominate, and these organisms provide important ecosystem services, not only as primary consumers of algae and detritus, but also as food sources for fish (Merritt and Cummins, 1996). Sensitive orders such as mayflies, stoneflies, and caddisflies (Orders Ephemeroptera, Plecoptera, and Trichoptera, respectively) are particularly responsive to insecticide exposure and habitat alteration and can serve as important sentinels for the potential for cascading effects on other organisms in the food web.
The widespread use of insecticides to control terrestrial insect pests poses a risk to non-target aquatic insects that have similar nervous systems to their terrestrial counterparts (Liess and von der Ohe, 2005; Alexander et al., 2007; Damalas and Eleftherohorinos, 2011; Main et al., 2014; Morrissey et al., 2015; Sheedy et al., 2019). Neonicotinoid insecticides such as clothianidin, imidacloprid, and thiamethoxam, may pose a particular risk to the aquatic environment as these compounds tend to co-occur, are persistent and highly soluble, readily leach from surrounding soils into the aquatic environment and, where present, are likely to cause adverse effects (Xu et al., 2016; Health Canada, 2021a,b; US EPA, 2023). Anthranilic diamide insecticides (e.g., chlorantraniliprole) are a new generation of chemical compounds which have been suggested as a potential replacement for neonicotinoids, as diamides are considered to have low toxicity to beneficial non-target organisms (Schmidt-Jeffris and Nault, 2016; Sanford et al., 2021). At present, the magnitude of the risk these new compounds pose individually, let alone in combination, is largely unknown. Despite the widespread detection of multiple insecticides in freshwaters (e.g., Gilliom, 2007; Goulson, 2013; Main et al., 2014; Morrissey et al., 2015; Health Canada, 2021a,b), only rarely are these substances investigated in combination to determine their long-term effects on the aquatic environment.
Due to several highly publicized fish kills on the Island, there has been a public outcry demanding the implementation of cutting-edge beneficial management practices (PEI Government, 2016; 2022). The overarching objective of our ongoing work in this area was to critically examine whether current surface water monitoring in these agroecosystems is capturing underlying patterns of effects and how greater integration, using approaches such as the One-Health Framework, could inform next steps, ultimately co-developing new beneficial management practices with local partners. In this manuscript, we explore the historical aspect of this work, where we have examined data from 10 focal watersheds over a 20-year period to better understand the interplay between the immense natural capital in the region (e.g., Atlantic salmon), environmental health, and the public’s growing concerns for the safety of their freshwater resources. Specifically, this study investigates the potential environmental effects of the four most common insecticides detected downstream of agricultural activity in Prince Edward Island (imidacloprid, thiamethoxam, clothianidin, and chlorantraniliprole) by assessing changes in habitat integrity, historical water quality, surface water insecticide concentrations, and changes in benthic macroinvertebrate assemblages at 10 focal watersheds over a 20-year period (2002–2022). We also highlight the challenges of sustainable food systems by integrating existing monitoring efforts conducted using a One-Health approach to assess the role of fish kills in these agroecosystems while recommending improved monitoring and water management practices in these important agricultural landscapes. We used: Principal Components Analysis (PCA), to compare patterns in habitat characteristics, surface water quality, insecticide concentrations, and benthic community composition within and between the 10 focal watersheds over time; secondly, we tested the extent of relationships between a major driver of habitat change, specifically, average daily discharge (m3/s) and surface water concentrations of the four commonly used insecticides in moderately vs. severely agriculturally impacted watersheds using Analysis of Covariance (ANCOVA) and regression techniques, and finally, explored how current monitoring approaches could be improved upon based on these findings.
Prince Edward Island (PEI) is a maritime province on the east coast of Canada and is the smallest (~5,600 km2) and most densely populated province in Canada (28 people/km2 vs. 17 people/km2 in neighboring Nova Scotia; Figure 1). The landscape on the island mainly consists of rolling, pastoral hills with a max elevation of 142 m above sea level. Of the total landmass, more than 1,100 km is coastline with many bays, coves, and inlets on the island. Due to the island’s small overall area combined with moderate slope, the average watershed size is also relatively small (~21 km2). There are an estimated 1,300 farms on the island and insecticide sales were estimated to be more than $430,000 CAD or 34% of the Canadian market total in 2019 (PEI Government, 2020). The most commonly purchased insecticides for the protection of potato crops in PEI were also the most commonly detected in PEI waterways and these four were selected for this study: chlorantraniliprole (69% as a foliar application for the protection of 26,422 acres, or 106 km2), clothianidin (95% foliar vs. 5% seed treatment to 4,639 acres, or 19 km2), imidacloprid (65% foliar vs. 15% seed treatment to 13,584 acres, or 55 km2), and thiamethoxam (>95% foliar vs. < 2% seed treatment to 1,360 acres, or 5 km2) (Crawford, unpublished data). Of the 10 focal watersheds examined in this study, four were in the eastern region of the island (Bear, Morell, Montague, and Valleyfield). Four watersheds were in the central region of the island (Wilmot, Dunk, West, and Clyde) and two were selected from the west (Miminegash and Mill) (Figure 1). All study watersheds were classified as either minimally impacted (< 35% of agricultural land use, or ‘min’), moderately impacted (35–50% agricultural land use, or ‘mod’), or severely impacted by agriculture (>50% of land use attributed to agriculture, or ‘sev’) based on their percent of land area attributed to agriculture (Supplementary Table S1).
Figure 1. Percentage of land use attributed to forested areas (green), agricultural development (black), or other activities (white) in ten (10) variably impacted focal watersheds in Prince Edward Island, Canada. In this study, watersheds minimally impacted watersheds (< 35% developed land area or ‘minimally’ disturbed) included the Bear, Miminegash, and Morell rivers, whereas the Montague, Valleyfield, and Mill rivers had more agricultural activity (35–50% or ‘moderately’ impacted), and the most ‘severely’ impacted watersheds included the West, Dunk, Wilmot, and Clyde rivers (> 50% agricultural activity in the watershed by land area).
Over the past two decades, extensive surface water quality sampling has been routinely conducted on the island by different government departments and agencies, with more than 5,800 surface water chemistry samples collected at 93 locations from 33 watersheds across PEI since 2002. For instance, surface water chemistry, benthic macroinvertebrate assemblages, water level and volume, and pesticide surface water concentrations were collected by the province or federal research teams.4 In this study, the focal ‘impacted’ watersheds were then selected based on locations where: (i) fish kills have previously occurred, (ii) insecticides were routinely detected, (iii) detections were above guideline limits, (iv) the percentage of land use within the watershed that is attributed solely to agriculture could be estimated, and (v) whether there was water quality data available for the watershed during the 20-year study period. Due to the selection process, 10 variably impacted watersheds were selected including the minimally impacted Bear, Miminegash, and Morell watersheds, the moderately impacted Montague, Valleyfield, and Mill watersheds, and the severely impacted West, Dunk, Wilmot, and Clyde watersheds (Figure 1; Supplementary Table S1). For 8 of the focal watersheds, Atlantic salmon surveys were also available, and this enabled additional comparisons with respect to historical water quality data (Supplementary Table S1).
Over the course of this study, alkalinity (mg/L), dissolved oxygen (mg/L), conductivity (μS/cm), nitrate (mg/L), pH, total phosphorous (mg/L), and water temperature (°C) were routinely measured. Throughout, only samples collected using standardized methods for environmental testing were used in the final historical water quality database (e.g., Keith, 1996; Eaton et al., 2005; Environment Canada, 2011). Pesticide samples (total analyses n = 10,370) of over 194 different compounds were collected during the open water period in 1-L amber glass sample bottles (EPA vials, Fisher Scientific, Fair Lawn, NJ, United States) from water flowing midstream in each of the study watersheds and analyzed at ISO 9001 certified analytical laboratories.5 Following field collection pesticide samples were processed and uploaded to the PEI open data portal6 and water quality samples to the Atlantic Datastream.7 Pesticide samples in the database contained, 784 insecticide measurements of the four insecticides selected for this study. For clothianidin the analytical limit of quantification was <0.01 ng/mL, for imidacloprid <0.1 ng/mL, chlorantraniliprole <0.1 ng/mL, and thiamethoxam <0.01 ng/mL. Limits of quantification decreased for three of the insecticides over the course of this study, post 2012 for both imidacloprid and chlorantraniliprole (both <0.02 ng/mL) and in 2018 for thiamethoxam to <0.005 ng/mL. When pesticide concentrations were measured at the limit of quantification in the initial historical water quality database, a value of one half of the limit value was used. Subsequent detailed comparisons for example, of concentration versus discharge, excluded insecticide concentrations that were measured at the limit of quantification. Average daily discharge (m3/s) data was obtained from the Water Survey of Canada, historical hydrometric data portal8 and the PEI Open Data Portal (see text footnote 6). Hydrological data was processed using Indicators of Hydrologic Alteration metrics on river flow regimes (Richter et al., 1996).
Benthic macroinvertebrate assemblages were collected at 81 locations in 34 watersheds since 2002 with benthos typically sampled during the fall, low flow period using the CABIN standardized method (see Environment Canada, 2011). As part of the CABIN field protocol, the area to be sampled must be defined before entering the stream by identifying an area of six times the bankfull width and containing shallow, rocky stream riffles (Environment Canada, 2011). The protocol also includes the standardized collection of surrounding habitat characteristics at the sample site, including canopy coverage, streamside vegetation composition, water depth, and substrate characteristics (e.g., pebble count and substrate embeddedness). The habitat data supported the determination of buffer zone width and complexity, as well as soil porosity of the surrounding catchment and substrate composition of the focal watersheds. To collect benthic macroinvertebrate samples, substrate was disturbed by walking upstream in a systematic, zig-zag pattern over a defined area for 3 min while feet are repeatedly kicked vigorously into the substrate to dislodge rock-clinging insects to float into the downstream ‘kick-net’ made of 400 μm mesh (Environment Canada, 2011). Upon collection, all materials inside the kick-net were rinsed down to the collection cup at the bottom of the net and emptied into a sieve to ensure that all materials are collected and transferred into a sample jar (Environment Canada, 2011). The bucket was swirled to separate sediments from samples prior to being preserved with 10% buffered formalin or 95% ethanol (Environment Canada, 2011). Once preserved, samples were then taken to the laboratory and subsampled using a Marchant box subsampler and a minimum of 300 individuals were identified from at least 7 random subsamples (ECCC, 2023). Where more than 50 of the 100 subsamples in the Marchant box were required to achieve a count of 300 organisms, the entire sample was processed (see protocols: ECCC, 2020). Once samples were processed, organisms were identified to family or to the lowest practical level (often genus), and then the total density, taxa richness, and as well as percent of each of the EPT Orders and richness was determined (e.g., diversity of specimens collected within the Orders: Ephemeroptera, Plecoptera, and Trichoptera) (ECCC, 2020). Additional metrics included the percent contribution of some Ephemeroptera families such as the percent of Baetidae. Some taxa are not included in the identification process; specifically, Ostracoda, Cladocera, Copepoda, Rotifera, Porifera, Nematoda, as well as non-aquatic taxa. Upon completion of sample sorting, identification and assessment, data was entered in the CABIN database.9
Principal Components Analysis (PCA), a multivariate technique, was used to compare patterns in habitat characteristics, surface water quality, insecticide concentration, and benthic community composition within and between the 10 focal watersheds. PCA enabled the determination of the variance within the dataset, and which variables (principal components) were contributing the most to variation by reducing many variables to a small number of factors to enable the testing of the strength of relationships among different parameters. Non-metric Multidimensional Scaling (nMDS) and Redundancy Analyses were also conducted to confirm patterns in the PCA and to assist with the removal of redundant and correlated variables in subsequent analyses. Mantel tests were used to evaluate whether spatial correlation contributed to overlapping patterns in variables such as between historical salmon surveys and different watershed locations as well as comparisons of the relative severity of agricultural activity using matrices. The extent of the relationships between average daily discharge (m3/s) and surface water insecticide concentrations (ng/ml or ppb) of insecticides was evaluated using Pearson’s product correlation coefficients, ANCOVA, and Tukey’s Post-Hoc tests were conducted, where appropriate. All analyses were conducted using R statistical software (e.g., version 4.1.2; 2021) and required the use of the {vegan}, {FactoMineR}, {factoextra}, {ggpubr}, and {ade4} packages (Oksanen, 2022). All maps were made using open content provided by the PEI government (Dibblee, 1990)10 and were assembled using QGIS.11
Habitat characteristics for the 10 focal watersheds selected were similar and had overlapping physical properties in each of the agricultural impact levels examined in this study (minimal or ‘min’ <35% developed; moderate or ‘mod’ 35–50% developed, and severe or ‘sev’ >50% agricultural development by land area, Supplementary Table S1). Although the level of agricultural impact significantly differed between agricultural land use intensity groupings (land use vs. impact level: F2,7 = 16.16, p < 0.01), levels of agricultural intensity did not vary with location, and all levels of agricultural development (‘min’, ‘mod’, or ‘sev’) could be found in all parts of the island (Mantel test, r = 0.16, p = 0.15, Figure 1). Minimally impacted streams such as the Bear, Miminegash, and Morell, encompassed the full range of variability in the physical characteristics of the impacted watersheds (e.g., Bear, 2nd order, 0.00617 ± 0.001017 m/m, and only 8.3 ± 1.0 m SE bankfull width vs. Morell, 3rd order, 0.00382 ± 0.00119 m/m, and 21.6 ± 0.9 m SE bankfull width). Minimally impacted streams were also among the watersheds with the greatest canopy coverage (Bear 51–75%, Morell 26–50% and Miminegash 51–75%) compared to more agriculturally impacted systems such as the Valleyfield, Wilmot, and Clyde watersheds that had no canopy coverage during the study period (Supplementary Table S1).
Collectively, differences in habitat characteristics between watersheds over time accounted for an estimated 47.2% of the variation in the habitat data (6.14 of 13 EV) with changes in slope (0.00034–0.00617 m/m), average velocity (0.21–0.83 m/s), substrate size (e.g., 2.5–6.2 cm median diameter or D50) and stream width (e.g., average wetted widths of 3.5–13.5 m) major drivers of the variation between watersheds e.g., (see Figure 2A). For example, significant variation in the habitat data could be correlated with parameters such as stream width (e.g., r wetted width = 0.74 vs. Factor 1, p < 0.001; Figure 2A). Slopes were steeper (>0.004 m/m, or 0.41% slope) in minimally disturbed watersheds, such as the Bear (0.00617 m/m), Morell (0.00382 m/m), and Miminegash (0.0059 m/m) whereas severely impacted watersheds such as the West, Dunk, and Wilmot tended to be flatter in grade compared to average (<0.0025 m/m or < 0.2% average slope of West, Dunk, Wilmot vs. the average grade of all watersheds; 0.0035 m/m or 0.3%). As expected, slope was inversely related to stream order, width, and depth - with steeper slopes associated with narrower, shallower, and minimally impacted upland streams (Supplementary Table S1). However, substrate size was inversely related to average stream velocity, a pattern driven by slower streams such as the Dunk watershed (0.21 ± 0.01 m/s) which had substrates at the upper 95% confidence interval of the median particle size of all streams studied (e.g., D50Dunk = 6.2 ± 1.1 cm vs. 0.72–6.35 cm 95% CI of all watersheds).
Figure 2. (A–D) Principal Components Analysis (PCA) of environmental factors contributing to patterns in PEI watersheds over time including (A) habitat characteristics [top left], (B) surface water quality [top right], (C) insecticide concentrations [bottom left], and (D) benthic macroinvertebrate assemblages [bottom right] in the 10 focal watersheds during the study period. The ellipses are 95% confidence ellipses around group centroids and reflect the level of variation in these systems during the study period. In (B) Alk indicates alkalinity, Cond indicates conductivity, DO indicates dissolved oxygen, NO3 indicates freshwater nitrate concentration, TP indicates total phosphorous concentration, and Temp indicates temperature of surface waters sampled. (C) Insecticide concentrations, as related to different sampling locations and impact gradients, are given in full. (D) Percent of Ephemeroptera, Plecoptera, and Trichoptera in the benthic samples are indicated by %E, %P, and %T whereas Ephemeroptera, Plecoptera and Trichoptera Richness ‘s’ are presented as Es, Ps, and Ts, respectively. Total abundance is represented by ‘N’, and the percent (%) of Oligochaetes, Chironomidae, and Baetidae mayflies are also indicated.
Surface water chemistry in the 10 focal watersheds also indicated differences between highly impacted watersheds such as the Wilmot, Dunk, and Clyde compared to minimally impacted systems such as the Miminegash, Morell, and Bear watersheds over time (Figure 2B; Supplementary Table S1). Severely impacted watersheds had greater nutrient concentrations that were routinely above guidance for nitrate (e.g., > 3 mg/L for Dunk, Wilmot, and Clyde) and total phosphorus (e.g., > 35 μg/L for >50% agriculturally developed watersheds; Figure 2B; Supplementary Table S1). In contrast, minimally impacted focal watersheds had lower nitrate concentrations (0.38–1.22 mg/L) and slightly lower total phosphorous concentrations (41.6–52.4 μg/L) compared to watersheds that were moderately (35–50%) or severely (>50%) impacted by agriculture (Figure 2B; Supplementary Table S1). An analysis of the principal components contributing to variation in these systems shows nitrate and alkalinity concentrations are strongly positively correlated to axis 1 (r NO3- = 0.71, p < 0.0001 vs. Factor 1 with an EV of 2.26 out of 7 total, 32.5% of total variance) while dissolved oxygen was strongly correlated to axis 2 (r = 0.90, p < 0.0001; Factor 2 EV of 1.58 out of 7 total, 22.5% of total variance). These patterns were driven by highly impacted systems such as the Wilmot that had the highest nitrate concentration (6.21 ± 0.18 mg/L vs. all watersheds, 0.38–6.21 mg/L) and was one of the warmest rivers (12.6 ± 0.3°C) and had the lowest dissolved oxygen concentrations (10.5 ± 0.2 mg DO/L) (Figure 2B; Supplementary Table S1). Total phosphorus (TP), by contrast, was inversely correlated to increasing nitrate concentrations (e.g., TP vs. NO3-: r = −0.49, p < 0.001) and TP enrichment was closely associated with systems such as the severely impacted West watershed (Figure 2B; Supplementary Table S1).
Elevated concentrations and frequent detections of insecticides were found in severely agriculturally impacted watersheds, such as the Wilmot, whereas few detections and no guidelines were exceeded in the Morell River, the only minimally impacted watershed with insecticide data (Supplementary Table S1). When clothianidin or imidacloprid were detected in the severely impacted Dunk watershed, concentrations were detected at or above guidance in 86% of samples collected (Supplementary Table S1). Insecticide concentrations also significantly differed over time in the dataset (Concentration vs. Year: F 1,7,423 = 60.56, p < 0.001) with increases in both the concentration and frequency of detection associated with more recent contemporary sampling events rather than historical measurements (p < 0.01). For example, concentrations of imidacloprid, thiamethoxam, clothianidin and chlorantraniliprole all increased an order of magnitude and were, on average, 260 times (95% CI 13.97–507.03) more likely to be detected above the limit of quantification post 2012, a period that overlaps with improved analytical limits of quantification of surface water samples (Observed vs. Expected Frequency: χ2 = 263.82, df = 3; p < 0.01). Temporal trends were particularly marked for more contemporary insecticides such as chlorantraniliprole (registered circa 2008) that were not detected at concentrations above the limit of quantification prior to July 2013, as opposed to the imidacloprid (registered in 1994) that was detected at levels exceeding guidelines even prior to improvements in analytical limits of quantification. Patterns of increased concentrations over time were most marked when discharge was included as a covariate (e.g., Dunk R., [chlorantraniliprole] vs. Discharge x Year: F 1,17 = 9.74, p < 0.01) with inter-annual variation in discharge significantly contributing to variation in concentration over time (e.g., ANCOVA: Wilmot R., [chlorantraniliprole] vs. Discharge x Year, F 14,15 = 6.18, p = 0.03).
Changes in insecticide concentrations in surface waters over time also explained 73.3% of the total variation in the dataset (e.g., first two factors plotted on x- and y-axis respectively, E.V. = 2.93 of 4, or 73.3%, see Figure 2C). An estimated 43.7% of this variation could be attributed to the detection of chlorantraniliprole and clothianidin in surface waters of the >50% impacted watersheds such as the Wilmot (Supplementary Table S1) whereas minimally disturbed systems such as the Morell had 4x lower concentrations of chlorantraniliprole (0.017 ± 0.003 ng/mL) and 21x lower concentrations of clothianidin (0.005 ± 0.0002 ng/mL) e.g., (see x-axis, Factor 1, 1.75 of 4, Figure 2C, Supplementary Table S1). Elevated concentrations of imidacloprid and thiamethoxam explained 29.6% of the total variation in the dataset and were associated with different river systems including the severely impacted Clyde, Dunk, and West, and the moderately impacted Montague and Mill watersheds, respectively (Figure 2C Supplementary Table S1). Notably, where thiamethoxam concentrations increased, imidacloprid concentrations remained among the lowest detected (e.g., Montague: 0.0074 ± 0.0001 and Mill: 0.0058 ± 0.0004 ng thiamethoxam/mL vs. Montague: 0.016 ± 0.003 and Mill: 0.016 ± 0.004 ng imidacloprid/mL; Figure 2C).
Five of the 10 focal watersheds (Clyde, Dunk, Mill, Montague, and Wilmot) had reduced richness overall of sensitive sentinel species such as the orders of Ephemeroptera, Plecoptera, and Trichoptera with 46.7% of the total variation explained by the first two factors over time (EV 5.61 of 12 total, see Figure 2D). The first axis (28.9% of variation) was predominantly associated with increased richness and percent relative abundance of Ephemeroptera and Trichoptera in minimally impacted systems such as the Morell watershed (e.g., Trichoptera Richness, Ts: r = 0.79, p < 0.0001; Ephemeroptera Richness, Es: r = 0.75, p < 0.0001; Percent Trichoptera, %T: r = 0.71, p < 0.0001; Figure 2D). Increased abundance and richness of Plecoptera was both strongly positively associated with the second axis (Percent Plecoptera or %P vs. Factor 2: r = 0.88, p < 0.0001; as well as Plecoptera Richness, Ps: r = 0.67, p < 0.0001, respectively) and this pattern was particularly evident in minimally disturbed watersheds such as the Bear watershed (e.g., 19.1% of variation in Factor 2, Figure 2D). By contrast, benthic assemblages from systems with greater agricultural activity, such as the Dunk, tended to be negatively correlated with the percent of Plecoptera (r = −0.71, p < 0.0001) and were strongly associated with increased percentages of oligochaetes. For example, 75% of all organisms collected in the Dunk River were oligochaetes in 2018.
Increases in stream discharge associated with increased precipitation had effects on stream water quality (e.g., increased turbidity) and these changes were visually apparent, particularly in severely impacted systems such as the Dunk (Figure 3). Further, given that significant variation in the habitat data from the 10 focal watersheds could be correlated with parameters such as increasing stream order, stream width (e.g., r wet width = 0.74 of Factor 1, p < 0.001; r bankfull = 0.61, p < 0.01), as well as decreasing velocity (e.g., r = −0.49, p = 0.02) (Supplementary Table S1, Figure 2), it was expected that discharge (e.g., 1961–2002 for Dunk R., Figure 3), due to the potential for overland flow draining agricultural fields, could have a role in the increased concentrations of the four commonly detected insecticides examined in this study. For instance, in the Dunk and Wilmot rivers, chlorantraniliprole (F1,35 = 14.75, p < 0.001) and clothianidin (F1,34 = 5.35, p = 0.03) concentrations significantly covary with changes in surface water discharge (ANCOVA p < 0.05). Neither imidacloprid (ANCOVA: F42,43 = 2.59, p = 0.12) nor thiamethoxam (ANCOVA: F38,39 = 1.48, p = 0.23) concentrations significantly covaried with average daily discharge, likely because of the high frequency that these insecticides were measured in surface waters at their analytical limits of quantification historically (see Discussion).
Figure 3. (A–D) Overview of Dunk River watershed. Photos of North Brook, a tributary of the Dunk River that is 650 m upstream of the Water Survey of Canada gaging station, #01CB002, on 5 October 2005 before (A), and the same site on 23 November 2005 after a nine-day series of >10 mm and two of those days with >65 mm in total precipitation. RapidEye® Remote Satellite imagery of the Dunk River watershed with the gaging station, 01CB002 (yellow star) and the North Brook photographs (red circle) indicated. (C) The median daily discharge for the Water Survey of Canada gaging station 01CB002 in m3/s ± the coefficient of dispersion. (D) The precipitation events in (B) coincided with daily discharge rates >2.11 and > 2.48 m3/s (upper 95% Confidence Interval) at the downstream gaging station, 01CB002, for October and November, respectively. (B,D) Discharge data for station 01CB002 is available at https://wateroffice.ec.gc.ca/ and climate data for Charlottetown https://climate.weather.gc.ca/. Photos provided with permission and courtesy of Eric Luiker.
For the Dunk and the Wilmot rivers, there was sufficient data to permit further comparisons with respect to whether discharge could be related to surface water concentrations of chlorantraniliprole and clothianidin (Figure 4). Specifically, chlorantraniliprole concentration were positively correlated with average daily discharge in the Wilmot (r linear = 0.66; r exp = 0.68; p < 0.01), as were clothianidin concentrations (Wilmot: r linear = 0.40, r logxlog = 0.64, p < 0.01; Figure 4). The relationship between concentration and discharge was more complex in the Dunk watershed and although both chlorantraniliprole (r linear = 0.30) and clothianidin (r linear = 0.26) were moderately positively correlated to average daily discharge, both insecticide subsets in the Dunk were bimodal, with stronger positive correlations to discharge when flow was above 1.5 m3/s (r chlorantraniliprole = 0.78; r clothianidin = 0.76; Figure 4). No surface water quality or pesticide samples have been collected at average daily discharge rates greater than 2.38 m3/s in either the Dunk of Wilmot watersheds. The average daily discharge during the pesticide surface water sampling was 1.35 ± 0.10 m3/s (1.15–1.55 m3/s 95% CI, n = 21) and 0.46 ± 0.05 m3/s (0.37–0.55 m3/s 95% CI, n = 18) for the Dunk and the Wilmot watersheds, respectively.
Figure 4. (A,B) Concentration in ng/ml, of (A) chlorantraniliprole [top panel], and (B) clothianidin [bottom panel] in the Wilmot [‘×’, see legend] and Dunk [gray circles] watersheds versus average daily discharge (m3/s). Linear correlations shown.
This study investigated historical changes in habitat integrity, water quality, insecticide concentrations, and benthic macroinvertebrate assemblages within the context of four commonly used insecticides (imidacloprid, thiamethoxam, clothianidin, and chlorantraniliprole) in Prince Edward Island at 10 focal watersheds. Study locations where fish kills have previously occurred (e.g., Mill, Wilmot, Dunk, and Clyde watersheds), and where concentrations of insecticides were detected above guideline levels (e.g., Montague, Mill, Dunk, Wilmot, and Clyde) were of particular interest for further study (number of fish kills, salmon redds, and insecticide detections are given in Supplementary Table S1). Multivariate analyses highlighted that extensive habitat modification of these waterways has occurred (Figure 2A), that elevated surface water concentrations of nitrate and total phosphorus were commonplace (Figure 2B), that insecticide concentrations were very strong predictors (> 73%) of variation in these systems (e.g., 2.93 of 4 EV, Figure 2C), and that benthic macroinvertebrate communities had greater richness and percent relative abundance of sensitive EPT taxa in minimally impacted watersheds such as the Morell and the Bear rivers (Figure 2D). This study also found a relationship between average daily discharge (m3/s) and the concentration of two insecticides (chlorantraniliprole and clothianidin) and that these relationships were exponential or log x log linear in the Wilmot River (e.g., chlorantraniliprole: r exp = 0.68, p < 0.01) (Figure 4). Patterns in the Dunk River, however, were more complex and although strong positive correlations to discharge were found, these relationships were stronger when average daily discharge was above 1.5 m3/s (r chlorantraniliprole = 0.78; r clothianidin = 0.76) (Figure 4). No relationship between habitat, surface water quality, or insecticide concentrations and estimates of Atlantic salmon success or density (e.g., fish/100 m2 or number of redds) could be discerned (Mantel r < 0.01, p > 0.60; Supplementary Table S1).
PEI’s soils are typically sandstone and tend to be highly permeable and erodible (Alberto et al., 2016). For instance, the Mill River has a hydraulic conductivity of 17.52 m/day with a very high runoff potential (Mockus et al., 1972; Bhatti et al., 2021). In this study, seven of the watersheds (minimally disturbed to severely impacted: Morell, Valleyfield, Montague, Mill, Wilmot, Dunk, and Clyde) have also been heavily modified via impoundments, numerous restoration activities, and agricultural activity (Figure 1). The high hydraulic connectivity and risk of soil erosion, combined with agricultural techniques such as low residue cropping and intensive tilling, have contributed to significant levels of sediment entering PEI watersheds historically (Nyiraneza et al., 2017). The resulting fine sediments in surrounding substrates in the more agriculturally impacted watersheds in this study are likely a potent barrier to the success of both benthic macroinvertebrates and fish (Supplementary Table S1; Newcombe, 2003; Guignion et al., 2019). However, the conventional approach to salmon conservation is that the highest densities of juvenile Atlantic salmon (Salmo salar) and salmon fry will be found in well sorted, unembedded, rounded gravel-cobble substrates e.g., (see Gage et al., 2004; Guignion et al., 2019). However, in this study, strong pattern between the parameters measured and salmon densities could not be discerned (e.g., Mantel r salmon density = −0.08, r redds = −0.09, p ≥ 0.60) (see also Supplementary Table S1). Rather, this 20-year dataset highlights that generations of restoration, conservation, and more recently, implementation of various beneficial management practices has contributed to significant changes in the size and distribution of dominant and surrounding substrates in these systems - such as the larger than expected dominant substrates in the intensively farmed, slow velocity Dunk River watershed (Supplementary Table S1; Figure 2A). Although a single metric (e.g., D50) has long been sought to assess habitat quality for salmon, that large organisms likely have varying gravel requirements over their complex life cycle, is as yet poorly integrated into current restoration efforts (see Kondolf, 2000 for a discussion on metrics).
Differences in surface water chemistry were noted between minimally (< 35% agriculturally developed) and severely degraded (> 50% agricultural) watersheds in this study (Figure 2B, Supplementary Table S1). Minimally impacted watersheds had lower alkalinity, conductivity, and nitrate concentrations compared to severely impacted systems such as the Wilmot that had elevated alkalinity, conductivity, and nitrate (Supplementary Table S1). Notably, the Wilmot watershed, a system 78% impacted by agriculture by land area, had some of the highest concentrations of all parameters measured (Supplementary Table S1; Figure 2B). Nitrate concentrations in the Mill, Dunk, Clyde, and Wilmot also routinely exceeded the recommended federal guideline of 3 mg [NO3−]/L during the study period (>88% of observations, or 143 occasions of n total = 162 samples) (CCME, 2007a, 2012). For some systems, nitrate levels exceeded guidelines in more than 90% of the samples taken [e.g., in severely impacted systems such as the Dunk (100% exc), Clyde (94%), and Wilmot (99%)]. PEI currently uses a ‘site-specific’ total phosphorus guideline of 70 μg TP/L (CCME, 2004; ECCC, 2023). The current site-specific total phosphorus guideline was exceeded even in moderately impacted watersheds [e.g., Montague (23%)] and, like surface water nitrate concentrations, frequently exceeded in severely impacted watersheds in this 20-year dataset [e.g., Clyde (37%), West (41%), and Wilmot (47%)].
Nitrate is the most common form of nitrogen in PEI freshwaters and is strongly associated with elevated agricultural activity (Somers et al., 1999; Danielescu and MacQuarrie, 2011; Lawniczak et al., 2016; Liang et al., 2020). In intensively farmed areas, such as PEI, where nitrate is used in excess as a fertilizer, as much as 60% of applied nitrate has the potential to leach from the application site and contaminate surface and groundwaters (Benson et al., 2006; Shukla and Saxena, 2018; Bijay-Singh and Craswell, 2021; Danielescu et al., 2024). Previous studies have also indicated that watersheds with greater than 35% land use attributed to agriculture experience increased concentrations of nitrate, phosphorus, and reduced dissolved oxygen which has been associated with an increased risk of eutrophication (Somers et al., 1999; Liu et al., 2022). In this study, concentrations of nitrate were 6x greater in >50% agriculturally developed watersheds such as the Wilmot (6.21 ± 0.18 mg/L), Dunk (4.67 ± 0.30 mg/L), and Clyde (3.53 ± 0.10 mg/L) compared to <35% minimally impacted Bear (0.38 ± 0.07 mg/L), Miminegash (1.22 ± 0.17 mg/L), and Morell (0.99 ± 0.07 mg/L) (Supplementary Table S1). Further, elevated nitrate levels in PEI groundwaters have been shown to persist for several decades (Jiang et al., 2007), and surface water nitrate concentrations in some of the study watersheds [such as the Morell (min), Mill (mod), Dunk (sev), and Wilmot (sev)] have been increasing since long term nitrate studies began in the 1960s (see Zebarth et al., 2015). As many of the focal watersheds are at least partially groundwater fed, particularly during the summer, low-flow, open water period, persistent groundwater concentrations likely influence stream water quality (e.g., Wilmot watershed is ~66% groundwater fed according to Jiang et al., 2007).
Surface water concentration of insecticides was a major driver of watershed impactedness and explained 73% of the total variation in the dataset (Figure 2C). Over 60% of this variation was explained by elevated concentrations of chlorantraniliprole and clothianidin in severely (>50%) impacted watersheds such as the Wilmot [0.121 ± 0.014 ng (chlorantraniliprole)/mL, and 0.134 ± 0.045 ng (clothianidin)/mL, respectively; Figure 2C; Supplementary Table S1]. In contrast, the lowest concentrations of chlorantraniliprole, clothianidin, imidacloprid, and thiamethoxam were found in the minimally impacted Morell watershed (Supplementary Table S1). The highest concentration of thiamethoxam was found in the 30–50% moderately impacted Montague watershed [0.0074 ± 0.0001 ng (thiamethoxam)/mL; Supplementary Table S1]. Both clothianidin and imidacloprid were measured at concentrations that are toxic to sensitive aquatic species such as Cloeon spp. mayflies and Chironomus midges (discussed below). For instance, clothianidin was frequently detected in focal watersheds and routinely (37% of observations) exceeded guidance (> 0.02 ng/mL; Health Canada, 2021c) (n detected = 114, n total = 196, n exceeded = 72). This pattern of clothianidin detections above guidance was most pronounced in the moderately 35–50% impacted Montague, and Mill River systems, as well as in the severely >50% impacted Dunk, Wilmot, and Clyde watersheds (93% obs, n = 113). As a highly soluble (327 mg/L) compound, clothianidin is thought to be less likely to bind to soils and is reported to have a higher risk of entering streams via run off as well as during extreme flow events (Miles et al., 2017; Yadav and Watanabe, 2018). Clothianidin is also the breakdown transformation product of thiamethoxam in some soils, plants, insects (see Liu et al., 2018), and thus the field application of either clothianidin or thiamethoxam could both be contributing to elevated levels of clothianidin in surface and groundwaters (US EPA, 2020; Health Canada, 2021a). The remaining 58% (n detected = 114 of 196) of clothianidin detections that did not exceed current guidance overlap with concentrations that affect the successful adult emergence of aquatic insects such as the Dipteran, Chironomus dilutus (7-d E20 = 0.02 ng a.i./ml, see Cavallaro et al., 2017). Reduced success of highly abundant species, such as Chironomidae, could dramatically reshape aquatic community composition (see Hulot et al., 2000).
Surface water samples analyzed for imidacloprid and thiamethoxam were frequently measured at or below the analytical limits of quantification in the historical dataset (94 and 93% of observations respectively). For example, prior to 2013, the analytical limit of quantification of imidacloprid is higher than current Canadian guidelines (DL <0.1 pre-2013 and < 0.02 ng/mL thereafter vs. current guidance of 0.041 ng/mL, formerly 0.23 ng/mL CCME, 2007b; Health Canada, 2016). Only 6 % (6%) of imidacloprid samples were above the analytical limit of quantification (n = 12 of 196 observations). Concentrations less than the current analytical limit of quantification of imidacloprid have been associated with 10% mortality of Cloeon spp. in a laboratory setting (96-h LC10 < 0.0001 ng/mL, Sumon et al., 2018). Imidacloprid concentrations in the range of the latest guidance (0.041 ng/mL) have also been associated with 10% mortality of the mayfly larvae Cloeon dipterum (28-d, LC10 = 0.041 ng/mL; Roessink et al., 2013). Although the concentration of imidacloprid in PEI surface waters is still largely unknown, these results suggest there could be very low mayfly density in watersheds where these substances are present. As preferred prey items of both salmon and trout, preventing reductions in mayfly biomass has previously been recommended as clearly needed for successful salmon restoration and management efforts (see Ward et al., 2009). The absence of these preferred food items could also be contributing to the cumulative risk of fish kills in these watersheds as the greater energetic costs of physiological detoxification post exposure to contaminants, combined with changes in food consumption, could work in combination to reduce the success of sensitive fishes in these watersheds.
Six of the focal watersheds, including the moderately impacted Mill, and Montague, as well as the severely impacted Dunk, Clyde, West, and Wilmot, had reduced richness of sensitive, sentinel EPT orders (Ephemeroptera, Plecoptera, and Trichoptera; Figure 2D). In contrast, minimally disturbed systems, such as the Morell and Miminegash, were strongly associated with greater species richness of Trichoptera, and somewhat of Ephemeroptera, while the Bear River was strongly associated with increased Plecoptera richness (or stoneflies) (Figure 2D). Restoration and remediation efforts in severely impacted systems such as the Clyde and the Dunk, are also clearly evident in the multivariate analysis (e.g., Figure 2A, Supplementary Table S1). Significant habitat alteration due to storm events and construction (e.g., Clyde) or restoration efforts (Dunk, West), act as major drivers in these systems making these watersheds appear extremely disturbed while these efforts are underway and in some cases for years following the restoration activity. For instance, severely, or > 50% impacted watersheds, such as the Clyde and the Dunk, were both strongly associated with long-term increases in the percent of oligochaetes (Figure 2D). Sensitive EPT assemblages may be especially vulnerable to significant habitat disturbances due to their relatively poor dispersal ability and reliance on high water and substrate quality such as well-sorted cobble (see Gage et al., 2004). However, stream restoration is a relatively new area of research and, to date, the effectiveness of this work to support macroinvertebrates has had mixed results with prolonged disruption to benthic communities commonly reported (e.g., Jähnig et al., 2010; Palmer et al., 2010; Bernhardt and Palmer, 2011). Further, the literature also suggests that restoration efforts that focus on the benefit of a single species, such as Atlantic salmon, although inherently worthwhile and warranted as important forms of natural capital, are thought to be among the least sustainable as these efforts, as these approaches may not sufficiently consider the needs of other flora and fauna within an interconnected food web (see Muotka et al., 2002; Louhi et al., 2011).
Differences between moderately and minimally disturbed watersheds were difficult to distinguish and there was considerable overlap in severely impacted watersheds with other groups e.g., (see 95% centroids in Figure 2). This is perhaps due in part to the abundance of cosmopolitan species with long-distance dispersal ability, such as Baetidae mayflies. Some species of Baetidae (e.g., Baetis alpinus, Baetis rhodani) are weak fliers and are thought to be limited to wind dispersal with active flying distances no greater than 3.7 km. Exceptionally strong fliers, such as the caddisfly, Mesophylax aspersus (Trichoptera, Limnephilidae) can fly distances greater than 65 km (e.g., Kelly et al., 2001; Arce et al., 2021). Dispersal abilities for other members of the EPT greater than 8 km are not unusual (summarized in Arce et al., 2021). As PEI is relatively small (< 65 km wide and 224 km long), and a mere 13 km from a mainland source population of flying insects (see: www.confederationbridge.com), adult EPT could potentially disperse from other areas to recolonize highly impacted watersheds (MacArthur and Wilson, 1967; Costanzi and Steifetten, 2019; Russell and Kueffer, 2019). Therefore, the presence of minimally disturbed source habitats may be of great importance toward the stabilization of benthic populations, as well as perhaps for fish reliant on these foodstuffs. To what extent these population-level invasions are occurring and the extent of endemism in aquatic insect taxa on the island merits further study. The latter also suggests that PEI watersheds may not be independent from one another - rather, conservation and restoration activities of these systems will require a more holistic and integrated multiple watershed approach.
Insecticide concentration and discharge covaried, particularly in the Dunk and Wilmot watersheds, when the level of agricultural activity was included in the analysis. Significant differences were found between moderately (35–50% agriculturally impacted: Mill, Montague) and severely impacted watersheds (>50% agriculturally impacted: West, Dunk, and Wilmot; p < 0.001). Sufficient insecticide monitoring data was not available to permit concentration versus discharge comparisons in minimally impacted watersheds such as the Bear, Miminegash, and Morell or the severely impacted watersheds Valleyfield and Clyde. However, in the severely impacted Wilmot watershed, the concentration of chlorantraniliprole exponentially increased with increasing discharge (r = 0.65, p < 0.01), while for clothianidin, the correlation was best described by a log–log linear relationship with concentration increasing 10-fold with corresponding increases in average daily discharge (Figure 4). Patterns were more complex in the Dunk, where responses appeared to potentially be bimodal with stronger positive correlations to discharge when flow was above 1.5 m3/s but less than 2.2 m3/s (r ≥ 0.76, p < 0.01, Figure 4). During the study period, surface water samples processed for insecticide concentration have almost exclusively been collected during late summer low flow periods (e.g., 0.23–1.83 m3/s, 95% CI of all watersheds between May to October). Although baseflow, or groundwater flow, can be as low as 0.4 ± 0.5 m3/s (Median ± Coef. Dispersion), 1-day maximum average daily discharge measurements of 22.7 ± 1.5 m3/s (average ± SE) in the Dunk have been reported e.g., (see Figure 3). Although there are very real logistical and safety constraints in conducting water sampling during high discharge events, more of these types of measurements will be necessary to inform loading estimates and future risk assessments due to climate change.
Global use of all pesticides has increased significantly (approximately 4.1 million tonnes each year) since 1990 (Sharma et al., 2019; Boedeker et al., 2020; Tao et al., 2020; FAO, 2023). This pattern was also found in Prince Edward Island and substantial changes in land use practices occurred over the same period (see Bugden et al., 2014). The 10 focal watersheds in this study varied in the extent of agricultural activity within their catchments and several have a troubling history of fish kills since the 1960s (Supplementary Table S1). Although no relationship between habitat, surface water quality, or insecticide concentrations and Atlantic salmon success or density could be found using the historical monitoring data in this study (Supplementary Table S1), a combination of factors has likely contributed to historical fish kills in PEI waterways including pesticides, increased nitrate concentrations, low dissolved oxygen, and increased water temperature, among others (e.g., CBC, 2022). The historical data examined in this study suggests that these conditions are interrelated for some systems (e.g., Wilmot, Dunk, and Clyde) but may have other components in others, even for apparently similar, severely agriculturally developed systems (> 50% by land area) such as the West River where lower alkalinity and nitrate concentrations coincide with greater total phosphorus (Figure 2B; Supplementary Table S1). Interestingly both the West River and the minimally impacted Morell, have among the highest estimated density of Atlantic salmon and number of redds in a 2018 survey conducted by Guignion et al. (2019) (Supplementary Table S1). Collectively, these findings suggest that watershed-specific approaches may be warranted to protect these systems moving forward.
Extensive restoration efforts have also severely impacted some of the focal watersheds e.g., (see Guignion et al., 2019) and the effects of these activities are evident in the multivariate analysis (e.g., Dunk, West), as are the catastrophic effects of climate change (e.g., severe storm events in Dunk in 2009) and anthropogenic disturbance (e.g., construction and landslides on Clyde 2017–2018). Unfortunately, estimating the total pesticide load in these systems is difficult at present because monitoring on the island is plagued by high historical limits of quantification, infrequent site visits, and sample collections that are routinely taken during low or baseflow periods when these watercourses are likely dominated by groundwater inputs, as opposed to overland flow and runoff mechanisms (Jiang et al., 2007, 2015). Despite public awareness that pesticides are part of the challenge in agroecosystems, very few studies have investigated the magnitude and extent of pesticides entering watercourses in the province (although see: Jackson et al., 1990; Gormley et al., 2005; Yao et al., 2006; Dunn et al., 2011; Taylor et al., 2021). Intensive agricultural activity, selective environmental monitoring, proprietary data controls, ongoing restoration efforts, and a changing climate, are all obscuring root causes in these systems all while benthic macroinvertebrate communities, and the fish that feed on them, are further degraded. Long-term, cumulative impacts on island assemblages of organisms also have an increased likelihood of local extinction events (e.g., MacArthur and Wilson, 1967; Costanzi and Steifetten, 2019) creating the potential for fish kill zones or population ‘sinks’ where water quality may be acceptable, but fish fail to thrive. Finally, to what extent PEI benthic communities depend on the influx of species from neighboring habitats is, at present, unknown. Although, the extent of overlap in community composition in the most severely impacted waterways in this study (> 50% agriculturally developed by land area), suggests that species influx from nearby streams may be commonplace. However, degradation of neighboring ‘source’ systems, beyond certain thresholds, could cause rapid, catastrophic shifts in these systems (see Scheffer et al., 2001).
Groundwater often supplies 100% of streamflow during the late summer sampling period in PEI (Jiang et al., 2007). Thus, groundwater may also be driving the influx of low concentrations of a variety of substances into even minimally impacted streams that are hydraulically connected to groundwater supplies impacted by adjacent, more intensively farmed watersheds. Further, this influx of nutrients and contaminants may continue for extended periods, particularly during low-flow, open water periods. Managing such a system, where historical and contemporary activities in neighboring watersheds may mask the beneficial management practices in sustainably farmed systems, will be difficult. Tools that capture the necessity to increase the scale of these efforts beyond the limited capacity and scope of individual watershed groups will be needed. One health frameworks - an approach that unifies the need to balance and optimize the health of people, animals and the environment is ideally suited to mobilizing the different sectors, disciplines, and communities that will be needed to address the underlying root causes of contamination at a broader ecosystem scale. In the absence of a more holistic approach, the success of efforts toward the re-establishment of Atlantic salmon may be limited. As 100% of the drinking water in PEI is sourced from groundwater (Jiang et al., 2007; PEI, 2020), the human health impacts of pesticides in island waterways may also be significant.
The datasets presented in this study can be found in online repositories. The names of the repository/repositories and accession number(s) can be found in the article/Supplementary material.
The manuscript presents research on animals that do not require ethical approval for their study.
MC: Conceptualization, Data curation, Formal analysis, Validation, Visualization, Writing – original draft. AA: Writing – review & editing, Conceptualization, Formal analysis, Funding acquisition, Investigation, Methodology, Project administration, Resources, Software, Supervision, Visualization.
The author(s) declare that financial support was received for the research, authorship, and/or publication of this article. This work was funded by an NSERC Discovery Launch (RGPIN 2018-03868) and Living Labs funding from Agriculture and Agri-Foods Canada to AA.
The authors are grateful for the contributions of a number of staff and students in the @ThisLabMayfly discussion group, notably Shauna Barry, Emma Bowser, and Brianna Levenstein.
The authors declare that the research was conducted in the absence of any commercial or financial relationships that could be construed as a potential conflict of interest.
All claims expressed in this article are solely those of the authors and do not necessarily represent those of their affiliated organizations, or those of the publisher, the editors and the reviewers. Any product that may be evaluated in this article, or claim that may be made by its manufacturer, is not guaranteed or endorsed by the publisher.
The Supplementary material for this article can be found online at: https://www.frontiersin.org/articles/10.3389/fsufs.2024.1356579/full#supplementary-material
1. ^https://www.princeedwardisland.ca/en/information/environment-energy-and-climate-action/annual-pesticides-sales-data
2. ^ https://www.canada.ca/en/health-canada/services/consumer-product-safety/reports-publications/pesticides-pest-management/corporate-plans-reports/pest-control-products-sales-report.html
3. ^Open Data Prince Edward Island, 2018; see https://data.princeedwardisland.ca/
4. ^see online repositories of this information: https://open.canada.ca/en/open-data; https://atlanticdatastream.ca; https://www.canada.ca/en/environment-climate-change/services/canadian-aquatic-biomonitoring-network/ the Government of Canada, 2022 - https://wateroffice.ec.gc.ca/
5. ^e.g., RPC Fredericton: https://www.rpc.ca/english/quality.html#:~:text=RPC%20is%20an%20ISO%209001,%2C%20value%2C%20and%20efficient%20service
6. ^https://data.princeedwardisland.ca/
7. ^https://atlanticdatastream.ca/
8. ^https://wateroffice.ec.gc.ca/search/historical_e.html
9. ^accessible at: https://cabin-rcba.ec.gc.ca/cabin/login?culture=en-CA
10. ^accessible at: http://www.gov.pe.ca/gis/
11. ^v. 3.10; https://qgis.org/en/site/
Alberto, A., St-Hilaire, A., Courtenay, S. C., and Van Den Heuvel, M. R. (2016). Monitoring stream sediment loads in response to agriculture in Prince Edward Island, Canada. Environ. Monit. Assess. 188:415. doi: 10.1007/s10661-016-5411-3
Alexander, A. C., Culp, J. M., Liber, K., and Cessna, A. J. (2007). Effects of insecticide exposure on feeding inhibition in mayflies and oligochaetes. Environ. Toxicol. Chem. 26, 1726–1732. doi: 10.1897/07-015R.1
Alexander, A. C., Luis, A. T., Culp, J. M., Baird, D. J., and Cessna, A. J. (2013). Can nutrients mask community responses to insecticide mixtures? Ecotoxicology 22, 1085–1100. doi: 10.1007/s10646-013-1096-3
Arce, P. A., Hörren, T., Schletterer, M., and Kail, J. (2021). How far can EPTs fly? A comparison of empirical flying distances of riverine invertebrates and existing dispersal metrics. Ecol. Indic. 125:107465. doi: 10.1016/j.ecolind.2021.107465
Benson, V. S., VanLeeuwen, J. A., Sanchez, J., Dohoo, I. R., and Somers, G. H. (2006). Spatial analysis of land use impact on ground water nitrate concentrations. J. Environ. Qual. 35, 421–432. doi: 10.2134/jeq2005.0115
Bernhardt, E., and Palmer, M. A. (2011). River restoration: the fuzzy logic of repairing reaches to reverse catchment scale degradation. Ecol. Appl. 21, 1926–1931. doi: 10.1890/10-1574.1
Bhatti, A. Z., Farooque, A. A., Krouglicof, N., Peters, W., Acharya, B., Li, Q., et al. (2021). Spatiotemporal hydrological analysis of streamflows and groundwater recharge for sustainable water management in Prince Edward Island, Canada. World Water Policy 7, 253–282. doi: 10.1002/wwp2.12065
Bijay-Singh, B., and Craswell, E. (2021). Fertilizers and nitrate pollution of surface and ground water: an increasingly pervasive global problem. SN Appl. Sci. 3:518. doi: 10.1007/s42452-021-04521-8
Boedeker, W., Watts, M., Clausing, P., and Marquez, E. (2020). The global distribution of acute unintentional pesticide poisoning: estimations based on a systematic review. BMC Public Health 20:1875. doi: 10.1186/s12889-020-09939-0
Brock, T., Van Wijngaarden, R., and Van Geest, G. (2000). Ecological risks of pesticides in freshwater ecosystems. Part 2: Insecticides. Alterra-Rapport 089. Wageningen, Netherlands. Available at: https://research.wur.nl/en/publications/ecological-risks-of-pesticides-in-freshwater-ecosystems-part-2-in
Bugden, G., Jiang, Y., Van den Heuvel, M. R., Vandermeullen, H., MacQuarrie, K. T. B., Crane, C. J., et al. (2014). Nitrogen loading criteria for estuaries in Prince Edward Island. Canadian technical report of fisheries and aquatic science 3066. Fisheries and Oceans Canada, pp. 1–52. Available at: https://www.princeedwardisland.ca/sites/default/files/publications/nitrogen_loading_criteria_for_estuaries_in_prince_edward_island.pdf (Accessed July 24, 2023).
Cavallaro, M. C., Morrissey, C. A., Headley, J. V., Peru, K. M., and Liber, K. (2017). Comparative chronic toxicity of imidacloprid, clothianidin, and thiamethoxam to Chironomus dilutus and estimation of toxic equivalency factors. Environ. Toxicol. Chem. 36, 372–382. doi: 10.1002/etc.3536
CBC (2022). Combination of factors likely led to fish kill in Morell River, says P.E.I. Fisheries biologist August 15, 2022. Available at: https://www.cbc.ca/news/canada/prince-edward-island/pei-fish-kill-morell-river-cause-1.6551876.
CCME (2004). CCME (Canadian Council for Ministers of the environment). Phosphorus: Canadian guidance framework for the management of freshwater systems. Ottawa, ON. Available at: https://ccme.ca/en/res/phosphorus-en-canadian-water-quality-guidelines-for-the-protection-of-aquatic-life.pdf.
CCME (2007a). Canadian water quality guidelines for the protection of aquatic life: nutrients. In: Canadian environmental quality guidelines. Available at: https://ccme.ca/en/res/nutrients-en-canadian-water-quality-guidelines-for-the-protection-of-aquatic-life.pdf.
CCME (2007b). Canadian water quality guidelines: Imidacloprid. Scientific supporting document. Canadian Council of Ministers of the environment. Available at: https://ccme.ca/en/res/2007-imidacloprid-cwqg-ssd-1388-en.pdf.
CCME (2012). Canadian water quality guidelines for the protection of aquatic life: Nitrate ion. Ottawa, ON. Available at: https://ccme.ca/en/res/nitrate-ion-en-canadian-water-quality-guidelines-for-the-protection-of-aquatic-life.pdf.
Costanzi, J., and Steifetten, Ø. (2019). Island biogeography theory explains the genetic diversity of a fragmented rock ptarmigan (Lagopus muta) population. Ecol. Evol. 9, 3837–3849. doi: 10.1002/ece3.5007
Damalas, C. A., and Eleftherohorinos, I. G. (2011). Pesticide exposure, safety issues, and risk assessment indicators. Int. J. Environ. Res. Pu. 8, 1402–1419. doi: 10.3390/ijerph8051402
Danielescu, S., and MacQuarrie, K. T. B. (2011). Nitrogen loadings to two small estuaries, Prince Edward Island, Canada: a 2-year investigation of precipitation, surface water and groundwater contributions. Hydrol. Process. 25, 945–957. doi: 10.1002/hyp.7881
Danielescu, S., MacQuarrie, K. T. B., Nyiraneza, J., Zebarth, B., Sharifi-Mood, N., Grimmett, M., et al. (2024). Development and validation of a crop and nitrate leaching model for potato cropping Systems in a Temperate–Humid Region. Water 16:475. doi: 10.3390/w16030475
Davidson, C., and Knapp, R. A. (2007). Multiple stressors and amphibian declines: dual impacts of pesticides and fish on yellow-legged frogs. Ecol. Appl. 17, 587–597. doi: 10.1890/06-0181
Dibblee, R. (1990). PEI watershed boundaries. Available at: www.gov.pe.ca/gis.
Dunn, A. M., Julien, G., Ernst, W. R., Cook, A., Doe, K. G., and Jackman, P. M. (2011). Evaluation of buffer zone effectiveness in mitigating the risks associated with agricultural runoff in Prince Edward Island. Sci. Total Environ. 409, 868–882. doi: 10.1016/j.scitotenv.2010.11.011
Eaton, A. D., Clesceri, L. S., Rice, E. W., and Greenberg, A. E. (2005). Standard methods for the examination of water and wastewater. 21st Edn. Baltimore: Port City Press.
ECCC . Canadian aquatic biomonitoring laboratory methods: Processing taxonomy, and quality control of benthic macroinvertebrates samples. Ottawa, ON, (2020). Available at: http://publications.gc.ca/site/eng/476513/publication.html.
ECCC (2023). Canadian environmental sustainability indicators: Water quality in Canadian rivers. Available at: https://www.canada.ca/content/dam/eccc/documents/pdf/cesindicators/water-quality/2023/water-quality-canadian-rivers-en.pdf.
Environment Canada (2011). Canadian aquatic biomonitoring network field manual - wadeable streams. Ottawa, ON. Available at: http://publications.gc.ca/site/eng/422979/publication.html.
FAO . More people, more food, worse water? A global review of water pollution from agriculture. Rome, Italy, (2018). Available at: https://www.fao.org/documents/card/en?details=CA0146EN
FAO . Pesticides used. In: The state of food security and nutrition in the world 2023. Rome, Italy, (2023). Available at: https://www.fao.org/documents/card/en?details=cc3017en
Gage, M. S., Spivak, A., and Paradise, C. J. (2004). Effects of land use and disturbance on benthic insects in headwater streams draining small watersheds north of Charlotte. N. C. Southeast. Nat. 3, 345–358. doi: 10.1656/1528-7092(2004)003[0345:EOLUAD]2.0.CO;2
Gagnon, P., Sheedy, C., Farenhorst, A., Cessna, A. J., Newlands, N. K., and McQueen, R. D. A. (2016). “Pesticides” in environmental sustainability of Canadian agriculture: Agri-environmental indicator report series report #4. eds. R. L. Clearwater, T. Martin, and T. Hoppe (Ottawa, ON: Agriculture and Agri-Food Canada), 153–165.
Gilliom, R. J. (2007). Pesticides in U.S. streams and groundwater. Environ. Sci. Technol. 41, 3408–3414. doi: 10.1021/es072531u
Gormley, K. L., Teather, K. L., and Guignion, D. L. (2005). Changes in salmonid communities associated with pesticide runoff events. Ecotoxicology 14, 671–678. doi: 10.1007/s10646-005-0017-5
Goulson, D. (2013). REVIEW: an overview of the environmental risks posed by neonicotinoid insecticides. J. Appl. Ecol. 50, 977–987. doi: 10.1111/1365-2664.12111
Guignion, D., Gaudet, C., and MacFarlane, R. (2019) A renewed conservation strategy for Atlantic salmon in Prince Edward Island. Oak meadows Inc., Central Queens wildlife federation, and PEI Department of communities, land and environment. Charlottetown, PE, 2019. Available at: https://www.salmonconservation.ca/wp-content/uploads/2019/04/Atlantic-Salmon-Strategy-April-2019.pdf
Health Canada (2016). Pest management regulatory agency. Proposed re-evaluation decision PRVD2016-20 Imidacloprid 23 November 2016. Ottawa, ON. Available at: https://health.canada.ca/en/health-canada/corporate/request-publication-form.html?title=PMRA%20(PRVD2016-20)%20Proposed%20Re-evaluation%20Decision,%20Imidacloprid
Health Canada (2021a). Pest management regulatory agency. Special review decision SRD2021-03 Clothianidin. Ottawa, ON. https://honeycouncil.ca/wp-content/uploads/2021/04/SRD2021-03-eng.pdf
Health Canada (2021b). Pest management regulatory agency. Special review decision SRD2021-04 Thiamethoxam. Ottawa, ON. Available at: https://publications.gc.ca/collections/collection_2021/sc-hc/h113-17/H113-17-2021-4-eng.pdf.
Health Canada (2021c). Pest management regulatory agency. Special review decision: Clothianidin risk to aquatic invertebrates SRD2021-03 (extended). Ottawa, ON. Available at: https://health.canada.ca/en/health-canada/corporate/request-publication-form.html?title=PMRA%20(SRD2021-03)%20Special%20Review%20Decision:%20Clothianidin%20Risk%20to%20Aquatic%20Invertebrates.
Health Canada (2024). Pest management regulatory agency. Pesticide use information framework development: Towards a strategic approach “what we heard” report from government partners and stakeholders (2021–2023). Ottawa, ON. Available at: https://www.canada.ca/content/dam/hc-sc/documents/services/consumer-product-safety/reports-publications/pesticides-pest-management/corporate-plans-reports/pesticide-use-information-framework-development-towards-strategic-approach-what-we-heard-report-from-government-partners-stakeholders-2021-2023/pesticide-use-information-framework-development-towards-strategic-approach-what-we-heard-report-from-government-partners-stakeholders-2021-2023-en.pdf.
Hulot, F., Lacroix, G., Lescher-Moutoué, F., and Loreau, M. (2000). Functional diversity governs ecosystem response to nutrient enrichment. Nature 405, 340–344. doi: 10.1038/35012591
IPBES (2018). “Secretariat of the intergovernmental science-policy platform on biodiversity and ecosystem services” in The IPBES assessment report on land degradation and restoration. eds. L. Montanarella, R. Scholes, and A. Brainich (Bonn, Germany: IPBES).
Jackson, R. E., Mutch, J. P., and Priddle, M. W. (1990). Persistence of aldicarb residues in the sandstone aquifer of Prince Edward Island, Canada. J. Contam. Hydrol. 6, 21–35. doi: 10.1016/0169-7722(90)90009-6
Jähnig, S., Brabec, K., Buffagni, A., Erba, S., Lorenz, A. W., Ofenböck, T., et al. (2010). A comparative analysis of restoration measures and their effects on hydromorphology and benthic invertebrates in 26 central and southern European rivers. J. Appl. Ecol. 47, 671–680. doi: 10.1111/j.1365-2664.2010.01807.x
Jiang, Y., Jamieson, T., Nyiraneza, J., Somers, G., Thompson, B., Murray, B., et al. (2015). Effects of fall vs. spring plowing forages on nitrate leaching losses to groundwater. Groundwater Monit. Remediat. 35, 43–54. doi: 10.1111/gwmr.12083
Jiang, Y., Somers, G., and Paradis, D. (2007). Estimation of groundwater residence times in the Wilmot River watershed on Prince Edward Island - implications for nutrient reduction. In: 60th Canadian Geotechnical Conference and 8th Joint CGS-IAH-CNC Conference. Ottawa, ON, 21–24 October 2007. Available at: http://www.gov.pe.ca/photos/original/cle_WA33.pdf.
Kelly, L. C., Bilton, D. S., and Rundle, S. D. (2001). Population structure and dispersal in the Canary Island caddisfly Mesophylax aspersus (Trichoptera, Limnephilidae). Heredity 86, 370–377. doi: 10.1046/j.1365-2540.2001.00839.x
Kondolf, G. M. (2000). Assessing salmonid spawning gravel quality. Trans. Am. Fish. Soc. 129, 262–281. doi: 10.1577/1548-8659(2000)129<0262:ASSGQ>2.0.CO;2
Lawniczak, A. E., Zbierska, J., Nowak, B., Achtenberg, K., Grześkowiak, A., and Kanas, K. (2016). Impact of agriculture and land use on nitrate contamination in groundwater and running waters in central-West Poland. Environ. Monit. Assess. 188:172. doi: 10.1007/s10661-016-5167-9
Liang, K., Jiang, Y., Qi, J., Fuller, K., Nyiraneza, J., and Meng, F. R. (2020). Characterizing the impacts of land use on nitrate load and water yield in an agricultural watershed in Atlantic Canada. Sci. Total Environ. 729:138793. doi: 10.1016/j.scitotenv.2020.138793
Liess, M., and von der Ohe, P. C. (2005). Analyzing effects of pesticides on invertebrate communities in streams. Environ. Toxicol. Chem. 24, 954–965. doi: 10.1897/03-652.1
Liu, F. S., Lockett, B. R., Sorichetti, R. J., Watmough, S. A., and Eimers, M. C. (2022). Agricultural intensification leads to higher nitrate levels in Lake Ontario tributaries. Sci. Total Environ. 830:154534. doi: 10.1016/j.scitotenv.2022.154534
Liu, N., Pan, X., Yang, Q., Ji, M., and Zhang, Z. (2018). The dissipation of thiamethoxam and its main metabolite clothianidin during strawberry growth and jam-making process. Sci. Rep. 8:15242. doi: 10.1038/s41598-018-33334-w
Louhi, P., Mykrä, H., Paavola, R., Huusko, A., Vehanen, T., Mäki-Petäys, A., et al. (2011). Twenty years of stream restoration in Finland: little response by benthic macroinvertebrate communities. Ecol. Appl. 21, 1950–1961. doi: 10.1890/10-0591.1
MacArthur, R. H., and Wilson, E. O. (1967). The theory of island biogeography. Princeton, NJ: Princeton University Press.
Main, A. R., Headley, J. V., Peru, K. M., Michel, N. L., Cessna, A. J., and Morrissey, C. A. (2014). Widespread use and frequent detection of neonicotinoid insecticides in wetlands of Canada’s prairie pothole region. PLoS One 9:e92821. doi: 10.1371/journal.pone.0092821
Merritt, R. W., and Cummins, K. W. (1996). An introduction to the aquatic insects of North America. 3rd Edn. Dubuque: Kendall Hunt.
Miles, J. C., Hua, J., Sepulveda, M. S., Krupke, C. H., and Hoverman, J. T. (2017). Effects ofclothianidin on aquatic communities: evaluating the impacts of lethal and sublethal exposure to neonicotinoids. PLoS One 12:e0174171. doi: 10.1371/journal.pone.0174171
Mockus, V., Werner, J., Woodward, D.E., Nielsen, R., Dobos, R., Hjelmfelt, A., et al. (1972). Hydrologic soil groups. In: Part 630 hydrology National Engineering Handbook. Washington, DC. Available at: https://directives.sc.egov.usda.gov/OpenNonWebContent.aspx?content=17757.wba.
Morrissey, C. A., Mineau, P., Devries, J. H., Sanchez-Bayo, F., Liess, M., Cavallaro, M. C., et al. (2015). Neonicotinoid contamination of global surface waters and associated risk to aquatic invertebrates: a review. Environ. Int. 74, 291–303. doi: 10.1016/j.envint.2014.10.024
Muotka, T., Paavola, R., Haapala, A., Novikmec, M., and Laasonen, P. (2002). Long-term recovery of stream habitat structure and benthic invertebrate communities from in-stream restoration. Biol. Conserv. 105, 243–253. doi: 10.1016/S0006-3207(01)00202-6
Newcombe, C. P. (2003). Impact assessment model for clear water fishes exposed to excessively cloudy water. J. Am. Water Resour. As. 39, 529–544. doi: 10.1111/j.1752-1688.2003.tb03674.x
Nyiraneza, J., Thompson, B., Geng, X., He, J., Jiang, Y., Fillmore, S., et al. (2017). Changes in soil organic matter over 18 years in Prince Edward Island, Canada. Can. J. Soil Science 97, 745–756. doi: 10.1139/cjss-2017-0033
Oksanen, J. (2022). Vegan: an introduction to ordination. Available at: https://cran.r-project.org/web/packages/vegan/vignettes/intro-vegan.pdf
Olaya-Arenas, P., Hauri, K., Scharf, M. E., and Kaplan, I. (2020). Larval pesticide exposure impacts monarch butterfly performance. Sci. Rep. 10:14490. doi: 10.1038/s41598-020-71211-7
Palmer, M. A., Menninger, H., and Bernhardt, E. (2010). River restoration, habitat heterogeneity and biodiversity: a failure of theory or practice? Freshw. Biol. 55, 205–222. doi: 10.1111/j.1365-2427.2009.02372.x
PEI (2016). Department of Transportation and Infrastructure. Trans Canada Highway Extension. Charlottetown, PE. Available at: https://www.princeedwardisland.ca/en/information/trans-canada-highway-extension.
PEI (2020). Strategic policy and evaluation division, Department of Agriculture and Land. The Prince Edward Island potato sector: An economic impact analysis. Charlottetown, PE. Available at: https://www.princeedwardisland.ca/sites/default/files/publications/af_potato_econ_impact_study.pdf.
Relyea, R. A. (2009). A cocktail of contaminants: how mixtures of pesticides at low concentrations affect aquatic communities. Oecologia 159, 363–376. doi: 10.1007/s00442-008-1213-9
Richter, B. D., Baumgartner, J. V., Powell, J., and Brawn, D. P. (1996). A method for assessing hydrologic alteration within ecosystems. Conserv. Biol. 10, 1163–1174. doi: 10.1046/j.1523-1739.1996.10041163.x
Roessink, I., Merga, L. B., Zweers, H. J., and van den Brink, P. J. (2013). The neonicotinoid imidacloprid shows high chronic toxicity to mayfly nymphs. Environ. Toxicol. Chem. 32, 1096–1100. doi: 10.1002/etc.2201
Rossi, A. S., Fantón, N., Michlig, M. P., Repetti, M. R., and Cazenave, J. (2020). Fish inhabiting rice fields: bioaccumulation, oxidative stress and neurotoxic effects after pesticides application. Ecol. Indic. 113:106186. doi: 10.1016/j.ecolind.2020.106186
Russell, J. C., and Kueffer, C. (2019). Island biodiversity in the anthropocene. Annu. Rev. Environ. Resour. 44, 31–60. doi: 10.1146/annurev-environ-101718-033245
Sanford, M., Washuck, N., Carr, K., and Prosser, R. S. (2021). Pulsed exposure of the macrophyte Lemna minor to herbicides and the mayfly Neocloeon triangulifer to diamide insecticides. Chemosphere 273:128582. doi: 10.1016/j.chemosphere.2020.128582
Schäfer, R. B. (2019). Responses of freshwater macroinvertebrates to pesticides: insights from field studies. Curr. Opin. Environ. Sci. Health 11, 1–7. doi: 10.1016/j.coesh.2019.06.001
Scheffer, M., Carpenter, S., Foley, J., Folke, C., and Walker, B. (2001). Catastrophic shifts in ecosystems. Nature 413, 591–596. doi: 10.1038/35098000
Schmidt-Jeffris, R. A., and Nault, B. A. (2016). Anthranilic Diamide insecticides delivered via multiple approaches to control vegetable pests: a case study in snap bean. J. Econ. Entomol. 109, 2479–2488. doi: 10.1093/jee/tow219
Sharma, A., Kumar, V., Shahzad, B., Tanveer, M., Sidhu, G. P. S., Handa, N., et al. (2019). Worldwide pesticide usage and its impacts on ecosystem. SN Appl. Sci. 1:1446. doi: 10.1007/s42452-019-1485-1
Sheedy, C., Kromrey, N., Nilsson, D., and Armitage, T. (2019). From peaks to prairies: a time-of-travel synoptic survey of pesticides in watersheds of southern Alberta, Canada. Inland Waters 9, 438–452. doi: 10.1080/20442041.2019.1634947
Shukla, S., and Saxena, A. (2018). “Global status of nitrate contamination in groundwater: its occurrence, health impacts, and mitigation measures” in Handbook of environmental materials management. ed. C. M. Hussain (New York: Springer), 869–888.
Somers, G., Raymond, B., and Uhlman, W. (1999). P.E.I. Water quality interpretive report prepared for the Canada-Prince Edward Island water annex to the federal/provincial framework agreement for environmental cooperation in Atlantic Canada. Charlottetown, PE. Available at: http://www.gov.pe.ca/photos/original/waterquality_99.pdf.
Sumon, K. A., Ritika, A. K., Peeters, E. T. H. M., Rashid, H., Bosma, R. H., Rahman, M. S., et al. (2018). Effects of imidacloprid on the ecology of sub-tropical freshwater microcosms. Environ. Pollut. 236, 432–441. doi: 10.1016/j.envpol.2018.01.102
Tao, M., Adler, P. R., Larsen, A. E., and Suh, S. (2020). Pesticide application rates and their toxicological impacts: why do they vary so widely across the U.S.? Environ. Res. Lett. 15:124049. doi: 10.1088/1748-9326/abc650
Taylor, L. J., Clark, K. F., Daoud, D., van den Heuvel, M. R., and Greenwood, S. J. (2021). Exposure of American lobster (Homarus americanus) to the pesticide chlorpyrifos results in changes in gene expression. Comp. Biochem. Phys. D. 40:100918. doi: 10.1016/j.cbd.2021.100918
Tilman, D., Fargione, J., Wolff, B., D’Antonio, C., Dobson, A., Haworth, R., et al. (2001). Forecasting agriculturally driven global environmental change. Science 292, 281–284. doi: 10.1126/science.1057544
Tudi, M., Daniel Ruan, H., Wang, L., Lyu, J., Sadler, R., Connell, D., et al. (2021). Agriculture development, pesticide application and its impact on the environment. Int. J. Environ. Res. Pub. He. 18:1112. doi: 10.3390/ijerph18031112
US EPA (2015). Insecticides. Caddis Volume 2. Washington, DC. Available at: https://www.epa.gov/caddis-vol2/insecticides.
US EPA (2020). Proposed interim registration review decision for neonicotinoids. Washington, DC. Available at: https://www.epa.gov/pollinator-protection/proposed-interim-registration-review-decision-neonicotinoids.
US EPA (2023). Imidacloprid, thiamethoxam and clothianidin: Draft predictions of likelihood of jeopardy and adverse modification for federally listed endangered and threatened species and designated critical habitats. Washington, DC. Available at: https://www.epa.gov/system/files/documents/2023-05/ESA-JAM-Analysis.pdf.
Ward, D. M., Nislow, K. H., and Folt, C. L. (2009). Increased population density and suppressed prey biomass: relative impacts on juvenile Atlantic salmon growth. Trans. Am. Fish. Soc. 138, 135–143. doi: 10.1577/T08-128.1
Xing, Z., Chow, L., Cook, A., Benoy, G., Rees, H., Ernst, B., et al. (2012). Pesticide application and detection in variable agricultural intensity watersheds and their river systems in the maritime region of Canada. Arch. Environ. Con. Tox. 63, 471–483. doi: 10.1007/s00244-012-9789-9
Xu, T., Dyer, D. G., McConnell, L. L., Bondarenko, S., Allen, R., and Heinemann, O. (2016). Clothianidin in agricultural soils and uptake into corn pollen and canola nectar after multiyear seed treatment applications. Environ. Toxicol. Chem. 35, 311–321. doi: 10.1002/etc.3281
Yadav, I. C., and Watanabe, H. (2018). Soil erosion and transport of imidacloprid and clothianidin in the upland field under simulated rainfall condition. Sci. Total Environ. 640-641, 1354–1364. doi: 10.1016/j.scitotenv.2018.06.008
Yao, Y., Tuduri, L., Harner, T., Blanchard, P., Waite, D., Poissant, L., et al. (2006). Spatial and temporal distribution of pesticide air concentrations in Canadian agricultural regions. Atmos. Environ. 40, 4339–4351. doi: 10.1016/j.atmosenv.2006.03.039
Keywords: river, pesticide and insecticide, one health, monitoring, climate, spatial and temporal distribution
Citation: Crawford M and Alexander AC (2024) Fish kills and insecticides: historical water quality patterns in 10 agricultural watersheds in Prince Edward Island, Canada (2002–2022). Front. Sustain. Food Syst. 8:1356579. doi: 10.3389/fsufs.2024.1356579
Received: 15 December 2023; Accepted: 11 July 2024;
Published: 26 July 2024.
Edited by:
David Lapen, Agriculture and Agri-Food Canada, CanadaReviewed by:
Justin Beneteau Renaud, Agriculture and Agri-Food Canada, CanadaCopyright © 2024 Crawford and Alexander. This is an open-access article distributed under the terms of the Creative Commons Attribution License (CC BY). The use, distribution or reproduction in other forums is permitted, provided the original author(s) and the copyright owner(s) are credited and that the original publication in this journal is cited, in accordance with accepted academic practice. No use, distribution or reproduction is permitted which does not comply with these terms.
*Correspondence: Alexa C. Alexander, YWxleGEuYWxleGFuZGVyQHVuYi5jYQ==
Disclaimer: All claims expressed in this article are solely those of the authors and do not necessarily represent those of their affiliated organizations, or those of the publisher, the editors and the reviewers. Any product that may be evaluated in this article or claim that may be made by its manufacturer is not guaranteed or endorsed by the publisher.
Research integrity at Frontiers
Learn more about the work of our research integrity team to safeguard the quality of each article we publish.