- 1School of Water, Energy, and Environment, Cranfield University, Bedford, United Kingdom
- 2Department of Geography and Environmental Science, University of Reading, Reading, United Kingdom
- 3Bioprocess Department, Future Biogas Ltd., Guildford, United Kingdom
Anaerobic digestate is a nutrient rich slurry by-product derived from biogas production, often used as a fertiliser due to its high nitrogen content. However, nitrogen losses from its application can lead to environmental pollution. In a laboratory experiment, the addition of high organic carbon materials to digestate-amended soil as a potential means to stimulate microbial immobilisation of digestate supplied nitrogen was investigated. Soil was incubated in pots for 5 months with digestate (equivalent to 250 kgN ha−1). The impact of adding carbon into the digestate (equivalent to 540 kgC ha−1) as either glycerol, straw, woodchip, or biochar on soil microbial and chemical parameters was quantified. Glycerol amended soils had significantly higher microbial biomass compared to digestate alone during the first month and at 30 days after application had a 4x higher on average microbial N. The digestate + straw treatment resulted in a 2.5x significantly greater nitrogen immobilisation compared to digestate alone after 3 months of incubation. The digestate + woodchip had a 2× higher mean microbial N after 5 months, whilst the biochar amendment did not stimulate significant nitrogen immobilisation at any time. These results suggest that mixing a labile to moderately labile organic carbon amendment, such as straw, with digestate has the greatest potential to reduce nitrogen losses following digestate application through microbial immobilisation.
1 Introduction
Anaerobic digestate is a nutrient-dense slurry generated from biogas production. The process to create biogas requires the breakdown of a biodegradable feedstock such as animal waste, purpose grown energy crops, sewage waste, and the organic fraction of household, municipal and industrial wastes, in the absence of oxygen. During anaerobic digestion between 20% and 95% of the carbon in the feedstock is converted into methane and carbon dioxide (Möller, 2015) and collected as biogas. As the organic matter is digestated, between 15% and 82% of the organic nitrogen bound within is mineralised into ammonium (Bareha et al., 2018). As this form of nitrogen is readily available for plants to utilise, digestate can be applied to land as a fertiliser. However, due to its high liquid content it is expensive for the biogas industry to store and transport the digestate (Al-Seadi and Lukehurst, 2012). A solution to reduce costs is to separate the digestate into its fibrous (solid) and liquid fractions, which reduces the volume of the liquid by 20%–30% (Lyons et al., 2021).
The liquid fraction of the separated digestate has been extensively studied for its use as a fertiliser and been found to produce yields comparable to synthetic nitrogen (Šimon et al., 2015; Riva et al., 2016; Walsh et al., 2018; Barzee et al., 2019). However, sustainable use of digestate as a fertiliser on agricultural land is dependent on good management practises. On average, over 80% of the nitrogen content of the liquid fraction is in the form of ammonium and nitrate (Czekała, 2022), which are the N forms most susceptible to loss processes by volatilisation, denitrification, nitrification and leaching. Therefore, land spreading digestate can result in groundwater and atmospheric pollution (Nkoa, 2014). These nitrogen losses from the soil reduce the nitrogen use efficiency of digestate fertilisers. Therefore, methods need to be developed to keep nitrogen supplied by the liquid digestate in the soil and available for crop uptake, whilst minimising losses and detrimental impacts on the environment.
Ammonia volatilisation losses represent a key nitrogen loss pathway when applying anaerobic digestate with 35%–60% of total nitrogen applied lost (Tiwary et al., 2015). However, these losses are reduced by 40%–50% using precision slurry spreading techniques such as band spreading or injecting digestate directly into the soil compared to the traditional practise of broadcast spreading (Nicholson et al., 2018). Similarly adding sulphuric acid into digestate to lower its pH can effectively reduce ammonia volatilisation with reductions ranging from 45% (Wagner et al., 2021) to 95% (Sánchez-Rodríguez et al., 2018) when compared to non-acidified digestate. Once in the soil, the ammonium nitrogen from digestate is converted by nitrifying and denitrifying microorganisms, resulting in nitrogen losses before plant uptake as nitrate leaching and N2O emissions. Research is ongoing on the applicability of using nitrification inhibitors, which are chemical compounds added to a fertiliser to delay the conversion of ammonium into nitrate and therefore reduce these losses. Huf and Olfs (2020) and Giacometti et al. (2020) observed that the addition of nitrification inhibitors to digestate reduced N2O and leaching losses, respectively, within a month of application. Hegewald et al. (2021) observed lower annual N2O emissions by 36% over a three-year period. However, the cost of using nitrification inhibitors can be significant because factors such as climate, soil type and crop influence their effectiveness (Macleod et al., 2015; Drame et al., 2023). Furthermore, these nitrification inhibitors are agrochemicals which cannot be used in organic farming systems. As such, it is necessary to investigate alternative methods of reducing nitrogen losses from the soil.
Soil microorganisms can immobilise nitrogen into their biomass, as they use nitrogen to build proteins, nucleic acids and other cellular components. This nitrogen forms part of the necromass when the microbes die, which is subsequently remineralised when the necromass is primed by plant root exudates (Meier et al., 2017). This provides plants with a source of nitrogen when they need it as opposed to farmers applying synthetic nitrogen, which can result in a mismatch between time of input and plant demand. Most soil microorganisms are heterotrophic and require an external source of organic carbon as a precursor to synthesising their own molecules. Digestate has a low total carbon content, typically between 0.43% and 3.4% (Risberg et al., 2017). The nutrient flush following digestate application causes temporary increases in microbial activity and abundance that subside within 24 h of application (Alburquerque et al., 2012; Iocoli et al., 2019) and disappear within a few weeks (Walsh et al., 2012; Johansen et al., 2013; Mortola et al., 2019; Gebremikael et al., 2020; Ren et al., 2020; Różyło and Bohacz, 2020). When digestate is separated into its solid and liquid fractions and applied separately to land, the organic carbon richer solid fraction increases microbial growth and immobilised nitrogen into microbial biomass, which is not seen when liquid digestate is applied (de la Fuente et al., 2013). This observation indicates that to stimulate microbial immobilisation of nitrogen in liquid digestate, more carbon is needed to satisfy microbial stoichiometry.
Organic materials high in carbon but low in nitrogen are known to immobilise nitrogen into soil microorganisms; as the microbes utilise the bioavailable carbon, they simultaneously use the material supplied nitrogen to meet their own, lower, carbon to nitrogen (C:N ratio) requirements (Robertson and Groffman, 2007). However, C:N ratio alone does not determine the rate of microbial growth, as the carbon in material can be of varying accessibility to microbes. For example, carbon that forms a simple monomer can be readily taken up through diffusion or active transport into microbial cells (da Silva et al., 2009; Dobson et al., 2012). More complex carbon structures include plant polymers such as hemicellulose, cellulose and lignin. To break down these materials into assimilable molecules, microbes require a variety of externally excreted enzymes, with residues containing a high lignin content predominately decomposed by enzymes secreted by fungi and bacterial cells that form multicellular assemblages such as Actinomycetes (Mekonnen, 2021).
This research aimed to investigate the effects of adding different sources of organic carbon to liquid digestate on soil microbial biomass, microbial nitrogen immobilisation and microbial community dynamics. The objective was to determine which type of organic material, in terms of carbon accessibility, was most effective at inducing nitrogen immobilisation. It was hypothesised that adding organic carbon additives to liquid digestate would stimulate microbial immobilisation of nitrogen, and that the magnitude and the timing of the effect would be influenced by the accessibility of the carbon substrates to soil microorganisms. As the additives vary in structural complexity, they are utilised by different microbial groups as a carbon source for growth and energy, it was predicted that the microbial community would shift to one with a higher fungi-to-bacteria ratio with the addition of complex carbon materials into the digestate. A pot experiment was established to test these hypotheses in the absence of confounding environmental variables, such as temperature.
2 Materials and methods
2.1 Soil, digestate and high organic carbon materials
A sandy loam topsoil (69% sand, 20% silt, 11% clay) bought from Bourne Amenity Ltd., was used for the study. Prior to the experiment the soil was passed through a 2 mm sieve to remove any stones and large debris and thoroughly mixed to homogenise. Liquid anaerobic digestate was supplied by Future Biogas Ltd. from a biogas plant, managed to BSI PAS110 standards, using a mixed feedstock of 85 tonne maize silage, 7.5 tonne cow manure, and 18 tonne chicken manure. The plant is mesophilic, operating at 43°C with a retention time of 98 days. The liquid fraction was mechanically obtained from the whole digestate after screw press separation. The biochar applied in the experiment (CreChar™, supplied by Carbogenics Ltd) was produced from office waste in a kiln run at 700–800°C for 60 min. Wheat straw was obtained from a farmer (Bedfordshire United Kingdom) and woodchips from the Milton Keynes council parks department. Straw and woodchips were air-dried and chopped into smaller pieces (1–2 cm) to fit into the pots. Glycerol was bought from Sigma Aldrich. Details of material properties are in Table 1.
2.2 Soil incubations
The incubation experiment was carried out in the dark at 20°C 4°C for 5 months. 250 g (dry weight basis) soil was added to 330 mL capacity PVC containers (top diameter 8 cm, bottom diameter 5 cm, height 12 cm) and pots were gently tapped on the worktop to ensure soil settled to a bulk density of 1 g cm3. Before the start of the experiment the soils were adjusted to 40% water holding capacity and pre-incubated at 20°C 4°C in the dark under aerobic conditions for a week, to allow soil microbial activity to recover after being sieved. Water holding capacity was determined using a saturate and drain method modified from Harding and Ross (1964). 50 g of soil was added to a stoppered funnel and saturated with 100 mL of deionised water for 30 min. The stopper was then removed, and the water drained for 30 min. The volume of water retained in the soil was combined with the known moisture content of the soil to calculate the water holding capacity.
The experiment consisted of six treatments, arranged in a randomised block design with four replications: (1) liquid digestate control (LD); (2) liquid digestate with glycerol (LD-G); (3) liquid digestate with straw (LD-S); (4) liquid digestate with woodchip (LD-W); (5) liquid digestate with biochar (LD-B); and (6) unfertilised control (CONT). Sufficient sets were set-up to allow for destructive sampling for soil biochemical analysis on four occasions: 3 h after application, then 30, 90 and 150 days after application. This gave a total of 96 experimental units (6 treatments × 4 replicates × 4 sampling times).
Digestate was applied at 23 mL per pot, a rate equivalent to 250 kg-N ha−1, which supplied 0.59 mg-N g−1 dry soil. This rate of nitrogen was selected as it is the maximum amount farmers are allowed to apply in a 12 month period from organic sources in areas designated as Nitrate Vulnerable Zones under UK law (DEFRA and EA, 2018). Prior to application, the digestate was mixed with additives at a rate of 12 kg-C m3 of digestate (equivalent to 540 kg-C ha−1), resulting in a material with a C:N ratio of approximately 5:1. This equalled an addition to the pots of 0.7 mL glycerol and 0.68, 0.59, or 0.51 g of straw, woodchip, and biochar, respectively. The treatments were then mixed into the soil. A volume of water equal to the volume of digestate was added to the non-amended control pots and similarly mixed. The rationale for this amendment rate is given in the supporting information. The pots were loosely covered with lids to reduce moisture loss and weighed twice a week to check the moisture content and deionised water was added to maintain soils at 40% water holding capacity, which is optimal for microbial development (Gulledge and Schimel, 1998). At each sampling time, the pots were destructively sampled and the soil passed through a 2 mm sieve to break down the aggregates that had formed when mixing treatments into the soil, in order to homogenise the sample ready for analysis.
2.3 Microbial analyses
Microbial biomass carbon and nitrogen (microbial C and N respectively) were determined following the fumigation-extraction method (Vance et al., 1987). After extraction, the extracts were analysed on a Shimadzu TOC with a TN module. The microbial C and N were calculated using KEC and KEN values of 0.45 and 0.54, respectively (Brookes et al., 1985; Vance et al., 1987). PLFA profiles were determined using a modified method from Frostegård et al. (1991) by freeze-drying the soil after sieving. Lipids were extracted from 10 g freeze-dried soil using the Bligh and Dyer (1959) solvent ratio 1:2:0.8 v/v/v of chloroform, methanol, and a pH 4 citrate buffer, fractionated, and the phospholipids derivatised by mild alkaline methanolysis. The resultant fatty acid methyl esters were separated by gas chromatography (Agilent Technologies, Santa Clara, CA, United States) using a HP-5 (Agilent Technologies) capillary column (30 m length, 0.32 mm ID, 0.25 μm film). The GC conditions were reported in Pawlett et al. (2013). Resultant peak areas were integrated using G2070 ChemStation (Agilent Technologies) for gas chromatography and calculated as relative abundance (mol %). Bacteria were identified by the PLFA bioindicators 14:00, 15:0i, 15:0ai, 15:00, 16:0i, 16:1ω7c, 16:00, 17:0i, cyc17:0, ai17:0, 17:0br, 17:1ω8c, 17:1ω8t, 17:1ω7, 18:00, 18:0 (10Me), 18:1ω13 and 20:00 (Frostegård and Bååth, 1996; White et al., 1996; Zelles, 1997, 1999; Bossio and Scow, 1998; Kourtev et al., 2002). Fungi were identified by the biomarker 18:2ω6,9 (Frostegård and Bååth, 1996). The fungi-to-bacteria ratio was calculated by dividing the mol % of the fungal biomarker (18:2ω6,9) by the summed mol % of bacterial fatty acids (Frostegård and Bååth, 1996).
2.4 Chemical analyses
Soil total nitrogen was determined by dry combustion according to the British Standard Institution (BS EN 13654-2:2001) and analysed using an elemental analyser (Elementar, Vario EL III). Available nitrogen as the sum of ammonia and total oxides of nitrogen was determined using the potassium chloride (KCl) extraction method (MAFF, 1986). 20 g of soil was eluted with 100 mL of 2 mol KCl solution and filtered, after which the extracts were analysed on an analytical segmented flow multi-chemistry analyser (Seal, AA3).
2.5 Statistics
Data were first tested for normality using Kolmogorov–Smirnov & Lilliefors test and homoscedasticity using Levene’s Test, following this the available N, microbial C and N datasets were box-cox transformed. As the independent experimental variable of interest was treatment, a one-way ANOVA was used determine treatment effects at each sampling time on the soil parameters, whilst time as factor was not included due to unequal variance in data between sampling times. Significant differences between treatments for available and total N, and fungi:bateria (F:B) ratio were determined by Tukey’s post hoc test. The microbial C and N datasets still failed homoscedasticity so a one-way Welch ANOVA was used as it can tolerate unequal variance (Wilcox, 2003) and significant differences between treatments were determined by Games-Howell’s post hoc test. Principle Component Analysis was run on the PLFA profile data for each timepoint, which was normalised by measuring each biomarker as the relative abundance (%mol) to all the biomarkers. The resultant factor scores for each timepoint were analysed using a one-way ANOVA. All differences were considered statistically significant if p < 0.05. All statistical analysis was carried out in Statistica version 14.
3 Results
3.1 Biological properties of the soil
The addition of digestate alone to the soil did not result in higher microbial biomass (C) than the soil only control treatment at any timepoint. At 3 h after application, the addition of glycerol to digestate resulted in a significantly (p = 0.004) higher microbial biomass (C) by 344% compared to the digestate only control (Figure 1A). At 90 days the digestate with either straw or woodchip had a higher microbial biomass than soil only, but not the digestate control. At 150 days, the ANOVA recorded a significant effect due to treatment (p = 0.001), but the post hoc test did not identify any significant differences between individual treatments.
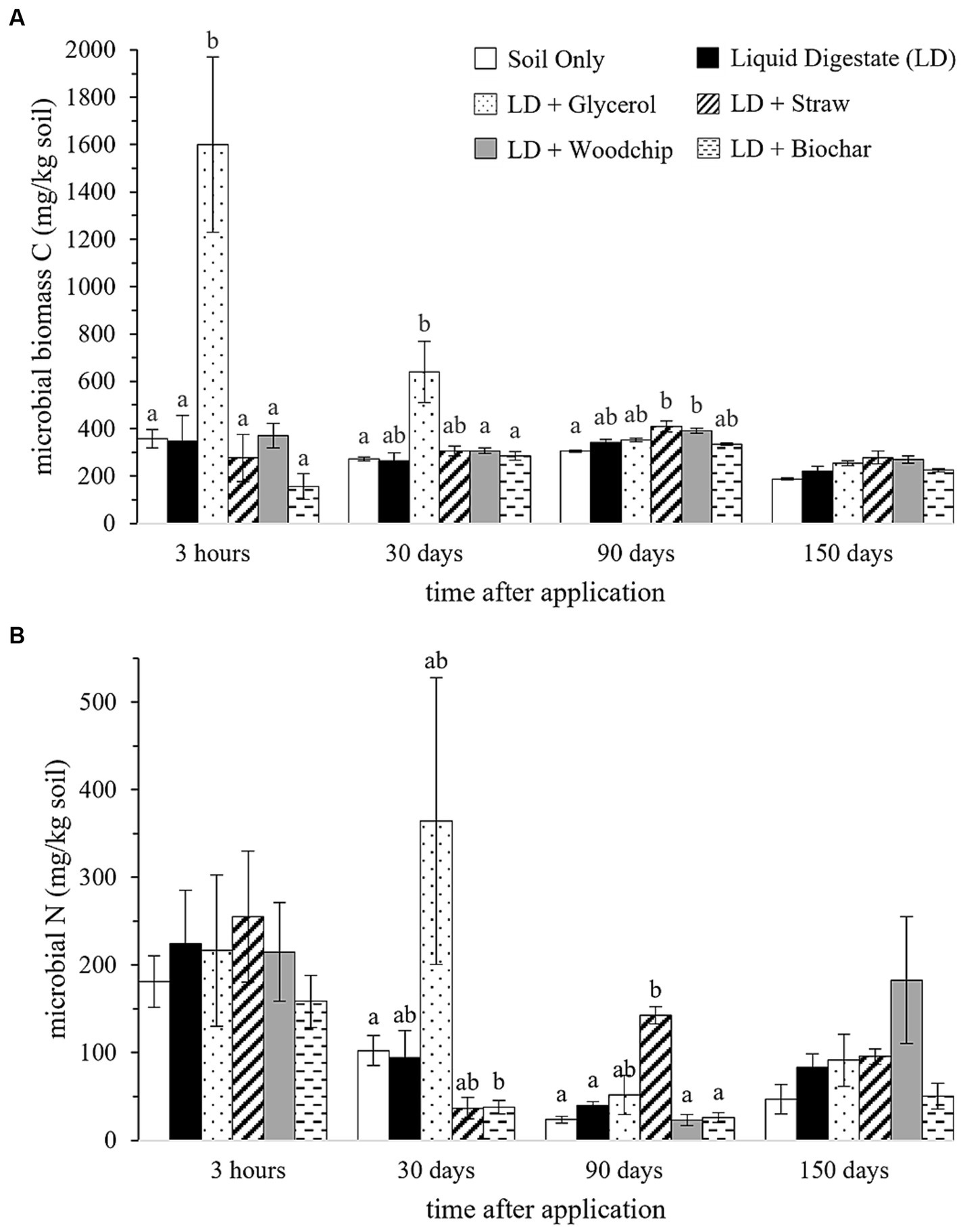
Figure 1. Changes in soil microbial biomass carbon (A) and microbial N (B). At each sampling time points denoting different lower-case letter have statistically different treatments effects according to Games-Howell’s test at 5% probability on box-cox transformed data. Error bars denote the standard error from the mean, n = 4. In graph B, LD + woodchip at 30 days was not included into the analysis, due to only one replicate producing analysable data.
Microbial N was not significantly (p = 0.95) affected by treatments at 3 h after application (Figure 1B). At 30 days the LD + biochar had a significantly (p < 0.05) lower concentration than soil only control, but not the digestate only control. At 90 days after application digestate applied with straw significantly (p = 0.009) immobilised 80 μg-N g−1 (dry weight soil), equivalent to 75 kg-N ha−1 (see Supplementary material for calculation) compared to the digestate only control, a difference of 309%. After 90 days no further significant treatment effect was observed, however digestate with woodchip had a 2x higher on average microbial N content compared to digestate only treatment.
The first two principal components (PC) on each of the PLFA datasets accounted for ≥50% of the total variation (Figure 2), with significant (p < 0.05) treatment effects on PC1 axis at every timepoint except 150 days after application. Fatty acid loadings (≥0.8 and ≤0.8) that contributed the most included 16:00, 16:1ω5, 17:0c, 18:2ω6,9 and 19:1ω6 for PC1 and 17:0br, 17:1ω8c and 18:1ω7t for PC2, for information on specific PLFAs for each PCA see Supplementary Table 1. At both 3 h and 30 days after application the microbial communities between the digestate and the soil only controls were distinctly separate (Figures 2A,B; p = 0.01) with a higher fungi:bacteria (F:B) ratio in the digestate control at day 30 (Table 2). At 30 days the addition of woodchips and biochar resulted in separate cluster with the soil only control, distinct from a group made of the glycerol additive and the digestate control (Figure 2B; p < 0.001), with a lower F:B ratio in the former treatments compared to the latter (Table 2), whilst the straw treatment lay non-distinctly between the two groups. At 90 days the woodchip amended grouped separately from the glycerol and straw amended digestate treatments (Figure 2C; p = 0.02), with a lower F:B ratio in the woodchip treatment compared to the straw and glycerol treatments (Table 2). By day 150 there were no distinct groupings between the digestate with and without additives (Figure 2D; p = 0.06) and no difference in the F:B ratios (Table 2).
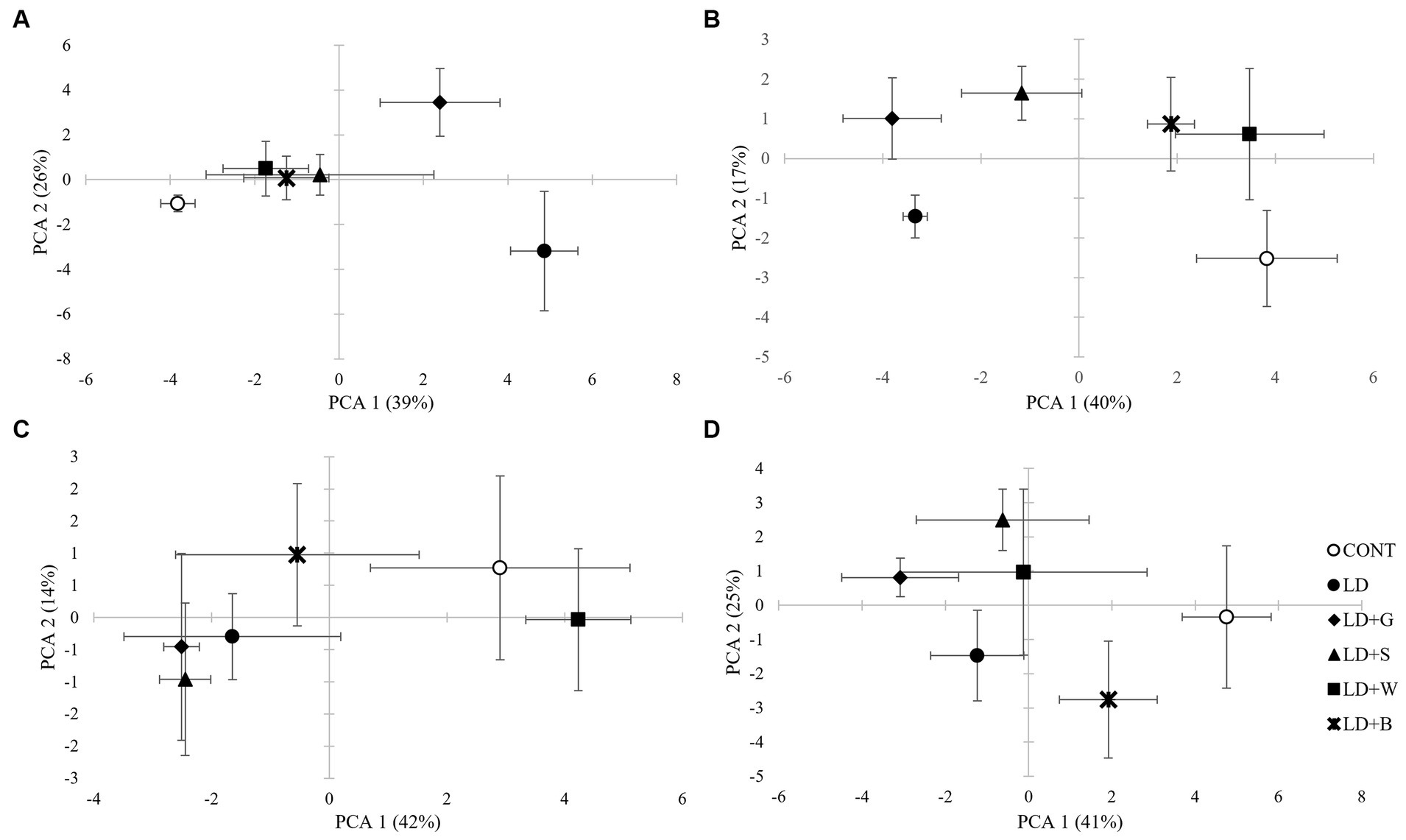
Figure 2. Principal component analysis on the PLFA profiles (mean ± SE, n = 4) of the microbial community as affected by the treatments at each sampling time: A = 3 hrs, B = 30 days, C = 90 days and D = 150 days, after application. Cont, soil only control; LD, liquid digestate control; LD + G, liquid digestate + glycerol; LD + S, liquid digestate + straw; LD + W, liquid digestate + woodchips; LD + B, liquid digestate + biochar.
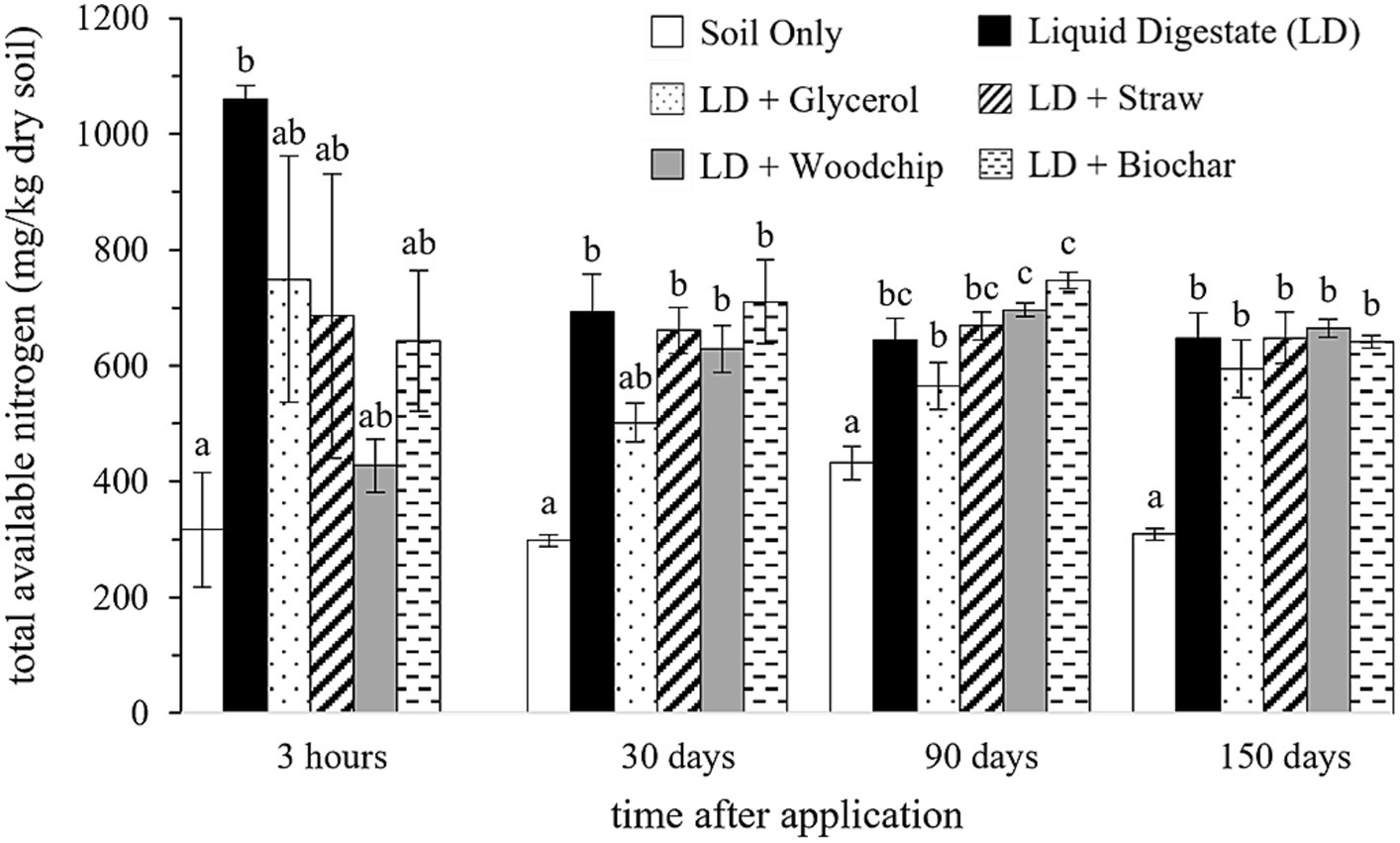
Figure 3. Changes in soil available nitrogen. At each sampling time point different lower-case letters between treatments have statistically different effects according to Tukey’s test at 5% probability on box-cox transformed data. Error bars denote the standard error from the mean (n = 4).
3.2 Chemical properties of the soil
3 h after treatment applications, the amount of total available nitrogen was significantly greater (p = 0.02) in the digestate (LD) control treatment compared to the soil only control and remained so for the experimental duration (Figure 3). The addition of additives into digestate had no effect on total available N compared to digestate control at all timepoints, however at day 90 the digestate with glycerol had a lower content compared to the digestate mixed with either woodchip or biochar.
The liquid digestate resulted in a significantly (p ≤ 0.004) greater amount of total soil nitrogen compared to the soil only control from 30 days onwards (Table 3). Mixing additives into digestate did not result in any further significant differences to the total soil nitrogen content apart from at 90 days after application where there was a lower total nitrogen in the digestate with straw treatment compared to woodchip and biochar amended digestates.
4 Discussion
The addition of straw to digestate elevated microbial N in the soil by 2.5× compared to digestate alone, by an amount equivalent to 75 kg-N ha−1, demonstrating that straw stimulated the immobilisation of N into microorganisms. This immobilisation is in agreement with studies by Cao et al. (2018) and Reichel et al. (2018) who applied straw with synthetic nitrogen fertilisers, Chaves et al. (2005) who added straw with nitrogen rich crop residue, and Wang et al. (2019) who applied digestate with rice straw. However, in this study, microbial growth cannot explain the uptake of N in biomass as the microbial biomass carbon under straw + digestate was not significantly greater than the digestate treatment. Microbes do not only uptake N as they grow and reproduce, but their C:N stoichiometry can change to adapt to nutrient limitations, or alleviation thereof (Heuck et al., 2015; Chen et al., 2019). In fungi, Khan and Joergensen (2019) suggested that N immobilisation can occur by storage of nitrogen-based compounds in vacuoles. Therefore, it is likely that a combination of both biomass incorporation and storage in fungal vacuoles caused the significant microbial N effect due to straw addition.
Contrary to the hypothesis, the addition of glycerol to digestate did not result in a significant microbial nitrogen immobilisation, despite the significantly greater growth in the first month from application. However, its addition did lead to a 4× higher on average microbial N at 30 days after application compared to digestate control. Significant results may be masked by experimental artefacts that can occur when comparing comparatively low amounts of extracted nitrogen between fumigated and non-fumigated soil relative to the large inputs of soluble N (Widmer et al., 1989), as from digestate. Time of sampling could also have been an issue, as de la Fuente et al. (2013) observed that digestates made from a feedstock mix of cattle slurry with 4% and 6% glycerol stimulated a peak nitrogen immobilisation within a week of application. Since we did not sample between 3 h and 30 days after application, it is possible that the peak of N immobilisation was missed.
The addition of woodchips to digestate had no significant effect on microbial growth or microbial N compared to digestate alone, although at 150 days microbial N was 2x higher on average than digestate alone. Similarly, Tahboub et al. (2007) observed no effect on nitrogen immobilisation when adding woodchips with synthetic fertiliser. However, Reichel et al. (2018) observed significant nitrogen immobilisation when adding sawdust with synthetic N, as did Chaves et al. (2005) when adding sawdust with nitrogen rich celery leaves. These significant effects could be due to the smaller particle size of sawdust (~1 mm) compared to woodchips (majority between 2.5–5 cm) used by Tahboub et al. (2007) and the 1–2 cm woodchips used in our study. This meant that our samples had less surface area per volume exposed for microbial colonisation which reduces decomposition rates (Idler et al., 2019). Furthermore, woodchips are mainly decomposed by fungi (Noll and Jirjis, 2012), yet the fungi to bacteria ratio in the woodchip amended soil of this study was never greater than digestate alone amended soil. This indicates that the fungal community in the soil did not include those that could effectively utilise the woodchips as carbon source. This could also have been a result of sampling bias, as the soil was sieved prior to PLFA analysis, removing pieces of woodchip greater than 2 mm and excluding any fungi colonising those woodchips from the analysis.
Mixing biochar into digestate did not have any effect on microbial biomass or nitrogen immobilisation. This corroborates results from Martin et al. (2015) who also observed no effect of adding biochar with digestate on either microbial C or N. In biochar the carbon is formed into complex aromatic structures (Schmidt et al., 2000) that are extremely resistant to decomposition (Wang et al., 2016). Additionally, the highly porous structure of biochar absorbs dissolved organic carbon, reducing the amount available to microbes to utilise (Mukherjee et al., 2016). Yet, Holatko et al. (2021) observed increased microbial biomass C from digestate amended with biochar after 6 weeks, with a correlated decrease in soil nitrogen that could indicate nitrogen immobilisation had occurred. It is possible that the differing effects of biochar addition on nitrogen immobilisation between the current study and Holatko et al. (2021) may be due to differences in the amount and type of biochar used. Holatko et al. (2021) applied twice the concentration of biochar (0.4 kg per litre of digestate compared to 0.2 kg per litre) and used agricultural grain-waste biochar pyrolysed at 600–650°C (cf 700°C–800°C office waste biochar used here). Biochar feedstock and processing temperatures, of which there are numerous options (Lehmann et al., 2011; Wang et al., 2016), determine the size of a small biochar-associated labile carbon pool. It is possible that the biochar used by Holatko et al. (2021) had a greater concentration of labile carbon and therefore stimulated microbial growth.
The immobilisation of nitrogen observed when digestate was mixed with straw demonstrates that microorganisms can be used as a potential mechanism to reduce the nitrogen losses from anaerobic digestate application. However, the quantity of N immobilised should be reflected in a lower concentration of soil available nitrogen. Yet contrary to this, the greater microbial N under straw addition did not result in a lower soil available nitrogen. This could be due to the extent of change in microbial N being too small to make a noticeable difference in the soil available N pool. There are several studies that supplied greater quantities of carbon than the 0.5 t-C ha−1 in this study. For example, 1.3 t-C ha−1 was applied by Cao et al. (2018) and 4.5 t-C ha−1 was applied by Reichel et al. (2018) as either straw or sawdust with synthetic nitrogen fertilisers, and 2.5 t-C ha−1 was applied by Chaves et al. (2005) who added straw and sawdust separately with nitrogen rich crop residue. All these studies recorded significant N immobilisation. de la Fuente et al. (2013) used digestates with a feedstock of 4% and 6% glycerol and saw N immobilisation, whereas our digestate mix contained 3% glycerol. However, for the amount of carbon added in this experiment (1,400 mg-C kg−1), the changes in microbial N and soil available N under the digestate and glycerol did meet expectations. Working on the assumption that microbes use 1 unit of N per 8 units of carbon to satisfy stoichiometry for growth (Sinsabaugh et al., 2016), then the increase of 1,200 mg-C kg−1 in microbial biomass due to glycerol addition at 3 h after application would immobilise 155 mg-N kg−1. The measured average reduction in available N due to glycerol addition compared to the digestate alone was higher than the estimate at 195 mg-N kg−1, due to variability in sample measurements between replicates. It appears that the high volume of nitrogen added by the digestate, requires a larger N uptake by microbes than what occurred in this study to significantly reduce the soil available N pool. This indicates that adding more carbon than we did into the digestate is necessary to increase the magnitude of microbial growth.
Whilst our system was carbon limited, we were still able to identify trends in the influence of carbon source on its bioavailability and subsequent impact on the magnitude and timing of soil microbial N uptake. Glycerol resulted in the greatest and quickest growth that occurred in the first month with a microbial N higher on average by 4× than digestate, followed by straw with a microbial N concentration greater by 2.5× than digestate. In an experiment comparing glycerol, straw and grass as N immobilisers, Redmile-Gordon et al. (2014) observed that the addition of glycerol with nitrogen had a quicker and greater magnitude of immobilisation compared to the straw-N mix, attributable to the greater lability, and therefore bioavailability, of carbon in glycerol compared to straw. Soil incubation experiments by Reichel et al. (2018) and Chaves et al. (2005), who used both straw and sawdust as immobilisers of nitrogen-rich sources, observed that nitrogen immobilisation under sawdust was of lower magnitude and occurred later compared to straw. Similarly, our woodchip-digestate had a later and lower on-average microbial N peak compared to the straw addition. This is attributable to the higher lignin content in woody material, which slows decomposition rates and therefore carbon availability to microbes (Melillo et al., 1982; Lehmann et al., 1995; Rahn and Lillywhite, 2002). Biochar was the most recalcitrant material used in our study and its addition to digestate had no positive influence on microbial N, as observed by Manirakiza et al. (2019) when they mixed biochar into biosolids. Alotaibi and Schoenau (2016) mixed biochar with urea to a C:N ratio of 20:1, higher than our digestate + biochar mix, and measured a reduction in microbial biomass after 4 months compared to urea alone. In contrast, the microbial biomass under their glycerol-urea mix was no different to urea control, which corroborates our results, confirming that glycerol has a quick and short-term influence. As such the hypothesis that the lability of carbon in the organic material influences the time and magnitude of microbial N uptake can be accepted.
The phenotypic (PLFA) profile of the soil community changed with the application of digestate, with the soil only treatment resulting in a group distinct from the digestate treated soil. A month after application two distinct groups emerged: digestate alone and with glycerol and straw, and digestate with woodchip and biochar and the unamended soil. A higher ratio of fungi to bacteria was present in the former group. This is contrary to the hypothesis, which expected that the additives containing more labile carbon would increase the relative abundance of bacteria compared to fungi. Approximately a third of the fungi in digestate are yeasts and moulds, which are quick growing (Coelho et al., 2020). The labile carbon supplied by the digestate, glycerol and straw provided a carbon source for these quick growing fungi from both the digestate and the soil. Meanwhile biochar, being able to sorb carbon and nutrients (Mukherjee et al., 2016; Ding et al., 2020), may have reduced the availability of these compounds, which may be a reason that digestate with biochar did not result in a change in the community composition compared to the soil only control. The merging of groups after 3 months is consistent with decreases in abundance of quick growing microorganisms as they exhaust their labile carbon supply (Meidute et al., 2008), shifting the community to a composition similar to the control soil.
There are practical implications for adding materials into digestate. In this study chopped straw was used, which resulted in significant immobilisation, however this is difficult in terms of mixing it into the digestate to ensure a homogeneous distribution followed by difficulties spreading it evenly onto a field, particularly with band or injection spreading equipment and systems where the digestate is transported to the applicator unit by an umbilical system, where straw addition may increase the chances of blockages occurring. An alternative would be to apply digestate and straw separately. Adding digestate to straw on the soil surface is problematic, as this reduces the infiltration rate of the digestate, resulting in increased ammonia gaseous losses (Cao et al., 2018). Whilst there are applicators that inject digestate straight into the soil through a straw layer to reduce ammonia losses, this results in spatial separation of the straw on the soil surface and the digestate below the surface. Therefore, microorganisms in the soil do not have access to the straw and cannot acquire the carbon they need to immobilise nitrogen from the digestate, as evidenced by Aita et al. (2012) who observed increased decomposition rates when slurry and straw were incorporated into the soil as opposed to straw remaining on soil surface. This would mean that the addition of straw to digestate is a mixture that is limited for use on crops that combine fertilisation with seedbed preparation.
This study demonstrated that the use of a high organic carbon material additives can result in microbial growth and uptake of digestate supplied nitrogen. To meet its potential as a mechanism to reduce nitrogen losses from the application of anaerobic digestate, more carbon is required than was applied in this study, however we have demonstrated that materials high in carbon, of which the majority is in an easily degradable form (i.e., low in lignin or char content) have the greatest potential to do this. Under field conditions the nitrogen immobilisation and remineralisation may be slower than found in this study, due to fluctuating temperatures and variable rainfall (Sun et al., 2019). Potentially less nitrogen would be immobilised overall as leaching and volatilisation would remove some nitrogen from the soil alongside immobilisation. Therefore, our findings that high organic carbon materials immobilise digestate supplied nitrogen need to be verified under field conditions. Should it prove possible to control digestate supplied nitrogen using this approach, then further work will be required to determine agronomic effects.
Data availability statement
The datasets presented in this study can be found in online repositories. The names of the repository/repositories and accession number(s) can be found at: https://doi.org/10.17862/cranfield.rd.24808605.
Author contributions
CM: Conceptualization, Investigation, Methodology, Project administration, Writing – original draft, Writing – review & editing, Formal analysis. JH: Conceptualization, Methodology, Supervision, Writing – review & editing. LS: Conceptualization, Methodology, Supervision, Writing – review & editing. TS: Conceptualization, Methodology, Supervision, Writing – review & editing. HM: Conceptualization, Funding acquisition, Methodology, Resources, Writing – review & editing. MP: Conceptualization, Funding acquisition, Methodology, Supervision, Writing – review & editing.
Funding
The author(s) declare that financial support was received for the research, authorship, and/or publication of this article. This research was funded by UKRI BBSRC FoodBioSystems Doctoral Training Partnership (DTP), grant number BB/T008776/1 and Future Biogas Ltd.
Acknowledgments
The authors would like to thank Denise Cysneiros at Future Biogas Ltd. for her input to the project. We are very grateful for the help and support by the EAF technical team, in particular Richard Andrews, Nuannat Simmons, Paul Barton, Maria Biskupska, Tammy Cowley, and Dave Sharpe. We would like to thank Jan Mumme at Carbogenics for providing us with the biochar used in this study.
Conflict of interest
HM was employed by Future Biogas Ltd., from whom this study received funding. The funder had the following involvement with the study: provision of digestate used.
The remaining authors declare that the research was conducted in the absence of any commercial or financial relationships that could be construed as a potential conflict of interest.
Publisher’s note
All claims expressed in this article are solely those of the authors and do not necessarily represent those of their affiliated organizations, or those of the publisher, the editors and the reviewers. Any product that may be evaluated in this article, or claim that may be made by its manufacturer, is not guaranteed or endorsed by the publisher.
Supplementary material
The Supplementary material for this article can be found online at: https://www.frontiersin.org/articles/10.3389/fsufs.2024.1356469/full#supplementary-material
References
Aita, C., Recous, S., Cargnin, R. H. O., da Luz, L. P., and Giacomini, S. J. (2012). Impact on C and N dynamics of simultaneous application of pig slurry and wheat straw, as affected by their initial locations in soil. Biol. Fertil. Soils 48, 633–642. doi: 10.1007/s00374-011-0658-x
Alburquerque, J. A., de la Fuente, C., and Bernal, M. P. (2012). Chemical properties of anaerobic digestates affecting C and N dynamics in amended soils. Agric. Ecosyst. Environ. 160, 15–22. doi: 10.1016/j.agee.2011.03.007
Alotaibi, K. D., and Schoenau, J. J. (2016). Application of two bioenergy byproducts with contrasting carbon availability to a prairie soil: three-year crop response and changes in soil biological and chemical properties. Agronomy 6:6010. doi: 10.3390/agronomy6010
Al-Seadi, T., and Lukehurst, C. (2012) ‘Quality management of digestate from biogas plants used as fertiliser’, IEA Bioenergy, Task, (Task 37-Energy from Biogas), p. 40. Available at: http://www.iea-biogas.net/files/daten-redaktion/download/publi-task37/digestate_quality_web_new.pdf
Bareha, Y., Girault, R., Jimenez, J., and Trémier, A. (2018). Characterization and prediction of organic nitrogen biodegradability during anaerobic digestion: a bioaccessibility approach. Bioresour. Technol. 263, 425–436. doi: 10.1016/j.biortech.2018.04.085
Barzee, T. J., Edalati, A., el-Mashad, H., Wang, D., Scow, K., and Zhang, R. (2019). Digestate biofertilizers support similar or higher tomato yields and quality than mineral fertilizer in a subsurface drip fertigation system. Front Sustain Food Syst 3, 1–13. doi: 10.3389/fsufs.2019.00058
Bligh, E., and Dyer, W. (1959). A rapid method of total lipid extraction and purification. Can. J. Biochem. Physiol. 37, 911–917. doi: 10.1139/y59-099
Bossio, D. A., and Scow, K. M. (1998). Impacts of carbon and flooding on soil microbial communities: phospholipid fatty acid profiles and substrate utilization patterns. Microb. Ecol. 35, 265–278. doi: 10.1007/s002489900082
Brookes, P. C., Landman, A., Pruden, G., and Jenkinson, D. S. (1985). Chloroform fumigation and the release of soil nitrogen: a rapid direct extraction method to measure microbial biomass nitrogen in soil. Soil Biol. Biochem. 17, 837–842. doi: 10.1016/0038-0717(85)90144-0
Cao, Y., Sun, H., Zhang, J., Chen, G., Zhu, H., Zhou, S., et al. (2018). Effects of wheat straw addition on dynamics and fate of nitrogen applied to paddy soils. Soil Tillage Res. 178, 92–98. doi: 10.1016/j.still.2017.12.023
Chambers, B., Nicholson, N., and Smith, K. (2007) ‘Spreading systems for slurries and solid manures ’. Mansfield: ADAS.
Chaves, B., de Neve, S., Boeckx, P., van Cleemput, O., and Hofman, G. (2005). Screening organic biological wastes for their potential to manipulate the N release from N-rich vegetable crop residues in soil. Agric. Ecosyst. Environ. 111, 81–92. doi: 10.1016/j.agee.2005.03.018
Chen, J., Seven, J., Zilla, T., Dippold, M. A., Blagodatskaya, E., and Kuzyakov, Y. (2019). Microbial C:N:P stoichiometry and turnover depend on nutrients availability in soil: a 14 C, 15 N and 33 P triple labelling study. Soil Biol. Biochem. 131, 206–216. Availabe at:. doi: 10.1016/j.soilbio.2019.01.017
Coelho, J. J., Hennessy, A., Casey, I., Bragança, C. R. S., Woodcock, T., and Kennedy, N. (2020). Biofertilisation with anaerobic digestates: a field study of effects on soil microbial abundance and diversity. Appl. Soil Ecol. 147:103403. doi: 10.1016/j.apsoil.2019.103403
Czekała, W. (2022). Digestate as a source of nutrients: nitrogen and its fractions. Water 14:4067. doi: 10.3390/w14244067
da Silva, G. P., Mack, M., and Contiero, J. (2009). Glycerol: a promising and abundant carbon source for industrial microbiology. Biotechnol. Adv. 27, 30–39. doi: 10.1016/j.biotechadv.2008.07.006
de la Fuente, C., Alburquerque, J. A., Clemente, R., and Bernal, M. P. (2013). Soil C and N mineralisation and agricultural value of the products of an anaerobic digestion system. Biol. Fertil. Soils 49, 313–322. doi: 10.1007/s00374-012-0719-9
DEFRA and EA (2018) ‘Collection-Nitrate vulnerable zones’. Available at: https://www.gov.uk/government/collections/nitrate-vulnerable-zones#full-publication-update-history.
Ding, J., Shen, Y., Ma, Y., Meng, H., Cheng, H., Zhang, X., et al. (2020). Study on dynamic sorption characteristics of modified biochars for ammonium in biogas slurry. Int J Agric Biol Engineer 13, 234–240. doi: 10.25165/j.ijabe.20201301.4697
Dobson, R., Gray, V., and Rumbold, K. (2012). Microbial utilization of crude glycerol for the production of value-added products. J. Ind. Microbiol. Biotechnol. 39, 217–226. doi: 10.1007/s10295-011-1038-0
Drame, M., Carswell, A., Roberts, W., Hood, J., Jemo, M., Heuer, S., et al. (2023). Effects of hotter, drier conditions on gaseous losses from nitrogen fertilisers. J. Environ. Manage. 345:118671. doi: 10.1016/j.jenvman.2023.118671
Frostegård, A., and Bååth, E. (1996). The use of phospholipid fatty acid analysis to estimate bacterial and fungal biomass in soil. Biol. Fertil. Soils 22, 59–65. doi: 10.1007/s003740050076
Frostegård, A., Tunlid, A., and Bååth, E. (1991). Microbial biomass measured as total lipid phosphate in soils of different organic content. J. Microbiol. Methods 14, 151–163. doi: 10.1016/0167-7012(91)90018-L
Gebremikael, M. T., Ranasinghe, A., Hosseini, P. S., Laboan, B., Sonneveld, E., Pipan, M., et al. (2020). How do novel and conventional Agri-food wastes, co-products and by-products improve soil functions and soil quality? Waste Manag. 113, 132–144. doi: 10.1016/j.wasman.2020.05.040
Giacometti, C., et al. (2020). ‘Potential Nitrate Leaching through Soil Columns Treated with Animal Slurries and Anaerobic Digestate’, Agronomy 10, 865. doi: 10.3390/agronomy10060865
Gulledge, J., and Schimel, J. (1998). Moisture control over atmospheric CH4 consumption and CO2 production in diverse Alaskan soils. Soil Biol. Biochem. 30, 1127–1132. doi: 10.1016/S0038-0717(97)00209-5
Harding, D. E., and Ross, D. J. (1964). Some factors in low-temperature storage influencing the mineralisable-nitrogen of soils. J. Sci. Food Agric. 15, 829–834. doi: 10.1002/jsfa.2740151203
Heuck, C., Weig, A., and Spohn, M. (2015). Soil microbial biomass C: N: P stoichiometry and microbial use of organic phosphorus. Soil Biol. Biochem. 85, 119–129. doi: 10.1016/j.soilbio.2015.02.029
Hegewald, H., et al. (2021). ‘Nitrification inhibitors reduce N 2 O emissions induced by application of biogas digestate to oilseed rape’, Nutrient Cycling in Agroecosystemst, 120, 99–118. doi: 10.1007/s10705-021-10127-8
Holatko, J., Hammerschmiedt, T., Kintl, A., Danish, S., Skarpa, P., Latal, O., et al. (2021). Effect of carbon-enriched digestate on the microbial soil activity. PLoS One 16, e0252262–e0252213. doi: 10.1371/journal.pone.0252262
Huf, M., and Olfs, H. (2020). Effect of the nitrification inhibitor DMPP on nitrous oxide emissions and the stabilization of ammonium following the injection of dairy slurry and digestate in a soil-column experiment. J. Plant Nutr. Soil Sci. 183, 129–135. doi: 10.1002/jpln.201900025
Idler, C., Pecenka, R., and Lenz, H. (2019). In fluence of the particle size of poplar wood chips on the development of mesophilic and thermotolerant mould during storage and their potential impact on dry matter losses in piles in practice. Biomass Bioenergy 127:105273. doi: 10.1016/j.biombioe.2019.105273
Iocoli, A. G., Zabaloy, M. C., Pasdevicelli, G., and Gómez, M. A. (2019). Use of biogas digestates obtained by anaerobic digestion and co-digestion as fertilizers: characterization, soil biological activity and growth dynamic of Lactuca sativa L. Sci. Total Environ. 647, 11–19. doi: 10.1016/j.scitotenv.2018.07.444
Johansen, A., Carter, M. S., Jensen, E. S., Hauggard-Nielsen, H., and Ambus, P. (2013). Effects of digestate from anaerobically digested cattle slurry and plant materials on soil microbial community and emission of CO2 and N2O. Appl. Soil Ecol. 63, 36–44. doi: 10.1016/j.apsoil.2012.09.003
Khan, K. S., and Joergensen, R. G. (2019). Stoichiometry of the soil microbial biomass in response to amendments with varying C/N/P/S ratios. Biol. Fertil. Soils 55, 265–274. doi: 10.1007/s00374-019-01346-x
Kourtev, P. S., Ehrenfeld, J. G., and Haggblom, M. (2002). Exotic plant species alter the microbial community structure and function in the soil. Ecology 83, 3152–3166. doi: 10.1890/0012-9658(2002)083[3152:EPSATM]2.0.CO;2
Lehmann, J., Rillig, M. C., Thies, J., Masiello, C. A., Hockaday, W. C., and Crowley, D. (2011). Biochar effects on soil biota—a review. Soil Biol. Biochem. 43, 1812–1836. doi: 10.1016/j.soilbio.2011.04.022
Lehmann, J., Schroth, G., and Zech, W. (1995). Decomposition and nutrient release from leaves, twigs and roots of three alley-cropped tree legumes in Central Togo. Agr. Syst. 29, 21–36. doi: 10.1007/BF00711279
Lyons, G. A., Cathcart, A., Frost, J. P., Wills, M., Johnston, C., Ramsey, R., et al. (2021). Review of two mechanical separation technologies for the sustainable management of agricultural phosphorus in nutrient-vulnerable zones. Agronomy 11:836. doi: 10.3390/agronomy11050836
MAFF: Ministry of Agriculture Fisheries and Food (1986). “Method 53 of MAAF reference book” in RB427 analysis of agricultural material. HM Stationary Office, London.
MacLeod, M., Eory, V., Gruere, G., and Lankoski, J. (2015). Cost-Efectiveness of greenhouse gas mitigation measures for agriculture: a literature review. OECD Food Agri and Fisher Papers 89. doi: 10.1787/5jrvvkq900vj-en
Manirakiza, E., Ziadi, N., St Luce, M., Hamel, C., Antoun, H., and Karam, A. (2019). Nitrogen mineralization and microbial biomass carbon and nitrogen in response to co-application of biochar and paper mill biosolids. Appl. Soil Ecol. 142, 90–98. doi: 10.1016/j.apsoil.2019.04.025
Martin, S. L., Clarke, M. L., Othman, M., Ramsden, S. J., and West, H. M. (2015). Biochar-mediated reductions in greenhouse gas emissions from soil amended with anaerobic digestates. Biomass Bioenergy 79, 39–49. doi: 10.1016/j.biombioe.2015.04.030
Meidute, S., Demoling, F., and Bååth, E. (2008). Antagonistic and synergistic effects of fungal and bacterial growth in soil after adding different carbon and nitrogen sources. Soil Biol. Biochem. 40, 2334–2343. doi: 10.1016/j.soilbio.2008.05.011
Meier, I. C., Finzi, A. C., and Phillips, R. P. (2017). Root exudates increase N availability by stimulating microbial turnover of fast-cycling N pools. Soil Biol. Biochem. 106, 119–128. doi: 10.1016/j.soilbio.2016.12.004
Mekonnen, A. (2021). A review on biodegradation and biological treatments of cellulose, hemicellulose and lignin. Glob J Biol 10, 10–12. doi: 10.35248/2319-5584.21.10.103
Melillo, J. M., Aber, J. D., and Muratore, J. F. (1982). Nitrogen and lignin control of hardwood leaf litter decomposition dynamics. Ecology 63, 621–626. doi: 10.2307/1936780
Möller, K. (2015). Effects of anaerobic digestion on soil carbon, nitrogen turnover, N emissions and soil biological activity: a review. Agron. Sustain. Dev. 35, 1021–1041. doi: 10.1007/s13593-015-0284-3
Mortola, N., Romaniuk, R., Cosentino, V., Eiza, M., Carfagno, P., Rizzo, P., et al. (2019). Potential use of a poultry manure Digestate as a Biofertiliser: evaluation of soil properties and Lactuca sativa growth. Pedosphere 29, 60–69. doi: 10.1016/S1002-0160(18)60057-8
Mukherjee, S., Weihermueller, L., Tappe, W., Vereecken, H., and Burauel, P. (2016). Microbial respiration of biochar- and digestate-based mixtures. Biol. Fertil. Soils 52, 151–164. doi: 10.1007/s00374-015-1060-x
Nicholson, F. A., Bhogal, A., Rollett, A., Taylor, M., and Williams, J. R. (2018). Precision application techniques reduce ammonia emissions following food-based digestate applications to grassland. Nutr. Cycl. Agroecosyst. 110, 151–159. doi: 10.1007/s10705-017-9884-4
Nkoa, R. (2014). Agricultural benefits and environmental risks of soil fertilization with anaerobic digestates: a review. Agron. Sustain. Dev. 34, 473–492. doi: 10.1007/s13593-013-0196-z
Noll, M., and Jirjis, R. (2012). Microbial communities in large-scale wood piles and their effects on wood quality and the environment. Appl. Microbiol. Biotechnol. 95, 551–563. doi: 10.1007/s00253-012-4164-3
Pawlett, M., Ritz, K., Dorey, R. A., Rocks, S., Ramsden, J., and Harris, J. A. (2013). The impact of zero-valent iron nanoparticles upon soil microbial communities is context dependent. Environ. Sci. Pollut. Res. 20, 1041–1049. doi: 10.1007/s11356-012-1196-2
Rahn, C. R., and Lillywhite, R. D. (2002). A study of the quality factors affecting the short-term decomposition of field vegetable residues. J. Sci. Food Agric. 82, 19–26. doi: 10.1002/jsfa.1003
Redmile-Gordon, M. A., Armenise, E., Hirsch, P. R., and Brookes, P. C. (2014). Biodiesel co-product (BCP) decreases soil nitrogen (N) losses to groundwater. Water Air Soil Pollut. 225:1831. doi: 10.1007/s11270-013-1831-7
Reichel, R., Wei, J., Islam, M. S., Schmid, C., Wissel, H., Schröder, P., et al. (2018). Potential of wheat straw, spruce sawdust, and lignin as high organic carbon soil amendments to improve agricultural nitrogen retention capacity: an incubation study. Front. Plant Sci. 9, 1–13. doi: 10.3389/fpls.2018.00900
Ren, A. T., Abbott, L. K., Chen, Y., Xiong, Y. C., and Mickan, B. S. (2020). Nutrient recovery from anaerobic digestion of food waste: impacts of digestate on plant growth and rhizosphere bacterial community composition and potential function in ryegrass. Biol. Fertil. Soils 56, 973–989. doi: 10.1007/s00374-020-01477-6
Risberg, K., Cederlund, H., Pell, M., Arthurson, V., and Schnürer, A. (2017). Comparative characterization of digestate versus pig slurry and cow manure—chemical composition and effects on soil microbial activity. Waste Manag. 61, 529–538. doi: 10.1016/j.wasman.2016.12.016
Riva, C., Orzi, V., Carozzi, M., Acutis, M., Boccasile, G., Lonati, S., et al. (2016). Short-term experiments in using digestate products as substitutes for mineral (N) fertilizer: agronomic performance, odours, and ammonia emission impacts. Sci. Total Environ. 547, 206–214. doi: 10.1016/j.scitotenv.2015.12.156
Robertson, G. P., and Groffman, P. M. (2007). “Nitrogen transformations” in Soil Microbiology, Ecology and Biochemistry. ed. P. A. Eldor. 4th ed (London: Elsevier), 421–446.
Różyło, K., and Bohacz, J. (2020). Microbial and enzyme analysis of soil after the agricultural utilization of biogas digestate and mineral mining waste. Int. J. Environ. Sci. Technol. 17, 1051–1062. doi: 10.1007/s13762-019-02522-0
Sánchez-Rodríguez, A. R., Carswell, A. M., Shaw, R., Hunt, J., Saunders, K., Cotton, J., et al. (2018). Advanced processing of food waste based Digestate for mitigating nitrogen losses in a winter wheat crop. Front Sustain Food Syst 2, 1–14. doi: 10.3389/fsufs.2018.00035
Schmidt, M. W. I., Noack, A. G., and Osmond, G. (2000). Black carbon in soil and sediments: analysis, distribution, implications, and current challenges. Glob Geochem Cycles 14, 777–793. doi: 10.1029/1999GB001208
Šimon, T., Kunzová, E., and Friedlová, M. (2015). The effect of digestate, cattle slurry and mineral fertilization on the winter wheat yield and soil quality parameters. Plant Soil Environ. 61, 522–527. doi: 10.17221/530/2015-PSE
Sinsabaugh, R. L., Turner, B. L., Talbot, J. M., Waring, B. G., Powers, J. S., Kuske, C. R., et al. (2016). Stoichiometry of microbial carbon use efficiency in soils. Ecological monographs 86, 172–189. doi: 10.1890/15-2110.1
Sun, L., Xia, Z., Sang, C., Wang, X., Peng, B., Wang, C., et al. (2019). Soil resource status affects the responses of nitrogen processes to changes in temperature and moisture. Biol. Fertil. Soils 55, 629–641. doi: 10.1007/s00374-019-01379-2
Tahboub, M. B., Lindemann, W. C., and Murray, L. (2007). Nutrient availability in soil amended with pecan wood chips. HortScience 42, 339–343. doi: 10.21273/hortsci.42.2.339
Tiwary, A., Williams, I. D., Pant, D. C., and Kishore, V. V. N. (2015). Assessment and mitigation of the environmental burdens to air from land applied food-based digestate. Environ. Pollut. 203, 262–270. doi: 10.1016/j.envpol.2015.02.001
Vance, E. D., Brooks, P. C., and Jenkinson, D. S. (1987). An extraction method for measuring soil microbial biomass C. Soil Biol. Biochem. 19, 703–707. doi: 10.1016/0038-0717(87)90052-6
Wagner, C., Nyord, T., Vestergaard, A. V., Hafner, S. D., and Pacholski, A. S. (2021). Acidification effects on in situ Ammonia emissions and cereal yields depending on slurry type and application method acidification effects on in situ Ammonia emissions and cereal yields depending on slurry type and application method. Agriculture 11:1053. doi: 10.3390/agriculture11111053
Walsh, J. J., Jones, D. L., Chadwick, D. R., and Williams, A. P. (2018). Repeated application of anaerobic digestate, undigested cattle slurry and inorganic fertilizer N: impacts on pasture yield and quality. Grass Forage Sci. 73, 758–763. doi: 10.1111/gfs.12354
Walsh, J. J., Rousk, J., Edwards-Jones, G., Jones, D. L., and Williams, A. P. (2012). Fungal and bacterial growth following the application of slurry and anaerobic digestate of livestock manure to temperate pasture soils. Biol. Fertil. Soils 48, 889–897. doi: 10.1007/s00374-012-0681-6
Wang, Y., Chikamatsu, S., Gegen, T., Sawada, K., Toyota, K., Riya, S., et al. (2019). Application of biogas Digestate with Rice straw mitigates nitrate leaching potential and suppresses root-knot nematode (Meloidogyne incognita). Agronomy 9:227. doi: 10.3390/agronomy9050227
Wang, J., Xiong, Z., and Kuzyakov, Y. (2016). Biochar stability in soil: Meta-analysis of decomposition and priming effects. GCB Bioenergy 8, 512–523. doi: 10.1111/gcbb.12266
White, D., Stair, J., and Ringelberg, D. (1996). Quantitative comparisons ofin situ microbial biodiversity by signature biomarker analysis. J. Ind. Microbiol. Biotechnol. 17, 185–196. doi: 10.1007/bf01574692
Widmer, P., Brookes, P. C., and Parry, L. C. (1989). Microbial biomass nitrogen measurements in soils containing large amounts of inorganic nitrogen. Soil Biol. Biochem. 21, 865–867. doi: 10.1016/0038-0717(89)90183-1
Wilcox, R. (2003). “One-way ANOVA” in Applying contemporary statistical techniques. ed. R. Wilcox (Elsevier Science, San Diego)
Zelles, L. (1997). Phospholipid fatty acid profiles in selected members of soil microbial communities. Chemosphere 35, 275–294. doi: 10.1016/S0045-6535(97)00155-0
Keywords: biogas residue, nitrogen immobilisation, microbial community, glycerol, straw, woodchip, biochar
Citation: van Midden C, Harris J, Shaw L, Sizmur T, Morgan H and Pawlett M (2024) Immobilisation of anaerobic digestate supplied nitrogen into soil microbial biomass is dependent on lability of high organic carbon materials additives. Front. Sustain. Food Syst. 8:1356469. doi: 10.3389/fsufs.2024.1356469
Edited by:
Tom Misselbrook, Rothamsted Research, United KingdomReviewed by:
Mesfin Tsegaye Gebremikael, Ghent University, BelgiumAna I. M. Natalio, Harper Adams University, United Kingdom
Copyright © 2024 van Midden, Harris, Shaw, Sizmur, Morgan and Pawlett. This is an open-access article distributed under the terms of the Creative Commons Attribution License (CC BY). The use, distribution or reproduction in other forums is permitted, provided the original author(s) and the copyright owner(s) are credited and that the original publication in this journal is cited, in accordance with accepted academic practice. No use, distribution or reproduction is permitted which does not comply with these terms.
*Correspondence: Christina van Midden, Y2hyaXN0aW5hLnZhbi1taWRkZW5AY3JhbmZpZWxkLmFjLnVr