- 1Faculty of Agricultural Sciences, Center for Post-Harvest Studies, University of Chile, Santiago, Chile
- 2Agriculture Production Department, Faculty of Agricultural Sciences, University of Chile, Santiago, Chile
Microgreens are vegetable or edible herb shoots harvested in the early stages of development. They have an important number of bioactive compounds and add color, texture, and flavor to dishes and salads. Given their benefits, small size, and high market prices, they can grow in indoor systems, where light is determinant. This study aimed to evaluate the effect of different light intensities on agronomic characteristics, color, chlorophylls and carotenoids content, and antioxidant activity represented by total phenolic content (TPC), eliminate, and antioxidant capacity (AC) in four Brassicaceae species in two colors (green and red). The experiment was conducted in a controlled light-emitting diode (LED) environment growth chamber (day/night temperatures of 25/20 ± 1.2°C, 16 h photoperiod, and 79 ± 2% relative humidity). Three light intensities were used for microgreen growth with the same LED light spectrum: low (120 ± 5.1 μmol m−2 s−1), medium (160 ± 3.6 μmol m−2 s−1), and high (210 ± 5.9 μmol m−2 s−1). Eight g of the seeds of green and red cultivars of cabbage, kale, mizuna, and mustard were sown in a plastic tray (64 cm x 35 cm x 6 cm) with a mixture of peat and perlite (1:2 = v: v). Overall, the high intensity increased dry matter percentage and dry weight, except in green and red kale and green cabbage cultivars. In contrast, low intensity promoted a larger hypocotyl in all species than with high intensity; moreover, it enhanced the cotyledon area in green and red mizuna. Cabbage, kale, and mustard green cultivars were greener under medium intensity, whereas the low intensity enhanced the purple color of mizuna. In addition, chlorophyll a and b increased under low intensity in most species except the red kale and mustard cultivars. The high intensity raises the antioxidant activity, promoting a higher TPC and AC. The findings revealed that the light intensity generated variations in agronomic characteristics, color, chlorophyll content, and antioxidant activity of Brassicaceae microgreens, and the changes were based on the specific species and cultivars.
1 Introduction
No formal definition of microgreens has been established, and there are almost as many definitions as there are articles written about these microgreens or micro-vegetables (Verlinden, 2020). Mir et al. (2017) described microgreens as a new class of edible vegetables harvested when the cotyledons have fully expanded and before true leaves have emerged. On the other hand, Toscano et al. (2021) described microgreens as young, tender greens of edible plants harvested at the first true leaf stage, and Verlinden (2020) defines them as germinated seedlings with fully developed and non-senescent cotyledons and the emergence and early development of one to two true leaves.
Microgreens are fast-growing; they can be harvested when plants are no taller than 5–10 cm (Toscano et al., 2021), taking about 1–3 weeks after seeding (Xiao et al., 2014; Di Gioia et al., 2016; Toscano et al., 2021). Compared to their adult counterparts or their seeds, microgreens have a significant number and diversity of phytochemicals with health functional properties (Kou et al., 2014; Brazaitytė et al., 2015). In the culinary field, microgreens have double function as food and garnish on plates (Toscano et al., 2021) and are frequently used to add variety to diets (Verlinden, 2020). They incorporate color, aroma, texture, and flavor, improving the sensory quality of the dishes (Xiao et al., 2012; Toscano et al., 2021). Due to these characteristics, microgreens have been incorporated into gourmet cuisine, where they have been described as “confetti” and as the “lingerie of the culinary” world (Riggio et al., 2019; Verlinden, 2020). Their popularity has increased drastically of late; they can be found even in casual dining establishments (Verlinden, 2020). In this light, the Knowledge Sourcing Intelligence (2020) indicated that the global microgreens market size reached US$ 1,522 million in 2022 and is expected to reach US$ 2,470 million by 2028, exhibiting a compound annual growth rate (CAGR) of 8.4% during 2022–2028.
Many vegetable and herb species can be grown as microgreens. Most commercial species are aromatic herbs or vegetables from the Brassicaceae family (such as broccoli, cabbage, cauliflower, arugula, radish, mizuna, and kale). The Brassicaceae family stands out among others because of its nutritional and functional benefits (Di Gioia et al., 2016; Toscano et al., 2021). They can be considered excellent dietary sources because they are good sources of both macro and micro elements and accumulate high concentrations of ascorbic acid, carotenoids, tocopherols, phenolic compounds, and glucosinolates, bioactive compounds associated with a reduction in the incidence of cancer, cardiovascular diseases, and other degenerative diseases (Xiao et al., 2019).
Microgreens can be grown in various settings, but most production occurs in greenhouses (Di Gioia et al., 2016). However, due to their rapid growth, small size, and high selling price, they are a good candidate for vertical farming and growth in enclosure-controlled environments without daylight (Verlinden, 2020). In these conditions, microgreens can be produced throughout the year (Toscano et al., 2021).
Light supplementation is a significant factor in the growth and development of microgreens within controlled environments. Light plays a fundamental role as one of the primary environmental factors governing plant growth and development (Huché-Thélier et al., 2016), serves as the energy source for and triggers various physiological responses in plants (Hasan et al., 2017). Light can be defined based on three main variables: intensity, understood as the amount of light that falls on a surface in a given time, measured in μmol m−2 s−1 (micromoles per square meter per second); photoperiod, or the number of hours of light and darkness, frequently expressed in hours; and spectrum, which describes the composition of colors or wavelengths (λ) of the light source and is expressed in nm (nanometers) (Son and Oh, 2015). In this indoor context, light-emitting diode (LED) lights have been applied for agricultural purposes and microgreen cultivation (Alrifai et al., 2019), providing tools to study the different light components separately. Some researchers have even assessed how LED light affects yield (Brazaitytė et al., 2021; Toscano et al., 2021; Demir et al., 2023), morphology (Brazaitytė et al., 2021), pigment content (Vaštakaitė et al., 2017; Brazaitytė et al., 2021; Toscano et al., 2021), sugar content (Gao et al., 2021; Toscano et al., 2021), macro and micronutrient content (Brazaitytė et al., 2021), and antioxidant capacity (Vaštakaitė et al., 2017; Toscano et al., 2021). To fully understand areas concerning plant physiology, yield optimization, and the concentration of functional compounds, among other relevant factors on different species and cultivars, each light factor must be studied separately.
Within the light parameters, spectrum and intensity play an important role in the vegetable quality. In general, the blue and red spectral regions are the most used for indoor cultivation (Brazaitytė et al., 2021) because both spectral regions are more efficient in driving the photosynthetic process (Dou et al., 2017; Rahman et al., 2021). Blue light allows the maximum absorption of chlorophylls, promoting photosynthesis, although blue is less efficient than red light in leading the photosynthetic process (Rahman et al., 2021). Nevertheless, blue light induces stomatal opening and CO2 fixation (Brazaitytė et al., 2021). Furthermore, blue light can increase the hypocotyl length and fresh weight of mustard and kale microgreens (Brazaitytė et al., 2021), and antioxidant capacity and total ascorbic acid concentration in parsley microgreens (Carillo et al., 2022). Moreover, the increase in the blue spectral region can ameliorate the accumulation of anthocyanins and carotenoids (Brazaitytė et al., 2021). On the other hand, the red spectral region can increase yield (Carillo et al., 2022), hypocotyl length (Brazaitytė et al., 2021; Carillo et al., 2022), and cotyledon length (Carillo et al., 2022) of different microgreens species. However, it must be considered that other regions of the spectrum, such as far-red and green, are useful in improving the performance of certain microgreen species (Brazaitytė et al., 2015; Gerovac et al., 2016). For example, the use of light with 23% blue, 75% red, and 2% far-red improved the performance of beet microgreens under low and medium intensities (120 and 180 μmol m−2 s−1) and short photoperiods of 12 h of light (Hernández-Adasme et al., 2023). In parsley microgreens, the light with 45% blue, 10% green, and 45% red improved dry weight percentage, leaf length, and leaf width vs. blue or red monochromatic light (Carillo et al., 2022). Likewise, Gerovac et al. (2016) mentioned that the addition of 18% green or 7% infrared fraction to the blue-red spectrum promoted hypocotyl length in kohlrabi, mizuna, and mustard microgreens at low intensity (105 μmol m−2 s−1) and leaf area of the same microgreens, regardless of intensity, against a blue-red spectrum light (Gerovac et al., 2016). Thus, a more complete spectrum that includes a greater proportion of the blue and red spectral regions may improve the performance of microgreens.
Conversely, intensity can control plant growth and phytochemical accumulation, making it a productive, manageable, and eco-friendly way to provide high-quality products (Gao et al., 2021). According to Brazaitytė et al. (2015, 2021), a normal or standard intensity for the growth of Brassicaceae microgreens is around 220 μmol m−2 s−1. Lower intensities, such as 105 μmol m−2 s−1, can promote hypocotyl length and leaf area of kohlrabi, mizuna, and mustard microgreens or maintain similar fresh weight of kohlrabi microgreens compared to intensities of 210 and 315 μmol m−2 s−1 (Gerovac et al., 2016). Meanwhile, intensities between 120 and 160 μmol m−2 s−1 can led to a greater accumulation of total betalains in beet microgreens compared to 220 μmol m−2 s−1 (Hernández-Adasme et al., 2023). It is important to consider that higher intensity is correlated with higher electricity consumption (Cui et al., 2021). Therefore, low intensities may eventually decrease the cost of electricity used for microgreen production under indoor conditions (Gao et al., 2021). However, it is essential to ascertain the lowest intensity needed to successfully grow high-quality microgreens.
To determine the quality of microgreen morphology, quality indicators have been suggested, where hypocotyl length was reported as a feature that could be useful in verifying quality (Vaštakaitė et al., 2015; Vaštakaitė and Viršilė, 2015). Antioxidants have also been featured as a determinant of quality (Sun et al., 2013; Ebert, 2022). However, to date, no visual, physical, physiological, or biochemical quality indices have been created (Verlinden, 2020).
This study aimed to determine the minimum intensity of light to be used in a vertical farm system for the correct cultivation of microgreens of different species of Brassicaceae, including green and red cultivars.
2 Methodology
2.1 Microgreen growth conditions
Four species of Brassicaceae: cabbage, kale, mizuna, and mustard in green and red cultivars were sown in a 64 cm × 35 cm × 7 cm high tray filled with a moist mixture of peat DSM2 W R0632 (Kekkilä, Vantaa, Finland) and perlite A6 (Harborlite, Santiago, Chile) in a 1:2 ratio (v/v) and grown in a vertical farm system. Eight g of seeds (Ortis Quality Seeds, Italy) were scatter-sown on the substrate, covered with a small layer of the same substrate, and put at 20°C into darkness for 2 days until radicle emergence. Then, the tray was put in an adapted refrigerated chamber where three shelves (170*180*45 cm) with three levels were placed. Each level was conditioned with two LED lamp tubes model GL-TL040P12BF-01 (Lighting Xiamen Technology, Xiamen, China) with a spectrum of 5% UV-A, 19% blue, 26% green, 44% red, and 6% far-red, as shown in Figure 1. The intensity of the light treatment was defined by the distance at which the plants were from the source of illumination in low, medium, and high (Table 1). The spectrum and intensities were recorded with an UPRtek MK350S LED meter (Miaoli Country, Taiwan). The photoperiod was 16/8 h, the chamber temperature was fixed at 20 ± 2°C, and relative humidity was 75–80%. These growing conditions were maintained until harvest, 14 days after sowing. The same procedure was repeated for each species.
2.2 Microgreen growth parameters
2.2.1 Fresh and dry weights (per m2)
Three trays per light intensity were fully harvested to determine total fresh weight (FW) per m2 using a precision analytical balance (AS 100/C/2, RADWAG, Radom, Poland). For dry weight (DW) measurements, a quarter of each tray was dried in a forced air oven (LDO-150F, Labtech, Gagok-ri, Korea) at 70°C until a constant mass was achieved. Both FM and DW values were recorded in grams (g). For dry matter content (DMC), the percentage of DW was calculated using the DW:FW ratio and expressed as a percentage.
2.2.2 Cotyledon thickness
Thirty cotyledons per light repetition (n = 90) were considered for cotyledon thickness. A micrometer with a 0.01 mm precision (JPT491, Mitutoyo, Japan) was used and expressed in millimeters (mm).
2.2.3 Microgreen length
A 0–150 mm digital Vernier was used to determine microgreen length. Thirty microgreens per light repetition (n = 90) were considered and expressed in millimeters (mm).
2.2.4 Leaf area
Cotyledon leaf area was determined by image analysis of 20 cotyledons per replicated (n = 60). Images were analyzed using the ImageJ software, an open-source image processing program designed by the National Institutes of Health (NIH) (Bethesda, Maryland, United States). The specific version used was v. 1.51j8 (Schneider et al., 2012).
2.3 Color properties
Color was assessed as a quality attribute of the cotyledons. To conduct these measurements, multiple cotyledons were affixed onto a strip to cover the objective of the device entirely. A compact tristimulus spectrophotometer (CM-2500d, Minolta, Japan) was used to record 10 readings for the three biological replicates (n = 30). The data underwent analysis using the SpectraMagic NX color data software and were subsequently presented in terms of luminosity (L), chroma (C*), and hue angle (Hue) (McGuire, 1992).
2.4 Pigment content determinations: chlorophyll a, b, and carotenoids
The amount of 0.1 g of dry microgreen was homogenized in a mortar and pestle with 2 mL of methanol (70%), and the resulting homogenized mixture was centrifuged at 7,300 g for 15 min at 4°C (Hermle Labortechnik, Z326K, Wehingen, Germany). Three samples per biological replicate (n = 9) were taken. The supernatant was set aside, and 0.5 mL was combined with 4.5 mL of methanol. This diluted solution was used to determine chlorophyll a, chlorophyll b, and total carotenoids using a UV/VIS spectrophotometer (Asys UVM 340, Biochrom, Cambridge, United Kingdom). The concentrations of chlorophyll a, chlorophyll b, and total carotenoids were expressed in μg*g−1 FW and determined using the equations proposed by Lichtenthaler and Buschmann (2001) for methanol extractions.
2.5 Extraction of antioxidant compounds
Fresh microgreens were harvested, rapidly frozen with liquid nitrogen, and stored at −80°C until lyophilization. Upon lyophilization, samples were processed into a fine powder using an electric mill. An exact amount of powder was mixed with methanol: water (MeOH: H2O) solution in a 70:30 ratio (Flores et al., 2022). The mixture underwent agitation through vortexing and ultrasonic treatment. Subsequently, the mixtures were centrifuged at 4,180 g for 10 min at 4°C (Hermle Labortechnik, Z326K, Wehingen, Germany). The resulting supernatant was filtered using a sterile syringe filter (0.45 μm PVDF membrane). Then, the filtered extract was transferred to amber tubes and stored at −20°C until analysis for total phenolic content and antioxidant activity.
2.5.1 Total phenolic content
The determination of total phenolic content (TPC) was conducted according to the procedure described by Ainsworth and Gillespie (2007) with some modifications (Flores et al., 2022). The hydro-methanolic extract obtained in the previous steps was mixed with 10% Folin–Ciocalteu reagent, followed by a 0.7 mol L−1 Na2CO3 solution. The reaction was left to react for 2 h, and 765 nm absorbance was recorded using a multi-plate spectrophotometer (Asys UVM 340, Biochrom, Cambridge, United Kingdom). A control mix without a sample was established for baseline correction purposes. The quantification of TPC was accomplished through a gallic acid calibration curve and was expressed in milligrams of gallic acid equivalent (GAE) per 100 g of fresh weight (FW). The final values were calculated as the average of nine data points, comprising three samples from each treatment replication.
2.5.2 Antioxidant capacity
Two distinct assays were conducted for the antioxidant capacity (AC) measurement. The ferric reducing antioxidant power (FRAP) and the 2,2-diphenyl-1-picrylhydrazyl (DPPH). A modified version of the method reported by Benzie and Strain (1996) was used for the FRAP assay. The FRAP reagent, comprised of 0.3 mol L−1 acetate buffer (pH 3.6), 0.01 mol L−1 2,4,6-Tripyridyl-s-triazine (TPTZ) dissolved in 0.04 mL L−1 HCl, and 0.02 mol L−1 FeCl3 6H2O, was prepared in a 10:1:1 ratio. The mixture was then heated at 37°C for 10 min. Subsequently, a sample aliquot was combined with the preheated FRAP reagent. The reaction mixture was transferred to a 96-well plate, and 593 nm absorbance was monitored with a multi-plate spectrophotometer (Asys UVM 340, Biochrom, Cambridge, United Kingdom) until it reached a stable reading.
The 2,2-diphenyl-1-picrylhydrazyl (DPPH) reagent was used for free radical scavenging activity, following the methodology that Gupta and Prakash (2009) described. In each reaction, an extract aliquot was mixed with a solution of 0.2 × 10−3 mol L−1 DPPH, and the absorbance at 517 nm was recorded with a multi-plate spectrophotometer (Asys UVM 340, Biochrom, Cambridge, United Kingdom) until it stabilized.
To account for baseline variations, a control lacking samples was prepared and utilized for correction in both AC determination methods. The equivalent antioxidant capacity was determined using a calibration curve established with Trolox (Merck KGaA, Darmstadt, Germany). The resultant AC values were expressed in milligrams of Trolox equivalent (Trolox eq) per 100 g of fresh weight (mg 100 g−1 FW).
2.6 Experimental design and statistical analysis
The experiment was arranged in three blocks with a divided plot design. Two factors were considered: the main plot was the light intensity of high (210 μmol m−2 s−1), medium (160 μmol m−2 s−1), or low (120 μmol m−2 s−1), and the subplot was the cultivar of green or red. Each block represented one replicate. So, three trays for each cultivar were considered for each light intensity. An analysis of variance (ANOVA) and a multiple range test using the LSD (Least Significant Difference) by Fisher were conducted. The first analysis aimed to compare the average values of each variable for both green and red cultivar colors, while the second analysis focused on assessing the significance of the differences. A significance level of p < 0.05 was employed to determine significant differences (Di Rienzo et al., 2017).
3 Results
3.1 Agronomic characteristics
3.1.1 Fresh weight
The yield on a fresh weight basis varied among species and cultivars (green or red). Green cabbage yield showed no significant differences among treatments; meanwhile, in red cabbage, the low intensity increased the yield compared to medium and high intensities by 17.9 and 5.7%, respectively (Table 2). In kale, significant differences were observed only for the red cultivar; specifically, the low and medium intensities raised the yield compared to the high intensity by 9.0 and 9.9%, respectively (Table 2). In mizuna, the yield did not show significant differences for the green and red cultivars (Table 2). On the other hand, in green mustard, the yield was significantly higher under medium and low intensities compared to high intensity by 26.6 and 16.1%, respectively. By contrast, high intensity produced the maximum yield of 46.7% for red mustard and 42.2% for low intensity, both of which were greater than the yield attained with medium intensity (Table 2).
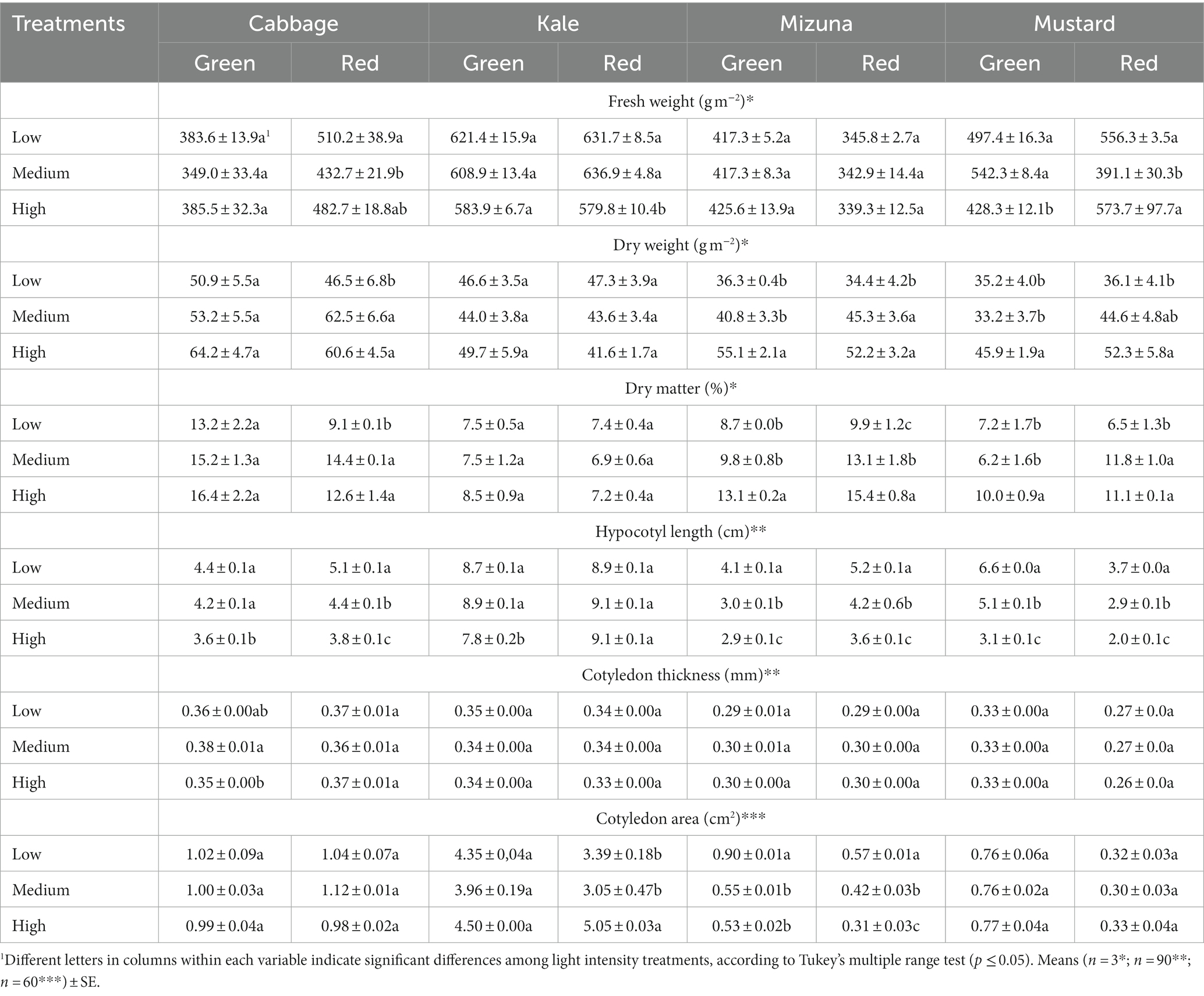
Table 2. Agronomic characteristics of Brassicaceae microgreens grown under low (120 ± 5.06 μmol m−2 s−1), medium (160 ± 3.60 μmol m−2 s−1), and high (210 ± 5.91 μmol m−2 s−1) intensities.
3.1.2 Dry weight
Green cabbage and kale showed no significant differences among treatments for dry weight (Table 2). In red cabbage, low intensity diminished the yield compared to medium and high intensities by 25.6 and 23.3%, respectively. In red kale, no significant differences were observed (Table 2). In green mizuna and mustard, high intensity promoted a higher yield than medium and low intensities; in the red cultivars of both species, dry weight decreased significantly at low intensity by about 30% (Table 2).
3.1.3 Dry matter content
Dry matter content showed similar results to dry weight. Green cabbage and kale cultivars showed no significant differences among treatments. On the other hand, low intensity significantly decreased DMC in red cabbage compared to medium and high intensities by 36.8 and 27.8%, respectively. Meanwhile, in red kale, no significant differences among treatments were found (Table 2). In green mizuna and mustard cultivars, high intensity promoted a higher DMC than low and medium intensities. In particular, DMC in green mizuna under high intensity was 50.6 and 33.7% higher than in low and medium intensities, respectively (Table 2). In green mustard, DMC under high intensity was 38.9 and 61.0% higher than in low and medium intensities, respectively (Table 2). In red mizuna and mustard cultivars, low intensity had a significant negative effect on DMC. The low intensity decreased the DMC in red mizuna compared to high and medium intensities by 35.7 and 24.4%, respectively. In addition, medium intensity significantly decreased DMC in red mizuna compared to high intensity by 14.9%. In red mustard, the low intensity diminished DMC by about 40% compared to the others intensities (Table 2).
3.1.4 Hypocotyl length
Overall, the low intensity promoted a greater length of the microgreens in all species compared to the high intensity, except in red kale (Table 2). For instance, the hypocotyl length in green and red cabbages at the low intensity was 22.2 and 34.2% greater than at the highest intensity, respectively. In green kale, the low intensity stimulated the hypocotyl length by 11.5% compared to the high intensity. Meanwhile, green and red mizuna showed increased hypocotyl length of over 40% under low compared to high-intensity. Among all species, mustard hypocotyl length, independent of cultivar, had the greatest difference between low and high intensity. Specifically, hypocotyl length was 112.9 and 85% greater in green and red mustard, respectively, when comparing low and high intensities (Table 2).
3.1.5 Cotyledon thickness
Cotyledon thickness generally did not show significant differences among light intensity treatments, except for green cabbage, where medium intensity significantly increased cotyledon thickness compared to high intensity by 8.6% (Table 2).
3.1.6 Cotyledon area
Cotyledon area showed significant differences only in green and red mizuna and red kale (Table 2). Particularly, the low intensity increased the cotyledon area of green mizuna compared to medium and high intensities by 63.7 and 69.8%, respectively. In red mizuna, low intensity promoted higher cotyledon area than medium and high intensity by 35.7 and 83.9%, respectively. In addition, medium intensity significantly increased cotyledon area compared to high intensity by 35.5%. On the other hand, high intensity promoted a larger cotyledon area of red kale compared to low and medium intensities by 49.0 and 65.6%, respectively (Table 2).
3.1.7 Cotyledon color
Color parameters, such as lightness, chroma, and hue, showed significant differences among intensity treatments for the different Brassicaceae species and cultivars, except for red mustard (Table 3). In general, green cabbage microgreens grown under medium intensity were greener than microgreens grown under low and high intensity (Table 3; Figure 2). Meanwhile, the low intensity promoted a lighter color of the microgreens in red cabbage (high lightness value) compared to medium and high intensities (Table 3; Figure 2). In green kale, medium intensity significantly increased the hue, turning it greener than the low and high intensities. In contrast, in red kale, the color remained green and enhanced under low intensity compared to medium and high intensities (Table 3; Figure 2). In green mizuna, microgreens grown under high intensity were greener than those grown under low and medium intensities. In contrast, in red mizuna, the low intensity increased the blue color of the cotyledons compared to medium and high intensities, which enhanced the purple color (Table 3; Figure 3). Finally, in green mustard, both medium and high intensities significantly increased the hue, which meant that microgreens were greener than microgreens grown under low intensity. In red mustard, the microgreens had a color closer to reddish, and no significant differences were observed among the intensity treatments (Table 3; Figure 3).
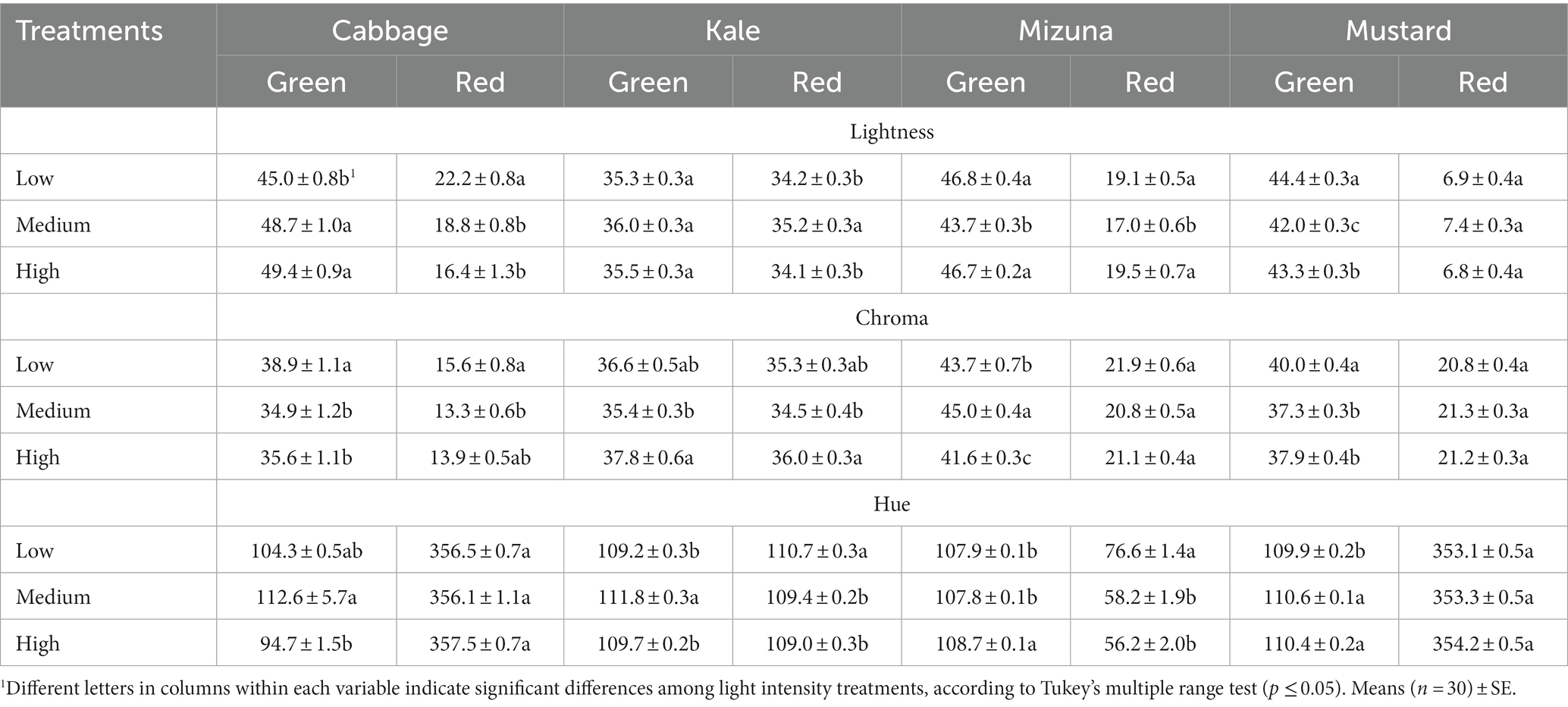
Table 3. Cotyledon color of Brassicaceae microgreens grown under low (120 ± 5.06 μmol m−2 s−1), medium (160 ± 3.60 μmol m−2 s−1), and high (210 ± 5.91 μmol m−2 s−1) intensities.
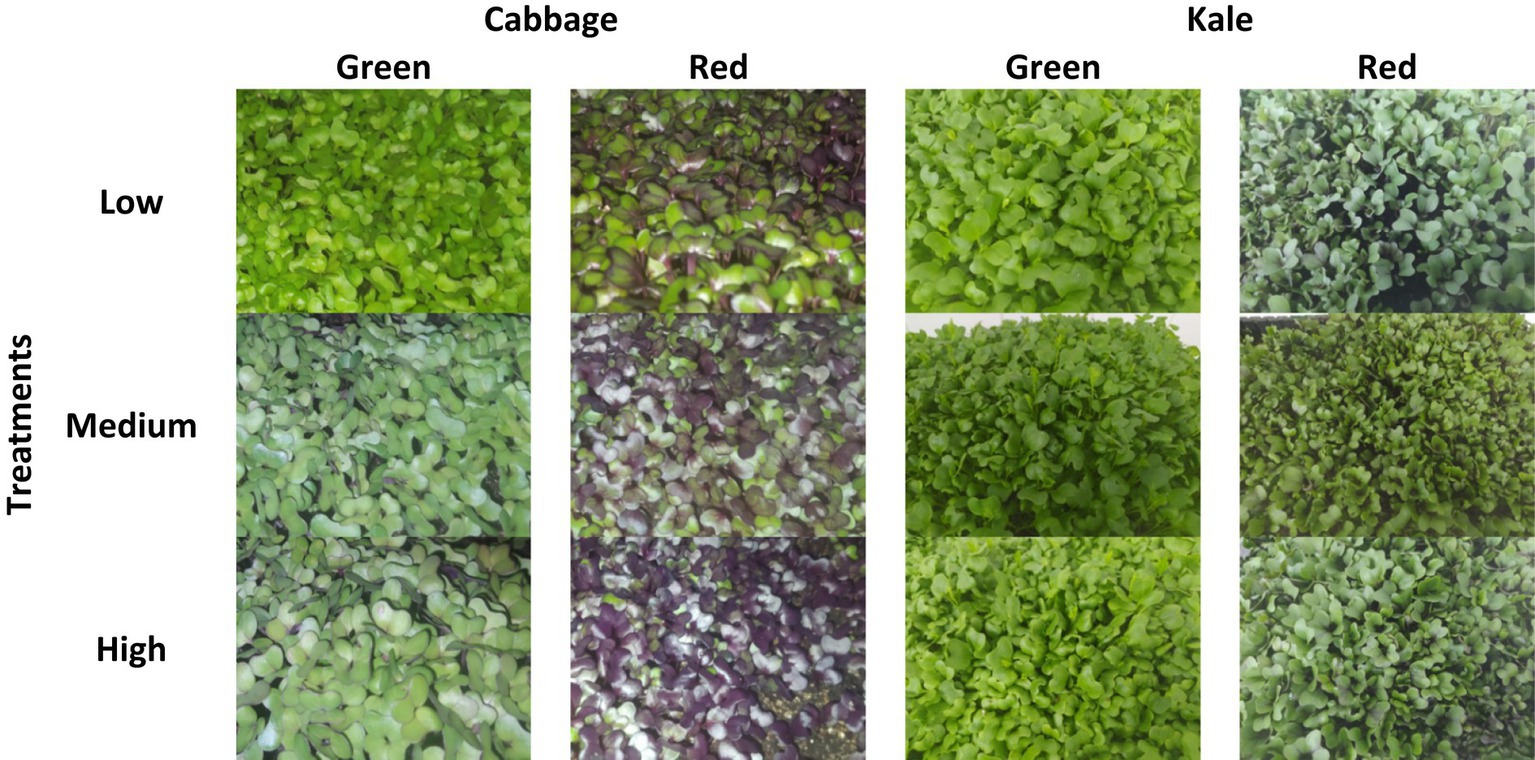
Figure 2. Green and red cabbage and kale microgreens at harvest previously grown under low (120 ± 5.06 μmol m−2 s−1), medium (160 ± 3.60 μmol m−2 s−1), and high (210 ± 5.91 μmol m−2 s−1) intensities.
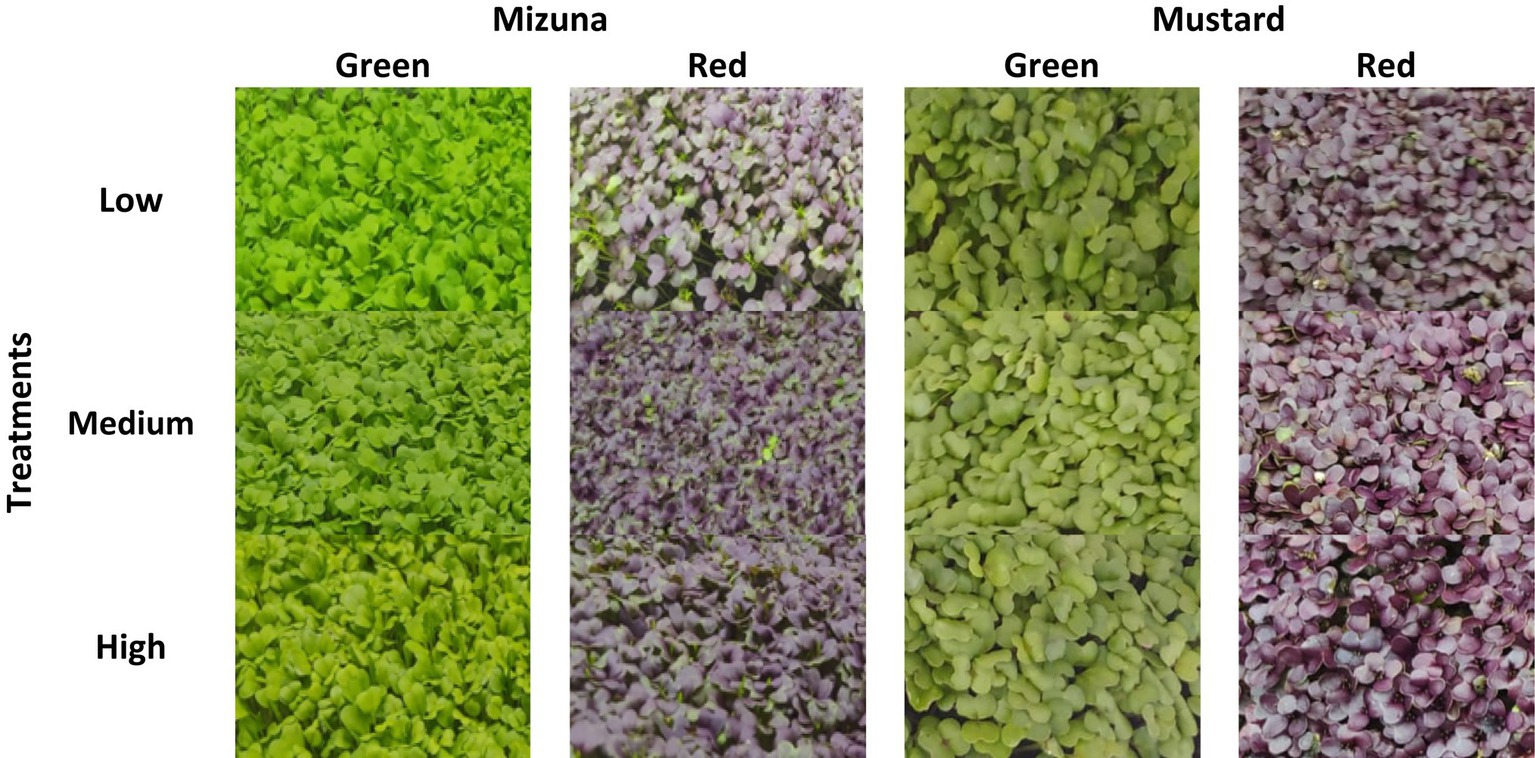
Figure 3. Green and red mizuna and mustard microgreens at harvest previously grown under low (120 ± 5.06 μmol m−2 s−1), medium (160 ± 3.60 μmol m−2 s−1), and high (210 ± 5.91 μmol m−2 s−1) intensities.
3.2 Pigment contents
3.2.1 Chlorophyll a
Brassicaceae microgreen chlorophyll a content varied between 55.6 and 212.2 μg g−1 FW, and overall, the low intensity promoted a higher content in most of the species than the other intensities, except for the red kale and mustard cultivars (Table 4). Specifically, low intensity significantly increased the chlorophyll a content in green cabbage compared to medium and high intensities by 38.7 and 65.7%, respectively. Similar results were observed in red cabbage; were low intensity stimulated the chlorophyll a content compared to medium and high intensities by 27.6 and 33.0%, respectively. In green kale, low intensity also significantly increased the chlorophyll a content compared to medium and high intensities by 23.8 and 45.9%, respectively. In green mizuna, the chlorophyll a content at low intensity reached 12.6 and 64.7% more than at medium and high intensities, respectively. On the other hand, red mizuna grown in low and medium intensities showed a similar chlorophyll a content and was significantly higher than high intensity by 29%. For green mustard, the chlorophyll a content was significantly enhanced under low intensity compared to medium and high intensities by 16.7 and 27.9%, respectively (Table 4).
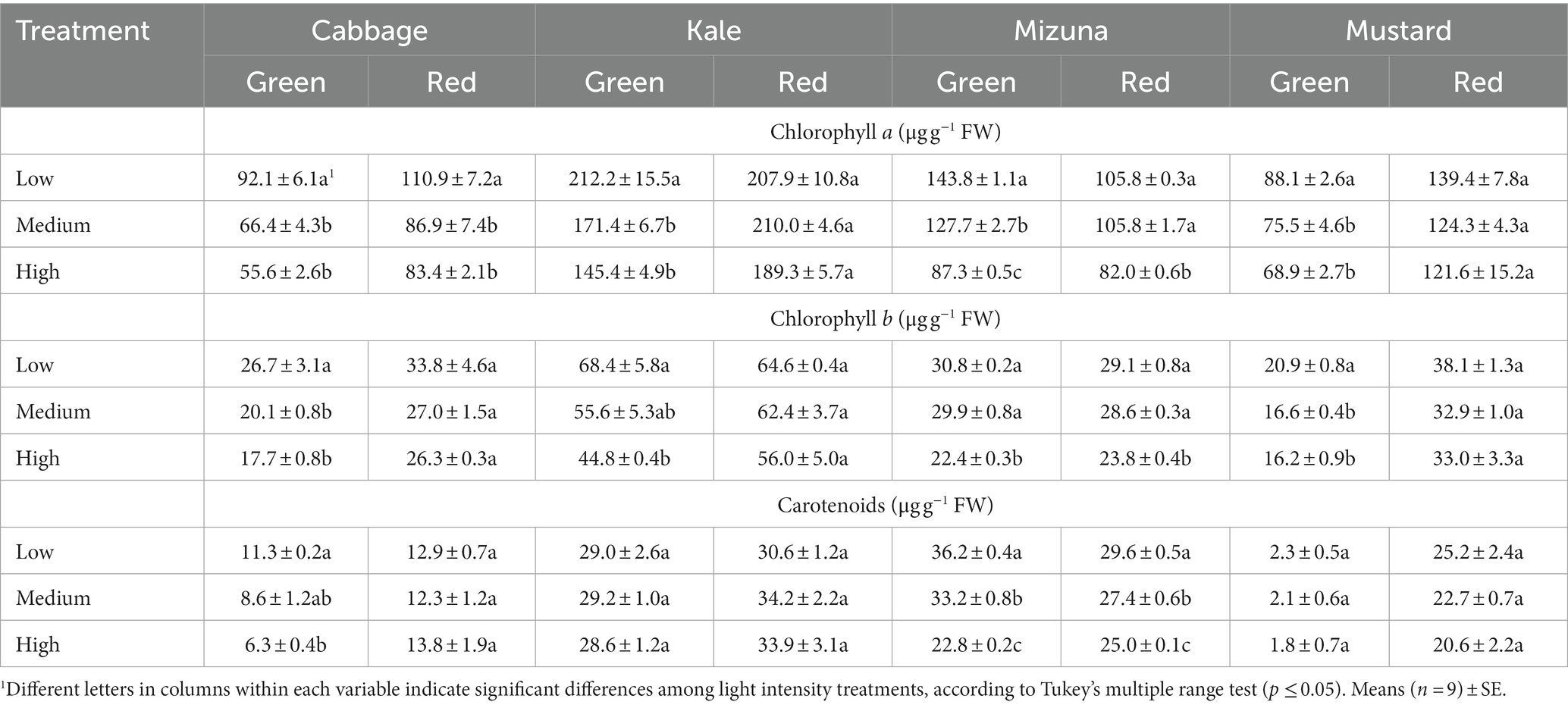
Table 4. Pigment contents of Brassicaceae microgreens grown under low (120 ± 5.06 μmol m−2 s−1), medium (160 ± 3.60 μmol m−2 s−1), and high (210 ± 5.91 μmol m−2 s−1) intensities.
3.2.2 Chlorophyll b
Chlorophyll b content showed higher values in all the microgreens of the species grown under the lowest intensity, but significant differences were observed only in the green cultivars and red mizuna (Table 4). Green cabbage grown under low intensity had higher chlorophyll b content than medium and high intensities by 32.8 and 50.9%, respectively. Meanwhile, in green kale, the content of chlorophyll b increased 52.7% when comparing low and high intensity. In green mizuna, both low and medium intensities significantly enhanced chlorophyll b content compared to high intensity by 37.5 and 33.5%, respectively. Similarly, the chlorophyll b content significantly increased by 22.3 and 20.2% in red mizuna cultivated in low and medium intensities compared to high intensity, respectively. The low intensity also improved chlorophyll b content in green mustard. In particular, the lowest intensity significantly increased chlorophyll b content by 25.9 and 29.0%, respectively, compared to medium and high intensities (Table 4).
3.2.3 Carotenoids
Carotenoid content was significantly modified only in green cabbage and both cultivars of mizuna (Table 4). In green cabbage, carotenoid content significantly increased under low intensity compared to high intensity by 79.4%. Meanwhile, the low intensity significantly improved the carotenoid contents in both mizuna cultivars. Specifically, the low intensity in green mizuna increased carotenoid content compared to the medium and high intensities by 9 and 58.8%, respectively. Likewise, the medium intensity showed a significantly higher content of carotenoids than the high intensity by 45.6%. In red mizuna, carotenoid content under low intensity was significantly enhanced compared to medium and high intensities by 8.0 and 18.4%. In addition, medium intensity also significantly increased carotenoid content compared to high intensity by 9.6% (Table 4).
3.3 Antioxidant compounds
3.3.1 Total phenol content
Total phenol content in Brassicaceae microgreens varied between 51.5 and 248.5 mg GAE 100 g−1 FW. Among the green cultivars, green mustard was the species with the lowest TPC values (51.5–90.3 mg GAE 100 g−1 FW), while green cabbage had the highest values (164.0–194.9 mg GAE 100 g−1 FW) (Table 5). Among the red cultivars, red kale showed the lowest TPC values (86.9–110.6 mg GAE 100 g−1 FW); the highest values were obtained in red cabbage (158.5–248.5 mg GAE 100 g−1 FW) (Table 5). Overall, TPC was improved under high intensity in all Brassicaceae species, except in red cabbage and mustard. In these red cultivars, the TPC was enhanced by the medium intensity. In particular, red cabbage and mustard significantly improved under medium compared to low intensity by 56.8 and 54.9%, respectively (Table 5). On the other hand, green cabbage grown under high intensity significantly raised TPC compared to low intensity by 18.7%. In green kale, TPC significantly increased compared to low and medium intensities by 28.6 and 27.7%, respectively. In red kale, TPC enhanced under high intensity compared to low intensity by 27.3%. Similarly, TPC significantly increased by 52.5% in green mizuna microgreens grown at high compared to low intensity. In red mizuna, the high intensity also significantly increased the TPC compared to low and medium intensities by 54.7 and 27.5%, respectively. In the same way, high intensity significantly enhanced TPC in green mustard versus low and medium intensities by 75.3 and 59.5%, respectively (Table 5).
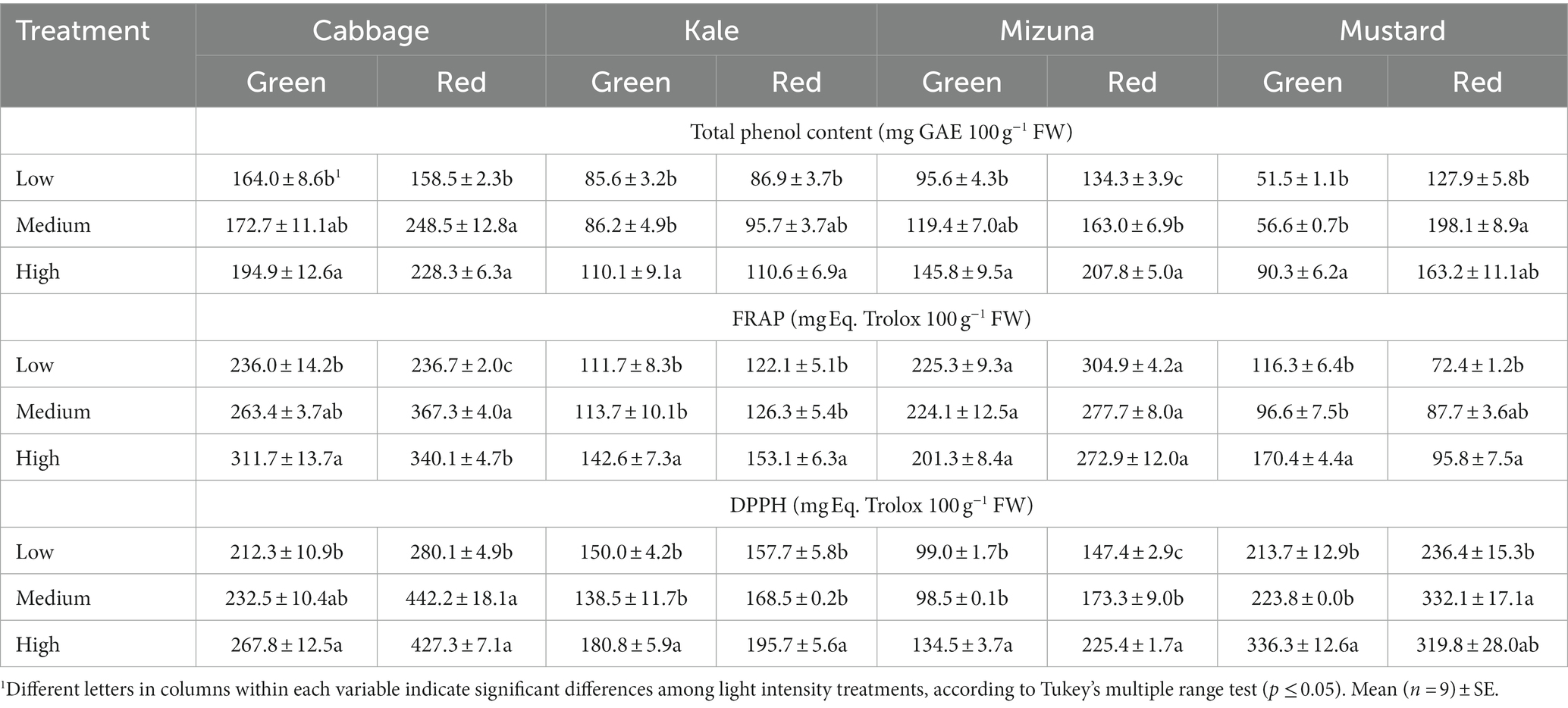
Table 5. Total phenol content and antioxidant capacity by FRAP and DPPH of Brassicaceae microgreens grown under low (120 ± 5.06 μmol m−2 s−1), medium (160 ± 3.60 μmol m−2 s−1), and high (210 ± 5.91 μmol m−2 s−1) intensities.
3.3.2 Antioxidant capacity
In green cabbage, the antioxidant capacity measured by FRAP and DPPH exhibited a similar trend across intensity treatments, significantly increasing AC at high intensity compared to low intensity. The increase by FRAP reached 32.1%, while by DPPH, it was 26.1% (Table 5). In red cabbage, both medium and high intensities raised AC compared to low intensity. Specifically, AC under medium significantly increased compared to low intensity by 55.2% (FRAP) and 57.9% (DPPH), respectively (Table 5). In both kale cultivars, high intensity promoted higher AC by FRAP and DPPH compared to low and medium intensities. Particularly, AC measured by FRAP increased under high intensity versus the other intensities in a range of 21.2–27.7%. Similarly, AC measured by DPPH enhanced under high compared to low and medium intensities in a range of 16.1–30.5% (Table 5). In both mizuna cultivars, AC measured by FRAP showed no significant differences among intensity treatments. Meanwhile, AC measured by DPPH showed a substantial worsening under high intensity compared to low and medium intensities. In green mizuna, high intensity significantly raised AC compared to the other intensities by about 36%. In red mizuna, the high intensity increased AC compared to low and medium intensities by 52.9 and 30.1%, respectively. Furthermore, medium intensity also significantly raised AC compared to low intensity by 17.6% (Table 5). In general, in both mustard cultivars, high intensity promoted AC by FRAP and DPPH compared to low and medium intensities. In green mustard, the AC by FRAP under high intensity was 46.5 to 76.4% higher than at low and medium intensities, respectively. Meanwhile, the AC by DPPH under high intensity was 57.4–50.3% higher than at low and medium intensities, respectively. In red mustard, AC by FRAP under high intensity significantly enhanced compared to low intensity by 32.3%. On the other hand, AC by DPPH significantly raised under medium intensity compared to low by 40.5% (Table 5).
4 Discussion
4.1 Agronomic characteristics
Microgreens are immature and tender edible vegetables of great importance in the market for their contribution to human well-being as functional foods (Hernández-Adasme et al., 2023). Among the various species, those of the Brassicaceae are characterized by secondary metabolites beneficial to health, such as polyphenols and glucosinolates (Ebert, 2022). The development, growth, and secondary metabolism of microgreens can be modulated by the intensity and spectrum of light (Flores et al., 2022; Hernández-Adasme et al., 2023). However, the impact of light on plants depends on the species and even the variety (Harakotr et al., 2019; Appolloni et al., 2021; Hernández-Adasme et al., 2022; Modarelli et al., 2022). According to Balázs et al. (2023), there was a linear relationship between light intensity and accumulated biomass in pea microgreens. Despite the significant variation in the fresh weight of microgreens under the different intensities, the authors concluded that only 31% of the variation in the fresh weight can be attributed to the PPFD changes. The results of this study showed that agronomic characteristics (fresh weight yield, dry weight yield, dry matter, hypocotyl length, and cotyledon area) differed under the light treatments depending on the species and cultivar (Table 2). In general, fresh weight yield was higher or similar for microgreens grown at low intensity (120 μmol m−2 s−1) compared to high intensity (210 μmol m−2 s−1), which coincided with a lower dry weight yield and DMC. Similar results were obtained for beet microgreens grown at low (120 μmol m−2 s−1) and medium (160 μmol m−2 s−1) intensities compared to high intensity (210 μmol m−2 s−1) (Hernández-Adasme et al., 2023). In Brassicaceae species like broccoli microgreens, one of the lowest intensities (50 μmol m−2 s−1) promoted the highest fresh weight, but also raised dry weight and moisture content compared to the other intensities of 30, 70, and 90 μmol m−2 s−1 (Gao et al., 2021). In radish, fresh weight yield decreased at an intensity of 50 μmol m−2 s−1 by 12%; when the intensity increased to 200 μmol m−2 s−1, fresh weight yield decreased by 22% compared to 100 μmol m−2 s−1. Additionally, increasing the intensity from 100 to 200 μmol m−2 s−1 for cabbage reduced fresh weight yield by 28% (Vetchinnikov et al., 2021). In other Brassicaceae microgreens (arugula, cabbage, kale, and mustard), biomass accumulation (i.e., fresh and dry weights) increased as the intensity increased from 100 to 600 μmol m−2 s−1 (Jones-Baumgardt et al., 2019). Harakotr et al. (2019) noted that among five indigenous vegetable microgreens, four species increased dry weight under high (330 μmol m−2 s−1) vs. low intensity (110 μmol m−2 s−1). Also, in cabbage and Chinese kale, the fresh weight per plant was raised under intensities between 50 and 70 μmol m−2 s−1 compared to lower or higher intensities like 30 and 90 μmol m−2 s−1, respectively (Liu et al., 2022). On the other hand, in the case of red and green amaranth cultivars at different intensities of 130–280 μmol m−2 s−1 did not promote significant differences in fresh weight (Meas et al., 2020), showing that the response to light intensity on fresh weight depends on species.
The higher or similar fresh weight yield, lower dry weight yield, and DMC of microgreens grown at low intensity (120 μmol m−2 s−1) would indicate higher water content. In contrast, the microgreens grown at high intensity (210 μmol m−2 s−1) showed a higher dry weight yield and DMC. According to Modarelli et al. (2022), the increased light intensity favors net photosynthesis and sustains the electron transport rate (ETR). In turn, the main process involved in producing dry matter is photosynthesis, whereby carbon dioxide is converted into carbohydrates (Bierhuizen, 1959). Nevertheless, recent studies have shown that the gradual increase in light intensity produced an increase in total plant dry weight compared to treatments with decreasing or constant light intensity (Jin et al., 2023). On the other hand, Mao et al. (2022) found that the expression of most Lhca and all Lhcb genes that code for light capture proteins in hypocotyls and cotyledons of pepper were significantly increased under low light conditions, while only Lhca2 and 5 were higher under high-intensity light. Hence, the expression of certain genes can preserve the normal photosynthetic capacity under different light-intensity conditions to capture more light.
The length of the hypocotyl of microgreens is a morphological characteristic that can be influenced by light. The results reported by Liu et al. (2022), Gerovac et al. (2016), Huang et al. (2021), and Samuolienė et al. (2013) showed a similar trend, namely the length of the hypocotyl of various Brassicaceae microgreens shortened with increasing light intensity. The results of Vetchinnikov et al. (2021) showed that the hypocotyl of radish microgreens decreased under intensities of 50 and 200 μmol m−2 s−1 vs. 100 μmol m−2 s−1. In the case of cabbage, increasing the intensity to 200 μmol m−2 s−1 caused a decrease in hypocotyl by 34%, whereas a low intensity (50 μmol m−2 s−1) increased hypocotyl length by 10% compared to the intensity of 100 μmol m−2 s−1. Johnson et al. (2020) noted a decrease in hypocotyl in arugula and mustard as the intensity increased from 20 to 650 μmol m−2 s−1, regardless of the light spectrum. In this study, lower intensity promoted a greater hypocotyl length (Table 2), which may indicate a promotion in the elongation of the cells that compose the hypocotyl. According to Bierhuizen (1959), a high-water content of the plant is favorable for cell elongation, which increases fresh weight, also observed in this study, but the size of some tissues, such as leaf area, showed no significant differences among the light treatments (Table 1). Wang and Shang (2020) pointed out that low light (50 μmol m−2 s−1; 16 h light) promotes hypocotyl elongation in Brassica rapa as it represses wall deposition by influencing the accumulation of cellulose, hemicellulose, and pectin. Moreover, these authors indicated light intensity significantly influenced the expression level of cell division cycle-associated (CDCA) family genes and cyclin-dependent kinase A1 (CDKA;1) gene involved in cell division. In transgenic Brassica napus plants overexpressing the CRY2a gene, which codes for cryptochrome 2, displayed the phenotype of short hypocotyl under low-intensity blue and white light relative to the wild type (Sharma et al., 2022). In other species, such as pepper seedlings, low light (50 μmol m−2 s−1; 16 h light) would induce rapid hypocotyl elongation due to increased expression of some family genes that code for auxins (AUX) and indole acetic acid (IAA) (Mao et al., 2022). Therefore, both cell division and elongation contributed to hypocotyl elongation under the influence of cryptochrome-mediated low light, which would benefit the harvesting of the microgreens, facilitating their management. However, low light promotes hypocotyl elongation both in isolation and in coordination with other variables, such as high temperature and high-water potential (Wang and Shang, 2020). The light spectrum is another variable to be considered since differential responses of Brassica species to different light sources have been observed for the same photoperiod. For example, Chen et al. (2021) noted that under combined red: blue (8:2) light, Chinese cabbage plant height was much greater in 9-day-old sprouts than in those cultivated under white light. Point to side color is an important attribute of microgreens that contributes to better sensory quality (Tan et al., 2020), popularity (Ebert, 2022) and can lead to an increase in consumption (Mir et al., 2017). This study reported differences in color for the different Brassicaceae species following no clear trend. Overall, the green cultivar’s species under medium intensity (160 μmol m−2 s−1) increased hue values of microgreens, whereas the low intensity (120 μmol m−2 s−1) reduced them. Hence, microgreens cultivated at medium intensity were greener than those at low intensity, except green mizuna (Table 3). On the other hand, the hue of red mizuna was lower at low intensity than at medium and high intensities, which promoted a less red color in the microgreens. In red kale, the hue values under the different intensities varied between 109.0 and 110.7°, denoting the absence of red-purple color. However, the low intensity significantly increased the greenness of these microgreens compared to the medium and high intensities (Table 3; Figure 2). No significant differences were observed in red cabbage and mustard (Table 3). According to Jones-Baumgardt et al. (2019), increasing light intensity between 100 and 600 μmol m−2 s−1 (photoperiod 16 h) of the blue: red (15:85) lamp decreased hue angle linearly in all Brassicaceae genotypes. Similarly, Johnson et al. (2020) observed a significant decrease in cotyledon hue in both species of arugula (Brassica eruca, “Rocket”) and mustard (Brassica juncea, “Ruby Streaks”) seedlings when light intensity increased between 20 and 650 μmol m−2 s−1 (photoperiod 24 h) irrespective of the light spectrum. However, the effect of intensity on vegetable color can interact with other factors, such as light spectrum, species (Johnson et al., 2020), and photoperiod (Hernández-Adasme et al., 2023). On the other hand, decreased hue is an indication of increased proportions of yellow and red pigments in green plants (Jones-Baumgardt et al., 2019) and serves as a measure of the absence of green in kale, cabbage, arugula, and mustard microgreens (Jones-Baumgardt et al., 2021). Chlorophylls impart vegetables a more vibrant color, contributing to a greater appreciation of their appearance (Tan et al., 2020). Likewise, a reduced hue indicates increased proportions of purple pigments in reddish-purple plants (Jones-Baumgardt et al., 2019). It is important to highlight that purple and blue colorations were correlated with higher anthocyanins and other flavonoid contents (Shibaeva et al., 2022). Thus, microgreens of green cultivars grown at low intensity would have a higher concentration of yellow-red pigments and a lower chlorophyll concentration, while there would be fewer anthocyanins in red mizuna microgreens. Nevertheless, the pigment concentrations obtained in this study did not coincide with the latter statement since, at low intensity, the chlorophyll contents (a and b) were higher. In contrast, carotenoid levels remained similar among treatments, except for mizuna microgreens (Table 4).
4.2 Pigment content
4.2.1 Chlorophylls and total carotenoids
Leaf pigments, such as chlorophylls and carotenoid concentration, can vary due to internal factors and as an indicator of response to light conditions (Sumanta et al., 2014). Choi (2021) showed in strawberries that photosynthetic pigments as chlorophyll a (Chl a), chlorophyll b (Chl b), and carotenoid contents changed under various light intensity conditions and presented significantly lower concentrations at high light intensity. On the other hand, Meas et al. (2020) show that the increase in light intensity between 130 and 280 μmol m−2 s−1 did not affect the concentration of total chlorophyll a, b, (a + b), and total carotenoids of red amaranth microgreens. These studies showed the differential response to light intensity of these pigments among species. In the current study, the content of Chl a showed a significant reduction at high light intensity (210 μmol m−2 s−1) in green and red cabbages, green kale, green and red mizuna, and green mustard (Table 4). On the other hand, Chl b obtained with high light intensity was significantly lower than at lower intensity in green cabbage, kale, mizuna, mustard, and red mizuna. Moreover, total carotenoids were reduced in green cabbage and green and red mizuna at high light intensity. Green cabbage reached 40 and 33% higher Chl a and Chl b, respectively, at low compared to high intensity. On the other hand, red cabbage had a higher content of Chl a and Chl b at low compared to high intensity by 25 and 22%, respectively. As cabbage, low intensity promoted a higher concentration of Chl a and Chl b in green and red kale grown at low compared to high intensity by 31 and 34%, respectively. Red kale also showed a reduction in Chl a and Chl b concentrations when grown at low intensity compared to high intensity by 9 and 13%, respectively (Table 4). For green mizuna, the reduction of Chl a and b between low and high intensity reached 39 and 24%, respectively, while for the red cultivar, it reached 22 and 18%, respectively. For mustard, only the green cultivar showed a significant decrease in Chl a and b due to the light intensity. In particular, the Chl a and Chl b concentrations decreased from low to high intensity by 21 and 22%, respectively. These results confirm those reported by Jones-Baumgardt et al. (2021), who observed that Chl a and Chl b content was reduced in the range of 24 and 30% between 100 and 400 μmol m−2 s−1 light intensities in cabbage, kale, mustard, and arugula.
In indoor conditions, 80 and 350 μmol m−2 s−1 were considered normal and high light intensity, respectively (Jiang et al., 2020). In this sense, high light exposure could disrupt the normal light reactions of photosynthesis. This disruption leads to an increase in the number of oxidized Photosystem I (PSI) reaction centers, which in turn contributes to photo-inhibition leading to the production of reactive oxygen species (ROS) such as singlet oxygen and superoxide radicals. These ROS can damage the chlorophyll molecules and other photosynthetic components, ultimately leading to a decrease in chlorophyll content (Janeeshma et al., 2022). In this study, the loss of chlorophyll a and b of microgreens exposed to high light intensities could likely attributable to enzyme-mediated degradation. Sato et al. (2015) report that in A. thaliana under high light intensities, the chlorophyll b is degraded by chlorophyll b reductase, which is proposed to be crucial for disassembling light-harvesting complexes as a strategy to minimize damage to light-capturing photosystems in the chloroplast.
Carotenoids are liposoluble pigments present in numerous fruits and vegetables. Some of these carotenoids, like β-carotene, serve as precursors to vitamin A and have antioxidant functions that effectively scavenge free radicals and extinguish singlet oxygen (Xiao et al., 2019). According to Xiao et al. (2012, 2019), the major carotenoids found in these Brassicaceae microgreens were β-carotene, lutein/zeaxanthin, and violaxanthin, which is very similar to the carotenoid profile of many mature Brassicaceae samples. The total carotenoid concentration was significantly reduced by light intensity in green cabbage and green and red mizuna (Table 4). Specifically, in green cabbage, the concentration of total carotenoids was reduced by 44% when they grow at high rather low intensity. Also, in green and red mizuna, the total carotenoid concentration decreased under low compared to high intensity by 37 and 15%, respectively. The reduction in β-carotene concentration can be attributed to the specific regulation of carotenoid biosynthesis and accumulation by light. Under high light stress, the activity of carotenoid cleavage dioxygenases (CCDs) is enhanced, thereby regulating carotenoid levels. Moreover, enzymatic oxidation via peroxidases/lipoxygenases or non-enzymatic photochemical processes in photosynthetic tissues also contributes to carotenoid homeostasis (Nisar et al., 2015). Furthermore, Arabidopsis leaves exposed to high light stress produce bioactive, volatile plant compounds such as β-cyclocitral and dihydroactinidiolide, which are derived from the oxidation of β-carotene. This oxidation process contributes to the observed reduction in β-carotene concentration. Also, these compounds, originating from carotene, function as stress signals, leading to the modulation of gene expression and activation of cellular defense mechanisms in plants (Nisar et al., 2015). The other evaluated species showed a similar trend, with no significant differences observed between light intensities. Likewise, Jones-Baumgardt et al. (2021) noted that total carotenoid concentrations were unaffected in kale, cabbage, mustard, and arugula microgreens exposed to variable PPFD from 100 to 600 μmol m−2 s−1.
4.3 Antioxidant compounds
4.3.1 Total phenol content
Consumption of microgreens has increased due to appreciation for their concentrated functional components, such as phenolics, compared to mature leafy greens (Mir et al., 2017). The phenolic content in the plant is determined by the plant’s biosynthetic processes, which are influenced by various factors, such as cultivar, climate, postharvest treatments, and agricultural and environmental conditions (Olsen et al., 2010). Among the phenols, flavonoids constitute the most abundant and varied category in our dietary intake (Olsen et al., 2010), where the most prevalent subgroups are flavanols and anthocyanins. The latter are responsible for the red and purple in the red cultivars of Brassicaceae species as cabbage, kale, mizuna, and mustard (Olsen et al., 2010; Sun et al., 2013). Total phenolic content in green and red cabbages increased under high compared to low intensity by 18 and 44%, respectively. As reported by Sun et al. (2013), different anthocyanins present in red cabbage microgreens correspond to cyanidin-3-diglucoside-5-glucoside derivatives. Compounds that, according to Olsen et al. (2010), represent 10.3% of the total phenol equivalents per 100 g of fresh weight. Also, Zhang et al. (2019) mentioned that light treatment, especially short-wavelength light, can improve anthocyanin accumulation and antioxidant capacity. In this context, the light spectrum used (Figure 1) contributed to the wavelength in the range between 380 and 420 nm, which corresponds to the UV-A region (Kozai and Zhang, 2016) and is thus capable of stimulating the synthesis of this kind of compound. This might explain the higher increase in total phenolics in the red cultivar over the green one.
Kale cultivars had similar total phenolic concentrations between 85 and 110 mg GAE 100 g−1 FW and showed an increase of 29 and 27% when high light intensities were compared with low for green and red cultivars, respectively (Table 5). Olsen et al. (2010) determined that red kale had a total phenolic content between 614 and 730 mg GAE 100 g−1 FW, and Xiao et al. (2019) found 220.8 ± 9.0 mg GAE 100 g−1 FW. These results were almost 7- and 2-fold, respectively, higher than values in this study for the same species at high light intensity (Table 5).
For green mizuna, the higher total phenolic concentration was registered at high light intensity and represented a 53% increase compared to low intensity. Also, the green mustard cultivated in this study had the most significant increase in total phenolic content, reaching an increase of 75% when cultivated at high over low intensity (Table 5). On the other hand, in red mizuna, total phenolic concentrations showed an increase of 55% when high and low intensities were compared and the total phenolic concentration in red mustard increase 28% at high intensity compared to low intensity. Lin et al. (2011) showed that, as in cabbage, the phenolic composition of red mustard was glycosylated hydroxycinnamic acid derivatives, and different flavonol glycoside derivatives that correspond to kaempferol quercetin or isorhamnetin. Lin et al. (2011) also showed that different anthocyanins present in red mustard correspond to acylated cyanidin 3-sophoroside-5-mono-di or tri-glucosides. These same authors mentioned that nearly all the structurally identified anthocyanins from the colored brassica plants corresponded to cyanidin with a similar chemical structure. Additionally, Zhang et al. (2019) said these compounds are accumulated by short-wavelength light (Figure 1).
Dannehl and Josuttis (2014) highlight that the quantity (intensity) and quality (spectrum) of light play a major role in terms of the accumulation of secondary plant compounds in fruits and vegetables. Also, according to Flores et al. (2022), high intensities can stimulate the increase in the concentration of total phenolic, total flavonoids, and the antioxidant capacity of lettuce and endive, especially when the light spectrum has an adequate intensity at wavelengths corresponding with blue color (400–500 nm) (Figure 1). Also, Appolloni et al. (2021) analyzed the effects of indoor LED lighting recipes and management on the specialized metabolite content in different groups of crop plants and found that most studies applied a combination of red and blue light (22%) or monochromatic blue (23%) with a 16 h day−1 photoperiod (78%) and an intensity greater than 200 μmol m−2 s−1 (77%) and concluded that these treatment features were often the most efficient in enhancing specialized metabolite content. Additionally, Verlinden (2020) mentioned that high light intensities and UV-A exposure treatments appear to trigger antioxidant accumulation, and wavelength in the range between 380 and 420 nm (Figure 1) can stimulate the synthesis of anthocyanins (Zhang et al., 2019). In addition, Oh et al. (2009) showed in lettuce that a high light intensity of 800 μmol m−2 s−1 applied for 1 day can increase the concentration of total phenolics and antioxidant capacity. The same authors showed that high light intensity, compared to other stresses, like chilling and heat shock, can be more effective in increasing the concentration of compounds such chlorogenic acid, caffeic acid, chicoric acid, quercetin 3-O-glucoside, and luteolin-7-O-glucoside, which belong to the phenylpropanoid pathway. These changes consequently can explain the increase in the recorded values of total phenolic content.
4.3.2 Antioxidant capacity
Dannehl and Josuttis (2014) emphasized that increased light intensity increases anthocyanins and flavonoids compounds, which is related to the antioxidant capacity. Therefore, the high antioxidant capacity found in Brassicaceae microgreens grown under high light intensity (210 μmol m−2 s−1) may be due to the accumulation of these molecules (Table 5). All Brassicaceae species evaluated in this study reached the highest antioxidant capacity by DPPH at 210 μmol m−2 s−1 and showed the same behavior as total phenolic compounds (Table 5). In general, red cultivars had higher antioxidant capacity by DPPH than green ones (Table 5). Only green and red mustards registered similar values among cultivars.
At high light intensity (210 μmol m−2 s−1), green and red cabbages showed a significant increase in DPPH values of 26 and 53% compared with low intensity (120 μmol m−2 s−1). Also, for green cabbage, AC by FRAP was higher at high light intensity, representing a 32% increase compared to low light intensity. Conversely, red cabbage reached the highest FRAP value at medium intensity, increasing 55% compared to low intensity and 8% compared to high intensity (Table 5).
Green and red kale also reached the highest DPPH values at 210 μmol m−2 s−1, which represents an increase of 21 and 24%, respectively, compared with the values reported at low intensity (Table 5). AC by FRAP also had higher values at high intensity, showing an increase of 28 and 25% in green and red kales, compared to low intensity. For this species, FRAP did not show differences among light-intensity treatments. Instead, DPPH showed an increase in green and red mizuna of 36 and 53% at high compared to low intensity (Table 5). According to Olsen et al. (2010), red kale had complex hydroxycinnamic acids derivatives, and disinapoyl-diglucoside was the main one, reaching 4.9% of the total amount of polyphenol equivalents per 100 g of fresh weight. These authors also showed that the main flavonol in red kale was kaempferol-3-sinapoyl-diglucoside-7-diglucoside, which represented 9.8% of the total amount of polyphenol equivalents per 100 g of fresh weight, being the mayor secondary compound responsible for the antioxidant capacity registered for this specie. Also, according to Verlinden (2020), this type of favonol can be accumulated at high light intensities. For green mustard, an increase in DPPH and FRAP of 47 and 57% at high intensity was observed compared to low intensity. Red mustard also showed the highest FRAP value at high intensity, increasing by 32% compared to low intensity. However, the highest DPPH values were reached at medium intensity representing an increase of 40 and 4% compared to low and high intensities, respectively.
Samuolienė et al. (2013) reported that a PPFD of 110 μmol m−2 s−1 suppresses normal growth and diminishes the nutritional value of Brassicaceae microgreens (Kohlrabi, mustard, red pak choi, and tatsoi). According to these authors, the most suitable conditions for growth and nutritional quality of the microgreens were higher intensities of 330–440 μmol m−2 s−1, resulting in a larger leaf surface area, higher total phenols, and antioxidant capacity. Similarly, in this study, 110 μmol m−2 s−1 reached the lowest concentration of total phenols and antioxidant capacity measured by DPPH and FRAP (Table 5).
Despite the high intensity increasing dry matter and dry weight percentage and also increase the TPC promoting a higher antioxidant activity, long photoperiods at low intensities can be better in terms of energy and financial efficiency than shorter photoperiods at high intensities (Filatov and Olonin, 2023; Lanoue et al., 2023).
5 Conclusion
Light intensity induced variations in the agronomic characteristics, color, chlorophyll content, and antioxidant activity of Brassicaceae microgreens. The changes were species- and cultivar-dependent. In particular, high intensity (210 μmol m−2 s−1) promoted an increase in antioxidant activity (phenolic content and antioxidant capacity) in Brassica microgreens. Consequently, high intensity positively impacted the antioxidant quality of Brassica microgreens grown in a vertical farm system. On the other hand, low (110 μmol m−2 s−1) and medium (160 μmol m−2 s−1) intensities promoted a higher or similar yield and chlorophyll pigment concentration in Brassica microgreens. Therefore, these levels of intensity are sufficient to achieve optimal quality in these types of vegetables, thereby enabling substantial energy conservation in vertical farm cultivation.
Data availability statement
The original contributions presented in the study are included in the article/supplementary material, further inquiries can be directed to the corresponding author.
Author contributions
MF: Data curation, Formal analysis, Investigation, Methodology, Writing – original draft, Writing – review & editing. CH-A: Data curation, Formal analysis, Writing – original draft, Writing – review & editing. MG: Methodology, Writing – original draft. VE: Conceptualization, Funding acquisition, Project administration, Resources, Supervision, Writing – review & editing.
Funding
The author(s) declare that financial support was received for the research, authorship, and/or publication of this article. This work was supported by FONDECYT 1230703, ANID, Chile.
Conflict of interest
The authors declare that the research was conducted in the absence of any commercial or financial relationships that could be construed as a potential conflict of interest.
Publisher’s note
All claims expressed in this article are solely those of the authors and do not necessarily represent those of their affiliated organizations, or those of the publisher, the editors and the reviewers. Any product that may be evaluated in this article, or claim that may be made by its manufacturer, is not guaranteed or endorsed by the publisher.
References
Ainsworth, E. A., and Gillespie, K. M. (2007). Estimation of total phenolic content and other oxidation substrates in plant tissues using Folin–Ciocalteu reagent. Nat. Protoc. 2, 875–877. doi: 10.1038/nprot.2007.102
Alrifai, O., Hao, X., Marcone, M., and Tsao, R. (2019). Current review of the modulatory effects of LED lights on photosynthesis of secondary metabolites and future perspectives of microgreen vegetables. J. Agric. Food Chem. 67, 6075–6090. doi: 10.1021/acs.jafc.9b00819
Appolloni, E., Pennisi, G., Zauli, I., Carotti, L., Paucek, I., Quaini, S., et al. (2021). Beyond vegetables: effects of indoor LED light on specialized metabolite biosynthesis in medicinal and aromatic plants, edible flowers, and microgreens. J. Sci. Food Agric. 102, 472–487. doi: 10.1002/jsfa.11513
Balázs, L., Kovács, G. P., Gyuricza, C., Piroska, P., Tarnawa, Á., and Kende, Z. (2023). Quantifying the effect of light intensity uniformity on the crop yield by pea microgreens growth experiments. Horticulturae 9:1187. doi: 10.3390/horticulturae9111187
Benzie, I. F., and Strain, J. J. (1996). The ferric reducing ability of plasma (FRAP) as a measure of ‘antioxidant power’: the FRAP assay. Anal. Biochem. 239, 70–76. doi: 10.1006/abio.1996.0292
Bierhuizen, J. F. (1959). "Plant growth and soil moisture relationships. Arid zone research, Unesco: Plant-water relationships in arid and semi-arid conditions” in Proceedings of the Madrid Symposium.
Brazaitytė, A., Miliauskienė, J., Vaštakaitė-Kairienė, V., Sutulienė, R., Laužikė, K., Duchovskis, P., et al. (2021). Effect of different ratios of blue and red LED light on Brassicaceae microgreens under a controlled environment. Plan. Theory 10:801. doi: 10.3390/plants10040801
Brazaitytė, A., Sakalauskienėe, S., Samuolienėe, G., Jankauskienė, J., Viršilė, A., Novickovas, A., et al. (2015). The effects of LED illumination spectra and intensity on carotenoid content in Brassicaceae microgreens. Food Chem. 173, 600–606. doi: 10.1016/j.foodchem.2014.10.077
Carillo, P., El-Nakhel, C., De Micco, V., Giordano, M., Pannico, A., De Pascale, S., et al. (2022). Morpho-metric and specialized metabolites modulation of parsley microgreens through selective LED wavebands. Agronomy 12:1502. doi: 10.3390/agronomy12071502
Chen, J., Chen, Z., Li, Z., Zhao, Y., Chen, X., Wang-Pruski, G., et al. (2021). Effect of photoperiod on Chinese kale (Brassica alboglabra) sprouts under white or combined red and blue light. Front. Plant Sci. 11:589746. doi: 10.3389/fpls.2020.589746
Choi, H. G. (2021). Correlation among phenotypic parameters related to the growth and photosynthesis of strawberry (Fragaria × ananassa Duch.) grown under various light intensity conditions. Front. Plant Sci. 12:647585. doi: 10.3389/fpls.2021.647585
Cui, J., Song, S., Yu, J., and Lui, H. (2021). Effect of daily light integral on cucumber plug seedlings in artificial light plant factory. Horticulturae 7:139. doi: 10.3390/horticulturae7060139
Dannehl, D., and Josuttis, M. (2014). “Cultivar and production effects on bioactive polyphenols” in Polyphenols in Plants, Isolation, Purification and Extract Preparation. ed. R. R. Watson. 1st ed (Academic Press), 3–13. doi: 10.1016/B978-0-12-397934-6.00001-2
Demir, K., Sarıkamış, G., and Seyrek, G. (2023). Effect of LED lights on the growth, nutritional quality and glucosinolate content of broccoli, cabbage and radish microgreens. Food Chem. 401:134088. doi: 10.1016/j.foodchem.2022.134088
Di Gioia, F., De Bellis, P., Mininni, C., Santamaria, P., and Serio, F. (2016). Physicochemical, agronomical, and microbiological evaluation of alternative growing media for the production of rapini (Brassica rapa L.) microgreens. J. Sci. Food Agric. 97, 1212–1219. doi: 10.1002/jsfa.7852
Di Rienzo, J. A., Casanoves, F., Balzarini, M. G., Gonzalez, L., Tablada, M., and Robledo, C. W. (2017). InfoStat Versión. Grupo InfoStat: FCA, Universidad Nacional de Córdoba, Córdoba, Argentina. Available at: http://www.infostat.com.ar
Dou, H., Niu, G., Gu, M., and Masabni, J. G. (2017). Effects of light quality on growth and phytonutrient accumulation of herbs under controlled environments. Horticulturae 3:36. doi: 10.3390/horticulturae3020036
Ebert, A. W. (2022). Sprouts and microgreens—novel food sources for healthy diets. Plan. Theory 11:571. doi: 10.3390/plants11040571
Filatov, D., and Olonin, I. (2023). Optimal ratio of spectrum, light intensity and photoperiod to minimize costs when growing microgreens. E3S Web Conf. 383:04074. doi: 10.1051/e3sconf/202338304074
Flores, M., Urrestarazu, M., Amorós, A., and Escalona, V. (2022). High intensity and red enriched LED lights increased the growth of lettuce and endive. Ital. J. Agron. 17:1915. doi: 10.4081/ija.2022.1915
Gao, M., He, R., Shi, R., Li, Y., Song, S., Zhang, Y., et al. (2021). Combination of selenium and UVA radiation affects growth and phytochemicals of broccoli microgreens. Molecules 26:4646. doi: 10.3390/molecules26154646
Gerovac, J. R., Craver, J. K., Boldt, J. K., and Lopez, R. G. (2016). Light intensity and quality from sole-source light-emitting diodes impact growth, morphology, and nutrient content of Brassica microgreens. HortScience 51, 497–503. doi: 10.21273/HORTSCI.51.5.497
Gupta, S., and Prakash, J. (2009). Studies on indian green leafy vegetables for their antioxidant activity. Plant Foods Hum. Nutr. 64, 39–45. doi: 10.1007/s11130-008-0096-6
Harakotr, B., Srijunteuk, S., Rithichai, P., and Tabunhan, S. (2019). Effects of light-emitting diode light irradiance levels on yield, antioxidants and antioxidant capacities of indigenous vegetable microgreens. Sci. Technol. Asia 4, 59–66. doi: 10.14456/scitechasia.2019.21
Hasan, M., Bashir, T., Ghosh, R., Lee, S. K., and Bae, H. (2017). An overview of LEDs’ effects on the production of bioactive compounds and crop quality. Molecules 22:1420. doi: 10.3390/molecules22091420
Hernández-Adasme, C., Palma-Dias, R., and Escalona, V. H. (2023). The effect of light intensity and photoperiod on the yield and antioxidant activity of beet microgreens produced in an indoor system. Horticulturae 9:493. doi: 10.3390/horticulturae9040493
Hernández-Adasme, C., Silva, H., and Escalona, V. (2022). In-door germination and seedling growth of green and red lettuce under LED-light spectrum and subsequent effect on baby leaf lettuce. Ital. J. Agron. 17:1982. doi: 10.4081/ija.2022.1982
Huang, J. J., D’Souza, C., and Zhou, W. (2021). Light-time-biomass response model for predicting the growth of choy sum (Brassica rapa var. parachinensis) in soil-based LED-constructed indoor plant factory for efficient seedling production. Front. Plant Sci. 12:623682. doi: 10.3389/fpls.2021.623682
Huché-Thélier, L., Crespel, L., Gourrierec, J., Morel, P., Sakr, S., and Leduc, N. (2016). Light signaling and plant responses to blue and UV radiations—perspectives for applications in horticulture. J. Exp. Bot. 121, 22–38. doi: 10.1016/j.envexpbot.2015.06.009
Janeeshma, E., Johnson, R., Amritha, M. S., Noble, L., Aswathi, K. P. R., Telesiński, A., et al. (2022). Modulations in chlorophyll a fluorescence based on intensity and spectral variations of light. Int. J. Mol. Sci. 23:5599. doi: 10.3390/ijms23105599
Jiang, Z., Zhu, L., Wang, Q., and Hou, X. (2020). Autophagy-related 2 regulates chlorophyll degradation under abiotic stress conditions in Arabidopsis. Int. J. Mol. Sci. 21:4515. doi: 10.3390/ijms21124515
Jin, W., Ji, Y., Larsen, D. H., Huang, Y., Heuvelink, E., and Marcelis, L. F. M. (2023). Gradually increasing light intensity during the growth period increases dry weight production compared to constant or gradually decreasing light intensity in lettuce. Sci. Hortic. 311:111807. doi: 10.1016/j.scienta.2022.111807
Johnson, R., Kong, Y., and Zheng, Y. (2020). Elongation growth mediated by blue light varies with light intensities and plant species: A comparison with red light in arugula and mustard seedlings. Environ. Exp. Bot. 169:103898. doi: 10.1016/j.envexpbot.2019.103898
Jones-Baumgardt, C., Llewellyn, D., Ying, Q., and Zheng, Y. (2019). Intensity of sole-source light-emitting diodes affects growth, yield, and quality of Brassicaceae microgreens. HortScience 54, 1168–1174. doi: 10.21273/HORTSCI13788-18
Jones-Baumgardt, C., Ying, Q., Zheng, Y., and Bozzo, G. G. (2021). The growth and morphology of microgreens is associated with modified ascorbate and anthocyanin profiles in response to the intensity of sole-source light-emitting diodes. Can. J. Plant Sci. 101, 212–228. doi: 10.1139/cjps-2020-0060
Knowledge Sourcing Intelligence (2020). Global Microgreens Market and Value Chain Analysis 2020–2025 Report. Available at: https://www.knowledge-sourcing.com/report/global-microgreensmarket?gclid=EAIaIQobChMIm7WQ5sPp7wIVWOqyCh0Klg09EAAYAiAAEgLw5fD_BwE (Accessed October 7, 2022)
Kou, L., Yang, T., Luo, Y., Liu, X., Huang, L., and Codling, E. (2014). Pre-harvest calcium application increases biomass and delays senescence of broccoli microgreens. Postharvest Biol. Technol. 87, 70–78. doi: 10.1016/j.postharvbio.2013.08.004
Kozai, T., and Zhang, G. (2016). “Some aspects of the light environment” in LED Lighting for Urban Agriculture. eds. T. Kozai, K. Fujiwara, and E. Runkle (Singapore: Springer), 49–55.
Lanoue, J., Louis, S., Little, C., and Hao, X. (2023). Continuous lighting can improve yield and reduce energy costs while increasing or maintaining nutritional contents of microgreens. Front. Plant Sci. 13:983222. doi: 10.3389/fpls.2022.983222
Lichtenthaler, H., and Buschmann, C. (2001). Extraction of photosynthetic tissue: chlorophylls and carotenoids. Curr. Protoc. Food Anal. Chem. 1, F4.2.1–F4.2.6. doi: 10.1002/0471142913.faf0403s01
Lin, L., Sun, J., Chen, P., and Harnly, J. (2011). UHPLC-PDA-ESI/HRMS/MSn analysis of anthocyanins, flavonol glycosides, and hydroxycinnamic acid derivatives in red mustard greens (Brassica juncea Coss variety). Agric Food Chem. 59, 12059–12072. doi: 10.1021/jf202556p
Liu, K., Gao, M., Jiang, H., Ou, S., Li, X., He, R., et al. (2022). Light intensity and photoperiod affect growth and nutritional quality of brassica microgreens. Molecules 27:883. doi: 10.3390/molecules27030883
Mao, L., Dai, Y., Huang, Y., Sun, H., Li, Z., Yang, B., et al. (2022). Effect of light intensity on gene expression in hypocotyl during the elongation in a leaf-yellowing mutant of pepper (Capsicum annuum L.). Agronomy 12:2762. doi: 10.3390/agronomy12112762
McGuire, R. (1992). Reporting of objective color measurements. Hortic. Sci. 27, 1254–1255. doi: 10.21273/HORTSCI.27.12.1254
Meas, S., Luengwilai, K., and Thongket, T. (2020). Enhancing growth and phytochemicals of two amaranth microgreens by LEDs light irradiation. Sci. Hortic. 265:109204. doi: 10.1016/j.scienta.2020.109204
Mir, S., Shah, M., and Mir, M. (2017). Microgreens: production, shelf life, and bioactive components. Crit. Rev. Food Sci. Nutr. 57, 2730–2736. doi: 10.1080/10408398.2016.1144557
Modarelli, G. C., Paradiso, R., Arena, C., De Pascale, S., and Van Labeke, M. C. (2022). High light intensity from blue-red LEDs enhances photosynthetic performance, plant growth, and optical properties of red lettuce in controlled environment. Horticulturae 8:114. doi: 10.3390/horticulturae8020114
Nisar, N., Li, L., Lu, S., Khin, N. C., and Pogson, B. J. (2015). Carotenoid metabolism in plants. Mol. Plant 8, 68–82. doi: 10.1016/j.molp.2014.12.007
Oh, M. M., Carey, E. E., and Rajashekar, C. B. (2009). Environmental stresses induce health-promoting phytochemicals in lettuce. Plant Physiol. Biochem. 47, 578–583. doi: 10.1016/j.plaphy.2009.02.008
Olsen, H., Aaby, K., and Borge, G. (2010). Characterization, quantification, and yearly variation of the naturally occurring polyphenols in a common red variety of curly kale (Brassica oleracea L. convar. Acephala var. sabellica cv. ‘Redbor’). J. Agric. Food Chem. 58, 11346–11354. doi: 10.1021/jf102131g
Rahman, M. M., Field, D. L., Ahmed, S. M., Hasan, M. T., Basher, M. K., and Alameh, K. (2021). LED illumination for high-quality high-yield crop growth in protected cropping environments. Plan. Theory 10:2470. doi: 10.3390/plants10112470
Riggio, G., Wang, Q., Kniel, K., and Gibson, K. E. (2019). Microgreens-a review of food safety considerations along the farm to fork continuum. Int. J. Food Microbiol. 290, 76–85. doi: 10.1016/j.ijfoodmicro.2018.09.027
Samuolienė, G., Brazaitytė, A., Jankauskienė, J., Viršilė, A., Sirtautas, R., Novičkovas, A., et al. (2013). LED irradiance level affects growth and nutritional quality of Brassica microgreens. Cent. Eur. J. Biol. 8, 1241–1249. doi: 10.2478/s11535-013-0246-1
Sato, R., Ito, H., and Tanaka, A. (2015). Chlorophyll b degradation by chlorophyll b reductase under high-light conditions. Photosynth. Res. 126, 249–259. doi: 10.1007/s11120-015-0145-6
Schneider, C. A., Rasband, W. S., and Eliceiri, K. W. (2012). NIH image to ImageJ: 25 years of image analysis. Nat. Methods. 9, 671–675. doi: 10.1038/nmeth.2089
Sharma, P., Mishra, S., Burman, N., Chatterjee, M., Singh, S., Pradhan, A. K., et al. (2022). Characterization of Cry2 genes (CRY2a and CRY2b) of B. Napus and comparative analysis of BnCRY1 and BnCRY2a in regulating seedling photomorphogenesis. Plant Mol. Biol. 110, 161–186. doi: 10.1007/s11103-022-01293-6
Shibaeva, T. G., Sherudilo, E. G., Rubaeva, A. A., and Titov, A. F. (2022). Continuous LED lighting enhances yield and nutritional value of four genotypes of Brassicaceae microgreens. Plan. Theory 11:176. doi: 10.3390/plants11020176
Son, K. H., and Oh, M. M. (2015). Growth, photosynthetic and antioxidant parameters of two lettuce cultivars as affected by red, green, and blue light-emitting diode. Hortic. Environ. Biotechnol. 56, 639–653. doi: 10.1007/s13580-015-1064-3
Sumanta, N., Imranul, C., Nishika, J., and Suprakash, R. (2014). Spectrophotometric analysis of chlorophylls and carotenoids from commonly grown fern species by using various extracting solvents. Res. J. Chem. Sci. 25, 97–101. doi: 10.1055/s-0033-1340072
Sun, J., Xiao, Z., Lin, L., Lester, G., Wang, Q., Harnly, J., et al. (2013). Profiling polyphenols in five Brassica species microgreens by UHPLC-PDA-ESI/HRMSn. J. Agric. Food Chem. 61, 10960–10970. doi: 10.1021/jf401802n
Tan, L., Nuffer, H., Feng, J., Kwan, S. H., Chen, H., Tong, X., et al. (2020). Antioxidant properties and sensory evaluation of microgreens from commercial and local farms. Food Sci. Human Wellness 9, 45–51. doi: 10.1016/j.fshw.2019.12.002
Toscano, S., Cavallaro, V., Ferrante, A., Romano, D., and Patané, C. (2021). Effects of different light spectra on final biomass production and nutritional quality of two microgreens. Plan. Theory 10:1584. doi: 10.3390/plants10081584
Vaštakaitė, V., and Viršilė, A. (2015). Light-emitting diodes (LEDs) for higher nutritional quality of Brassicaceae microgreens. Res. Rural. Dev. 1, 111–117.
Vaštakaitė, V., Viršilė, A., Brazaitytė, A., Samuolienė, G., Jankauskienė, J., Novickovas, A., et al. (2017). Pulsed light-emitting diodes for higher phytochemical level in microgreens. J. Agric. Food Chem. 65, 6529–6534. doi: 10.1021/acs.jafc.7b01214
Vaštakaitė, V., Viršilė, A., Brazaitytė, A., Samuolienė, G., Jankauskienė, J., Sirtautas, R., et al. (2015). The effect of blue light dosage on growth and antioxidant properties of microgreens. Sodin. Daržinink. 34, 25–35.
Verlinden, S. (2020). “Microgreens. Definitions, product types, and production practices” in Horticultural Reviews. ed. I. Warrington, vol. 47 (New Jersey, United States: John Wiley & Sons, Inc.), 85–124.
Vetchinnikov, A., Filatov, D., Olonina, S., Kazakov, A., and Olonin, I. (2021). Influence of the radiation intensity of LED light sources of the red-blue spectrum on the yield and energy consumption of microgreens. IOP Conf. Ser. Earth Environ. Sci. 723:032046. doi: 10.1088/1755-1315/723/3/032046
Wang, H., and Shang, Q. (2020). The combined effects of light intensity, temperature, and water potential on wall deposition in regulating hypocotyl elongation of Brassica rapa. PeerJ 8:e9106. doi: 10.7717/peerj.9106
Xiao, Z., Lester, G., Luo, Y., and Wang, Q. (2012). Assessment of vitamin and carotenoid concentrations of emerging food products: edible microgreens. J. Agric. Food Chem. 60, 7644–7651. doi: 10.1021/jf300459b
Xiao, Z., Luo, Y., Lester, F. E., Kou, L., Yang, T., and Wang, Q. (2014). Postharvest quality and shelf life of radish microgreens as impacted by storage temperature, packaging film, and chlorine wash treatment. LWT-Food Sci. Tech. 55, 551–558. doi: 10.1016/j.lwt.2013.09.009
Xiao, Z., Rausch, S., Luo, Y., Sun, J., Yu, L., Wang, Q., et al. (2019). Microgreens of Brassicaceae: genetic diversity of phytochemical concentrations and antioxidant capacity. LWT 101, 731–737. doi: 10.1016/j.lwt.2018.10.076
Zhang, X., Bian, Z., Li, S., Chen, X., and Lu, C. (2019). Comparative analysis of phenolic compound profiles, antioxidant capacities, and expressions of phenolic biosynthesis-related genes in soybean microgreens grown under different light spectra. J. Agric. Food Chem. 67, 13577–13588. doi: 10.1021/acs.jafc.9b05594
Keywords: LED, Brassicaceae , light intensity, total phenolic content, antioxidant capacity
Citation: Flores M, Hernández-Adasme C, Guevara MJ and Escalona VH (2024) Effect of different light intensities on agronomic characteristics and antioxidant compounds of Brassicaceae microgreens in a vertical farm system. Front. Sustain. Food Syst. 8:1349423. doi: 10.3389/fsufs.2024.1349423
Edited by:
José Pinela, Instituto Politécnico de Bragança, PortugalReviewed by:
Rajendra Persaud, Guyana Rice Development Board, GuyanaNorhayati Hashim, Sultan Idris University of Education, Malaysia
Aušra Brazaitytė, Lithuanian Research Centre for Agriculture and Forestry, Lithuania
Copyright © 2024 Flores, Hernández-Adasme, Guevara and Escalona. This is an open-access article distributed under the terms of the Creative Commons Attribution License (CC BY). The use, distribution or reproduction in other forums is permitted, provided the original author(s) and the copyright owner(s) are credited and that the original publication in this journal is cited, in accordance with accepted academic practice. No use, distribution or reproduction is permitted which does not comply with these terms.
*Correspondence: Víctor Hugo Escalona, dmVzY2Fsb25hQHVjaGlsZS5jbA==