- 1School of Chemistry and Chemical Engineering, University of Surrey, Guildford, United Kingdom
- 2School of Water, Energy and Environment, Cranfield University, Cranfield, United Kingdom
- 3Department of Chemical Engineering, Indian Institute of Technology Hyderabad, Sangareddy, Telangana, India
- 4Centre for Environment and Sustainability, School of Sustainability, Civil and Environmental Engineering, University of Surrey, Guildford, United Kingdom
Bread waste (BW) poses a significant environmental and economic challenge in the United Kingdom (UK), where an estimated 20 million slices of bread are wasted daily. BW contains polysaccharides with great potential for its valorization into building block chemicals. While BW valorization holds tremendous promise, it is an emerging field with low technology readiness levels (TRLs), necessitating careful consideration of sustainability and commercial-scale utilization. This review offers a comprehensive assessment of the sustainability aspects of BW valorization, encompassing economic, environmental, and social factors. The primary objective of this review article is to enhance our understanding of the potential benefits and challenges associated with this approach. Incorporating circular bioeconomy principles into BW valorization is crucial for addressing global issues stemming from food waste and environmental degradation. The review investigates the role of BW-based biorefineries in promoting the circular bioeconomy concept. This study concludes by discussing the challenges and opportunities of BW valorization and waste reduction, along with proposing potential strategies to tackle these challenges.
1 Introduction
Excessive food production to feed the world’s growing population, combined with wasteful consumer behavior, has resulted in massive food waste generation, posing a major challenge to sustainability and resource efficiency. With global population expecting to reach 9.6 billion by 2050 (Burke et al., 2023), ensuring food security will become increasingly challenging due to resource limitations. Agricultural systems need to achieve three objectives simultaneously: increase food production, create economic opportunities for rural communities, and reduce the adverse effects on the environment. Based on the Food and Agricultural Organization (FAO), the food waste generated globally is estimated at 1.3 billion tons per year, resulting in unfavorable economic and environmental consequences, leading to an economic loss of $990 billion and the release of 4.4 gigatons of CO2-eq (FAO, 2013). Within the European Union, an alarming quantity of 89 million tons of food is wasted annually, contributing to the emission of approximately 170 million tons of CO2-eq (Bräutigam et al., 2014). The UK alone witnesses the disposal of £20 billion worth of food and beverages each year. Notably, bread emerges as a significant contributor to food waste generation across Europe and North America, given its status as a staple food (Kumar et al., 2022).
According to the Waste and Resources Action Programme (WRAP), BW accounts for 10% of the total food waste in the UK (WRAP, 2021). Bread stands out as the second most wasted food in the UK, accounting for 20 million slices discarded daily. This results in an annual waste of 292,000 tons and emissions of 584,000 tons of CO2-eq (Narisetty et al., 2021), impacting both the environment and the economy significantly. While this waste primarily refers to the final product, the bread-making process and its supply chain introduce additional unaccounted waste, such as flour, dough, and trimmings. Addressing BW carbohydrates at its source is the most optimal solution (Brancoli et al., 2020), but predicting consumer attitudes within the food supply chain poses a challenge. Hence, alternative strategies must be explored to manage this waste.
In the current era, the extensive use of non-renewable fossil resources for energy and commodity has adversely impacted the environment. Consequently, researchers are exploring alternative and sustainable feedstocks for biorefineries. BW, characterized by its starchy composition and cost-effectiveness, has emerged as a highly promising and environmentally sustainable source for producing high-value biochemicals and bioenergy through fermentative processes (Kumar and Longhurst, 2018; Jung et al., 2022; Swetha et al., 2023). Unlike lignocellulosic biomass, which requires harsh pre-treatment, BW is readily accessible and serves as a sustainable feedstock for bioenergy and biochemicals (Narisetty et al., 2021), with lower carbon emissions and a greener profile (Yaashikaa et al., 2020; Brandão et al., 2021). However, it is crucial to thoroughly investigate the modes of BW generation at every stage of the supply chain and identify potential products that can be derived from it.
The concept of a circular bioeconomy presents a promising approach to enhance environmental well-being through the establishment of new value chains and the implementation of cleaner and more economically viable industrial practices. This approach also enables the transformation of waste into valuable resources, inspiring innovation and motivating retailers and consumers to attain a 50% decrease in food waste by the year 2030 (European Commission, 2018). The previous reviews have demonstrated the benefit of BW as a valuable source for producing high-value products within the circular bioeconomy. The previous works have further demonstrated the untapped potential of BW as a valuable source for producing high-value products within the circular bioeconomy. For instance, Narisetty et al. (2021) discussed BW generation across the supply chain and its associated logistical challenges. The study also revealed numerous potential applications, such as the production of bioplastics, biochemicals, biofuels, pharmaceuticals, and other renewable products, using the clean and high-quality fermentable sugars derived from BW through microbial fermentation. Similarly, Ben Rejeb et al. (2022) emphasized the valorization of BW into various valuable chemical building blocks, including aroma compounds, biohydrogen, enzymes, ethanol, glucose–fructose syrup, 5-hydroxymethylfurfural, lactic acid (LA), proteins, pigments, and succinic acid (SA). Another study by Kumar et al. (2022) highlighted that BW is a sustainable feedstock to produce a wide range of platform chemicals, including SA, LA, ethanol, 2,3-butanediol (BDO), and syngas. The study also examined the current challenges related to BW logistics and supply chain, as well as a comparison of life cycle assessments between BW-based production and alternative feedstocks. These studies underscore the potential of utilizing a fermentative approach to convert BW into valuable chemical building blocks, thereby maximizing resource recovery and minimizing the harmful environmental impact of BW disposal.
Despite the potential benefits, the valorization of BW is an emerging field with low technology readiness levels (TRLs), highlighting the need to explore commercial-scale production of biochemicals from BW while carefully considering technology readiness, economics, and sustainability aspects (Murthy, 2019). In particular, evaluating the sustainability of resource recovery opportunities is crucial. Silk et al. (2020) recommended taking into account environmental, economic, and societal factors when evaluating sustainability, as this aids in understanding the potential impacts and benefits of waste valorization technologies. Therefore, this review offers a distinctive perspective by highlighting the crucial need for a thorough assessment of the sustainability of BW valorization technologies and an integrated BW valorization strategy—an aspect that has not been extensively discussed in prior studies. The review is organized into six sections. Firstly, it offers an overview of the current state of BW generation. Following that, it explores the role of BW biorefinery in fostering a circular bioeconomy and discusses various biochemical and biofuel products derived from BW. An evaluation of the sustainability aspects associated with BW valorization is then presented. Subsequently, the challenges related to BW valorization are addressed, and potential strategies to overcome these challenges are proposed. Lastly, the review concludes by discussing the future strategic approach of BW valorization.
2 BW generation
Bread holds significant importance in the human diet due to its nutritional composition. For instance, 100 g of bread typically contains approximately 59.8 g of starch, 22.3 g of moisture, 1.56 g of total organic nitrogen, and around 8.9 g of protein (Leung et al., 2012; Yusufoğlu et al., 2021). As a staple food in developed nations, particularly North America and Europe, bread is produced in large quantities to meet global consumer demands.
Despite its importance in the human diet, bread has a short shelf life of around 3–6 days at room temperature. This is due to its high nutrient content, which makes it susceptible to decay and staling (Taglieri et al., 2021; Bhardwaj et al., 2023; Immonen, 2023). Moreover, the baking process transforms the starch into a digestible gelatinized form in bread, thereby making it vulnerable to microbial attack (Ben Rejeb et al., 2022). Consequently, a staggering amount of bread, amounting to millions of tons, is wasted on a daily basis in numerous developed countries as illustrated in Figure 1. A significant amount of BW is resulted at different stages of the supply chain, from primary production to manufacturing, distribution, and consumption by the end consumer (Figure 2).
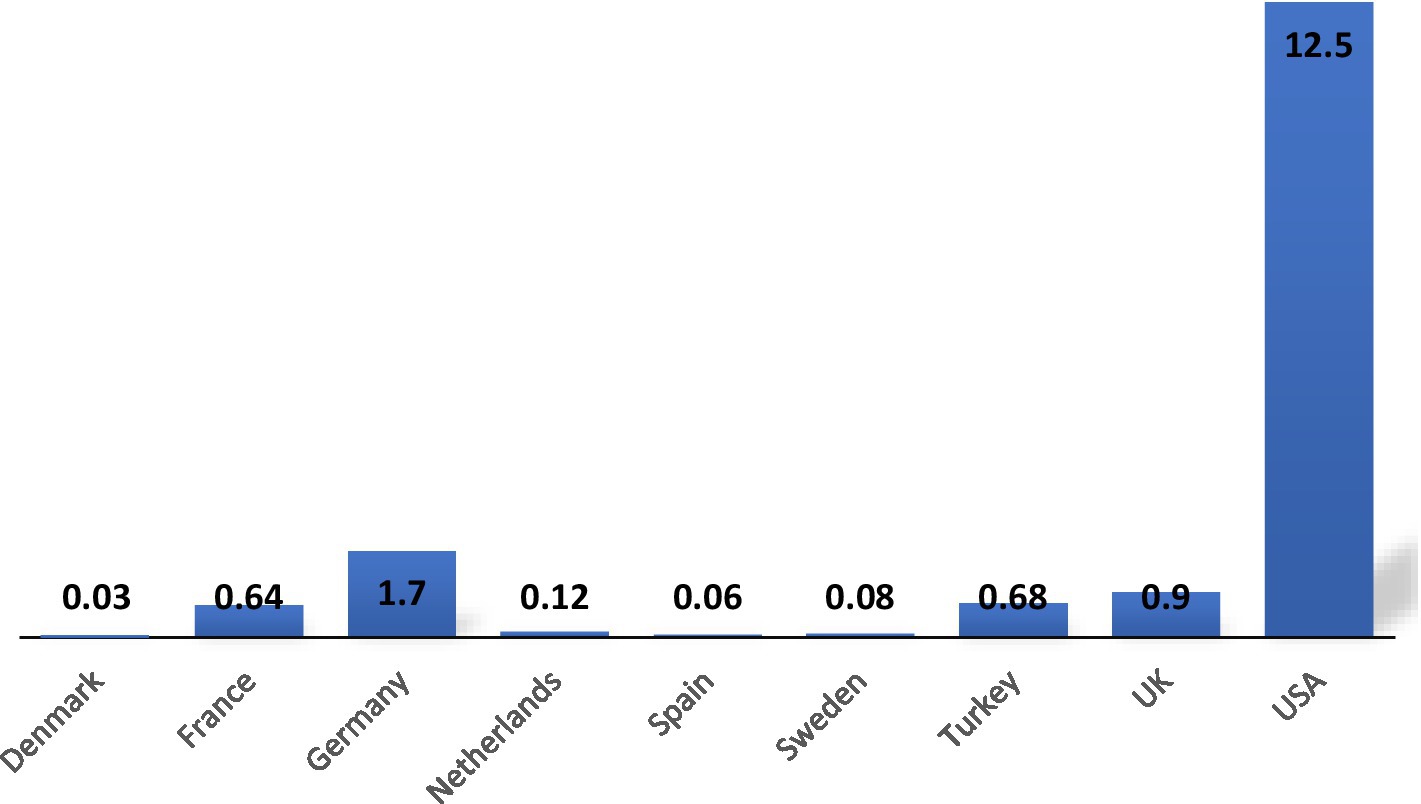
Figure 1. BW generation in different countries (million tons) (Kumar et al., 2022).
In the UK, a significant portion of bread, approximately 44%, is wasted during the manufacturing process, primarily due to factors that are difficult to avoid, such as overproduction, equipment breakdowns, and improper handling (Goryńska-Goldmann et al., 2020; Narisetty et al., 2021). Similarly, wastage can also occur during distribution due to challenges like inadequate transportation and storage, inaccurate forecasting, poor stock rotation, expired shelf life, break in the cold chain, suboptimal storage, and inadequate packaging (Jeswani et al., 2021). Consumer behavior is also a critical factor in BW generation at the household level, driven by factors, such as excessive purchasing, failure to consume bread before its expiration date, lack of knowledge about storage conditions and bread shelf life, and cooking or serving in excess, leading to leftovers that become inedible due to mold or spoilage (López-Avilés et al., 2019; Jeswani et al., 2021). Consequently, bakeries are under pressure to deliver fresh bread to meet consumer demand, leading to short lead times for order placement and product delivery.
To promote sustainability, it is thus important to actively address BW considering its substantial environmental implications. To achieve this, it is crucial to identify the various stages of the supply chain where wastage occurs. Several research studies have analyzed the environmental impact of various phases of the bread supply chain, commencing from wheat cultivation to consumption (Table 1). These studies revealed that some hotspots, such as agricultural production, baking, and electricity consumption, have been identified as targeted areas of improvement to reduce the environmental burden of bread production.
3 BW biorefinery promoting a circular bioeconomy
Currently, the world is facing two interconnected challenges: the generation of a substantial amount of waste and the diminishing availability of fossil fuels. The conventional approach to waste reduction involves implementing waste management based on a hierarchical framework directive, which raises ecological and environmental concerns as depicted in Figure 3. The waste framework directive has been identified as a viable solution for decreasing food waste, including BW (de Sadeleer et al., 2020). Research conducted by Eriksson et al. (2015) highlights the potential of BW in reducing greenhouse gas (GHG) emissions. However, waste prevention should be given priority over waste management options, as it has a more positive impact on the environment. The study by Slorach et al. (2020) supports this notion, as they demonstrate that waste prevention is six times more effective in reducing GHG compared to targeted collection for anaerobic digestion (AD). Vandermeersch et al. (2014) also found that utilizing BW for animal feed had a more favorable environmental impact than anaerobic digestion. Similarly, Brancoli et al. (2020) concluded that reducing BW through donation, source reduction, and animal feed production yielded significant environmental benefits compared to AD and incineration. Although preventing BW is important, it may not always be feasible. In such cases, it is crucial to develop effective strategies for valorizing the surplus. One potential approach is to utilize excess bread flour by employing alternative methods, such as enzymatic and/or fermentation processes, to create valuable new products (Figure 3). This idea has been suggested by Gómez and Martinez (2023) as an area for future research. Overall, prioritizing the reduction of food waste, including BW, should be a top priority in waste management strategies to minimize environmental impacts and mitigate climate change.
As an alternative, the biorefinery approach has emerged as a promising solution for reducing dependence on fossil-fuel-based feedstocks by producing chemical and energy products from renewable feedstocks in a sustainable manner. The term “biorefinery” is a concept inspired by crude oil refineries and analogous to petroleum refinery and petrochemical industry with the aim of creating effective biomass processing from plant, algae, agricultural waste, municipal waste, animal, and food waste into various products (i.e., energy, fuels, polymers, food additives, etc.) (Sadhukhan et al., 2014). Furthermore, the biorefinery represents a strategic approach that promots sustainability of the plant as a whole by integrating process to enhance energy efficiency, reduce water consumption, and mitigate emissions (Ubando et al., 2020; Thongchul et al., 2022). Currently, the commercial use of first-generation biorefineries is focused on the production of bioethanol and biodiesel. However, the use of edible substrates in these biorefineries encompases an ongoing debate regarding the balance between meeting the needs of the human population and addressing global food shortages (Malode et al., 2021; Thongchul et al., 2022). To circumvent this issue, the use of non-edible feedstocks in second-generation (2G) and third-generation (3G) biorefinery approaches has gained attention as a potential alternative (Hassan et al., 2019; Tong et al., 2022). Lignocellulosic biomass (LCB) has been the primary focus of research in 2G biorefineries. However, the commercialization of LCB-based biorefineries has associated challenges due to its recalcitrant nature and the high costs associated with its pretreatment for efficient valorization (Chandel et al., 2020). On the other hand, algal biomass is being explored for use in third-generation (3G) biorefineries offering potential for sustainable bio-production. However, it is important to note that this technology has thus far only been tested at the laboratory scale and has not yet been implemented on an industrial scale due to its cost ineffectivency (Kumar et al., 2022). For biobased products to be commercially feasible, they must be cost-competitive (Doddapaneni and Kikas, 2021). Of particular interest are platform chemicals derived from unavoidable food waste, as they have the potential to replace primary chemicals. For example, converting food waste into levulinic acid (Sadhukhan et al., 2016) and utilizing seaweed for nutraceuticals, pharmaceuticals, cosmeceuticals, and health, personal and home care products (Sadhukhan et al., 2019) are highly desirable. Utilizing waste materials that are abundant, cost-effective, and rich in renewable and fermentable carbon represents a significant opportunity for expanding the biomass feedstock sector. Waste streams with high sugar content, including food, bakery, bread, fruit, and beverage waste, have gained popularity due to their suitability as carbon feedstocks for biorefineries.
As mentioned previously, BW is a starchy material and provides a clean and easily extractable source of fermentable sugars, unlike LCB feedstocks that require harsh physical, chemical, and enzymatic treatments. An additional advantage of using BW-based feedstock is that no inhibitors are found in the produced sugars (Dymchenko et al., 2023). This feature makes BW a promising carbon source for new commercial processes; alternative methods utilizing chemical and enzymatic pre-treatment processes have been developed to release fermentable sugars. BW mostly consists of polysaccharides and small amounts of disaccharides, which normally comprises 50–70% starch, 8–10% protein, and 1–5% fat (Nair et al., 2017; Jung et al., 2022; Kumar et al., 2022; Narisetty et al., 2022a).
In recent years, several successful works have explored alternative methods for recycling BW into marketable biochemicals and biofuels (Table 2). The findings from these studies highlight the high potential of BW as a promising substrate for producing a diverse range of valuable fermentable sugars, biochemicals, and biofuels. Moreover, the derived biochemical products from BW, which are discussed in this article, are regarded as promising. They rank among the top 12 platform chemicals, according to the United States Department of Energy (US DOE) (Werpy and Petersen, 2004), and are under investigation as attractive bio-based chemical products by E4TECH, considering market attractiveness and UK strengths (LBNet, 2017). Furthermore, two currently promising biofuels derived from BW are bioethanol and hydrogen (H2), anticipated to replace fossil-fuel-based energy in the future. Bioethanol is currently used as a blend in the transportation industry, aligning with the European Union’s Renewable Energy Directive, which aims to replace 10% of transport fuel with biofuels like bioethanol (van Niekerk and Kay, 2020). Similarly, in the UK, the Renewable Transport Fuel Obligation (RTFO) mandates fuel suppliers to secure a proportion of their fuel supply from renewable sources, with an escalating biofuel production target from 4.75% in 2020 to 12.4% by 2032 (Küfeoğlu and Khah Kok Hong, 2020). Moreover, H2 is recognized as a promising future source of clean energy, offering the potential to generate no pollutants upon combustion. Establishing a low-carbon H2 sector is crucial for the UK’s net-zero goals. According to the Energy Research Partnership, the current demand for H2 in the UK is estimated to be around 27 terawatt-hours per year (Chari et al., 2023).
4 Biochemicals and biofuels from BW
This section predominantly highlights a range of economically viable biochemicals and biofuels derived from BW. It explores the methodologies applied in the conversion of BW into diverse bioproducts.
4.1 Biochemicals
4.1.1 2,3-Butanediol
2,3-BDO is considered as high-value chemical, which has been widely used in the food application, chemicals, cosmetics, and pharmaceutical industries (Gadkari et al., 2023). The 2,3-BDO market is projected to reach approximately $220 million by 2027, with a compound annual growth rate (CAGR) of 3% from 2019 to 2027 (Tinôco et al., 2021; Maina et al., 2022). The study of Narisetty et al. (2022b) was aimed to produce BDO sustainably from BW using Enterobacter ludwigii. The findings indicated that enzymatic hydrolysis, under optimal conditions of 10% w/v solid loading and an enzyme loading of 0.6 mg/g, resulted in a BDO yield of 0.43 g/g glucose. In fed-batch cultivation, BDO reached 138.8 g/L after 96 h, with a yield of 0.48 g/g brewery waste.
4.1.2 Succinic acid
SA is a key compound, which is considered one of the 12 main platform chemical according to US DOE. In 2022, the worldwide market for SA was worth $160.8 million, and it is projected to grow at a 6.5% annual rate, reaching $301.4 million by 2032 (Escanciano et al., 2023). The potential economic and sustainable impact of producing SA through fermentation using renewable carbohydrate feedstocks is attractive, which has the ability to replace current petroleum-based production. Leung et al. (2012) utilized enzymes from Aspergillus awamori and Aspergillus oryzae to extract sugars and amino acids from BW. This hydrolysate was then employed for SA production by Actinobacillus succinogenes through solid-state fermentation. The bacterial fermentation resulted in 47.3 g/L of SA, with an overall yield of 0.55 g/g BW.
4.1.3 Lactic acid
LA is a versatile platform chemical with a market potential of $2.64 billion, attributed to its wide range of applications (Shoaf and Engelberth, 2022). Cox et al. (2022) investigated different hydrolysis (acid and enzymatic) processes of BW to produce LA. The enzymatic hydrolysis produced highest glucose release and yield with 98.6 g/L and 0.49 g glucose/g BW using Dextrozyme from Novozymes. In addition, the fed-batch fermentation by B. coagulans resulted in titer of 155.4 g/L LA, with a productivity and LA yield of 1.30 g/L and 0.42 g/g BW, respectively. Sadaf et al. (2021) explored BW as a rich source of reducing sugars for the production of LA. Under conditions of simultaneous saccharification and fermentation, SKL-11 demonstrated the highest LA production, reaching 56 mg/g of bakery waste, equivalent to 28 g/L.
4.1.4 5-Hydroxymethylfurfural
5-Hydroxymethylfurfural (5-HMF) is a versatile platform chemical that can be turned into various useful materials, which is used in different areas like medicine, chemicals, food, pesticides, and making diesel fuel (Kong et al., 2020). The expected global market value for 5-HMF is projected to reach EUR 55 million by 2024, with CAGR of 1.4% (Albonetti et al., 2022). Yu et al. (2018) explored the use of propylene carbonate (PC) and γ-valerolactone (GVL) as environmentally friendly co-solvents in a binary solvent-water medium for the conversion of BW to 5-HMF over SnCl4 as the catalyst. The study revealed that the PC/H2O solvent system outperformed the others, achieving a notable 5-HMF yield of 20 mol%. In comparison, the GVL/H2O solvent system produced a lower 5-HMF yield of 13.5 mol%. Iris et al. (2017) investigated the effects of selected mediums on the desirable pathways (hydrolysis, isomerization, and dehydration). The polar aprotic solvent of ACN/H2O was the highest rate of 5-HMF production from BW with 0.217 g/g BW. Furthermore, 5-hydroxymethyl-2-furfurylamine (HMFA) emerges as a key byproduct of 5-HMF, with notable applications in the synthesis of pharmaceutical hardeners, epoxy resins, biofuels, and chemicals derived from furan (Gao et al., 2023b). Wu et al. (2023) transformed BW into HMFA using a cascade reaction in betaine:malonic acid (B:MA)-water. They achieved a 30.3% yield of 5-HMF from BW in 45 min at 190°C. By using a specially engineered E. coli as a biocatalyst, they converted the 5-HMF into HMFA with a yield of 0.282 g HMFA/g BW at 35°C for 12 h. Gao et al. (2023a) proposed an innovative approach for converting bakery waste into HMFA using a two-step method. This method involves tandem catalysis utilizing deep eutectic solvent betaine/formic acid as the chemical catalyst and Escherichia coli HILF as the biocatalyst. Initially, a chemical catalyst, betaine/formic acid, was used on the BW, yielding 0.269 g 5-HMF/g BW. Subsequently, employing a specialized Escherichia coli HILF biocatalyst, which was efficiently converted 5-HMF into HMFA with a remarkable yield of 0.243 g HMFA/g BW within 6 h. Zhang et al. (2023) employed a chemoenzymatic approach to synthesize 5-HMF from BW for the production of 2,5-furandimethanol (FDM). This process involved a combination of catalysis, utilizing the deep eutectic solvent lactic acid:betaine as the chemocatalyst, and the HMFOMUT cell (biocatalyst: E. coli HMFMOUT whole-cell). The findings indicated that, under the conditions of lactic acid:betaine (15 wt%) at 180°C for 15 min, the 5-HMF yield reached 44.2 Cmol% of BW. In the presence of lactic acid:betaine–H2O, the HMFOMUT efficiently converted 5-HMF derived from BW into FDM, achieving a notable productivity of 700 kg of FDM per kg of 5-HMF (equivalent to 230 kg of FDM per kg of BW).
4.2 Biofuels
4.2.1 Bioethanol
Bioethanol stands out as a promising biofuel with several favorable attributes, involving a combustion efficiency and high octane number and heat vaporization (Narisetty et al., 2021). At the moment, the number of studies have been performed to improve the bioethanol production from BW. Nikolaou et al. (2023) investigated the viability of bioethanol production from BW through the examination of two distinct experimental processes: Separate Hydrolysis and Fermentation (SHF) and Simultaneous Saccharification and Fermentation (SSF). The investigation revealed that the optimal conditions for bioethanol production occurred under SSF conditions, specifically with 20% solid loading, an enzyme loading of 20 µL/g starch, and 2% w/w saccharomyces cerevisiae, maintained at 35°C for 48 hours. These conditions resulted in a bioethanol concentration of 87.5 g/L. Narisetty et al. (2022a), conducted a comprehensive studies of bioethanol production from BW, where the optimization of saccharification process both acid and enzymatic hydrolysis was also done through solid and acid/enzyme loading. The enzymatic hydrolysis demonstrated optimal glucose release, reaching 97.9 g/L at a solid loading of 20% w/v, equivalent to 95.9% of the maximum theoretical yield. Subsequently, the derived hydrolysate underwent fermentation to yield ethanol, which resulted in ethanol titers, yield and productivity of 114.9 g/L, 0.49 g/g and 3.2 g/L.h, respectively. The improvement of bioethanol production was also performed by Ünal et al. (2022) which was investigated the effect of temperature (25 and 30°C) and nitrogen supplementation (with 20 g/L peptone or without) on bioethanol production from BW. The mean ethanol yield using BW at 30°C was 0.49 ± 0.005 g ethanol/ g BW with 95.75% ± 0.93 of the maximum theoretical ethanol yield with nitrogen supplementation. Mihajlovski et al. (2020) performed the enzymatic hydrolysis of BW by a newly isolated Hymenobacter sp. CKS3 strain. The use of new strain resulted in 19.89 g/L of reducing sugars with maximum bioethanol concentration of 17.3 g/L after 24 h of fermentation. Han et al. (2019) developed a two-step enzymatic hydrolysis and ethanol fermentation along with its effect of enzymes volume on the performances. The maximum ethanol production and yield (46.6 g/L and 1.12 g/g dry cake) was achieved after 40 h of fermentation time.
4.2.2 Hydrogen
Hydrogen is recognized as a promising future clean energy carrier, as it produces no pollutants upon combustion, which posses higher mass caloric value (120–142 MJ/kg) surpasses that of methane (50 MJ/kg) and ethanol (26.8 MJ/kg) (Sillero et al., 2022). Adessi et al. (2018) conducted a two-stage process involving lactic fermentation and photo-fermentation to transform BW into H2. The most favorable outcomes were achieved using Lactobacillus amylovorus DSM20532, followed by photo-fermentation employing Rhodopseudomonas palustris 42OL, along with ferric citrate and magnesium sulfate supplementation. This method yielded 3.1 mol H2/mol glucose, with an energy recovery of 54 MJ/t dry BW. Han et al. (2017) studied an acceleration of the hydrolysis to enhance substrate conversion efficiency through a two-stage enzymatic hydrolysis prior to dark fermentation for H2 production using BW. The two-stage enzymatic hydrolysis showed an improvement of the nutrient conversion efficiency producing starch conversion of 96.6% and glucose yield of 0.521 g/g BW and the maximum H2 yield was obtained at 103 mL H2/g BW. Han et al. (2016b) proposed a novel bio-hydrogen production from BW using continuous stirred tank reactor (CSTR). Under a chemical oxygen demand (COD) concentration of 6,000 mg/L, the system achieved a H2 production rate of 7.4 L/(ld) and a H2 yield of 109.5 mL hydrogen/g BW.
5 Sustainability assessments of BW valorization for chemical production
Creating a sustainable biorefinery requires a systematic approach that involves designing, analyzing, and assessing the economic, environmental and social impacts of the process (Figure 4). Sustainable development must meet current needs while preserving natural resources, protecting the environment, achieving economic prosperity, and improving quality of life without compromising future generations (Posada and Osseweijer, 2016). A comprehensive sustainability assessment is necessary to ensure investment decision-making and societal acceptance. This assessment should encompass economic, environmental, and social impact considerations. Early-stage monitoring is necessary to avoid undesirable developments, and decision-making should consider multiple factors simultaneously to capture synergies. Thorough studies on systematic data collection and analysis methodology are required to ensure an accurate sustainability assessment (Thongchul et al., 2022). Evaluating sustainability can aid in understanding the potential impacts and benefits of BW valorization technologies, as it integrates economic, environmental, and social evaluations (Giraldo et al., 2020; Sadhukhan et al., 2021).
5.1 Life-cycle assessment
The objective of biorefineries is to produce products with lower carbon emissions compared to fossil-based refineries. However, the inherent complexity of the process presents sustainability challenges and potential environmental impacts. Therefore, it is crucial to employ a systematic methodology for identifying and evaluating their impacts. The sustainability of biorefineries, in comparison to products derived from fossil fuels is typically evaluated employing a life cycle approach (Collotta et al., 2022). Life-cycle assessment (LCA) has been widely used to quantify the environmental burden of certain product or process and is a tool for decision-making of sustainable process development. The evaluation of environmental impact in BW biorefineries is crucial, and a comprehensive life cycle impact assessment considering various factors is necessary. Recently, several researchers have conducted studies on the environmental impact assessment of BW valorization using LCA, as summarized in Table 3. This table represents the key features of the articles reviewed, including region and year, functional unit, system boundary, type of products, life cycle impact assessment (LCIA) method and database. To facilitate comparison, the environmental impact is primarily focused on the Global Warming Potential (GWP) score.
These studies aim to assess the environmental consequences of using BW to produce biochemical products, such as LA, bioethanol, SA, and fungal products. However, a comparative LCA for BW valorization remains challenging due to variations in functional units. Vanapalli et al. (2023) conducted a study that revealed producing LA from BW via low pH fermentation to be more environmentally friendly than using neutral pH fermentation. This study underscored strategies for reducing environmental impact, including employing pinch technology for enhanced energy efficiency and implementing a closed-loop system to mitigate water consumption. Conducted an LCA on the co-production of bioethanol and biomethane from BW. They identified electricity production as the most significant contributor to GHG emissions, followed by the use of chemicals, transportation, and water. The study found that improving process yield was effective in reducing the overall electricity requirements, particularly during fermentation and downstream processing. Moreover, a shift toward renewable energy sources for electricity generation was suggested as a promising strategy for further reducing the GWP and enhancing process sustainability. Gadkari et al. (2021) compared fermentative SA production from BW with fossil-based SA production, revealing a lower environmental impact for the former. The primary GHG emission sources were identified as steam and heating oil used in the process. Additionally, the study highlighted the potential of repurposing solid biomass waste as fish feed, which could contribute to environmental savings. Targeting steam and heating oil usage could significantly reduce energy requirements and GHG emissions. Brancoli et al. (2021) investigated the environmental burden of producing fungal food products using surplus bread. The study examined four scenarios: using stale bread (Scenario 1), molasses and ammonium chloride (Scenario 2), glucose (Scenario 3), and recirculated fermented product (Scenario 4). The findings showed that Scenario 4, followed by Scenario 1, had the lowest environmental impacts. These scenarios, which replaced glucose or molasses with stale bread, significantly reduced the environmental impacts associated with inoculum production. Conversely, Scenarios 2 and 3, which involved inoculum production and solid-state fermentation, demonstrated higher environmental impacts, underscoring the importance of optimizing electricity consumption. Furthermore, research on BW management by Eriksson et al. (2015) and Brancoli et al. (2020) highlighted donation and source reduction as the most environmentally favorable options, resulting in the lowest GHG emissions. These options stood out due to their minimal process-associated impacts and ease of distribution. However, the studies offered differing recommendations for subsequent waste management strategies. However, the studies differ in their recommendations for subsequent waste management options after donation and source reduction. Brancoli et al. (2020) favored converting BW into bioethanol, feed, and beer, citing benefits like the use of dried distillers grains with solubles (DDGS) as animal feed and the substitution of ingredients in feed and beer production. On the other hand, Eriksson et al. (2015) suggested incineration and AD as viable alternatives. The least favorable options were identified as landfilling (Eriksson et al., 2015) due to methane emissions, and incineration (Brancoli et al., 2020) due to low environmental savings from the bread’s low heating value and minimal energy substitution credit. In a recent study, we described an integrated biorefinery approach using BW, co-producing bioethanol and SA (Hafyan et al., 2024). The study systematically investigated four distinct scenarios, with Scenario 4 specifically incorporating AD, combined heat and power (CHP) generation, carbon capture (CC) technology, and SA production. This particular scenario stood out by delivering exceptional results, as it maximized the utilization of BW materials and generated marketable products including bioethanol, SA, and fertilizer. Notably, the integrated approach exhibited a remarkably low GWP value, measuring at −0.344 kg CO2-eq, thereby underscoring its significant environmental benefits.
These findings highlight the substantial potential of BW to replace non-renewable products in the long run, particularly when integrated with strategies to reduce environmental impact. Additionally, the outcomes involve the identification of environmental hotspots in the process of enhancing the value of waste, proposing solutions to address these issues, identifying more effective methods for value enhancement across various options, and pinpointing the most environmentally friendly technology for this purpose. Hence, LCA proves highly beneficial in recognizing potential trade-offs during the design phase and ensuring environmentally advantageous decisions are prioritized (Caldeira et al., 2020).
5.2 Techno-economic assessment
Techno-economic assessment (TEA) offers comprehensive insights into a technology or process, encompassing aspects like process and energy efficiency, product output, emissions, and the associated costs required to achieve specific objectives. It represents a cost-effective approach for evaluating the overall performance and commercial viability of novel technologies (Rout et al., 2023). Within an economic system, the ability to deliver desired products at competitive prices while considering production costs and profitability is of paramount importance. To assess a project’s feasibility, various economic indicators are examined, including net present value (NPV), internal rate of return (IRR), return on investment (ROI), and payback period (PBP). TEA has provided stakeholders with valuable insights into the enduring economic effects of technologies at diverse stages of commercialization (Almada et al., 2023). Based on the existing literature, economic assessment is often given the highest priority in the valorization of waste, followed by environmental assessment and the combination of both (Rana et al., 2020).
The production of bio-based products encounters substantial competition from petrochemicals, primarily in terms of market costs. Therefore, TEA plays a crucial role in forecasting the economic competitiveness of a production process. However, it’s worth noting that only a limited number of studies have undertaken the economic evaluation of BW valorization technologies. Mailaram et al. (2023) explored the economic feasibility of valorizing BW feedstock for the large-scale production of two distinct fermentation processes for LA—one operating under acid-neutral conditions and the other at a low pH level, both with a capacity of 100 metric tons per day. The study unveiled that the microbial LA production proved to be capital-intensive, attributed to the prolonged fermentation of BW to LA and the complex reactive separation of LA. In addition, the substantial total operating cost was primarily influenced by a high BW price, considering collection and transportation costs, as well as the use of energy-intensive separation process. Nevertheless, the implementation of pinch technology managed to reduce utility cost for both scenarios of acid neutral and low PH, with around 15 and 11%, respectively. As a result, the minimum selling prices estimated for acid-neutral and low pH scenarios were $3.52/kg and $3.22/kg, respectively, considering 5 years of PBP and IRR set at 8.5%. However, the study highlighted the imperative need for cost reduction strategies, advocating for the development of a more efficient LA production process, cost-effective downstream LA purification processes, and governmental subsidies to mitigate BW costs. Such strategies were shown to potentially decrease the production cost of LA by approximately 12–14%. Han et al. (2016a) conducted an economic analysis of hydrogen production derived from BW in a CSTR with a daily BW processing capacity of 2 metric tons. The total investment and annual production cost were estimated at $931,020 and $299,746/year, respectively. The primary constraint identified was the installation cost of the H2-producing plant, with the grinding and purification system representing the major components of equipment cost. Utilities (electricity and process water) and labor costs constituted significant contributions to the total operating cost. The study revealed that H2 yield constituted a substantial portion of revenue, and the inclusion of co-products, such as CO2, solid biomass (fish feed), and treated BW, contributed to an increased total annual revenue of $639,920/year. H2 yield and market price emerged as pivotal factors influencing industrial-scale hydrogen production. The analysis demonstrated the attractiveness of the plant, with a NPV of $1,266,654 and PBP of 4.8 years. Furthermore, the study emphasized that a lower discount rate and a hydrogen processing plant capacity exceeding 0.58 MT/day were prerequisites for profitability. Additionally, enhancements in energy efficiency and reductions in operating labor costs were identified as pivotal factors for improving the economic viability of hydrogen production from BW. Lam et al. (2014) investigated a profitability analysis of SA production from 1 metric tons of BW daily in Hong Kong. The capital investment required amounted to $1,118,243, with the SA fermenter constituting the primary contributor to the total equipment cost. It is noteworthy that the total production cost reached $230,750, primarily attributed to the substantial allocation of expenses—95%—toward the procurement of raw materials, specifically for the purchase of CO2. Moreover, the high steam consumption contributed to elevated utility costs, and the associated operating labor costs were also identified as significant contributors. All revenue generated emanated from the SA product, which constituted the primary revenue stream. Additionally, two co-products, namely solid biomass (utilized as fish feed) and treated BW, contributed to an overall revenue of $374,041/year. Furthermore, the profitability analysis disclosed a ROI of 12.8%, PBP of 7.2 years, and IRR of 15.3%. The study underscored key thresholds for ensuring profitability, emphasizing that the selling price of SA and the plant capacity for SA production should not fall below 0.26 MT/day. In our recent study, in addition to studying the environmental performance of the integrated biorefinery based on co-production of bioethanol and SA from BW, we also investigated the economic feasibility of the proposed plant (Hafyan et al., 2024). Once again, Scenario 4, which exhibited the most exceptional environmental performance, also demonstrated outstanding economic viability. It boasted a brief PBP of 2.2 years, a robust IRR of 33% after tax, a ROI of 32%, and a noteworthy NPV of 163 M$. The study provided compelling evidence that optimizing the utilization of all BW materials through an integrated biorefinery significantly enhanced the profitability of the process.
In summary, the reviewed studies point to feedstock quantity and cost, energy consumption, labor cost, and reactor yield as key factors influencing economic uncertainties and fluctuations in bioproducts from BW. Future research should prioritize better control over these factors. Overall, the TEA results support the commercial viability of BW valorization. However, it is crucial to note that the economic success of these processes at an industrial scale depends on specific pathways, with market prices and economies of scale playing a significant role in determining profitability.
5.3 Social life cycle assessment
Social impact assessment (S-LCA) is an essential aspect of sustainability evaluation, but it is often the least studied. S-LCA studies encompass five primary social categories, namely labor rights, health and safety, human rights, governance, and community infrastructure, along with 22 sub-categories which are discussed in the work of Sadhukhan et al. (2014) and (2021). S-LCA is a systems analysis tool used to analyze and evaluate the impact of changes in a product or service’s life cycle. It evaluates the social impacts, which is widely accepted approach for sustainability trade-off analysis. Andrews (2009) revealed that S-CLA is a technique that assesses the social and economic impacts of products throughout their entire life cycle, including raw material extraction, manufacturing, distribution, use, and disposal stages. It aims to identify both positive and negative impacts of a product on society. Vance et al. (2022) stated that social sustainability is the assessment of the social costs and benefits of a product, process, or system using s-LCA. However, it is important to notice that the social impact indicators are difficult to measure them per functional unit, unlike environmental and economic impacts. It is typically qualitative and semi-quantitative data according to physical output (Shemfe et al., 2018). Aristizábal-Marulanda et al. (2020) emphasized that when analyzing the social impact of biorefineries, a diverse set of indicators should be used, tailored to the geopolitical and public conditions of the facility’s location. This should include aspects, such as fair working conditions, contributions to community development, inclusion of minority groups, transparency, and responsibility for end-of-life management. However, to the best of our knowledge, no studies have been reported so far on the social impact assessment of BW valorization technologies.
6 Addressing the challenges of BW valorization
The valorization process of BW encounters challenges in establishing a robust commercial process, with contamination posing a significant hurdle. The fermentation process employed in BW valorization becomes vulnerable to contamination when BW is mixed with other types of waste. To tackle this issue, it is crucial to establish a systematic distribution scheme for managing BW collection system would prove advantageous for sourcing BW, minimizing the risk of contamination, and ensuring the success of the fermentation process.
Collecting segregated BW from various supply chains can be challenging, particularly when it comes to households, which generate substantial amounts of BW. The complexity and cost of waste separation make households less feasible as a source (Kumar et al., 2022). Instead, surplus bread collected from places, such as bakeries and retailers, could be good sources of segregated BW. In some European countries, a take-back agreement (TBA) service scheme has been implemented to prevent a significant increase in BW. Under this scheme, bakeries take responsibility for their unsold goods and their collection and treatment (Soni et al., 2022). This approach establishes a return system between retailers and bakeries, which creates a contamination-free flow with other food waste products, minimizing challenges for separation (Brancoli et al., 2019, 2020). The reverse supply chain created by this scheme compels bakeries to forecast bread stock, thereby reducing waste and maintaining shelf life. Furthermore, it facilitates the segregation of uncontaminated BW, opening up opportunities for its valorization in other processes. Meanwhile, Bhardwaj et al. (2023) proposed a strategic approach which concerns the issue of BW returned from retailers under the TBA scheme as well as maintaining the quality of returned BW and creating a hygienic environment. The study involved five Indian bakery manufacturers (A, B, C, D, E) implementing BW reduction strategies. The results revealed that returning BW to the manufacturing after 3 days instead of 6 days reduced the number of expired bread and improved the quality of BW, which could be used for making other products, such as breadcrumbs, rusk, pastry, and other baked items. Furthermore, companies A and E effectively encouraged retailers to reduce waste by offering discounts on bill invoices and offering affordable bread portion to attract customers on limited budget. Additionally, they focused on targeting small towns and villages, collaborating closely with retailers to increase bread sales. This approach not only reduced waste but also helped mitigate business losses.
Although the TBA scheme is an effective approach in reducing bread surplus and bakery waste, it presents challenges in terms of transportation and environmental sustainability. The transportation of BW can lower the environmental benefits of BW valorization and poses challenges in handling large quantities that require prompt transportation to the fermentation site. If not processed promptly, bread can decay, creating an environmental hazard, attracting rodents, and rendering it unsuitable as a feedstock (Narisetty et al., 2021). This issue has been studied by Weber et al. (2023), who examined the impact of the TBA system on GWP and evaluated alternative bread supply chain scenarios. They found that long-distance transport was the main contributor to climate impact, accounting for 68% of the overall impact. Short-distance delivery and waste transportion contributed 26% and 6%, respectively. The study identified a collaborative transport strategy as the most effective approach, reducing the reliance on small vehicles and decreasing transport distances. Addressing issue of logistic for BW valorization is essential to reduce production cost. On top of that, López-Avilés et al. (2019) investigated the impact of re-distributed manufacturing (RDM) on the energy consumption and emissions in the UK’s bread supply chain. RDM presented economic and political advantages, with potential for energy reduction. The use of low carbon vehicles, local bakery purchases, and optimized manufacturing technologies contributed to decreased energy consumption and emissions. The research emphasized the importance of localizing bread production for environmental and socio-economic benefits. While centralized industrial production might increase energy use, RDM had the potential to mitigate overall energy consumption, especially in transportation. The study also highlighted the role of technological advancements in smaller-scale operations and suggests additional benefits, such as energy recovery from food waste, though these aspects were not thoroughly explored.
7 Future strategic approach of BW valorization
The problem of food waste generation demands an innovative and comprehensive approach that involves the integration of sustainable biorefinery systems. Such an approach should encompass knowledge from various disciplines to develop sustainable solutions for promoting sustainable diets, repurposing food waste, and promoting circularity in food value chains (Sadhukhan et al., 2020). The configuration of a sustainable biorefinery should aim to produce bio-products in conjunction with bioenergy and biofuel (Sadhukhan et al., 2018).
Fermentation, carried out by bacteria, yeast, fungi, and other microorganisms, is the process of decomposing organic substrates into valuable products. However, the fermentation process generates a significant amount of sludge and CO2 as by-products. The by-products can be utilized and transformed into valuable products, adding economic value in the process. The recovery strategy primarily focuses on the recovery of sludge waste by producing biogas as fuel for energy generation (steam and electricity). This is achieved through a process called AD, which breaks down waste into methane for energy and bio-fertilizer. Several studies have demonstrated the potential biogas production from various food waste sources, such as BW 421 ± 10 mL/g vs. (Narisetty et al., 2022a), fruit waste 285.7 mL/g COD (Ambaye et al., 2020), mixed food waste 450–500 mL/g vs. (Shamurad et al., 2020), citrus peel 318–500 L/kg vs. (Martínez et al., 2018), and walnut shell 492–600 mL/g vs. (Linville et al., 2017).
The biogas production from the AD process can be integrated with heat recovery steam generation (HRSG) to generate steam and electricity. HRSG allows recovery of heat from the exhaust gas from turbine, which can be utilized to produce steam at temperatures reaching approximately 650°C and pressures ranging from 13 to 20 MPa (Rackley, 2017). Additionally, some fermentative processes yields carbon dioxide (CO2) by-product, which can be utilized in the food industry as supplements due to its high purity (Rodin et al., 2020). High-quality CO2 can also be utilized in CC applications to produce various products, such as SA (Gadkari et al., 2021), cryogenic-based CO2 (Song et al., 2019), methanol (Cordero-Lanzac et al., 2022), urea (Chehrazi and Moghadas, 2022), dimethyl carbonate (Kontou et al., 2022), and dimethyl ether (Michailos et al., 2019). CCU technologies not only help mitigate climate change but also open up new synthesis routes with possible economic benefits. Figure 5 illustrates the integration strategy of BW valorization, including the production of biochemical products, such as bioethanol and SA, AD, HRSG, and methanol as CO2 upgrading process.
Based on the abovementioned strategy, future studies could be focused on the integration and energy efficiency of BW valorization. For commercialization and industrialization, the process of valuable product production from BW should be optimized to minimize energy consumption. This aim can be achieved through integration process of BW valorization. Applying process integration results in better environmental and economic aspect (Almada et al., 2023). Solomou et al. (2022) demonstrated the potential of utilizing arabinoxylan (AX) fractions extracted from sugarcane bagasse as ingredients in bread. The findings emphasized that integrated biorefineries offer opportunities to introduce novel ingredients to the food industry that were currently unavailable. The study suggested the feasibility of commercially producing a range of AX products through integration with bioethanol production, utilizing ethanol for the precipitation of AX. Sheppard et al. (2019) investigated the potential benefits of co-locating a food product, specifically coffee bean roasting, with the lignocellulosic biorefining of its by-product, spent coffee grounds (SCG). The analysis suggested that combining coffee been roasting and SCG processing could lead to financial gains and improved energy efficiency, contributing to a substantial increase in site income. Martinez-Hernandez et al. (2018) presented the crucial role of process integration in designing integrated biorefineries for retrofitting, involving the co-production of bioethanol, DDGS, AX, and AXOS precipitated at higher ethanol concentrations. The study revealed that the mass pinch analysis proved effective in retrofitted bioethanol-producing biorefineries, minimizing fresh, high purity bioethanol use and reducing losses, strengthening the technical and economic foundations for producing various arabinoxylan-based products.
Furthermore, the sustainability assessment in terms of economic, environmental, and social aspect are factors that need to be incorporated to develop integrated BW valorization. It is generally assumed that the BW valorization is expected to bring economic, environmental, and social benefits. The use of BW as feedstock for producing biochemical and biofuel products pose a huge potential to create circular bioeconomy.
8 Conclusion
Recent studies have demonstrated the potential of BW as a valuable resource for producing marketable building block chemicals, thereby supporting the advancement of the circular bioeconomy. However, the successful implementation of this approach relies heavily on the technological readiness of BW biorefineries. Integrated sustainable biorefinery systems is critical for an energetically viable scheme, as they must effectively produce bio-products alongside bioenergy.
The adoption of BW biorefinery technology is facing several key challenges related to cost-effectiveness, efficiency, environmental sustainability, and social acceptability. In order to achieve sustainable development, it is essential to conduct a comprehensive sustainability evaluation that encompasses economic, environmental, and social factors. By addressing these multifaceted considerations, the utilization of BW in biorefineries has the potential to contribute significantly to a more sustainable and resource-efficient future.
Author contributions
RHH: Conceptualization, Data curation, Formal analysis, Methodology, Writing – original draft, Writing – review & editing. JM: Data curation, Methodology, Writing – original draft, Writing – review & editing. MU: Data curation, Methodology, Writing – original draft, Writing – review & editing. VK: Methodology, Writing – review & editing. VN: Methodology, Writing – review & editing. SKM: Data curation, Methodology, Writing – review & editing. JS: Data curation, Methodology, Project administration, Writing – review & editing, Supervision. SG: Conceptualization, Data curation, Formal analysis, Funding acquisition, Investigation, Methodology, Project administration, Resources, Supervision, Writing – review & editing.
Funding
The author(s) declare financial support was received for the research, authorship, and/or publication of this article. SG acknowledged the financial support from the Natural Environment Research Council (NERC) UK project (grant no. NE/W003627/1).
Conflict of interest
The authors declare that the research was conducted in the absence of any commercial or financial relationships that could be construed as a potential conflict of interest.
The author(s) declared that they were an editorial board member of Frontiers, at the time of submission. This had no impact on the peer review process and the final decision.
Publisher’s note
All claims expressed in this article are solely those of the authors and do not necessarily represent those of their affiliated organizations, or those of the publisher, the editors and the reviewers. Any product that may be evaluated in this article, or claim that may be made by its manufacturer, is not guaranteed or endorsed by the publisher.
References
Adessi, A., Venturi, M., Candeliere, F., Galli, V., Granchi, L., and De Philippis, R. (2018). Bread wastes to energy: sequential lactic and photo-fermentation for hydrogen production. Int. J. Hydrog. Energy 43, 9569–9576. doi: 10.1016/j.ijhydene.2018.04.053
Albonetti, S., Hu, C., and Saravanamurugan, S. (2022). Preface to special issue on green conversion of HMF. ChemSusChem 15:e202201057. doi: 10.1002/cssc.202201057
Almada, D. P., Galán-Martín, Á., Gámez, M. D. M. C., and Galiano, E. C. (2023). Integrated techno-economic and environmental assessment of biorefineries: review and future research directions. Sustain Energy Fuel 7, 4031–4050. doi: 10.1039/D3SE00405H
Ambaye, T. G., Rene, E. R., Dupont, C., Wongrod, S., and van Hullebusch, E. D. (2020). Anaerobic digestion of fruit waste mixed with sewage sludge Digestate biochar: influence on biomethane production. Front. Energy Res. 8:31. doi: 10.3389/fenrg.2020.00031
Andrews, E. S. (2009). Guidelines for social life cycle assessment of products: social and socio-economic LCA guidelines complementing environmental LCA and life cycle costing, contributing to the full assessment of goods and services within the context of sustainable development. Geneva, Switzerland: UNEP/Earthprint.
Aristizábal-Marulanda, V., Solarte-Toro, J. C., and Cardona Alzate, C. A. (2020). Economic and social assessment of biorefineries: The case of Coffee Cut-Stems (CCS) in Colombia. Bioresour. Technol. Rep. 9:100397. doi: 10.1016/j.biteb.2020.100397
Ben Rejeb, I., Charfi, I., Baraketi, S., Hached, H., and Gargouri, M. (2022). Bread surplus: a cumulative waste or a staple material for high-value products? Molecules 27:8410. doi: 10.3390/molecules27238410
Bhardwaj, A., Soni, R., Singh, L. P., and Mor, R. S. (2023). A simulation approach for waste reduction in the bread supply chain. Logistics 7:2. doi: 10.3390/logistics7010002
Brancoli, P., Bolton, K., and Eriksson, M. (2020). Environmental impacts of waste management and valorisation pathways for surplus bread in Sweden. Waste Manag. 117, 136–145. doi: 10.1016/j.wasman.2020.07.043
Brancoli, P., Gmoser, R., Taherzadeh, M. J., and Bolton, K. (2021). The use of life cycle assessment in the support of the development of fungal food products from surplus bread. Fermentation 7:173. doi: 10.3390/fermentation7030173
Brancoli, P., Lundin, M., Bolton, K., and Eriksson, M. (2019). Bread loss rates at the supplier-retailer interface – analysis of risk factors to support waste prevention measures. Resour. Conserv. Recycl. 147, 128–136. doi: 10.1016/j.resconrec.2019.04.027
Brandão, A. S., Gonçalves, A., and Santos, J. M. R. C. A. (2021). Circular bioeconomy strategies: from scientific research to commercially viable products. J. Clean. Prod. 295:126407. doi: 10.1016/j.jclepro.2021.126407
Bräutigam, K.-R., Jörissen, J., and Priefer, C. (2014). The extent of food waste generation across EU-27: different calculation methods and the reliability of their results. Waste Manag. Res. 32, 683–694. doi: 10.1177/0734242X14545374
Burke, D. T., Hynds, P., and Priyadarshini, A. (2023). Quantifying farm-to-fork greenhouse gas emissions for five dietary patterns across Europe and North America: a pooled analysis from 2009 to 2020. Resourc. Environ. Sustain. 12:100108. doi: 10.1016/j.resenv.2023.100108
Caldeira, C., Vlysidis, A., Fiore, G., De Laurentiis, V., Vignali, G., and Sala, S. (2020). Sustainability of food waste biorefinery: a review on valorisation pathways, techno-economic constraints, and environmental assessment. Bioresour. Technol. 312:123575. doi: 10.1016/j.biortech.2020.123575
Chandel, A. K., Garlapati, V. K., Jeevan Kumar, S. P., Hans, M., Singh, A. K., and Kumar, S. (2020). The role of renewable chemicals and biofuels in building a bioeconomy. Biofuels Bioprod. Biorefin. 14, 830–844. doi: 10.1002/bbb.2104
Chari, S., Sebastiani, A., Paulillo, A., and Materazzi, M. (2023). The environmental performance of mixed plastic waste gasification with carbon capture and storage to produce hydrogen in the U.K. ACS Sustain. Chem. Eng. 11, 3248–3259. doi: 10.1021/acssuschemeng.2c05978
Chehrazi, M., and Moghadas, B. K. (2022). A review on CO2 capture with chilled ammonia and CO2 utilization in urea plant. J. CO2 Utiliz. 61:102030. doi: 10.1016/j.jcou.2022.102030
Collotta, M., Champagne, P., Tomasoni, G., and Mabee, W. (2022). “Life cycle approach for the sustainability assessment of intensified biorefineries” in Biofuels and biorefining. (Netherlands: Elsevier), 389–404.
Cordero-Lanzac, T., Ramirez, A., Navajas, A., Gevers, L., Brunialti, S., Gandía, L. M., et al. (2022). A techno-economic and life cycle assessment for the production of green methanol from CO2: catalyst and process bottlenecks. J. Energy Chem. 68, 255–266. doi: 10.1016/j.jechem.2021.09.045
Cox, R., Narisetty, V., Nagarajan, S., Agrawal, D., Ranade, V. V., Salonitis, K., et al. (2022). High-level fermentative production of lactic acid from bread waste under non-sterile conditions with a circular biorefining approach and zero waste discharge. Fuel 313:122976. doi: 10.1016/j.fuel.2021.122976
de Sadeleer, I., Brattebø, H., and Callewaert, P. (2020). Waste prevention, energy recovery or recycling - directions for household food waste management in light of circular economy policy. Resour. Conserv. Recycl. 160:104908. doi: 10.1016/j.resconrec.2020.104908
Doddapaneni, T. R. K. C., and Kikas, T. (2021). “Chapter 39 - thermochemical and biochemical treatment strategies for resource recovery from Agri-food industry wastes” in Valorization of Agri-food wastes and by-products. ed. R. Bhat (United Kingdom: Academic Press), 787–807.
Dymchenko, A., Geršl, M., and Gregor, T. (2023). Trends in bread waste utilisation. Trends Food Sci. Technol. 132, 93–102. doi: 10.1016/j.tifs.2023.01.004
Eriksson, M., Strid, I., and Hansson, P.-A. (2015). Carbon footprint of food waste management options in the waste hierarchy – a Swedish case study. J. Clean. Prod. 93, 115–125. doi: 10.1016/j.jclepro.2015.01.026
Escanciano, I. A., Santos, V. E., Blanco, Á., and Ladero, M. (2023). Bioproduction of succinic acid from potato waste. Kinetic modeling. Industr. Crops Produ. 203:117124. doi: 10.1016/j.indcrop.2023.117124
Espinoza-Orias, N., Stichnothe, H., and Azapagic, A. (2011). The carbon footprint of bread. Int. J. Life Cycle Assess. 16, 351–365. doi: 10.1007/s11367-011-0271-0
European Commission (2018). A sustainable bioeconomy for Europe: strengthening the connection between economy, society and the environment European Commission Available at: https://eur-lex.europa.eu/legal-content/EN/TXT/?uri=CELEX:52018DC0673.
FAO . (2013). Food wastage footprint impacts on natural resources. Food and Agricul- ture Organization of the United Nations. www.fao.org/3/i3347e/i3347e.pdf
Gadkari, S., Kumar, D., Qin, Z.-H., Ki Lin, C. S., and Kumar, V. (2021). Life cycle analysis of fermentative production of succinic acid from bread waste. Waste Manag. 126, 861–871. doi: 10.1016/j.wasman.2021.04.013
Gadkari, S., Narisetty, V., Maity, S. K., Manyar, H., Mohanty, K., Jeyakumar, R. B., et al. (2023). Techno-economic analysis of 2,3-Butanediol production from sugarcane bagasse. ACS Sustain. Chem. Eng. 11, 8337–8349. doi: 10.1021/acssuschemeng.3c01221
Gao, R., Li, Q., Chai, H., He, Y., Yang, Z., and Ma, C. (2023a). Synthesis of 5-Hydroxymethyl-2-furfurylamine from bread waste via two-step reaction. ACS Sustain. Chem. Eng. 11, 15315–15327. doi: 10.1021/acssuschemeng.3c04032
Gao, R., Li, Q., Di, J., Li, Q., He, Y.-C., and Ma, C. (2023b). Improved 5-hydroxymethyl-2-furfurylamine production from D-fructose-derived 5-hydroxymethylfurfural by a robust double mutant aspergillus terreus ω-transaminase biocatalyst in a betaine-formic acid medium. Ind. Crop. Prod. 193:116199. doi: 10.1016/j.indcrop.2022.116199
Giraldo, J. A. P., Sanchez, M. O., Solarte-Toro, J. C., and Alzate, C. A. C. (2020). “Economic and social aspects of biorefineries” in Recent advances in bioconversion of lignocellulose to biofuels and value-added chemicals within the biorefinery concept (Netherlands: Elsevier), 199–231.
Gómez, M., and Martinez, M. M. (2023). Redistribution of surplus bread particles into the food supply chain. LWT 173:114281. doi: 10.1016/j.lwt.2022.114281
Goryńska-Goldmann, E., Gazdecki, M., Rejman, K., Kobus-Cisowska, J., Łaba, S., and Łaba, R. (2020). How to prevent bread losses in the baking and confectionery industry?—measurement, causes, management and prevention. Agriculture 11:19. doi: 10.3390/agriculture11010019
Hafyan, R. H., Mohanarajan, J., Uppal, M., Kumar, V., Narisetty, V., Maity, S. K., et al. (2024). Integrated biorefinery for bioethanol and succinic acid co-production from bread waste: techno-economic feasibility and life cycle assessment. Energy Convers. Manag. 301:118033. doi: 10.1016/j.enconman.2023.118033
Han, W., Hu, Y. Y., Li, S. Y., Li, F. F., and Tang, J. H. (2016a). Biohydrogen production from waste bread in a continuous stirred tank reactor: a techno-economic analysis. Bioresour. Technol. 221, 318–323. doi: 10.1016/j.biortech.2016.09.055
Han, W., Huang, J., Zhao, H., and Li, Y. (2016b). Continuous biohydrogen production from waste bread by anaerobic sludge. Bioresour. Technol. 212, 1–5. doi: 10.1016/j.biortech.2016.04.007
Han, W., Liu, W.-X., Yu, C.-M., Huang, J.-G., Tang, J.-H., and Li, Y.-F. (2017). BioH2 production from waste bread using a two-stage process of enzymatic hydrolysis and dark fermentation. Int. J. Hydrog. Energy 42, 29929–29934. doi: 10.1016/j.ijhydene.2017.06.221
Han, W., Xu, X., Gao, Y., He, H., Chen, L., Tian, X., et al. (2019). Utilization of waste cake for fermentative ethanol production. Sci. Total Environ. 673, 378–383. doi: 10.1016/j.scitotenv.2019.04.079
Hassan, S., Williams, G., and Jaiswal, A. (2019). Moving towards the second generation of lignocellulosic biorefineries in the EU: drivers, challenges, and opportunities. Renew. Sust. Energ. Rev. 101, 590–599. doi: 10.1016/j.rser.2018.11.041
Immonen, M. (2023). Valorization of surplus bread through bioprocessing-from waste to value. University of Helsinki, Finland: YEB.
Iris, K., Tsang, D. C., Chen, S. S., Wang, L., Hunt, A. J., Sherwood, J., et al. (2017). Polar aprotic solvent-water mixture as the medium for catalytic production of hydroxymethylfurfural (HMF) from bread waste. Bioresour. Technol. 245, 456–462. doi: 10.1016/j.biortech.2017.08.170
Jensen, J. K., and Arlbjørn, J. S. (2014). Product carbon footprint of rye bread. J. Clean. Prod. 82, 45–57. doi: 10.1016/j.jclepro.2014.06.061
Jeswani, H. K., Figueroa-Torres, G., and Azapagic, A. (2021). The extent of food waste generation in the UK and its environmental impacts. Sustain. Prod. Consumpt. 26, 532–547. doi: 10.1016/j.spc.2020.12.021
Jung, J.-M., Kim, J. Y., Kim, J.-H., Kim, S. M., Jung, S., Song, H., et al. (2022). Zero-waste strategy by means of valorization of bread waste. J. Clean. Prod. 365:132795. doi: 10.1016/j.jclepro.2022.132795
Kong, Q.-S., Li, X.-L., Xu, H.-J., and Fu, Y. (2020). Conversion of 5-hydroxymethylfurfural to chemicals: a review of catalytic routes and product applications. Fuel Process. Technol. 209:106528. doi: 10.1016/j.fuproc.2020.106528
Kontou, V., Grimekis, D., Braimakis, K., and Karellas, S. (2022). Techno-economic assessment of dimethyl carbonate production based on carbon capture and utilization and power-to-fuel technology. Renew. Sust. Energ. Rev. 157:112006. doi: 10.1016/j.rser.2021.112006
Korsaeth, A., Jacobsen, A. Z., Roer, A.-G., Henriksen, T. M., Sonesson, U., Bonesmo, H., et al. (2012). Environmental life cycle assessment of cereal and bread production in Norway. Acta Agric. Scand. A Anim. Sci. 62, 242–253. doi: 10.1080/09064702.2013.783619
Küfeoğlu, S., and Khah Kok Hong, D. (2020). Emissions performance of electric vehicles: a case study from the United Kingdom. Appl. Energy 260:114241. doi: 10.1016/j.apenergy.2019.114241
Kulak, M., Nemecek, T., Frossard, E., Chable, V., and Gaillard, G. (2015). Life cycle assessment of bread from several alternative food networks in Europe. J. Clean. Prod. 90, 104–113. doi: 10.1016/j.jclepro.2014.10.060
Kumar, V., Brancoli, P., Narisetty, V., Wallace, S., Charalampopoulos, D., Dubey, B. K., et al. (2022). Bread waste–a potential feedstock for sustainable circular biorefineries. Bioresour. Technol. 369:128449. doi: 10.1016/j.biortech.2022.128449
Kumar, V., and Longhurst, P. (2018). Recycling of food waste into chemical building blocks. Curr. Opin. Green Sustain. Chem. 13, 118–122. doi: 10.1016/j.cogsc.2018.05.012
Lam, K. F., Leung, C. C. J., Lei, H. M., and Lin, C. S. K. (2014). Economic feasibility of a pilot-scale fermentative succinic acid production from bakery wastes. Food Bioprod. Process. 92, 282–290. doi: 10.1016/j.fbp.2013.09.001
LBNet . (2017). UK top bio-based chemicals opportunities. Available at: https://www.bbnet-nibb.co.uk/resource/uk-top-bio-based-chemicals-opportunities
Leung, C. C. J., Cheung, A. S. Y., Zhang, A. Y.-Z., Lam, K. F., and Lin, C. S. K. (2012). Utilisation of waste bread for fermentative succinic acid production. Biochem. Eng. J. 65, 10–15. doi: 10.1016/j.bej.2012.03.010
Linville, J. L., Shen, Y., Ignacio-de Leon, P. A., Schoene, R. P., and Urgun-Demirtas, M. (2017). In-situ biogas upgrading during anaerobic digestion of food waste amended with walnut shell biochar at bench scale. Waste Manag. Res. 35, 669–679. doi: 10.1177/0734242X17704716
López-Avilés, A., Veldhuis, A. J., Leach, M., and Yang, A. (2019). Sustainable energy opportunities in localised food production and transportation: a case study of bread in the UK. Sustain. Prod. Consumpt. 20, 98–116. doi: 10.1016/j.spc.2019.05.004
Mailaram, S., Narisetty, V., Maity, S. K., Gadkari, S., Thakur, V. K., Russell, S., et al. (2023). Lactic acid and biomethane production from bread waste: a techno-economic and profitability analysis using pinch technology. Sustain. Energy Fuels 7, 3034–3046. doi: 10.1039/D3SE00119A
Maina, S., Prabhu, A. A., Vivek, N., Vlysidis, A., Koutinas, A., and Kumar, V. (2022). Prospects on bio-based 2,3-butanediol and acetoin production: recent progress and advances. Biotechnol. Adv. 54:107783. doi: 10.1016/j.biotechadv.2021.107783
Malode, S. J., Prabhu, K. K., Mascarenhas, R. J., Shetti, N. P., and Aminabhavi, T. M. (2021). Recent advances and viability in biofuel production. Energy Convers. Manag. 10:100070. doi: 10.1016/j.ecmx.2020.100070
Martínez, E. J., Rosas, J. G., Sotres, A., Moran, A., Cara, J., Sánchez, M. E., et al. (2018). Codigestion of sludge and citrus peel wastes: evaluating the effect of biochar addition on microbial communities. Biochem. Eng. J. 137, 314–325. doi: 10.1016/j.bej.2018.06.010
Martinez-Hernandez, E., Tibessart, A., and Campbell, G. M. (2018). Conceptual design of integrated production of arabinoxylan products using bioethanol pinch analysis. Food Bioprod. Process. 112, 1–8. doi: 10.1016/j.fbp.2018.08.005
Michailos, S., McCord, S., Sick, V., Stokes, G., and Styring, P. (2019). Dimethyl ether synthesis via captured CO2 hydrogenation within the power to liquids concept: a techno-economic assessment. Energy Convers. Manag. 184, 262–276. doi: 10.1016/j.enconman.2019.01.046
Mihajlovski, K., Rajilić-Stojanović, M., and Dimitrijević-Branković, S. (2020). Enzymatic hydrolysis of waste bread by newly isolated Hymenobacter sp. CKS3: statistical optimization and bioethanol production. Renew. Energy 152, 627–633. doi: 10.1016/j.renene.2020.01.101
Murthy, G. S. (2019). “Chapter 3 - systems analysis frameworks for biorefineries” in Biofuels: alternative feedstocks and conversion processes for the production of liquid and gaseous biofuels. eds. A. Pandey, C. Larroche, C.-G. Dussap, E. Gnansounou, S. K. Khanal, and S. Ricke. 2nd ed (United Kingdom: Academic Press), 77–92.
Nair, R. B., Eh-Hser Nay, T., Lennartsson, P. R., and Taherzadeh, M. J. (2017). Waste bread valorization using edible filamentous Fungi. EUBCE 2017–25th European Biomass Conference and Exhibition, Stockholm, Sweden, June 12–15, 2017.
Narisetty, V., Cox, R., Willoughby, N., Aktas, E., Tiwari, B., Matharu, A. S., et al. (2021). Recycling bread waste into chemical building blocks using a circular biorefining approach. Sustain. Energy Fuels 5, 4842–4849. doi: 10.1039/d1se00575h
Narisetty, V., Nagarajan, S., Gadkari, S., Ranade, V. V., Zhang, J., Patchigolla, K., et al. (2022a). Process optimization for recycling of bread waste into bioethanol and biomethane: a circular economy approach. Energy Convers. Manag. 266:115784. doi: 10.1016/j.enconman.2022.115784
Narisetty, V., Zhang, L., Zhang, J., Lin, C. S. K., Tong, Y. W., Show, P. L., et al. (2022b). Fermentative production of 2, 3-Butanediol using bread waste–a green approach for sustainable management of food waste. Bioresour. Technol. 358:127381. doi: 10.1016/j.biortech.2022.127381
Nikolaou, M., Stavraki, C., Bousoulas, Ι., Malamis, D., Loizidou, M., Mai, S., et al. (2023). Valorisation of bakery waste via the bioethanol pathway. Energy 280:128185. doi: 10.1016/j.energy.2023.128185
Notarnicola, B., Tassielli, G., Renzulli, P. A., Castellani, V., and Sala, S. (2017). Environmental impacts of food consumption in Europe. J. Clean. Prod. 140, 753–765. doi: 10.1016/j.jclepro.2016.06.080
Posada, J. A., and Osseweijer, P. (2016). “Chapter 26 - socioeconomic and environmental considerations for sustainable supply and fractionation of lignocellulosic biomass in a biorefinery context” in Biomass fractionation technologies for a lignocellulosic feedstock based biorefinery. ed. S. I. Mussatto (Netherlands: Elsevier), 611–631.
Rackley, S. A. (2017). “3 - Power generation fundamentals” in Carbon capture and storage. ed. S. A. Rackley . 2nd ed (United Kingdom: Butterworth-Heinemann), 37–71.
Rana, M. S., Bhushan, S., Prajapati, S. K., Preethi,, and Kavitha, S. (2020). “Chapter 15 - techno-economic analysis and environmental aspects of food waste management” in Food waste to valuable resources. eds. J. R. Banu, G. Kumar, M. Gunasekaran, and S. Kavitha (United Kingdom: Academic Press), 325–342.
Rodin, V., Lindorfer, J., Böhm, H., and Vieira, L. (2020). Assessing the potential of carbon dioxide valorisation in Europe with focus on biogenic CO2. J. CO2 Utiliz. 41:101219. doi: 10.1016/j.jcou.2020.101219
Rout, P. R., Goel, M., Pandey, D. S., Briggs, C., Sundramurthy, V. P., Halder, N., et al. (2023). Technological advancements in valorisation of industrial effluents employing hydrothermal liquefaction of biomass: strategic innovations, barriers and perspectives. Environ. Pollut. 316:120667. doi: 10.1016/j.envpol.2022.120667
Sadaf, A., Kumar, S., Nain, L., and Khare, S. K. (2021). Bread waste to lactic acid: applicability of simultaneous saccharification and solid state fermentation. Biocatal. Agric. Biotechnol. 32:101934. doi: 10.1016/j.bcab.2021.101934
Sadhukhan, J., Dugmore, T. I. J., Matharu, A., Martinez-Hernandez, E., Aburto, J., Rahman, P. K. S. M., et al. (2020). Perspectives on “game changer” global challenges for sustainable 21st century: plant-based diet, unavoidable food waste biorefining, and circular economy. Sustainability 12:1976. doi: 10.3390/su12051976
Sadhukhan, J., Gadkari, S., Martinez-Hernandez, E., Ng, K. S., Shemfe, M., Torres-Garcia, E., et al. (2019). Novel macroalgae (seaweed) biorefinery systems for integrated chemical, protein, salt, nutrient and mineral extractions and environmental protection by green synthesis and life cycle sustainability assessments [10.1039/C9GC00607A]. Green Chem. 21, 2635–2655. doi: 10.1039/C9GC00607A
Sadhukhan, J., Martinez-Hernandez, E., Murphy, R. J., Ng, D. K. S., Hassim, M. H., Siew Ng, K., et al. (2018). Role of bioenergy, biorefinery and bioeconomy in sustainable development: strategic pathways for Malaysia. Renew. Sust. Energ. Rev. 81, 1966–1987. doi: 10.1016/j.rser.2017.06.007
Sadhukhan, J., Ng, K. S., and Hernandez, E. M. (2014). Biorefineries and chemical processes: design, integration and sustainability analysis. United Kingdom: John Wiley & Sons.
Sadhukhan, J., Ng, K. S., and Martinez-Hernandez, E. (2016). Novel integrated mechanical biological chemical treatment (MBCT) systems for the production of levulinic acid from fraction of municipal solid waste: a comprehensive techno-economic analysis. Bioresour. Technol. 215, 131–143. doi: 10.1016/j.biortech.2016.04.030
Sadhukhan, J., Sen, S., and Gadkari, S. (2021). The mathematics of life cycle sustainability assessment. J. Clean. Prod. 309:127457. doi: 10.1016/j.jclepro.2021.127457
Shamurad, B., Sallis, P., Petropoulos, E., Tabraiz, S., Ospina, C., Leary, P., et al. (2020). Stable biogas production from single-stage anaerobic digestion of food waste. Appl. Energy 263:114609. doi: 10.1016/j.apenergy.2020.114609
Shemfe, M. B., Gadkari, S., and Sadhukhan, J. (2018). Social hotspot analysis and trade policy implications of the use of bioelectrochemical Systems for Resource Recovery from wastewater. Sustainability 10:3193. doi: 10.3390/su10093193
Sheppard, P., Garcia-Garcia, G., Angelis-Dimakis, A., Campbell, G. M., and Rahimifard, S. (2019). Synergies in the co-location of food manufacturing and biorefining. Food Bioprod. Process. 117, 340–359. doi: 10.1016/j.fbp.2019.08.001
Shoaf, T. J., and Engelberth, A. S. (2022). “Recycling of multiple organic solid wastes into chemicals via biodegradation” in Production of biofuels and chemicals from sustainable recycling of organic solid waste. eds. Z. Fang, R. L. SmithJr., and L. Xu (Singapore: Springer Nature Singapore), 205–242.
Silk, D., Mazzali, B., Gargalo, C. L., Pinelo, M., Udugama, I. A., and Mansouri, S. S. (2020). A decision-support framework for techno-economic-sustainability assessment of resource recovery alternatives. J. Clean. Prod. 266:121854. doi: 10.1016/j.jclepro.2020.121854
Sillero, L., Sganzerla, W. G., Forster-Carneiro, T., Solera, R., and Perez, M. (2022). A bibliometric analysis of the hydrogen production from dark fermentation. Int. J. Hydrog. Energy 47, 27397–27420. doi: 10.1016/j.ijhydene.2022.06.083
Slorach, P. C., Jeswani, H. K., Cuéllar-Franca, R., and Azapagic, A. (2020). Assessing the economic and environmental sustainability of household food waste management in the UK: current situation and future scenarios. Sci. Total Environ. 710:135580. doi: 10.1016/j.scitotenv.2019.135580
Solomou, K., Alyassin, M., Angelis-Dimakis, A., and Campbell, G. M. (2022). Arabinoxylans: a new class of food ingredients arising from synergies with biorefining, and illustrating the nature of biorefinery engineering. Food Bioprod. Process. 132, 83–98. doi: 10.1016/j.fbp.2021.12.007
Song, C., Liu, Q., Deng, S., Li, H., and Kitamura, Y. (2019). Cryogenic-based CO2 capture technologies: state-of-the-art developments and current challenges. Renew. Sust. Energ. Rev. 101, 265–278. doi: 10.1016/j.rser.2018.11.018
Soni, R., Bhardwaj, A., and Jarangal, L. P. S. (2022). Bread waste and mitigation strategies: a review. IOP Conf. Ser. 1248:012010. doi: 10.1088/1757-899X/1248/1/012010
Swetha, T. A., Ananthi, V., Bora, A., Sengottuvelan, N., Ponnuchamy, K., Muthusamy, G., et al. (2023). A review on biodegradable polylactic acid (PLA) production from fermentative food waste - its applications and degradation. Int. J. Biol. Macromol. 234:123703. doi: 10.1016/j.ijbiomac.2023.123703
Taglieri, I., Macaluso, M., Bianchi, A., Sanmartin, C., Quartacci, M. F., Zinnai, A., et al. (2021). Overcoming bread quality decay concerns: main issues for bread shelf life as a function of biological leavening agents and different extra ingredients used in formulation. A review. J. Sci. Food Agric. 101, 1732–1743. doi: 10.1002/jsfa.10816
Thongchul, N., Charoensuppanimit, P., Anantpinijwatna, A., Gani, R., and Assabumrungrat, S. (2022). “1 - overview of biorefinery” in A-Z of biorefinery. eds. N. Thongchul, A. Kokossis, and S. Assabumrungrat (Netherlands: Elsevier), 3–32.
Tinôco, D., Borschiver, S., Coutinho, P. L., and Freire, D. M. G. (2021). Technological development of the bio-based 2,3-butanediol process. Biofuels Bioprod. Biorefin. 15, 357–376. doi: 10.1002/bbb.2173
Tong, K. T. X., Tan, I. S., Foo, H. C. Y., Lam, M. K., Lim, S., and Lee, K. T. (2022). Advancement of biorefinery-derived platform chemicals from macroalgae: a perspective for bioethanol and lactic acid. Biomass Convers Bioref. 14, 1–37. doi: 10.1007/s13399-022-02561-7
Ubando, A. T., Felix, C. B., and Chen, W.-H. (2020). Biorefineries in circular bioeconomy: a comprehensive review. Bioresour. Technol. 299:122585. doi: 10.1016/j.biortech.2019.122585
Ünal, M. Ü., Chowdhury, G., and Şener, A. (2022). Effect of temperature and nitrogen supplementation on bioethanol production from waste bread, watermelon and muskmelon by Saccharomyces cerevisiae. Biofuels 13, 395–399. doi: 10.1080/17597269.2020.1724440
van Niekerk, A. S., and Kay, P. J. (2020). A holistic evaluation of the impact of UK renewable strategy on emissions from compression ignition engines. Fuel 271:117586. doi: 10.1016/j.fuel.2020.117586
Vanapalli, K. R., Bhar, R., Maity, S. K., Dubey, B. K., Kumar, S., and Kumar, V. (2023). Life cycle assessment of fermentative production of lactic acid from bread waste based on process modelling using pinch technology. Sci. Total Environ. 905:167051. doi: 10.1016/j.scitotenv.2023.167051
Vance, C., Sweeney, J., and Murphy, F. (2022). Space, time, and sustainability: the status and future of life cycle assessment frameworks for novel biorefinery systems. Renew. Sust. Energ. Rev. 159:112259. doi: 10.1016/j.rser.2022.112259
Vandermeersch, T., Alvarenga, R. A. F., Ragaert, P., and Dewulf, J. (2014). Environmental sustainability assessment of food waste valorization options. Resour. Conserv. Recycl. 87, 57–64. doi: 10.1016/j.resconrec.2014.03.008
Weber, L., Bartek, L., Brancoli, P., Sjölund, A., and Eriksson, M. (2023). Climate change impact of food distribution: the case of reverse logistics for bread in Sweden. Sustain. Prod. Consumpt. 36, 386–396. doi: 10.1016/j.spc.2023.01.018
Werpy, T., and Petersen, G. (2004). Top value added chemicals from biomass: volume I--results of screening for potential candidates from sugars and synthesis gas. Golden, CO, United States: National Renewable Energy Lab (NREL).
WRAP . (2021). Food surplus and waste in the UK –key facts. www.wrap.org.uk/food-drink. The Waste and Resources Action Programme.
Wu, C., Ma, C., Li, Q., Chai, H., and He, Y.-C. (2023). Efficient production of hydroxymethyl-2-furfurylamine by chemoenzymatic cascade catalysis of bread waste in a sustainable approach. Bioresour. Technol. 385:129454. doi: 10.1016/j.biortech.2023.129454
Yaashikaa, P. R., Kumar, P. S., Saravanan, A., Varjani, S., and Ramamurthy, R. (2020). Bioconversion of municipal solid waste into bio-based products: a review on valorisation and sustainable approach for circular bioeconomy. Sci. Total Environ. 748:141312. doi: 10.1016/j.scitotenv.2020.141312
Yu, I. K. M., Tsang, D. C. W., Yip, A. C. K., Hunt, A. J., Sherwood, J., Shang, J., et al. (2018). Propylene carbonate and γ-valerolactone as green solvents enhance Sn(iv)-catalysed hydroxymethylfurfural (HMF) production from bread waste [10.1039/C8GC00358K]. Green Chem. 20, 2064–2074. doi: 10.1039/C8GC00358K
Yusufoğlu, B., Yaman, M., and Karakuş, E. (2021). Glycemic evaluation of some breads from different countries via in vitro gastrointestinal enzymatic hydrolysis system. Food Sci. Technol. 42:e34920. doi: 10.1590/fst.34920
Keywords: bread waste, sustainability assessment, circular bioeconomy, platform chemicals, management waste, biorefinery, food waste valorization
Citation: Hafyan RH, Mohanarajan J, Uppal M, Kumar V, Narisetty V, Maity SK, Sadhukhan J and Gadkari S (2024) Bread waste valorization: a review of sustainability aspects and challenges. Front. Sustain. Food Syst. 8:1334801. doi: 10.3389/fsufs.2024.1334801
Edited by:
Shalini Gaur Rudra, Indian Agricultural Research Institute, IndiaReviewed by:
Poonam Singh, LaserLeap Technologies, PortugalDillirani Nagarajan, National Cheng Kung University, Taiwan
Copyright © 2024 Hafyan, Mohanarajan, Uppal, Kumar, Narisetty, Maity, Sadhukhan and Gadkari. This is an open-access article distributed under the terms of the Creative Commons Attribution License (CC BY). The use, distribution or reproduction in other forums is permitted, provided the original author(s) and the copyright owner(s) are credited and that the original publication in this journal is cited, in accordance with accepted academic practice. No use, distribution or reproduction is permitted which does not comply with these terms.
*Correspondence: Siddharth Gadkari, cy5nYWRrYXJpQHN1cnJleS5hYy51aw==