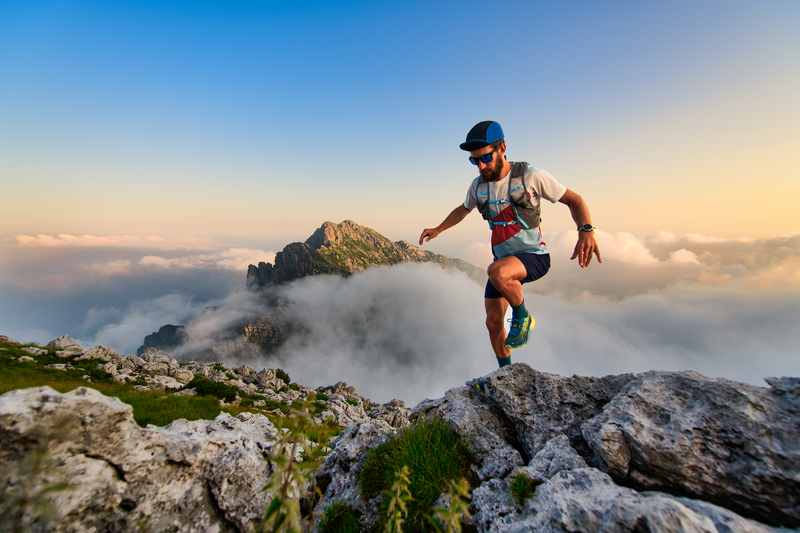
95% of researchers rate our articles as excellent or good
Learn more about the work of our research integrity team to safeguard the quality of each article we publish.
Find out more
ORIGINAL RESEARCH article
Front. Sustain. Food Syst. , 23 January 2024
Sec. Waste Management in Agroecosystems
Volume 8 - 2024 | https://doi.org/10.3389/fsufs.2024.1326304
This article is part of the Research Topic Circular Economy and Food Systems: Challenges and Opportunities in Waste Management, with Reference to Developing Economies View all 8 articles
Introduction: Considering the valuable organic fraction contained, restaurant food waste (RFW) has attracted more attention as an alternative substrate for animal feed production. In this work, a new enzyme-fermentation process (EFP) for diverting RFW into synbiotic animal feed was developed, and its economic and environmental benefits were evaluated.
Methods: The process initiated with enzymatic hydrolysis of RFWs, intending to convert starch into isomaltooligosaccharides (IMOs) via simultaneous saccharification and transglycosylation (SST). Subsequently, the hydrolysate underwent fermentation with engineered Pichia pastoris GSL to form L-lactic acid (L-LA) from the free glucose and to biologically enhance the nutritional value.
Results and discussion: The results indicated that employing the EFP yielded the highest IMOs levels, ranging from 17.10–38.00 g/L. Simultaneously, the process achieved the maximum L-LA concentration (20.75–27.16 g/L), with a conversion efficiency of 0.64–0.78 g/g. Additionally, 5.0–8.5 g/L of yeast biomass was generated. Economic estimates elucidated that the cost of RFW-derived animal feed through EFP was about $0.16/kg, signifying a substantial cost reduction (≥ 70%) compared to traditional feeds. Achieving complete conversion of RFW into animal feed while eliminating residual waste highlights the significant environmental benefits and the compatibility of the present technology with the zero-waste concept.
Globally, about 1.3 billion tons of food waste is generated annually, with restaurant food waste (RFW) contributing to nearly 40% of total waste (FAO, 2011; Nasser et al., 2018). RFW is a putrescible organic waste that mainly consists of uneaten food and unusable leftovers (Chen et al., 2015; Garnida et al., 2022) and is associated with adverse health, socioeconomic, and environmental impacts due to its high moisture content and perishability (Ojha et al., 2020; Sobol et al., 2023). Nevertheless, RFW also contains valuable ingredients such as carbohydrates, protein, fats, vitamins, fiber, and minerals, making it a promising source of nutrients (Noori et al., 2022). Therefore, there is a critical need to recycle and resource RFW to create value from it in an ecofriendly and economical way.
Considering its rich nutrient profile, feeding animals with RFW provides a viable substitute for conventional feeds, such as corn and soybeans, promoting resource conservation and mitigating the environmental impact of RFW (Chen et al., 2015). Circular integration of RFW as livestock feed by ranchers not only enhances profitability but also minimizes ecological repercussions associated with feed crop production, including greenhouse gas (GHG) emissions, chemical input, land utilization, energy, and water consumption (Nath et al., 2023). The transition from traditional feeds to feed production based on RFW offers a safer, more economical, and environmentally friendly alternative (Zhao et al., 2017). Although RFW is a nutrient-dense feedstock capable of producing a balanced animal feed, the heterogeneous nature and high moisture content are the main challenges to its conversion. The concerns regarding the feed’s nutritional value, contamination risks, and conversion techniques have hindered its market acceptance (Suwannarat and Ritchie Ajila, 2015; Sindhu et al., 2019). Additionally, unprocessed RFW is characterized by a lower nutritional density and higher fiber content, adversely affecting digestibility, energy availability, and its overall applicability within the feed industry (Ajila et al., 2012).
To address these concerns, modern feed systems highly favor biological treatments and prioritize natural feed additives, such as enzymes, prebiotics, probiotics, and organic acids, to ameliorate feed quality and enhance animal health and productivity (Mladenovic et al., 2019; Carranza-Mendez et al., 2022). Isomaltooligosaccharides (IMOs) are a well-known prebiotic produced from various starch sources through an enzymatic process, which is highly preferred over chemical methods due to its selectivity, efficiency, and sustainability (Niu et al., 2017; Tiangpook et al., 2023). IMOs display bifidogenic effects, thus promoting gut microbiota and intestinal fermentation (Plongbunjong et al., 2017; Mistry et al., 2020). These prebiotics enhance cell-mediated and humoral immunity, improve mineral absorption and lipid metabolism, increase antioxidant capacity, and reduce cholesterol (Li et al., 2010; Wang et al., 2016).
Furthermore, the biological processes optimize the hydrolysis of complex carbohydrates in RFW, converting them into water-soluble sugars that can be transformed into value-added products (Hafid et al., 2017). Microbial fermentation is a promising approach that improves the nutritional quality of RFW-derived feed by reducing antinutritional compounds and enhancing sensory characteristics (Yang et al., 2006; Wang et al., 2018; Du et al., 2021). The incorporated microorganisms (probiotics) facilitate the nutrient flow throughout the gastrointestinal tract, improving feed utilization, absorption, and digestibility (Pang et al., 2018). The technological additives, such as L-lactic acid (L-LA), generated during fermentation, lower the pH to crowd out pathogens of importance for livestock animals, preserve feed through acidification, and decrease animal mortality rates (Suiryanrayna and Ramana, 2015; Yousuf et al., 2018). L-LA also facilitates the extraction of bioactive compounds from RFW and significantly influences both the nutritional and organoleptic characteristics of animal feed, which alters the gastrointestinal attributes, thus promoting livestock health (Rollán et al., 2019; Azimonti et al., 2022). In addition, microbial biomass, particularly yeast, generated during fermentation, is a rich natural source of organic nutrients and is widely utilized as a valuable ingredient in feed formulations (Werten et al., 2019).
The present study aims to introduce a novel enzyme-fermentation process (EFP) to efficiently convert RFW into synbiotic feed fortified with IMOs, L-LA, and yeast biomass. The study utilized an enzyme cocktail for IMOs synthesis and investigated the effects of various parameters. Afterwards, a recombinant Pichia pastoris strain capable of producing L-LA was developed and employed in L-LA production from free glucose in the hydrolysate. The effects of operation on L-LA production, IMOs concentration, yeast biomass, and nutritional value were thoroughly assessed. The study presents an innovative and sustainable methodology for achieving a comprehensive closed-loop system and an effective recycling process for RFW management.
RFWs (R1–R6) were collected from local restaurants at Tianjin University of Science and Technology, Tianjin, China. First, clamshells, papers, toothpicks and chopsticks, animal bones and other inorganic materials were manually screened out. The sorted RFWs mostly comprised rice, noodles, potatoes, meat, fruit, and vegetable waste. Thereafter, RFWs were crushed using an electric blender (JYL-C012, Joyoung Co., Ltd., China) to obtain a homogenized slurry (Hafid et al., 2017) and stored at −20°C for a maximum of one month. Before use, samples were thawed overnight at 5°C.
To facilitate IMOs preparation from RFWs, an enzyme cocktail consisting of Bacillus amyloliquefaciens α-amylase (10,000 U/g), barley bran β-amylase (500 oDP/g), Bacillus naganoensis pullulanase (1,000 U/g), and Aspergillus niger transglucosidase (500,000 U/g) (Jiangsu Ruiyang Biotech, China) was developed according to the previous work (Niu et al., 2017). For starch liquefaction, thermophilic α-amylase from B. licheniformis (40,000 U/mL) was selected.
Experiments were performed in 500-mL Erlenmeyer flasks containing 100 g of blended RFWs, diluted to a final reaction volume of 100 mL with distilled water. Initially, RFWs were adjusted to pH 5.5–6.0 and subjected to liquefaction using thermophilic α-amylase with an initial enzyme dosage of 200 U/g (w/w, on a dry basis of starch) at 80°C and 100 rpm for about 1 h. Samples were collected periodically to analyze the residual starch using KI/I2 solution (Basu and Prapulla, 2016). Subsequently, IMOs synthesis from the liquefied starch was carried out through a one-step simultaneous saccharification and transglycosylation (SST) process as described by Niu et al. (2017), with slight modifications. For all the experiments performed, the enzyme cocktail at a dosage of 0.1% (w/w, dried starch basis) was added. The SST process was carried out at 58–60°C, pH 5.8, and 100 rpm for 6 h. Samples withdrawn at regular intervals were mixed with 3 mol/L HCl to terminate the reaction and were then centrifuged at 4,000 × g for 5 min. The supernatant was stored at −20°C for subsequent determination of the contents of IMOs and sugar components (see Section 2.5).
The effect of the percentage of total solid (TS) content on IMOs synthesis was studied. Initially, RFW–R1 was selected to investigate various parameters, and the optimized conditions were then applied to further samples. This sample was categorized into three groups based on different TS content: 11.00, 16.00, and 24.25%. The latter was the maximum possible TS content in the RFWs used in this study (see Supplementary Table S1). To attain TS content of 11.00, 16.00, and 24.25%, a fixed amount of 100 g RFW slurry was added to flasks and then diluted with varying amounts of distilled water. The resulting total solid concentrations for the respective 11.00, 16.00, and 24.25% TS solutions were determined to be 165 g TS/L, 216 g TS/L, and 254 g TS/L, respectively. The enzymatic treatment was performed as described above.
The enzymatic hydrolysis of RFWs was carried out, as discussed in Section 2.2.1. The determination of dextrose equivalent (DE) values was carried out during the starch liquefaction step, as previously described (Niu et al., 2017). Unhydrolyzed RFWs were used as the experimental control, and the levels of IMOs and glucose were assessed for comparison.
To evaluate the effect of SST duration on IMOs synthesis, a single factor experiment was conducted using RFW–R1. The reaction temperature, pH, and enzyme cocktail were maintained at 58°C, 5.8, and 0.1% (w/w), respectively. After starch liquefaction, the experiment was conducted for varying reaction times (0–6 h). Following the determination of the optimal SST duration, the same conditions were applied to other RFWs, and the IMOs contents were evaluated.
A recombinant P. pastoris expressing Bacillus coagulans L-lactate dehydrogenase (L-LDH) was developed using conventional cloning techniques (Sambrook et al., 1989). In brief, an integration expression vector pPGK901 was first constructed by recombining a 2.4 kb pUC-BglII from pPIC9k, a 640 bp PPGK promoter from P. pastoris GS115, and a 300 bp CYC1t terminator from Saccharomyces cerevisiae 303A. A 942 bp ldh gene encoding L-LDH of B. coagulans (Niu et al., 2014) was subsequently cloned into the BamHI and SmaI sites of pPGK901 to create the plasmid pPGK.BcoaLDH. A 1.9 kb ldh expression cassette, including the promoter PPGK, ldh, and the terminator CYC1t, was recovered and inserted into the SmaI site of pPIC9k to yield a 11.5 kb plasmid pPIC-BcoaLDH. It was then linearized by SalI, electroporated, and integrated by homologous recombination into the chromosome of P. pastoris GS115 following the Easy Select P. pastoris Expression kit protocol (Invitrogen, United States). Finally, the resulting strain was obtained and designated as P. pastoris GSL (Supplementary Figure S1).
Inoculum cultures of P. pastoris GSL were prepared in 250 mL Erlenmeyer flasks containing 30 mL YPD broth (10 g/L yeast extract, 20 g/L peptone, and 20 g/L D-glucose). After inoculating with single colonies, the flasks were incubated at 30°C and 200 rpm overnight. Yeast cells were harvested by centrifugation at 4000 × g for 10 min, washed twice, and resuspended in sterile water to serve as a starter culture for L-LA fermentation. The L-LA productivity of the recombinant yeast was first evaluated in 500 mL shake flasks containing 100 mL of BMDY medium (YPD + 13.4 g/L YNB, 0.2 g/L biotin, and 50 mmol/L phosphate buffer, pH 6.0) at 30°C and 200 rpm for up to 60 h. Afterwards, L-LA fermentation was conducted in a 5-L bioreactor (Baoxing, Biological Equipment, Shanghai, China) with a 2.5-L BMDY medium. Samples were withdrawn at regular intervals. The supernatant was recovered by centrifugation and stored at −20°C for subsequent analysis.
The IMOs-enriched RFW hydrolysates (Section 2.2.1) served as the fermentation substrates. The fermentation experiments were performed in triplicate. The freshly prepared resuspended culture of P. pastoris GSL was inoculated into flasks to obtain a 3.6 × 105 colony forming unit (CFU)/mL of the cell population. Fermentations were conducted at 30°C, pH 5.5 and 200 rpm for 48 h. Samples were collected at certain intervals, and the supernatant was isolated by centrifugation at 4,000 × g for 5 min and stored at −20°C for later analysis.
The chemical composition of ground RFWs was initially assessed (see Supplementary Table S1). The protein content as the total nitrogen was estimated by the Kjeldahl method and multiplied by a factor of 6.25. Crude lipid was measured as reported earlier (Hafid et al., 2017). The ash content was quantified by incineration of a sample of known weight at 600°C for 2.5 h and weighting of the residues. To determine the amount of total starch, the enzymatic hydrolysis method specified in the Chinese National Standard (GB 5009.9–2016) was used (Zhang et al., 2022). Free phosphorus was measured using the malachite green assay method (Rahutomo et al., 2019). The free amino acids (FAAs) content was determined using a 4% ninhydrin assay (Navarrete-del-Toro and García-Carreño, 2003).
The contents of IMOs and the sugar profiles were analyzed using high performance liquid chromatography (HPLC) as previously described (Niu et al., 2017). Briefly, the HPLC system (Agilent 1,200 Series) was equipped with a refractive index detector (RI-201H, SHOKO, Science Co. Ltd., Yokohama, Japan) and a Prevail Carbohydrate J16Q0033 column (4.6 mm internal diameter (ID) × 250 mm, 5 μm, Tosoh, Japan). The analysis was performed using acetonitrile water (65:35, v/v) as solvent at a flow rate of 1 mL/min at 35°C. Isomaltose (IG2), isomaltotriose (IG3), and panose (P) used as HPLC standards were obtained from Sigma (St. Louis, MO, United States). The sugar standards (glucose and maltose) were purchased from Shanghai BioTech Co. Ltd., China. The IMOs content (%, w/w) was calculated as a percentage of the total amounts of IG2, IG3, and P in the dried matter (Niu et al., 2017).
The L-LA content in the fermentation broths was quantified by HPLC as previously described (Niu et al., 2014).
The phenolic compounds were identified and quantified by HPLC (Chen et al., 2021), while the total phenolic content (TPC) was determined using the Folin–Ciocalteu method (Shao et al., 2014), and total flavonoid content was measured as previously reported (Bakar et al., 2009). The antioxidant activities were evaluated by the 2,2-diphenyl-1-picrylhydrazyl (DPPH) radical-scavenging assay (Chen et al., 2021), 2,2-azino-bis-(3-ethylbenzothiazoline-6-sulphonic acid) (ABTS+) radical cation method (Pang et al., 2018), and the ferric reducing antioxidant power (FRAP) assay (Liao et al., 2012). The antioxidant reference (Trolox) was purchased from Shanghai BioTech Co. Ltd., China, and results were expressed as mM Trolox equivalents (TE) per 100 g RFW.
The viable cell count (CFU/mL) was manually enumerated from 0.1 mL serially diluted fermentation samples on YPD agar plates and incubated at 30°C for 48 h (Wang et al., 2021). To determine the dry cell weight, yeast cells were dried at 70°C to a constant weight. The nucleic acid content was quantified as previously reported (Zhang et al., 2023).
All other chemicals or reagents not mentioned were of analytical research grade and purchased from Shanghai BioTech Co. Ltd., China.
The effects of TS concentration on IMOs production were evaluated using RFW–R1 as the core sample. For 11.00% TS content, the IMOs concentration reached 11.55 g/L, with corresponding IG2, IG3, and P concentrations of 8.25 g/L, 2.01 g/L, and 1.29 g/L, respectively. Increasing the TS content to 16.00% resulted in the final concentration of IMOs reaching 20.22 g/L. The values recorded for IG2, IG3 and P were 12.12 g/L, 5.87 g/L, and 2.23 g/L, respectively. The impact of increasing the TS content from 11.00 to 16.00% represented a 75% increase in IMOs concentration compared to the initial level. Conversely, at 24.25% TS content, the IMOs level reached 38 g/L, with IG2, IG3, and P concentrations achieving 21.09 g/L, 10.46 g/L, and 6.45 g/L, respectively. Elevating the TS content from 16.00 to 24.25% resulted in a significant 88% increase in IMOs concentration. The total yield of IMOs from RFW analyzed at 11.00, 16.00, and 24.25% TS was 0.07, 0.09, and 0.14 g product/g TS. These findings are consistent with Yousuf et al. (2018) research, as we observed that increasing TS positively impacted the concentration and yield of the resulting product. However, these results contradict previous literature (Staley et al., 2011), which suggested that higher solid content leads to depletion zones at the microscopic level, causing lower substrate degradation and conversion rates. Based on the obtained results, our study employed higher available TS (20 to 22%) for the bio-treatment of other RFWs (see Supplementary Table S1), which can potentially enhance production processes, making them more efficient and sustainable.
Enzymatic hydrolysis is a promising pretreatment technique that operates under mild environmental conditions to enhance the substrate’s digestibility and increase the final product’s yield (Han et al., 2016). Figure 1 illustrates the IMOs synthesis from RFWs through a one-step enzymatic hydrolysis process. Initially, the degree of starch liquefaction was determined, resulting in DE values ranging from 12 to 16. It is worth noting that the increased DE value shortens the transglycosylation duration and maximizes the yield of IMOs (Niu et al., 2017). Subsequently, the enzyme cocktail degraded the liquefied starch, resulting in the formation of α-(1,4)-linked α-D-glucooligosaccharides, which were then converted into α-(1,6)-oligosaccharides (IMOs) through transglycosylation activity. The maximum concentration of IMOs in hydrolyzed RFWs (R1, R2, R3, R4, R5, and R6) reached 38 g/L, 17.06 g/L, 33.52 g/L, 18.60 g/L, 17.50 g/L, and 21.91 g/L, respectively. Starch conversion rates to IMOs measured 23, 18, 25, 18, 17, and 20% (w/w). The yield of IMOs per gram of RFWs ranged between 0.09 to 0.16 g. In contrast, untreated RFWs exhibited relatively low concentrations of IMOs (Figure 1), glucose, and maltose (Supplementary Table S2), with values ranging from 1.03 to 1.98 g/L, 1.71 to 4.26 g/L, and 1.06 to 4.46 g/L, respectively. The presence of sugars in the control groups can be attributed to either their inherent presence or their release due to the activity of naturally occurring microorganisms, resulting in alterations in the nutritional and biochemical characteristics of RFWs (Kim et al., 2021). Studies (Cho et al., 2014; Tiangpook et al., 2023) have demonstrated that deploying microorganisms engineered to directly express the requisite enzymes for IMOs synthesis results in lower IMOs output than enzymatic hydrolysis. This reduction is due to an imbalance in the microbial population, which hinders key biochemical reactions, reducing IMOs yield. Our findings demonstrate that enzymatic hydrolysis significantly improved the concentration of IMOs, highlighting the potential of this approach for valorizing RFWs and obtaining high-value products.
Figure 1. Effect of one-step enzymatic hydrolysis (SST) on the production of IMOs from RFWs, with unhydrolyzed RFWs used as experimental controls. Data are reported as mean ± standard deviation (n = 3).
Furthermore, the economic benefits of utilizing RFW as feedstock material in the IMOs synthesis were assessed. Table 1 displays the IMOs production from different substrates and their economic relevance to the current study. The cost of substrates used in IMOs production ranged from $300 to $3,500 per ton. Some substrates, notably agricultural wastes, require additional treatment, such as the addition of ascorbic acid, ethylenediaminetetraacetic acid, and calcium hydroxide, to eliminate heavy metals and viscous substances. Additionally, downstream processing, particularly S. cerevisiae fermentation, is adopted to purify IMOs syrup, which concurrently generates ethanol as an additional byproduct (Basu and Prapulla, 2016; Kruschitz and Nidetzky, 2020). All these factors contribute to the overall economic burden of the process.
On the other hand, the present study reports the IMOs production from RFWs within the framework of a multi-step approach for the first time. RFW, an abundant and readily available resource that might otherwise be destined for landfills or costly disposal, can be obtained at a significantly lower cost and utilized in producing high-value IMOs, thereby reducing the overall production expenses. By upcycling RFW into IMOs, the process not only minimizes the negative environmental consequences associated with waste disposal but also generates a valuable product, contributing to a more sustainable and responsible approach to resource utilization. Although a higher IMOs yield was not attained from RFW (38 g/L) compared to other studies solely targeting IMOs as the end-product, RFW stands out as a zero-or low-cost feedstock, offering compelling economic advantages over other expensive substrates (Table 1). Importantly, RFW requires minimal treatments, with enzymatic hydrolysis being the primary process for IMOs synthesis. The findings strongly support the feasibility of utilizing RFW as an economical and efficient substrate for large-scale IMOs production, as well as for related products enriched with IMOs.
The RFW–R1 underwent prior testing to ascertain the optimal duration for IMOs synthesis. The corresponding outcomes presented in Figure 2A showed that the SST process consisted of two distinct phases. The initial phase, lasting from 0 to 2 h, was characterized by dominant transglycosylation. The major transglycosylation products detected were IG2, IG3, and P, and their concentrations were steadily increased, with IG2 exhibiting the highest concentration (24.77 g/L), followed by IG3 (7.81 g/L) and P (5.42 g/L). The final concentration of IMOs reached 38 g/L, and the conversion rate from starch to IMOs was measured at 22.68% (w/w).
Figure 2. Profile of IMO contents and glucose (byproduct) obtained from different RFWs by deploying SST process under optimal conditions. All experiments were carried out in triplicate. [(A–F): RFW-R1 to RFW-R6].
From 2 to 6 h, the second phase was initiated. The concentration of IMOs started to decline progressively, with IG2, IG3, and P concentrations reaching 21.45 g/L, 6.05 g/L, and 4.60 g/L, respectively (6 h). The observed decrease can be attributed to the transglucosidase activity, which also can facilitate the conversion of IMOs into various byproducts by cleaving α-1,4- and α-1,6-glycosidic bonds. This transformation primarily leads to the release of glucose as the main byproduct, predominantly from maltose, resulting in a significant reduction in the overall IMOs content. Moreover, panose undergoes extensive conversion into a range of oligosaccharides with increasing degrees of polymerization, including tetrasaccharides, pentasaccharides, hexasaccharides, and higher-degree oligosaccharides (Chockchaisawasdee and Poosaran, 2013; Cui et al., 2017). To maintain the IMOs concentration, it is highly recommended to regulate the transglucosidase dosage in the enzyme cocktail and effectively manage the processing time. While the literature addresses the management of transglucosidase dosage (Basu et al., 2016; Niu et al., 2017), our study specifically focused on controlling processing time. Remarkably, we identified an SST duration of 2 h as optimal to achieve maximum IMOs yield from RFW, with the study reporting the shortest synthesis duration compared to other studies (Table 1).
Afterwards, the SST process under optimal operational conditions was applied to further RFWs (R2–R6), and results are shown in Figures 2B–F. The analysis of the IMO contents exhibited a consistent pattern, with IG2 being the predominant transglycosylation product, followed by IG3 and P. The concentrations of IG2, IG3, and P ranged from 9.77 to 19.48 g/L, 4.23 to 9.19 g/L, and 2.08 to 4.85 g/L, respectively. Meanwhile, glucose as a byproduct of the SST process with continuously increasing concentration was released, and maximum concentrations of glucose reached 40.43 g/L, 32.23 g/L, 30.82 g/L, 28.34 g/L, 36.20 g/L, and 29.31 g/L, respectively.
Pichia pastoris GSL, previously evaluated for L-LA production (Supplementary Figures S2, S3), was deployed in IMOs enriched RFWs, which efficiently consumed all the free glucose within 44–48 h of fermentation. Figure 3 illustrates the concentrations of fermentative products obtained from the treated RFWs. The highest recorded L-LA titers in RFWs (R1, R2, R3, R4, R5, and R6) were 25.92 g/L, 23.11 g/L, 22.20 g/L, 22.35 g/L, 27.16 g/L, and 20.75 g/L, respectively. The corresponding conversion rates of glucose into L-LA were 64.10, 71.70, 72.10, 78.80, 75.10, and 70.80% (w/w). Incorporated into the animal diet, L-LA elicits a decrease in gastric pH to establish an unfavorable local microenvironment, delaying the multiplication of pathogens. Owing to its robust antimicrobial properties, the ban on antibiotics in animal production has prompted the adoption of L-LA as a viable alternative (Zamojska et al., 2021). A significant reduction in the pH of treated RFWs (4.3–4.5) was observed. Engineered yeast exhibits high tolerance to these low pH levels compared to lactic acid bacteria (Abdel-Rahman et al., 2013). Its utilization not only reduces chemical and utility requirements through low-pH fermentation but also facilitates the conversion of RFWs into probiotic-rich feeds, offering economic and ecological benefits.
Figure 3. Evaluating fermentation profile and L-LA production from RFWs by P. pastoris GSL: Illustrating distribution and quantification of other fermentative products.
Apart from L-LA, other beneficial organic acids, including acetic acid, pyruvic acid, and succinic acid, were generated at relatively modest levels, exhibiting concentrations ranging from 1.20 to 2.87 g/L, 0.70 to 2.61 g/L, and 0.20 to 0.88 g/L, respectively (Figure 3). The biosynthesis of organic acids enhances the sensory attributes of the feed, resulting in pleasant aromas and flavors that augment animals’ interest in consuming the fermented feed. Combined with other feed additives, organic acids synergistically stimulate the immune system and gut microbiota, overcoming nutritional and environmental stresses and improving animal growth performance (Suiryanrayna and Ramana, 2015; Kim et al., 2021). The experimental confirmations demonstrated that only glucose present in the hydrolyzed RFWs was converted into L-LA during the fermentation process, while the levels of IMOs remained nearly constant in the final product (see Supplementary Table S3). This outcome is attributed to the complex structure of disaccharides and higher oligosaccharides, which renders them unfermentable by P. pastoris, as previously discussed by Cervantes et al. (2022). In contrast to S. cerevisiae, P. pastoris GSL exhibited a distinct metabolic pathway, favoring L-LA production over ethanol when employed in IMOs syrup. This deviation from ethanol production eliminates the need for costly purification processes. Moreover, the GRAS (generally recognized as safe) status granted by the Food and Drug Administration enhances the safety profile of P. pastoris application in animal feed (Ahmad et al., 2014). These findings provide novel evidence that genetically modified P. pastoris can be utilized for RFW valorization, signifying its potential as a robust biocatalyst in developing an environmentally friendly and sustainable recycling approach. Furthermore, the EFP aligns with the overarching goals of achieving a circular economy by efficiently utilizing waste resources and reducing environmental impacts.
Despite significant compositional variation among RFWs sourced from different restaurants (Supplementary Table S1), P. pastoris GSL, during fermentation, retained its viability and thrived on the residual RFWs. Figure 4 depicts the cell viability and the biomass concentration of yeast. The cell viability of yeast in bio-treated RFWs (R1–R6) reached levels of 9.5, 8.7, 8.1, 7.6, 9.0, and 7.9 × 108 CFU/mL, respectively. These values align with the range recommended for animal feed (106 to 109 CFU/mL) by Minelli and Benini (2009). Livestock farming contributes 14 to 18% of global anthropogenic GHG emissions (FAO, 2006). Supplementing feed with live yeast cells has shown the potential to reduce GHG emissions, particularly methane, by competing methanogens for hydrogen (Gong et al., 2013; Che et al., 2017). The presence of oil compounds in RFW hydrolysates (RFW − R3 and RFW − R6) was observed to prolong the fermentation duration (~51 h), resulting in a slight reduction in yeast cell viability. These findings are coherent with previous work (Hafid et al., 2017), which demonstrated that such compounds generally create flotation, mass transfer, and clogging problems, thus hindering the fermentation efficiency by decreasing the yield. Moreover, the biomass concentration of P. pastoris GSL attained during fermentation was 8.5 g/L, 7.5 g/L, 6.2 g/L, 5.0 g/L, 8.2 g/L, and 7.2 g/L, accompanied by respective yields of 0.21 g/g, 0.23 g/g, 0.20 g/g, 0.17 g/g, 0.22 g/g, and 0.24 g/g glucose. The obtained biomasses are comparable to those reported by Choi et al. (2002) for various yeasts using Chinese cabbage waste as a substrate.
Figure 4. Illustration of viable cell count and biomass production of P. pastoris GSL during RFW fermentations. Solid lines (cell viability) and dotted lines (biomass concentration). (Blue circle: RFW R1; black square: RFW-R2; green diamond: RFW-R3; dark red triangle: RFW-R4; Orange triangle: RFW-R5; red circle: RFW-R6.
To serve as a healthy feed supplement, biomass must meet certain nutritional criteria, including elevated concentrations of essential compounds (e.g., total nitrogen) while minimizing the levels of antinutritional compounds like nucleic acid. Regarding the latter, ingested nucleic acids break down into purine and pyrimidine bases, with a further breakdown of purine resulting in the production of uric acid, which leads to the development of gout and calculi within the urinary tract (Silva et al., 2011). The incorporated yeast biomass effectively improved the crude protein content in treated RFWs, with final concentrations of crude protein reaching levels of 17.29, 21.21, 13.76, 19.61, 18.09, and 19.43% (w/w), respectively. The measured nucleic acid content (ranging between 4.61 to 5.80%) was lower than that usually acceptable for animal feed (≤ 10% dry weight). These results are in line with Ergün et al. (2022), who identified P. pastoris as a robust producer of single-cell protein characterized by low nucleic acid content and proposed its incorporation into animal feed. Utilizing feedstock like RFW in yeast biomass production yields economic benefits while simultaneously enhancing the nutritional quality of RFW for use as animal feed. Our findings provide compelling evidence endorsing the utilization of IMOs and L-LA-enriched RFWs, supplemented with probiotic biomass, as a viable substitute for animal feed.
The chemical composition and nutritional value of RFWs experienced substantial modifications during the EFP (Table 2). The starch content was significantly converted into IMOs, resulting in the complete absence of residual starch in the treated RFWs. Reducing the amount of starch in feed by converting it into other value-added compounds is important to mitigate the risks of subclinical ruminal acidosis in animals, which may result in reduced product yield (Gott et al., 2015). The addition of yeast biomass increased the crude protein content by 20 to 43% (w/w). This is in conformity with the outcomes of Jan et al. (2022), who reported that an increase in crude protein is credited to the utilization of carbohydrates and the degradation of complex protein by yeast. The observed decline in crude fat content (13–38%) can be attributed to various physiological and biochemical transformations, including lipid utilization for energy production and the degradation of fatty acids and glycerol to enhance sensory qualities. This decrease in fat level significantly minimizes the risk of rancidity and extends the shelf-life of feed. The crude fiber level was reduced by 33 to 76%, which could be associated with the fermenting organism’s capacity to metabolize fiber, as suggested by Jan et al. (2022). Ash content was increased by 33 to 70% from the initial, which is due to the reduction of carbohydrates and fat through microbial activities.
As a promising nitrogen source for microbial cell growth and fermentative lactic acid production, the concentration of FAAs was reduced by 41 to 80% (w/w) during RFW fermentations. In addition, P. pastoris GSL exhibited a high utilization rate of free phosphorus, estimated to range from 38 to 97% (w/w). Fermentation efficiently regulates the concentration of essential nutrients in livestock nutrition, and their mitigated excretion contributes to reducing environmental pollution and tackling potential challenges in animal husbandry, including GHG emissions and eutrophication.
Furthermore, RFWs used in this study primarily consisted of rice (≥ 60%), which contains abundant bioactive compounds (Pang et al., 2018). The biological processes, such as enzymatic hydrolysis and microbial fermentation, facilitate the liberation of these compounds, thus enhancing the nutritional value, functional properties, antioxidant activity, and organoleptic qualities; lipid peroxidation inhibition; mineral binding regulation of feed; and eventually contributing to the overall enhancement of animal health (Adebo and Medina-Meza, 2020; Khubber et al., 2022). Compared to control groups, the concentration of individual phenolic compounds in the treated RFWs was significantly increased. The levels of TPC and total flavonoids reached the highest concentrations of 54 mg Gallic acid Equivalent and 71 mg Rutin Equivalent per 100 g RFW (w/w), respectively. Besides, a range of high antioxidant activities were exhibited, as measured by the DPPH, ABTS+, and FRAP assays, ranging from 0.16 to 0.66 mM TE, 0.24 to 0.82 mM TE, and 0.11 to 0.53 mM TE per 100 g RFW, respectively (Table 2). For animal diets, however, defining the optimum dosage level of bioactive compounds proves challenging due to their wide variety and composition (Serra et al., 2021). Overall, the comparative analysis revealed that the EFP enhanced the nutritional value of RFWs, making them a more suitable option for animal feed.
The comprehensive mass balance was conducted to evaluate the amount of animal feed produced from RFW using the experimental results obtained in this study. A theoretical RFW of 1,000 L containing 167.50 kg of starch was considered as feedstock, and the mass balances of the EFP are summarized in Figure 5. After enzymatic hydrolysis, a maximum IMOs concentration was achieved, comprising 24.77 g/L IG2, 7.81 g/L IG3, and 5.42 g/L panose. Additionally, the hydrolysate contained glucose as a transglycosylation byproduct, with a final concentration of 40.43 g/L. The absence of maltose in the transglycosylation (IMOs) mixture confirmed the preservation of most oligosaccharides using the current technology and pretreatment conditions. The analysis of remaining sugars or oligosaccharides was not conducted as the focus was primarily on the IMOs synthesis from starch present in RFW.
The reuse of hydrolysate as a medium in food waste management contributes to the establishment of an economically efficient process. The glucose found in hydrolysate is typically considered undesirable in animal diets due to its potential to facilitate pathogenic growth, which is detrimental to animals and product storage. The recombinant P. pastoris GSL converted overall glucose specifically into 25.92 g/L L-LA with a yield of 0.64 g/g sugar consumed. During fermentation, yeast biomass (13 g/L) was produced as a supplementary probiotic in the treated RFW. Based on the experimental and theoretical findings, 1,000 liters of RFW slurry could yield 257 kg (dried) animal feed containing 0.15 kg.kg−1 IMOs, 0.10 kg.kg−1L-LA, and 0.05 kg.kg−1 yeast biomass. The content of crude protein (0.06 kg.kg−1) and ash (0.02 kg.kg−1) showed significant amelioration, with respective increases of 1.87 and 2.10 folds. In Figure 5, the materials balance showed a loss of some mass due to either loss of water residues or the conversion of one compound into another, which remained undetermined in this study. The EFP effectively inhibited microbial contamination compared to control groups. The treated RFWs can be used either as fermented liquid feed or stored for long-term usage as dried feed (see Figure 6). We consider this mass balance as a significant step toward the commercialization of RFW-derived feed, with the potential for broader applications in environmentally sustainable processes.
Figure 6. Demonstration of EFP converting RFWs (R1-R6) into synbiotic animal feed. (A) Homogenized RFWs. (B) Experimental controls. (C) IMOs enriched RFWs after yeast fermentation (as fermented liquid feed). (D) Treated RFWs dried at medium temperature (≤ 65°C).
Conventional feeds contribute up to 70% of the total cost in livestock production systems, whereas the incorporation of commercial additives further escalates the total cost (Yang et al., 2006; Yunus et al., 2015; Wang et al., 2016; Alqaisi et al., 2017). The overall cost of EFP, including expenses for power consumption, enzyme, and fermentation, amounted to around $157.59 per ton (Supplementary Table S4). A substantive amount ($93) of this cost is attributed to the enzyme cocktail required for IMOs synthesis. The production cost of the acquired feed is, respectively, 56 and 70% lower compared to corn ($359.46/ton) and soybean ($525.36/ton). It is noteworthy to emphasize that the EFP, as the prevailing recycling technology, guarantees the complete conversion of the entire RFW into a valuable synbiotic feed, resulting in no residual waste and solidifying the efficacy of our zero-waste approach. However, further endeavors are necessitated to explore renewable energy sources and low-cost enzyme reservoirs, aiming to reduce energy costs and alleviate the overall expenses associated with this technology.
In conclusion, the novel EFP presented in this study offers a sustainable and innovative solution for the bioconversion of RFW into synbiotic animal feed. The technology not only addresses the issue of food waste management but also provides an economically and environmentally beneficial approach. Analysis of enzymatically treated RFW exhibited that starch was efficiently converted into IMOs (17–25%, w/w) by the optimized SST process. The free glucose yielded 64–79% (w/w) L-LA, through recombinant P. pastoris GSL. Yeast biomass as an additional feed additive was produced in RFWs during fermentation. The comprehensive estimates demonstrated that EFP considerably reduced feed costs by 56 to 70% compared to conventional feed sources. The technology was found to be reliable in enhancing the nutritional value of RFWs and adhered to the zero-waste concept by generating no residual waste. Altogether, our results suggest that EFP could be a promising and practical model for reducing waste, promoting a circular bioeconomy, and enhancing animal nutrition.
The datasets presented in this study can be found in online repositories. The names of the repository/repositories and accession number(s) can be found in the article/Supplementary material.
MB: Conceptualization, Project administration, Supervision, Validation, Writing – review & editing. DN: Data curation, Investigation, Methodology, Writing – original draft. ZW: Supervision, Writing – review & editing.
The author(s) declare financial support was received for the research, authorship, and/or publication of this article. This work was supported by the Tianjin Outstanding Talent Program (Grant No.: JC20200309) to ZW, the Intergovernmental International Scientific and Technological Innovation Cooperation Program, MOST, China (Grant No.: 2018YFE0100400) to ZW, the Intergovernmental International Scientific and Technological Innovation Cooperation Program, MOST, China (Grant No.:2021YFE0106200) to DN.
The authors declare that the research was conducted in the absence of any commercial or financial relationships that could be construed as a potential conflict of interest.
All claims expressed in this article are solely those of the authors and do not necessarily represent those of their affiliated organizations, or those of the publisher, the editors and the reviewers. Any product that may be evaluated in this article, or claim that may be made by its manufacturer, is not guaranteed or endorsed by the publisher.
The Supplementary material for this article can be found online at: https://www.frontiersin.org/articles/10.3389/fsufs.2024.1326304/full#supplementary-material
Abdel-Rahman, M. A., Tashiro, Y., and Sonomoto, K. (2013). Recent advances in lactic acid production by microbial fermentation processes. Biotechnol. Adv. 31, 877–902. doi: 10.1016/j.biotechadv.2013.04.002
Adebo, O. A., and Medina-Meza, G. I. (2020). Impact of fermentation on the phenolic compounds and antioxidant activity of whole cereal grains: a mini review. Molecules 25:927. doi: 10.3390/moslecules25040927
Ahmad, M., Hirz, M., Pichler, H., and Schwab, H. (2014). Protein expression in Pichia pastoris: recent achievements and perspectives for heterologous protein production. Appl. Microbiol. Biotechnol. 98, 5301–5317. doi: 10.1007/s00253-014-5732-5
Ajila, C. M., Brar, S. K., Verma, M., Tyagi, R. D., Godbout, S., and Valero, J. R. (2012). Bio-processing of agro-byproducts to animal feed. Crit. Rev. Biotechnol. 32, 382–400. doi: 10.3109/07388551.2012.659172
Alqaisi, O., Ndambi, O. A., and Williams, R. B. (2017). Time series livestock diet optimization: cost-effective broiler feed substitution using the commodity price spread approach. Agric. Food Econ. 5:25. doi: 10.1186/s40100-017-0094-9
Azimonti, G., Bampidis, V., Bastos, M. D. L., Christensen, H., Dusemund, B., Durjava, M. F., et al. (2022). Safety and efficacy of a feed additive consisting of lactic acid produced by Weizmannia coagulans (synonym Bacillus coagulans) DSM 32789 for all animal species except for fish (Jungbunzlauer SA). EFSA J. 20:e07268. doi: 10.2903/j.efsa.2022.7268
Bakar, M. F. A., Mohamed, M., Rahmat, A., and Fry, J. (2009). Phytochemicals and antioxidant activity of different parts of bambangan (Mangifera pajang) and tarap (Artocarpus odoratissimus). Food Chem. 113, 479–483. doi: 10.1016/j.foodchem.2008.07.081
Basu, A., Mutturi, S., and Prapulla, S. G. (2016). Production of isomaltooligosaccharides (IMO) using simultaneous saccharification and transglucosylation from starch and sustainable sources. Process Biochem. 51, 1464–1471. doi: 10.1016/j.procbio.2016.06.008
Basu, A., and Prapulla, S. G. (2016). “Sustainable utilization of food industry waste and by-products for the production of prebiotic isomaltooligosaccharides (IMO)” in Biotechnology and biochemical engineering. eds. B. D. P. Gummadi and P. Vadlani (Singapore: Springer).
Carranza-Mendez, R. C., Chávez-González, M. L., Sepúlveda-Torre, L., Aguilar, C. N., Govea-Salas, M., and Ramos-González, R. (2022). Production of single cell protein from orange peel residues by Candida utilis. Biocat Agri Biotechnol. 40:102298. doi: 10.1016/j.bcab.2022.102298
Cervantes, F. V., Fernandez-Polo, D., Merdzo, Z., Miguez, N., Garcia-Gonzalez, M., Ballesteros, A. O., et al. (2022). Reuse of immobilized Komagataella phaffii cells for the elimination of d-glucose in syrups of bioactive carbohydrates. ACS Food Sci. Technol. 2, 682–690. doi: 10.1021/acsfoodscitech.2c00008
Chen, Y., Qin, L., Wen, A., Mazhar, M., Wang, H., and Zhu, Y. (2021). Three-solvent extracting method comprehensively evaluates phenolics profile and antioxidant activities of Tartary buckwheat. J. Food Process. Preserv. 45:e15020. doi: 10.1111/jfpp.15020
Chen, T., Jin, Y., and Shen, D. (2015). A safety analysis of food waste-derived animal feeds from three typical conversion techniques in China. Waste Manag. 45, 42–50. doi: 10.1016/j.wasman.2015.06.041
Che, L., Xu, Q., Wu, C., Luo, Y., Huang, X., Zhang, B., et al. (2017). Effects of dietary live yeast supplementation on growth performance, diarrhoea severity, intestinal permeability and immunological parameters of weaned piglets challenged with enterotoxigenic Escherichia coli K88. Br. J. Nutr. 118, 949–958. doi: 10.1017/S0007114517003051
Cho, S. K., Eom, H. J., Moon, J. S., Lim, S. B., Kim, Y. K., Lee, K. W., et al. (2014). An improved process of isomaltooligosaccharide production in kimchi involving the addition of a Leuconostoc starter and sugars. Int. J. Food Microbiol. 170, 61–64. doi: 10.1016/j.ijfoodmicro.2013.10.027
Chockchaisawasdee, S., and Poosaran, N. (2013). Production of isomaltooligosaccharides from banana flour. J. Sci. Food Agric. 93, 180–186. doi: 10.1002/jsfa.5747
Choi, M. H., Ji, G. E., Koh, K. H., Ryu, Y. W., Jo, D. H., and Park, Y. H. (2002). Use of waste Chinese cabbage as a substrate for yeast biomass production. Bioresour. Technol. 83, 251–253. doi: 10.1016/s0960-8524(01)00232-2
Cui, J., Li, Y., Wang, Q., Li, J., Ou, Y., Wang, J., et al. (2017). Production, purification and analysis of the isomalto-oligosaccharides from Chinese chestnut (Castanea mollissima Blume) and the prebiotics effects of them on proliferation of Lactobacillus. Food Bioprod. Process. 106, 75–81. doi: 10.1016/j.fbp.2017.08.003
Du, G., Shi, J., Zhang, J., Ma, Z., Liu, X., Yuan, C., et al. (2021). Exogenous probiotics improve fermentation quality, microflora phenotypes, and trophic modes of fermented vegetable waste for animal feed. Microorganisms 9:644. doi: 10.3390/microorganisms9030644
Duong Hong, Q., Hoang Minh, T., Nguyen Thi, H. G., Nguyen Thi, T. H., Nguyen, N. H., Nguyen Thi, T. L., et al. (2021). Synthesis of isomaltooligosaccharides (IMOs) from sweet potato starch by simultaneous saccharification and transglycosylation using Saccharomyces cerevisiae Var. diastaticus BE 134 to improve purity of IMOs. J. Food Qual. 2021, 1–12. doi: 10.1155/2021/1987219
Ergün, B. G., Laçın, K., Çaloğlu, B., and Binay, B. (2022). Second generation Pichia pastoris strain and bioprocess designs. Biotechnol. Biofuels 15, 150–119. doi: 10.1186/s13068-022-02234-7
Garnida, Y., Rudiansyah, M., Yasin, G., Mahmudiono, T., Kadhim, A. J., Sharma, S., et al. (2022). Investigation of parameters in restaurant food waste for use as poultry rations. Food Sci. Technol. 42:e118621. doi: 10.1590/fst.118621
Gómez, J. A., Berni, P., Matallana, L. G., Sánchez, Ó. J., Teixeira, J. A., and Nobre, C. (2022). Towards a biorefinery processing waste from plantain agro–industry: process development for the production of an isomalto–oligosaccharide syrup from rejected unripe plantain fruits. Food Bioprod. 133, 100–118. doi: 10.1016/j.fbp.2022.03.005
Gong, Y. L., Liao, X. D., Liang, J. B., Jahromi, M. F., Wang, H., Cao, Z., et al. (2013). Saccharomyces cerevisiae live cells decreased in vitro methane production in intestinal content of pigs. Asian Australas. J. Anim. Sci. 26, 856–863. doi: 10.5713/ajas.2012.12663
Gott, P. N., Hogan, J. S., and Weiss, W. P. (2015). Effects of various starch feeding regimens on responses of dairy cows to intramammary lipopolysaccharide infusion. J. Dairy Sci. 98, 1786–1796. doi: 10.3168/jds.2014-8638
Hafid, H. S., Nor'Aini, A. R., Mokhtar, M. N., Talib, A. T., Baharuddin, A. S., and Kalsom, M. S. U. (2017). Over production of fermentable sugar for bioethanol production from carbohydrate-rich Malaysian food waste via sequential acid-enzymatic hydrolysis pretreatment. Waste Manag. 67, 95–105. doi: 10.1016/j.wasman.2017.05.017
Han, W., Yan, Y., Shi, Y., Gu, J., Tang, J., and Zhao, H. (2016). Biohydrogen production from enzymatic hydrolysis of food waste in batch and continuous systems. Sci. Rep. 6:38395. doi: 10.1038/srep38395
Jan, S., Kumar, K., Yadav, A. N., Ahmed, N., Thakur, P., Chauhan, D., et al. (2022). Effect of diverse fermentation treatments on nutritional composition, bioactive components, and anti-nutritional factors of finger millet (Eleusine coracana L.). J. Appl. Biol. Biotechnol. 10, 46–52. doi: 10.7324/JABB.2022.10s107
Kaulpiboon, J., Rudeekulthamrong, P., Watanasatitarpa, S., Ito, K., and Pongsawasdi, P. (2015). Synthesis of long-chain isomaltooligosaccharides from tapioca starch and an in vitro investigation of their prebiotic properties. J. Mol. Catal. B Enzym. 120, 127–135. doi: 10.1016/j.molcatb.2015.07.004
Khubber, S., Marti-Quijal, F. J., Tomasevic, I., Remize, F., and Barba, F. J. (2022). Lactic acid fermentation as a useful strategy to recover antimicrobial and antioxidant compounds from food and by-products. Curr. Opin. Food Sci. 43, 189–198. doi: 10.1016/j.cofs.2021.11.013
Kim, D., Jung, J. S., and Choi, K. C. (2021). A preliminary study on effects of fermented feed supplementation on growth performance, carcass characteristics, and meat quality of Hanwoo steers during the early and late fattening period. Appl. Sci. 11:5202. doi: 10.3390/app11115202
Kruschitz, A., and Nidetzky, B. (2020). Downstream processing technologies in the biocatalytic production of oligosaccharides. Biotechnol. Adv. 43:e107568:107568. doi: 10.1016/j.biotechadv.2020.107568
Li, M., Liu, W., Zhao, G., Yu, L., Wu, Y., Wu, Y., et al. (2010). Effect of different oligosaccharides on immunity and production performance of piglets. Anim. Husb. Feed Sci. 2, 9–11.
Liao, H., Dong, W., Shi, X., Liu, H., and Yuan, K. (2012). Analysis and comparison of the active components and antioxidant activities of extracts from Abelmoschus esculentus L. Pharmacogn. Mag. 8, 156–161. doi: 10.4103/0973-1296.96570
Minelli, E. B., and Benini, A. (2009). Relationship between number of bacteria and their probiotic effects. Microb. Ecol. Health Dis. 20, 180–183. doi: 10.1080/08910600802408095
Mistry, R. H., Borewicz, K., Gu, F., Verkade, H. J., Schols, H. A., Smidt, H., et al. (2020). Dietary isomalto/malto-polysaccharides increase fecal bulk and microbial fermentation in mice. Mol. Nutr. Food Res. 64:e2000251. doi: 10.1002/mnfr.202000251
Mladenovic, D., Djukic-Vukovic, A., Stanković, M., Milasinovic-Seremesic, M., Radosavljevic, M., Pejin, J., et al. (2019). Bioprocessing of agro-industrial residues into lactic acid and probiotic enriched livestock feed. J. Sci. Food Agric. 99, 5293–5302. doi: 10.1002/jsfa.9759
Nasser, N., Abiad, M. G., Babikian, J., Monzer, S., and Saoud, I. P. (2018). Using restaurant food waste as feed for Nile tilapia production. Aquac. Res. 49, 3142–3150. doi: 10.1111/are.13777
Nath, P. C., Ojha, A., Debnath, S., Sharma, M., Nayak, P. K., Sridhar, K., et al. (2023). Valorization of food waste as animal feed: a step towards sustainable food waste management and circular bioeconomy. Animals 13:1366. doi: 10.3390/ani13081366
Navarrete-del-Toro, M. A., and García-Carreño, F. L. (2003). Evaluation of the progress of protein hydrolysis. Ed. current protocols in food analytical chemistry (New York: Wiley)
Niu, D., Qiao, J., Li, P., Tian, K., Liu, X., Singh, S., et al. (2017). Highly efficient enzymatic preparation of isomalto-oligosaccharides from starch using an enzyme cocktail. Electron. J. Biotechnol. 26, 46–51. doi: 10.1016/j.ejbt.2016.12.002
Niu, D., Tian, K., Prior, B. A., Wang, M., Wang, Z., Lu, F., et al. (2014). Highly efficient L-lactate production using engineered Escherichia coli with dissimilar temperature optima for L-lactate formation and cell growth. Microb. Cell Factories 13, 78–11. doi: 10.1186/1475-2859-13-78
Noori, A. W., Royen, M. J., Medved Ova, A., and Haydary, J. (2022). Drying of food waste for potential use as animal feed. Sustainability 14:5849. doi: 10.3390/su14105849
Ojha, S., Bubler, S., and Schlüter, O. K. (2020). Food waste valorization and circular economy concepts in insect production and processing. Waste Manag. 118, 600–609. doi: 10.1016/j.wasman.2020.09.010
Pang, Y., Ahmed, S., Xu, Y., Beta, T., Zhu, Z., Shao, Y., et al. (2018). Bound phenolic compounds and antioxidant properties of whole grain and bran of white, red and black rice. Food Chem. 240, 212–221. doi: 10.1016/J.FOODCHEM.2017.07.095
Plongbunjong, V., Graidist, P., Knudsen, K. E. B., and Wichienchot, S. (2017). Starch-based carbohydrates display the bifidogenic and butyrogenic properties in pH-controlled faecal fermentation. Int. J. Food Sci. Technol. 52, 2647–2653. doi: 10.1111/ijfs.13553
Rahutomo, S., Kovar, J. L., and Thompson, M. L. (2019). Malachite green method for determining phosphorus concentration in diverse matrices. Commun. Soil Sci. Plant Anal. 50, 1743–1752. doi: 10.1080/00103624.2019.1635140
Rollán, G. C., Gerez, C. L., and LeBlanc, J. G. (2019). Lactic fermentation as a strategy to improve the nutritional and functional values of pseudocereals. Front. Nutr. 6:98. doi: 10.3389/fnut.2019.00098
Sambrook, J., Fritsch, E. F., and Maniatis, T. E. (1989). Molecular cloning: A laboratory manual, second ed. New York: Cold spring harbor laboratory press.
Serra, V., Salvatori, G., and Pastorelli, G. (2021). Dietary polyphenol supplementation in food producing animals: effects on the quality of derived products. Animals 11:401. doi: 10.3390/ani11020401
Shao, Y., Xu, F., Sun, X., Bao, J., and Beta, T. (2014). Phenolic acids, anthocyanins, and antioxidant capacity in rice (Oryza sativa L.) grains at four stages of development after flowering. Food Chem. 143, 90–96. doi: 10.1016/j.foodchem.2013.07.042
Silva, C. F., Arcuri, S. L., Campos, C. R., Vilela, D. M., Alves, J. G., and Schwan, R. F. (2011). Using the residue of spirit production and bio-ethanol for protein production by yeasts. Waste Manag. 31, 108–114. doi: 10.1016/j.wasman.2010.08.015
Sindhu, R., Gnansounou, E., Rebello, S., Binod, P., Varjani, S., Thakure, I. S., et al. (2019). Conversion of food and kitchen waste to value-added products. J. Environ. Manag. 241, 619–630. doi: 10.1016/j.jenvman.2019.02.053
Sobol, L., Dyjakon, A., and Soukup, K. (2023). Dioxins and furans in biochars, hydrochars and torreficates produced by thermochemical conversion of biomass: a review. Environ. Chem. Lett. 21, 2225–2249. doi: 10.1007/s10311-023-01600-7
Staley, B. F., Iii, F. L. D. L. R., and Barlaz, M. A. (2011). Effect of spatial differences in microbial activity, pH, and substrate levels on methanogenesis initiation in refuse. Appl. Environ. Microbiol. 77, 2381–2391. doi: 10.1128/AEM.02349-10
Suiryanrayna, M. V., and Ramana, J. V. (2015). A review of the effects of dietary organic acids fed to swine. J. Anim. Sci. Biotechnol. 6:45. doi: 10.1186/s40104-015-0042-z
Suwannarat, J., and Ritchie Ajila, R. J. (2015). Anaerobic digestion of food waste using yeast. Waste Manag. 42, 61–66. doi: 10.1016/j.wasman.2015.04.028
Tiangpook, S., Nhim, S., Prangthip, P., Pason, P., Tachaapaikoon, C., Ratanakhanokchai, K., et al. (2023). Production of a series of long-chain isomaltooligosaccharides from maltose by Bacillus subtilis AP-1 and associated prebiotic properties. Foods 12:1499. doi: 10.3390/foods12071499
Wang, R., Lorantfy, B., Fusco, S., Olsson, L., and Franzen, C. J. (2021). Analysis of methods for quantifying yeast cell concentration in complex lignocellulosic fermentation processes. Sci. Rep. 11, 11293–11212. doi: 10.1038/s41598-021-90703-8
Wang, C., Shi, C., Zhang, Y., Song, D., Lu, Z., and Wang, Y. (2018). Microbiota in fermented feed and swine gut. Appl. Microbiol. Biotechnol. 102, 2941–2948. doi: 10.1007/s00253-018-8829-4
Wang, X. X., Song, P. X., Wu, H., Xue, J. X., Zhong, X., and Zhang, L. Y. (2016). Effects of graded levels of isomaltooligosaccharides on the performance, immune function and intestinal status of weaned pigs. Asian Australas. J. Anim. Sci. 29, 250–256. doi: 10.5713/ajas.15.0194
Werten, M. W., Eggink, G., Stuart, M. A. C., and de Wolf, F. A. (2019). Production of protein-based polymers in Pichia pastoris. Biotechnol. Adv. 37, 642–666. doi: 10.1016/j.biotechadv.2019.03.012
Yang, S. Y., Ji, K. S., Baik, Y. H., Kwak, W. S., and McCaskey, T. A. (2006). Lactic acid fermentation of food waste for swine feed. Bioresour. Technol. 97, 1858–1864. doi: 10.1016/j.biortech.2005.08.020
Yousuf, A., Bastidas-Oyanedel, J. R., and Schmidt, J. E. (2018). Effect of total solid content and pretreatment on the production of lactic acid from mixed culture dark fermentation of food waste. Waste Manag. 77, 516–521. doi: 10.1016/j.wasman.2018.04.035
Yunus, F. N., Nadeem, M., and Rashid, F. (2015). Enhancement of protein contents of rice bran for animal feed by solid state fermentation. Biomed. Lett. 1, 31–36.
Zamojska, D., Nowak, A., Nowak, I., and Macierzyńska-Piotrowska, E. (2021). Probiotics and postbiotics as substitutes of antibiotics in farm animals: a review. Animals 11:3431. doi: 10.3390/ani11123431
Zhang, L., Dong, W., Yao, Y., Chen, C., Li, X., Yin, B., et al. (2022). Analysis and research on starch content and its processing, structure and quality of 12 adzuki bean varieties. Foods 11:3381. doi: 10.3390/foods11213381
Zhang, C., Hao, E., Chen, X., Huang, C., Liu, G., Chen, H., et al. (2023). Dietary fiber level improve growth performance, nutrient digestibility, immune and intestinal morphology of broilers from day 22 to 42. Animals 13:1227. doi: 10.3390/ani13071227
Keywords: restaurant food waste, resourcesization, Isomaltooligosaccharides, L-lactic acid, Pichia pastoris GSL, synbiotic animal feed
Citation: Bilal M, Niu D and Wang Z (2024) Novel enzyme-fermentation process for bioconversion of restaurant food waste into isomaltooligosaccharide-and L-lactic acid-enriched animal feed. Front. Sustain. Food Syst. 8:1326304. doi: 10.3389/fsufs.2024.1326304
Received: 23 October 2023; Accepted: 05 January 2024;
Published: 23 January 2024.
Edited by:
Silvia Fiore, Polytechnic University of Turin, ItalyReviewed by:
Muthukumar Serva Peddha, Central Food Technological Research Institute (CSIR), IndiaCopyright © 2024 Bilal, Niu and Wang. This is an open-access article distributed under the terms of the Creative Commons Attribution License (CC BY). The use, distribution or reproduction in other forums is permitted, provided the original author(s) and the copyright owner(s) are credited and that the original publication in this journal is cited, in accordance with accepted academic practice. No use, distribution or reproduction is permitted which does not comply with these terms.
*Correspondence: Zhengxiang Wang, enh3YW5nMDUxOUB0dXN0LmVkdS5jbg==
Disclaimer: All claims expressed in this article are solely those of the authors and do not necessarily represent those of their affiliated organizations, or those of the publisher, the editors and the reviewers. Any product that may be evaluated in this article or claim that may be made by its manufacturer is not guaranteed or endorsed by the publisher.
Research integrity at Frontiers
Learn more about the work of our research integrity team to safeguard the quality of each article we publish.