- School of Public Health, Shandong First Medical University and Shandong Academy of Medical Sciences, Jinan, Shandong, China
The maize starch (MS)-stearic acid (SA) and MS-SA-whey protein (WP) complexes were prepared using the high-pressure homogenization (HPH). Results from X-ray diffraction (XRD) showed that MS-SA complexes presented an increase in the long-range molecular order with increasing the homogenization pressure, and MS-SA-WP complexes showed only an increase with increasing the homogenization pressure from 10 to 50 MPa. Results from differential scanning calorimetry (DSC) and Raman spectroscopy showed that the amount of complexes and the short-range order of both MS-SA and MS-SA-WP complexes increased with increasing the homogenization pressure. The addition of WP to MS-SA altered significantly the structure and digestion of complexes. Results revealed that MS-SA-WP complexes have more ordered structure and lower digestion than the corresponding MS-SA complexes. The digestibility of all complexes decreased with increasing the homogenization pressure. There was a significant correlation between the digestibility and structural characteristics of complexes. Complexes with better structural stability have better anti-digestion properties. The obtained results are helpful in understanding the structure and digestibility of complexes prepared by HPH, which is valuable for controlling the quality and nutrition of starchy food.
1 Introduction
Starch is the main energy source of the human diet. It is a natural, renewable, inexpensive and biodegradable raw material that can be widely used for thickener, stabilizer and binder (Wang et al., 2021, 2022). However, higher digestibility of starch was found due to the disruption of starch structure after processing, leading to a higher glycemic index (Liu et al., 2022). Based on the glucose release rate of starch, starch is divided into three types of rapidly digestible starch (RDS, digest within 20 min), slowly digestible starch (SDS, digest within 20 min to 120 min) and resistant starch (RS, unable to be digested after 120 min; Englyst et al., 1992). RS is not digested in the small intestine but fermented in the large intestine to produce short-chain fatty acids beneficial for human. RS, which can be divided into five types depending on their interaction pattern with the starch digestive enzymes and termed them as physically inaccessible starch (RS1), natural resistant starch granules (RS2), retrograded starch (RS3), chemically modified starch (RS4) and starch-lipid complexes (RS5; Bojarczuk et al., 2022). The latter RS (RS5) was attracted and studied widely due to its outstanding functional and nutritional properties (Guo et al., 2021). RS5, known as V-type complex, is a single-helical inclusion formed by the insertion of lipids into the spiral cavities of amylose (Putseys et al., 2010; Wang et al., 2020). The stable structure V-type starch-lipid complex is resistant to enzyme hydrolysis, which is effective in stabilizing postprandial blood glucose (Gutiérrez and Tovar, 2021). The addition of protein to the mixture of starch and lipids facilitates the further formation of complexes with lower digestibility (Zheng et al., 2018; Gutiérrez and Tovar, 2021).
There are many methods for preparing V-type complexes, such as extrusion cooking, frying, steam cooking, ultrasound, microwave and high-pressure homogenization (HPH; Liu et al., 2018; Cervantes-Ramírez et al., 2020; Shi et al., 2020; Guo et al., 2021; Li et al., 2021). Recently, HPH has received much attention due to environment-friendly and low-cost. High speed shear generated by HPH can degrade starch polymers to release more amylose, improve the dispersion of lipid with low water solubility and enhance the chances of reaction between lipid and amylose (Shuang et al., 2014; Chen et al., 2017). Previous studies found that as increased homogenization pressure and the addition content of lipids, the complex index increased generally (Shuang et al., 2014; Chen et al., 2017; Cui et al., 2021). Following the HPH treatment, starch-lipid complexes showed an obvious decrease in the hydrolysis content (Chen et al., 2018). Protein can also interact with starch and lipids by hydrophobic forces and van der Waals forces to form starch-lipid-protein complexes (Wang et al., 2022). And the starch-lipid-protein ternary complex has lower digestibility than that of the corresponding starch-lipid binary complex (Zheng et al., 2018; Duan et al., 2023).
The starch-lipid complexes prepared by HPH have been studied extensively, but studies on the interactions among starch, lipid and protein using the HPH are still limited. Protein often coexists with starch and lipids in starchy foods. Therefore, the influence of HPH on maize starch-stearic acid and maize starch-stearic acid-whey protein complexes were investigated. Furthermore, correlations between the structural properties and digestibility of complexes were established. The dates from the present study are helpful to better understand the interactions among starch, lipid and protein under HPH treatment, as well as to process and prepare starchy food with controllable digestibility.
2 Materials and methods
2.1 Materials
The maize starch (MS, 11.9% water content, 0.36% protein content, 0.11% lipid content, 0.02% ash content, 23.7% amylose content) was purchased from the Shandong Rising Group Co., Ltd. (Shandong province, China). Whey protein (Hilmar9410, WP, 90.4% protein content, 1.4% lipid content, 0.3% ash content) was acquired from the Hilmar Ingredients Company (Hilma, California, United States). Stearic acid (SA, lab grade) was obtained from the Maclin Biochemical Technology Co., Ltd. (Shanghai, China). α-amylase (Sigma, EC 3.2.1.1, type VI-B from porcine pancreas, 10 units/mg) were purchased from Sigma Chemical Co. (St. Louis, MO, United States). Maltose was acquired from China National Medicines Co., Ltd. Other chemical reagents were all of analytical grades.
2.2 Preparation of MS-SA and MS-SA-WP complexes
The MS-SA and MS-SA-WP complexes were prepared by the high-pressure homogenizer according to a previous method with slight modifications (Guo et al., 2021). MS was mixed with SA (5%, w/w, dry starch base) and/or WP (10%, w/w, dry starch base) to achieve a weight ratio of 20: 1: 2, and deionized water was added to make the water content of 92%. Then the starch slurry was constantly stirred for 2 h at 25°C to make the SA and WP better disperse in starch slurry. The MS-SA-WP mixtures were incubated at 95°C for 30 min, and then cooled to 60°C for another 30 min. The mixed slurry was homogenized using a laboratory-scale high-pressure homogenizer (GYB 40-10S; Donghua Technology Co., Ltd., Shanghai, China) at 10, 30, 50, 70, and 90 MPa for three passes. Samples were collected, freeze-dried, ground into powder and passed through a 100-mesh sieve.
2.3 Differential scanning calorimetry
According to previous research with minor modifications as follows (Chao et al., 2020; Liu et al., 2021), the thermal properties of the MS, MS-SA and MS-SA-WP complexes were examined using a differential scanning calorimeter (204, Netzsch, Germany). 3 mg samples were weighed into a 40 μL aluminum pan, and 9 μL distilled water was added. The pans were sealed and allowed to stand overnight at room temperature before measurement. The samples were heated from 20 to 130°C at a heating rate of 10°C/min. An empty aluminum pan was used as the reference. The onset (To), peak (Tp), conclusion (Tc) temperatures and enthalpy change (ΔH) of complexes were obtained through data recording software.
2.4 X-ray diffraction analysis
The MS, MS-SA and MS-SA-WP complexes were analyzed using a Bruker D8 Advance X-ray diffractometer (Bruker, Germany) operating at 40 kV and 40 mA. The samples were scanned from 5° to 35° (2θ) with a speed of 2°/min and a step size of 0.06°. The relative crystallinity of samples were calculated using the TOPAS V.5.0 software.
2.5 Laser confocal micro-Raman spectroscopy
Raman spectra were obtained using a Renishaw Invia Raman microscope system equipped with a Leica microscope (Leica Biosystems, Wetzlar, Germany); a 785 nm green diode laser source was used. The MS-SA and MS-SA-WP complexes were pressed into a flat surface using two glass slides. Spectra were collected at least six different spots in the range of 3,200–100 cm−1 with a resolution of approximately 7 cm−1. The full width at half maximum (FWHM) of the band at 480 cm−1 was calculated using the WiRE 2.0 software to characterize the short-range ordered structure in complexes (Wang et al., 2017a).
2.6 Fourier transform infrared spectroscopy
The FTIR data of MS, MS-SA, and MS-SA-WP complexes were collected by a Fourier transform infrared spectroscopy (FTIR) spectrometer (Bruker, Germany). The mixture of 2 mg samples and 100 mg KBr powder were grinded using a quartz mortar and a transparent pellet was obtained by pressing at a pressure of 150 MPa for 30 s. The scanning range is from 4,000 to 400 cm−1 with an accumulation of 32 scans at a resolution of 4 cm−1.
2.7 Scanning electron microscope
The morphology of MS, MS-SA and MS-SA-WP complexes were obtained using high resolution field emission scanning electron microscope (SEC; Regulus8100, Tokyo, Japan) at an accelerating voltage of 1 kV. The representative micrographs were recorded at 1,000× magnifications.
2.8 In vitro starch digestion
The enzymatic hydrolysis of complexes was done using a previous method with slight modifications (Xu et al., 2020). Starch (100 mg, wet-weight basis) was suspended in 9.0 mL of sodium acetate buffer (pH 6.0), and 1.0 mL of freshly prepared enzyme solution containing 10 units of α-amylase was added and mixed in a 50 mL centrifuge tube. The centrifuge tubes were placed in a water-bath shaker (37°C, 260 r/min) and incubated for the corresponding times. At 20, 40, 60, 80, 100, 120, 160, 200, and 240 min, 100 μL of the hydrolysate was withdrawn into 1 mL centrifuge tube with 900 μL of 0.5 M Na2CO3. After centrifugation at 13,000 × g for 3 min, the reducing sugar content in the supernatant was determined using the PAHBAH method described previously (Xu et al., 2020). Starch digestograms obtained by plotting the percent of hydrolyzed starch as a function of hydrolysis time. The content of RDS, RDS and RS were calculated.
2.9 Statistical analysis
Except for XRD, all the other experimental tests were repeated at least three times. The mean values and standard deviation and the significant differences of the data were calculated using SPSS 25.0 software (SPSS, Inc. Chicago, IL, United States). Data in the same column labeled with different letters have significant differences.
3 Results and discussion
3.1 Long-range order of complexes
The long-range structural order of the MS, MS-SA, and MS-SA-WP complexes prepared by different homogeneous pressure was characterized by the X-ray diffraction, and the results were shown in Figure 1. Native MS displayed a typical A-type diffraction pattern with peaks at 15.0, 17.0, 18.0 and 23.0° (2θ). The relative crystallinity (RC) of MS was 22.1% (Table 1). After the treatment of high-pressure homogenization, the main diffraction peaks of the MS disappeared. Three distinct diffraction peaks at 19.7°, 21.5°, and 24.1° (2θ) appeared for the MS-SA complexes prepared under the pressure of 10 MPa and 30 MPa. With increasing pressure to 50 MPa, diffraction peaks at 12.7o (2θ) appeared for MS-SA complexes. The peaks at 21.5° and 24.1° (2θ) are attributed to free SA aggregates, and peaks at 12.7° and 19.7° (2θ) are attributed to the formation of the V-type crystallites. The high-pressure homogenization significantly affected the long-range order of complexes. The RC increases from 3.36% to 17.92% with the increasing pressure from 10 to 90 MPa (Table 1), suggesting that the long-range order of MS-SA complexes significantly increase with the increasing pressure. This is consistent with previous studies on the effect of HPH on the long-range ordered structure of rice starch-fatty acid complexes (Cui et al., 2021).
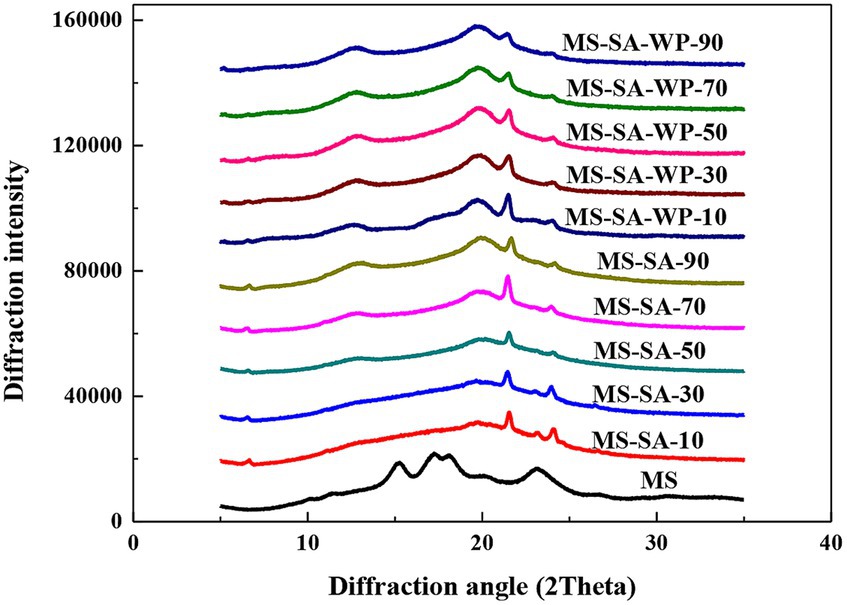
Figure 1. X-ray diffraction patterns of MS, MS-SA, and MS-SA-WP complexes prepared at different homogeneous pressures. MS-SA-WP-n represents the maize starch-stearic acid-whey protein complexes prepared under n MPa homogeneous pressure. MS, maize starch; SA, stearic acid; WP, whey protein.
The MS-SA-WP complexes prepared by different homogeneous pressures showed similar diffraction patterns with four obvious diffraction peaks at 12.7°, 19.7°, 21.5°, and 24.1° (2θ). The relative crystallinity of V-type crystallites also increased from 13.02% to 20.06% with the increasing homogeneous pressure from 10 to 50 MPa, but it did not change significantly with increasing the pressure from 50 to 90 MPa (Table 1). The above results suggest that the long-range ordered structure of MS-SA-WP complexes increases with the increase of the homogeneous pressure within a certain pressure range (10–50 MPa), which is attributed to the release of amylose and the better dispersibility of SA in starch dispersion by the homogeneous pressure (Chen et al., 2017). Despite an increase in homogeneous pressure, the long-range order of the MS-SA-WP complexes remained unchanged, suggesting that a sustained increase in the homogeneous pressure does not consistently improve the long-range order of the MS-SA-WP complexes. Under the same homogeneous pressure, the peak intensity and relative crystallinity of the MS-SA-WP complexes were significantly higher than that of the MS-SA complexes, which can be explained that the increased dispersity of SA in the system caused by the emulsification of whey protein.
3.2 Short-range order of MS and complexes
The full width at half maximum of the Raman band at 480 cm−1 (FWHM480) can be used to characterize the short-range order of the starch and starch-lipid complexes (Wang et al., 2017b; Qin et al., 2019). The FWHM480 of MS is 15.10. There were obvious differences in the FWHM480 of the MS-SA complexes prepared by different homogeneous pressures, so does the MS-SA-WP complexes (Table 1). The larger value of FWHM480 corresponds to the smaller the short-range molecular ordering of the complexes. With increasing homogeneous pressure, the value of FWHM480 decreases from 19.84 to 14.70 and from 18.22 to 13.56 for MS-SA and MS-SA-WP complexes, respectively, indicating that the short-range order of MS-SA and MS-SA-WP complexes increases with increasing homogeneous pressure. The results of short-range order of MS-SA complexes were consistent with the long-range order, but the changes of the short-range order of MS-SA-WP complexes with the increasing homogeneous pressure were different from the long-range order. This may be due to the fact that in the presence of WP, the increase of homogeneous pressure can only increase the short-range order of the complex, which is not enough to further arrange to form more ordered crystal structure. For MS-SA and MS-SA-WP complexes prepared with the same homogeneous pressure, the MS-SA-WP complexes had a smaller value of FWHM480, indicating the MS-SA-WP complexes have a greater short-range order, consistent with the XRD results.
3.3 FTIR spectra
FTIR can be used to identify functional groups and the presence of guest molecules in complexes. The FTIR spectra of MS and complexes are shown in Figure 2. In comparison with native MS, the bands at 2,857 and 1,710 cm−1 were observed in the spectra of MS-SA complexes, which were attributed to asymmetric C-H stretching and carbonyl groups stretching vibration of SA, respectively (Guillen and Cabo, 1997). This finding suggests the formation of starch-lipid complexes. However, the peak at 1,710 cm−1 was not observed in MS-SA-WP complexes due to the formation of the MS-SA-WP complexes attenuating the FTIR absorbance of the carbonyl group of SA (Wang et al., 2017c; Zheng et al., 2018; Niu et al., 2020). A new absorption band in 1,542 cm−1 appeared in MS-SA-WP complexes, which was attributed to the deformation vibration of amino acids in WP and the absorption of the amide II region (Zhen et al., 2022).
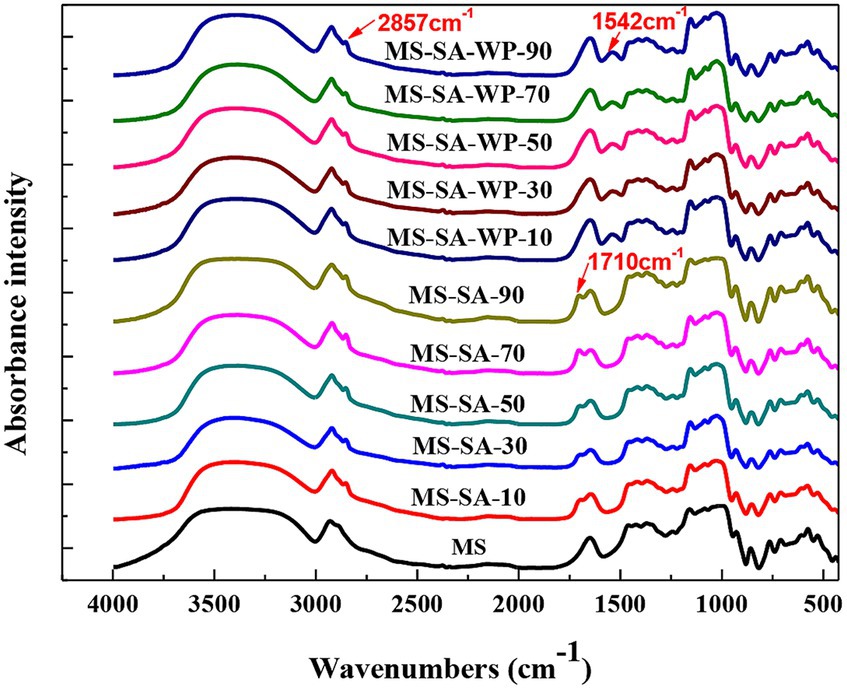
Figure 2. FTIR spectra of MS and complexes prepared at different homogeneous pressures. MS-SA-WP-n represents the maize starch-stearic acid-whey protein complexes prepared under n MPa homogeneous pressure. MS, maize starch; SA, stearic acid; WP, whey protein.
3.4 Thermal properties of complexes
The thermograms of MS, MS-SA and MS-SA-WP complexes are presented in Figure 3. The native MS presented an obvious gelatinization endothermic transition, with thermal transition temperatures in the range of 68.4–78.3°C and enthalpy change (ΔH) of 11.2 J/g. This endothermic transition was not observed in the complexes prepared by the high-pressure homogenization, suggesting the complete gelatinization of starch. Other two endothermic transitions were showed for all complexes samples, the transitions at 65°C–75°C represents the melting of the recrystallized SA, and the one at 96°C–113°C attributed to the dissociation of complexes. Thermal transition parameters of the MS-SA and MS-SA-WP complexes were obtained by analyzing thermograms, as shown in Table 2. With the homogenization pressure increasing from 10 to 90 MPa, the thermal transition peak temperature (Tp) of MS-SA complexes and MS-SA-WP complexes increased from 103.6°C to 107.6°C, and from 104.9°C to 108.3°C, respectively, suggesting that the thermal stability of the complexes improved with the increasing homogeneous pressure. With the increasing homogeneous pressure, the enthalpy change (∆H) of MS-SA complexes and MS-SA-WP complexes increased from 1.2 to 3.3 J/g, and from 1.6 to 3.5 J/g, respectively, indicating that the number of complexes also increases with the increasing homogeneous pressure. The ∆H of the MS-SA-WP complexes is higher than corresponding MS-SA complexes prepared with the same homogeneous pressure, for example, the ∆H of the MS-SA complexes and the MS-SA-WP complexes prepared with 10 MPa is 1.2 and 1.6 J/g, respectively, which can be explained that the increasing emulsifying ability due to the presence of WP facilitate the formation of the complexes among MS, SA and WP (Du et al., 2023). The ∆H results of the MS-SA-WP complexes are consistent with the result of short-range order but inconsistent with the result of long-range order. That is, the higher homogeneous pressure (50–90 MPa) only forms more complexes with better short-range order, but those does not further arrange into a crystal structure with better long-range order.
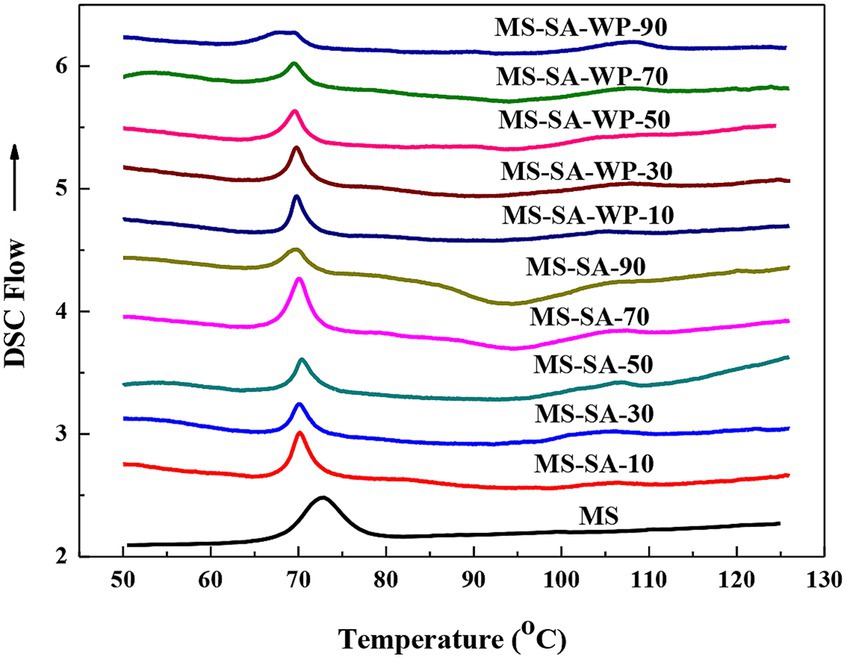
Figure 3. DSC spectra of MS and complexes prepared at different homogeneous pressures. MS-SA-WP-n represents the maize starch-stearic acid-whey protein complexes prepared under n MPa homogeneous pressure. MS, maize starch; SA, stearic acid; WP, whey protein.
3.5 Granules morphology
The SEM micrographs of MS, MS-SA and MS-SA-WP complexes are shown in Figure 4. Native maize starch is composed of granules with different shapes, such as spherical and polyhedral. And the pores and holes with different sizes were observed on the surface of some granules. The starch granules in all the complex samples were completely destroyed into debris with different sizes due to the high temperature and pressure during the preparation of the complex. The debris were getting smaller gradually with the increase of homogeneous pressure from 10 to 90 MPa for MS-SA complexes, and the surface of debris were covered with some irregularly-shaped fragments (red arrows). This observation was in accordance with previous studies, which represented the appearance of the V-type complex (Chen et al., 2017, 2018). Differently, MS-SA-WP complexes prepared with different homogeneous pressure showed relatively uniform debris, this can be attributed to the fact that the presence of WP makes MS and SA better dispersed and then more evenly subjected to shear forces by high-pressure homogenization. The regularly-shaped fragments (green arrows) were still observed for MS-SA-WP complexes.
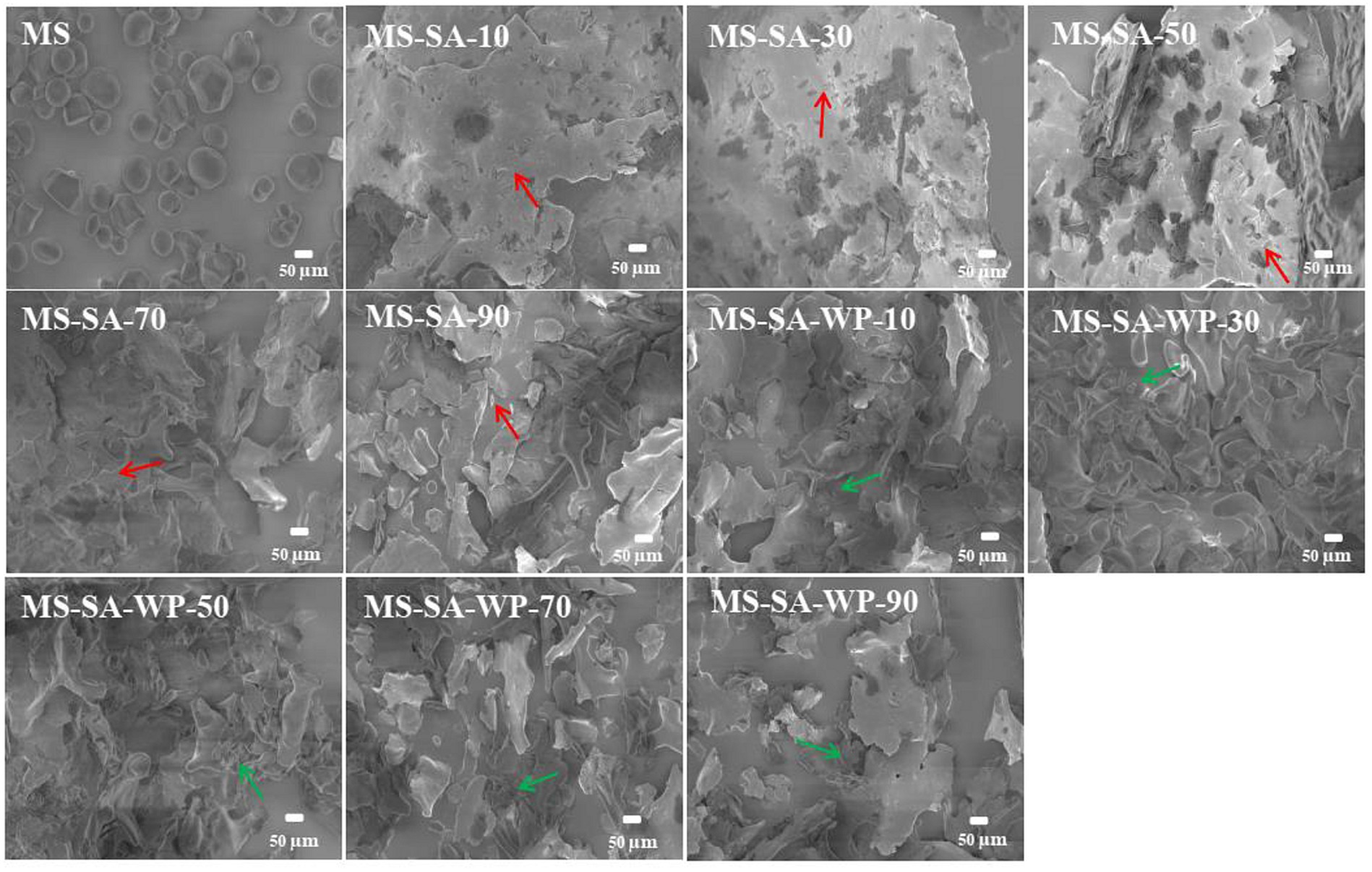
Figure 4. The scanning electronic micrographs of MS and complexes prepared at different homogeneous pressures at 1,000× magnification. MS-SA-WP-n represents the maize starch-stearic acid-whey protein complexes prepared under n MPa homogeneous pressure. MS, maize starch; SA, stearic acid; WP, whey protein.
3.6 In vitro digestibility of complexes
Figure 5 displays the digestion curves of the MS-SA and the MS-SA-WP complexes prepared with the different homogenization pressure. The digestion curves of all complexes are similar, but digestion extent at each digestion time shows differences. All samples showed a gradual increase in the degree of digestion at 0–240 min, with a rapidly increased digestion rate for the first 60 min, subsequently slowly increased rate and leveled off at 200 min. The MS-SA complexes prepared by 10–90 MPa showed no significant difference in the digestion degree within 40 min. The degree of digestion of MS-SA complexes prepared at different homogeneous pressures showed increasingly distinct differences with increasing digestion time. The degree of hydrolysis reached 83.55%, 82.37%, 79.59%, 79.28%, and 77.48% at 240 min, respectively, for MS-SA complexes prepared with 10–90 MPa. That is, the final digestion extent of the MS-SA complexes was gradually decreased with increasing pressure. These results were in agreement of XRD, DSC and Raman results, suggesting that the digestion of MS-SA complexes is correlated with the degree of structural order. And the digestion degree of MS-SA-WP complexes prepared by different homogeneous pressures showed similar changes trends to that of the MS-SA complexes, but the digestion degree at 240 min were lower. Moreover, the MS-SA-WP complexes have lower hydrolysis content than the corresponding MS-SA complexes prepared with the same homogenization pressure, indicating that the addition of WP increased enzymatic resistance of complexes.
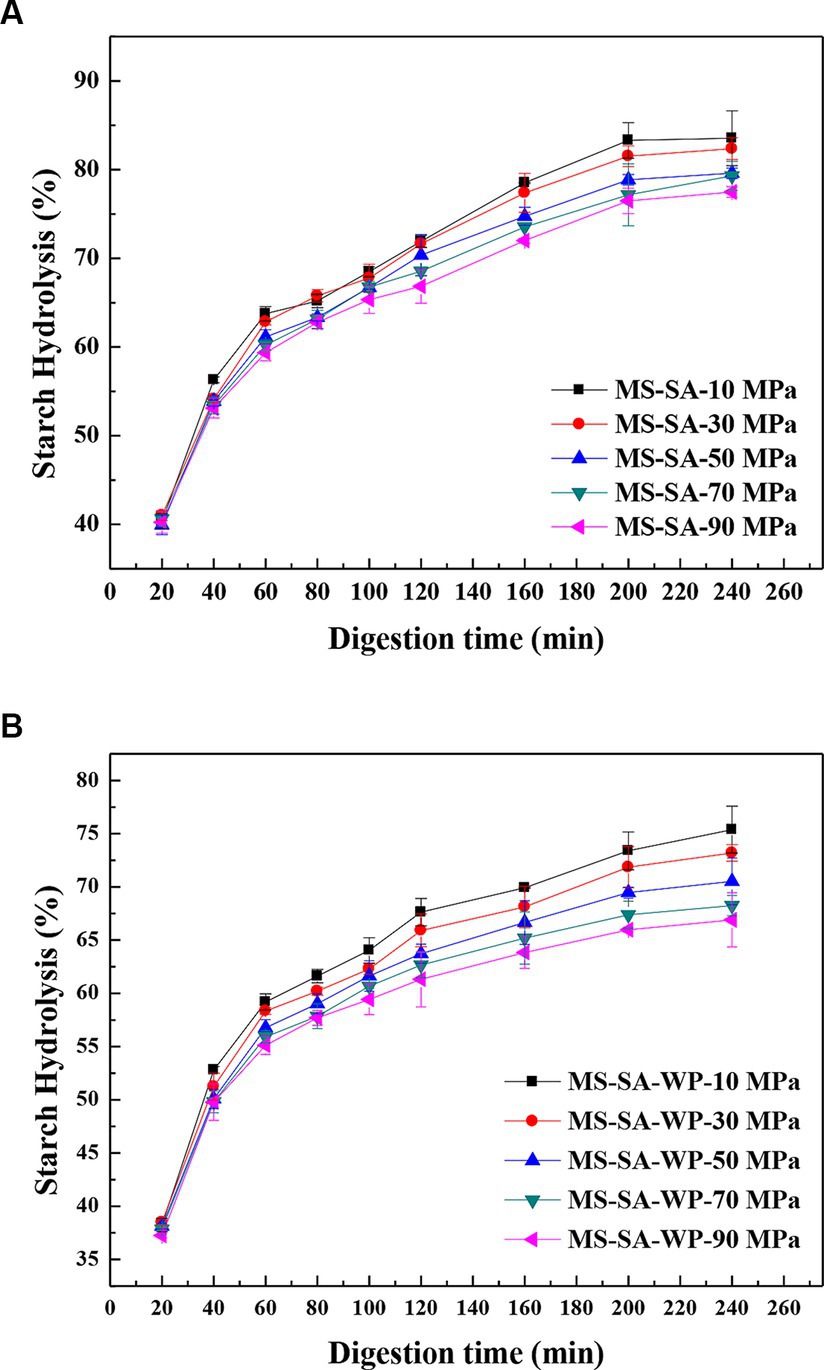
Figure 5. In vitro starch digestograms of complexes prepared under different homogeneous pressures. MS-SA-WP-n represents the maize starch-stearic acid-whey protein complexes prepared under n MPa homogeneous pressure. MS, maize starch; SA, stearic acid; WP, whey protein.
To further analyze the digestion of the complexes, the content of RDS, SDS and RS of the complexes were calculated based on the digestion curves, and the results are appeared in Table 3. For the MS-SA complexes prepared with different homogeneous pressures, the RDS content has no significant difference, but the amount of SDS decreased from 31.55 to 26.62 and RS increased from 28.08 to 33.15 with increasing pressure from 10 to 90 MPa, and the MS-SA-WP complexes showed a similar change pattern. The amount of RDS, SDS and RS show obvious differences between the MS-SA and MS-SA-WP complexes prepared with the same homogeneous pressure. Specifically, the MS-SA-WP complexes had less RDS and SDS and more RS compared to the MS-SA complexes. These results can guide us to design anti-digested starchy foods by regulating homogeneous pressure and components, which is very beneficial for slow the rising of postprandial glycemia index, especially for diabetics.
4 General discussion
In this study, the effect of HPH on structure and digestibility of MS-SA and MS-SA-WP complexes were studied. Results of XRD and Raman showed that the degree of short-range order and long-range order of MS-SA increased with increasing the homogeneous pressure. The result of DSC analyses suggested that greater amounts of MS-SA complexes with better thermal stability were formed as the homogeneous pressure increased. These can be attributed to the strong shear force generated by the higher pressure homogenization, which makes MS and SA better dispersed so that they can interact better (Guo et al., 2021). For MS-SA-WP complexes, the short-range order, amount and thermal stability also increased with increasing the homogeneous pressure, but the long-range order only increased with increasing the homogeneous pressure from 10 to 50 MPa. It is considered that the emulsification of whey protein and the shear action of high-pressure will synergically increase the solubility of lipid (Shuang et al., 2014; Zheng et al., 2018), and the better shear action at higher homogeneous pressure and emulsification of whey protein are sufficient to disperse the lipids well, so the increasing continuously the homogeneous pressure in the presence of WP will not significantly increase the diffraction peak intensities of V-type crystallites. This also suggested that the simply increasing the homogeneous pressure cannot always improve the long-range order of the MS-SA-WP complexes, and other preparation conditions, such as treatment temperature, can be considered if necessary. The MS-SA-WP complexes presented the better short-range and long-range order and higher enthalpy change compared with MS-SA complexes prepared with the same homogeneous pressure, which can be attributed to the emulsification of whey protein.
To gain a better understanding of structure-digestion relationships for these complexes, Pearson correlation analyses were performed (Figure 6). The in vitro digestibility is closely related to the amount and structural order of complexes. The complexes with better long-range and short-range ordered structures had greater barrier effect on enzyme access and better enzymatic resistance. Results of correlation analyses showed the content of RS was positively correlated with the gelatinization peak temperature (Tp; r = 0.765, p < 0.01), the enthalpy change (∆H; r = 0.775, p < 0.01), the relative crystallinity (r = 0.919, p < 0.01), negatively correlated with the FWHM480 (r = −0.876, p < 0.01). The content of SDS was negatively correlated with Tp (r = −0.736, p < 0.05), ∆H (r = −0.93, p < 0.01) and RC (r = −0.819, p < 0.01), but positively correlated with FWHM480 (r = 0.933, p < 0.01). The RDS content was negatively correlated with RC (r = −0.71, p < 0.05). These results indicate that complexes with better structural stability prepared by HPH have better anti-digestion properties rather than the slow digestion, and the MS-SA-WP complexes have more RS than the MS-SA complexes.
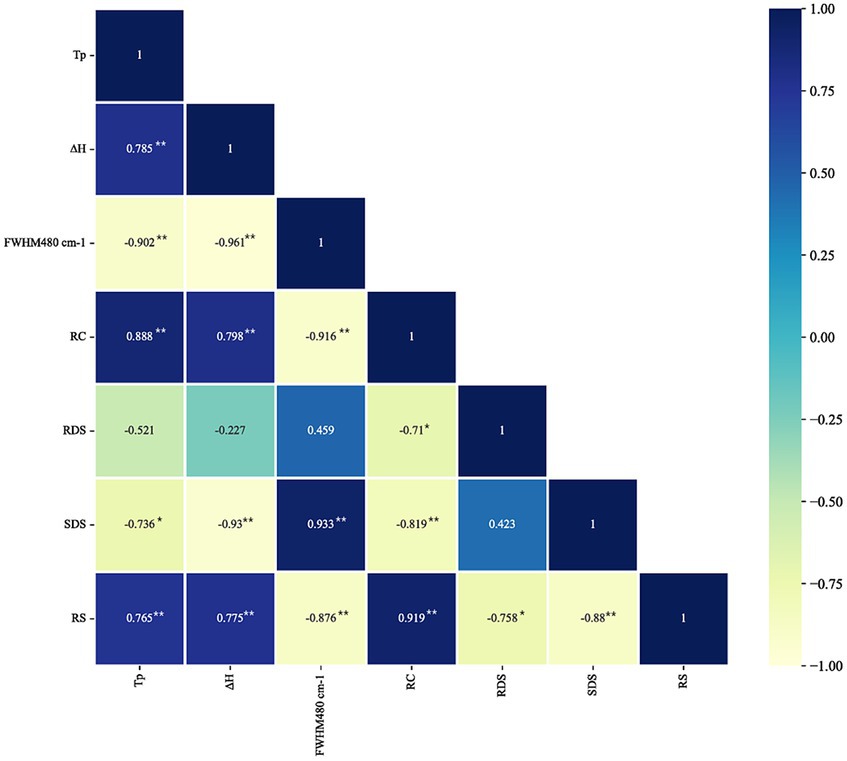
Figure 6. Pearson correlation coefficient matrix among the variables of MS-SA and MS-SA-WP complexes. * and ** represent significance at the p < 0.05 and p < 0.01 levels, respectively.
5 Conclusion
In conclusion, high-pressure homogeneity can affect the structure of MS-SA and MS-SA-WP complexes, further affecting the digestion. With increasing homogeneity pressure, the amount, short-range order, long-range order and thermal stability of MS-SA complexes increased and the in vitro digestibility decreased. The amount, short-range order and thermal stability of MS-SA-WP complexes also increased with increasing homogeneity pressure, but the long-range order of MS-SA-WP complexes does not show a continuous improvement as the increased homogeneity pressure. Compared with MS-SA complexes, the addition of WP could form more MS-SA-WP complexes with more stable ordered structure. The MS-SA-WP complexes have a lower digestibility than corresponding MS-SA complexes prepared with the same homogenization pressure. This study deepens the understanding of the structure and digestibility of starch-lipid and starch-lipid-protein complexes prepared by high-pressure homogenization.
Data availability statement
The original contributions presented in the study are included in the article/supplementary material, further inquiries can be directed to the corresponding author.
Author contributions
KH: Data curation, Formal analysis, Investigation, Writing – original draft. YW: Data curation, Formal analysis, Investigation, Writing – original draft. XP: Data curation, Formal analysis, Investigation, Writing – original draft. BZ: Investigation, Software, Writing – original draft. FW: Validation, Writing – review & editing. XL: Conceptualization, Formal analysis, Funding acquisition, Supervision, Validation, Writing – review & editing.
Funding
The author(s) declare financial support was received for the research, authorship, and/or publication of this article. The authors gratefully acknowledge the financial support from the Shandong Provincial Natural Science Foundation (ZR2022QC015) and the Student Research Training Program of the Shandong First Medical University (2022104391080).
Conflict of interest
The authors declare that the research was conducted in the absence of any commercial or financial relationships that could be construed as a potential conflict of interest.
Publisher’s note
All claims expressed in this article are solely those of the authors and do not necessarily represent those of their affiliated organizations, or those of the publisher, the editors and the reviewers. Any product that may be evaluated in this article, or claim that may be made by its manufacturer, is not guaranteed or endorsed by the publisher.
References
Bojarczuk, A., Skąpska, S., Mousavi Khaneghah, A., and Marszałek, K. (2022). Health benefits of resistant starch: A review of the literature. J. Funct. Foods 93:105094. doi: 10.1016/j.jff.2022.105094
Cervantes-Ramírez, J. E., Cabrera-Ramirez, A. H., Morales-Sánchez, E., Rodriguez-García, M. E., Reyes-Vega, M., Ramírez-Jiménez, A. K., et al. (2020). Amylose-lipid complex formation from extruded maize starch mixed with fatty acids. Carbohydr. Polym. 246:116555. doi: 10.1016/j.carbpol.2020.116555
Chao, C., Huang, S., Yu, J., Copeland, L., Wang, S., and Wang, S. (2020). Molecular mechanisms underlying the formation of starch-lipid complexes during simulated food processing: A dynamic structural analysis. Carbohydr. Polym. 244:116464. doi: 10.1016/j.carbpol.2020.116464
Chen, B., Jia, X., Miao, S., Zeng, S., Guo, Z., Zhang, Y., et al. (2018). Slowly digestible properties of lotus seed starch-glycerine monostearin complexes formed by high pressure homogenization. Food Chem. 252, 115–125. doi: 10.1016/j.foodchem.2018.01.054
Chen, B., Zeng, S., Zeng, H., Guo, Z., Zhang, Y., and Zheng, B. (2017). Properties of lotus seed starch–glycerin monostearin complexes formed by high pressure homogenization. Food Chem. 226, 119–127. doi: 10.1016/j.foodchem.2017.01.018
Cui, J., Zheng, B., Liu, Y., Chen, L., Li, B., and Li, L. (2021). Insights into the effect of structural alternations on the digestibility of rice starch-fatty acid complexes prepared by high-pressure homogenization. LWT. 136:110294. doi: 10.1016/j.lwt.2020.110294
Du, J., Lv, M. Y., Zhang, H. L., Xiao, S. S., Zheng, S. Y., and Wang, X. D. (2023). Synergistic effect of endogenous gluten and oleic acid on wheat starch digestion by forming ordered starch-fatty acid-protein complexes during thermal processing. Curr Res Food Sci. 6:100422. doi: 10.1016/j.crfs.2022.100422
Duan, Y., Chao, C., Yu, J., Liu, Y., and Wang, S. (2023). Effects of different sources of proteins on the formation of starch-lipid-protein complexes. Int. J. Biol. Macromol. 253:126853. doi: 10.1016/j.ijbiomac.2023.126853
Englyst, H. N., Kingman, S. M., and Cummings, J. H. (1992). Classification and measurement of nutritionally important starch fractions. Eur. J. Clin. Nutr. 46, 33–50.
Guillen, M., and Cabo, N. (1997). Infrared spectroscopy in the study of edible oils and fats. J. Sci. Food Agric. 75, 1–11. doi: 10.1002/(SICI)1097-0010(199709)75:1<1::AID-JSFA842>3.0.CO;2-R
Guo, T., Hou, H., Liu, Y., Chen, L., and Zheng, B. (2021). In vitro digestibility and structural control of rice starch-unsaturated fatty acid complexes by high-pressure homogenization. Carbohydr. Polym. 256:117607. doi: 10.1016/j.carbpol.2020.117607
Gutiérrez, T. J., and Tovar, J. (2021). Update of the concept of type 5 resistant starch (RS5): self-assembled starch V-type complexes. Trends Food Sci. Technol. 109, 711–724. doi: 10.1016/j.tifs.2021.01.078
Li, Q., Shi, S., Du, S.-k., Dong, Y., and Yu, X. (2021). Starch–palmitic acid complex formation and characterization at different frying temperature and treatment time. LWT. 136:110328. doi: 10.1016/j.lwt.2020.110328
Liu, X., Chao, C., Yu, J., Copeland, L., and Wang, S. (2021). Mechanistic studies of starch retrogradation and its effects on starch gel properties. Food Hydrocoll. 120:106914. doi: 10.1016/j.foodhyd.2021.106914
Liu, X., Huang, S., Chao, C., Yu, J., Copeland, L., and Wang, S. (2022). Changes of starch during thermal processing of foods: current status and future directions. Trends Food Sci. Technol. 119, 320–337. doi: 10.1016/j.tifs.2021.12.011
Liu, P., Wang, R., Kang, X., Cui, B., and Yu, B. (2018). Effects of ultrasonic treatment on amylose-lipid complex formation and properties of sweet potato starch-based films. Ultrason. Sonochem. 44, 215–222. doi: 10.1016/j.ultsonch.2018.02.029
Niu, B., Chao, C., Cai, J., Yan, Y., Copeland, L., Yu, J., et al. (2020). Effect of pH on formation of starch complexes with lauric acid and β-lactoglobulin. LWT 132:109915. doi: 10.1016/j.lwt.2020.109915
Putseys, J. A., Lamberts, L., and Delcour, J. A. (2010). Amylose-inclusion complexes: formation, identity and physico-chemical properties. J. Cereal Sci. 51, 238–247. doi: 10.1016/j.jcs.2010.01.011
Qin, R., Yu, J., Li, Y., Copeland, L., Wang, S., and Wang, S. (2019). Structural changes of starch-lipid complexes during postprocessing and their effect on in vitro enzymatic digestibility. J. Agric. Food Chem. 67, 1530–1536. doi: 10.1021/acs.jafc.8b06371
Shi, S., Dong, Y., Li, Q., Liu, T., and Yu, X. (2020). Morphology, structural, thermal and rheological properties of wheat starch–palmitic acid complexes prepared during steam cooking. RSC Adv. 10, 30087–30093. doi: 10.1039/d0ra05954d
Shuang, M., Ying, M., Sun, D. W., Wang, L., and Liu, T. (2014). Properties of starch-palmitic acid complexes prepared by high pressure homogenization. J. Cereal Sci. 59, 25–32. doi: 10.1016/j.jcs.2013.10.012
Wang, S., Chao, C., Cai, J., Niu, B., Copeland, L., and Wang, S. (2020). Starch–lipid and starch–lipid–protein complexes: A comprehensive review. Compr. Rev. Food Sci. Food Saf. 19, 1056–1079. doi: 10.1111/1541-4337.12550
Wang, C., Chao, C., Yu, J., Copeland, L., Huang, Y., and Wang, S. (2022). Mechanisms underlying the formation of amylose-Lauric acid-beta-Lactoglobulin complexes: experimental and molecular dynamics studies. J. Agric. Food Chem. 70, 10635–10643. doi: 10.1021/acs.jafc.2c04523
Wang, Y., Chen, L., Yang, T., Ma, Y., McClements, D. J., Ren, F., et al. (2021). A review of structural transformations and properties changes in starch during thermal processing of foods. Food Hydrocoll. 113:106543. doi: 10.1016/j.foodhyd.2020.106543
Wang, S., Wang, S., Guo, P., Liu, L., and Wang, S. (2017a). Multiscale structural changes of wheat and yam starches during cooking and their effect on in vitro enzymatic digestibility. J. Agric. Food Chem. 65, 156–166. doi: 10.1021/acs.jafc.6b04272
Wang, S., Wang, S., Liu, L., Wang, S., and Copeland, L. (2017b). Structural orders of wheat starch do not determine the in vitro enzymatic digestibility. J. Agric. Food Chem. 65, 1697–1706. doi: 10.1021/acs.jafc.6b04044
Wang, S., Zheng, M., Yu, J., Wang, S., and Copeland, L. (2017c). Insights into the formation and structures of starch-protein-lipid complexes. J. Agric. Food Chem. 65, 1960–1966. doi: 10.1021/acs.jafc.6b05772
Xu, H., Zhou, J., Yu, J., Wang, S., Copeland, L., and Wang, S. (2020). Revealing the mechanisms of starch amylolysis affected by tea catechins using surface plasmon resonance. Int. J. Biol. Macromol. 145, 527–534. doi: 10.1016/j.ijbiomac.2019.12.161
Zhen, Y., Wang, K., Wang, J., Qiao, D., Zhao, S., Lin, Q., et al. (2022). Increasing the pH value during thermal processing suppresses the starch digestion of the resulting starch-protein-lipid complexes. Carbohydr. Polym. 278:118931. doi: 10.1016/j.carbpol.2021.118931
Keywords: high-pressure homogenization, structure, maize starch-stearic acid complexes, maize starch-stearic acid-whey protein complexes, digestibility
Citation: Han K, Wu Y, Peng X, Zhou B, Wang F and Liu X (2024) Effect of high-pressure homogenization on maize starch-stearic acid and maize starch-stearic acid-whey protein complexes. Front. Sustain. Food Syst. 8:1324217. doi: 10.3389/fsufs.2024.1324217
Edited by:
Haiteng Li, Jiangsu University, ChinaReviewed by:
Asad Mohammad Amini, University of Kurdistan, IranGiovanni Barone, University of Copenhagen, Denmark
Copyright © 2024 Han, Wu, Peng, Zhou, Wang and Liu. This is an open-access article distributed under the terms of the Creative Commons Attribution License (CC BY). The use, distribution or reproduction in other forums is permitted, provided the original author(s) and the copyright owner(s) are credited and that the original publication in this journal is cited, in accordance with accepted academic practice. No use, distribution or reproduction is permitted which does not comply with these terms.
*Correspondence: Xia Liu, liuxia@sdfmu.edu.cn
†These authors have contributed equally to this work and share first authorship