- 1Division of Environment Science, ICAR-Indian Agricultural Research Institute, New Delhi, India
- 2INTI International University, Persiaran Perdana BBN, Putra Nilai, Negeri Sembilan, Malaysia
- 3ICAR-Indian Institute of Maize Research, New Delhi, India
- 4Indira Gandhi National Open University, New Delhi, India
- 5Tamil Nadu Agricultural University, Coimbatore, India
- 6Division of Agronomy, ICAR-Indian Agricultural Research Institute, New Delhi, India
- 7ICAR-Directorate of Weed Research, Jabalpur, Madhya Pradesh, India
- 8Anbil Dharmalingam Agricultural College, Tamil Nadu Agricultural University, Trichy, India
- 9Division of Agricultural Physics, ICAR-Indian Agricultural Research Institute, New Delhi, India
- 10ICAR-Indian Institute of Soil Science, Bhopal, Madhya Pradesh, India
- 11ICAR-Indian Institute of Soil and Water Conservation, Research Centre, Udhagamandalam, Tamil Nadu, India
- 12Forest College and Research Institute, Tamil Nadu Agricultural University, Coimbatore, India
- 13National Agro Foundation, Chennai, India
- 14Tamilnadu Pollution Control Board, Chennai, India
- 15ICAR-National Academy of Agricultural Research Management, Hyderabad, Telangana, India
- 16ICAR Research Complex for Eastern Region, Patna, India
- 17ICAR – Indian Institute of Maize Research, Ludhiana, India
Maize-based crop systems are promoted in large scale in South Asia because they are more sustainable and efficient than rice-based systems. In the present study, using two combinations of crop residue management practices (CRM) with four precision nitrogen (N) management (PNM) systems, we assessed the impacts on soil physicochemical characteristics [soil organic carbon (SOC), bulk density (BD), soil penetration resistance (PR)] and crop yields in 6 years old continuous zero tillage (ZT) practices under maize-wheat-mungbean cropping system in a sandy loam soil of northwestern India. The highest SOC (5.73 g/kg) was observed in Zero Tillage with Residue Retention (ZT + R) plots. Zero-tillage with residue retention (ZT + R) significantly reduced the bulk density over the zero-tillage with no residue retention (ZT-R) across the soil depth. The bulk density in ZT + R was 6.5 and 10.7% lower at 0–15 cm and 15–30 cm soil depth, respectively, than under ZT-R. The penetration resistance (PR) was significantly lower in ZT + R than in ZT-R across the soil depth. Soil organic carbon (SOC) in ZT + R was 7.4% higher at 0–15 cm depth and 11.9% higher at 15–30 cm depth than under ZT-R treatment. Among PNM treatments, the sequence of treatments in SOC content was 50%N + Green Seeker (GS) >33%N + GS > RDN > 70%N + GS. The system productivity (maize equivalent yield) under ZT + R in combination with 50%BN + GS was 15.0% higher than crops grown under ZT-R with RDN. The wheat equivalent yield under the ZT + R treatment is found to be higher (5.97) in the 50%BN + GS, which was 18% higher than the recommended dose of nitrogen treatment (5.04) and 28% higher than the 70%BN + GS treatment (4.68). Results demonstrated that plots with residue retention performed better, showing a 10% increase in system productivity. The study concludes that a ZT-based system with maize-based crop rotations (MWMb) with crop residue retention and precision nitrogen management can improve soil properties and system productivity in northwestern India.
1 Introduction
Agricultural intensification in the Indo-Gangetic plains of Northwestern India to meet the growing food demand is often accompanied by negative effects such as declining soil fertility, crop residue burning, biodiversity loss, soil degradation, and increase in greenhouse gas (GHG) emissions (Venkatramanan et al., 2021; Reddy et al., 2023). Farmers in the region clear crop residues more often because it is easier to manage and process the land for the next crop and provides short-term economic benefits. By 2030, about 207 million tonnes of maize (Zea mays L.) biomass will be removed for biofuel production (Muth Jr et al., 2013). On the other hand, increased residue removal threatens soil fertility and productivity (Mrunalini et al., 2022). As a result, there is a surge of interest in reusing agricultural wastes and employing conservation agriculture (CA) to sustain agricultural productivity (Chalise et al., 2019). Conservation agriculture is a climate-smart practice emphasizing low soil disturbance, long-term organic residue cover, and crop diversification (Eze et al., 2020).CA is a crucial instrument for sustainably securing future food production and protecting soil resources from extreme climatic events like drought and heatwaves (Page et al., 2020). The agricultural production system, to increase food production, minimize GHG emissions, and adapt to changing climate conditions, has to incorporate smart agricultural practices (Venkatramanan and Shah, 2019). Resilient agricultural practices like conservation agriculture, precision nutrient management, and crop residue management have been recommended to improve soil properties and achieve soil security (Venkatramanan et al., 2020). Crop residue removal decreases soil organic carbon (SOC) and increases soil penetration resistance (PR) and bulk density (BD), which has a significant negative impact on soil productivity. However, the CA effectively utilizing the previous crop residues (Dinesh et al., 2023) and long-term residue cover might increase SOC and improve soil-water dynamics, eventually boosting crop output and productivity (Basche et al., 2016). Many ecosystem services and benefits have been linked to conservation agriculture, including increased soil water storage (Page et al., 2019), improved soil quality (Jayaraman et al., 2019; Jayaraman and Dalal, 2022), reduced erosion, higher yields (Kadam et al., 2022) and net agricultural income (Page et al., 2019). Hence, smallholder adoption of CA is being promoted by both governmental and non-governmental organizations in developing nations around the world (Eze et al., 2020), and there is a lot of scope for payment for ecosystem services to conservation agriculture-practicing farmers in the near future (Dinesh et al., 2021; Rasheed et al., 2021).
In tillage investigations, parameters such as BD, PR, and SOC influence the system’s productivity and soil properties. Crop residue incorporation through conservation tillage practices decreases soil bulk density and increases soil biota activity (Sharma et al., 2017). The bulk density will be much greater in the first few years (up to five) of conservation agriculture (CA). However, compared to conventional tillage, CA will have a lower soil bulk density after stabilization. Hence, the effect of conservation tillage on BD was not instantaneous; compared to conventional tillage (plowing), it may take a few years to show noticeable results in dropping BD. Because crop residues are lighter than minerals, their breakdown products induce more aggregation (Shaver, 2010). Previous research has found that diverse zero-till (ZT) systems had a lower PR value in the upper soil layers (0–20 cm) than conventional tillage systems (Singh and Malhi, 2006). The crop rotation and addition of crop residues reduced PR value, implying that cultivation systems significantly influence soil compaction (Indoria et al., 2017). Like BD, increased PR will negatively affect root growth (Lampurlanés and Cantero-Martínez, 2003). As the SOC improves, higher bulk densities can drop with time (Blanco-Canqui and Ruis, 2018). However, in some cases, increased BD limits production (Page et al., 2020) because of decreased air permeability (Nyagumbo et al., 2016).
Nitrogen significantly increases plant yield but it must be handled cautiously to prevent negative environmental effects. Depending on the crop and the amount of N fertilization, different crops respond differently to N treatment regarding physico-chemical characteristics and system productivity. In addition to lowering the potassium content of the soil that is accessible for use, high N fertilizer rates may also hinder photosynthesis and radiation efficiency, which lowers grain yield (Sun et al., 2020). On the other hand, effective N fertilization may enhance starch’s structure and physico-chemical characteristics, as well as protein’s water-holding capacity and thermal stability, all of which contribute to higher crop quality (Noor et al., 2023). Higher nutrient use efficiency may reduce the need for high fertilizer-N rate application and have a good effect on biodiversity, human health, and the quality of the air and water (Tian-yang et al., 2022). Therefore, to maximize crop output while reducing the detrimental effects on the ecosystem by N fertilizer, precise N management is essential to avoid N loss.
The soil texture of Indo-Gangetic Plains (IGP) is primarily sandy loam (Typic Haplustept; Inceptisols). Higher BD, low water retention capacity, and lower SOC are the critical constraints of this type of soil (Singh et al., 2016). These concerns have forced the Indian government to prioritize diversification with maize and legumes, particularly in the rice-wheat rotation in northwestern Indo-Gangetic plains (IARI, 2012). However, compared to conventional tillage, no-tillage techniques and diverse maize cropping systems improve soil physical, chemical, and biological qualities and overall soil health (Dinesh et al., 2022a). Fertilizer-N has been shown to impact several plant physiological processes and metabolic activities, including respiration rate, water balance, and signaling pathways. The sustainability of maize-based agroecosystems and the most accurate way to calculate fertilizer N use efficiency have been researched in light of the likelihood that N fertilizer enhances soil organic matter (SOM) mineralization and, as a consequence, diminishes SOM stocks. The fact that synthetic N fertilizer may alter SOM mineralization in both good and negative ways via several direct and indirect channels is a major factor in this argument (Mahal et al., 2019). Thus, the study’s hypothesis was crop residue, and N management (precision N application with green seeker) coupled with zero tillage would positively impact crucial soil properties compared to conventional practices without crop residue addition with the recommended dose of fertilizer. Though many studies on CA and soil quality have been reported, there is still a significant knowledge gap about CA coupled with PNM and CRM, particularly its impacts on soil properties and their interrelationship with crop yields and system productivity (Dougill et al., 2017). In light of this, this research was carried out with the objective of studying the effects of crop residue retention and precision N management on soil organic carbon, bulk density, soil penetration resistance, crop yields and system productivity in a six-year-old conservation agriculture experiment on sandy loam soil in northwestern Indo-Gangetic plains.
2 Materials and methods
2.1 Experimental site
A field experiment was conducted in the long-term conservation agriculture-based research farm in the Northwestern Indo-Gangetic plains at ICAR-Indian Agricultural Research Institute, New Delhi, India (28° 40′ N, 77° 12′ E, and 228.6 m above MSL) from 2018 to 2020. The experimental farm has sandy loam soil (Typic Haplustept; Inceptisols) and experienced a semiarid climate with hot and dry summer and cold winter. The meteorological conditions that prevailed during the study period are shown in Figures 1, 2. The average annual rainfall during 2018 and 2019 was 966.80 mm and 859.40 mm, respectively. During the Southwest monsoon, 80% of the annual rainfall is received, and the remaining is received during the winter months as western disturbances.
2.2 Experimental details
The field trial was conducted in a long-term experimental site since 2012, and the current study was conducted for 2 years with three seasons, i.e., 2018–19 and 2019–20 (6th and 7th year of a long-term experiment). Table 1 presents experimental details, including the tillage, crop establishment, and residue retention under maize-wheat-mungbean (MWMb) systems. The study was initiated with the sowing of maize (cv. PMH 1) in mid-July, followed by wheat (cv. HD 2967) in the first week of November, and summer mungbean (cv. Pusa Vishal) in mid-April. The experiment was carried out with two main-plot treatments (main factor) (Crop residue management (CRM) options), namely zero tillage with residue retention (ZT + R) and zero tillage without residue retention (ZT-R), along with four sub-plot treatments (subfactor) of precision nitrogen management (PNM) options namely (i) The recommended dose of nitrogen (RDN) (150, 120 and 18 kg/ha for maize, wheat, and mungbean, respectively), (ii) 33% basal RDN followed by Green Seeker N application (33%N + GS), (iii) 50% basal RDN followed by Green Seeker N application (50%N + GS), (iv) 70% basal RDN followed by Green Seeker N application (70%N + GS). The recommended amount of fertilizer (as per recommendations of IARI, New Delhi package of practices based on soil test) applied was 150:60:40, 120:60:40, and 18:46:0 (N: P2O5:K2O kg/ha) for maize, wheat, and mungbean, respectively. The nitrogen doses varied across the treatments based on the green seeker readings; the phosphorous and potassium remained equivalent in all the treatments. The calculations are done by Sensor-Based Nitrogen Rate Calculator developed by Oklahoma State University.1 For maize, fertilizers are applied at three times. Basal dose at 0 DAS, Knee High stage (35 DAS), Silking stage (65 DAS). For wheat, fertilizers are applied at Basal (0 DAS), Crown Root Initiation stage (25 DAS), active tillering stage (70 DAS). P and K nutrients are applied based on blanket recommendations. For Mungbean, only basal dose (18 kg N and 46 Kg P) is applied. In zero tillage without residues plots (ZT-R), under 33%BN + GS, 50%BN + GS, and 70%BN + GS treatments, 33, 50 and 70% of N is applied as basal (50, 75, 105 kg N for maize and 40, 65, 95 kg N for wheat) and remaining will be based on the green seeker readings at critical stages mentioned above. Detailed application of fertilizers is mentioned in the Table 2.
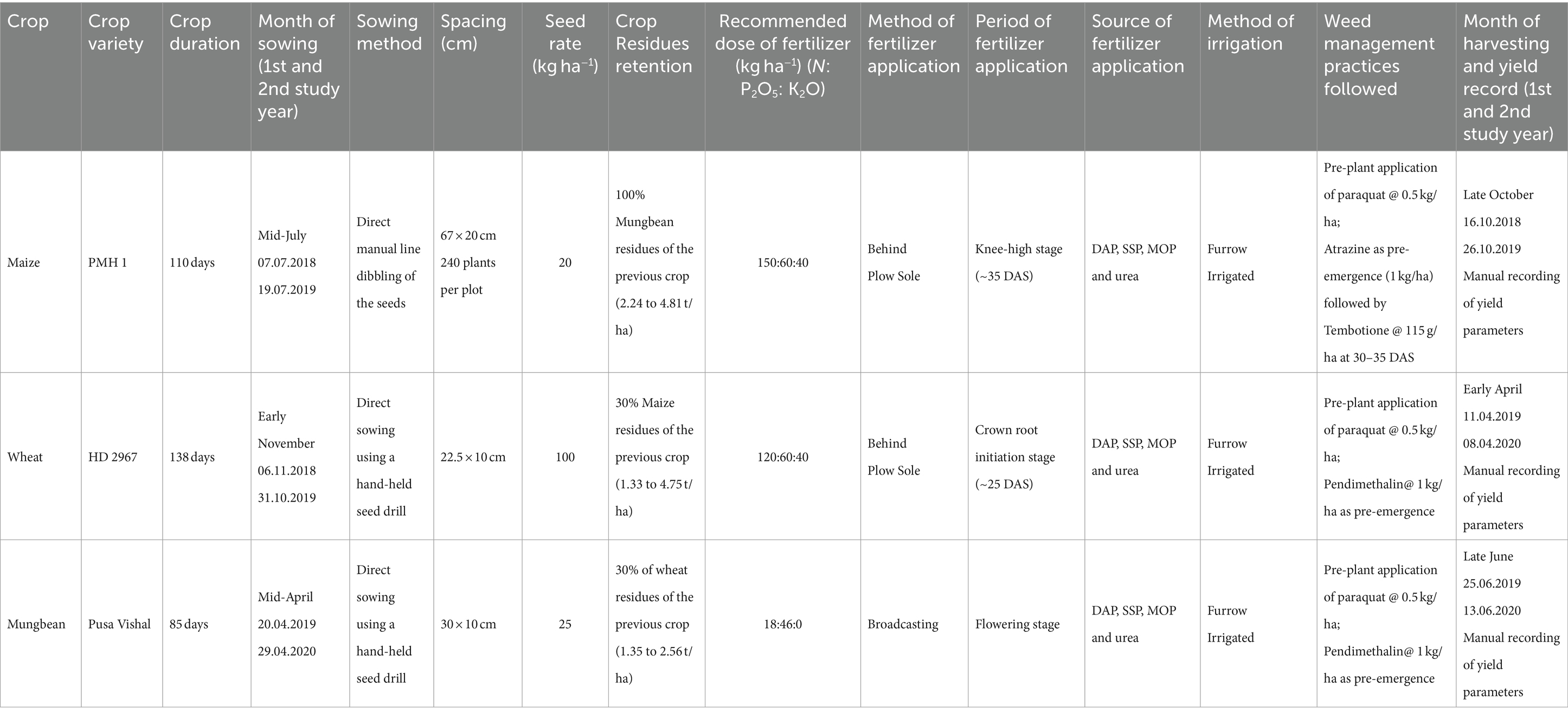
Table 1. Description of tillage, crop establishment, and residue practices under maize-wheat-mungbean (MWMb) systems.
2.3 Collection and processing of soil samples
In July 2018 and 2019 (before the sowing, typically after harvesting of the previous crop), the soil samples were taken from each plot (2 main plots (CRM) × 4 subplots (PNM) × 3 replications = 24 plots) at 3–5 random locations covering 25% of the plot. A composite soil sample was prepared using the quadrant method to analyze various soil parameters. The samples were collected at two depths of 0–15 and 15–30 cm, using a core auger (with two divisions of 0–15 and 15–30 cm, 5 cm diameter). The soil samples were air-dried, sieved through a 2 mm sieve and then used to analyze soil parameters (Page, 1982).
2.3.1 Soil characteristics
For the initial soil characteristics, soil samples (0–15 cm layer) were collected randomly in triplicate from the 24 plots of the experimental farm before the start of the maize cropping season in 2018. The soil samples were analyzed for soil organic carbon (Walkley and Black, 1934), available soil nitrogen (Subbaiah and Asija, 1956), available phosphorous (Olsen et al., 1954), and available potassium (Prasad, 1998). The soil pH was 7.8, and the electrical conductivity was 0.42 dS/m, the soil organic carbon (SOC) was 4.69 g/kg, the available nitrogen was 162.8 kg/ha, the available phosphorus was 15.2 kg/ha and the available potassium was 152.2 kg/ha.
2.3.2 Soil analysis
Soil organic carbon and bulk density were estimated using the standard procedures (Walkley and Black, 1934). Soil samples were taken from a depth of 0–15 and 15–30 cm. Soil organic carbon was determined by the chromic acid wet oxidation. For bulk density, undisturbed soil samples were taken from a depth of 0 to 30 cm at a distance of 15 cm with the core sampler. Fresh soil core samples were processed in the laboratory and oven-dried for 48 h at 105°C. Soil bulk density was calculated by dividing the core volume by the dry weight of the soil (Blake and Hartge, 1986). Soil strength (cone penetration resistance) was measured when the profile moisture content was close to field capacity, using a hand-held recording penetrometer (RIMIK CP40II, Australia) fitted with a 12.8 mm diameter cone with an area of 130 mm2 with a maximum cone index of 5,600 kPa (Parihar et al., 2020), which recorded the PR at intervals of 10 mm up to a soil depth of 700 mm. Resistance data from three equal positions (between rows) for each treatment per plot were averaged for each depth, and the mean was expressed in kilopascals (kPa) (Anderson et al., 1980). SOC, BD, and PR are measured and analysed at 25 DAS to determine soil properties’ status during the active crop growth stage after retaining previous crop residues. Soil moisture was measured using a Delta moisture meter ranging from 16.83 to 34.06% (Delta-T Devices Ltd, 2006).
2.4 Maize, wheat, mungbean grain yield and system productivity (maize equivalent yield)
Crops were harvested after the physiological maturity stage, and grain yield was measured by calculating the weight of harvested economic part produced from each plant in the grown area using the conversion factor, which is presented as tonnes per hectare. System productivity of the cropping systems was calculated based on the crop yields and minimum support price of maize and wheat by using the following equation as mentioned in Parihar et al. (2016a):
2.5 Statistical analysis
The data were analyzed using the Microsoft Excel ver. 2021 and Indian NARS Statistical Computing Portal2 developed by ICAR – Indian Agricultural Statistical Research Institute, New Delhi, India, based on SAS ver. 9.2 (SAS Institute Inc., 2002) for a split-plot design (Rangaswamy, 2018). The data on SOC, bulk density, penetration resistance, maize, wheat, mungbean yield and system productivity were subjected to the Barlett test for homogeneity of variance. The error variances for almost all parameters (i.e., SOC, bulk density, penetration resistance, maize, wheat, mungbean yield and system productivity) were homogeneous over the years. Hence, pooled analysis was done to find out the effects of the year (Y) and interactions between Y × crop residue management (CRM), Y × precision nitrogen management (PNM), CRM × PNM and Y × CRM × PNM on the studied variables of soil physico-chemical properties and crops productivity. The significance of treatment means was appraised using least significance difference test at p ≤ 0.05. Pearson’s correlation analysis was done using the R studio ver. 2021.09.4 (R Core Team, 2013).
The multivariate statistical technique was used to perform principal component analysis (PCA) by PAST (Andrews et al., 2002). First, the principal components (PCs) with the greatest Eigenvalues were selected. Consequently, PCs with high Eigenvalues were deemed to be best principal components (Kaiser, 1960). Next, the score was determined using the variation (percent) of each principal component investigated. Then, components were selected and taken for further analysis based on the Eigenvalues and the percentage of variance. In addition to Eigenvalues, a scree plot was used to sort out the principal components. Finally, Biplot analysis was performed utilizing the components, Eigenvalues, and loadings.
3 Results and discussion
3.1 Soil organic carbon
Pooled analysis of variance (ANOVA) of soil organic carbon (SOC), bulk density (BD), penetration resistance (PR), maize grain yield, wheat grain yield, mungbean grain yield and system productivity (MEY) showing the effects of years, crop residue management (CRM), precision nitrogen management (PNM) and their interactions are shown in the Table 3. From the analysis, the study found that the combined effect between main plot (CRM), subplot (PNM) and years (Y) are mostly non-significant and only in SOC it is statistically significant. Soil organic carbon (SOC) in the topsoil layer (0–15 cm) of zero tillage without residue retention (ZT-R) plots increased from 5.10 g/kg to 5.20 g/kg between 2018 and 2020. Meanwhile, in the zero tillage with residue retention (ZT + R) treatment, the SOC increased from 5.33 g/kg to 5.73 g/kg between 2018 and 2020 (Table 4). The ZT + R increased SOC from 4.69 to 5.73 g/kg, amounting to a 22% increase in SOC. Long-term studies on conservation agriculture in South Asia have reported that system-based conservation tillage improves resource-use efficiency, conserves biological and natural resources, and improves available soil nutrients (Jayaraman et al., 2020). A study conducted on a corn-soybean-wheat-cowpea cropping system observed that no-tillage practices enhanced the total soil C by 30% and active carbon by 10% (Aziz et al., 2015). While intensive soil tillage increases the oxidation and decomposition of organic matter (SOM) and decreases the soil C content (Dinesh et al., 2022b; Sinduja et al., 2022), conservation agricultural techniques increase C content in the soil (Kaiser et al., 2014). However, the increase in soil carbon content is influenced by crop rotations, the amount and chemical composition of leftover crop biomass/residues, and root exudates (Congreves et al., 2015). Minimum tillage practices have been observed to improve the soil structure and other physical properties due to the maintenance of soil aggregates and reduced oxidation of SOM (Srinivasarao et al., 2021). Conservation tillage ensures minimum soil disturbance, and the plant roots in the root zone (up to 30 cm) slowly decompose to increase organic C content. Nevertheless, the soil C content is less influenced in the deeper layers (30–45 cm) due to the absence of root biomass (Parihar et al., 2016b; Dinesh et al., 2022a,b).
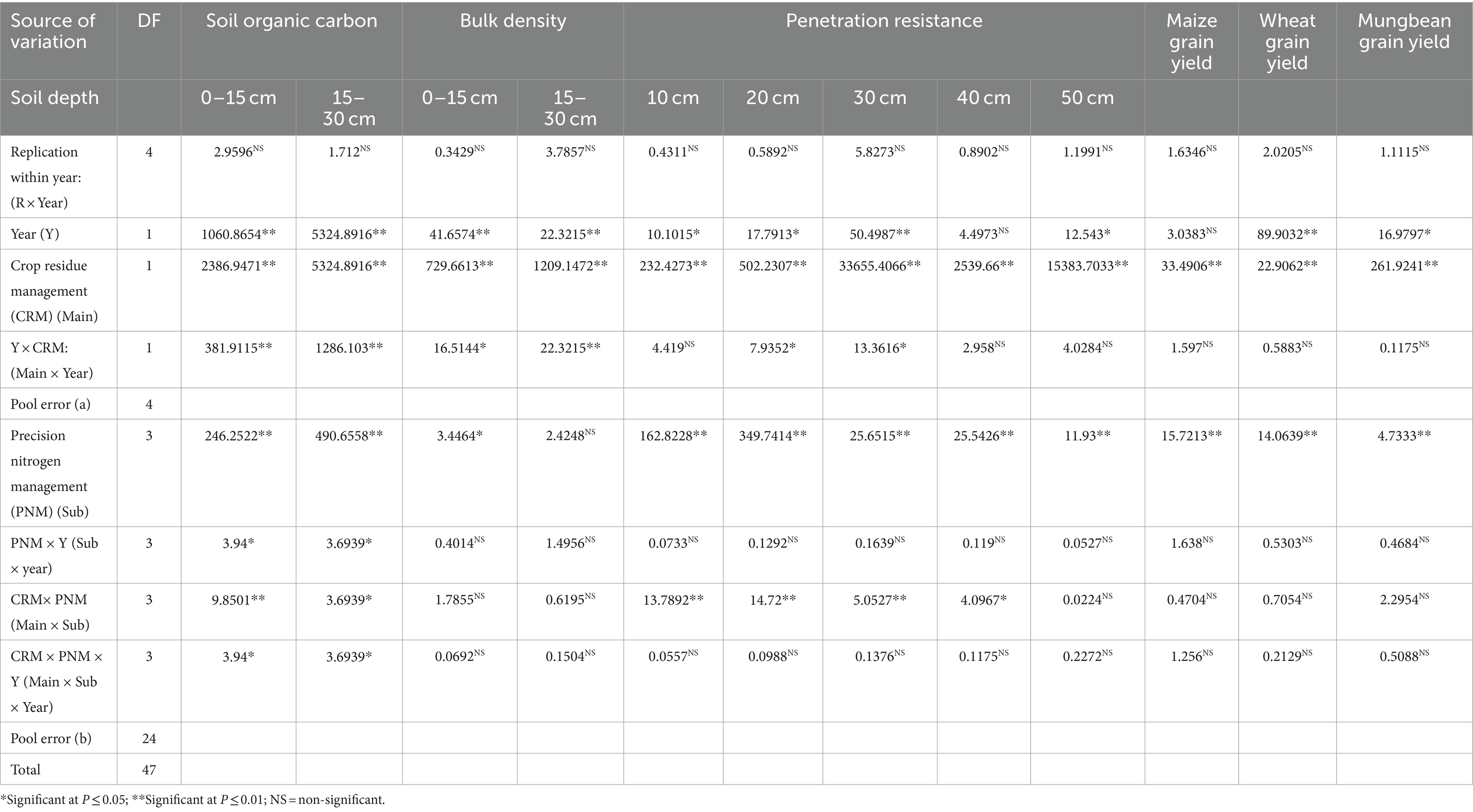
Table 3. Pooled analysis of variance (ANOVA) of soil organic carbon (SOC), bulk density (BD), penetration resistance (PR), maize grain yield, wheat grain yield, mungbean grain yield and system productivity (MEY) showing the effects of years, crop residue management (CRM), precision nitrogen management (PNM) and their interactions.
In the sub-surface soil layer (15–30 cm), the SOC in ZT-R plots increased from 3.52 g/kg to 4.23 g/kg of soil during the study period. In the ZT + R treatment plots, the SOC increased from 4.23 g/kg to 4.46 g/kg of soil (Table 4). The increase in the SOC in the sub-surface soil layer is due to the root exudates and decomposition of root residue biomass. Studies have reported that the root residues and dissolved organic carbon transport drive the sub-surface soil C dynamics, and the crops with the deeper root system improve SOC in the deeper layers of the soil (Juma et al., 2019). A similar study reported that wheat straw incorporation and decomposition contribute to a higher SOC content in the surface soil layer than in other deeper soil layers (Liu et al., 2020). The pooled mean analysis during the study period 2018–20 in both the main plot treatments was statistically significant, with the critical difference ranging from 0.01 to 0.09. The crop residue effect was significant, possibly caused by residue-based soil fertility enhancement and increased moisture availability. Hence, residue retention is an important and integral practice of zero tillage systems. The effects of residue retention (residue removal treatments of 0, 25, 50, 75, and 100%) under long-term no-till continuous corn on SOC sequestration. They found a higher SOC with a greater residue retention rate in silt loam soils (Blanco-Canqui and Lal, 2007). The magnitude of the residue impacts depends on the soil type and topographic factors. Another study observed that the rise in SOC was attributed to crop residue retention on the soil surface, where microorganisms break down the residues and store higher C. Legume residues with a low C: N ratio increased the SOC due to the quick degradability of residues. At deeper soil layers, leguminous taproot residues increased the SOC content (Yadav et al., 2017). Thierfelder et al. (2012) discovered that adding cowpea and sun hemp to corn-based crop rotations increased soil carbon by 31%. Saha and Ghosh (2013) have found that applying organic manures to grain-growing systems positively influenced SOC content. The study conducted in China reported that, following the addition of wheat straw, the NH4+ concentration increased by 40 to 80% due to the quick mineralization of the straw and soil organic matter (SOM).
In the precision N management treatments, in the topsoil layer (0–15 cm), the highest SOC was observed in 50%BN + GS treatment with 5.40 g/kg of soil, and in the second year (2019), the highest SOC was observed in 50%BN + GS with 5.65 g/kg of soil. This increase in the SOC is due to the precision application of nitrogen based on the requirements. A study conducted in North China plains reported that the precision application of nitrogenous fertilizers increased SOC and SOM (Li et al., 2019).
In the sub-surface soil layer (15–30 cm) in 2018, the highest SOC was observed in 50%BN + GS with 4.05 g/kg, and in 2019, the highest SOC was observed in 50%BN + GS with 4.54 g/kg. The increase in the SOC at subsurface soil layers may be attributed to the proper and required quantity of fertilizer application through green seeker-based precision techniques. A global meta-analysis to quantify the relationship between SOM and crop yields revealed that the yields of maize and wheat crops are greater with higher SOC. Increasing the SOC levels will potentially reduce reliance on N fertilizers and also reduce the global yield gaps (Oldfield et al., 2019).
SOC levels in the subplot treatments showed an observable trend. SOC was highest at 50%BN + GS, followed by 33%BN + GS, RDN, and 70%BN + GS. However, in some cases, especially in the rooting zone soil layer (15–30 cm), RDN and 33%BN + GS values were closely related and significantly at par. Pooled analysis between subplot treatments revealed a significant difference between the two study years. Furthermore, statistical analysis between the subplot treatments also revealed a significant difference, with the critical difference values ranging from 0.03 to 0.09. Interaction analysis between crop residue and N management was non-significant in the 2018–19 topsoil layer (0-15 cm) and the 2018–19 and 2019–20 bottom (15-30 cm) soil layers. A significant difference was observed only in the 2019 topsoil layer. Pooled analysis in both study years also revealed a significant difference.
3.2 Bulk density
In the topsoil layer (0–15 cm), the BD was 1.32 Mg/m3 in the ZT + R plots in 2018, and it was further reduced to 1.28 Mg/m3 in 2019 (Table 4). The very meager reduction in the BD in ZT-R plots could be attributed to the soil’s decomposition of crop roots (belowground biomass; Labelle and Kammermeier, 2019). In the ZT-R plots, the bulk density was 1.40 Mg/m3 in 2018 and slightly reduced to 1.39 Mg/m3 in 2019. In the bottom soil layer (15–30 cm), the bulk density was 1.50 Mg/m3 in both years of ZT-R plots and ZT + R plots, having 1.36 Mg/m3 in 2018 and 1.32 in 2019. There was a significant difference in BD between ZT + R and ZT-R main plot treatments in both years at 0–15 cm and 15–30 cm soil layer (p = 0.02), and pooled analysis also showed a significant difference (p ≤ 0.05) in both years and both soil layers. There was a slight variation between N management subplot treatments in both study years. However, there was a notable year-to-year variation between the subplot treatments. In a study conducted by (Butterly et al., 2013), it was observed that forest litter amendment and soil compaction influenced the microbial biomass carbon, nitrogen, and SOC. Soil compaction reduced the soil aeration due to a 13–36% reduction in porosity. Soil compaction changes soil structure and reduces water infiltration and root penetration into the soil. Proper N management reduced the soil bulk density due to higher root biomass production (Nawaz et al., 2013). Chalise et al. (2019) in their study on the effect of cover crops and residue retention, found that residue returned (residue retained) and cover crops are beneficial for improving the physical environment of soil and reduced bulk density compared to residue not returned (Chalise et al., 2019).
The lowest BD (1.32 Mg/m3) was observed in the topsoil layer (0–15 cm), in 70%N + GS subplot treatment, and the highest was observed in RDN (1.36 Mg/m3), 33%BN + GS and 50%BN + GS. On the other hand, in the bottom soil layer (15–30 cm), the lowest bulk density (1.40 Mg/m3) was observed in 33%BN + GS, and the highest was observed in RDN (1.44 Mg/m3). A statistically significant difference (p = 0.01) existed between the subplot treatments. Furthermore, pooled analysis between the subplot treatments was also statistically significant (p = 0.04). The interaction effects between the residue and nutrient management were statistically non-significant in both study years. However, pooled analysis reveals that there was a significant difference between them.
Compared to ZT + R plots, the ZT-R plots had 8% more BD, possibly due to residue retention in the field (Parihar et al., 2016b). Similar results have been reported by Chalise et al. (2019) from the three-year-old experiment (2014, 2015, and 2016), which showed that residue retained plots (1.30 Mg/m3) had a 7% lower bulk density (BD) compared to the residue removed plots (1.40 Mg/m3) (Chalise et al., 2019). The highest variation was seen in the 50 cm soil layer. Hence, the residue retention significantly affects the ZT-R and ZT + R plots, especially in the 50 cm soil layer. In the long run, the BD of the conventional tilled soil may increase, which might cause restriction to root penetration to crops and create much more complex, ultimately resulting in the reduction of crop yields (Orzech et al., 2021). Furthermore, the increase in the BD also caused a drastic reduction in crop growth (Parlak and Parlak, 2011). But in this current zero tillage with residue retention study, the bulk density will decrease over the long run. Higher SOC content, improved aggregation, enhanced root development, and increased biomass might decrease BD under CA (Unger and Jones, 1998). Some studies reported similar findings of lower BD values under ZT (Salem et al., 2015). However, in clay/silty loam soils, several researchers observed greater BD values under ZT (Wilkins et al., 2002). Crop rotation impacts do not substantially influence oil BD (Unger and Jones, 1998). Long-term investigations by Parihar et al. (2016b) and Parihar et al. (2016a) reported that BD of sandy loam soil (Inceptisols; Typic Haplustept) increases with depth but reduces with crop residues retained and no-tillage practices. Research in Minnesota and Iowa found that high residue removal rates resulted in a 7% greater BD than no residue harvest (Tormena et al., 2017). Chalise et al. (2019) reported that reduced BD at both soil depths was attributable to root contributions. Residue retention was found to lower BD by 7 and 3.7% for depths of 0–5 and 5–15 cm, respectively, compared to residue non-retained treatments.
3.3 Soil penetration resistance
Soil Penetration Resistance (PR) in the topsoil layer (10 cm) of ZT-R plots was 463.4 kPa in 2018 and 461.7 kPa in 2019 (Table 4). Meanwhile, in the ZT + R plots, the PR was 442.4 kPa in 2018 and 433.9 kPa in 2019, showing that the residue retention had reduced the PR. Similar trends were observed at 20 cm, 30 cm, 40 cm, and 50 cm soil depth levels. In a study conducted by Acuña and Villamil (2014) in Illinois, similar results were observed. In another study by Chalise et al., residue retained with crop cover increased the SOC and lowered the PR (Chalise et al., 2019). In the deeper soil depths, the variation in PR between ZT-R and ZT + R plots was clear and robust, which again confirmed that the effect of residue retention positively influences the soil penetration resistance in the experimental site. Research in Ames, Iowa, found that the PR value was lowered at a depth of 5 cm, and high residue removal boosted penetration resistance by 39 percent compared to no shrub residue removal (Tormena et al., 2017). Similar findings were seen in long-term research on silt-loam soil in Ohio, where the PR value improved by 17 to 24 percent when more than half of the residues were removed, compared to no residue removal (Blanco-Canqui and Lal, 2007). The difference in soil penetration resistance between the ZT-R and ZT + R plots ranged between 3 to 18%. The statistical analysis showed a significant difference between ZT + R and ZT-R plots. In addition, the pooled analysis also revealed a significant difference between the two study years.
The subplot treatments showed a significant difference between the precision N management in the top few soil layers. In the topsoil layer, the highest soil penetration resistance was observed in 70%BN + GS (476.23 kPa), and the lowest was observed in 50%BN + GS (423.84 kPa). In the 50 cm soil layer, the soil PR was lowest in the 50%BN + GS subplot treatment (1202.36 kPa), and the highest PR was observed in the 70%BN + GS subplot (1222.39 kPa). From the results, it was clear and visible that the 50%BN + GS subplot treatment had the lowest PR values in all the five soil depths, and in contrast, the 70%BN + GS subplot had the highest PR values in all the five soil depths. A residue retention study by Dolan et al. (2006) revealed that Pisum sativum plant residues reduced the penetration resistance value more than the Brassica napus or Triticum aestivum plant residues (Doan et al., 2005). In addition, using long and robust root crops in crop rotation has been shown to overcome soil compaction limitation (Liu et al., 2021). Because deeper soil layers had a greater intrinsic BD, the PR increased with depth (up to 40 cm). The compaction created by plow pan under conventional tillage methods increased soil resistance, resulting in greater PR in repeatedly tilled soil (Parihar et al., 2016b). Hence, from the perspective of soil penetration resistance, 50%BN + GS was the best treatment among the four subplot treatments, followed by RDN or 33%BN + GS subplot treatments. However, 70%BN + GS is the least-performing treatment among all the soil depths. The variation among the subplot treatments was reduced in the deeper soil depths, which implied the effect of residue retention in the topsoil depths till up to 30 cm was enormously prominent. If the residue were incorporated into deeper soil depths using any minor manually hand-operated drilling, the PR might also decrease in deeper soil depths (Parihar et al., 2016b).
Interaction analysis revealed a significant difference between year-residue management and year-precision N management. There was a significant difference between year, residue management, and precision N management in the three-way interaction analysis but not in the pooled analysis between them. In other studies, CA methods reduced soil PR (Saha et al., 2010). A higher BD could be another reason for an increased PR in conventional tillage techniques. Parihar et al. (2016b) reported that BD could account for 84% of the variation in PR; hence, PR and BD had a strong relationship in soil. Sharma and De Datta (1986) also found a significant positive relationship between PR and BD.
3.4 Crop yields and system productivity
Table 5 presents the effect of residue and precision nitrogen management on maize, wheat, and mungbean crop yields. The yield of kharif sown maize (5.73 t/ha), rabi sown wheat (5.80 t/ha), and summer mungbean (1.07 t/ha) are higher in the residue retained fields rather than residue non-retained fields, which is statistically significant (p ≤ 0.05). The grain yields of maize, wheat, and mungbean were 9, 9, and 15%, respectively, higher in the residue-retained fields. Among the subplot treatments, the higher yields were observed in the 50%BN + GS treatments in maize (5.90 t/ha), 33%BN + GS in wheat (5.84 t/ha), and 70%BN + GS in mungbean (1.02 t/ha) (Table 6). However, ANOVA showed significant differences only in the case of maize and wheat but not in mungbean. ANOVA showed no significant interaction effects of crop residue management and precision nitrogen management on maize, wheat, and mungbean yield during the study period.
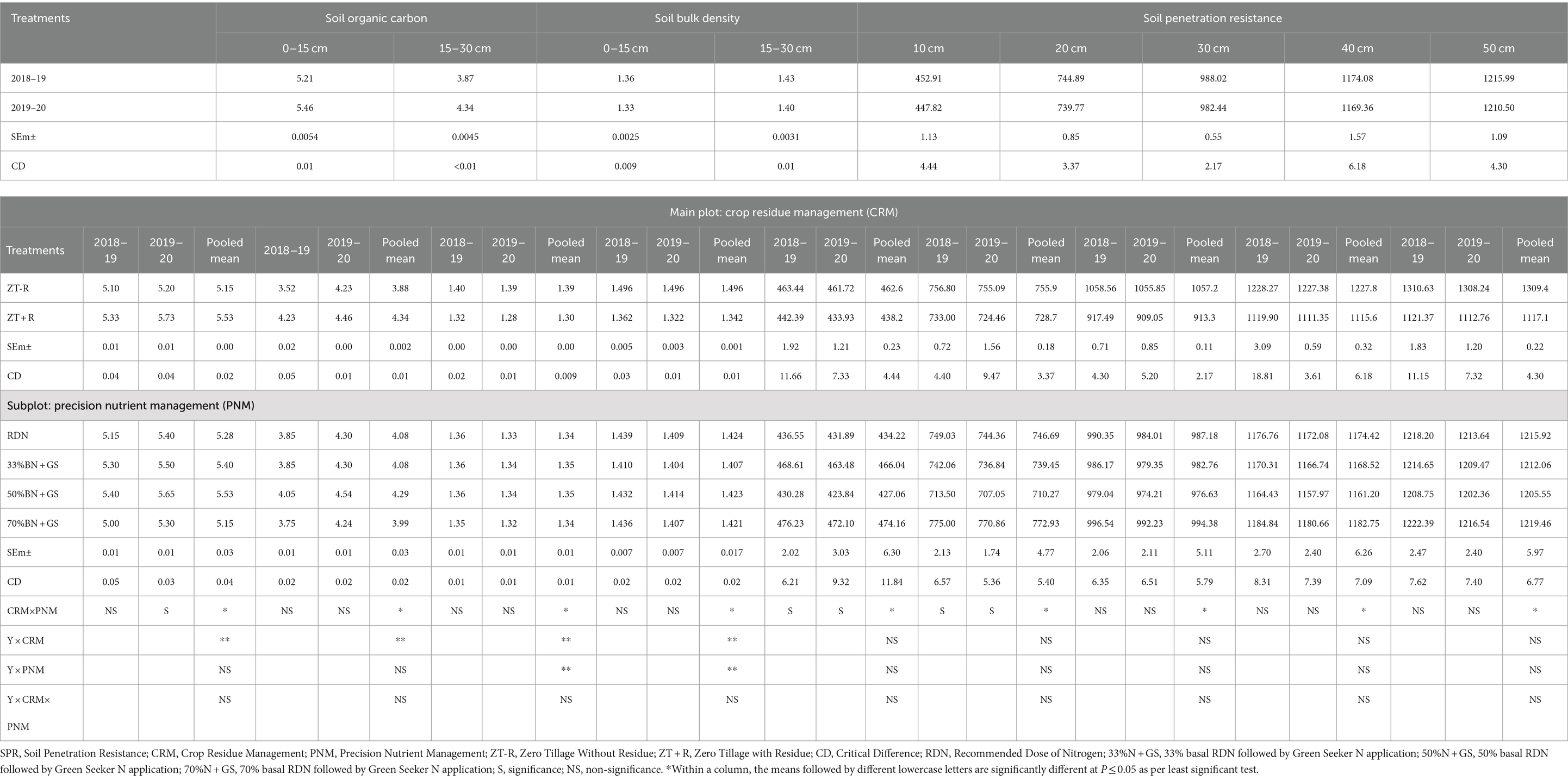
Table 4. Soil Organic Carbon (SOC), Bulk Density (BD) and Penetration Resistance (PR) across the treatments over the years.
The maize equivalent yield under the ZT + R treatment is found to be higher (6.05) in the 50%BN + GS plot, which is 15% higher than the recommended dose of nitrogen treatment (5.26) and 20% higher than the 70%BN + GS treatment (5.04) (Table 6). It signifies the importance of precision N management using green seeker. Compared to non-residue plots, residue retention plots outperformed well with 7% more system productivity; this outcome signifies the prominence and need for residue retention in conservation agriculture. The utilization of green seeker highlights the significance of precision nitrogen management. The results indicate that residue retention plots exhibit superior performance compared to non-residue plots, with a 10% increase in system productivity. This underscores the significance and necessity of crop residue retention in the context of conservation agriculture (Table 6).
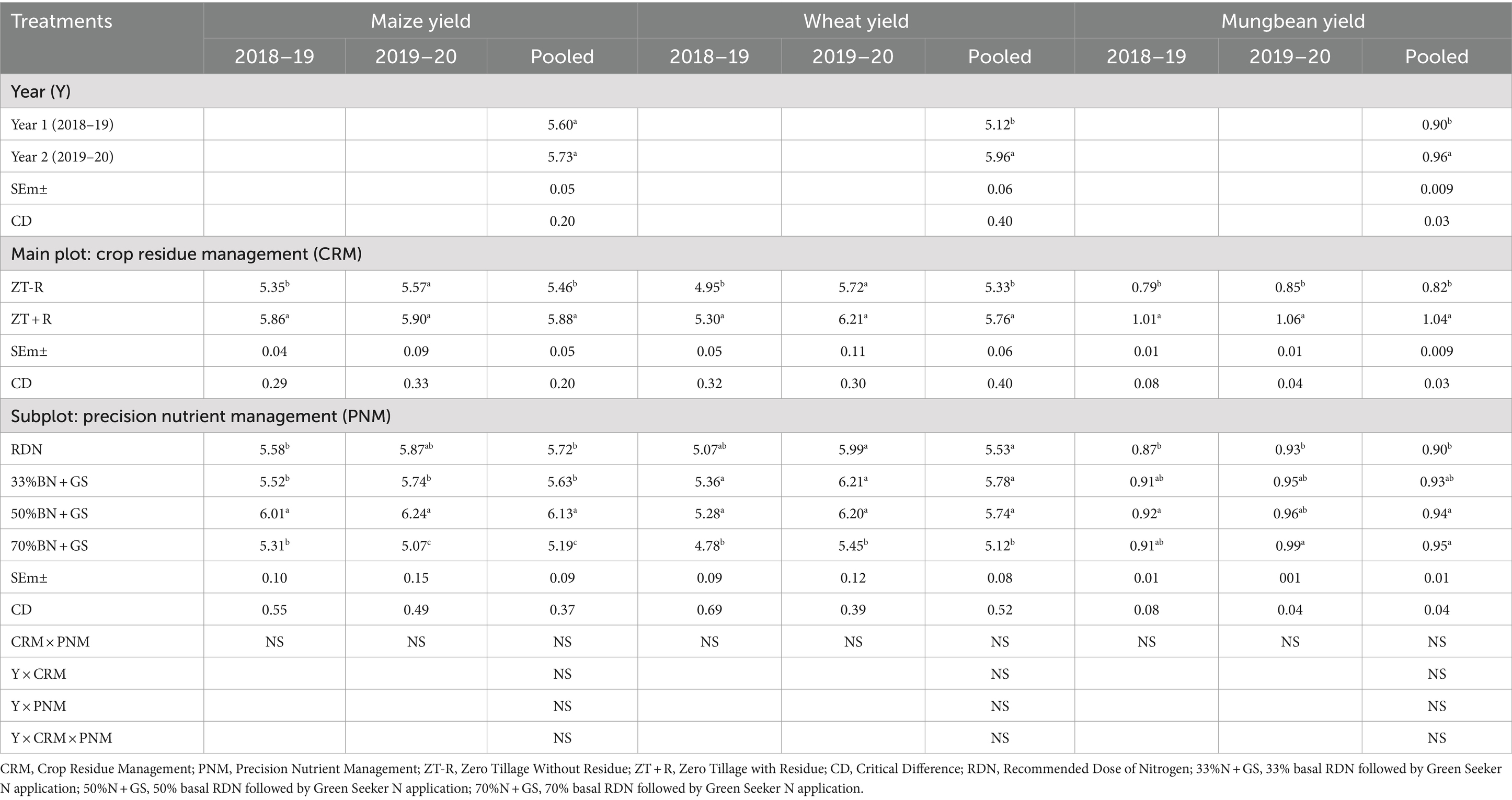
Table 5. Effect of residue and precision nitrogen management on maize, wheat, and mungbean crop yields (t/ha).
The outcomes of our study align with prior research conducted in South Asia, which demonstrated that zero tillage with residue retention resulted in greater crop yields in rice-wheat and maize-wheat systems (Parihar et al., 2017, 2018; Sapkota et al., 2020; Jat H. S. et al., 2021). The increased yield of maize, wheat, and mungbean in the zero tillage (ZT) system may be attributed to the combined effects of supplementary nutrients, reduced weed population, enhanced soil physical health, improved water regimes, and increased nutrient use efficiency (Parihar et al., 2016). The increased grain yield and grain equivalent yield (GEY) are ascribed to the legumes’ inclusion in the cropping system, as it will increase soil fertility and soil nitrogen (Lipper, 2010; Congreves et al., 2015). The ZT plots with residue retention exhibited a marked increase in grain and GEY compared to the ZT non-residue retained plots. This outcome may be ascribed to the greater spike density, number of grains per spike, and 1,000-grain weight (Parihar et al., 2016).
3.5 Pearson’s correlation and principal component analysis
From Pearson’s correlation analysis, SOC has a highly negative correlation with soil BD and PR; it agreed with the previous studies (Yu et al., 2014) and had a weak positive correlation with maize and wheat yield. However, it had a high positive correlation with mungbean yields. Hence, increased SOC might improve crop yields (Somasundaram et al., 2020; Jat R. A. et al., 2021). On the other hand, BD had a highly negative correlation with SOC and mungbean yields (Figure 3). It also had a weak negative correlation with maize and wheat yield but a very high positive correlation with PR. Previous studies also reported that increased soil BD would reduce crop yields (Liu et al., 2021; Obour et al., 2021).
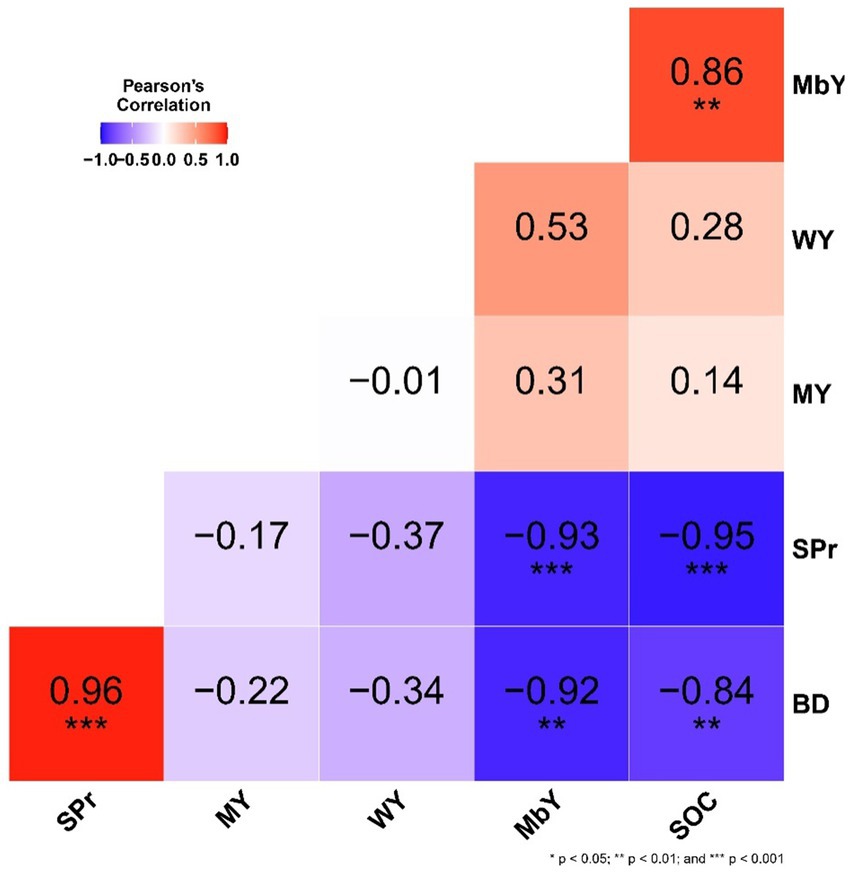
Figure 3. Relationship of various soil parameters with crop yields by Pearson’s correlation analysis.
Soil penetration resistance negatively correlated with SOC and mungbean yields. It had a weak negative correlation with maize and mungbean yields Arruda et al. (2021) also reported that increasing soil penetration resistance would reduce crop yields. SOC had a very strong level of significance with soil bulk density (p ≤ 0.01) and an extreme level of significance with soil penetration resistance (p ≤ 0.001). Mungbean yields had a very strong level of significance with soil bulk density (p ≤ 0.01) and an extreme level of significance with soil penetration resistance (p ≤ 0.001). Soil penetration resistance was extremely significant with soil bulk density (p ≤ 0.001). Soil organic carbon has a very strong significance level with mungbean yields (p ≤ 0.01).
The PCA analysis clustered all observations into six Principal Components (PC). PC 1 and PC 2 contributed 83.08% of the percentage variance, and PC 1 and PC2 had an Eigenvalue of 3.97 and 1.01 respectively, which was considered the best components. The scree plot also revealed that over 80% of the Eigenvalues were observed in PC 1 and PC 2. Hence, PC 1 and 2 were taken for further biplot analysis. The Biplot analysis between PC 1 and PC 2 revealed that ZT + R 33%BN + GS are observed with negative dispersions, and ZT + R 50%BN + GS, ZT + R 70%BN + GS, and ZT + R RDN are observed with positive dispersions (Figure 4). Soil organic carbon, Maize and mungbean yields are toward positive dispersions, PR, and BD toward negative dispersions. Hence, it may be concluded that from the PCA analysis, crop yields increased when PR and BD decreased. Previous research also revealed increasing crop yields and plant and root growth when BD and soil PR decreased (Colombi et al., 2018; Getahun et al., 2018).
4 Conclusion
The two-year study in a six-year-old long-term conservation agriculture experiment demonstrated that maize-based crop rotations with ZT-based systems coupled with precise nitrogen management/crop residue retention not only improved the soil organic carbon (SOC) but also significantly impact physical attributes of sandy loam soil. In comparison to non-residue retained plots, we found a decreased bulk density (by 6 to 8%), soil penetration resistance (by 5 to 18%), and an increased SOC (by 4 to 20%) in residue retained plots, which are statistically significant. Among the precision nitrogen management practices, 50% basal nitrogen, followed by green seeker, proved to be the most promising treatment in improving SOC and physical properties. The maize equivalent yield under the ZT + R treatment was found to be higher (6.05 t/ha) in the 50%BN + GS plot, which is 15% higher than the recommended dose of nitrogen treatment (5.26 t/ha) and 20% higher than the 70%BN + GS treatment (5.04 t/ha). Compared to non-residue retention plots, residue retention plots outperformed well, with 7% more system productivity. Study findings indicated that long–term ZT coupled with residue retention and precision N management in maize-wheat-mungbean rotations could enhance soil health and resilience, which ultimately results in sustainable crop production in sandy loam soil (Typic Haplustept; Inceptisols) of the north-west Indo-Gangetic plains. Farmers and policymakers can consider implementing these sustainable practices to enhance soil health, increase crop yields, and ultimately improve food security. Further research could explore the long-term effects of these practices and their potential to reduce greenhouse gas emissions and mitigate the negative impacts of climate change on agriculture. Additionally, precision nitrogen management practices could be further optimized to achieve even better soil health and system productivity.
Data availability statement
The original contributions presented in the study are included in the article/supplementary material, further inquiries can be directed to the corresponding authors.
Author contributions
GKD: Conceptualization, Data curation, Formal analysis, Investigation, Methodology, Software, Validation, Visualization, Funding acquisition, Writing – original draft. DKS: Conceptualization, Supervision, Validation, Writing – review & editing. SLJ: Conceptualization, Supervision, Validation, Writing – review & editing. VV: Writing – review & editing, Methodology, Validation. KB: Writing – review & editing, Methodology, Validation. PVK: Data curation, Formal analysis, Writing – review & editing. SP: Writing- review & editing, Methodology, Validation. AA: Writing – review & editing. SS: Writing – review & editing. SR: Writing – review & editing. TR: Writing – review & editing. KKY: Writing – review & editing, Methodology, Validation. SJ: Writing – review & editing, Methodology, Validation. KRR: Writing – review & editing. MS: Writing – review & editing. VS: Writing – review & editing. CSR: Writing – review & editing. RD: Writing – review & editing. SMM: Writing – review & editing. SK: Writing – review & editing. AKS: Writing – review & editing. BK: Writing – review & editing. DMM: Writing – review & editing.
Funding
The author(s) declare financial support was received for the research of this article. The authors would like to thank ICAR – Indian Agricultural Research Institute, New Delhi, for providing the student research fellowship to the author GKD. The funding received from ICAR-National Agricultural Science Fund, New Delhi (F. No. NASF/CA-7022/2018-19) to carry out the study is duly acknowledged.
Acknowledgments
We sincerely thank the Division of Environment Science, ICAR-IARI, Director, ICAR-IARI, and ICAR-IIMR, New Delhi, for providing field and laboratory facilities. Special thanks to the University Grants Commission, Government of India, for supporting this work in the form of a Junior Research Fellowship.
Conflict of interest
The authors declare that the research was conducted in the absence of any commercial or financial relationships that could be construed as a potential conflict of interest.
The reviewer PG declared a shared affiliation with the authors KB, SS, SR, TR, and KRR to the handling editor at the time of review.
Publisher’s note
All claims expressed in this article are solely those of the authors and do not necessarily represent those of their affiliated organizations, or those of the publisher, the editors and the reviewers. Any product that may be evaluated in this article, or claim that may be made by its manufacturer, is not guaranteed or endorsed by the publisher.
Footnotes
References
Acuña, J. C. M., and Villamil, M. B. (2014). Short-term effects of cover crops and compaction on soil properties and soybean production in Illinois. Agron. J. 106, 860–870. doi: 10.2134/agronj13.0370
Anderson, G., Pidgeon, J. D., Spencer, H. B., and Parks, R. (1980). A new hand-held recording penetrometer for soil studies. J. Soil Sci. 31, 279–296. doi: 10.1111/j.1365-2389.1980.tb02081.x
Andrews, S. S., Karlen, D. L., and Mitchell, J. P. (2002). A comparison of soil quality indexing methods for vegetable production systems in northern California. Agric. Ecosyst. Environ. 90, 25–45. doi: 10.1016/S0167-8809(01)00174-8
Arruda, A. B., de Souza, R. F., Brito, G. H. M., de Moura, J. B., de Oliveira, M. H. R., Dos Santos, J. M., et al. (2021). Resistance of soil to penetration as a parameter indicator of subsolation in crop areas of sugar cane. Sci. Rep. 11, 1–9. doi: 10.1038/s41598-021-91186-3
Aziz, I., Bangash, N., Mahmood, T., and Islam, K. R. (2015). Impact of no-till and conventional tillage practices on soil chemical properties. Pak. J. Bot. 47, 297–303. doi: 10.1016/j.iswcr.2020.02.002
Basche, A. D., Kaspar, T. C., Archontoulis, S. V., Jaynes, D. B., Sauer, T. J., Parkin, T. B., et al. (2016). Soil water improvements with the long-term use of a winter rye cover crop. Agric. Water Manag. 172, 40–50. doi: 10.1016/j.agwat.2016.04.006
Blake, G. R., and Hartge, K. H. (1986). “Bulk density” in Methods of soil analysis. ed. G. R. Blake (United States: SSSA Book Series), 363–375.
Blanco-Canqui, H., and Lal, R. (2007). Soil and crop response to harvesting corn residues for biofuel production. Geoderma 141, 355–362. doi: 10.1016/j.geoderma.2007.06.012
Blanco-Canqui, H., and Ruis, S. J. (2018). No-tillage and soil physical environment. Geoderma 326, 164–200. doi: 10.1016/j.geoderma.2018.03.011
Butterly, C. R., Baldock, J. A., and Tang, C. (2013). The contribution of crop residues to changes in soil pH under field conditions. Plant Soil 366, 185–198. doi: 10.1007/s11104-012-1422-1
Chalise, K. S., Singh, S., Wegner, B. R., Kumar, S., Pérez-Gutiérrez, J. D., Osborne, S. L., et al. (2019). Cover crops and returning residue impact on soil organic carbon, bulk density, penetration resistance, water retention, infiltration, and soybean yield. Agron. J. 111, 99–108. doi: 10.2134/agronj2018.03.0213
Colombi, T., Torres, L. C., Walter, A., and Keller, T. (2018). Feedbacks between soil penetration resistance, root architecture and water uptake limit water accessibility and crop growth – a vicious circle. Sci. Total Environ. 626, 1026–1035. doi: 10.1016/j.scitotenv.2018.01.129
Congreves, K. A., Hayes, A., Verhallen, E. A., and Van Eerd, L. L. (2015). Long-term impact of tillage and crop rotation on soil health at four temperate agroecosystems. Soil Tillage Res. 152, 17–28. doi: 10.1016/j.still.2015.03.012
Delta-T Devices Ltd (2006). User manual for the SM200 soil moisture sensor. SM200-UM-1st Edn. United Kingdom: Delta-T Devices Ltda Cambridge.
Dinesh, G. K., Sharma, D. K., Jat, S. L., Bandyopadhyay, K., Srinivasa Rao, C., Venkatramanan, V., et al. (2023). Effect of conservation agriculture practices on carbon pools in a Sandy loam soil of indo-Gangetic Plains. Commun. Soil Sci. Plant Anal. 54, 2845–2862. doi: 10.1080/00103624.2023.2241513
Dinesh, G. K., Sharma, D. K., Jat, S. L., Sri, K. S. K., Bandyopadhyay, K. K., Bhatia, A., et al. (2022a). Ecological relationship of earthworms with soil physicochemical properties and crop yields in conservation agriculture. Indian J. Ecol. 49, 2135–2139. doi: 10.55362/IJE/2022/3798
Dinesh, G. K., Sharma, D. K., Kadam, P. V., Bhatia, A., Bandhopadhyay, K. K., Kumar, P., et al. (2021). “Valuation of carbon stock and its ecosystem services from conservation agriculture” in First NABS-international conference on “life sciences: Contemporary approaches in biological science for food, health, nutrition security and conservation of biodiversity”. eds. V. A. Parthasarathy, T. Marimuthu, S. Nakkeeran, M. Prakash, S. Arivudainambi, and S. Rameshkumar (Chidambaram, Tamil Nadu: Faculty of Agriculture, Annamalai University and National Academy of Biological), 111–112.
Dinesh, G. K., Sinduja, M., Priyanka, B., Sathya, V., Karthika, S., Meena, R. S., et al. (2022b). “Enhancing soil organic carbon sequestration in agriculture: plans and policies” in Plans and policies for soil organic carbon Management in Agriculture. eds. R. S. Meena, C. S. Rao, and A. Kumar (Singapore: Springer Nature Singapore), 95–121. doi: 10.1007/978-981-19-6179-3_4
Doan, V., Chen, Y., and Irvine, B. (2005). Effect of residue type on the performance of no-till seeder openers. Can. Biosyst. Eng. 47, 29–35.
Dolan, M. S., Clapp, C. E., Allmaras, R. R., Baker, J. M., and Molina, J. A. E. (2006). Soil organic carbon and nitrogen in a Minnesota soil as related to tillage, residue and nitrogen management. Soil Tillage Res. 89, 221–231. doi: 10.1016/j.still.2005.07.015
Dougill, A. J., Whitfield, S., Stringer, L. C., Vincent, K., Wood, B. T., Chinseu, E. L., et al. (2017). Mainstreaming conservation agriculture in Malawi: knowledge gaps and institutional barriers. J. Environ. Manag. 195, 25–34. doi: 10.1016/j.jenvman.2016.09.076
Eze, S., Dougill, A. J., Banwart, S. A., Hermans, T. D. G., Ligowe, I. S., and Thierfelder, C. (2020). Impacts of conservation agriculture on soil structure and hydraulic properties of Malawian agricultural systems. Soil Tillage Res. 201:104639. doi: 10.1016/j.still.2020.104639
Getahun, G. T., Kätterer, T., Munkholm, L. J., Parvage, M. M., Keller, T., Rychel, K., et al. (2018). Short-term effects of loosening and incorporation of straw slurry into the upper subsoil on soil physical properties and crop yield. Soil Tillage Res. 184, 62–67. doi: 10.1016/j.still.2018.06.007
IARI (2012). Crop residues management with conservation agriculture: potential, constraints and policy needs. 1st Edn. New Delhi, India: Indian Agricultural Research Institute.
Indoria, A. K., Rao, C. S., Sharma, K. L., and Reddy, K. S. (2017). Conservation agriculture–a panacea to improve soil physical health. Curr. Sci. 112, 52–61. doi: 10.18520/cs/v112/i01/52-61
Jat, H. S., Datta, A., Choudhary, M., Sharma, P. C., and Jat, M. L. (2021). Conservation agriculture: factors and drivers of adoption and scalable innovative practices in indo-Gangetic plains of India– a review. Int. J. Agric. Sustain. 19, 40–55. doi: 10.1080/14735903.2020.1817655
Jat, R. A., Reddy, K. K., Choudhary, R. R., Rawal, S., Thumber, B., Misal, N., et al. (2021). Effect of conservation agriculture practices on soil quality, productivity, and profitability of peanut-based system of Saurashtra, India. Agron. J. 113, 2102–2117. doi: 10.1002/agj2.20534
Jayaraman, S., and Dalal, R. C. (2022). No-till farming: prospects, challenges–productivity, soil health, and ecosystem services. Soil Res. 60, 435–441. doi: 10.1071/SR22119
Jayaraman, S., Salikram, M., Sinha, N. K., Mohanty, M., Chaudhary, R. S., Dalal, R. C., et al. (2019). Corrigendum to: conservation agriculture effects on soil properties and crop productivity in a semiarid region of India. Soil Res. 57:200. doi: 10.1071/SR18145_CO
Jayaraman, S., Sinha, N. K., Dalal, R. C., Lal, R., Mohanty, M., Naorem, A. K., et al. (2020). No-till farming and conservation agriculture in South Asia–issues, challenges, prospects and benefits. Crit. Rev. Plant. Sci. 39, 236–279.
Juma, N. G., Izaurralde, R. C., Robertson, J. A., and McGill, W. B. (2019). Crop yield and soil organic matter trends over 60 years in a Typic Cryoboralf at Breton, Alberta. In: Soil organic matter in temperate agroecosystems. Boca Raton, FL: CRC Press, pp. 273–282.
Kadam, P. V., Jat, S., Parihar, C., Singh, A., Mahala, D., Kumar, A., et al. (2022). Effect of residue and nitrogen management in maize (Zea mays) on mustard (Brassica juncea) productivity and profitability under conservation agriculture. Indian J. Agric. Sci. 92, 637–642. doi: 10.56093/ijas.v92i5.124789
Kaiser, H. F. (1960). The application of electronic computers to factor analysis. Educ. Psychol. Meas. 20, 141–151. doi: 10.1177/001316446002000116
Kaiser, M., Piegholdt, C., Andruschkewitsch, R., Linsler, D., Koch, H., and Ludwig, B. (2014). Impact of tillage intensity on carbon and nitrogen pools in surface and sub-surface soils of three long-term field experiments. Eur. J. Soil Sci. 65, 499–509. doi: 10.1111/ejss.12146
Labelle, E. R., and Kammermeier, M. (2019). Above- and belowground growth response of Picea abies seedlings exposed to varying levels of soil relative bulk density. Eur. J. For. Res. 138, 705–722. doi: 10.1007/s10342-019-01201-6
Lampurlanés, J., and Cantero-Martínez, C. (2003). Soil bulk density and penetration resistance under different tillage and crop management systems and their relationship with barley root growth. Agron. J. 95, 526–536. doi: 10.2134/agronj2003.5260
Li, Z., Li, D., Ma, L., Yu, Y., Zhao, B., and Zhang, J. (2019). Effects of straw management and nitrogen application rate on soil organic matter fractions and microbial properties in North China plain. J. Soils Sediments 19, 618–628. doi: 10.1007/s11368-018-2102-4
Lipper, L. (2010). Climate-smart agriculture: Policies, practice and financing for food security, adaptation and Mitigation. Rome: FAO.
Liu, Z., Cao, S., Sun, Z., Wang, H., Qu, S., Lei, N., et al. (2021). Tillage effects on soil properties and crop yield after land reclamation. Sci. Rep. 11, 4611–4612. doi: 10.1038/s41598-021-84191-z
Liu, Z., Gao, T., Tian, S., Hu, H., Li, G., and Ning, T. (2020). Soil organic carbon increment sources and crop yields under long-term conservation tillage practices in wheat-maize systems. Land Degrad. Dev. 31, 1138–1150. doi: 10.1002/ldr.3531
Mahal, N. K., Osterholz, W. R., Miguez, F. E., Poffenbarger, H. J., Sawyer, J. E., Olk, D. C., et al. (2019). Nitrogen fertilizer suppresses mineralization of soil organic matter in maize agroecosystems. Front. Ecol. Evol. 7:59. doi: 10.3389/fevo.2019.00059
Mrunalini, K., Behera, B., Jayaraman, S., Abhilash, P. C., Dubey, P. K., Swamy, G. N., et al. (2022). Nature-based solutions in soil restoration for improving agricultural productivity. Land Degrad. Dev. 33, 1269–1289. doi: 10.1002/ldr.4207
Muth, D. J. Jr., Bryden, K. M., and Nelson, R. G. (2013). Sustainable agricultural residue removal for bioenergy: a spatially comprehensive US national assessment. Appl. Energy 102, 403–417. doi: 10.1016/j.apenergy.2012.07.028
Nawaz, M. F., Bourrié, G., and Trolard, F. (2013). Soil compaction impact and modelling. A review. Agron. Sustain. Dev. 33, 291–309. doi: 10.1007/s13593-011-0071-8
Noor, H., Yan, Z., Sun, P., Zhang, L., Ding, P., Li, L., et al. (2023). Effects of nitrogen on photosynthetic productivity and yield quality of wheat (Triticum aestivum L.). Agronomy 13:1448. doi: 10.3390/agronomy13061448
Nyagumbo, I., Mkuhlani, S., Pisa, C., Kamalongo, D., Dias, D., and Mekuria, M. (2016). Maize yield effects of conservation agriculture based maize–legume cropping systems in contrasting agro-ecologies of Malawi and Mozambique. Nutr. Cycl. Agroecosyst. 105, 275–290. doi: 10.1007/s10705-015-9733-2
Obour, A. K., Holman, J. D., Simon, L. M., and Schlegel, A. J. (2021). Strategic tillage effects on crop yields, soil properties, and weeds in dryland no-tillage systems. Agronomy 11:662. doi: 10.3390/agronomy11040662
Oldfield, E. E., Bradford, M. A., and Wood, S. A. (2019). Global meta-analysis of the relationship between soil organic matter and crop yields. Soil 5, 15–32. doi: 10.5194/soil-5-15-2019
Olsen, S. R., Cole, C. V., Watnab, F. S., and Decan, L. A. (1954). Estimation of available phosphorous in soil byextra action with sodium bicarbonate. Washington, DC: US Department of Agriculture.
Orzech, K., Wanic, M., and Załuski, D. (2021). The effects of soil compaction and different tillage systems on the bulk density and moisture content of soil and the yields of winter oilseed rape and cereals. Agriculture 11:666. doi: 10.3390/agriculture11070666
Page, A. L. (1982). Methods of soil analysis: part 2 chemical and microbiological properties, 9.2.2. 2nd Edn. Wisconsin, USA: The American Society of Agronomy, Inc., Soil Science Society of America, Inc.
Page, K. L., Dang, Y. P., and Dalal, R. C. (2020). The ability of conservation agriculture to conserve soil organic carbon and the subsequent impact on soil physical, chemical, and biological properties and yield. Front. Sustain. Food Syst. 4:31. doi: 10.3389/fsufs.2020.00031
Page, K. L., Dang, Y. P., Dalal, R. C., Reeves, S., Thomas, G., Wang, W., et al. (2019). Changes in soil water storage with no-tillage and crop residue retention on a vertisol: impact on productivity and profitability over a 50 year period. Soil Tillage Res. 194:104319. doi: 10.1016/j.still.2019.104319
Parihar, C. M., Jat, S. L., Singh, A. K., Kumar, B., Rathore, N. S., Jat, M. L., et al. (2018). Energy auditing of long-term conservation agriculture based irrigated intensive maize systems in semi-arid tropics of India. Energy 142, 289–302. doi: 10.1016/j.energy.2017.10.015
Parihar, C. M., Jat, S. L., Singh, A. K., Kumar, B., Yadvinder-Singh, Y., Pradhan, S., et al. (2016). Conservation agriculture in irrigated intensive maize-based systems of North-Western India: effects on crop yields, water productivity and economic profitability. Field Crop Res. 193, 104–116. doi: 10.1016/j.fcr.2016.03.013
Parihar, C. M., Jat, S. L., Singh, A. K., Majumdar, K., Jat, M. L., Saharawat, Y. S., et al. (2017). Bio-energy, water-use efficiency and economics of maize-wheat-mungbean system under precision-conservation agriculture in semi-arid agro-ecosystem. Energy 119, 245–256. doi: 10.1016/j.energy.2016.12.068
Parihar, C. M., Singh, A. K., Jat, S. L., Dey, A., Nayak, H. S., Mandal, B. N., et al. (2020). Soil quality and carbon sequestration under conservation agriculture with balanced nutrition in intensive cereal-based system. Soil Tillage Res. 202:104653. doi: 10.1016/j.still.2020.104653
Parihar, C. M., Yadav, M. R., Jat, S. L., Singh, A. K., Kumar, B., Pradhan, S., et al. (2016b). Long term effect of conservation agriculture in maize rotations on total organic carbon, physical and biological properties of a sandy loam soil in north-western indo-Gangetic Plains. Soil Tillage Res. 161, 116–128. doi: 10.1016/j.still.2016.04.001
Parlak, M., and Parlak, A. Ö. (2011). Effect of soil compaction on root growth and nutrient uptake of forage crops. J. Food Agric. Environ 9, 275–278.
Prasad, R. (1998). A practical manual for soil fertility. New Delhi: Division of Agronomy, Indian Agricultural Research Institute, 110012.
R Core Team (2013). R: A language and environment for statistical computing. Vienna, Austria: R Core Team.
Rangaswamy, R. (2018). A text-book of agricultural statistics. Second. New Delhi, India: New Age International Publishers.
Rasheed, S., Venkatesh, P., Singh, D. R., Renjini, V. R., Jha, G. K., and Sharma, D. K. (2021). Ecosystem valuation and eco-compensation for conservation of traditional paddy ecosystems and varieties in Kerala, India. Ecosyst. Serv. 49:101272. doi: 10.1016/j.ecoser.2021.101272
Reddy, S. B., Srinivasarao, C., Rao, P. C., Lal, R., Rakesh, S., Kundu, S., et al. (2023). Greenhouse gases emissions and agronomic productivity as influenced by varying levels of N fertilizer and tank silt in degraded semiarid Alfisol of southern India. Land Degrad. Dev. 34, 943–955. doi: 10.1002/ldr.4507
Saha, S., Chakraborty, D., Sharma, A. R., Tomoar, R. K., Bhadraray, S., Sen, U., et al. (2010). Effect of tillage and residue management on soil physical properties and crop productivity in maize (Zea mays)–Indian mustard (Brassica juncea) system. Indian J. Agric. Sci. 80, 679–685.
Saha, R., and Ghosh, P. K. (2013). Soil organic carbon stock, moisture availability and crop yield as influenced by residue management and tillage practices in maize–mustard cropping system under hill agro-ecosystem. Natl. Acad. Sci. Lett. 36, 461–468. doi: 10.1007/s40009-013-0158-7
Salem, H. M., Valero, C., Muñoz, M. Á., Rodríguez, M. G., and Silva, L. L. (2015). Short-term effects of four tillage practices on soil physical properties, soil water potential, and maize yield. Geoderma 237-238, 60–70. doi: 10.1016/j.geoderma.2014.08.014
Sapkota, T. B., Singh, L. K., Yadav, A. K., Khatri-Chhetri, A., Jat, H. S., Sharma, P. C., et al. (2020). Identifying optimum rates of fertilizer nitrogen application to maximize economic return and minimize nitrous oxide emission from rice–wheat systems in the indo-Gangetic Plains of India. Arch. Agron. Soil Sci. 66, 2039–2054. doi: 10.1080/03650340.2019.1708332
Sharma, P. K., and De Datta, S. K. (1986). Physical properties and processes of puddled rice soils. Adv. Soil Sci. 1, 139–178. doi: 10.1007/978-1-4613-8660-5_3
Sharma, D. K., Tomar, S., and Chakraborty, D. (2017). Role of earthworm in improving soil structure and functioning. Curr. Sci. 113, 1064–1071. doi: 10.18520/cs/v113/i06/1064-1071
Shaver, T. (2010). Crop residue and soil physical properties. In: Proceedings of the 2010 Central Plains irrigation conference, Kearney, Nebraska, February 24–25 (Colorado State University. Libraries).
Sinduja, M., Sathya, V., Maheswari, M., Kalpana, P., Dhevagi, P., Dinesh, G. K., et al. (2022). Chemical transformation and bioavailability of chromium in the contaminated soil amended with bioamendments. Biorem. J. 27, 229–250. doi: 10.1080/10889868.2022.2049677
Singh, B., and Malhi, S. S. (2006). Response of soil physical properties to tillage and residue management on two soils in a cool temperate environment. Soil Tillage Res. 85, 143–153. doi: 10.1016/j.still.2004.12.005
Singh, V. K., Yadvinder-Singh, D., Dwivedi, B. S., Singh, S. K., Majumdar, K., Jat, M. L., et al. (2016). Soil physical properties, yield trends and economics after five years of conservation agriculture based rice-maize system in North-Western India. Soil Tillage Res. 155, 133–148. doi: 10.1016/j.still.2015.08.001
Srinivasarao, C., Singh, S. P., Kundu, S., Abrol, V., Lal, R., Abhilash, P. C., et al. (2021). Integrated nutrient management improves soil organic matter and agronomic sustainability of semiarid rainfed Inceptisols of the indo-Gangetic Plains. J. Plant Nutr. Soil Sci. 184, 562–572. doi: 10.1002/jpln.202000312
Somasundaram, J., Sinha, N. K., Dalal, R. C., Lal, R., Mohanty, M., Naorem, A. K., et al. (2020). No-Till Farming and Conservation Agriculture in South Asia – Issues, Challenges, Prospects and Benefits. Critical Reviews in Plant Sciences, 39, 236–279. doi: 10.1080/07352689.2020.1782069
Subbaiah, V. V., and Asija, G. K. (1956). A rapid procedure for utilization of available nitrogen in soil. Curr. Sci. 26, 258–260.
Sun, J., Li, W., Li, C., Chang, W., Zhang, S., Zeng, Y., et al. (2020). Effect of different rates of nitrogen fertilization on crop yield, soil properties and leaf physiological attributes in banana under subtropical regions of China. Front. Plant Sci. 11:613760. doi: 10.3389/fpls.2020.613760
Thierfelder, C., Cheesman, S., and Rusinamhodzi, L. (2012). A comparative analysis of conservation agriculture systems: benefits and challenges of rotations and intercropping in Zimbabwe. Field Crop Res. 137, 237–250. doi: 10.1016/j.fcr.2012.08.017
Tian-yang, Z., Zhi-Kang, L. I., En-Peng, L. I., Wei-lu, W., Li-min, Y., Hao, Z., et al. (2022). Optimization of nitrogen fertilization improves rice quality by affecting the structure and physicochemical properties of starch at high yield levels. J. Integr. Agric. 21, 1576–1592. doi: 10.1016/S2095-3119(21)63678-X
Tormena, C. A., Karlen, D. L., Logsdon, S., and Cherubin, M. R. (2017). Corn Stover harvest and tillage impacts on near-surface soil physical quality. Soil Tillage Res. 166, 122–130. doi: 10.1016/j.still.2016.09.015
Unger, P. W., and Jones, O. R. (1998). Long-term tillage and cropping systems affect bulk density and penetration resistance of soil cropped to dryland wheat and grain sorghum. Soil Tillage Res. 45, 39–57. doi: 10.1016/S0167-1987(97)00068-8
Venkatramanan, V., and Shah, S. (2019). Climate smart agriculture technologies for environmental management: the intersection of sustainability, resilience, wellbeing and development. Sustain. Green Technol. Environ. Manag. 1, 29–51. doi: 10.1007/978-981-13-2772-8_2
Venkatramanan, V., Shah, S., and Prasad, R. (2020). Global Climate Change: Resilient and Smart Agriculture. Singapore: Springer.
Venkatramanan, V., Shah, S., Prasad, S., Singh, A., and Prasad, R. (2021). Assessment of bioenergy generation potential of agricultural crop residues in India. Circ. Econ. Sustain. 1, 1335–1348. doi: 10.1007/s43615-021-00072-7
Walkley, A., and Black, I. A. (1934). An examination of the degtjareff method for determining soil organic matter, and a proposed modification of the chromic acid titration method. Soil Sci. 37, 29–38. doi: 10.1097/00010694-193401000-00003
Wilkins, D. E., Siemens, M. C., and Albrecht, S. L. (2002). Changes in soil physical characteristics during transition from intensive tillage to direct seeding. Trans. ASAE 45:877. doi: 10.13031/2013.9933
Yadav, M. R., Parihar, C. M., Jat, S. L., Singh, A. K., Kumar, R., Yadav, R. K., et al. (2017). Long term effect of legume intensified crop rotations and tillage practices on productivity and profitability of maize Vis-a-Vis soil fertility in North-Western indo-Gangetic Plains of India. Legume Res. Int. J. 40, 282–290. doi: 10.18805/lr.v0i0.7583
Keywords: soil bulk density, soil penetration resistance, soil organic carbon, residue retention, nitrogen management, inceptisols, system productivity, greenseeker
Citation: Dinesh GK, Sharma DK, Jat SL, Venkatramanan V, Boomiraj K, Kadam P, Prasad S, Anokhe A, Selvakumar S, Rathika S, Ramesh T, Bandyopadhyay K, Jayaraman S, Ramesh KR, Sinduja M, Sathya V, Rao CS, Dubey R, Manu SM, Karthika S, Singh AK, Kumar B and Mahala DM (2024) Residue retention and precision nitrogen management effects on soil physicochemical properties and productivity of maize-wheat-mungbean system in Indo-Gangetic Plains. Front. Sustain. Food Syst. 8:1259607. doi: 10.3389/fsufs.2024.1259607
Edited by:
Ashish Kumar Gupta, National Institute for Plant Biotechnology, Indian Council of Agricultural Research, IndiaReviewed by:
P. Sivasakthivelan, Annamalai University, IndiaThangasamy Arunachalam, Directorate of Onion and Garlic Research, India
Poovizhi Sindhu G., Tamil Nadu Agricultural University, India
Copyright © 2024 Dinesh, Sharma, Jat, Venkatramanan, Boomiraj, Kadam, Prasad, Anokhe, Selvakumar, Rathika, Ramesh, Bandyopadhyay, Jayaraman, Ramesh, Sinduja, Sathya, Rao, Dubey, Manu, Karthika, Singh, Kumar and Mahala. This is an open-access article distributed under the terms of the Creative Commons Attribution License (CC BY). The use, distribution or reproduction in other forums is permitted, provided the original author(s) and the copyright owner(s) are credited and that the original publication in this journal is cited, in accordance with accepted academic practice. No use, distribution or reproduction is permitted which does not comply with these terms.
*Correspondence: Govindaraj Kamalam Dinesh, Z2tkaW5lc2hpYXJpQGdtYWlsLmNvbQ==; Dinesh Kumar Sharma, ZGluZXNocHVzYTY5QGdtYWlsLmNvbQ==; Shankar Lal Jat, c2xpYXJpQGdtYWlsLmNvbQ==
‡Present address: Govindaraj Kamalam Dinesh, Department of Soil Science and Agricultural Chemistry, Faculty of Agricultural Sciences, SRM College of Agricultural Sciences, SRM Institute of Science and Technology, Chengalpattu, Tamil Nadu, India
†ORCID: Govindaraj Kamalam Dinesh, https://orcid.org/0000-0002-6605-9561
Shankar Lal Jat, https://orcid.org/0000-0002-3816-2318
Veluswamy Venkatramanan, https://orcid.org/0000-0003-1798-3755
Kovilpillai Boomiraj, https://orcid.org/0000-0002-8112-929X
Praveen Kadam, https://orcid.org/0000-0002-5249-9769
Shiv Prasad, https://orcid.org/0000-0003-4741-2603
Archana Anokhe, https://orcid.org/0000-0003-3906-3132
Somasundaram Jayaraman, https://orcid.org/0000-0003-3486-4109
Murugaiyan Sinduja, https://orcid.org/0000-0001-9051-5523
Velusamy Sathya, https://orcid.org/0000-0003-3153-7196
Cherukumalli Srinivasa Rao, https://orcid.org/0000-0003-0635-3141