- 1Departamento de Investigación y Posgrado en Alimentos, Universidad de Sonora, Hermosillo, Sonora, Mexico
- 2Departamento de Tecnología Agroalimentaria, Universidad Miguel Hernández de Elche, Orihuela, Spain
Phytopathogenic fungi are a constant danger in the production of different crops around the world, especially in melons, since they can cause significant economic losses during the harvest, affecting the quality and shelf life. In recent years, producers have increasingly used chemical pesticides indiscriminately, causing environmental problems and damage to public health. For this reason, phytopathogenic fungi become more resistant. However, it is essential to guarantee the safety, quality, and shelf life of food after harvest, during transportation, storage and marketing. The presence of fungi in food can cause diseases transmitted through the production of toxins. Most producers depend on the discriminated use of chemical pesticides, which is a great challenge to guarantee food safety and sustainable agricultural production. To solve this problem, some extracts derived from tomato plants after harvest containing bioactive compounds have been implemented. These compounds can be natural antifungal agents as they contain phenols, flavonoids, and vitamins. Bioactive compounds emerge as a sustainable and safe opportunity in the search for new antifungal and antimicrobial agents. Therefore, the objective of this study was to determine the in vitro antifungal activity of whole tomato plant extracts on three phytopathogenic fungi. The research findings indicated that a concentration of 74.7 μg/mL of TPE resulted in a complete inhibition of mycelial growth in Fusarium oxysporum, Fusarium graminearum, and Fusarium verticillioides. Additionally, TPE exhibited both fungistatic and fungicidal effects on these Fusarium species, with a MIC50 of 30.7, 31.5, and 29.5, and a MFC of 82.4, 78.6, and 75.8 μg/mL, respectively. As a result, this study suggests that TPE can be considered as an environmentally friendly solution for extracting tomato plants, which can be applied to the surface of whole fruits or incorporated into semi-processed foods.
1 Introduction
Postharvest losses are mainly due to microorganisms and the environment, affecting functionality, quality, and appearance. The effect of these factors is extensive and can generate symptoms ranging from very subtle and not visible to severe damage such as malformations, tissue disintegration, or others that are perfectly visible. Moreover, in the course of their postharvest maturation, phytopathogenic fungi can invade the fruits. These fungi can cause irreversible damage, rot, having a bad smell and internal and external damage. In addition to producing mycotoxins, they can contaminate adjacent foods (Molina et al., 2015; Bautista-Baños et al., 2016).
The growth of microorganisms, primary fungi on fruits, in addition to causing rot, can make them unsuitable for consumption due to the presence of mycotoxins. These mycotoxins are secondary metabolites produced by the metabolism of certain fungi, which are toxic to animals and humans when consumed. They can even be immunosuppressive, carcinogenic, nephrotoxic, and neurotoxic, especially when they occur in repeated exposure (Feijó et al., 2018). Fungi that develop mycotoxins are known as toxigenic fungi. This group is mainly dominated by Penicillium, Fusarium, and Aspergillus, at a lower level by Claviceps, Stachybotrys, and Alternaria (Greff-Laubscher et al., 2020).
The economic impact of postharvest rot caused by fungi is unpredictable and lacks official documentation. However, according to Pedraza-Zapata et al. (2017) global economic losses reach between 30 and 50%, an important challenge is to reduce the environmental impact by improving agriculture, health, commerce and industries, as well as using biological alternatives that ensure control of these fungi. But on some occasions, rotting can lead to the total loss of horticultural products. Both fungi and bacteria produce rot; however, it has been reported that fungi infect hosts more postharvest than bacteria (Bautista-Baños et al., 2016).
Pesticides have been used to control or inhibit fungi that severely affect crops. However, the excessive use of these products has been increasing year after year causing the load of these substances in the atmosphere, hydrosphere, soil and sediments causing environmental pollution and in public health (Hernández-Castellanos et al., 2021). Therefore, recently, research has been carried out to obtain natural substances that can inhibit the growth of these fungi that can be obtained from different sources including plants, animals, bacteria, algae and fungi (Añibarro-Ortega et al., 2020).
Worldwide, the production of tomato (Solanum lycopersicum L.) generates a large amount of vegetable by-products at the end of the harvest, without commercial value which are discarded as waste and sometimes left outdoors (Tej et al, 2018). This is considered a serious problem of contamination, due to the production of methane and a possible phytopathogenic contamination for the surrounding fields. In this sense, the by-products can be recovered to obtain bio-based ingredients with bioactive properties (Independent Group of Scientists appointed by the Secretary-General, 2019; Añibarro-Ortega et al., 2020). Different parts of plants, such as leaves, cuticles, peels, pulp, seeds, stems and roots, are rich in bioactive substances. These substances may have natural antioxidant, antimicrobial, antiviral, and antifungal activities, including phenolic compounds, flavonoids, and vitamins. Bioactive substances emerge as a sustainable and safe opportunity in the search for new antifungal agents (Silva-Beltrán et al., 2015; Shirahigue and Ceccato-Antonini, 2020).
Nowadays, the generation of by-products or agro-industrial waste produced through the growth, and development of plants is a global problem. In most cases, this waste is not processed and is left out in the open, a situation that contributes to the process of environmental contamination. Agro-industrial residues have a high potential to be used in different strategies that include producing new products, giving them value-added and the bioactive properties that they may have (Rosas et al., 2016; Vargas and Pérez, 2018).
Bioactive compounds are characterized by being components with biological activity capable of adapting to metabolic processes, acting to promote health conditions. Their benefits include antioxidant, antimicrobial, antifungal activity, induction or inhibition of enzymes, gene expression, and inhibition of receptor activities (Vuolo et al., 2019).
A natural alternative to inhibit fungi after harvest is the use of extracts obtained from various whole plants since they contain bioactive substances, such as tomato plant extract. Research describing the antimicrobial and antioxidant activities of plant by-products has been increasing, particularly with extracts from grape seeds, olive residues and different anatomical regions of tomato plants, which could contribute to improving the safety and food quality (Coelho et al., 2023). Therefore, the objective of this study was to determine the in vitro antifungal activity of whole tomato plant extracts on three phytopathogenic fungi.
2 Materials and methods
2.1 Plant material
Tomato plants (Lycopersicon esculentum) obtained from the Vigo Produce greenhouse in the La Rosita ejido in the municipality of Francisco I. Madero, Coahuila, Mexico (Latitude N: 28°17′20.779″ and Longitude W: 103°17′ 16.476″ 1,320 masl) were used in this study. Twenty complete fresh plants of the Prunaxx variety were manually collected. The plants were transported in plastic bags to the Food Safety Laboratory of the Experimental Field La Laguna-INIFAP, Matamoros, Coahuila; Mexico for processing. The leaves, pedicles, stems and roots of each one were separated and washed with distilled water. Subsequently, the plants were placed in a vacuum oven at 45°C for 24 h to dry and then milled for later use.
2.2 Extract obtaining
Tomato plant extract (TPE) was obtained following the methodology proposed by Silva-Beltrán et al. (2015) with some modifications. 35 g of the tomato plant were weighed and 280 mL of ethanol-acetic acid (95:5, v/v) was added. The mixture was homogenized for 72 h at 28°C and in complete darkness. Subsequently, the resulting solution was filtered using Whatman # 1 filter paper. The solution was concentrated on a rotary evaporator. The extract obtained was stored at −20°C until use.
2.3 Antioxidant activity and total phenolic compounds of tomato plant extract
2.3.1 DPPH˙assay
This assay was conducted according to Prior et al. (2005) with some modifications. An aliquot of 200 μL of a solution of DPPH˙ radical (0.001 mg/mL in methanol) was mixed with 20 μL/mL of TPE and shaken. It was left to stand for 30 min at 28°C. Subsequently, the absorbance at 515 nm was measured using a using a microplate reader (Thermo Fisher Scientific Inc. Multiskan GO, New York, NY, United States). The control sample was prepared with 200 μL of DPPH˙ and 20 μL/mL of ethanol. Antioxidant activity was calculated using a Trolox calibration curve and results were expressed as Trolox equivalents per g extract (μmol/TE) per g extract (ge). Measurements were made in triplicate.
2.3.2 ABTS·+ assay
This assay was conducted according to Toor et al. (2009) with some modifications. 0.02 g of ABTS·+ cationic radicals were weighed out and dissolved in 5 mL of distilled water. Subsequently, they were mixed with 88 μL of a sodium persulfate solution and left to stand in the dark for between 12 and 16 h at 28°C. Then, in 88 mL of ethanol, 1 mL of the prepared solution was added. An aliquot of 270 μL of the prepared cationic moiety solution was mixed with 20 μL of TPE and shaken. It was left to stand for 30 min at 28°C. Subsequently, the absorbance at 734 nm was measured. The control sample was prepared with 270 μL of the cationic moiety and mixed with 20 μL of ethanol. The Trolox equivalent antioxidant capacity (TEAC) value was expressed as trolox equivalents(μmol/TE) per g of extract (ge). Measurements were made in triplicate.
2.3.3 FRAP assay
The reductant power of TPE was determined by the FRAP assay described by Benzie and Strain (1996), with some modifications. Stock solutions were sodium acetate buffer (300 mM, pH 3.6), FeCl3 (20 mM) and TPTZ (2,4,6-tripyridyl-s-triazine) solution (10 mM) in 36% HCl (40 mM). The FRAP solution was prepared in a ratio of 10:1:1 (Buffer: FeCl3: TPTZ). An aliquot of 20 μL of TPE was mixed with 280 μL of FRAP and incubated at 37°C for 30 min. Subsequently, its absorbance at 638 nm was measured. The control sample was prepared with 20 μL of ethanol and 280 μL of the FRAP solution. The results were expressed in Trolox equivalents (μmol/TE) per g of extract (ge). Measurements were performed in triplicate.
2.3.4 Total phenolics content
The concentration of total phenolic content was determined using Folin–Ciocalteu technique described by Magalhães et al. (2010) with some modifications. An aliquot of 10 μL of TPE was mixed with 25 μL of Folin–Ciocalteu 1 N reagent and allowed to stand for 5 min at 28°C. Then, 25 μL of 20% (w/v) Na2CO3 and 140 μL of distilled water were added. It was left to stand for 30 min at 28°C. The absorbance was read at 765 nm using a spectrophotometer. The control sample used ethanol. The total concentration of phenolic compounds was calculated using a gallic acid equivalent (GAE) standard curve and expressed as milligrams per gram of extract (mg GAE/ge). Analyzes were performed in triplicate.
2.3.5 Total flavonoids content
The flavonoid content was determined based on the technique described by Chen et al. (2014) with some modifications. An aliquot of 80 μL of TPE was mixed with 80 μL of ethanolic solution of aluminum trichloride (20 w/v). Afterwards, it was shaken for 30 s, covered with parafilm and incubated for 1 h at 25°C. Its absorbance at 415 nm was read in a spectrophotometer, using quercetin as the standard. The total concentration of flavonoid compounds was calculated using a quercetin equivalent (QE) standard curve and expressed as milligrams per gram of extract (mg EQ/ge). Analyzes were performed in triplicate.
2.4 Antifungal activity
2.4.1 Fusarium strains
The strains of Fusarium oxysporum, Fusarium verticillioides, and Fusarium graminearum were provided by the Laboratory of Plant Biotechnology, and Postharvest of the Food and Development Research Center, A.C., the Laboratory of Microbiology of the Department of Research, and Postgraduate Studies in Food of the University of Sonora, and by the Faculty of Veterinary Medicine, and Animal Husbandry of the Department of Microbiology, and Immunology of the UNAM, respectively.
2.4.2 Spore suspension
Spores were obtained from a 10-day-old culture of F. oxysporum, F. graminearum, and F. verticillioides, respectively, grown on potato dextrose agar (PDA) medium at 28°C. The spore was harvested by pouring 10 mL of physiological saline solution (PSS) containing 1% (v/v) Tween 80 and the surface of the mycelium was scraped with an L-shaped glass spreader. The resulting spores were counted using a Neubauer chamber (depth 0.1 mm, 0.0025 mm2) and the final concentration was adjusted to 1×104 spores/mL (Lam-Gutiérrez et al., 2018).
2.4.3 Agar disk-diffusion method
The antifungal activity was determined by measuring the inhibition zone with different concentrations of PTE on F. oxysporum, F. graminearum and F. verticillioides according to the technique described by Balouiri et al. (2016) with some modifications. Three 6 mm filter paper disks were individually impregnated with 20 μL of TPE at concentrations of 20, 30, 40, 50, 60 and 70 mg/mL, and placed in each plate on the solidified medium. A negative control was used using disks without TPE and with ethanol. They were incubated at 28°C for 14 days. The inhibition area was measured in perpendicular diameters in millimeters, obtaining an average value at 24 h. Three plates were used for all the treatments (Arce-Araya et al., 2019).
2.4.4 Poisoned food method
Tomato plant extract antifungal activity was determined with the poisoned food method proposed by Balouiri et al. (2016) and Medina-López et al. (2016) with modifications. One mL of TPE at concentrations of 4.9, 9.9, 19.6, 47.6, 74.7 and 90.9 μg/mL was poured into Petri dishes and then 10 mL of PDA medium was added. One mL of ethanol was added with 10 mL of PDA as a negative control. Disks of 5 mm diameter mycelium taken from the edge of the dishes were transferred from each eight-day-old Fusarium. They were placed in the center of the dishes containing poisoned PDA medium and incubated at 25°C for 14 days. A mycelium disk from each fungus was used with PDA medium without TPE was used as a positive control (Duarte et al., 2014; Medina-López et al., 2016; Chacón et al., 2021). The antifungal activity was evaluated as a percentage of mycelial growth inhibition, and was calculated with the following equation:
where: C.C., average diameter in the colonies of the control sample, and C.T., mean diameter of colony inhibition in the treated sample. Three plates were used for all the treatments (Arce-Araya et al., 2019).
2.4.5 Determination of minimum inhibitory concentration (MIC50) and maximum fungicidal concentration (MFC)
MIC50 and MFC of the TPE were determined based on the results obtained from the percentage of mycelial inhibition against Fusarium using a polynomial function. MIC50 is defined as the lowest concentration of TPE that inhibits or retards 50% of mycelial growth and MFC is the highest concentration of TPE that inhibits or eliminates 100% of mycelial growth (Rosas-Burgos et al., 2009).
2.4.6 Fungistatic and fungicidal activity
The fungal disks that inhibited 100% mycelial growth were reseeded in Petri plates with PDA medium without TPE and incubated at 28°C for 8 days. A fungistatic effect was determined when a temporary inhibition of mycelial growth was observed, while a fungicidal effect was detected as there was no growth of the fungus after 8 days of incubation (Askarne et al., 2012).
2.5 Statistical analysis
The results were expressed as mean values ± SD using ANOVA, and the statistical difference was established at p < 0.05 using the Tukey test. A correlation analysis was performed between the antioxidant activity of TPE and phenolic and flavonoid compounds based on Pearson’s correlation coefficient. In the determination of the MIC50 and MFC, a polynomial function analysis was used. The INFOSTAT Software version 2017 was used.
3 Results and discussion
3.1 Antioxidant activity and total phenolic compounds of tomato plant extract (TPE)
By-products from tomato plants contain a wide variety of phenolic compounds, vitamins, and carotenoids. Some of these phenolic compounds have antioxidant activity that can capture free radicals due to their structural characteristics as they contain one or more aromatic rings together with one or more hydroxyl groups (Silva-Beltrán et al., 2015; Silva et al., 2020). Also, due to their structural characteristics, these phenolic compounds may have other activities such as antifungal and antibacterial. The results obtained in the antioxidant activity of the TPE of the Prunaxx variety in ABTS·+, DPPH˙ and FRAP were 29.6 ± 0.21, 25.01 ± 0.31 and 22.83 ± 0.21 μmol TE/ge, respectively. For the quantification of the content of phenolic compounds and flavonoids, they were 394.78 ± 11.11 mg GAE/ge and 208.63 ± 5.78 mg QE/ge, respectively.
Based on these results, a Pearson correlation coefficient analysis was performed between phenolic and flavonoid compounds and their TPE antioxidant activity. Table 1 shows the correlation between the phenolic and flavonoid content and the antioxidant activity.
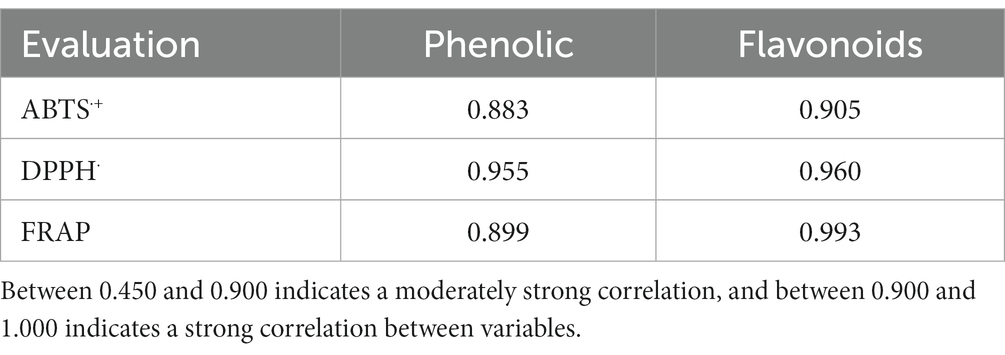
Table 1. Pearson correlation coefficient between antioxidant activity and phenolic compounds of TPE.
It has been reported that the antioxidant capacity of an extract obtained from agricultural by-products is directly correlated with the phenolic content (Spencer et al., 2005). Likewise, a moderately strong Pearson correlation coefficient was observed for the phenolic content in ABTS·+ and FRAP with values of 0.883 and 0.899, respectively, and for flavonoids in ABTS·+ with a value of 0.673. On the other hand, a strong correlation was observed in the phenolic content in DPPH˙ with a value of 0.955 and for flavonoids in DPPH˙ and FRAP with values of 0.905 and 0.995, respectively. It was shown that there is a satisfactory correlation between the phenolic content and flavonoids of the TPE with the values of ABTS·+, DPPH˙ and FRAP.
The results obtained indicate that the phenolic and flavonoid compounds of tomato by-products satisfactorily collaborated with their antioxidant activity by reducing the levels of free radicals. Similar results were obtained by Silva-Beltrán et al. (2015), who obtained a strong Pearson correlation coefficient between the phenolic content with a value of 0.944 in DPPH˙. A moderately strong correlation in phenols was shown with values of 0.783 in ABTS·+ and for flavonoids values of 0.842 and 0.586 in DPPH˙ and ABTS·+, respectively, in extracts from two whole tomato plants.
In a previous study, Añibarro-Ortega et al. (2020) implemented a Pearson correlation analysis obtaining very strong correlations between total phenolic compounds and flavonoids and DPPH˙ activity with values of −0.901 and − 0.904, respectively, in whole tomato plant extracts. These significant correlations were negative, since the lower the Catechin equivalent (EC50) values per mg, the higher the antioxidant activity of the whole tomato plant extracts.
Several studies have determined the chemical aspects and bioactive properties of tomato leaves, stems, roots and whole plants; as well as the seeds and skin of the tomato fruit (Taveira et al., 2012; Silva-Beltrán et al., 2015; Figueiredo-González et al., 2017; Añibarro-Ortega et al., 2020). These studies propose that tomato plant by-products are promising sources of bioactive components such as phenolic compounds, carotenoids, and alkaloids with antioxidant, antibacterial, and antifungal activity, as well as cardioprotective and anticancer actions (Franco-Bañuelos et al., 2017; Añibarro-Ortega et al., 2020). Results obtained in our study showed that TPE contains bioactive components such as phenolic compounds and flavonoids and that they are related to antioxidant activity and antifungal activity.
3.2 Evaluation of antifungal activity of TPE
3.2.1 Agar disk-diffusion method
The agar disk diffusion method is commonly employed to assess the susceptibility of bacteria and fungi to antibiotics, plant extracts, and essential oils. This method involves observing the formation and persistence of inhibition halos at various concentrations, which indicate the effect of these substances on microbial growth. In Table 2, the results of the antifungal activity of TPE on Fusarium strains are presented.

Table 2. Inhibition halos (mm) in fungi at different concentrations of TPE after 8 days of incubation at 28°C.
When TPE was tested against F. graminearum, the highest inhibition effect was observed at a concentration of 70 mg/mL, resulting in a halo with a diameter of 16.3 ± 1.2a mm. In contrast, F. oxysporum showed the least inhibition effect with a halo diameter of 12.8 ± 0.3b mm at a concentration of 50 mg/mL. This difference in inhibition diameter between the two Fusarium species was found to be statistically significant (p < 0.05). However, there was no significant difference (p < 0.05) in inhibition diameter between the 70 and 60 mg/mL concentrations of TPE when tested against F. oxysporum and F. graminearum. On the other hand, concentrations of 20, 30, 40 mg/mL, as well as the control, did not demonstrate any inhibition effect on fungal growth after 8 days of incubation.
The antifungal activity of TPE is not well-documented, although there is existing research on its effects against gram-positive and gram-negative bacteria. In a study conducted by Silva-Beltrán et al. (2015), the inhibitory effects of TPE were evaluated on S. aureus, L. ivanovii, E. coli O157:H7, and S. typhimurium. The results showed that TPE produced inhibition halos with diameters of 9.9 mm, 8.9 mm, 8.2 mm, and 8 mm, respectively. Another study conducted by Añibarro-Ortega et al. (2020) assessed the antifungal activity of six different tomato whole plant extracts against Aspergillus fumigatus, A. ochraceus, A. niger, Penicillium ochrochloron, P. funiculosum, and P. verrucosum. However, no antifungal activity was observed against these fungi.
Various extracts derived from different types of biologically active tomato plants have been found to exhibit varying effects on microorganisms. The impact of these extracts is contingent upon the specific species of fungus, and the effects may differ in terms of inhibiting the growth of mycelium or the germination of spores. This discovery aligns with the findings of the present study, which suggest that the antifungal activity observed in the (TPE) could be attributed to its phenolic compounds.
3.2.2 Mycelial growth inhibition percentage by the poisoned food method
The poisoned food method is mainly used to evaluate antifungal activity against phytopathogenic fungi. The extract is incorporated into the PDA culture medium with the desired concentrations. Likewise, the diameters of the growth of the fungus under study and of the control are measured, and subsequently, the percentage of mycelial growth inhibition is calculated (Ali-Shtayeh and Abu-Ghdeib, 1999; Balouiri et al., 2016).
The antifungal activity of TPE on mycelial growth in three phytopathogenic fungi was evaluated using six different concentrations. The results for F. oxysporum, F. verticillioides, and F. graminearum after 14 days of incubation, can be seen in Table 3. It was found that a concentration of 47.6 μg/mL of TPE inhibited more than 50% of mycelial growth in all three Fusarium species. Additionally, concentrations of 74.7 and 90.9 μg/mL completely inhibited mycelial growth in the three Fusarium species after 14 days of incubation as seen on Figure 1.

Table 3. Percentage of inhibition of mycelial growth of F. oxysporum, F. verticillioides, and F. graminearum with TPE at different concentrations.
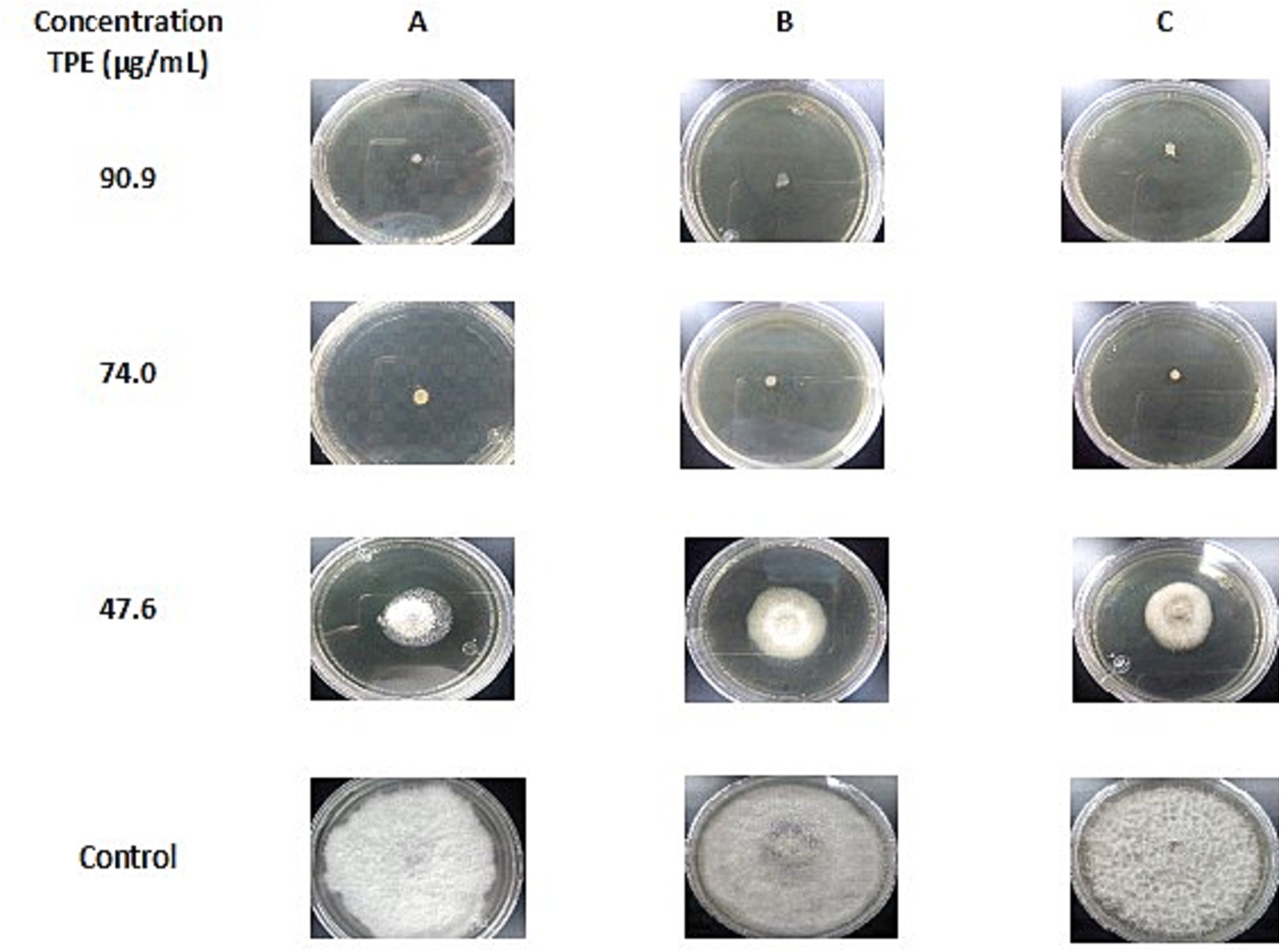
Figure 1. Inhibition of mycelial growth of (A) Fusarium oxysporum, (B) F. verticillioides and (C) F. graminearum, in PDA with different concentrations of TPE after 14 days of incubation at 28°C compared to the control.
Recent research indicates that the antifungal properties of plant extracts are primarily attributed to phenolic and flavonoid compounds. Similar findings were reported by Valencia-Botin et al. (2018), who linked the antifungal activity of phenolic compounds, tannins, and flavonoids present in Vitex mollis extracts to their effectiveness against F. verticillioides. Another study by Salhi et al. (2017) examined the effects of Asphodelus tenuifolius extracts containing phenolic compounds, flavonoids, tannins, alkaloids, and steroids on F. graminearum. In addition to ethanolic extracts, researchers have also explored the inhibitory effects of oils on mycelial growth. For instance, Chacón et al. (2021) investigated the oil derived from Piper auritum Kuntch and found that the presence of alkaloid phenolic compounds, safrole, amines, butenolides, flavonoids, and terpenes contributed to its inhibition of F. oxysporum.
3.2.3 Determination of minimum inhibitory concentration (MIC50) and maximum fungicidal concentration (MFC)
Based on the results obtained from the percentage of mycelial inhibition on F. oxysporum, F. verticillioides and F. graminearum, the MIC50 and MFC of the TPE were determined. Values were determined by polynomial function analysis and are shown in Table 4.
Different levels of mycelial growth inhibition against fungi were observed in the results of the MIC50 and MFC tests conducted on TPE. The MIC50 values ranged from 31.5 to 29.5 μg/mL, within a concentration range of 19.6 to 47.6 μg/mL. For F. oxysporum, F. verticillioides, and F. graminearum, the MFC values were 82.4, 78.6, and 75.8 μg/mL, respectively, within a concentration range of 47.6 to 74.7 μg/mL. In the case of F. graminearum, an MIC50 and MFC with greater inhibition activity can be achieved with concentrations of 29.5 and 75.8 μg/mL of TPE. However, a higher concentration of TPE is required to inhibit the mycelial growth of F. oxysporum and F. verticillioides. Therefore, the effectiveness of TPE on F. graminearum is greater at lower concentrations compared to F. oxysporum and F. verticillioides.
3.2.4 Fungistatic and fungicidal activity
A fungistatic activity refers to the temporary inhibition or slowing down of mycelial growth, while a fungicidal activity indicates a complete inhibition of mycelial growth after 8 days of incubation at 28°C. The results presented in Table 4 demonstrate the antifungal activity of different concentrations of TPE against the Fusarium species as measured by the percentage of mycelial growth inhibition. Concentrations of 74.7 and 90.9 μg/mL were able to completely inhibit mycelial growth. As a result, the fungal disks were re-inoculated in Petri dishes with PDA medium without TPE and incubated for 8 days at 28°C. When the concentration of 74.7 μg/mL was used, mycelial growth was observed after 48 h of incubation, indicating a fungistatic effect. In contrast, the disks with a concentration of 90.9 μg/mL showed a fungicidal effect with no growth observed after 8 days of incubation. Various studies have investigated the fungicidal and fungistatic effects of extracts obtained from different plant varieties on mycelial growth. However, there is limited research on TPE demonstrating its fungicidal effect on Fusarium.
Askarne et al. (2012) evaluated extracts from 50 different plants on P. italicum. Which, 21 plants reduced mycelial growth by more than 50% and only two plants showed fungistatic activity. Research carried out with essential oil leaves of E. camaldulensis Dehnh showed a fungicidal effect on F. oxysporum, F. verticillioides, F. proliferatum and F. subglutinans. However, the essential oil did not show fungicidal activity against F. solani (Gakuubi et al., 2017). These results agree with a study by El-Khateeb et al. (2013) who showed that the highest antifungal activity of the extract obtained from the leaves of Zizyphus spinachrist was due to the effect of the phenolic compounds on the fungi B. fabae, B. cinerea, A. solani, F. solani and F. oxysporum.
These results support the present work showing that the different polyphenolic compounds of TPE are responsible for the antifungal activity. A correlation of antifungal activity with the content of total phenolic compounds and flavonoids has been determined. It can be inferred that the inhibitory activity is due to the presence of hydroxyl groups in the structure of phenolic and flavonoid compounds, since these groups can act on the cell membrane by binding to their protein molecules, acting as chelating agents to modify the composition of the membrane and cause the loss of structural components, changing its physiological state (Rongai et al., 2015; Silva-Beltrán et al., 2015).
4 Conclusion
The findings of this research show that the tomato plant extract exhibits a strong fungicidal effect on F. oxysporum, F. verticillioides, and F. graminearum. The inhibition of mycelial growth was observed in all cases, indicating that the high concentration of phenolic compounds present in this agricultural by-product extract contributes to their potent antifungal properties. Therefore, based on the results of the TPE obtained from the agricultural by-products after the tomato harvest, a natural fungicide could be obtained on the phytopathogenic fungi F. oxysporum, F. verticillioides and F. graminearum which can be beneficial for producers and contribute to reduce agricultural waste by reusing it and obtaining bioactive compounds. In the same way, TPE could be used as an antifungal preservative in whole foods, minimally processed foods and even in the pharmaceutical industry. It is important to note that most of the available studies focus on antimicrobial activity, leaving antifungal activity aside.
Data availability statement
The raw data supporting the conclusions of this article will be made available by the authors, without undue reservation.
Author contributions
LI-R: Investigation, Writing – original draft. EM-R: Investigation, Supervision, Writing – review & editing. CT-S: Investigation, Supervision, Writing – review & editing. SR-C: Investigation, Supervision, Writing – review & editing. DV-G: Investigation, Supervision, Writing – review & editing. GS-J: Investigation, Methodology, Resources, Supervision, Writing – review & editing.
Funding
The author(s) declare that no financial support was received for the research, authorship, and/or publication of this article.
Acknowledgments
The authors thank Rosalba Troncoso-Rojas, from the Laboratory of Plant Biotechnology, and Post-harvest of the Food and Development Research Center, A.C., Hermosillo, and Carolina Segundo-Zaragoza, from the Faculty of Veterinary Medicine, and Animal Husbandry, Department of Microbiology, and Immunology, Universidad Nacional Autónoma de México, for the donations of F. oxysporum, and F. graminearum, respectively. To CONAHCyT and INIFAP for granting a scholarship to study the Ph.D. in Food Science at the University of Sonora, Hermosillo, Mexico.
Conflict of interest
The authors declare that the research was conducted in the absence of any commercial or financial relationships that could be construed as a potential conflict of interest.
Publisher’s note
All claims expressed in this article are solely those of the authors and do not necessarily represent those of their affiliated organizations, or those of the publisher, the editors and the reviewers. Any product that may be evaluated in this article, or claim that may be made by its manufacturer, is not guaranteed or endorsed by the publisher.
References
Ali-Shtayeh, M. S., and Abu-Ghdeib, S. I. (1999). Antifungal activity of plant extracts against dermatophytes. Mycoses 42, 665–672. doi: 10.1046/j.1439-0507.1999.00499.x
Añibarro-Ortega, M., Pinela, J., Ciric, A., Martins, V., Rocha, F., MD, S., et al. (2020). Valorisation of table tomato crop by-products: phenolic profiles and in vitro antioxidant and antimicrobial activities. Food Bioprod. Process. 124, 307–319. doi: 10.1016/j.fbp.2020.09.006
Arce-Araya, C., Varela-Benavides, I., and Torres-Portuguez, S. (2019). Inhibition of mycelial growth of fungi associated with anthracnose in yam (Dioscorea alata). Agronomía Mesoamericana 30, 381–393. doi: 10.15517/am.v30i2.32653
Askarne, L., Talibi, I., Boubaker, H., Boudyach, E., Msanda, F., Saadi, B., et al. (2012). In vitro and in vivo antifungal activity of several Moroccan plants against Penicillium italicum, the causal agent of citrus blue mold. Crop Prot. 40, 53–58. doi: 10.1016/j.cropro.2012.04.023
Balouiri, M., Sadiki, M., and Ibnsouda, S. (2016). Methods for in vitro evaluating antimicrobial activity: a review. J. Pharmaceutical Analysis 6, 71–79. doi: 10.1016/j.jpha.2015.11.005
Bautista-Baños, S., Romanazzi, G., and Jiménez-Aparicio, A. (2016). Chitosan in the preservation of agricultural commodities. 1st Edition. USA: Academic Press/Elsevier.
Benzie, I. F. F., and Strain, J. J. (1996). The ferric reducing ability of plasma (FRAP) as a measure of “antioxidant power”: the FRAP assay. Anal. Biochem. 239, 70–76. doi: 10.1006/abio.1996.0292
Chacón, C., Miranda-Granados, J., Ruiz-Lau, N., Lagunas-Rivera, S., Ruíz-Valdiviezo, V. M., and Gutiérrez-Miceli, F. A. (2021). In vitro antifungal activity and chemical composition of Piper auritum Kunth essential oil against fusarium oxysporum and fusarium equiseti. Agronomy 11:1098. doi: 10.3390/agronomy11061098
Chen, L., Xin, X., Yuan, Q., Su, D., and Liu, W. (2014). Phytochemical properties and antioxidant capacities of various colored berries. J. Sci. Food Agric. 94, 180–188. doi: 10.1002/jsfa.6216
Coelho, M. C., Rodrigues, A. S., Teixeira, J. A., and Pintado, M. E. (2023). Integral valorisation of tomato by-products towards bioactive compounds recovery: human health benefits. Food Chem. 410:135319. doi: 10.1016/j.foodchem.2022.135319
Duarte, Y., Pino, O., and Martínez, B. (2014). Effect of four essential oils on phytopathogenic fungi associated with spotted rains in rice. Revista Protección Vegetal 29, 62–65.
El-Khateeb, A. Y., Elsherbiny, E. A., Tadros, L. K., Ali, S. M., and Hamed, H. B. (2013). Phytochemical analysis and antifungal activity of fruit leaves extracts on the mycelial growth of fungal plant pathogens. J. Plant Pathol. Microbiol. 4, 1–6. doi: 10.4172/2157-7471.1000199
Feijó, C. J., Orso, P. B., Bordin, K., Hara, R. V., and Luciano, F. B. (2018). Toxicological effects of fumonisin B1in combination with other fusarium toxins. Food Chem. Toxicol. 121, 483–494. doi: 10.1016/j.fct.2018.09.043
Figueiredo-González, M., Valentão, P., Pereira, D. M., and Andrade, P. B. (2017). Further insights on tomato plant: cytotoxic and antioxidant activity of leaf extracts in human gastric cells. Food Chem. Toxicol. 109, 386–392. doi: 10.1016/j.fct.2017.09.018
Franco-Bañuelos, A., Contreras-Martínez, C. S., Carranza-Téllez, J., and Carranza-Concha, J. (2017). Total phenolic content and antioxidant capacity of non-native wine grapes grown in Zacatecas, Mexico. Agrociencia 51, 661–671.
Gakuubi, M. M., Maina, A. W., and Wagacha, J. M. (2017). Antifungal aActivity of essential oil of Eucalyptus camaldulensis Dehnh. Against selected fusarium spp. Int. J. Microbiol. 2017, 1–7. doi: 10.1155/2017/8761610
Greff-Laubscher, M. R., Beukes, I., Marais, G. J., and Jacobs, K. (2020). Mycotoxin production by three different toxigenic fungi genera on formulated abalone feed and the effect of an aquatic environment on fumonisins. Mycology 11, 105–117. doi: 10.1080/21501203.2019.1604575
Hernández-Castellanos, J. L., Cuervo González, R., Montañez Soto, J. L., Hernández Castellanos, N. D., Pérez Vargas, M. A., Cruz, H. A., et al. (2021). Biodegradation of organophosphate and organochlorine pesticides by Candida tropicalis and Stenotrophomonas maltophilia in soil microcosms. Int. J. Environ. Pollut. 37, 553–564. doi: 10.20937/RICA.53889
Independent Group of Scientists appointed by the Secretary-General. (2019). Global sustainable development report. The Future is Now – Science for Achieving Sustainable Development. United Nations, New York.
Lam-Gutiérrez, A., Ayora-Talavera, T. R., Garrido-Ramírez, E. R., Gutiérrez-Miceli, F. A., Montes-Molina, J. A., Lagunas-Rivera, S., et al. (2018). Phytochemical profile of methanolic extracts from Chilca (Baccharis glutinosa) roots and its activity against aspergillus ochraceus and fusarium moniliforme. J. Environ. Biol. 40, 302–308.
Magalhães, L. M., Santos, F., Segundo, M. A., Reis, S., and Lima, J. L. F. C. (2010). Rapid microplate high-throughput methodology for assessment of Folin-Ciocalteu reducing capacity. Talanta 83, 441–447. doi: 10.1016/j.talanta.2010.09.042
Medina-López, C. F., Plascencia-Jatomea, M., Cinco-Moroyoqui, F. J., Yépiz-Gómez, M. S., Cortez-Rocha, M. O., and Rosas-Burgos, E. C. (2016). Potentiation of antifungal effect of a mixture of two antifungal fractions obtained from Baccharis glutinosa and Jacquinia macrocarpa plants. J. Environ. Sci. Health B 51, 760–768. doi: 10.1080/03601234.2016.1198641
Molina, B. E., Zaldivar, P. C., and Yañez, L. M. (2015). Physiological studies and postharvest technology of fruits and vegetables. Iztapalapa, México. Casa abierta. Universidad Autónoma Metropolitana. UAM. pp. 57.
Pedraza-Zapata, D. M., Sanchez-Garibello, M., Quevedo-Hidalgo, B., Moreno-Sarmiento, N., and Gutierrez-Rojas, I. (2017). Promising cellulolytic fungi isolates for rice straw degradation. J. Microbiol. 55, 711–719. doi: 10.1007/s12275-017-6282-1
Prior, R. L., Wu, X., and Schaich, K. (2005). Standardized methods for the determination of antioxidant capacity and phenolics in foods and dietary supplements. J. Agric. Food Chem. 53, 4290–4302. doi: 10.1021/jf0502698
Rongai, D., Pulcini, R., Pesce, B., and Milano, F. (2015). Antifungal activity of some botanical extracts on fusarium oxysporum. Open Life Sci. 10, 409–416. doi: 10.1515/biol-2015-0040
Rosas, D., Ortiz, H., Herrera, J., and Leyva, O. (2016). Revaluation of some agro-industrial waste and its potential for application to agricultural soils. Agroproductividad 9, 18–23.
Rosas-Burgos, E. C., Cortez-Rocha, M. O., Cinco-Moroyoqui, F. J., Robles-Zepeda, R. E., López-Cervantes, J., Sánchez-Machado, D. I., et al. (2009). Antifungal activity in vitro of Baccharis glutinosa and Ambrosia confertiflora extracts on aspergillus flavus, aspergillus parasiticus and fusarium verticillioides. World J. Microbiol. Biotechnol. 25, 2257–2261. doi: 10.1007/s11274-009-0116-1
Salhi, N., Mohammed, S. S. A., Terzi, V., Brahmi, I., Ghedairi, N., and Bissati, S. (2017). Antifungal activity of aqueous extracts of some dominant algerian medicinal plants. Biomed. Res. Int. 2017:6. doi: 10.1155/2017/7526291
Shirahigue, L. D., and Ceccato-Antonini, S. R. (2020). Agro-industrial wastes as sources of bioactive compounds for food and fermentation industries. Ciência Rural 50, 1–17. doi: 10.1590/0103-8478cr20190857
Silva, J. S., Ortiz, D. W., Garcia, L. G. C., Asquieri, E. R., Becker, F. S., and Damiani, C. (2020). Effect of drying on nutritional composition, antioxidant capacity and bioactive compounds of fruits co-products. Food Sci. Technol. 40, 810–816. doi: 10.1590/fst.21419
Silva-Beltrán, N. P., Ruiz-Cruz, S., Cira-Chávez, L. A., Estrada-Alvarado, M. I., Ornelas-Paz, J. J., López-Mata, M. A., et al. (2015). Total phenolic, flavonoid, tomatine, and tomatidine contents and antioxidant and antimicrobial activities of extracts of tomato plant. Int. J. Analytical Chem. 4, 1–10. doi: 10.1155/2015/284071
Spencer, J. P. E., Kuhnle, G. G. C., Hajirezaei, M., Mock, H. P., Sonnewald, U., and Rice-Evans, C. (2005). The genotypic variation of the antioxidant potential of different tomato varieties. Free Radic. Res. 39, 1005–1016. doi: 10.1080/10715760400022293
Taveira, M., Ferreres, F., Gil-Izquierdo, A., Oliveira, L., Valentão, P., and Andrade, P. B. (2012). Fast determination of bioactive compounds from Lycopersicon esculentum mill. leaves. Food Chem. 135, 748–755. doi: 10.1016/j.foodchem.2012.05.016
Tej, R., Rodríguez-Mallol, C., Rodríguez-Arcos, R., Karray-Bouraoui, N., and Molinero-Ruíz, L. (2018). Inhibitory effect of Lycium europaeum extracts on phytopathogenic soil-borne fungi and the reduction of late wilt in maize. Eur. J. Plant Pathol. 152, 249–265. doi: 10.1007/s10658-018-1469-9
Toor, R. K., Savage, G. P., and Lister, C. E. (2009). Release of antioxidant components from tomatoes determined by an in vitro digestion method. Int. J. Food Sci. Nutr. 60, 119–129. doi: 10.1080/09637480701614121
Valencia-Botin, A. J., Gutiérrez-Lomelí, M., Morales-Del-Rio, J. A., Guerrero-Medina, P. J., Robles-García, M. A., Ruiz-Cruz, S., et al. (2018). Inhibitory effect of Vitex mollis kunth extracts against bacteria and fusarium species of human and agricultural importance. Rev. Fitotec. Mex. 41, 353–363. doi: 10.35196/rfm.2018.4.353-363
Vargas, C. Y. A., and Pérez, P. L. I. (2018). Use of agro-industrial waste in improving the quality of the environment. Facultad de Ciencias Básicas 14, 59–72. doi: 10.18359/rfcb.3108
Keywords: tomato plant, antifungal activity, antioxidant, agroindustrial byproducts, Fusarium
Citation: Isidro-Requejo LM, Márquez-Ríos E, Del Toro-Sánchez CL, Ruiz-Cruz S, Valero-Garrido D and Suárez-Jiménez GM (2023) Tomato plant extract (Lycopersicon esculentum) obtained from agroindustrial byproducts and its antifungal activity against Fusarium spp. Front. Sustain. Food Syst. 7:1323489. doi: 10.3389/fsufs.2023.1323489
Edited by:
Carlos Gregorio Barreras-Urbina, National Council of Science and Technology (CONACYT), MexicoReviewed by:
Miguel Angel Robles Garcia, University of Guadalajara, MexicoIdalia Osuna-Ruiz, Polytechnic University of Sinaloa, Mexico
Juan José Reyes Pérez, Universidad Técnica Estatal de Quevedo, Ecuador
Copyright © 2023 Isidro-Requejo, Márquez-Ríos, Del Toro-Sánchez, Ruiz-Cruz, Valero-Garrido and Suárez-Jiménez. This is an open-access article distributed under the terms of the Creative Commons Attribution License (CC BY). The use, distribution or reproduction in other forums is permitted, provided the original author(s) and the copyright owner(s) are credited and that the original publication in this journal is cited, in accordance with accepted academic practice. No use, distribution or reproduction is permitted which does not comply with these terms.
*Correspondence: Guadalupe M. Suárez-Jiménez, miroslava.suarez@unison.mx