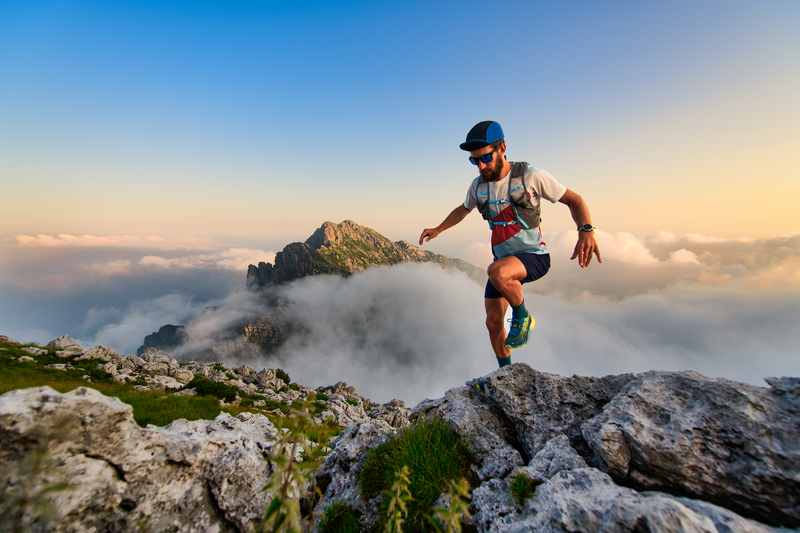
94% of researchers rate our articles as excellent or good
Learn more about the work of our research integrity team to safeguard the quality of each article we publish.
Find out more
ORIGINAL RESEARCH article
Front. Sustain. Food Syst. , 14 November 2023
Sec. Agro-Food Safety
Volume 7 - 2023 | https://doi.org/10.3389/fsufs.2023.1303753
This article is part of the Research Topic The Hazards and Nutritional Benefits of Metal(loid)s in Food and Environment View all 7 articles
Cadmium (Cd) is a highly toxic heavy metal that can severely hinder plant growth and development. Tomato is one of the most important economical crops in the world, and its quality and safety are closely related to human health. Therefore, it is important to elucidate the molecular mechanisms involved in tomato plant responses to Cd stress. In this study, tomato plants were treated with or without 100 μM Cd2+ in hydroponic culture for 3 days. Transcriptional changes in tomato roots and shoots were examined by transcriptome sequencing techniques. A total of 1,123 differentially expressed genes (DEGs) were identified in roots and 159 DEGs were identified in shoots after Cd treatment, including 15 DEGs were upregulated and 24 DEGs were downregulated in both roots and shoots. KEGG enrichment analysis showed that DEGs in the roots and shoots under Cd stress were significantly enriched in the glutathione metabolism pathway, sulfur metabolism pathway, phenylpropanoid biosynthesis, plant-pathogen interaction cutin pathway, suberine and wax biosynthesis pathway, and photosynthesis-antenna proteins pathway. 15 DEGs were further validated by quantitative real-time RT-PCR, including ABC transporter genes, WRKY transcription factors, and NAC transcription factors, among others. This study will provide a theoretical basis for further research on the molecular mechanisms involved in tomato responses to Cd stress, and genetic improvement of Cd tolerance.
With the rapid development of industrial and agricultural production in recent years, abiotic stresses such as drought, cold, high temperatures, high salt, and heavy metals have become increasingly serious environmental problems that restrict crop yields all over the world (Ashraf and Foolad, 2007; Lata and Shivhare, 2021). Cadmium (Cd) is a highly toxic heavy metal element that is harmful to plant growth and development, and is increasingly causing grain production losses and economic losses (Li et al., 2023). Cd concentrations in the natural environment are usually low, but the expansion of human activities such as mineral mining, smelting, and the use of phosphate fertilizers containing Cd has led to the increase Cd pollution in soil. Plants growing in soils with excessive Cd exhibit obvious symptoms of damage such as yellowing, root growth inhibition, and impaired photosynthesis (Alcántara et al., 1994; Zhang et al., 2014, 2015; Chiao et al., 2020; Zhao et al., 2021). Understanding the process of plant Cd accumulation or molecular mechanisms is critical importance for plant growth and crop yield.
Many studies have investigated the physiological mechanisms involved in Cd tolerance in plants, and a large number of Cd tolerance genes have been cloned and identified, including complex transporters, metallothionein synthase, and transcription factors (Zhang et al., 2022). Cd is a non-essential element in plants. Cd enters root cells mainly through transporters/channels of essential elements (such as iron, manganese, zinc, and calcium), such as zinc/iron-regulated transporter-like proteins (ZIPs), and natural resistance-associated macrophage protein (Nramp) transporter (Asgher et al., 2015). For example, OsNramp5 was localized in rice root cell membrane, and the Cd content in plants and grains was significantly decreased after OsNramp5 deletion (Tang et al., 2022). Cd or Cd complexes that enter root cells can be excreted from the cell via Cd transporters on the cell membrane. ATP-binding cassette (ABC) transporters are one of the largest protein families. They use the energy generated by ATP hydrolysis to transport various solutes and catalyze the input of many primary metabolites (Kang et al., 2011; Orelle et al., 2018). Kim et al. (2007) reported that ABC transporter AtABCG36/AtPDR8 is related to plant Cd tolerance in addition to pathogen defense. Overexpression of AtABCG36 in Arabidopsis improved Cd tolerance by excretion of Cd or Cd complexes (Bovet et al., 2003; Kim et al., 2007). Some transporters are evidently involved in the transport of Cd from roots to shoots. OsHMA2 was localized in rice sheath cells, and overexpression of OsHMA2 reduced Cd content in rice grains. In addition, vacuole isolation plays an important role in Cd detoxification. For example, both OsHMA3 and OsABCC9 were localized in the vacuolar membrane of root cells, and through sequestration of Cd into the root vacuoles involved in rice cadmium tolerance (Sasaki et al., 2014; Yang et al., 2021). The migration of Cd in cells promotes the detoxification of Cd in roots, which improves the tolerance of plants response to Cd toxicity (Bali et al., 2020).
Transcriptional regulation of the defense gene expression is a key step in plants resistance to abiotic stress, such as NAC, MYB, and WRKY family has been reported play an important role in plant resistance to abiotic stress. For example, the MYB transcription factor OsMYB45 was localized in the nucleus, and mutation of OsMYB45 leads to decreased expression of OsCATA and OsCATC, decreased catalase activity in plants, and increased sensitivity of rice to Cd (Hu et al., 2017). Overexpression PyWRKY75 improved Cd tolerance and enhanced the accumulation of Cd in transgenic poplars (Wu et al., 2022). Rice OsNAC15 regulates zinc and Cd tolerance by binding to the ZDRE motif in the promoters of OsZIP7 and OsZIP10, and inhibiting their transcription (Zhan et al., 2022). However, little is known about the role of transcription factors in tomato response to Cd stress.
Tomato is one of the most largest horticultural crop in the world. Many studies investigating tomato response to salt, drought, and heat stress have been reported. However, the transcriptional response of tomato to Cd accumulation is still unclear. Transcriptome sequencing is an effective technique for examining molecular mechanisms of gene expression networks and complex biological pathways by systematically studying gene transcription at the entire transcriptional level. In order to investigate potential mechanisms involved in tomato response to Cd stress, comparative transcriptome analysis of tomato roots and shoots under Cd stress was conducted in the current study. Differentially expressed genes (DEGs) were then further verified by qRT-PCR. The results of the present study will accelerate research-investigating responses of tomato to Cd stress, have practical reference significance in the field of tomato Cd tolerance breeding, and promote the effective utilization of tomato resources for human life.
Seeds of Solanum lycopersicum Mill. cv. Ailsa Craig were surface sterilized using 75% ethanol and 1% sodium hypochlorite, then rinsed thoroughly with distilled H2O. They were then germinated on 1/2 MS medium and cultivated under controlled conditions (16-h photoperiod at 28 ± 2°C) until two true leaves had grown. The resulting seedlings were carefully uprooted, washed thoroughly with ddH2O, and then transferred to 1/2 Hoagland nutrient solution (pH 5.8) which was replaced every 4 days. 30-day-old seedlings were then divided into two groups treated with 0 or 100 μM Cd2+. After 72 h roots and shoots were sampled and recorded as CK_R (roots of the control group), CK_S (shoots of the control group), Cd_R (roots of the Cd treatment group), and Cd_S (shoots of the Cd treatment group). Each group was composed of three biological replicates. The samples were rapidly frozen in liquid nitrogen and stored at −80°C. All the samples were sent to Shanghai Majorbio for transcriptome sequencing.
Total RNA was extracted from samples using TRIzol reagent (Amboin, United States) in accordance with the manufacturer’s instructions. The purity and concentration of total RNA in each sample was detected by Nanodrop spectrophotometer (Termo Scientifc). cDNA library construction and RNA-seq were performed by Shanghai Majorbio Bio-pharm Technology Co., Ltd. (Shanghai, China). The main cDNA library processes were as follows: (1) mRNA was enriched with Oligo (dT) magnetic beads. (2) mRNA was randomly broken into short fragments with fragmentation buffer. (3) The first cDNA strand was synthesized using random hexamer primers, then the second cDNA strand was synthesized using DNA polymerase I, dNTPs, and RNase H. The double-stranded cDNA was purified with AMPure XP beads. (4) The purified double-stranded cDNA was subjected to end reparation, including addition of an “A” tail and ligation to the sequencing adaptors, then AMPure XP beads were used for fragment size selection. (5) The purified cDNA template was enriched via PCR amplification. (6) Twelve cDNA libraries were constructed and sequenced using the Illumina HiSeq platform.
For Illumina RNA-seq, the raw RNA-seq reads were trimmed and quality controlled by fastp.1 Then clean reads were separately aligned to reference genome of Solanum lycopersicum2 with orientation mode using Hisat23 software. The mapped reads of each sample were assembled by StringTie4 software. GO was annotated using Blast2GO5 software. KOBAS 2.1.1 software6 was used to obtain unigene Genes and Genomes orthology results in Kyoto encyclopedia of genes and genomes (KEGG) pathway analysis. The predicted amino acid sequences of unigenes were aligned with the Protein Family (Pfam) database using HMMER 3.2.1 software (https://selab.janelia.org/pub/software/hmmer3/3.0/hmmer-3.0.tar.gz; E value <10−10) to acquire unigene annotation information.
DESeq27 software was used for the screening of DEGs. Based on the alignment results, gene expression levels were normalized using fragments per kilobase of transcript per million mapped reads (FPKM) values. In statistical analysis, the corrected p value, i.e., false discovery rate (FDR), was used as the key factor for DEG screening. An FDR < 0.05 and a fold change (FC) ≥ 2 were set as threshold parameters in the screening process. Volcano plots combining the statistical significance of the FDR with FC were generated. The abscissa was represented by log2 FC, and the ordinate was represented by −log10 FDR. Genes in the upper left and upper right parts of the volcano plot represent the most statistically significant DEGs with the largest FCs. Goatools8 software was used to analyze the functional GO enrichment of DEGs. KEGG pathway enrichment analysis of DEGs was conducted using Python scipy9 software. The enrichment degree of KEGG pathways was analyzed using the enrichment factor, and the significance of enrichment was calculated via Fisher’s exact test.
The protein–protein interaction (PPI) network of DEGs was constructed by Cytoscape (v3.9.1) using the STRING online database.
To verify the reliability of the sequencing results of DEGs, 15 DEGs from roots were detected by quantitative real-time RT-PCR (qRT-PCR). Total RNA (1 μg) was reverse transcribed using a reverse transcriptase. First-strand cDNA was synthesized using HiScriptII 1st Strand cDNA Synthesis Kit (+gDNA), HiScriptII Q RT SuperMix for qPCR (Vazyme), and Q RT SuperMix for qPCR (Vazyme). qRT-PCR experiments were analyzed using the ChamQ SYBR qPCR Master Mix (Vazyme) in accordance with the manufacturer’s instructions. The specific primers used in the qRT-PCR analysis were designed using Primer 5.0 sofware (Premier Biosof International) as shown in Supplementary Table 1. SlCAC was used as an internal gene. Three technical replicates were performed for each gene. Expression levels were calculated by 2−ΔΔCt method.
The transcriptomes of 12 samples were sequenced via the Illumina Hiseq high throughput-sequencing platform, and a total of 89.54 Gb of clean data were obtained. At least 6.66 Gb of clean data were obtained per sample, the average GC content was 43.36%, and the ratio of Q30 was >93.56%. All clean reads were mapped to the tomato reference transcriptome. The percentages of mapped reads were similar among the 12 libraries (90.35–97.25%; Table 1).
The DEGs were identified by pairwise comparisons of libraries from Cd stress and the control in roots and shoots. A total of 1,123 DEGs were detected in the roots (CK_R vs. Cd_R), of which 554 DEGs were upregulated and 569 DEGs were downregulated (Figure 1A). 159 DEGs were detected in the shoots (CK_S vs. Cd_S), of which 57 DEGs were upregulated and 102 DEGs were downregulated. Among them, there were 15 DEGs that were upregulated and 24 DEGs that were downregulated in both roots and shoots (Supplementary Table 2). In intra-group comparisons of different group, the number of DEGs that were downregulated in shoots was higher than that of upregulated, and that trend was the same in roots (Figures 1B,C).
Figure 1. Venn diagrams and volcano plots of DEGs in different comparative groups. (A) Venn diagrams of DEGs in different comparative groups. (B) Volcano plot of significantly up- or downregulated genes in CK_R vs. Cd_R. (C) Volcano plot of significantly up- or downregulated genes in CK_S vs. Cd_S.
Gene ontology functional enrichment analysis was carried out to further characterize the main biological functions of DEGs in tomato roots and shoots under Cd stress. In GO analysis 1,123 DEGs with at least one annotation were obtained in tomato roots and shoots under Cd stress. These DEGs were classified into three categories, including biological process (BP), cellular component (CC), and molecular function (MF). In tomato roots, a total of 114 GO terms were significant enriched under Cd stress (p adjust < 0.05), including 58 biological process, 13 cellular components, and 43 molecular function terms (Supplementary Table 3). The top 10 GO terms of BP, CC, and MF categories of significantly enriched DEGs in roots were shown in Figure 2A. The biological processes related to ion transport, such as iron ion transport (GO:0006826), metal ion transport (GO:0030001), phosphate ion transport (GO:0006817), and several molecular functions, such as glutathione transferase activity (GO:0004364), ion transmembrane transporter activity (GO:0015075), and manganese ion transmembrane transporter activity (GO:0005384), may play important roles in the tomato resistance to Cd stress. In shoots, a total of 141 GO terms were identified, 29 GO terms were significant enriched in shoots under Cd stress (p adjust < 0.05), including 17 biological process, seven cellular components, and five molecular function terms (Supplementary Table 4). The top 10 GO terms of BP, CC, and MF categories of DEGs in shoots were shown in Figure 2B. In roots and shoots, 13 GO terms were significant enriched in both comparisons (CK_R vs. Cd_R and CK_S vs. Cd_S), such as cell wall organization or biogenesis (GO:0071554), cell wall (GO:0005618), and polysaccharide metabolic process (GO:0005976).
Figure 2. The top 10 GO terms of BP, CC, and MF categories of significantly impacted DEGs. (A) CK_R vs. Cd_R. (B) CK_S vs. Cd_S.
Gene ontology enrichment was also conducted to analysis the 15 DEGs that were upregulated and 24 DEGs that were downregulated in both roots and shoots. A total of 21 GO terms were identified in 15 DEGs and 53 GO terms were identified in 24 DEGs, respectively. In upregulated DEGs, the top three GO terms were “response to stimulus (GO:0050896),” “response to stress (GO:0006950),” and “response to oxidative stress (GO:0006979)” (Supplementary Figure 1A). In downregulated DEGs, the top three GO terms were “glucosidase activity (GO:0015926),” “cell wall (GO:0005618),” and “external encapsulating structure (GO:0030312)” (Supplementary Figure 1B).
Kyoto encyclopedia of genes and genomes pathway enrichment analysis was performed to estimate the number of DEGs contained at different levels. A total of 79 and 26 KEGG pathways were identified in roots and shoots DEGs analysis, of which 21 pathways were both exist in roots and shoots (Supplementary Tables 5, 6). In roots, five KEGG pathways were significantly enriched under Cd stress (adjusted p < 0.05), including phenylpropanoid biosynthesis, glutathione (GSH) metabolism, plant-pathogen interaction, sulfur metabolism, and cutin, suberine, and wax biosynthesis (Figure 3A). In the shoots, two KEGG pathways were significantly enriched under Cd stress (adjusted p < 0.05), including photosynthesis-antenna proteins and phenylpropanoid biosynthesis (Figure 3B; Table 2).
A PPI network based on STRING database was constructed by Cytoscape. After filtering out the non-interacting proteins, we were left with a PPI network with 245 nodes and 300 edges for the DEGs from the CK_R vs. Cd_R comparison, and the top five sub-network were shown in Figure 4A. In shoots, a PPI network was analyzed with 46 nodes and 32 edges for the DEGs from the CK_S vs. Cd_S comparison, and the top three sub-network were shown in Figure 4B.
Figure 4. PPI network analysis. (A) The top five modules of differentially expressed genes of CK_R vs. Cd_R. (B) The top three modules of differentially expressed genes of CK_S vs. Cd_S.
When plants are subjected to Cd stress, transcription factors (TFs) combine with Cd-related genes to regulate expression of related genes at the transcriptional level. In the roots, the 1,123 DEGs included 20 TF families, and the distribution of these TFs was shown in Figure 5. The top 5 TF families were MYB (21.54%), WRKY (16.92%), ERF (10.77%), NAC (12.31%), and bHLH (9.23%) families (Figure 6). In shoots under Cd stress, 159 DEGs included three ERF transcription factors (Solyc08g082210.4, Solyc12g009240.1, and Solyc11g012980.1), one bHLH transcription factor (Solyc01g106460.4), one HSF transcription factor (Solyc04g016000.3), one Trihelix transcription factor (Solyc09g005560.4), and one GATA transcription factor (Solyc04g015360.3). The results indicated that TF families such as NAC, WRKY, bHLH, and MYB superfamily might play important roles in the regulation of tomato response to Cd stress. Differential expression levels of TFs in the same family demonstrated that different members might have distinct mechanisms in plant responses to Cd stress, and appear to exhibit different biological functions.
Figure 6. The expression pattern of transcription factor and transporter genes in the root of tomato after Cd treatment. Transcription levels were verified by qRT-PCR with SlCAC as an internal control. (A-J) The relative gene expression of transcription factor genes examined by qRT-PCR. (K-O) The relative gene expression of transporter genes examined by qRT-PCR.
To further verify the reliability of gene changes identified in comparative transcriptome analyses, several key genes with significant differences were analyzed via qRT-PCR. Expression levels under Cd treatment and the control condition were shown in Figure 6, including 10 transcription factors (Figures 6A–J) and five transporter genes (Figures 6K–O). Expression levels of these genes were all consistent with the transcriptome analysis (Supplementary Table 7), indicating that the DEGs identified via comparative transcriptome analysis were reliable.
Tomato is a highly nutritious fruit and vegetables that is widely consumed in the world. Fruit quality and metabolite biosynthesis of tomato are affected by adverse plant growth conditions, including water scarcity, soil salinization, and other abiotic stresses such as Cd (Alyemeni et al., 2018; Diouf et al., 2018). Tomato is a vegetable that accumulates Cd readily in the fruit, and the Cd concentration in tomato after Cd treatment is two or even three times the limit of edible concentration in vegetables (Hussain et al., 2015; Xu Z. M. et al., 2018). Tomato yields and fruit quality decrease after Cd stress, substantially reducing global yields (Hussain et al., 2017). Currently most research on tomato is focused on domestication, breeding, fruit development and ripening, and defense regulation against biological and abiotic stress (Xie et al., 2021; Wei et al., 2022). However, there were few studies on the genes and molecular mechanisms of tomato response to cadmium stress. In tomato, Cd toxicity markedly inhibits its growth and development (Hussain et al., 2019). Reducing Cd accumulation elucidating the mechanism of tomato in Cd stress are of great significance for improving yield and quality and food safety.
In recent years, various omics technologies have emerged and been widely used in plant science, life science and other fields. Among them, transcriptomics is considered one of the most widely used techniques to study the adaptation mechanism of plants to stress and the mining of stress-response genes. It has been reported that RNAseq-based methods were used to detect arsenic-responsive miRNAs in rice roots, and found that miRNAs were involved in the responses of rice to arsenic (Liu and Zhang, 2012). In the current study transcriptome analysis identified 1,123 DEGs in roots, of these, 554 genes were upregulated and 569 were downregulated. In shoots under Cd, 159 DEGs were identified, of which 57 genes were upregulated and 102 were downregulated in shoots (Figure 1). Among them, there were 15 DEGs that were upregulated and 24 DEGs that were downregulated in both roots and shoots under Cd stress (Supplementary Table 2). It is suggested that these genes may play an important role in tomato response to cadmium stress. However, the mechanisms of these DEGs under Cd stress in tomato require further investigation.
Plants have evolved well-developed mechanisms in response to Cd stress, such as cell wall binding, redistribution of transporters, compartmentalization in vacuoles, chelation with phytochelatins, recovery of damaged proteins, and antioxidant defense (Xu et al., 2017; Rui et al., 2018; Sidhu et al., 2019; Szerement and Szatanik-Kloc, 2022). In plants Cd absorption and distribution are related to many metal-regulated transporters, including heavy metal ATPase, ABC transporter, NRAMP, and ZIP family, which are important to metal homeostasis (Yu et al., 2017; Zhao et al., 2018). For example, ABC transporters AtABCC33 is considered a key factor in plant chelatin-mediated Cd tolerance in Arabidopsis (Brunetti et al., 2015). To further clarify gene functions in tomato under Cd stress in the current study, identified DEGs were analyze by GO enrichment (Figure 3). Based on GO enrichment analysis, all of these DEGs in roots and shoots were distributed in molecular function, biological process, and cellular component under Cd stress. The GO terms related to ion transport, such as iron ion transport, metal ion transport in biological processes, and glutathione transferase activity, ion transmembrane transporter activity, and manganese ion transmembrane transporter activity in molecular functions were analyzed in roots. This indicated that tomato initiated complex responsive approaches to adapt to external Cd stress. In addition, five DEGs annotated as transporter genes were also verified by qRT-PCR, Four genes encoding ABC transporter (Solyc12g013640 and Solyc07g065320), oligo peptide transporter (Solyc11g012700), and high-affinity nitrate transporter (Solyc11g069735) were found to be upregulated and one gene encoding vacuolar iron transporter (Solyc01g104820) was downregulated under Cd stress (Figure 6), which indicate that transporters may be directly involved in Cd response in tomato plants.
Glutathione can act as a substrate of GSH S-transferase, participating in the reduction of hydroperoxide, and its synthesis in plants also depends on sulfur assimilation (Edwards et al., 2000; Hernández et al., 2017). Cd often induces the production of toxic reactive oxygen species such as H2O2, -OH, and O2−, then induces oxidation of biological macromolecules including proteins, lipids, and nucleic acids, resulting in enzyme inactivation, lipid peroxidation, and membrane damage (Nagajyoti et al., 2010; Daud et al., 2016). Plants have evolved antioxidant defense mechanisms to maintain normal function, including superoxide dismutase, peroxidase, and GSH, to avoid the toxic effects of reactive oxygen species (Wang et al., 2020). In current study, KEGG enrichment analysis showed five KEGG pathways and two KEGG pathways were significantly enriched in roots and shoots under Cd stress, respectively (Figure 3). In roots, the top three KEGG pathways were phenylpropanoid biosynthesis, glutathione (GSH) metabolism, and plant-pathogen interaction. It can be speculated that tomato plants may adapting to a high Cd environment by promoting GSH accumulation, or upregulate GSH synthesis.
Transcription factors, such as WRKY, bZIP, NAC, and MYB proteins plays an important role in response to Cd stress by controlling the expression of their specific related genes (DalCorso et al., 2010; Tokumoto et al., 2019). It has been reported that JrVHAG1 participated in Cd stress by MYB transcription regulatory network and Abscisic acid (ABA) signaling pathway (Xu Z. et al., 2018). Overexpression AemNAC2 increased the cadmium tolerance of transgenic wheat (Du et al., 2020). In current study, transcriptome sequencing indicated that DEGs induced by Cd stress in tomato roots and shoots belong to multiple transcription factor families, such as MYB, WRKY, ERF, NAC, and bHLH (Figure 5). Given this and the above-described findings, it is speculated that these DEGs may improve the tolerance of tomato plants to Cd by promoting GSH accumulation, or participating in regulatory networks such as the ethylene signaling pathway. Combined with functional annotation, 10 DEGs annotated as transcription factors were verified by qRT-PCR. Nine genes encoding ERF, MYB, WRKY, and bHLH in the pathway of signal transduction were found to be upregulated and one gene encoding MYB (Solyc05g051550) was downregulated under Cd stress (Figure 6), which indicate that TFs may be directly involved in Cd response in tomato plants. Further study is required to determine the key genes and regulatory pathways of tomato response under cadmium stress. In conclusion, through this study, we hope to enhance the understanding of the mechanism involved in tomato plants response to Cd stress, provide reference for further gene function research, and provide reference for further research on the response genes and molecular mechanisms of tomato under Cd stress.
The original contributions presented in the study are publicly available. This data can be found at: https://www.ncbi.nlm.nih.gov/sra/?term=PRJNA1030794, PRJNA1030794.
LC: Methodology, Validation, Writing – original draft. MW: Data curation, Writing – original draft, Writing – review & editing. WJ: Data curation, Formal Analysis, Writing – original draft. TL: Data curation, Formal Analysis, Writing – original draft. YL: Writing – original draft, Data curation, Methodology. XW: Supervision, Validation, Writing – original draft. SF: Supervision, Validation, Writing – review & editing.
The author(s) declare financial support was received for the research, authorship, and/or publication of this article. This research was supported by the Natural Science Foundation of Hubei Province (grant number: 2022CFB990) and Hubei Key Laboratory of Edible Wild Plants Conservation and Utilization (grant number: EWPL202005).
The authors declare that the research was conducted in the absence of any commercial or financial relationships that could be construed as a potential conflict of interest.
All claims expressed in this article are solely those of the authors and do not necessarily represent those of their affiliated organizations, or those of the publisher, the editors and the reviewers. Any product that may be evaluated in this article, or claim that may be made by its manufacturer, is not guaranteed or endorsed by the publisher.
The Supplementary material for this article can be found online at: https://www.frontiersin.org/articles/10.3389/fsufs.2023.1303753/full#supplementary-material
1. ^https://github.com/OpenGene/fastp
2. ^https://solgenomics.net/organism/Solanum_lycopersicum/genome/
3. ^http://ccb.jhu.edu/software/hisat2/index.shtml
4. ^http://ccb.jhu.edu/software/stringtie/
6. ^http://kobas.cbi.pku.edu.cn/download.php
7. ^http://bioconductor.org/packages/stats/biocDESeq2
Alcántara, E., Romera, F. J., Cañete, M., and De la Guardia, M. D. (1994). Effects of heavy metals on both induction and function of root Fe (III) reductase in Fe-deficient cucumber (Cucumis sativus L.) plants. J. Exp. Bot. 45, 1893–1898. doi: 10.1093/jxb/45.12.1893
Alyemeni, M. N., Ahanger, M. A., Wijaya, L., Alam, P., Bhardwaj, R., and Ahmad, P. (2018). Selenium mitigates cadmium-induced oxidative stress in tomato (Solanum lycopersicum L.) plants by modulating chlorophyll fluorescence, osmolyte accumulation, and antioxidant system. Protoplasma 255, 459–469. doi: 10.1007/s00709-017-1162-4
Asgher, M., Khan, M. I. R., Anjum, N. A., and Khan, N. A. (2015). Minimising toxicity of cadmium in plants-role of plant growth regulators. Protoplasma 252, 399–413. doi: 10.1007/s00709-014-0710-4
Ashraf, M., and Foolad, M. R. (2007). Roles of glycine betaine and proline in improving plant abiotic stress resistance. Environ. Exp. Bot. 59, 206–216. doi: 10.1016/j.envexpbot.2005.12.006
Bali, A. S., Sidhu, G. P. S., and Kumar, V. (2020). Root exudates ameliorate cadmium tolerance in plants: a review. Environ. Chem. Lett. 18, 1243–1275. doi: 10.1007/s10311-020-01012-x
Bovet, L., Eggmann, T., Meylan-bettex, M., Polier, J., Kammer, P., Marin, E., et al. (2003). Transcript levels of AtMRPs after cadmium treatment: induction of AtMRP3. Plant Cell Environ. 26, 371–381. doi: 10.1046/j.1365-3040.2003.00968.x
Brunetti, P., Zanella, L., Paolis, A. D., Litta, D. D., Cecchetti, V., Falasca, G., et al. (2015). Cadmium-inducible expression of the ABC-type transporter AtABCC3 increases phytochelatin-mediated cadmium tolerance in Arabidopsis. J. Exp. Bot. 66, 3815–3829. doi: 10.1093/jxb/erv185
Chiao, W. T., Chen, B. C., Syu, C. H., and Juang, K. W. (2020). Aspects of cultivar variation in physiological traits related to cd distribution in rice plants with a short-term stress. Bot. Stud. 61:27. doi: 10.1186/s40529-020-00304-3
DalCorso, G., Farinati, S., and Furini, A. (2010). Regulatory networks of cadmium stress in plants. Plant Signal. Behav. 5, 663–667. doi: 10.4161/psb.5.6.11425
Daud, M. K., Mei, L., Azizullah, A., Dawood, M., Ali, I., and Mahmood, Q. (2016). Leaf-based physiological, metabolic, and ultrastructural changes in cultivated cotton cultivars under cadmium stress mediated by glutathione. Environ. Sci. Pollut. Res. Int. 23, 15551–15564. doi: 10.1007/s11356-016-6739-5
Diouf, I. A., Derivot, L., Bitton, F., Pascual, L., and Causse, M. (2018). Water deficit and salinity stress reveal many specific QTL for plant growth and fruit quality traits in tomato. Front. Plant Sci. 9:279. doi: 10.3389/fpls.2018.00279
Du, X., He, F., Zhu, B., Ren, M., and Tang, H. (2020). NAC transcription factors from Aegilops markgrafii reduce cadmium concentration in transgenic wheat. Plant Soil 449, 39–50. doi: 10.1007/s11104-019-04419-w
Edwards, R., Dixon, D. P., and Walbot, V. (2000). Plant glutathione S-transferases: enzymes with multiple functions in sickness and in health. Trends Plant Sci. 5, 193–198. doi: 10.1016/s1360-1385(00)01601-0
Hernández, L. E., Sobrino-Plata, J., Montero-Palmero, M. B., Carrasco-Gil, S., Flores-Cáceres, M. L., Ortega-Villasante, C., et al. (2017). Contribution of glutathione to the control of cellular redox homeostasis under toxic metal and metalloid stress. J. Exp. Bot. 66, 2901–2911. doi: 10.1093/jxb/erv063
Hussain, A., Ali, S., Zia-ur-Rehman, M., Qayyum, M., Wang, H., and Rinklebe, J. (2019). Responses of wheat (Triticum aestivum) plants grown in a cd contaminated soil to the application of iron oxide nanoparticles. Ecotox Environ Safe. 173, 156–164. doi: 10.1016/j.ecoenv.2019.01.118
Hussain, I., Ashraf, M., Rasheed, R., Iqbal, M., Ibrahim, M., Zahid, T., et al. (2017). Cadmium-induced perturbations in growth, oxidative defense system, catalase gene expression and fruit quality in tomato. Int. J. Agric. Biol. 19, 61–68. doi: 10.17957/IJAB/15.0242
Hussain, M. M., Saeed, A., Khan, A. A., Javid, S., and Fatima, B. (2015). Differential responses of one hundred tomato genotypes grown under cadmium stress. Genet. Mol. Res. 14, 13162–13171. doi: 10.4238/2015
Hu, S., Yu, Y., Chen, Q., Mu, G., Shen, Z., Zheng, L., et al. (2017). OsMYB45 plays an important role in rice resistance to cadmium stress. Plant Sci. 264, 1–8. doi: 10.1016/j.plantsci.2017.08.002
Kang, J., Park, J., Choi, H., Burla, B., Kretzschmar, T., Lee, Y., et al. (2011). Plant ABC Transporters. Arabidopsis Book 9:e0153. doi: 10.1199/tab.0153
Kim, D. Y., Bovet, L., Maeshima, M., Martinoia, E., and Lee, Y. (2007). The ABC transporter AtPDR8 is a cadmium extrusion pump conferring heavy metal resistance. Plant J. 50, 207–218. doi: 10.1111/j.1365-313X.2007.03044.x
Lata, C., and Shivhare, R. (2021). Engineering cereal crops for enhanced abiotic stress tolerance. Proc. Indian Natl. Sci. Acad. 87, 63–83. doi: 10.1007/s43538-021-00006-9
Li, Y., Rahman, S. U., Qiu, Z., Shahzad, S. M., Nawaz, M. F., Huang, J., et al. (2023). Toxic effects of cadmium on the physiological and biochemical attributes of plants, and phytoremediation strategies: a review. Environ. Pollut. 325:121433. doi: 10.1016/j.envpol.2023.121433
Liu, Q., and Zhang, H. (2012). Molecular identification and analysis of arsenite stress-responsive miRNAs in rice. J. Agric. Food Chem. 60, 6524–6536. doi: 10.1021/jf300724t
Nagajyoti, P. C., Lee, K. D., and Sreekanth, T. V. M. (2010). Heavy metals, occurrence and toxicity for plants: a review. Environ. Chem. Lett. 8, 199–216. doi: 10.1007/s10311-010-0297-8
Orelle, C., Durmort, C., Mathieu, K., Duchêne, B., Aros, S., and Fenaille, F. (2018). A multidrug ABC transporter with a taste for GTP. Sci. Rep. 8:2309. doi: 10.1038/s41598-018-20558-z
Rui, H., Zhang, X., Shinwari, K. I., Zheng, L., and Shen, Z. (2018). Comparative transcriptomic analysis of two Vicia sativa L. varieties with contrasting responses to cadmium stress reveals the important role of metal transporters in cadmium tolerance. Plant Soil 423, 241–255. doi: 10.1007/s11104-017-3501-9
Sasaki, A., Yamaji, N., and Ma, J. F. (2014). Overexpression of OsHMA3 enhances cd tolerance and expression of Zn transporter genes in rice. J. Exp. Bot. 65, 6013–6021. doi: 10.1093/jxb/eru340
Sidhu, G. P. S., Bali, A. S., and Bhardwaj, R. (2019). “Chapter 16-use of fungi in mitigating cadmium toxicity in plants” in Cadmium Toxicity Tolerance Plants. eds. M. Hasanuzzaman, M. N. V. Prasad, and M. Fujita (USA: Academic Press), 397–426.
Szerement, J., and Szatanik-Kloc, A. (2022). Cell-wall pectins in the roots of Apiaceae plants: adaptations to cd stress. Acta Physiol. Plant. 44:53. doi: 10.1007/s11738-022-03386-7
Tang, L., Dong, J., Qu, M., Lv, Q., Zhang, L., Peng, C., et al. (2022). Knockout of OsNRAMP5 enhances rice tolerance to cadmium toxicity in response to varying external cadmium concentrations via distinct mechanisms. Sci. Total Environ. 832:155006. doi: 10.1016/j.scitotenv.2022.155006
Tokumoto, M., Lee, J. Y., and Satoh, M. (2019). Transcription factors and downstream genes in cadmium toxicity. Biol. Pharm. Bull. 42, 1083–1088. doi: 10.1248/bpb.b19-00204
Wang, Q., Ge, C., Xu, S., Wu, Y., Sahito, Z. A., Ma, L., et al. (2020). The endophytic bacterium Sphingomonas SaMR12 alleviates cd stress in oilseed rape through regulation of the GSH-AsA cycle and antioxidative enzymes. BMC Plant Biol. 20:63. doi: 10.1186/s12870-020-2273-1
Wei, T., Sun, Y., Yashir, N., Li, X., Guo, J., Liu, X., et al. (2022). Inoculation with rhizobacteria enhanced tolerance of tomato (Solanum lycopersicum L.) plants in response to cadmium stress. J. Plant Growth Regul. 41, 445–460. doi: 10.1007/s00344-021-10315-4
Wu, X., Chen, Q., Chen, L., Tian, F., Chen, X., Han, C., et al. (2022). A WRKY transcription factor, PyWRKY75, enhanced cadmium accumulation and tolerance in poplar. Ecotoxicol. Environ. Saf. 239:113630. doi: 10.1016/j.ecoenv.2022.113630
Xie, Y., Su, L., He, Z., Zhang, J., and Tang, Y. (2021). Selenium inhibits cadmium absorption and improves yield and quality of cherry tomato (Lycopersicon esculentum) under cadmium stress. J. Soil Sci. Plant Nutr. 21, 1125–1133. doi: 10.1007/s42729-021-00427-x
Xu, Z., Ge, Y., Zhang, W., Zhao, Y., and Yang, G. (2018). The walnut JrVHAG1 gene is involved in cadmium stress response through ABA-signal pathway and MYB transcription regulation. BMC Plant Biol. 18:19. doi: 10.1186/s12870-018-1231-7
Xu, Z. M., Tan, X. Q., Mei, X. Q., Li, Q. S., Zhou, C., Wang, L. L., et al. (2018). Low-cd tomato cultivars (Solanum lycopersicum L.) screened in non-saline soils also accumulated low cd, Zn, and cu in heavy metal-polluted saline soils. Environ. Sci. Pollut. Res. Int. 25, 27439–27450. doi: 10.1007/s11356-018-2776-6
Xu, Q., Wang, C., Li, S., Li, B., Li, Q., Chen, G., et al. (2017). Cadmium adsorption, chelation and compartmentalization limit root-to-shoot translocation of cadmium in rice (Oryza sativa L.). Environ. Sci. Pollut. Res. 24, 11319–11330. doi: 10.1007/s11356-017-8775-1
Yang, G., Fu, S., Huang, J., Li, L., Long, Y., Wei, Q., et al. (2021). The tonoplast-localized transporter OsABCC9 is involved in cadmium tolerance and accumulation in rice. Plant Sci. 307:110894. doi: 10.1016/j.plantsci.2021.110894
Yu, R., Li, D., Du, X., Xia, S., Liu, C., and Shi, G. (2017). Comparative transcriptome analysis reveals key cadmium transport-related genes in roots of two pak choi (Brassica rapa L. ssp. chinensis) cultivars. BMC Genomics 18:587. doi: 10.1186/s12864-017-3973-2
Zhang, A., Bian, R., Li, L., Wang, X., Zhao, Y., Hussain, Q., et al. (2015). Enhanced rice production but greatly reduced carbon emission following biochar amendment in a metal-polluted rice paddy. Environ. Sci. Pollut. Res. 22, 18977–18986. doi: 10.1007/s11356-015-4967-8
Zhang, F., Wan, X., Zheng, Y., Sun, L., Chen, Q., Guo, Y., et al. (2014). Physiological and related anthocyanin biosynthesis genes responses induced by cadmium stress in a new colored-leaf plant “Quanhong poplar”. Agrofor. Syst. 88, 343–355. doi: 10.1007/s10457-014-9687-4
Zhang, J., Zhu, Y., Yu, L., Yang, M., Zou, X., Yin, C., et al. (2022). Research advances in cadmium uptake, transport and resistance in rice (Oryza sativa L.). Cells 11:569. doi: 10.3390/cells11030569
Zhan, J., Zou, W., Li, S., Tang, J., Lu, X., Meng, L., et al. (2022). OsNAC15 regulates tolerance to zinc deficiency and cadmium by binding to OsZIP7 and OsZIP10 in rice. Int. J. Mol. Sci. 23:11771. doi: 10.3390/ijms231911771
Zhao, X., Luo, L., Cao, Y., Liu, Y., Li, Y., Wu, W., et al. (2018). Genome-wide association analysis and QTL mapping reveal the genetic control of cadmium accumulation in maize leaf. BMC Genomics 19:91. doi: 10.1186/s12864-017-4395-x
Zhao, L., Zhu, Y. H., Wang, M., Ma, L. G., Han, Y. G., Zhang, M. J., et al. (2021). Comparative transcriptome analysis of the hyperaccumulator plant Phytolacca americana in response to cadmium stress. Three Biotech 11:327. doi: 10.1007/s13205-021-02865-x
Keywords: tomato, cadmium stress, transcriptome, qRT-PCR, transcription factor
Citation: Chen L, Wu M, Jin W, Lei T, Li Y, Wu X and Fu S (2023) Gene identification and transcriptome analysis of cadmium stress in tomato. Front. Sustain. Food Syst. 7:1303753. doi: 10.3389/fsufs.2023.1303753
Received: 28 September 2023; Accepted: 27 October 2023;
Published: 14 November 2023.
Edited by:
Juan Han, Chinese Academy of Agricultural Sciences, ChinaReviewed by:
Sheng Qian, Huazhong Agricultural University, ChinaCopyright © 2023 Chen, Wu, Jin, Lei, Li, Wu and Fu. This is an open-access article distributed under the terms of the Creative Commons Attribution License (CC BY). The use, distribution or reproduction in other forums is permitted, provided the original author(s) and the copyright owner(s) are credited and that the original publication in this journal is cited, in accordance with accepted academic practice. No use, distribution or reproduction is permitted which does not comply with these terms.
*Correspondence: Shan Fu, c2hhbmZ1NzdAaGJudS5lZHUuY24=; Xuan Wu, d3V4dWFuNTg1QDE2My5jb20=
Disclaimer: All claims expressed in this article are solely those of the authors and do not necessarily represent those of their affiliated organizations, or those of the publisher, the editors and the reviewers. Any product that may be evaluated in this article or claim that may be made by its manufacturer is not guaranteed or endorsed by the publisher.
Research integrity at Frontiers
Learn more about the work of our research integrity team to safeguard the quality of each article we publish.