- 1School of Water Stress Management, Indian Agricultural Research Institute (ICAR)-National Institute of Abiotic Stress Management, Baramati, Maharashtra, India
- 2Division of Soil Chemistry & Fertility, Indian Agricultural Research Institute (ICAR)-Indian Institute of Soil Science, Bhopal, Madhya Pradesh, India
Sequestration of carbon (C) in arable cropping systems is considered one of the potential climate change mitigation strategies. In this context, assessing the potential of sugarcane cropping systems should be a priority, as it leaves substantial amounts of recyclable residues essential for maintaining soil organic carbon (SOC), improving soil health, and strengthening overall resources. We evaluated the impacts of residue retention and nutrient management practices on SOC and its pools, storage, soil biology, and yield in a multi-ratooning sugarcane system. A field experiment was conducted in the split-plot design with residue burning (RB) and residue retention (RR) as the main plot treatments and three nutrient management practices, that is, 25% of the recommended dose of fertilizers (RDF, i.e., 300:150:150 kg of N, P2O5, and K2O kg ha−1, respectively) as basal + 75% through fertigation (N1); 50% of RDF as basal + 50% through fertigation (N2); and 75% of RDF as basal + 25% through fertigation (N3) as subplot treatments in ratoon sugarcane. Soil samples were collected initially and after 6 years of multi-ratooning (one plant and four ratoon crops) from a soil depth of 0–30 cm. The results indicated that RR plots had 21% higher total SOC with 42, 47, 17, and 13% higher very labile, labile, less labile, and non-labile C pools, respectively, than RB plots (P < 0.05). RR also had a higher lability and recalcitrant index than RB. Of the total SOC stock, the contribution of passive pools was higher (72–75%) than active pools. Significantly higher dehydrogenase activity (DHA) (86%), alkaline phosphatase activity (APA) (16%), and ß-glucosidase activity (BGA) (22%) were observed in RR plots as compared to RB plots, whereas for nutrient management practices, it followed the order of N2 > N3> N1. Microbial counts also followed the same trend as that of enzyme activities. Residue retention practices reported higher C sequestration (0.68 Mg C ha−1 yr−1), carbon retention efficiency (37%), and yield (38%) with a potential to reduce GHG emissions by 2.72 Mg CO2 ha−1 yr−1 as compared to traditional practices. Residue retention and 50–75% RDF as basal is recommended for higher soil C retention and soil biology for sustained sugarcane productivity.
Introduction
Rising levels of atmospheric carbon dioxide (CO2) have drawn global attention to the increasing potential of soils to store carbon (C). For this reason, resource conservation technologies have been applied to different cropping systems (Das et al., 2013; Parihar et al., 2018; Datta et al., 2019; Jat S. L. et al., 2019; Somasundaram et al., 2019) where regular addition of crop residues was quite effective in C sequestration and enhancing nutrient availability. In addition to being the primary indicator of soil health, soil organic carbon (SOC) provides energy and substrates to promote biological diversity and ecosystem functionality (Wendling et al., 2010). In this context, assessing the potential of cropping systems with large biomass production ability, such as rice–wheat and sugarcane-based, should be a priority, though the former has been evaluated at large (Gathala et al., 2013; Singh and Sidhu, 2014; Choudhary et al., 2018; Jat H. S. et al., 2019; Singh et al., 2020; Datta et al., 2022). Sugarcane is a long-duration crop (12–18 months) with the possibility of taking multiple ratoons (Suman et al., 2009). It leaves substantial amounts of recyclable residues (8–10 t ha−1), which are essential for maintaining SOC, improving soil health, and strengthening overall resources. Moreover, the SOC stocks are comprised of labile or active pools and stable, passive, or recalcitrant pools with varying residence times. In general, labile C pools have rapid turnover rates (<5 years) and act as a source of food for soil microbes, thus influencing soil nutrient cycling and productivity, whereas passive C pools with longer stability help in higher C sequestration (Datta et al., 2018; Jat H. S. et al., 2019). However, further evaluation of the dynamics of sequestered C to assess the temporal fate of different pools should help in developing strategies to counter the challenges posed by climate change.
Declining soil health in many sugarcane-growing areas is also a major concern among farmers. In general, a sugarcane crop yielding 100 ton ha−1 removes approximately 200–250 kg of nitrogen, 120–150 kg of phosphorous, and 175–225 kg of potassium from the soil. In India, an estimated plant nutrient removal of 34 million tons is reported as against the estimated nutrient consumption of 18 million tons, thus indicating an erratic and imbalanced crop nutrient management (Yadav et al., 2019). This imbalanced management, in turn, has resulted in a decline in the average cane productivity of plant crops and ratoons to 78–90 and 48–60 Mg ha−1, respectively, over the last two decades. A normal yielding sugarcane crop (100 Mg ha−1) produces 8–10 Mg of recyclable residues in the form of dried leaves (trash), root biomass, and stubbles, in addition to rhizodeposition, which are essential for maintaining SOC (Suman et al., 2009). However, residue burning either before or after harvest is a common practice among farmers (Bhuvaneshwari et al., 2019; Dutta et al., 2022), which not only results in losses of surface organic matter, SOC, essential nutrients, soil enzymatic activities, and soil microflora but also causes environmental pollution. If returned to the soil, these residues will enrich the soil with nutrients and organic C. Later, these residues also play a major role in diversified and abundant microbial populations that act as agents of transformation of soil organic matter, nutrient cycling, and energy flow among other ecosystem functions (Six et al., 2002). Soil enzyme activity is one of the potential indicators of soil health due to its rapid response to management changes and environmental factors (Mohammadi, 2011). Changes in residue recycling and nutrient management practices induce significant changes in the quantity and quality of plant residue entering into the soil, their seasonal and spatial distribution, the ratio between shoot and root inputs, and nutrient dynamics, all of which influence soil microorganisms and soil microbial processes (Govaerts et al., 2009). In this context, the present study was conducted to assess the effect of different residue and nutrient management practices on the SOC, its pools, soil biology, and yield in a multi-ratooning system of sugarcane in black clayey soils under hot semi-arid regions. The specific objectives were as follows: (i) to assess the impacts of residue and nutrient management practices on total SOC stock, size of its pools and their dynamics, soil enzymatic activity, microbial count, and yield, and (ii) to study the effect of C addition on SOC storage in black clayey soils of hot semi-arid regions.
Materials and methods
Experimental site, climate, and soil
A field experiment was conducted for 6 consecutive years (2016–2022) at a research farm (18°09′30.62″ N, 74°30′ 03.08″E and 570 m above mean sea level) of ICAR–National Institute of Abiotic Stress Management (NIASM), Baramati, Maharashtra, India (Figure 1). The site is part of a hot semi-arid agro-ecological region (AER-6) in the Deccan Plateau of India and is characterized with extremely high temperatures, erratic rainfalls, and prolonged dry spells. The long-term average annual rainfall is 576 mm, which is restricted to the southwest and retreating monsoon. The contribution of southwest monsoon (June–September) and post-monsoon (October–December) rainfall is approximately 70 and 21%, respectively. The maximum and minimum temperatures, the maximum and minimum values of relative humidity, and average values for rainfall and sunshine hours during the six consecutive study periods were 32.8°C and 18.8°C, 70 and 42%, 617 mm, and 6.5 h, respectively (ICAR–NIASM Agromet Observatory). The soil type at the study site is black with a clayey texture (13% sand, 20% silt, and 67% clay), which was determined according to the International Pipette Method (Baruah and Barthakur, 1999). The pH (soil: water 1: 2.5) and electrical conductivity (EC) were calculated following standard methods, and the values were 8.1 and 0.24 dS m−1, respectively (Jackson, 1973). The bulk density (BD) (1.28 Mg m−3) and soil organic carbon (0.58%) were determined using a core sampler (Blake and Hartge, 1986) and wet oxidation method (Walkley and Black, 1934), respectively.
Experimental layout, soil, and crop management
The experiment was laid out in a split-plot design comprised of two residue management practices (RB: residue burning and RR: residue retention) in main plots and three nutrient management practices (N1: 25% recommended dose of fertilizer [RDF i.e., 300: 150:150 kg of N, P2O5, and K2O kg ha−1, respectively] as basal + 75% by fertigation; N2: 50% RDF as basal + 50% by fertigation; and N3: 75% RDF as basal + 25% by fertigation) in sub plots having three replications. The experiment was initiated on July 2016 using the sugarcane variety MS−10001, which was transplanted at 120 × 60 cm spacing. The gross and net plot area under each treatment was 10 × 4 m and 8.5 × 3.5 m, respectively. The fresh crop of sugarcane was harvested in January 2018 (18 months), whereas subsequent four ratoon crops were harvested in January of each year until 2022 (Figure 2). The irrigation method applied was through drip, and all other recommended crop production practices required for the crop (weeding, earthing up, and crop protection measures) were followed. The cultivation procedures including planting and harvesting time, tillage, residue, and nutrient management schedules are described in Table 1.
Biomass and yield measurement
At harvest of plant and ratoon crop, quadrats of 2 × 2 m were selected in each plot for estimating above-ground biomass, with each quadrat comprising dried leaves, green tops, and leaf sheaths, which were chopped, homogenized, and dried at 70°C to obtain a constant weight. Contribution of biomass through the fall of dry leaves (trash) during crop growth in residue retention plots was accounted to be approximately 3.7% of harvested above-ground biomass (Suman et al., 2009). A root:shoot ratio of 0.30 (Suman et al., 2009) and rhizodeposition constituting approximately 0.15 g g−1 of the above-ground biomass (Bronson et al., 1988) were assumed for the calculation of below-ground biomass contribution. The C input into the soil from shoots, leaves, and roots was calculated by assuming 40% C present in various plant parts (Dubey and Lal, 2009). The contribution of weed biomass to SOC was found to be negligible as they were removed periodically across all the treatments. Following the procedures as stated above, an estimate of plant-derived C inputs into the soils was made (Table 2).
The mean annual input of organic biomass/residues to soil from sugarcane crops varied with aboveground yield responses of the crop and the type of treatment. Hence, there was a yearly variation in the total amounts of added residues under different treatments (Table 2). A higher amount of gross C input through crop biomass was reported in residue retention plots, ranging from 10.30 to 10.82 Mg ha−1 yr−1, whereas plots with residue burning had an annual C input ranging from 3.32 to 3.62 Mg ha−1 yr−1, which was contributed by roots and rhizodeposition.
The crops were harvested from the net plot (8.5 × 3.5 m) for yield assessment, leaving two border rows. The cane yield under each treatment was recorded for all the study years and then averaged for 6 years to represent the effect of residue and nutrient management practices on overall cane yield.
Soil sampling, processing, and analysis
Replicated soil samples (4 sub-replications from each replicated field × 6 replications × 6 treatments) were collected from a soil depth of 0–30 cm using a soil core sampler (10 cm height; 7 cm diameter; and 385 cm3 volume) after completion of one fresh and four ratoon cycles of sugarcane during the 1st week of February 2022. One part of the samples was air-dried in shade, passed through a 2-mm sieve, and used for the analysis of soil organic carbon and its pools. The other part of the fresh soil sample was kept undisturbed in polyethylene bags at 4°C and used for soil biochemical analysis. The wet digestion method was used to estimate the organic carbon content of soil samples (Walkley and Black, 1934). Soil organic carbon (SOC) stock was calculated using Equation (1).
Oxidizable SOC, its pools, and mineralizable C
Different pools of SOC with varying lability were estimated following the modified Walkley and Black method as described by Chan et al. (2001), which allowed partitioning of total SOC into four different pools of decreasing oxidizability as described below.
Very labile (VL) pool: organic C oxidizable by 12 N H2SO4.
Labile (L) pool: the difference in organic C oxidizable by 18 N and that under 12 N H2SO4.
Less labile (LL) pool: the difference in organic C oxidizable by 24 N and that under 18 N H2SO4.
Non-labile (NL) pool: the difference in total SOC and organic C oxidizable under 24 N H2SO4.
Total SOC was estimated by the modified wet oxidation method as described by Nelson and Sommers (1982).
All four fractions were grouped into active pools (very labile and labile pools) and passive pools (less labile and non-labile) of organic C in soil (Datta et al., 2015). Similarly, the lability index (LI) for SOC was calculated by first expressing the amounts of each of the three labile pools, namely, VL, L, and LL as the fraction of the amount of total carbon and then multiplying the fractions with their respective weightages of 3, 2, and 1 based on their ease of oxidation followed by their addition (Datta et al., 2015). The LI was computed using Equation 2. The values obtained represent the relative performance of different treatments in maintaining labile soil organic C at different depths. Further recalcitrant index (RI) of SOC was derived in two ways following Equations (3) and (4) given by Datta et al. (2017).
where VL, very labile C; L, labile C; LL, less labile C; NL, non-labile C; SOC, total soil organic C.
Mineralizable C of soil from the 0–30 cm soil depth under different treatments was estimated by the CO2-C evolution method. The amount of CO2 evolved during the 30-day incubation period was absorbed in 10 ml of 0.5 N NaOH solutions. Evolved CO2 was estimated by titrating the alkali in the traps with 0.5 N HCl using a phenolphthalein indicator (Anderson, 1982).
Soil microbial count and enzyme activity
Estimation of different functional groups of microbes in each treatment was performed using the serial dilution technique and standard plate counts. The total bacterial count was done using a nutrient agar medium (Zuberer, 1994), whereas Rose Bengal and Actinomycetes isolation agar media were used for the count of total fungus and actinomycetes counts, respectively (Martin, 1950; Himedia Manual, 2009). Data from triplicate readings were expressed as colony-forming units (CFU) g−1 dry soil. Dehydrogenase activity (DHA) was determined from the conversion of 2, 3, 5-triphenyl tetrazolium chloride (TTC) to triphenyl formazan (TPF) over a 24-h period (Dick et al., 1996). Alkaline phosphatase activity (APA) was determined as described by Dick et al. (1996) and is expressed as micrograms of p-nitrophenol formed per gram of oven-dried soil. β-glucosidase activity was determined by the method of Eivazi and Tabatabai (1988).
Carbon sequestration and carbon retention efficiency
The carbon sequestration rate was calculated using Equation 5 (Kumara et al., 2014) as follows:
where final and initial SOC stock represent SOC (Mg C ha−1) in the final and initial soils, respectively, and duration is the study period in years.
Carbon retention efficiency (CRE) was calculated by following Equation 6 given by Bhattacharyya et al. (2009).
where final and initial SOC stock represent SOC (Mg C ha−1) in the final and initial soils, respectively, and cumulative C input is the total estimated C input (Mg C ha−1) to the soil between the initial and final year of experimentation, as depicted in Table 2.
Statistical analysis
The recorded data were statistically analyzed using analysis of variance for split-plot design using the “Agricolae” package of R (R Development Core Team, 2015). A mixed model was used considering residue as the main plot factor and nutrient management as the subplot factor and analyzed in a split-plot design. The F-test and least significant difference (LSD) (P < 0.05) were used to decipher the significance of the means of residue and nutrient management practices and their interactions. A correlation matrix was constructed among different soil parameters and yields using the same statistical package.
The carbon mineralization data were fitted with a first-order exponential model expressed as:
where Y = cumulative CO2-C emission (μg of CO2-C g−1 of soil), C0 = potentially mineralizable C (μg of CO2-C g−1 of soil), k = decomposition rate constant (day−1), and t = time of incubation (days). The model was evaluated using the goodness-of-fit test through the estimation of root mean square error (RMSE) and the Nash–Sutcliffe model efficiency coefficient (Ef) (Datta et al., 2019; Govindasamy et al., 2021). Furthermore, model significance was tested using the sum of square reduction test as described in the study of Govindasamy et al. (2021). The cumulative C-mineralization data after eliminating the CO2-C values during the initial 3 days of incubation (as they accounted for nearly 50% of the total mineralizable carbon) was fit to a linear model, that is,
where Y = cumulative CO2-C emission (μg of CO2-C g−1 of soil), Y0 is the overall intercept, b is the slope, and x is time (days).
Results
Total soil organic carbon, its pools, lability, and recalcitrant indices
The total SOC was increased by 21% in residue retention plots as compared to residue-burning plots (21.97 Mg C ha−1) from a 0–30 cm surface soil (P < 0.05) (Figure 3). Significant variations existed in different pools of SOC values ranging between 3.43 and 4.87, 1.76 and 2.58, 2.52 and 2.95, and 14.26 and 16.15 Mg C ha−1 for very labile, labile, less labile, and non-labile C pools, respectively. Plots with residue retention had 42, 47, 17, and 13% higher very labile, labile, less labile, and non-labile C pools, respectively, as compared with residue burning. However, nutrient management practices had a comparable effect on SOC and its pools. Furthermore, residue retained plots had 44 and 14% higher active and passive pools of C, that is, 5.19 and 16.78 Mg C ha−1, respectively, than RB plots. On average, active and passive pools contributed approximately 25–28% and 72–75% to the total SOC, respectively.
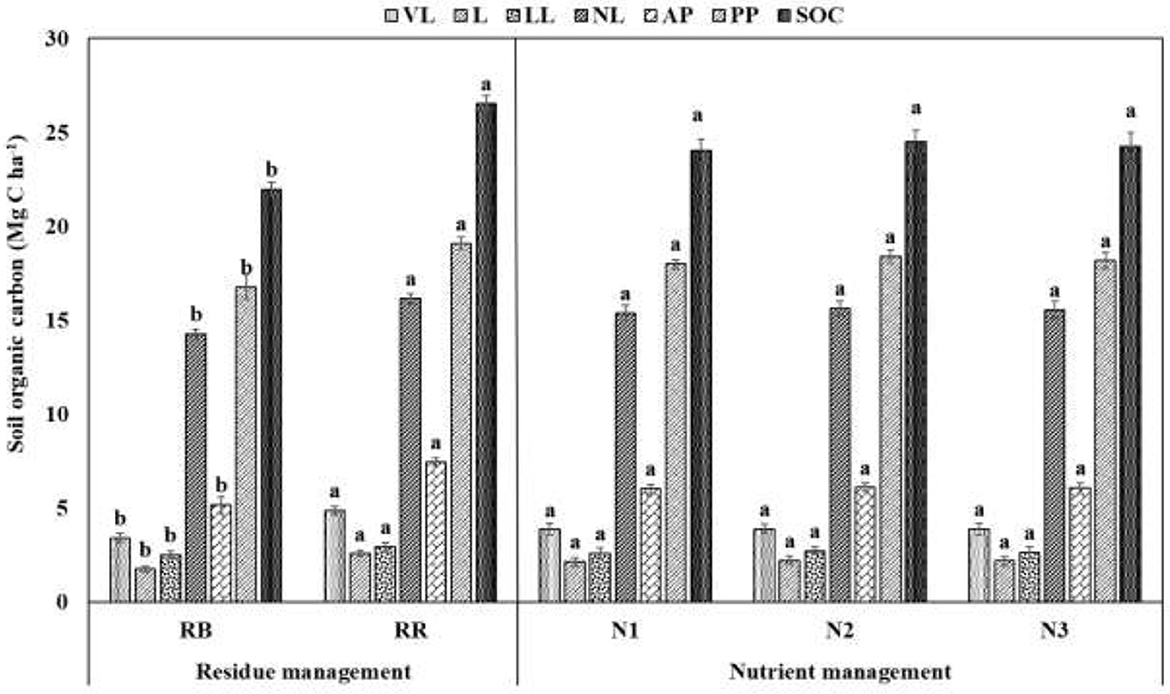
Figure 3. Effect of residue and nutrient management on the distribution of SOC (Mg ha−1) into different pools of oxidizability in the 0–30 cm surface soil. Values with different lowercase letters are significantly different between the treatment levels (Tukey's HSD test for mean separation at p = 0.05). Vertical bars represent S.E. ± mean of the observed values. VL, very labile C; L, labile C; LL, less labile C; NL, non-labile C; AP, active pool; PP, passive pool; SOC, soil organic carbon; RB, residue burning; RR, residue retention; N1: 25% recommended dose of fertilizer (RDF) as basal + 75% by fertigation; N2: 50% RDF as basal + 50% by fertigation; and N3: 75% RDF as basal + 25% by fertigation.
The lability index (LI) was higher for RR plots than RB plots, whereas it was almost similar for all the nutrient management treatments (Table 3). The recalcitrant index 1 (RI1) was higher for RR (3.05) than RB (2.60), indicating the stability of SOC under residue retention. Recalcitrant index 2 (RI2) remained unaffected by various treatments (Table 3).
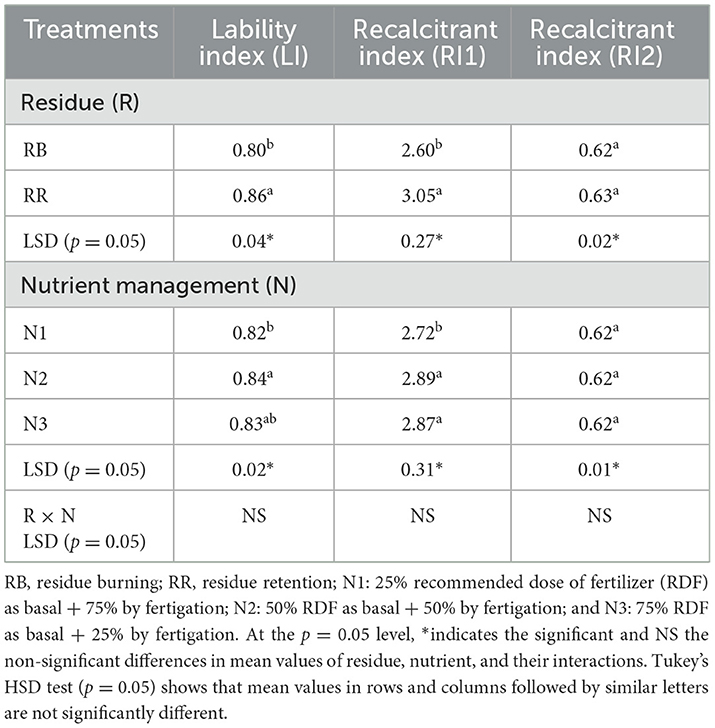
Table 3. Lability index (LI) and recalcitrant indices (RIs) of soil organic carbon as affected by residue and nutrient management practices.
Mineralizable carbon
The cumulative C mineralization in the 0–30 cm surface soil over 30 days was significantly affected by residue management practices (Figure 4, Table 4). The C mineralization data were well fitted to a first-order exponential model (RMSE value = 6–15 and Ef = 0.80–0.83) (Table 4). The model indicated that the total mineralizable carbon at the 0–30 cm surface soil was higher in RR plots (619.30 μg of CO2-C g−1 of soil) than in RB plots (226.93 μg of CO2-C g−1 of soil). The mineralization rate was highest during the initial 3 days of incubation and decreased gradually with the progress of incubation time, irrespective of the treatments (Figure 4). The exclusion of the initial 3 days of mineralizable C values from the cumulative data provided a better fit to a linear model with R2 values of 0.93 and 0.94 for RB and RR, respectively (Figure 5).
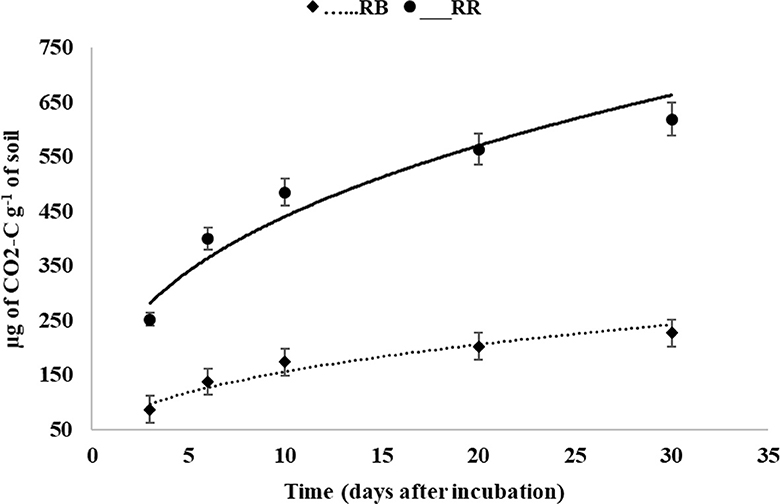
Figure 4. Carbon mineralization (μg of CO2-C g−1 of soil) as affected by residue management in the 0–30 cm surface soil (RB, residue burning; RR, residue retention).
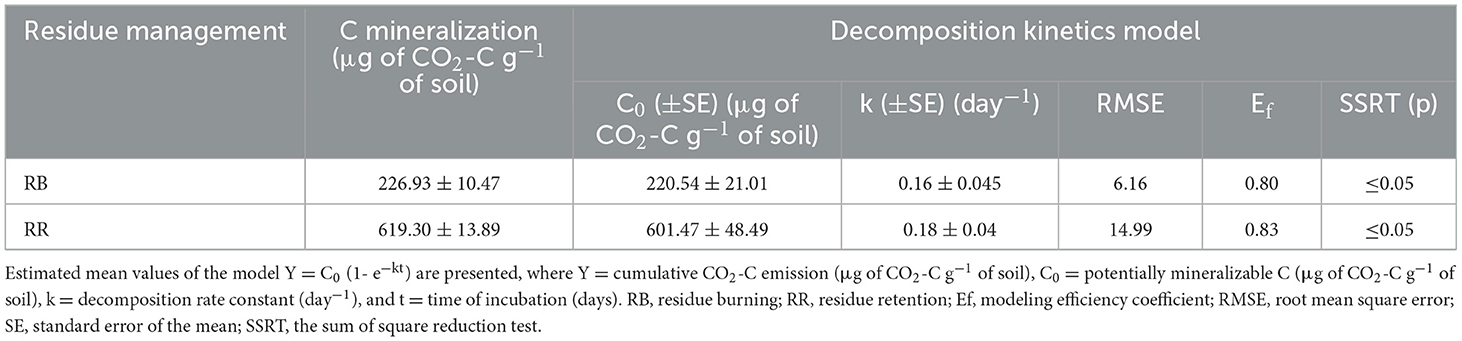
Table 4. Parameter estimates and model goodness-of-fit for the first-order exponential function fitted to the cumulative carbon-mineralization data under residue management in multi-ratoons of sugarcane in the 0–30 cm surface soil.
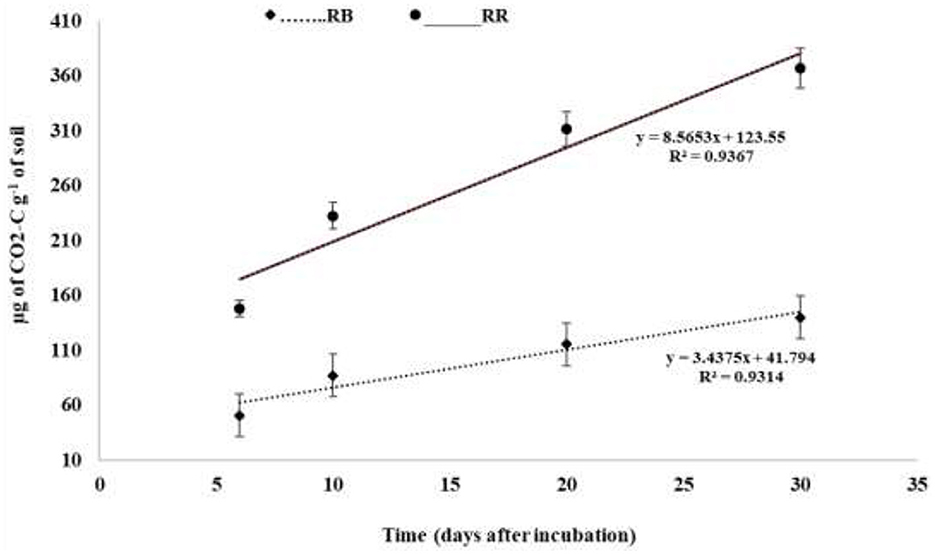
Figure 5. Carbon mineralization (μg of CO2-C g−1 of soil) as affected by residue management in the 0–30 cm surface soil [linear model Y = Y0 + b*x, where Y = cumulative CO2-C emission (μg of CO2-C g−1 of soil), Y0 is the overall intercept, b is the slope, and x is time (days)]. RB, residue burning; RR, residue retention.
Soil enzymes
Soil dehydrogenase activity (DHA), alkaline phosphatase activity (APA), and ß-glucosidase activity (BGA) were significantly affected by residue and nutrient management treatments (Table 5). Plots with residue retention had 86, 16, and 22% higher DHA, APA, and BGA, respectively, than residue-burning plots. For nutrient management practices, the enzyme activities followed the order: N2 > N3 > N1. DHA, APA, and BGA improved by 82, 14, and 26% under N2, and 67, 11, and 19% under N3, respectively, compared to N1.
Microbial population
The microbial population, namely, bacteria, fungi, and actinomycetes, were significantly affected by residue and nutrient management treatments (Table 6). The population of bacteria was higher compared to fungi and actinomycetes irrespective of the treatments. Compared to RB, the counts of bacteria, fungi, and actinomycetes were 30, 31, and 21%, respectively, higher in RR. The treatments having application of >50% of RDF as basal (N2 and N3) had 8, 23, and 5% higher populations of bacteria, fungi, and actinomycetes, respectively, as compared to N1.
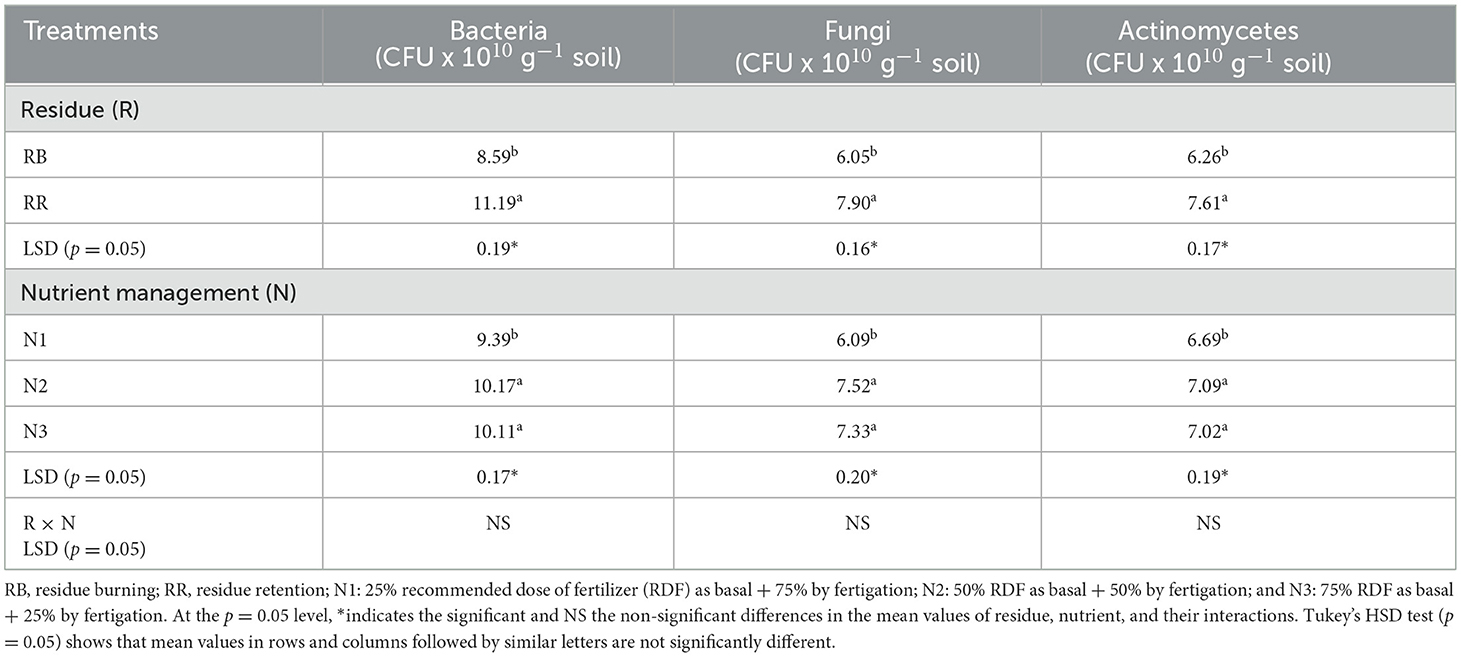
Table 6. Effect of residue and nutrient management on soil microbial population in 0–30 cm surface soil layers.
Soil carbon sequestration, carbon retention efficiency, and its environmental implications
During the 6 years of study, the total amount of C added through crop residues (root, rhizodeposition, and above-ground biomass) was 11–12 Mg C ha−1 for residue retention plots, whereas it ranged between 3.4 and 3.8 Mg C ha−1 for residue-burning plots (only through root and rhizodeposition) (Table 7). A build-up in SOC was observed with RR (4.05 Mg C ha−1), but it declined in RB plots (−1.06 Mg C ha−1) (Table 6). Furthermore, N2 plots resulted in 20 and 43% higher rates of C sequestration than N3 and N1, respectively.
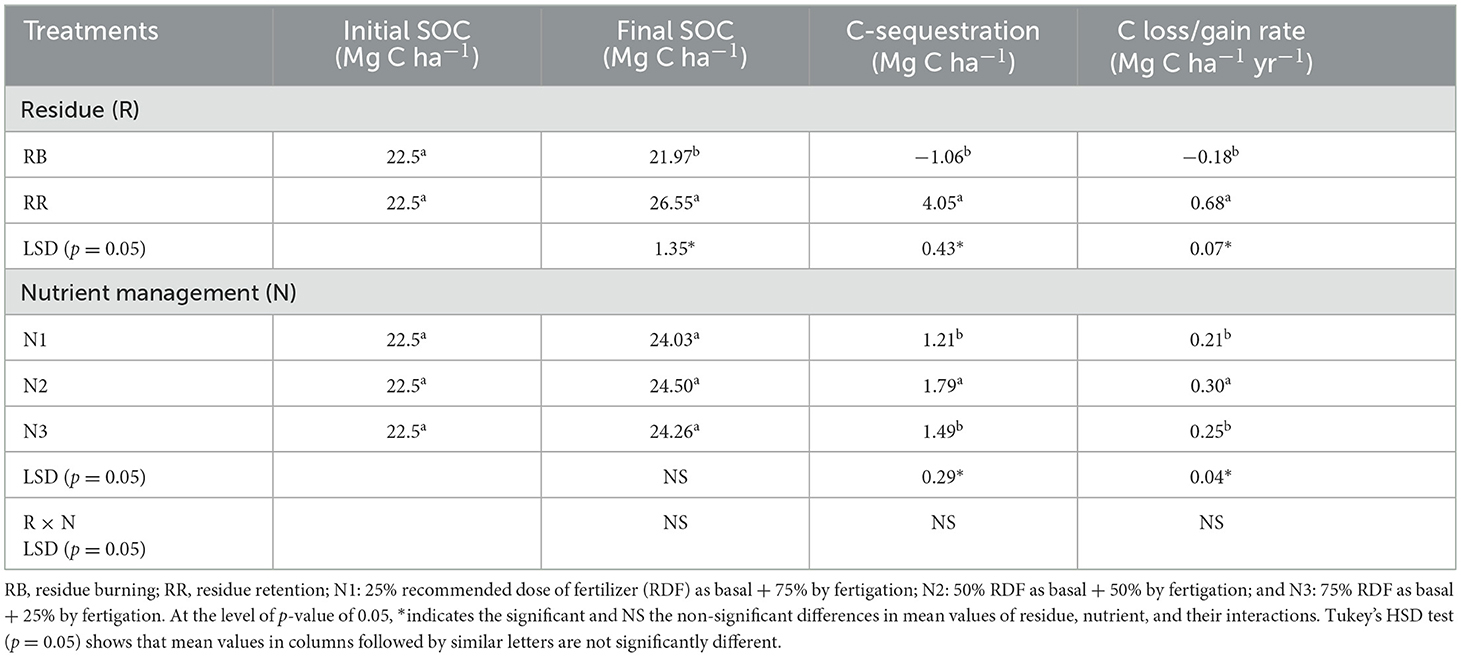
Table 7. Impact of treatments on soil organic carbon sequestration at a surface soil depth of 0–30 cm.
Soil carbon retention efficiency (CRE) varied between 11 and 37% among the treatments (Figure 6). Highest CRE was observed under RR-N2 followed by RR-N3 ≥ RR-N1 > RB-N2 > RB-N3 > RB-N1. Understandably, there was a loss in CRE of up to 18% with residue burning.
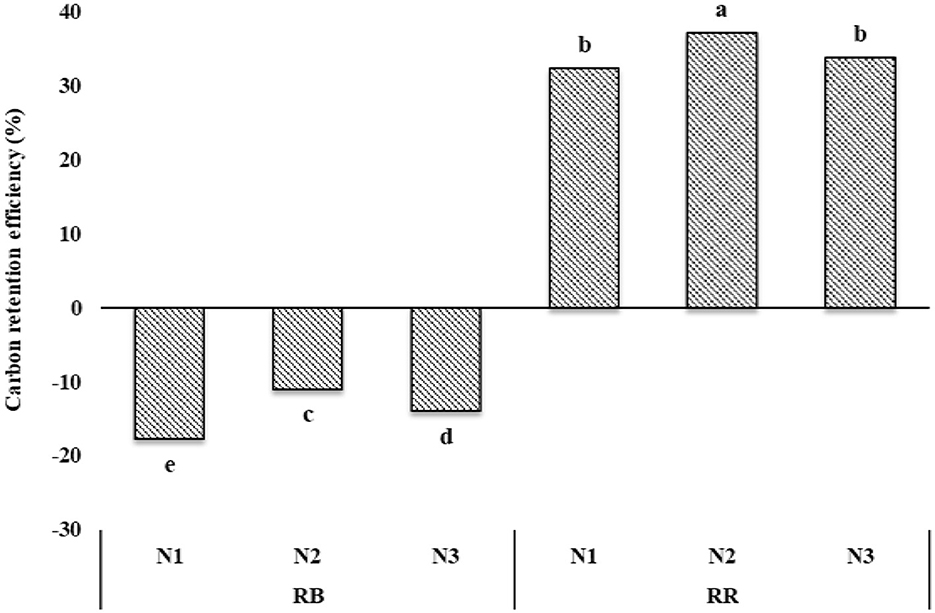
Figure 6. Carbon retention efficiency as affected by residue and nutrient management practices. RB, residue burning; RR, residue retention; N1: 25% recommended dose of fertilizer (RDF) as basal + 75% by fertigation; N2: 50% RDF as basal + 50% by fertigation; and N3: 75% RDF as basal + 25% by fertigation; bars with different lowercase letters are significantly different among the treatments for Tukey's HSD test (p = 0.05).
Sugarcane yield and its relationship with the soil properties
Sugarcane yield averaged over 6 years of cultivation (2016–22) was affected significantly by residue and nutrient management practices (Figure 7). Plots with RR had 38% higher cane yield than RB (93.12 Mg ha−1), whereas plots with N2 and N3 produced 9–10% higher cane yield (i.e., 128.24 and 126.74 Mg ha−1, respectively) than N1 (116.21 Mg ha−1).
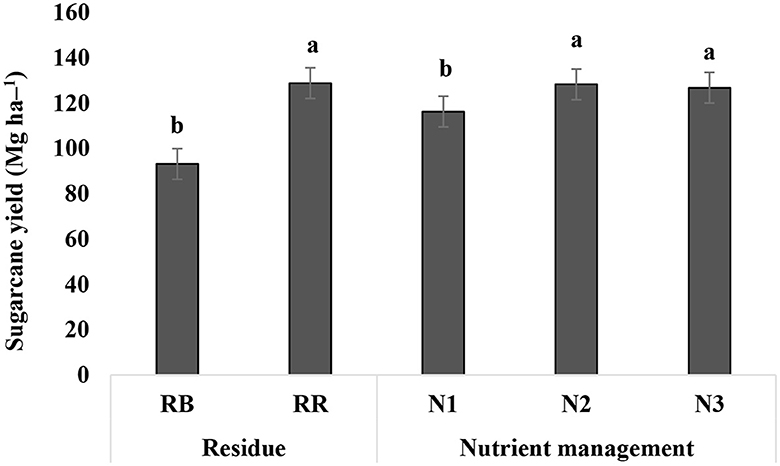
Figure 7. Sugarcane yield under different residue and nutrient management practices in 6 years (mean of 6 years). RB, residue burning; RR, residue retention; N1: 25% recommended dose of fertilizer (RDF) as basal + 75% by fertigation; N2: 50% RDF as basal + 50% by fertigation; and N3: 75% RDF as basal + 25% by fertigation; bars with different lowercase letters are significantly different among the treatments for Tukey's HSD test (p = 0.05).
Most of the C pools and biological soil properties showed significant correlations (P < 0.01 and P < 0.05) among each other (Table 8). Very labile and labile pools were significantly correlated with active C pool (r = 0.99, p < 0.01 and r = 0.94, p < 0.01), whereas less labile and non-labile pools were significantly positively correlated with passive C pool (r = 0.65, p < 0.01 and r = 0.81, p < 0.01), respectively. SOC significantly positively correlated with very labile (r = 0.70, p < 0.01), non-labile (r = 0.91, p < 0.01), active (r = 0.61, p < 0.01), and passive (r = 0.88, p < 0.01) pools of C. All the enzymes, namely, DHA, APA, and BGA, were significantly and positively correlated with each other (P < 0.01). Mineralizable C was significantly positively correlated with very labile, labile, and active pools of C (r = 0.94, p < 0.01; r = 0.92, p < 0.01; and r= 0.89, p < 0.01, respectively). Sugarcane yield had significantly positive correlation with labile pool (r = 0.68, p < 0.05), active pool (r = 0.70, p < 0.05), DHA (r = 0.88, p < 0.01), APA (r = 0.93, p < 0.01), BGA (r = 0.93, p < 0.01), bacteria (r = 0.96, p < 0.01), fungi (r = 0.69, p < 0.05), and actinomycetes (r = 0.87, p < 0.05), respectively.
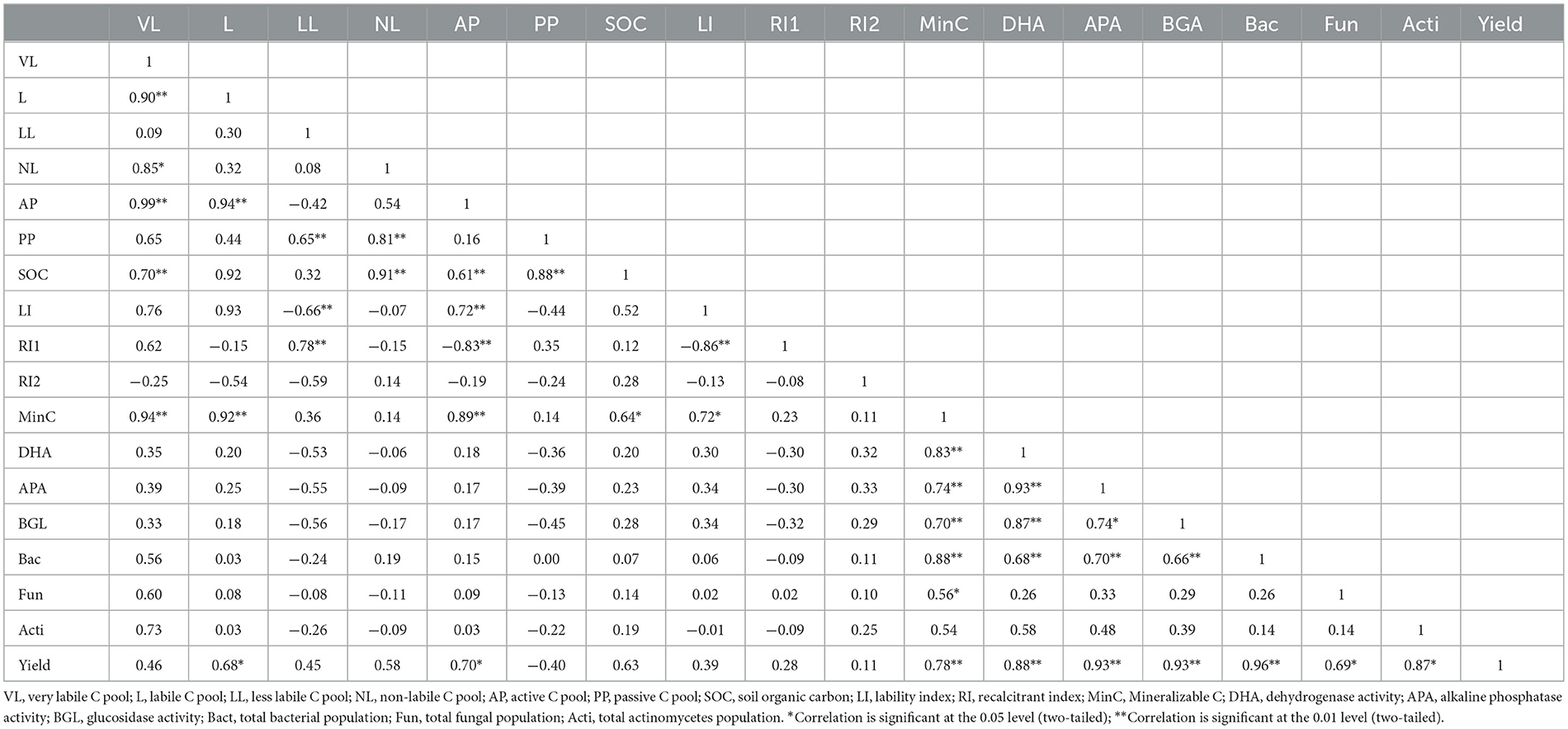
Table 8. Pearson's correlations among the soil C pools and biological properties with crop yield irrespective of scenarios.
Discussion
Crop residues are a prerequisite for the SOC pool. Therefore, retaining more crop residues in the soil is closely related to an increase in SOC concentration (Dolan et al., 2006). Our results of residue retention plots having higher total SOC as well as increased C pool sizes are in line with the findings of Razafimbelo et al. (2006), Galdos et al. (2009), and Tenelli et al. (2019). Active C pools (very labile and labile) serve as readily available food for microbial communities governing nutrient cycling processes in soils. In contrast, passive C (less labile and non-labile) pools are relatively resistant to decomposition and thus contribute to long-term C sequestration. Similar contributions of the two pools were earlier reported by Majumder et al. (2008), Datta et al. (2018), and Somasundaram et al. (2019). High contents of C in the passive pool are ascribed to the higher surface area of black clayey soils that safeguard its degradation (Datta et al., 2018). Additionally, high lignin contents of sugarcane trash appear to increase the half-life of the passive pool. Higher quantity of residue additions and their slow decomposition due to lower soil disturbance for ratoon crops and quantities of trash being high are the other reasons for higher SOC as well as the different pools (Saha et al., 2008; Dikgwatlhe et al., 2014; Jat H. S. et al., 2019). Higher LI in RR might be due to higher decomposable carbon, which is also manifested in higher AP and labile pools of C, as previously reported by Fang et al. (2005) and Datta et al. (2017, 2018). Indeed, the higher quantities of passive C pool than the active ones resulted in increased values of RI1 in RR (Datta et al., 2017). The nutrient management practice had no effect on SOC and its pools, which might be due to the relatively short duration of the experiment but was significantly associated with soil enzymes and microbial population. The higher rate of CO2 flux in the first few days after incubation was due to the rapid decomposition of labile carbon and increased agitation of microbes at the time of sampling, resulting in a greater availability of metabolically accessible compounds (Saggar et al., 2001). Consistent with the results of many studies, residue retention significantly increased mineralizable C at all incubation stages, as residue organic C can greatly promote microbial growth and activity (Cheng et al., 2014; Zang et al., 2016). Further application of 50–75% RDF as basal might have facilitated the decomposition of crop residues in the soil (Liang et al., 2022).
The enzyme activity usually becomes enhanced with the availability of labile carbon and nitrogen to the microbes. A higher basal dose of fertilizer appears to cause a surge in soil mineral N at the initial crop stage. On the contrary, the retention of residues with a high C:N ratio (100:1 here) results in temporary N immobilization (Oliveira et al., 2017). This immobilization increases competition for the available N between plant roots and soil microorganisms (Jingguo and Bakken, 1997). Thus, higher basal dose appears to have helped to overcome this situation by boosting both the initial root growth and microbial growth. Fertigation with the remaining half nutrients subsequently improved the synchrony between the nutrient demands of the crop and microbial populations (Gehl et al., 2005). Soil enzyme activities have been reported to be highly related to the amount of substrate (organic matter) available for microbial growth (Chandra, 2011; Wang et al., 2011; Stott et al., 2013). Higher microbial activities, in turn, create a positive “rhizosphere effect” through the release of organic substance, thereby resulting in higher enzyme secretion in soil (Roldan et al., 2005). Similar findings were also reported by Choudhary et al. (2018), Jat H. S. et al. (2019), and Roy et al. (2022). Furthermore, the residues acted as a food source and, when coupled with nutrient management, influenced the C:N ratio for increasing the activity of microbes. Increased microbial population by providing stimulating substrates through residue retention or incorporation was reported earlier (Ghimire et al., 2014; Choudhary et al., 2018; Jat H. S. et al., 2019). The application of more than half a dose of nutrients as basal and the rest through fertigation maintains their availability as per the demand of both the plants and microorganisms during crop growth (Awale et al., 2013; Jat S. L. et al., 2019). This strategy further ensures sufficient soil nutrients, especially N, for optimizing the residue decomposition and, in turn, the build-up of stable pools of SOC (Cotrufo et al., 2013).
Similarly, residue retention plots exhibited a higher rate of C sequestration (0.68 Mg C ha−1 yr−1) than other treatments, which might be attributed to a higher amount of above- and below-ground crop residue C inputs left over the soils, which subsequently became a part of SOC. Similar results on crop residue retention in paddy–wheat cropping systems have been reported by Das et al. (2013), Datta et al. (2015), Jat H. S. et al. (2019), and Roy et al. (2022). This effect was also reflected in increased CRE in residue-retained plots after 6 years of the experimental period. In general, sugarcane trash burning in India leads to emissions of CO2, CO, SOX, NOx, NH3, and particulate matter, which have been estimated at 28.23, 1.7, 0.01, 0.05, 0.02, and 0.24 Mt, respectively (Jain et al., 2014). As the increase in 1 Mg C retention in soil equals a decrease in 3.67 Mg CO2 released into the atmosphere, the residue retention with nutrient management has the potential to lower CO2 emissions by 2.72 Mg CO2 ha−1 yr−1, as compared to traditional practices.
Higher sugarcane yield under residue retention and more than 50% RDF as basal application might be attributed to higher carbon mineralization, better nutrient availability, and improved soil biological properties. Similar reports of higher system yield under crop residue C inputs through residue retention and proper nutrient management practices were also reported by Majumder et al. (2008), Surendran et al. (2016), Datta et al. (2018), and Jat et al. (2018).
Most of the C pools in the soil were related to each other, indicating the existence of a dynamic equilibrium between these pools. Thus, a practice that causes the depletion or enrichment in one pool would influence the equilibrium and affect the size of others. The high correlation values, particularly between active pools and labile pools and between non-labile and less labile pools, indicate their active participation in C dynamics of soils. Labile C and active pools C as well as enzyme and microbial population were significantly correlated with sugarcane yield, suggesting their contribution to crop growth and thus cane yield. Overall, it can be stated that the C inputs and C pools influence the yield of the crops, possibly through maintaining better soil health, and therefore, proper nutrient management practices are essential for the maintenance of the SOC level and sustainability of the production systems.
Conclusion
Residue retention practices during 6 years of sugarcane cultivation increased the cane yield by 38% compared to the farmer's practice of burning trash. Furthermore, soil organic carbon stocks, especially the non-labile pools, were further improved, and the C sequestration rate was estimated to be 0.68 Mg C ha−1 yr−1, while there was a net loss of 0.18 Mg C ha−1 yr−1 under farmer's practice. Thus, the practice of residue retention could lower CO2 emissions by 2.72 Mg CO2 ha−1 yr−1. Increasing the basal dose of nutrients to 50–75% of the recommended fertilizers improved the cane yield by 8–10%. Positive effects of residue retention and nutrient management were attributed to improvements in microbial populations and enzymatic activities. Sustainable agricultural residue management has become a major concern, particularly for developing countries such as India, which has a growing population, production rates, and economic growth. Due to a lack of technical awareness and adequate disposal options, small-scale farmers, in particular, resort to agricultural residue burning as an economical alternative. However, any solution involving long-haul transportation, expensive technology, or high capital investment is less likely to succeed. Sustainable solutions that involve the methods of in situ management of crop residues show better promise to be successful. Thus, the practices of residue retention and proper nutrient management have the potential for long-term sustainability of sugarcane cultivation (in an area of 27 m ha including India) in view of declining soil fertility and forecasted global warming with climate change.
Data availability statement
The original contributions presented in the study are included in the article/supplementary material, further inquiries can be directed to the corresponding author.
Author contributions
AP: Writing—original draft, Writing—review & editing. GW: Project administration, Supervision, Writing—review & editing. DS: Formal analysis, Writing—review & editing. PM: Conceptualization, Funding acquisition, Project administration, Resources, Visualization, Writing—review & editing. AB: Funding acquisition, Project administration, Supervision, Writing—review & editing. KR: Project administration, Supervision, Writing—review & editing.
Funding
The author(s) declare financial support was received for the research, authorship, and/or publication of this article. The study was conducted as a part of ICAR research program on Agri–Consortia Research Platform Project on Conservation Agriculture (CRPCA) (Grant No. 1007382). The authors also acknowledge the funding and technical support received from ICAR–IISS, Bhopal and ICAR–NIASM, Baramati.
Conflict of interest
The authors declare that the research was conducted in the absence of any commercial or financial relationships that could be construed as a potential conflict of interest.
Publisher's note
All claims expressed in this article are solely those of the authors and do not necessarily represent those of their affiliated organizations, or those of the publisher, the editors and the reviewers. Any product that may be evaluated in this article, or claim that may be made by its manufacturer, is not guaranteed or endorsed by the publisher.
References
Anderson, J. E. E. (1982). “Soil respiration,” in Methods of Soil Analysis, part 2, Chemical and Microbiological Properties (Madison, Wisconsin: American Society of Agronomy), 831–871. doi: 10.2134/agronmonogr9.2.2ed.c41
Awale, R., Chatterjee, A., and Franzen, D. (2013). Tillage and N–fertilizer influences on selected organic carbon fractions in a North Dakota silty clay soil. Soil Tillage Res. 134, 213–222. doi: 10.1016/j.still.2013.08.006
Baruah, T. C., and Barthakur, H. P. (1999). A Text Book of Soil Analysis. New Delhi: Vikas Publishing Houses Pvt. Ltd.
Bhattacharyya, R., Ved–Prakash Pandey, S. C., Kundu, S., Srivastva, A. K., and Gupta, H. S. (2009). Effect of fertilization on carbon sequestration in soybean–wheat rotation under two contrasting soils and management practices in the Indian Himalayas. Aus. J. Soil Res. 47, 592–601. doi: 10.1071/SR08236
Bhuvaneshwari, S., Hettiarachchi, H., and Meegoda, J. N. (2019). Crop Residue Burning in India: Policy Challenges and Potential Solutions. Int. J. Environ. Res. Public Health. 16, 832. doi: 10.3390/ijerph16050832
Blake, G. R., and Hartge, K. H. (1986). “Bulk density,” in Methods of Soil Analysis, Part I. Physical and Mineralogical Methods, ed. A. Klute (Madison, Wisconsin: American Society of Agronomy), 363–375. doi: 10.2136/sssabookser5.1.2ed.c13
Bronson, K. F., Cassman, K. G., Wassman, R., Olk, D. C., Noordwik, M. V., and Garrity, D. P. (1988). “Soil carbon dynamics in different cropping systems in principal ecoregions of Asia,” in Management of carbon sequestration in soil, eds. R. Lal, J. M. Kimble, R. F. Follet, and B. A. Stewart (Boco Raton, FL: CRC Press), 35–37.
Chan, K. Y., Bowman, A., and Oates, A. (2001). Oxidizable organic carbon fractions and soil quality changes in an oxic paleustalf under different pastures leys. Soil Sci. 166, 61–67. doi: 10.1097/00010694-200101000-00009
Chandra, R. (2011). Effect of summer crops and their residue management on yield of succeeding wheat and soil properties. J. Indian Soc. Soil Sci. 59, 37–42.
Cheng, W., Parton, W. J., and Gonzalez–Meler, M. A. (2014). Synthesis and modelling perspectives of rhizosphere priming. New Phytol. 201, 31–44. doi: 10.1111/nph.12440
Choudhary, M., Datta, A., Jat, H. S., Yadav, A. K., Gathala, M. K., Sapkota, T. B., et al. (2018). Changes in soil biology under conservation agriculture based sustainable intensification of cereal systems in Indo–gangetic plains. Geoderma 313, 193–204. doi: 10.1016/j.geoderma.2017.10.041
Cotrufo, M. F., Wallenstein, M. D., Boot, C. M., Denef, K., and Paul, E. (2013). The Microbial Efficiency–Matrix Stabilization (MEMS) framework integrates plant litter decomposition with soil organic matter stabilization: do labile plant inputs form stable soil organic matter? Glob. Change Biol. 19, 988–995. doi: 10.1111/gcb.12113
Das, T. K., Bhattacharya, R., Sharma, A. R., Das, S., Saad, A. A., and Pathak, H. (2013). Impacts of conservation agriculture on total soil organic carbon retention potential under an irrigated agro–ecosystem of the western Indo–gangetic plains. Eur. J. Agron. 51, 34–42. doi: 10.1016/j.eja.2013.07.003
Datta, A., Basak, N., Chudhari, S. K., and Sharma, D. K. (2015). Soil properties and organic carbon distribution under different land use in reclaimed sodic soils of North–West India. Geoderma Reg. 4, 134–146. doi: 10.1016/j.geodrs.2015.01.006
Datta, A., Choudhury, M., Sharma, P. C., Priyanka, J.at, H. S., Jat, M. L., and Kar, S (2022). Stability of humic acid carbon under conservation agriculture practices. Soil Tillage Res. 216, 105240. doi: 10.1016/j.still.2021.105240
Datta, A., Jat, H. S., Yadav, A. K., Choudhary, M., Sharma, P. C., Rai, M., et al. (2019). Carbon mineralization in soil as influenced by crop residue type and placement in an Alfisols of Northwest India. Carbon Manag. 10, 37–50. doi: 10.1080/17583004.2018.1544830
Datta, A., Mandal, B., Badole, S., Chaitanya, K., Majumdar, S. P., Padhan, D., et al. (2018). Interrelationship of biomass yield, carbon input, aggregation, carbon pools and its sequestration in Vertisols under long–term sorghum–wheat cropping system in semi–arid tropics. Soil Tillage Res. 184, 164–175. doi: 10.1016/j.still.2018.07.004
Datta, A., Mandal, B., Basak, N., Badole, S., Krishna Chaitanya, A., Majumder, S. P., et al. (2017). Soil carbon pools under long–term rice–wheat cropping system in Inceptisols of Indian Himalayas. Arch. Agron. Soil Sci. 64, 1315–1320. doi: 10.1080/03650340.2017.1419196
Dick, R. P., Breakwell, D. P., Turco, R. F., Doran, J. W., and Jones, A. J. (1996). “Soil enzyme activities and biodiversity measurements as integrative microbiological indicators,” in Methods for Assessing Soil Quality 247–271. doi: 10.2136/sssaspecpub49.c15
Dikgwatlhe, S. B., Chen, Z., Lal, R., Zhang, H., and Chen, F. (2014). Changes in soil organic carbon and nitrogen as affected by tillage and residue management under wheat–maize cropping system in the North China Plain. Soil Tillage Res. 144, 110–118. doi: 10.1016/j.still.2014.07.014
Dolan, M. S., Clapp, C. E., Allmaras, R., Baker, J. M., and Molina, J. A. E. (2006). Soil organic carbon and nitrogen in a Minnesota soil as related to tillage, residue and nitrogen management. Soil Tillage Res. 89, 221–231. doi: 10.1016/j.still.2005.07.015
Dubey, A., and Lal, R. (2009). Carbon footprint and sustainability of agricultural production systems in Punjab, India, and Ohio, USA. J. Crop Improv. 23, 332–350. doi: 10.1080/15427520902969906
Dutta, A., Patra, A., Hazra, K. K., Nath, C. P., Kumar, N., and Rakshit, A. (2022). A state of the art review in crop residue burning in India: previous knowledge, present circumstances and future strategies. Environ. Chall. 8, 100581. doi: 10.1016/j.envc.2022.100581
Eivazi, F., and Tabatabai, M. A. (1988). Glucosidases and galactosidases in soils. Soil Biol. Biochem. 20, 601–606. doi: 10.1016/0038-0717(88)90141-1
Fang, C., Smith, P., Moncrieff, J. B., and Smith, J. U. (2005). Similar response of labile and resistant soil organic matter pools to changes in temperature. Nature 433, 57–59. doi: 10.1038/nature03138
Galdos, M. V., Cerri, C. C., and Cerri, C. E. P. (2009). Soil carbon stocks under burned and unburned sugarcane in Brazil. Geoderma 153, 347–352. doi: 10.1016/j.geoderma.2009.08.025
Gathala, M. K., Kumar, V., Sharma, P. C., Saharawat, Y. S., Jat, H. S., Singh, M., et al. (2013). Optimizing intensive cereal–based cropping systems addressing current and future drivers of agricultural change in the north western indo–gangetic plains of India. Agri. Ecosyst. Environ. 177, 85–97. doi: 10.1016/j.agee.2013.06.002
Gehl, R. J., Schmidt, J. P., Maddux, L. D., and Gordon, W. B. (2005). Corn yield response to nitrogen rate and timing in sandy irrigated soils. Agron. J. 97, 1230–1238. doi: 10.2134/agronj2004.0303
Ghimire, R., Norton, J. B., Stahl, P. D., and Norton, U. (2014). Soil microbial substrate properties and microbial community responses under irrigated organic and reduced–tillage crop and forage production systems. PLoS ONE 9, e103901. doi: 10.1371/journal.pone.0103901
Govaerts, B., Sayre, K. D., Goudeseune, B., De Corte, P., Litcher, K., Dendooven, L., et al. (2009). Conservation agriculture as a sustainable option for the central Mexican highlands. Soil Tillage Res. 103, 222–230. doi: 10.1016/j.still.2008.05.018
Govindasamy, P., Mowrer, J., Rajan, N., Provin, T., Hons, F., and Bagavathiannan, M. (2021). Soil carbon dynamics is improved under long–term (36 years) no–till sorghum production in a sub–tropical environment. Soil Use Manag. 37, 37–48. doi: 10.1111/sum.12636
Himedia Manual (2009). Himedia Manual for Microbiology Laboratory Practice. Thane, India: HiMedia Laboratories Pvt. Ltd.
Jain, N., Bhatia, A., and Pathak, H. (2014). Emission of air pollutants from crop residue burning in India. Aerosol Air Qual. Res. 14, 422–430. doi: 10.4209/aaqr.2013.01.0031
Jat, H. S., Datta, A., Choudhary, M., Sharma, P. C., Yadav, A. K., Choudhary, V., et al. (2019). Climate smart agriculture practices improve soil organic carbon pools, biological properties and crop productivity in cereal–based systems of North–West India. Catena 181, 104059. doi: 10.1016/j.catena.2019.05.005
Jat, H. S., Datta, A., Sharma, P. C., Kumar, V., Yadav, A. K., Choudhary, M., et al. (2018). Assessing soil properties and nutrient availability underconservation agriculture practices in a reclaimed sodic soil in cereal–based systems of North–West India. Arch. Agron. Soil Sci. 64, 531–545. doi: 10.1080/03650340.2017.1359415
Jat, S. L., Parihar, C. M., Dey, A., Nayak, H. S., Ghosh, A., Parihar, N., et al. (2019). Dynamics and temperature sensitivity of soil organic carbon mineralization under medium–term conservation agriculture as affected by residue and nitrogen management options. Soil Tillage Res. 190, 175–185. doi: 10.1016/j.still.2019.02.005
Jingguo, W., and Bakken, L. R. (1997). Competition for nitrogen during mineralization of plant residues in soil: microbial response to C and N availability. Soil Biol. Biochem. 29, 163–170. doi: 10.1016/S0038-0717(96)00292-1
Kumara, B. H., Antil, R. S., and Raj, D. (2014). Impact of long–term manures and fertilizers application on carbon sequestration and its efficiency under pearl millet wheat cropping sequence. Int. J. Farm Sci. 4, 21–26.
Liang, Z., Cao, B., Jiao, Y., Liu, C., Li, X., Meng, X., et al. (2022). Effect of the combined addition of mineral nitrogen and crop residue on soil respiration, organic carbon sequestration, and exogenous nitrogen in stable organic matter. Appl. Soil Ecol. 171, 104322. doi: 10.1016/j.apsoil.2021.104324
Majumder, B., Mandal, B., Bandypadhyay, P. K., Gangopadhyay, A., Mani, P. K., Kundu, A. L., et al. (2008). Organic amendments influence soil organic carbon pools and rice–wheat productivity. Soil Sci. Soc. Am. J. 72, 775–785. doi: 10.2136/sssaj2006.0378
Martin, J. P. (1950). Use of acid, rosebengal and streptomycin in the plate method for estimating soil fungi. Soil Sci. 69, 215–232. doi: 10.1097/00010694-195003000-00006
Mohammadi, K. (2011). Soil microbial activity and biomass as influenced by tillage and fertilization in wheat production. Am. Eurasian J. Agric. Environ. Sci. 10, 330–337.
Nelson, D. W., and Sommers, L. E. (1982). “Total carbon, organic carbon, and organic matter,” in Methods of soil analysis. Part 2. 2nd ASA and SSSA, eds. A. L. Page, R. H. Miller, D. R. Keeney (Madison, WI: Agron. Monogr), 539–579. doi: 10.2134/agronmonogr9.2.2ed.c29
Oliveira, D. M. S., Williams, S., Cerri, C. E. P., and Paustian, K. (2017). Predicting soil C changes over sugarcane expansion in Brazil using the DayCent model. Glob. Change Biol. Bioener. 9, 1436–1144. doi: 10.1111/gcbb.12427
Parihar, C. M., Jat, S. L., Singh, A. K., Datta, A., Parihar, M. D., Varghese, E., et al. (2018). Changes in carbon pools and biological activities of a sandy loam soil under medium–term conservation agriculture and diversified cropping systems. Eur. J. Soil Sci. 69, 902–912. doi: 10.1111/ejss.12680
R Development Core Team (2015). R: a language and environment for statistical computing. Vienna, Austria: R Foundation for Statistical Computing. Available online at: http://www.r--project.org/ (accessed November 18, 2023).
Razafimbelo, T., Bernard Barthe's, B., Larre'–Larrouy, M. C., De Luca, E. F., Laurent, J. Y., Cerri, C. C., et al. (2006). Effect of sugarcane residue management (mulching versus burning) on organic matter in a clayey Oxisol from southern Brazil. Agric. Ecosyst. Environ. 115, 285–289. doi: 10.1016/j.agee.2005.12.014
Roldan, J. R., Salinas–Garcia, J. R., Alguacil, M. M., Diaz, E., and Caravaca, F. (2005). Soil enzyme activity suggests advantages of conservation tillage practices in sorghum cultivation under subtropical condition. Geoderma 129, 178–185. doi: 10.1016/j.geoderma.2004.12.042
Roy, D., Datta, A., Jat, H. S., Choudhary, M., Sharma, P. C., Singh, P. K., et al. (2022). Impact of long term conservation agriculture on soil quality under cereal based systems of North West India. Geoderma 405, 115391. doi: 10.1016/j.geoderma.2021.115391
Saggar, S., Headley, C. B., and Salt, G. J. (2001). Soil microbial biomass, metabolic quotient and carbon and nitrogen mineralization in 25–year–old Pinus radiate agroforestry regimes. Aus. J. Agril. Res. 39, 491–504. doi: 10.1071/SR00012
Saha, S., Prakash, V., Kundu, S., Kumer, N., and Mina, B. L. (2008). Soil enzymatic activity as affected by long term application of farm yard manure and mineral fertilizer under a rainfed soybean–wheat system in N–W Himalaya. Euro. J. Soil Biol. 44, 147–157. doi: 10.1016/j.ejsobi.2008.02.004
Singh, P., Singh, G., and Sodhi, G. P. S. (2020). Energy and carbon footprints of wheat establishment following different rice residue management strategies vis–à–vis conventional tillage coupled with rice residue burning in north–western India. Energy. 200, 117554. doi: 10.1016/j.energy.2020.117554
Singh, Y., and Sidhu, H. S. (2014). Management of cereal crop residues for sustainable rice–Wheat production system in the Indo–Gangetic plains of India. Proc. Ind. Nat. Sci. Acad. 80, 95–114. doi: 10.16943/ptinsa/2014/v80i1/55089
Six, J., Feller, C., Denef, K., Ogle, S. M., deMoraesSa, J. C., and Albrecht, A. (2002). Soil organic matter, biota and aggregation in temperate and tropical soils–effects of no–tillage. Agron. Sustain. Dev. 22, 755–775. doi: 10.1051/agro:2002043
Somasundaram, J., Salikram, M., Sinha, N. K., Mohanty, M., Chaudhary, R. S., Dalal, R. C., et al. (2019). Conservation agriculture effects on soil properties and crop productivity in a semiarid region of India. Soil Res. 57, 187–199. doi: 10.1071/SR18145
Stott, D. E., Karlen, D. L., Cambardella, C. A., and Harmel, R. D. (2013). A soil quality and metabolic activity assessment after fifty–seven years of agricultural management. Soil Sci. Soc. Am. J. 77, 903–913. doi: 10.2136/sssaj2012.0355
Suman, A., Singh, K. P., Singh, P., and Yadav, R. L. (2009). Carbon input, loss and storage in sub–tropical Indian Inceptisol under multi–ratooning sugarcane. Soil Tillage Res. 104, 221–226. doi: 10.1016/j.still.2009.02.008
Surendran, U., Ramesh, V., Jayakumar, M., Marimuthu, S., and Sridevi, G. (2016). Improved sugarcane productivity with tillage and trash management practices in semi arid tropical agro ecosystem in India. Soil Tillage Res. 158, 10–21. doi: 10.1016/j.still.2015.10.009
Tenelli, S., Bordonal, R. O. B., Barbosa, L. C., and Carvalho, J. L. N. (2019). Can reduced tillage sustain sugarcane yield and soil carbon if straw is removed? Bioenergy Res. 12, 764–777. doi: 10.1007/s12155-019-09996-3
Walkley, A., and Black, I. A. (1934). An examination of the Degtjareff method for determining soil organic matter and a proposed modification of the chromic acid titration methods. Soil Sci. 37, 29–38. doi: 10.1097/00010694-193401000-00003
Wang, J. B., Chen, Z. H., Chen, L. J., Zhu, A. N., and Wu, Z. J. (2011). Surface soil phosphorous and phosphatase activities affected by tillage and crop residue input amounts. Plant Soil Environ. 57, 251–257. doi: 10.17221/437/2010-PSE
Wendling, B., Jucksch, I., Mendonca, E. S., and Alvarenga, R. C. (2010). Organic–matter pools of soil under pines and annual cultures. Commun. Soil Sci. Plant Anal. 41, 1707–1722. doi: 10.1080/00103624.2010.489135
Yadav, S. K., Singh, S. C., Yadav, S., Yadav, S. K., Tiwari, A. K., and Sharma, B. L. (2019). Integrated nutrient management approaches for enhancing production potential and sustainability of sugarcane (Saccharum spp. hybrid) plant–ratoon system in North region of India. Sugar Tech. 21, 170–175. doi: 10.1007/s12355-018-0641-z
Zang, H., Wang, J., and Kuzyakov, Y. (2016). N fertilization decreases soil organic matter decomposition in the rhizosphere. Appl. Soil Ecol. 108, 47–53. doi: 10.1016/j.apsoil.2016.07.021
Keywords: crop residue, carbon retention efficiency, enzyme activities, microbial count, multi-ratooning sugarcane, nutrient management, soil organic carbon
Citation: Pradhan A, Wakchaure GC, Shid D, Minhas PS, Biswas AK and Reddy KS (2023) Impact of residue retention and nutrient management on carbon sequestration, soil biological properties, and yield in multi-ratoon sugarcane. Front. Sustain. Food Syst. 7:1288569. doi: 10.3389/fsufs.2023.1288569
Received: 04 September 2023; Accepted: 21 November 2023;
Published: 14 December 2023.
Edited by:
Prabhu Govindasamy, Indian Agricultural Research Institute (ICAR), IndiaReviewed by:
Sandeep Kumar Sihag, Indian Agricultural Research Institute (ICAR), IndiaVikash Kumar, Sardarkrushinagar Dantiwada Agricultural University, India
Copyright © 2023 Pradhan, Wakchaure, Shid, Minhas, Biswas and Reddy. This is an open-access article distributed under the terms of the Creative Commons Attribution License (CC BY). The use, distribution or reproduction in other forums is permitted, provided the original author(s) and the copyright owner(s) are credited and that the original publication in this journal is cited, in accordance with accepted academic practice. No use, distribution or reproduction is permitted which does not comply with these terms.
*Correspondence: Aliza Pradhan, YWxpemFwcmFkaGFuQGdtYWlsLmNvbQ==