- 1Instituto de Ecología Aplicada, Universidad Autónoma de Tamaulipas (UAT), Ciudad Victoria, Mexico
- 2Facultad de Ciencias de la Nutrición y Alimentos, Universidad de Ciencias y Artes de Chiapas (UNICACH), Tuxtla Gutiérrez, Mexico
- 3Escuela de Ciencias Químicas, Universidad Autónoma de Chiapas (UNACH), Tuxtla Gutiérrez, Mexico
- 4Unidad Académica de Trabajo Social y Ciencias para el Desarrollo Humano, Universidad Autónoma de Tamaulipas (UAT), Ciudad Victoria, Mexico
- 5Department of Molecular and Cell Biology, The University of Connecticut (UConn), Storrs, CT, United States
The cheese-making process generates large amounts of cheese whey wastewater (CWW), which is abundant in nutrients but difficult to dispose of, contributing to the eutrophication of natural environments due to inadequate waste management. Here we show the anaerobic digestion of CCW by syntrophy between bacteria and archaea in an expanded granular sludge bed (EGSB) bioreactor as a low-cost alternative for bioremediation and biofuel production. The performance of the EGSB bioreactor and the composition of the natural microbial community were evaluated. During the operation of the EGSB bioreactor, physicochemical parameters such as alkalinity ratio (0.25), pH (7.5), and temperature (26°C) were attained and maintained, as well as light- and oxygen-free conditions, which favored the metabolism of oxygen-sensitive bacteria and methane-producing archaea (methanogens). Under these conditions, the chemical oxygen demand (COD) removal rate was highly efficient (> 89%). Methane (CH4) was produced from organic matter degradation by a few methanogens, mainly from Methanosaeta spp., and was enhanced by the metabolic interaction between bacteria and archaea. The biochemical methane potential (BMP) was >335 mL CH4/gCOD, indicating that the syntrophic microbial community is very efficient in removing organic matter and CH4 produced from CWW. Our results suggest that CWW could be treated in EGSB bioreactors and used as a sustainable alternative to CH4 production and also provide insights for the design of synthetic microbial communities (SynComs) for bioremediation, biogas production, and other biotechnological processes.
1 Introduction
The food industry is one of the main industrial sectors of the worldwide economy, but it also generates large amounts of waste, contributing to the eutrophication of diverse environments when waste is not properly treated. The dairy agro-industry encompasses the activity dedicated to the development of products from milk, especially cheese (Fernández-Rodríguez et al., 2016) with a world production of 23.5 million tons/year from cows’ milk (FAOSTAT, 2023). During the cheese-making process, cheese whey is generated as a by-product, which is the liquid fraction of milk that is obtained after the precipitation and recovery of casein by acid or enzymatic action (Cruz-Salomón et al., 2020).
Cheese whey represents approximately 90% of the total volume of cow’s milk used and retains approximately 55% of the nutrients (Pires et al., 2021). In the cheese-making process, between 8 and 9 liters of cheese whey are generated for every kilogram of cheese produced (Asunis et al., 2019), resulting in a worldwide production of around 211.3 million m3/year. Therefore, cheese whey is considered the main by-product of the cheese agro-industry but also one of the main sources of waste and environmental pollution (Rincón-Catalán et al., 2022). The composition and physicochemical properties of cheese whey wastewater (CWW) generally depend on manufacturing practices used in cheese-making and other dairy processes (Carvalho et al., 2013).
Currently, the most common methods for CWW disposal are: (1) direct discharge into water bodies, such as rivers, lakes, oceans, or into municipal drainage/sewer systems (Prazeres et al., 2012), (2) used as a fertilizer or soil amendment in agriculture (Aboukila et al., 2018), (3) used as a food supplement for fattening animals, and (4) biological pre-treatment prior to end disposals. Consequently, these types of disposal can have several negative effects, such as reducing the quality of water and soils (inducing anoxia, eutrophication, acidification, toxicity, and pH imbalance), affecting metabolic interactions in aquatic and semi-aquatic environments (Tirado et al., 2018; Mostafa-Imeni et al., 2019), increasing contamination of nutrient runoff and groundwater (Ghaly et al., 2007), and generating digestive problems in animals due to an unbalanced diet (Sutera et al., 2023). For these reasons, the concern of the agro-industry for the treatment and reuse of CWW promotes the constant search for technologies that provide viable alternatives for the chemical or biological degradation of the pollutants contained in CWW, which can seriously impact the environment and economic activities (Coelho et al., 2019).
Anaerobic digestion (AD) in the expanded granular sludge bed (EGSB) bioreactor has been satisfactorily evaluated on a laboratory scale as a low-cost bioremediation alternative for the treatment of CWW (Lopes et al., 2017; Ramos and Silva, 2017; Cruz-Salomón et al., 2017a, 2020; Ramos et al., 2020). The EGSB bioreactor shows technical feasibility due to its low operating costs, simplicity in design and customization, robust technology, and easy operation for biofuel production from different wastewater. EGSB bioreactors operate efficiently under oxygen-free conditions, which reduces energy consumption and costs associated with aeration. EGSB bioreactors are highly efficient in producing methane by removing organic matter from wastewater, especially when chemical oxygen demand (COD) is >1,500 mgO2/L. Since methane can be used as an alternative biofuel for low-cost power generation, EGSB bioreactors are more attractive than aerobic bioreactors. Additionally, this technology reduces costs associated with sludge handling, treatment, and disposal (Cruz-Salomón et al., 2019).
EGSB bioreactors have been primarily designed for organic matter removal but also remove other nutrients such as nitrogen and phosphorus. While aerobic bioreactors excel in nutrient removal, the ability of EGSB bioreactors to achieve simultaneous organic matter and nutrient removal provides additional advantages. The efficiency of EGSB bioreactors depends on the amount of nutrients that could potentially be bio-transformed into renewable methane (Cajacuri et al., 2013; Wu and Yin, 2020), but also on the composition of the microbial community that usually coexists in anaerobic granular sludge (AnGS). However, the archaeal diversity of this complex microbial community has not been characterized when EGSB bioreactors are fed with CWW.
The biological production of methane in bioreactors is very sensitive to environmental and nutritional changes (e.g., oxygen availability, pH variation, accumulation of products, metals, and other compounds). The efficiency of EGSB bioreactors depends on inoculation, stability, and metabolic interactions (hydrolysis, acidogenesis, and methanogenesis) in the complex microbial communities (Nettmann et al., 2010; Jiménez-Hernández et al., 2014). Therefore, monitoring the diversity and abundance of microbial communities and metabolic processes associated with anaerobic biodegradation of cheese wastewater could help to predict and visualize the actions to be taken during EGSB bioreactor operation. This information can also help maximize organic matter removal from cheese wastewater and the production of biomolecules from wastewater by oxygen-sensitive microorganisms.
Here we report the performance of an EGSB bioreactor fed by CWW and the composition of the microbial community responsible for the biosynthesis of methane from complex organic matter. These microbiological parameters have not been previously considered but enhance the performance of our EGSB bioreactor and the removal of CWW.
2 Materials and methods
2.1 Materials
The CWW was collected from a cheese-processing factory located in Chiapas, Mexico (latitude 16°45′41.26″N and longitude 93°22′32.35″W) and stored at −20°C until used. All the chemicals used during the experiments were of analytical grade purchased from J.T. Baker (Mexico City, Mexico). Tri-distilled water was used.
The inoculum for anaerobic digestion in an EGSB bioreactor consists of an anaerobic granular sludge (AnGS) obtained from a full-scale Upflow Anaerobic Sludge Blanket Digestion (UASB) bioreactor treating effluent from a soft drink industry in Chiapas, Mexico (latitude 16°42′30″ N and longitude 93°1′1″W).
2.2 Experimental setup and operation
The laboratory-scale EGSB bioreactor and its configuration are depicted schematically in Figure 1. It was made of fiberglass with a volume of 3.3 L, a height of 146 cm, an inner diameter of 5 cm, and height/diameter ratio of 29.2. The total working, reaction zone, and settling volume were 2.9, 2.3, and 1 L, respectively. At the top of the bioreactor, a gas–liquid–solid separator was installed. The bioreactor was covered with black cardboard and aluminum foil to avoid methane production inhibition caused by light (Chen et al., 2011). The biogas discharged from the top of the bioreactor passed through a polyethylene terephthalate gas scrubber of cylindrical shape (height 50 cm and diameter 3 cm) loaded with a 3% sodium hydroxide solution and phenolphthalein (Cruz-Salomón et al., 2020). The bioreactor was inoculated with AnGS (30% of bioreactor working volume) and operated for 130 days under a mesophilic condition (26°C), at a hydraulic retention time (HRT) of 6 days and an organic loading rate (OLR) of 6.1 Kg COD/m3 day. For bioreactor feeding, the CWW was diluted to 50% with tri-distilled water (36.66 ± 2.45 g O2/L) and adjusted to a pH between 6.8 and 7.2 by adding sodium bicarbonate (NaHCO3) (Xia et al., 2016). One peristaltic pump to load influent into the EGSB bioreactor was used.
2.3 Analytical methods
The feedstock sample was analyzed immediately according to Standard Methods for Examination of Water and Wastewater (APHA, 2005), in order to determine temperature, color, turbidity, pH, oxidation/reduction potential (ORP), electrical conductivity (EC), floating matter (FM), settleable solids (SS), total solids (TS), total volatile solids (TVS), total suspended solids (TSS), total dissolved solids (TDS), acidity, alkalinity, fats, oil, and grease (FOG), chemical oxygen demand (COD), biochemical oxygen demand (BOD5), total organic carbon (TOC), total Kjeldahl nitrogen (TKN), ammonia-N, nitrate-N, nitrite-N, total phosphorus (TP), sulfates, Enterococcus faecalis, Escherichia coli, and Helminth eggs. The organic and inorganic matter, moisture, protein, ash, carbohydrate, lactose, dietary fiber, and total polyphenols content (TPC) content were determined according to the Association of Official Analytical Chemists (AOAC, 2019). The biodegradability (BI), competitiveness (CI), and FOG-wastewater (FWI) indexes were determined according to the reports by Cruz-Salomón et al. (2019). The metal ion (zinc, copper, magnesium, potassium, calcium, sodium, and iron) content was analyzed by using an inductively coupled plasma optical emission spectrophotometer (ICP-OES, Optima 7000 DV, PerkinElmer, United States).
The physicochemical characteristics of AnGS, such as pH, EC, ORP, TS, TVS, TVS/TS ratio, color, and odor, were analyzed according to APHA (2005). The sludge volume index (SVI), specific methanogenic activity (SMA), and density were determined according to Cruz-Salomón et al. (2017b), Wongburi and Park (2022), and van den Berg et al. (2023), respectively. All the parameters were measured during the next 24 h.
The morphology of the AnGS was observed using a scanning electron microscope (SEM) (JEOL, Model IT300, Boston, MA, United States) with an acceleration voltage of 15 kV. Specimen preparation was as follows. Firstly, the AnGS samples were suspended with anhydrous ethane to obtain a 1% (w/v) suspension; a drop of this suspension was taken by the aluminum slide and adhered with double-sided graphite tape and subsequently covered with a thin gold film to make them conductive to the electron beam. Measurements were taken using the SEM image scale bars.
Methane production was measured using Milligascounter (Ritter, MGC-1 V3.3 PMMA) as shown in Figure 1. In addition, the biochemical methane potential (BMP) value was determined according to the reports by Cruz-Salomón et al. (2017b). All the tests were performed in triplicate.
2.4 DNA extraction, library construction, and sequencing
For the microbial analysis, total DNA was extracted to survey the bacterial and archaeal communities, using two types of primers (see below). Samples from the low anaerobic granular sludge (AnGS) were taken by triplicate during the initial step (on day 0) and final step (after 130 days). A total of six DNA samples were extracted. For the AnGS, DNA was extracted from 0.2 g of material using the commercial ZymoBIOMICS™ DNA Miniprep Kit and following the manufacturer’s protocol.
DNA purity and concentration were analyzed with NanoDrop™ One (ThermoFisher Scientific). The DNA yield was 40–80 ng/μL of AnGS on average. The extracted DNA was stored at −20°C until required for PCR amplification and sequencing.
The DNA gene obtained was used to amplify using the primers 314F(5′-CCT ACG GGN GGC WGC AG-3′) and 805R(5′-GAC TAC HVG GGT ATC TAA TCC-3′) for bacteria, and 21F(5′-TCC GGT TGA TCC YGC CGG-3′) y 516R(GGT DTT ACC GCG GCK GCT G-3′) for archaea. Barcodes DNA libraries were sequenced through the Illumina (Macrogen, Seoul, Korea) MiSeq 2 × 300 bp platform.
2.5 Sequence filtering and taxonomic assignment
Raw FASTQ reads were quality-filtered with FASTQC v 0.11.91 and DADA2 (Callahan et al., 2016). Reads smaller than 36 bp and those of low quality according to the Phred scale were eliminated. Subsequently, amplicons and chimeras were removed, read binding was performed, and amplicon sequence variants (ASVs) were obtained. The tables of ASVs were used as input in the phyloseq (McMurdie and Holmes, 2013) R package for microbiome analysis2 to generate plots with ggplot2 (Wickham, 2016). Various R packages were used to build graphics, such as ggplot23 and ape.4
Taxonomic assignment was based on Silva v138 (SILVA, 2023),5 looking at counts in phylum, order, class, and family. Alpha diversity was calculated using the Shannon, Simpson, Inv Simpson, Chao, and evenness_pielou indices (Supplementary Figure S1). The beta diversity was obtained through Principal Coordinate Analysis (PcoA) plots to observe the distribution of the samples based on their similarities, and the Weighted Unifrac method was obtained (Supplementary Figure S2).
The difference in composition of bacterial and archaeal communities was assessed by permutational multivariate analysis of variance (PERMANOVA) with 10,000 permutations (Kelly et al., 2015).
3 Results
3.1 Cheese whey wastewater characterization
The physical, chemical, and microbiological characterization of CWW was performed in accordance with standardized methods of APHA (2005) and AOAC (2019), and the results obtained are shown in Table 1. Our data show that several of the analyzed parameters exceed the maximum limits set by World Health Organization (1995) and Official Mexican Environmental Regulations (2015). Consequently, these findings indicate high pollution associated with this agro-industry and limited residual management.
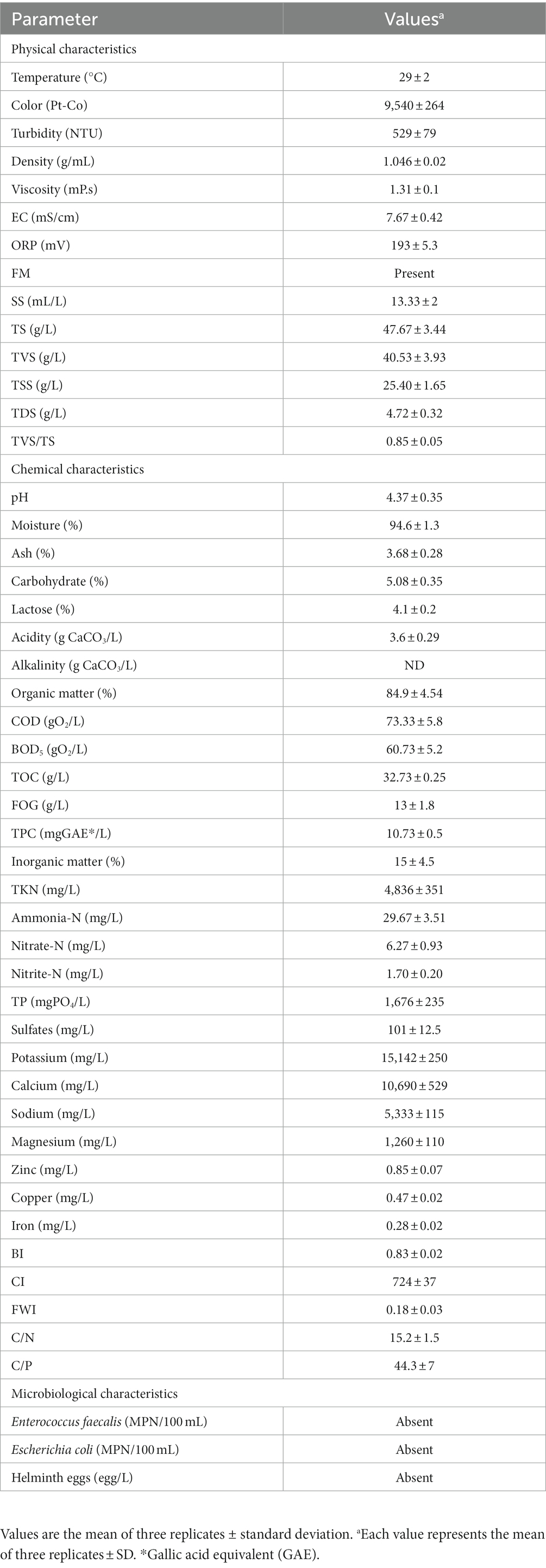
Table 1. Physicochemical and microbiological characterization of cheese whey wastewater (CWW) samples.
3.2 Anaerobic granular sludge characterization
The anaerobic granular sludge (AnGS) was comprised of irregularly shaped granules (∅, c. 0.5–1 mm) (Figure 2), with typical sewage odor (foul-smelling hydrogen sulfide gas), gray-black color, and with the following parameters: pH (7.45 ± 0.25), EC (7.01 ± 0.15 mS/cm), ORP (−414.66 ± 30 mV), TS (148.75 ± 4.6 g/L), TVS (76.67 ± 9.4 g/L), TVS/TS ratio (0.51 ± 0.06), sludge volume index (SVI, 8.1 ± 0.34 mL/gTS), specific methanogenic activity (SMA, 0.345 ± 0.02 gCOD/gTVS day), and density (1.26 ± 0.03 g/mL). This AnGS was used in the laboratory-scale EGSB bioreactor, and these physical and chemical properties of the AnGS positively influenced its performance and stability.
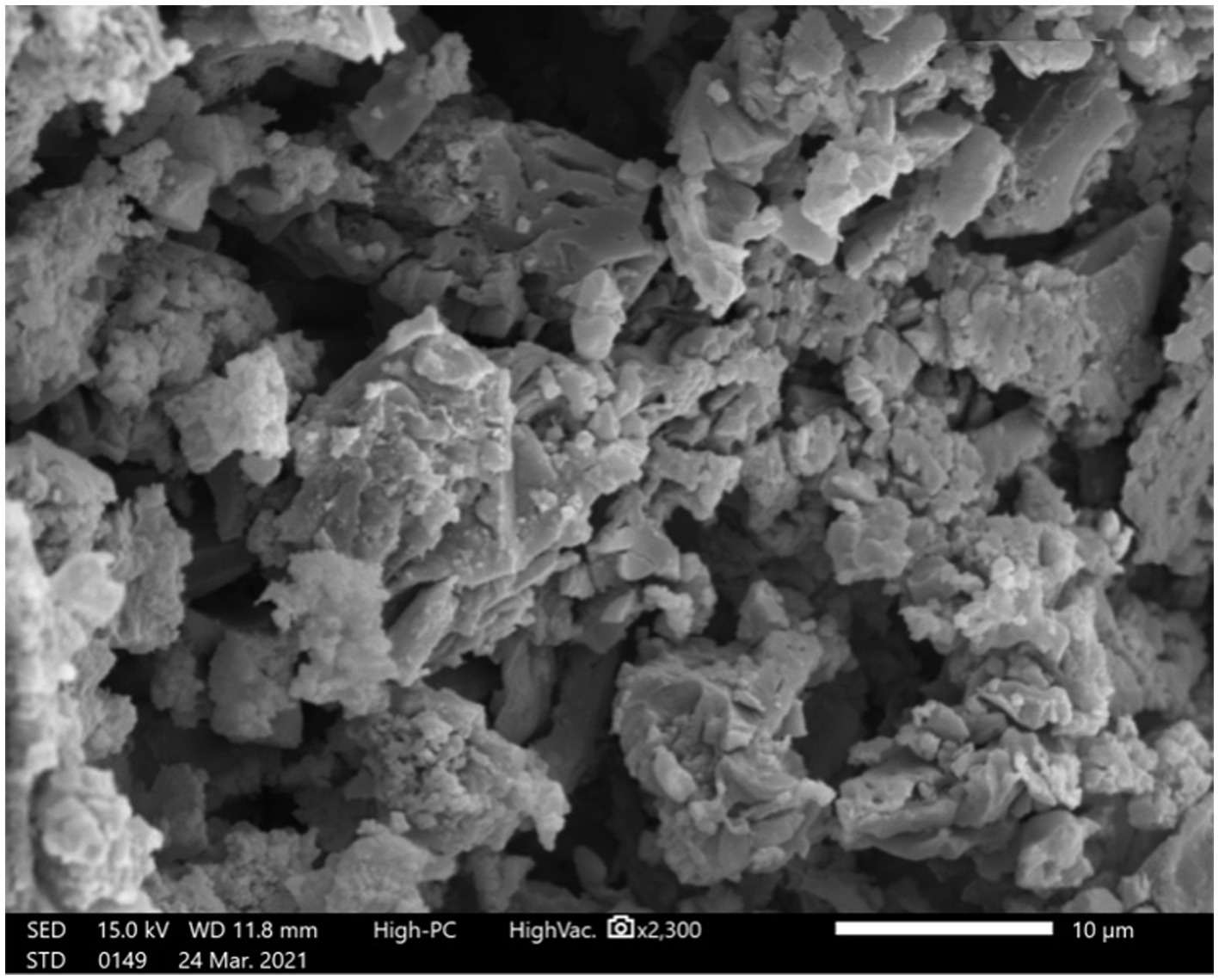
Figure 2. Micrograph of the anaerobic granular sludge (AnGS) used for the EGSB bioreactor. Image obtained by scanning electron microscope (SEM).
3.3 EGSB bioreactor performance
The pH, temperature, alkalinity ratio, and COD removal efficiency were monitored during the EGSB bioreactor operation (130 days) (Figure 3). These parameters helped to identify three phases during the EGSB bioreactor operation: start-up (days 0–24), stabilization (day 24–45), and stable conditions (day 45–130). The pH (7.5) and temperature (26°C) were stable during the incubation time (Figure 3A). The alkalinity ratio (AI/TA) was controlled to avoid acidification of the bioreactor and was less than 0.3 from day 48 to 130 (Figure 3B). The efficiency of this anaerobic bioreactor was evaluated using several parameters, with COD removal rate and methane production being the two crucial indicators. In Figure 3C, we show that the assessment of this parameter remained as high as 89%, with an organic matter concentration of the effluent reaching 4,032 mgO2/L, suggesting highly efficient performance.
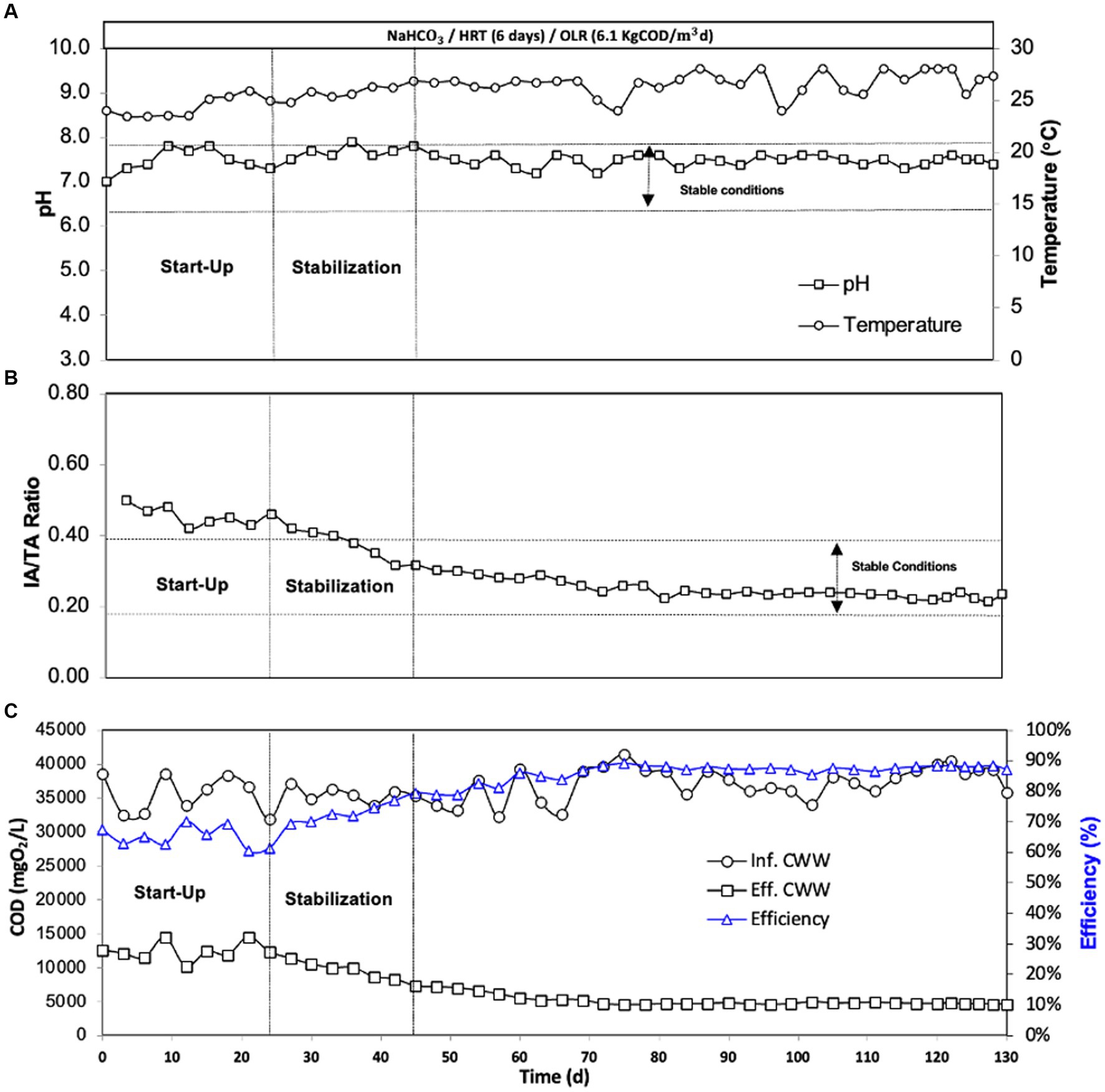
Figure 3. Evolution of the performance EGSB bioreactor during incubation: (A) pH and temperature; (B) the alkalinity ratio (AI/TA); (C) COD removal efficiency.
Also, during the EGSB bioreactor operation, the methane production was constant and lineal. To evaluate the efficiency in the transformation of organic matter into methane by the microbial community associated with the bioreactor, methane production was monitored for 24 h in the stable conditions phase (Figure 4). The actual methane production was higher than expected (around 6,900 mL CH4/day). The biochemical methane potential (BMP) value, which determines the maximum methane potential and biodegradability of added organic substrates, was also high (335 mL CH4/gCOD). These results suggest that the methanogens used other energy and carbon sources to produce more methane, potentially hydrogen and carbon dioxide, all of which are typically products of bacterial metabolism.
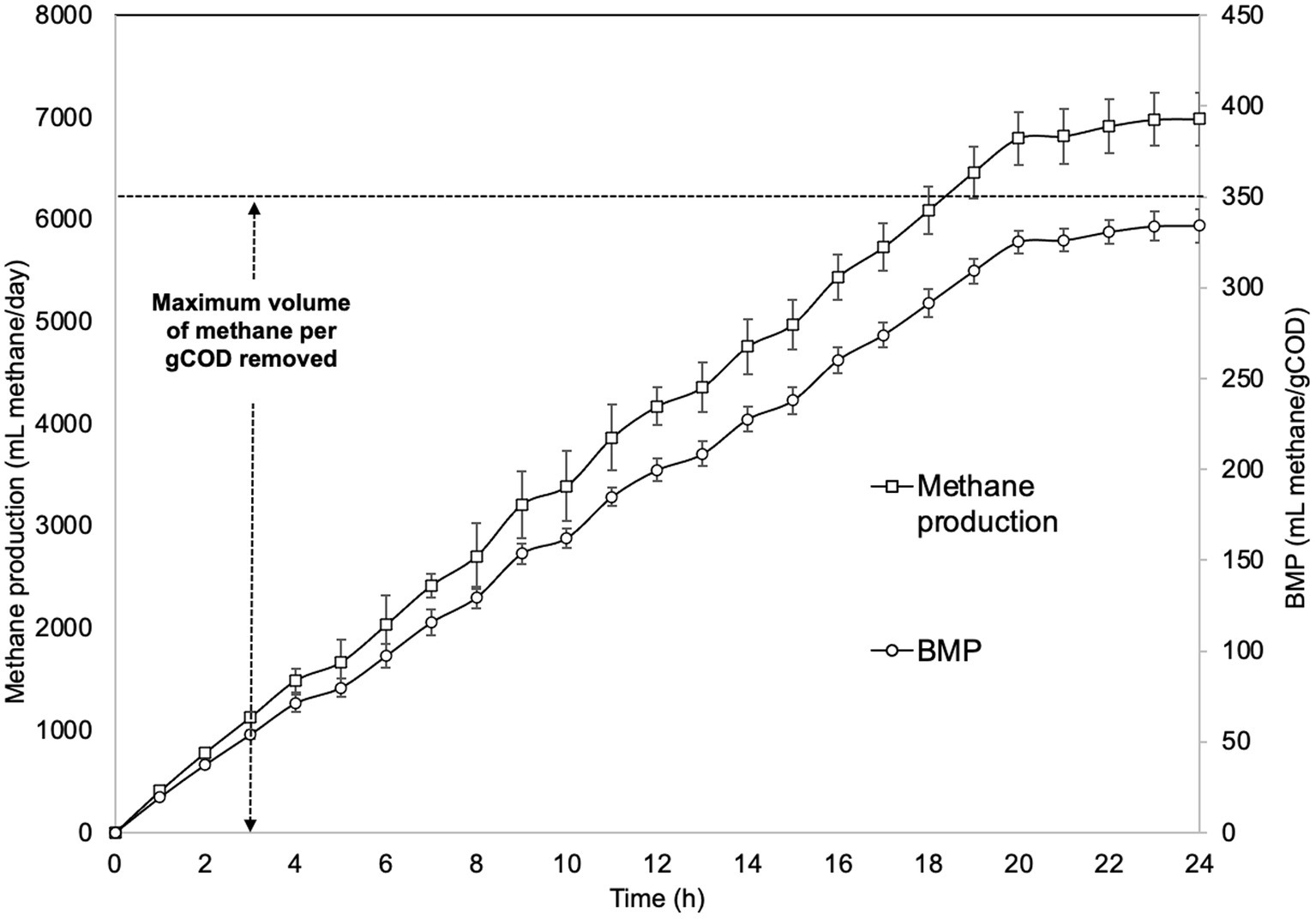
Figure 4. Methane production from cheese whey wastewater in the EGSB bioreactor. BMP = biochemical methane potential value. Values are the mean of three biological replicates and the standard deviation.
3.4 Microbial community analysis
We extracted DNA from three independent samples from the EGSB bioreactor. We obtained a total of 706,062 raw reads from the six samples, approximately 117,000 per sample. Once the cleaning was completed, we rescued 553,826 reads, and the average number of reads per sample was 92,304 (ranging from 87,045 to 100,330) (Supplementary Table S1). Extra filtering of the clean sequences was conducted to obtain external contamination.
To facilitate comparisons, we displayed the data using the same nomenclature. We analyzed three samples: A1, A2, and A3. All three samples were sequenced using primers commonly used for bacterial identification and other primers for archaeal identification. By that, we mean that S1A1 and S2A1 are the same samples, but different primers were used (S1 = primers for bacteria, and S2 = primers for archaea).
3.4.1 Initial microbial community
We analyzed the initial microbial composition of the EGSB bioreactor. In total, 7,567 OTUs were assigned. The most abundant phyla are shown in Figure 5, where Halobacterota and Thermoplasmatota are the only two phyla of the Archaea domain. Halobacterota was detected when primers for bacteria (S1A1, S1A2, and S1A3) and archaea (S2A1, S2A2, and S2A3) were used. Bacteroidota (synonym Bacteroidetes), Chloroflexi, and Desulfobacterota were the most abundant phyla from the Bacteria domain.
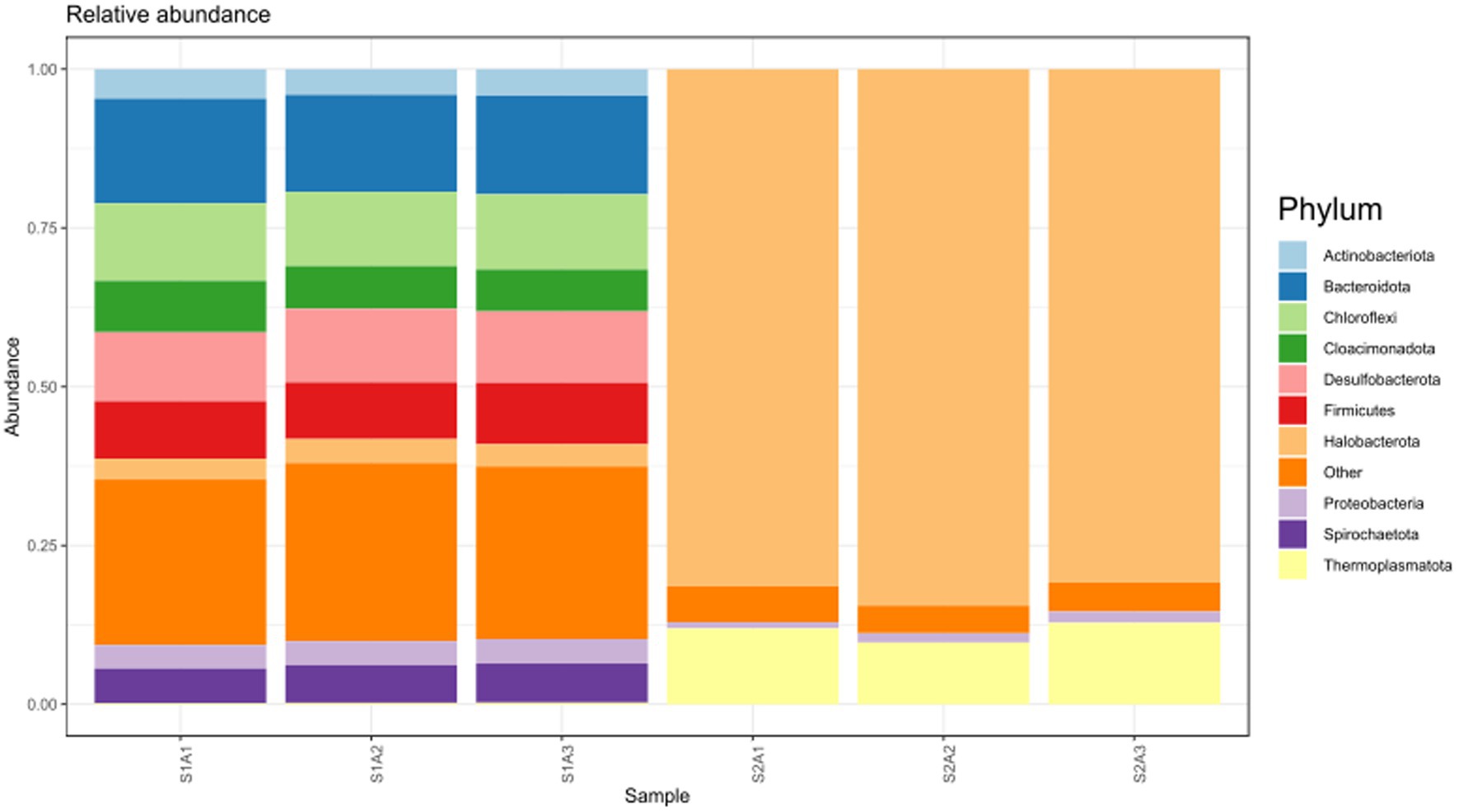
Figure 5. The most abundant phyla of the bacteria and archaea domain found in the initial anaerobic granular sludge (AnGS) from the EGSB bioreactor.
When the same data were analyzed at the genus level, Methanosaeta spp. was the most abundant archaea group and was identified when bacteria and archaea primers were used. Methanocorpusculum spp., Methanolinea spp., and Methanoregula spp. were also identified using both primers (Supplementary Figure S3). When only archaea data was analyzed, Methanosaeta spp. and Methanosarcina spp. were the most abundant groups, with a very clear difference in their relative abundance (Figure 6). All these genera are methane-producing archaea (methanogens) usually associated with oxygen-free environments and have high specificity for energy and carbon sources. These two genera have the unique ability to produce methane from acetate degradation via aceticlastic methanogenesis pathways that can be hydrogen-dependent or independent.
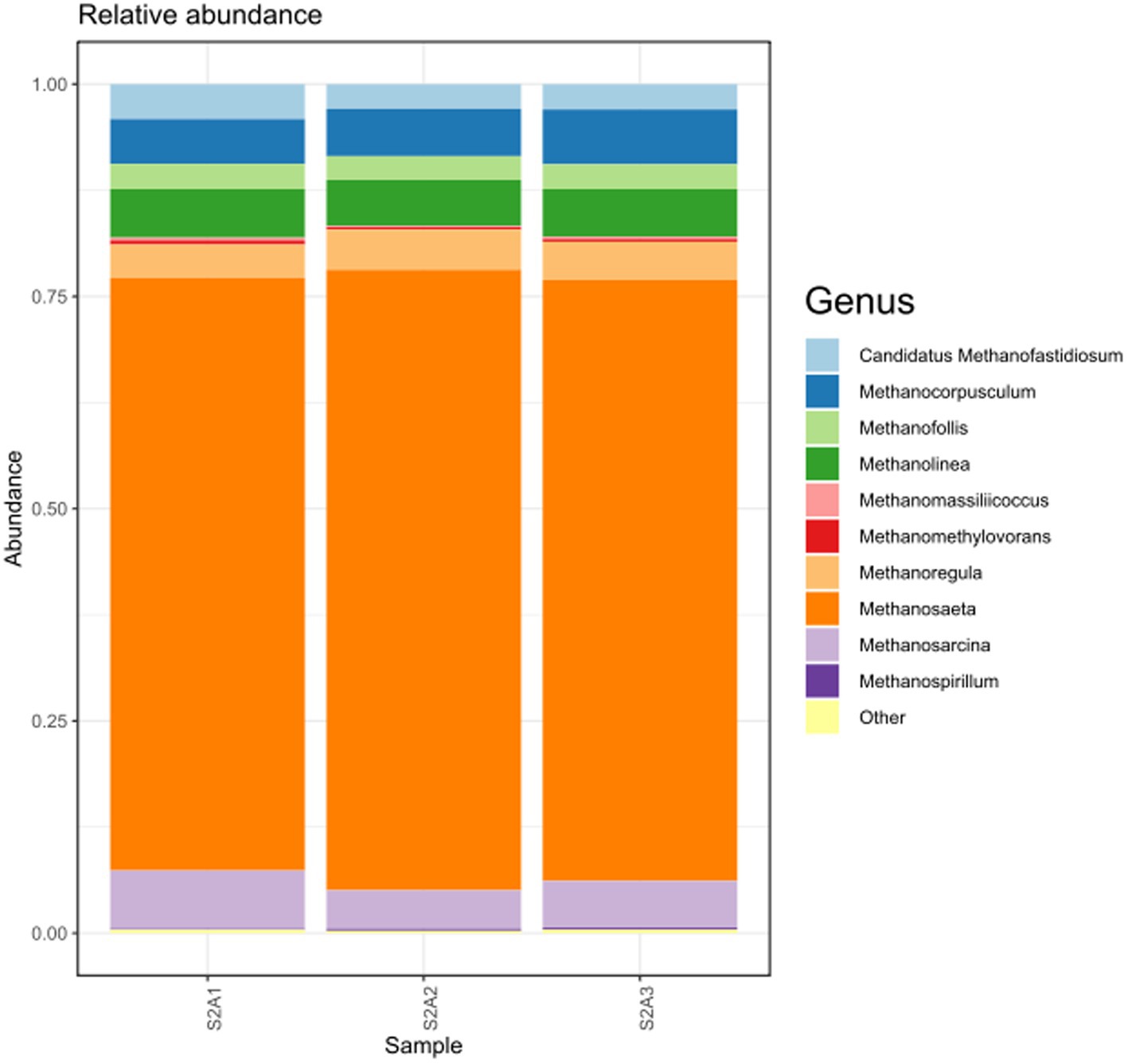
Figure 6. The most abundant genus of the archaea domain found in the initial anaerobic granular sludge (AnGS) from the EGSB bioreactor.
3.4.2 Enrichment of microbial community
After 130 days of incubation, three new samples from the EGSB bioreactor were taken, and their microbial composition was analyzed (Figure 7). A change in the microbial composition was observed, mainly in the bacterial members. Firmicutes (synonym Bacillota), Bacteroidota (synonym Bacteroidetes), and Cloacimonadota (synonym Cloacimonetes) were the most abundant phyla of the Bacteria domain, whereas Halobacterota was the most abundant archaea phylum. These data suggest that the EGSB bioreactor conditions favored the growth of Firmicutes, probably due to low oxygen availability.
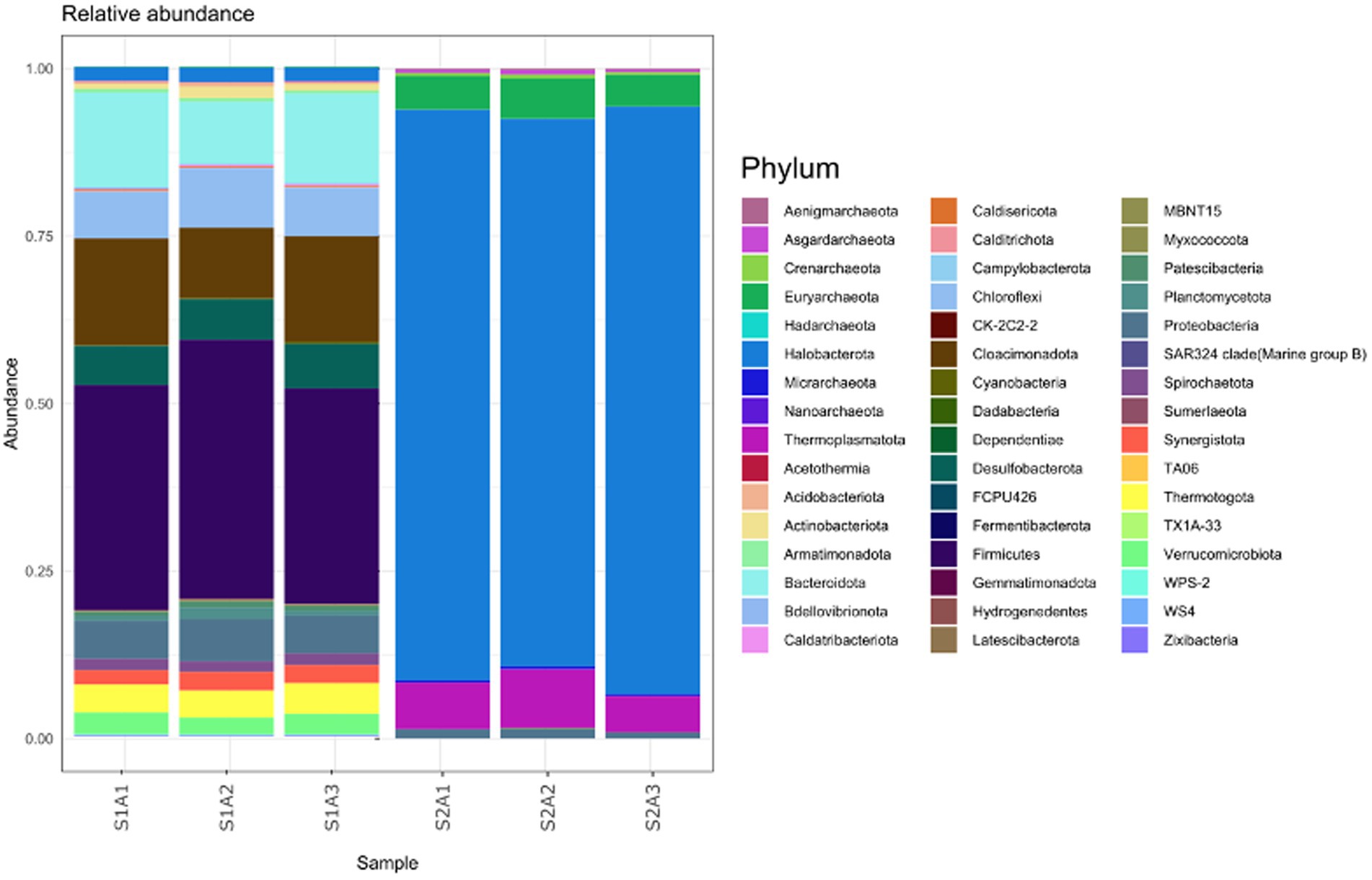
Figure 7. The most abundant phyla of the bacteria and archaea domain found in the EGSB bioreactor after 130 days of incubation.
When archaea data was reanalyzed, Methanosaeta spp. was the most abundant genus with a very clear difference in its relative abundance (Figure 8). These results suggest that Methanosaeta spp. (acetate-utilizing methanogens) was the main methane contributor during the EGSB bioreactor operation, but other methylotrophic and hydrogen-dependent methanogens (Methanolinea spp., Methanoregula spp., and Methanosarcina spp.) also contributed to the total methane production.
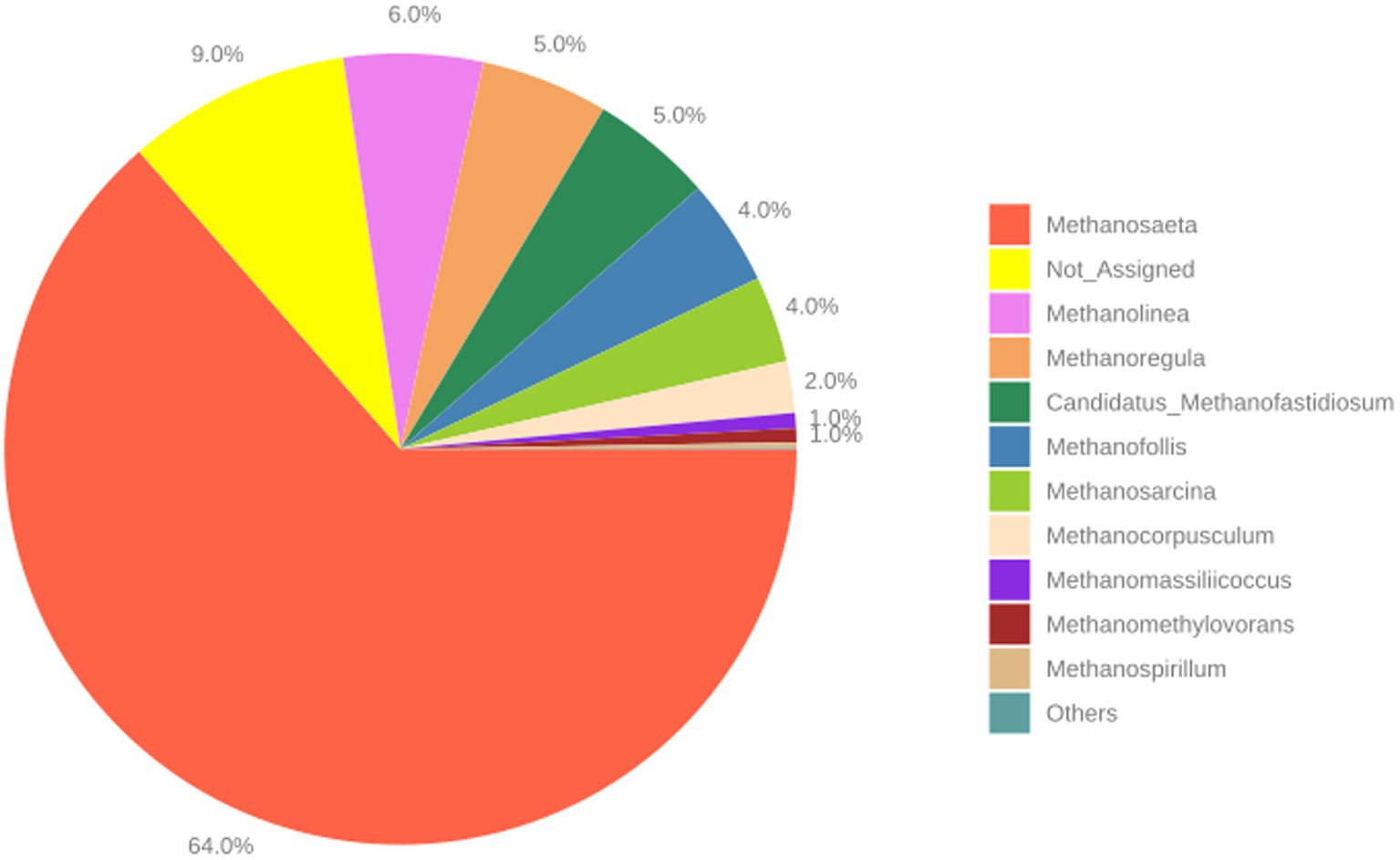
Figure 8. The most abundant genera of the archaea domain found in the EGSB bioreactor after 130 days of incubation. Values are the mean of three biological replicates.
4 Discussion
4.1 Cheese whey wastewater analysis
The CWW used here was generated from the cheese agro-industry and showed special characteristics that make its treatment and decontamination more difficult. Our CWW showed COD values of 73.33 g/L, BOD5 values of 60.73 g/L, and TOC values of 33.73 g/L. This high concentration of organic matter translates to a significant demand for dissolved oxygen in aquatic ecosystems, which can severely impact local municipal sewage treatment systems (Janczukowicz et al., 2008) and the natural environment. Furthermore, this CWW contained substantial levels of nutrients, such as TKN (4,836 mg/L), Ammonia-N (29.67 mg/L), Nitrate-N (6.27 mg/L), Nitrite-N (1.7 mg/L), and TP (1,676 mg/L). These high nutrient levels promote eutrophication in receiving waters, particularly in lakes and slow-moving rivers (Prazeres et al., 2012). Therefore, the discharge of this type of wastewater into bodies of water could cause various problems in water quality, such as rapid proliferation of toxic phytoplankton and algae, aesthetic pollution, loss of plant and animal diversity, alterations in abundance and distribution of microbial communities, oxygen depletion, and potential mortality of higher organisms due to neurotoxins (Prazeres et al., 2012; Osorio-González et al., 2018; Rincón-Catalán et al., 2022).
Moreover, CWW showed a pH of 4.33 and acidity of 3.6 gCaCO3/L, which could be harmful to aquatic life and organisms sensitive to pH changes. In addition, water acidification increases the solubility and availability of heavy metals such as aluminum, iron, and manganese, and makes them more ecotoxic if they accumulate in water and sediments (Lükewille et al., 1997; Eze et al., 2014). Taking all these characteristics and risk factors into account, if these wastes are not properly treated, CWWs can cause serious negative impacts when released freely into the natural environment.
On the other hand, this CWW showed great potential for treatment in EGSB anaerobic bioreactors due to its physicochemical profile and its suitability for application in various biotechnological processes (Cruz-Salomón et al., 2019). This conclusion is supported by key indicators such as a high BI (0.83) exceeding 0.3, low FWI (0.18) below 0.2, high CI (724) surpassing 10, favorable C/N relationship (1/15), high concentration of organic matter (84.9%), and high TVS/TS relation (0.85). Furthermore, CWW did not show inhibition by metal ions, as their concentrations fall below the recommended inhibitory levels (Parkin and Owen, 1986). Otherwise, metals such as iron (Fe), zinc (Zn), nickel (Ni), and copper (Cu) were presented at low concentrations in our CWW. At low concentrations, these metals enhance anaerobic digestion (Chen and Lee, 2014). Consequently, CWW is a suitable substrate for anaerobic treatment in EGSB bioreactors and has great potential for biogas production.
4.2 Anaerobic granular sludge characteristics and morphology analyses
The anaerobic granular sludge (AnGS) used in this project came from a UASB bioreactor. This AnGS showed biogranular characteristics, which are beneficial for EGSB bioreactors. Granular AnGS has higher sedimentability, more SMA, better TVS/TS ratio, and avoids cell washing compared to flocculant sludge. It has been proposed (Hulshoff et al., 2004) that an AnGS must present the following characteristics for use in upflow bioreactors: TS (50–250 g/L), TVS/TS ratio (0.3–0.85), SVL (<20 mL/g), and SMA (0.3–2.0 gCOD/gTVS day). Therefore, our AnGS has the appropriate characteristics and efficiency to be used in EGSB bioreactors. In addition, since the TVS/TS ratio is an indirect measure of the degree of biological activity of the inoculum and depends on the concentration of potential microbial substrates, the organic content must be higher than 50% to have high yields in biogas production (Liao and Li, 2015). Our AnGS had an organic content of 51%; therefore, it can be used as inoculum in EGSB bioreactors to produce high amounts of methane.
Sludge pH is one of the most important parameters influencing anaerobic digestion in bioreactors, since it affects both chemical reactions and the activity of microbial community in the sludge (Mirzoyan et al., 2008). Our sludge pH (7.45 ± 0.25) was a weak alkaline environment (commonly found in inoculums with a high organic matter content), which prevents excessive acidification, avoids microbial growth inhibition, and promotes the production of greater amounts of methane (Liu et al., 2016). In addition, anaerobic digestion operates efficiently under low ORP values (reducing conditions), typically between −200 mV and −400 mV (millivolts) (Gutu et al., 2021). However, other factors influence the variations of the ORP such as the type of feedstock, operating temperature, pH stability, and chemical composition of biogas produced. Our AnGS maintained the appropriate range of ORP values for microbial activity during the anaerobic digestion process. Finally, EC values are used as indicators of sludge salinity and are rarely discussed in the literature as a factor influencing methanogenesis in anaerobic bioreactors. The EC of our AnGS (7.01 ± 0.15 mS/cm) was moderately saline (4–8 mS/cm); however, it did not show any inhibitory effect on the anaerobic digestion process in our EGSB bioreactor.
4.3 EGSB bioreactor performance
The performance of an EGSB bioreactor is influenced by a variety of factors, such as wastewater characteristics, particle size distribution, acclimatization of the AnGS, environmental conditions (temperature and pH), as well as operational parameters such as OLR, HRT, SRT, and Vup (Cruz-Salomón et al., 2019; Wang et al., 2020). The pH within our EGSB bioreactor was 7.5 ± 0.2 over the course of 130 days of continuous operation. Different pH values (7.8 and 7.9) were recorded only during start-up and acclimatization steps. The addition of a pH stabilizer to EGSB bioreactors has been proposed to produce methane from wastewater with properties like CWW (Agbor et al., 2014; Ramos et al., 2020). Due to the low pH and alkalinity content of CWW and similar substrates, their addition to the bioreactor can exhaust the buffering capacity, leading acidification, volatile fatty acids (VFA) accumulation, excess of viscous extracellular polymeric materials, and subsequent bioreactor failure. In addition, the associated microorganisms could overproduce exopolysaccharides (biofilms) due to the high concentration of carbon and nitrogen sources, affecting the performance of the bioreactor and even clogging the circulating system (Mostafa-Imeni et al., 2019; Cruz-Salomón et al., 2020). Several compounds can be used to buffer the bioreactor and avoid these potential issues; among the most important are sodium bicarbonate (NaHCO3) calcium carbonate (CaCO3), and dipotassium phosphate (K2HPO4) (Osorio-González et al., 2022). Likewise, the control of this factor is crucial to achieving the economic feasibility of bioremediation and biogas production. Our results confirm that the use of NaHCO3 allowed the EGSB bioreactor to operate in stable conditions avoiding acidification and increasing the methane production and COD removal efficiency.
Temperature is also an important parameter for the efficient performance of anaerobic bioreactors (Mao et al., 2015). Our EGSB bioreactor maintained stable operation within the mesophilic range (26°C) over a 130-day period, which favored microbial metabolism, decomposition of organic matter, and methane bioproduction. While most studies have reported an optimal temperature of 35°C, our EGSB bioreactor showed a high organic matter removal efficiency (>89%) and substantial methane production (>335 mL CH4/gCOD) at 26°C. Since no heating system was used, operation and maintenance costs were lower.
The alkalinity ratio serves as a fundamental and dependable control parameter for assessing the stability of EGSB bioreactor and other anaerobic bioreactors. This parameter evaluates the interaction between the alkalinity of volatile fatty acids (VFA) and total alkalinity. Ideally, the appropriate range for this ratio is between 0.2 and 0.4 and indicates that a minimum of 60% of the total alkalinity within the system should be in the form of bicarbonate alkalinity. Lower values can cause a drop in pH, potentially inhibiting microbial activity and altering microbial metabolism, while higher values can indicate an excessive level of alkalinity, which can also negatively affect the process (Pérez and Torres, 2008). The alkalinity ratios of our EGSB bioreactor were tracked to evaluate its performance. Within the initial 24 days, our bioreactor displayed instability due to the start-up phase, reflected in alkalinity ratio values falling outside the range previously reported. The reduction in alkalinity ratio and stabilization were observed from day 42. Subsequently, our EGSB bioreactor continued to operate stably throughout the evaluation period.
The methane generated by our EGSB bioreactor is a renewable natural gas resulting from the anaerobic digestion of wastewater by a complex microbial community and under controlled conditions. This biogas can be used as biofuel or in the generation of heat and electricity, making it a compelling alternative energy source that could significantly reduce greenhouse gas (GHG) emissions. Our EGSB produced large amounts of methane (6,900 mLCH4/day) and removed significant amounts of organic matter (BMP: 335 mLCH4/gCOD). This substantial methane bioproduction can be largely attributed to the high biodegradability (0.83) of our CWW and its organic matter content (84.9%). This methane production is not only a consequence of the intrinsic characteristics of wastewater but is also influenced by the regulation of environmental factors during its operation, including temperature and pH, as well as key operating parameters such as OLR and HRT.
Furthermore, the BMP is defined as the volume of methane generated in relation to the amount of organic matter removed by biological activity. Therefore, BMP reflects the activity of the anaerobic microbial community (i.e., catabolism and fermentation) and depends on the proportion of biodegradable substances and the chemical composition of these compounds (Michaud et al., 2002). For anaerobic bioreactors, the theoretical maximum methane production is 350 mL of CH4/gCOD and can be computed based on the stoichiometry of methane produced from a mole of organic matter mineralization (Rincón-Catalán et al., 2022). The BMP value in our EGSB bioreactor was similar to previously reported values in other anaerobic bioreactors (Labatut et al., 2011; Mainardis et al., 2017; Rincón-Catalán et al., 2022).
Finally, the EGSB bioreactor is a low-cost and robust technology compared to other types of bioreactors. It operates using low nutrients and chemicals requirement and has low AnGS production and a compact design suitable for small spaces, with higher efficiency of organic matter removal and methane bioproduction compared to first- and second-generation anaerobic bioreactors (Cruz-Salomón et al., 2019). Due to these economic and operational advantages, this third-generation technology is highly recommended for the treatment of wastewater with a high concentration of biodegradable organic matter (BI>0.4 and COD >1,500 mgO2/L), such as CWW, with the beneficial concomitant production of methane and other biomolecules of commercial interest. Based on the results of this project, we recommend the use of the EGSB bioreactor as a low-cost alternative for efficient CWW treatment, linked to methane production as an additional benefit. Additionally, the characterization of the biochemical composition of the CWW and the microbial diversity associated with the EGSB bioreactor allowed us to better understand the microbial activity in the anaerobic system, nutrient recycling, and methane production.
4.4 Metabolic diversity of methane-producing archaea
Methane-producing archaea (methanogens) live in wide variety of environments and host-associated microbiomes (Moissl-Eichinger et al., 2018; Baker et al., 2020; Borrel et al., 2020) and have diverse evolutionary history and ecophysiological roles in nutrient cycles.
For many years, methanogens have been restricted to a metabolically specialized group of archaea, within the phylum Euryarchaeota; however, a new classification suggests that methanogens are grouped into three phyla: Euryarchaeota, Halobacterota, and Thermoplasmatota (Bräuer et al., 2020), being the In this work, we used SILVA ribosomal RNA database for the taxonomic assignment. Recently, the new species Candidatus Methanosuratincola yellowstonensis has been classified within the phylum Thermoproteota (Hatzenpichler et al., 2023), suggesting that the description of new archaeal species and their taxonomic assignment are changing and need to be constantly reviewed.
Independently of the taxonomy, so far, all methanogens belong to the domain Archaea. Methanogens have the unique ability to produce energy and methane through diverse methanogenesis pathways (electron transfer systems); however, around two-thirds of biomethane comes from the degradation of acetate (aceticlastic methanogenesis) in oxygen-free environments (Ferry, 2020). Methanogens are generally associated with strict anaerobic environments due to the high sensitivity to oxygen exposure of the enzymes involved in methanogenesis and the lack of genes encoding for proteins involved in protection against oxidative stress (Jasso-Chávez et al., 2015). Methanogens are unable to take carbohydrates as energy/carbon sources but use amino acids, peptone, pyruvate, formate, hydrogen/carbon dioxide, carbon monoxide, acetate, methanol, and other methylated compounds to grow.
Methanosaeta spp. was the most abundant genus found in our samples (from days 0 and 130). This acetate-utilizing methanogen uses the hydrogen-independent aceticlastic methanogenesis to grow and produce methane. Despite its slow growth and low biomass formation, Methanosaeta spp. shows a high affinity for acetate, and the final methane yield is directly related to the amount of acetate that is added to the culture (Smith and Ingram-Smith, 2007). In other words, about 100% of the carbon of the methyl group (CH3) of the acetate (CH3COO−) is used for the synthesis of methane, while the carbon of the carbonyl group (COO−) is used to produce biomass and others metabolic functions (Ferry, 2020).
Non-acetotrophic methanogens (Methanolinea spp. and Methanoregula spp.) can use carbon dioxide (CO2) and formate (HCOO−) as energy sources in hydrogen-dependent methanogenesis pathways. These microorganisms have been found in methanogenic sewage sludge, rice field soils, and anaerobic bioreactors (Imachi et al., 2008; Yashiro et al., 2011; Sakai et al., 2012). Methanosarcina spp. is the most metabolic diverse genus since species can use one or more energy sources, for example, acetate (CH₃COO−), methanol (CH3OH), methylamines (CH3NH2), and carbon dioxide (CO2), in hydrogen-dependent or independent methanogenesis pathways. These methanogens are usually found in sewage sludge, sediments from freshwater and marine environments, and soils (Smith and Ingram-Smith, 2007; Ferry, 2020).
The diversity and abundance of methanogens found in this work suggest a complex syntrophic interaction between bacteria and archaea. The results also help us understand the ecophysiological role of methanogens in nutrient recycling in the bioremediation of CWW. In addition, results suggest that there is no competition for food between bacteria, methanogens, and non-methanogenic archaea, due to the well-established difference in preference for energy and carbon sources.
4.5 Use of methanogenic archaea in bioprocesses
Many archaeal species have potential biotechnological applications, such as production of unusual or thermoresistant enzymes and unique metabolites (Amoozegar et al., 2017; Martínez-Espinosa, 2020; Zhao et al., 2020), biofuel production (e.g., bioethanol, methane, biodiesel, and other oils) (Carr and Buan, 2022), bioremediation of contaminated environments by heavy metals and other toxic compounds (Krzmarzick et al., 2018), and mitigation of global warming through carbon sequestration (McGlynn, 2017).
Diverse studies on methanogens and other archaea have suggested that the use of genetically modified microorganisms is not necessary to overproduce metabolites, since exposing them to stressful factors could be sufficient to induce the synthesis of biomolecules with commercial interests (Basen et al., 2014; Amoozegar et al., 2017; Zhao et al., 2020) as well as to modify the components of the culture media or use reactor-scale cultivation to improve the final yield of biomass (Mukhopadhyay et al., 1999; Palabikyan et al., 2022; Ngoumelah et al., 2023). For example, when exposed to stress conditions, Methanosarcina acetivorans increases the synthesis of metabolites, such as methane, HS-CoM (2-mercaptoethanesulfonate or Coenzyme M), polyphosphate, glycogen, and biofilms. In addition, pre-adaptation to stress conditions helps to increase the synthesis of metabolites (Jasso-Chávez et al., 2019). Methanosarcina spelaei RK-23, a recent methanogen isolated from anaerobic digestor, was the first methanogen reported with the unique ability to secrete bioflocculants (biosurfactants) (Zhao et al., 2020).
These examples suggest that in methanogens: (a) optimal conditions for the overproduction of metabolites could be different from the physiological growth conditions, (b) the ability of metabolite production depends on the environment from which the microorganism was isolated, and (c) biomolecule synthesis can be a protective or self-isolating mechanism against exposure to toxic components (sodium sulfide, high salinity, or low pH) common in biodigesters or natural environments.
Cultivation of microorganisms on small and large scales is a great tool to evaluate microbial physiology and metabolic diversity; however, the isolation and maintenance of new species remain a challenge and an experimental limitation. Recently, new metabolic predictions derived from genomic data of uncultured archaeal lineages have also helped to guide culture strategies (Adam et al., 2017; Hatzenpichler et al., 2020), for example when Candidatus Prometheoarchaeum syntrophicum strain MK-D1, an archaeon at the prokaryote–eukaryote interface, was isolated (Imachi et al., 2020).
Future comprehensive studies, including the detailed characterization of metabolic pathways, will provide a better understanding of microbial metabolism, especially oxygen-sensitive microorganisms such as anaerobic bacteria and methanogens. In addition, these studies could help to optimize and increase the biotechnological processes, using pre-adapted microorganisms or genetically modified strains in axenic cultures or microbial consortia.
5 Conclusion
Heterotrophic microorganisms (archaea and bacteria) can break down complex carbon sources into small molecules to grow, favoring syntrophic relationships with more specialized metabolism microorganisms such as anaerobic bacteria and methanogens. Genomic and physiological data from axenic cultures or natural microbial consortia guide and improve biotechnological processes, as well as help design synthetic microbial communities (SynComs) for more complex applications.
CWW can be treated in EGSB bioreactors by using these oxygen-sensitive microorganisms (methanogens and bacteria) and produce methane as a secondary metabolite. This low-cost strategy can be used for bioremediation, the production of biomass, biogas (methane and hydrogen), and other metabolites of commercial and biotechnological interest, and stimulate the enrichment of microorganisms of complex microbial communities.
Data availability statement
The raw data supporting the conclusions of this article will be made available by the authors, without undue reservation. The raw sequence data has also been deposited at the following link: https://drive.google.com/drive/folders/1EOQqNhsKbzFdWQqGvOHEOTWNNUKf4-1X?usp=sharing.
Author contributions
MD-E, AC-S and MGS-M planned the experiments. MD-E and JH-M performed the experiments. AC-S and MGS-M analyzed, interpreted the data, and wrote the draft manuscript. All authors contributed to the project and approved the final version of the manuscript.
Funding
This work was partially supported by UConn MCB StartUp funds (#4171510) and Scholarship Facilitation Fund (SFF #4656550) Award from UConn Office of the Vice President for Research to MGS-M.
Acknowledgments
The authors are grateful for the access granted to the laboratories of the Facultad de Ciencias de la Nutrición y Alimentos of the Universidad de Ciencias y Artes de Chiapas and the laboratory of the Escuela de Ciencias Químicas of the Universidad Autónoma de Chiapas, and Consejo Nacional de Humanidades, Ciencia y Tecnología (CONAHCyT). We would also like to thank the editor and reviewers for their suggestions to improve this manuscript.
Conflict of interest
The authors declare that the research was conducted in the absence of any commercial or financial relationships that could be construed as a potential conflict of interest.
Publisher’s note
All claims expressed in this article are solely those of the authors and do not necessarily represent those of their affiliated organizations, or those of the publisher, the editors and the reviewers. Any product that may be evaluated in this article, or claim that may be made by its manufacturer, is not guaranteed or endorsed by the publisher.
Supplementary material
The Supplementary material for this article can be found online at: https://www.frontiersin.org/articles/10.3389/fsufs.2023.1244691/full#supplementary-material
Footnotes
1. ^https://www.bioinformatics.babraham.ac.uk/projects/fastqc/
2. ^https://bioconductor.org/packages/release/bioc/html/microbiome.html
3. ^https://ggplot2.tidyverse.org/
References
Aboukila, E. F., Abdelraouf, E. A. A., and Gomma, I. (2018). Effects of cheese whey on some chemical and physical properties of calcareous and clay soils. Int. J. Plant Sci. 21, 1–12. doi: 10.1016/j.jssas.2016.09.005
Adam, P. S., Borrel, G., Brochier-Armanet, C., and Gribaldo, S. (2017). The growing tree of Archaea: new perspectives on their diversity, evolution and ecology. ISME J. 11, 2407–2425. doi: 10.1038/ismej.2017.122
Agbor, V., Carere, C., Cicek, N., Sparling, R., and Levin, D. (2014). “Biomass pretreatment for consolidated bioprocessing (CBP)” in Advances in biorefineries: biomass and waste supply chain exploitation Ed. K. W. Waldron (Amsterdam: Elsevier), 234–258.
Amoozegar, M. A., Siroosi, M., Atashgahi, S., Smidt, H., and Ventosa, A. (2017). Systematics of haloarchaea and biotechnological potential of their hydrolytic enzymes. Microbiology 163, 623–645. doi: 10.1099/mic.0.000463
AOAC. (2019). Official methods of analysis, 21th ed. Association of Analytical Chemists: Arlington, VA.
APHA. (2005). Standard methods for the examination of water and wastewater, 20th ed. American Public Health Association/American Water Works Association/Water Environment Federation: Washington, DC.
Asunis, F., De Gioannis, G., Isipato, M., Muntoni, A., Polettini, A., Pomi, R., et al. (2019). Control of fermentation duration and pH to orient biochemicals and biofuels production from cheese whey. Bioresour. Technol. 289:121722. doi: 10.1016/j.biortech.2019.121722
Baker, B. J., De Anda, V., Seitz, K. W., Dombrowski, N., Santoro, A. E., and Lloyd, K. G. (2020). Diversity, ecology and evolution of Archaea. Nat. Microbiol. 5, 887–900. doi: 10.1038/s41564-020-0715-z
Basen, M., Schut, G. J., Nguyen, D. M., Lipscomb, G. L., Benn, R. A., Prybol, C. J., et al. (2014). Single gene insertion drives bioalcohol production by a thermophilic archaeon. Proc. Natl. Acad. Sci. U. S. A. 111, 17618–17623. doi: 10.1073/pnas.1413789111
Borrel, G., Brugère, J. F., Gribaldo, S., Schmitz, R. A., and Moissl-Eichinger, C. (2020). The host-associated archaeome. Nat. Rev. Microbiol. 18, 622–636. doi: 10.1038/s41579-020-0407-y
Bräuer, S. L., Basiliko, N., Siljanen, H. M. P., and Zinder, S. H. (2020). Methanogenic archaea in peatlands. FEMS Microbiol. Lett. 367:fnaa172. doi: 10.1093/femsle/fnaa172
Cajacuri, M. P., Rincón, N., Araujo, I., Behling, E., Colina, G., and Marín, J. C. (2013). Microbiological diversity of the anaerobic sludge during treatment of Venezuelan oilfield produced waters. Ing. Invest. Tecnol. 14, 325–334. doi: 10.1016/S1405-7743(13)72247-9
Callahan, B. J., McMurdie, P. J., Rosen, M. J., Han, A. W., Johnson, A. J., and Holmes, S. P. (2016). DADA2: high-resolution sample inference from Illumina amplicon data. Nat. Methods 13, 581–583. doi: 10.1038/nmeth.3869
Carr, S., and Buan, N. R. (2022). Insights into the biotechnology potential of Methanosarcina. Front. Microbiol. 13:1034674. doi: 10.3389/fmicb.2022.1034674
Carvalho, F., Prazeres, A. R., and Rivas, J. (2013). Cheese whey wastewater: characterization and treatment. Sci. Total Environ. 445–446, 385–396. doi: 10.1016/j.scitotenv.2012.12.038
Chen, H. H., and Lee, A. H. I. (2014). Comprehensive overview of renewable energy development in Taiwan. Renew. Sust. Energ. Rev. 37, 215–228. doi: 10.1016/j.rser.2014.04.055
Chen, T., Zheng, P., Tang, C., Wang, S., and Ding, S. (2011). Performance of ANAMMOX-EGSB reactor. Desalination 278, 281–287. doi: 10.1016/j.desal.2011.05.038
Coelho, F., Teles de Faria, J., Fernandes da Silva, M., de Souza, P., Oliveira, R., and Converti, A. (2019). Cheese whey permeate fermentation by Kluyveromyces lactis: a combined approach to wastewater treatment and bioethanol production. Environ. Technol. 41, 3210–3218. doi: 10.1080/09593330.2019.1604813
Cruz-Salomón, A., Meza-Gordillo, R., Rosales-Quintero, A., Ventura-Canseco, C., Lagunas-Rivera, S., and Carrasco-Cervantes, J. (2017b). Biogas production from a native beverage vinasse using a modified UASB bioreactor. Fuel 198, 170–174. doi: 10.1016/j.fuel.2016.11.046
Cruz-Salomón, A., Ríos-Valdovinos, E., Pola-Albores, F., Lagunas-Rivera, S., Cruz-Rodríguez, R. I., Cruz-Salomón, K. D. C., et al. (2020). Treatment of cheese whey wastewater using an expanded granular sludge bed (EGSB) bioreactor with biomethane production. PRO 8:931. doi: 10.3390/pr8080931
Cruz-Salomón, A., Ríos-Valdovinos, E., Pola-Albores, F., Lagunas-Rivera, S., Meza-Gordillo, R., Ruíz-Valdiviezo, V. M., et al. (2019). Expanded granular sludge bed bioreactor in wastewater treatment. Glob. J. Environ. Sci. Manag. 5, 119–138. doi: 10.22034/gjesm.2019.01.10
Cruz-Salomón, A., Ríos-Valdovinos, E., Pola-Albores, F., Meza-Gordillo, R., Lagunas-Rivera, S., and Ruíz-Valdiviezo, V. M. (2017a). Anaerobic treatment of agro-industrial wastewaters for COD removal in expanded granular sludge bed bioreactor. Biofuel Res. J. 4, 715–720. doi: 10.18331/BRJ2017.4.4.3
Eze, V. C., Onwuakor, C. E., and Orok, F. E. (2014). Microbiological and physicochemical characteristics of soil contaminated with used petroleum products in Umuahia, Abia state, Nigeria. Appl. Environ. Microbiol. 2, 281–286. doi: 10.12691/jaem-2-6-3
FAOSTAT (2023). Crops and livestock products. Available at: https://www.fao.org/faostat/en/#data/QCL/visualize
Fernández-Rodríguez, C., Martínez-Torrez, E. J., Morán-Palao, A., and Gómez-Barrios, X. (2016). Biological treatments of cheese whey for biogas and hydrogen production. Revista Ion. 29, 47–62. doi: 10.18273/revion.v29n1-2016004
Ferry, J. G. (2020). Methanosarcina acetivorans: a model for mechanistic understanding of aceticlastic and reverse methanogenesis. Front. Microbiol. 11:1806. doi: 10.3389/fmicb.2020.01806
Ghaly, A. E., Mahmoud, N. S., Rushton, D. G., and Arab, F. (2007). Potential environmental and health impacts of high land application of cheese whey. Am. J. Agric. Biol. Sci. 2, 106–117. doi: 10.3844/ajabssp.2007.106.117
Gutu, L., Basitere, M., Harding, T., Ikumi, D., Njoya, M., and Gaszynski, C. (2021). Multi-integrated Systems for Treatment of abattoir wastewater: a review. Water 13:2462. doi: 10.3390/w13182462
Hatzenpichler, R., Kohtz, A., Krukenberg, V., Petrosian, N., Jay, Z., and Pilhofer, M. (2023). Cultivation and visualization of a methanogen of the phylum Thermoproteota. Research Square. Preprint.
Hatzenpichler, R., Krukenberg, V., Spietz, R. L., and Jay, Z. J. (2020). Next-generation physiology approaches to study microbiome function at single cell level. Nat. Rev. Microbiol. 18, 241–256. doi: 10.1038/s41579-020-0323-1
Hulshoff, L. W., de Castro, S., and Lettinga, P. (2004). Anaerobic sludge granulation. Water Res. 38, 1376–1389. doi: 10.1016/j.watres.2003.12.002
Imachi, H., Nobu, M. K., Nakahara, N., Morono, Y., Ogawara, M., Takaki, Y., et al. (2020). Isolation of an archaeon at the prokaryote–eukaryote interface. Nature 577, 519–525. doi: 10.1038/s41586-019-1916-6
Imachi, H., Sakai, S., Sekiguchi, Y., Hanada, S., Kamagata, Y., Ohashi, A., et al. (2008). Methanolinea tarda gen. Nov., sp. nov., a methane-producing archaeon isolated from a methanogenic digester sludge. Int. J. Syst. Evol. Microbiol. 58, 294–301. doi: 10.1099/ijs.0.65394-0
Janczukowicz, W., Zielinski, M., and De Bowski, M. (2008). Biodegradability evaluation of dairy effluents originated in selected sections of dairy production. Bioresour. Technol. 99, 4199–4205. doi: 10.1016/j.biortech.2007.08.077
Jasso-Chávez, R., Lira-Silva, E., González-Sánchez, K., Larios Serrato, V., Mendoza-Monzoy, D. L., Pérez-Villatoro, F., et al. (2019). Marine archaeon Methanosarcina acetivorans enhances polyphosphate metabolism under persistent cadmium stress. Front. Microbiol. 10:2432. doi: 10.3389/fmicb.2019.02432
Jasso-Chávez, R., Santiago-Martínez, M. G., Lira-Silva, E., Pineda, E., Zepeda-Rodríguez, A., Belmont-Díaz, J., et al. (2015). Air-adapted Methanosarcina acetivorans shows high methane production and develops resistance against oxygen stress. PLoS One 10:e0117331. doi: 10.1371/journal.pone.0117331
Jiménez-Hernández, J., Guerra-Rivera, G., Morgan-Sagastume, J. M., Noyola-Robles, A., Theuerl, S., and Klocke, M. (2014). Caracterización microbiológica y molecular de la comunidad anaerobia durante la co-fermentación de residuos agropecuarios con adición de arcillas para la obtención de metano. Rev. Cenic. Cien. Biol. 45, 10–17.
Kelly, B. J., Gross, R., Bittinger, K., Sherrill-Mix, S., Lewis, J. D., Collman, R. G., et al. (2015). Power and sample-size estimation for microbiome studies using pairwise distances and PERMANOVA. Bioinformatics 31, 2461–2468. doi: 10.1093/bioinformatics/btv183
Krzmarzick, M. J., Taylor, D. K., Fu, X., and McCutchan, A. L. (2018). Diversity and niche of archaea in bioremediation. Archaea 2018:3194108. doi: 10.1155/2018/3194108
Labatut, R. A., Largus, T. A., and Norman, R. S. (2011). Biochemical methane potential and biodegradability of complex organic substrates. Bioresour. Technol. 102, 2255–2264. doi: 10.1016/j.biortech.2010.10.035
Liao, X., and Li, H. (2015). Biogas production from low-organic-content sludge using a high-solids anaerobic digester with improved agitation. Appl. Energy 148, 252–259. doi: 10.1016/j.apenergy.2015.03.082
Liu, C., Li, H., Zhang, Y., and Liu, C. (2016). Improve biogas production from low-organic-content sludge through high-solids anaerobic co-digestion with food waste. Bioresour. Technol. 219, 252–260. doi: 10.1016/j.biortech.2016.07.130
Lopes, H. J. S., Ramos, L. R., and Silva, E. L. (2017). Co-fermentation of cheese whey and crude glycerol in EGSB reactor as a strategy to enhance continuous hydrogen and propionic acid production. Appl. Biochem. Biotechnol. 183, 712–728. doi: 10.1007/s12010-017-2459-7
Lükewille, A., Jeffries, D., Johannessen, M., Raddum, G., Stoddard, J., and Traaen, T. (1997). Transboundary air pollution. International cooperative programmed on assessment and monitoring of acidification of Rivers and lakes. The nine-year report: acidification of surface water in Europe and North America – Long-term developments (1980s and 1990s). NIVA-report n° 3637–97:168.
Mainardis, M., Cabbai, V., Zannier, G., Visintini, D., and Goi, D. (2017). Characterization and BMP tests of liquid substrates for high-rate anaerobic digestion. Chem. Biochem. Eng. Q. 31, 509–518. doi: 10.15255/CABEQ.2017.1083
Martínez-Espinosa, R. M. (2020). Heterologous and homologous expression of proteins from Haloarchaea: denitrification as case of study. Int. J. Mol. Sci. 21:82. doi: 10.3390/ijms21010082
McGlynn, S. E. (2017). Energy metabolism during anaerobic methane oxidation in ANME archaea. Microbes Environ. 32, 5–13. doi: 10.1264/jsme2.ME16166
McMurdie, P. J., and Holmes, S. (2013). Phyloseq: an R package for reproducible interactive analysis and graphics of microbiome census data. PLoS One 8:e61217. doi: 10.1371/journal.pone.0061217
Michaud, S., Berneta, N., Buffiére, P., Roustan, M., and Moletta, R. (2002). Methane yield as a monitoring parameter for the start-up of anaerobic fixed film reactors. Water Res. 36, 1385–1391. doi: 10.1016/S0043-1354(01)00338-4
Mirzoyan, N., Parnes, S., Singer, A., Tal, Y., Sowers, K., and Gross, A. (2008). Quality of brackish aquaculture sludge and its suitability for anaerobic digestion and methane production in an upflow anaerobic sludge blanket (UASB) reactor. Aquaculture 279, 35–41. doi: 10.1016/j.aquaculture.2008.04.008
Mao, C., Feng, Y., Wang,, and Ren, G. (2015). Review on research achievements of biogas from anaerobic digestion. Renewable and sustainable energy reviews. 45, 540–555. doi: 10.1016/j.rser.2015.02.032
Moissl-Eichinger, C., Pausan, M., Taffner, J., Berg, G., Bang, C., and Schmitz, R. A. (2018). Archaea are interactive components of complex microbiomes. Trends Microbiol. 26, 70–85. doi: 10.1016/j.tim.2017.07.004
Mostafa-Imeni, S., Pelaz, L., Corchado-Lopo, C., Busquets, A. M., Ponsá, S., and Colón, J. (2019). Techno-economic assessment of anaerobic co-digestion of livestock manure and cheese whey (cow, Goat & Sheep) at small to medium dairy farms. Bioresour. Technol. 291:121872. doi: 10.1016/j.biortech.2019.121872
Mukhopadhyay, B., Johnson, E. F., and Wolfe, R. S. (1999). Reactor-scale cultivation of the hyperthermophilic methanarchaeon Methanococcus jannaschii to high cell densities. Appl. Environ. Microbiol. 65, 5059–5065. doi: 10.1128/AEM.65.11.5059-5065.1999
Nettmann, E., Bergmann, I., Pramschüfer, S., Mundt, K., Plogsties, V., Herrmann, C., et al. (2010). Polyphasic analyses of methanogenic archaeal communities in agricultural biogas plants. Appl. Environ. Microbiol. 76, 2540–2548. doi: 10.1128/AEM.01423-09
Ngoumelah, D. D., Harnisch, F., Sulheim, S., Heggeset, T. M. B., and Haugnes, I. (2023). A unified and simple medium for growing model methanogens. Front. Microbiol. 13:1046260. doi: 10.3389/fmicb.2022.1046260
Official Mexican Environmental Regulations. NOM-001- SEMARNAT-1996, Comisión Nacional del Agua. Comisión Nacional del Agua: Mexican institution. (2015). Available at: https://www.conagua.gob.mx/CONAGUA07/Publicaciones/Publicaciones/SGAA-15-13.pdf.
Osorio-González, C. S., Gómez-Falcon, N., Brar, S. K., and Ramírez, A. A. (2022). Cheese whey as a potential feedstock for producing renewable biofuels: a review. Energies 15:6828. doi: 10.3390/en15186828
Osorio-González, C. S., Sandoval-Salas, F., Hernández-Rosas, F., Hidalgo-Contreras, J. V., Gómez-Merino, F. C., and Ávalos de la Cruz, D. A. (2018). Potential for the use of cheese whey in Mexico. Agroproductividad 11, 101–106. doi: 10.3168/jds.2015-9388
Palabikyan, H., Ruddyard, A., Pomper, L., Novak, D., Reischl, B., and Simon, K. M. R. (2022). Scale-up of biomass production by Methanococcus maripaludis. Front. Microbiol. 13:1031131. doi: 10.3389/fmicb.2022.1031131
Parkin, G. F., and Owen, W. F. (1986). Fundamentals of anaerobic digestion of wastewater sludges. Int. J. Environ. Eng. 112, 867–920.
Pérez, A., and Torres, P. (2008). Alkalinity indices for control of anaerobic treatment of readily acidifiable wastewaters. Ingen. Compet. 10, 41–52. doi: 10.25100/iyc.v10i2.2473
Pires, A. F., Marnotes, N. G., Rubio, O. D., Garcia, A. C., and Pereira, C. D. (2021). Dairy by-products: a review on the valorization of whey and second cheese whey. Foods 10:1067.1. doi: 10.3390/foods10051067
Prazeres, A. R., Carvalho, F., and Rivas, J. (2012). Cheese whey management: a review. Environ. Manag. 110, 48–68. doi: 10.1016/j.jenvman.2012.05.018
Ramos, L. R., de Menezes, C. A., Soares, L. A., Sakamoto, I. K., Amâncio-Varesche, M. B., and Silva, E. L. (2020). Controlling methane and hydrogen production from cheese whey in an EGSB reactor by changing the HRT. Bioprocess Biosyst. Eng. 43, 673–684. doi: 10.1007/s00449-019-02265-9
Ramos, L. R., and Silva, E. L. (2017). Improving EGSB reactor performance for simultaneous bioenergy and organic acid production from cheese whey via continuous biological H2 production. Biotechnol. Lett. 39, 983–991. doi: 10.1007/s10529-017-2323-4
Rincón-Catalán, N. I., Pérez-Fabiel, S., Mejía-González, G., Herrera-López, D., Castro-Chan, R., Cruz-Salomón, A., et al. (2022). Power generation from cheese whey treatment by anaerobic digestion and microbial fuel cell. Waste Biomass Valor 13, 3221–3231. doi: 10.1007/s12649-022-01720-1
Sakai, S., Ehara, M., Tseng, I. C., Yamaguchi, T., Bräuer, S. L., Cadillo-Quiroz, H., et al. (2012). Methanolinea mesophila sp. nov., a hydrogenotrophic methanogen isolated from rice field soil, and proposal of the archaeal family Methanoregulaceae fam. Nov. within the order Methanomicrobiales. Int. J. Syst. Evol. Microbiol. 62, 1389–1395. doi: 10.1099/ijs.0.035048-0
SILVA. High quality ribosomal RNA databases. (2023). Available at: https://www.arb-silva.de/.
Smith, K. S., and Ingram-Smith, C. (2007). Methanosaeta, the forgotten methanogen? Trends Microbiol. 15, 150–155. doi: 10.1016/j.tim.2007.02.002
Sutera, A. M., Arfuso, F., Tardiolo, G., Riggio, V., Fazio, F., Aiese Cigliano, R., et al. (2023). Effect of a co-feed liquid whey-integrated diet on crossbred pigs’ fecal microbiota. Animals 13:1750. doi: 10.3390/ani13111750
Tirado, L., Gökkuş, Ö., Brillas, E., and Sirés, I. (2018). Treatment of cheese whey wastewater by combined electrochemical processes. J. Appl. Electrochem. 48, 1307–1319. doi: 10.1007/s10800-018-1218-y
van den Berg, L., Pronk, M., van Loosdrecht, M. C. M., and de Kreuk, M. K. (2023). Density measurements of aerobic granular sludge. Environ. Technol. 44, 1985–1995. doi: 10.1080/09593330.2021.2017492
Wang, W. K., Ni, C. H., Guo, Y. J., Lin, Y. C., and Chun-Te Lin, J. (2020). Long-term performance and metagenomic analysis of full-scale anaerobic granular sludge bioreactors for low aerobically-biodegradable synthetic fiber manufacturing wastewater treatment. Int. Biodeterior. Biodegradation 153:105046. doi: 10.1016/j.ibiod.2020.105046
Wongburi, P., and Park, J. K. (2022). Prediction of sludge volume index in a wastewater treatment plant using recurrent neural network. Sustainability 14:6276. doi: 10.3390/su14106276
World Health Organization. Guideline for discharge of industrial effluent characteristics. WHO, Geneva (1995).
Wu, G., and Yin, Q. (2020). Microbial niche nexus sustaining biological wastewater treatment. npj Clean Water. 3:33. doi: 10.1038/s41545-020-00080-4
Xia, T., Gao, X., Wang, C., Xu, X., and Zhu, L. (2016). An enhanced anaerobic membrane bioreactor treating bamboo industry wastewater by bamboo charcoal addition: performance and microbial community analysis. Bioresour. Technol. 220, 26–33. doi: 10.1016/j.biortech.2016.08.057
Yashiro, Y., Sakai, S., Ehara, M., Miyazaki, M., Yamaguchi, T., and Imachi, H. (2011). Methanoregula formicica sp. nov., a methane-producing archaeon isolated from methanogenic sludge. Int. J. Syst. Evol. Microbiol. 61, 53–59. doi: 10.1099/ijs.0.014811-0
Keywords: archaea, bacteria, syntrophy, methane, methanogenesis, EGSB-bioreactor, cheese whey, bioremediation
Citation: Domínguez-Espinosa ME, Cruz-Salomón A, Ramírez de León JA, Hernández-Méndez JME and Santiago-Martínez MG (2023) Syntrophy between bacteria and archaea enhances methane production in an EGSB bioreactor fed by cheese whey wastewater. Front. Sustain. Food Syst. 7:1244691. doi: 10.3389/fsufs.2023.1244691
Edited by:
Xiaowu Huang, Guangdong Technion-Israel Institute of Technology (GTIIT), ChinaReviewed by:
Seung Gu Shin, Gyeongsang National University, Republic of KoreaYaoyu Zhou, Hunan Agricultural University, China
Copyright © 2023 Domínguez-Espinosa, Cruz-Salomón, Ramírez de León, Hernández-Méndez and Santiago-Martínez. This is an open-access article distributed under the terms of the Creative Commons Attribution License (CC BY). The use, distribution or reproduction in other forums is permitted, provided the original author(s) and the copyright owner(s) are credited and that the original publication in this journal is cited, in accordance with accepted academic practice. No use, distribution or reproduction is permitted which does not comply with these terms.
*Correspondence: Abumalé Cruz-Salomón, YWJ1bWFsZS5jcnV6QHVuYWNoLm14; Michel Geovanni Santiago-Martínez, Z2VvX3NhbnRpYWdvbUB1Y29ubi5lZHU=