- 1Department of Food Science, College of Agriculture and Veterinary Medicine, United Arab Emirates University, Al-Ain, United Arab Emirates
- 2Zayed Center for Health Science, United Arab Emirates University, Al-Ain, United Arab Emirates
Mungbean and pumpkin are rich source of proteins and nutrients which could be utilized in novel food formulations. This study involves formulation of meat analog using mungbean protein isolate (MBPI) and pumpkin protein isolates (PPI) through optimization process using Box–Behnken Design (BBD) of response surface methodology (RSM). MBPI and PPI were used as base ingredients for the development of meat alternatives using an innovative heat-induced gelation process. Methylcellulose (MC) and gum Arabic were used as supporting matrices for obtaining desired texture of the meat analog. The emulsifying activity, water-holding capacity, and oil-holding capacity of MBPI and PPI were analyzed. The set of physicochemical response factors used in RSM was moisture content, protein content, color, and textural properties of the formulated meat analogs. The selected independent variables were set at three levels (−1, 0, 1) with protein ratio (20:10, 15:15, and 10:20 of MBPI-PPI), Water (32, 37, and 42%), and MC (5, 6, and 7%). RSM results showed that the model effectively described the correlation between the independent variables (protein ratio, water percentage, and MC percentage) and the response factors. The microstructure of the analog showed porous and fibrous structures. It was observed that the degree of cross-linking between protein molecules could have impacted the textural properties that were associated with viscoelastic characteristics as reflected in the rheological analysis. Overall, the study shows that the mungbean and pumpkin seed proteins could be utilized as a potential ingredient to improve the textural properties of the meat analog, while it is also recommended to explore such proteins with other mechanical processing techniques like extrusion.
1. Introduction
Recently, increasing numbers of consumers globally are adopting plant-based diets as a substitute for traditional animal-based foods due to the negative impact of animal-based foods, on human health and the environment (He et al., 2020; Singh et al., 2021). Consuming processed red meat has been connected to health concerns, particularly those related to coronary artery disease, cancer, and cardiovascular disease, with putative processes related to the amount of saturated fat, cholesterol, iron, phosphatidylcholine, and carnitine in the meat (Herz et al., 2021). In addition, apprehensions towards the ethical issues associated with animal welfare is on a rapid rise. Due to these concerns, there have been more studies in recent times on exploring plant-based ingredients as alternative sources to formulate meat alternatives (Yuliarti et al., 2021). Currently, plant proteins are employed most frequently to create meat substitutes, which are typically used as isolates and concentrates in powdered form. According to research by Gu et al. (2022) eating plant-based meals high in polyunsaturated fatty acids, oligosaccharides, and dietary fiber greatly lowers the risk of obesity and cardiovascular illnesses. Additionally, consuming more plant-based proteins (particularly those found in legumes) instead of red meat may lower the chance of developing type II diabetes (Gu et al., 2022). To create a balanced overall amino acid profile, producers of plant-based foods may either employ mixtures of proteins (such as legume and cereal proteins) or may supplement their products with the essential amino acids that are absent in the plant protein. One of the most effective approaches for developing a functional plant-based diet is the fabrication and restructuring of plant proteins to resemble the textural properties of meat, which can greatly reduce the ubiquitous health complications linked with red meat consumption.
Plant-based protein composites known as “meat analogs (MAs)” generally contain ingredients from non-meat sources and are designed into a matrix to imitate the textural and organoleptic characteristics of animal meat. Several studies reported the production of meat analogs using different plant proteins having nutritive and techno-functional properties such as soy (Chiang et al., 2019), pea (Zhu et al., 2021), rice (Lee et al., 2022), oat (De Angelis et al., 2020), peanut (Rehrah et al., 2009). The formation of a fibrous structure within the plant protein matrix is the basis for maintaining the unique juiciness and chewiness often sought after in animal meat. However, restructuring plant protein ingredients is one of the challenges in terms of MAs production (Palanisamy et al., 2018). Interestingly, different approaches including protein ingredient combinations, physical structuring techniques, and inclusion of gelling ingredients can be employed to overcome this challenge (Dekkers et al., 2018; Singh et al., 2021). Heat-induced gelation of proteins involves the linkage of non-polar surface groups through hydrophobic interactions. The heating of proteins causes the globular protein molecules to unfold and exposes the non-polar surface groups (Herz et al., 2021). Plant proteins mostly comprise of globular proteins (Mcclements and Grossmann, 2021). Formation of protein gel matrix in the presence of polysaccharides like pectin (Moll et al., 2023), guar gum (Nanta et al., 2021), and products produced from cellulose such as MC (Bakhsh et al., 2021), could help in the restructuring of proteins. MC is a hydrophilic cellulose derivative that consists of 1,4-β-D-glucan monomers, in which-OH is partially replaced by CH2COOH groups (Michelin et al., 2020). It is widely utilized due to its structuring, thickening, or gelling ability in the aqueous phase. It can operate as an emulsifier in oil-in-water emulsions (Pirsa and Hafezi, 2022). These characteristics of MC attracted researchers to investigate its potential in creating robust texture and elasticity in MAs, in recent years. Furthermore, the formation of protein-rich gels through the interactions with MC, to provide improved fibrous texture of the MAs is well documented (Bakhsh et al., 2021; Zhao et al., 2021; Taghian Dinani et al., 2023).
Moreover, the appropriate selection of protein source is critical in impacting the desirable textural properties of the MAs which consequently plays an important role in the final structure and mouthfeel of the MAs. Therefore, it is pivotal to select a plant protein with excellent functionality to mimic conventional animal proteins. While soybean meal and wheat gluten have been widely used as the main plant-based protein ingredients for “MAs” because of their high-quality essential amino acids that are bioavailable for human nutrition (Kumar et al., 2022), but these ingredients have certain drawbacks eg. allergic proteins (Ozturk et al., 2023). For this reason, exploring novel proteins that can replace existing sources in the production of high-quality MAs is the utmost.
Mungbean and pumpkin seed proteins are currently being used as preferred protein ingredients in the food industry (Shrestha et al., 2023) due to their functionality, economical, and sustainable features. Hence, proteins from mungbean and pumpkin might have a huge potential in developing meat analogs. Numerous benefits of mungbean protein isolate (MBPI) have been demonstrated in processed foods, in terms of foaming, emulsifying, and water-absorbing properties (Du et al., 2022). However, its potential for meat analogs is scarcely explored. Pumpkin seeds have a protein content between 31.5 and 51%, making them also a potential source for plant-based meat substitutes (Batool et al., 2022). Even though there is a plethora of knowledge regarding the functional aspects of different plant-derived proteins, the functional characteristics of pumpkin seed protein fractions are not well understood and their application in the fabrication of MAs has not been explored so far (Vinayashree and Vasu, 2021). Considering all these factors, the design of this study was centered on the use of mungbean and pumpkin seed protein isolates along with MC to produce potential MAs.
2. Materials and methods
2.1. Materials
Food-grade mungbean protein (Munptein™ with 80% protein content) and pumpkin seed protein (PumpteinX™, with 74% protein content) isolates were obtained from ET protein (Xinping Street, Suzhou, China). The ingredients and chemicals including potato starch, methylcellulose (MC), gum arabic, and calcium chloride were procured from Sigma-Aldrich, Inc., United States. The sunflower oil (refined), salt, and baking powder were procured from the local market of Al Ain, United Arab Emirates (UAE).
2.2. Estimation of functional properties of protein isolates
2.2.1. Emulsifying activity
The methodology described by O'sullivan et al. (2016) was adopted with slight modifications. Five sets of protein solutions were prepared by briefly dissolving 300.0 mg of protein isolates in 30.0 mL deionized water (1% protein equivalent) and the pH was adjusted to 2, 4, 6, 8, and 10 using 1 M HCl and 1 M NaOH. The resulting solutions were blended with 10.0 mL sunflower oil and homogenized using a high-speed homogenizer (ULTRA TURRAX® T 25 digital homogenizer IKA®-Werke GmbH & Co., Staufen, Germany) at 20,500 rpm for 1 min at room temperature to form emulsions. Subsequently, a 50.0 μL aliquot (avoidance of the supernatant foam) was then taken carefully from the bottom of each tube by a micropipette and mixed with 5 mL of 0.1% SDS solution immediately. The absorbance of the mixture, which indicated the emulsifying ability, was determined at 500 nm (A0) (Multiskan Sky, ThermoFisher Scientific, United States). Each sample was prepared in duplicate and each of these duplicates was measured three times. The emulsion activity index (EAI) was calculated using the following formula stated by Pearce and Kinsella (1978):
2.2.2. Water and oil holding capability
The water and oil holding capacity of the protein isolates were established using the following procedure. In brief, 1.0 g of protein (W0) was placed in centrifugal tubes and weighed together (W1). Then, 10 mL of distilled water or oil was added to the tube and vortexed. The solution was shaken at room temperature for 1 h. After standing at ambient temperature for 30 min, the tube was centrifuged at 5000× g for 20 min. The supernatant was decanted and the tube with sediment was weighed (W2). Water holding capability (WHC) and oil holding capability (OHC) were calculated as:
2.3. Experimental design and optimization of meat analog preparation
Design expert software (version 13.0, Stat Ease Inc., Minneapolis, United States) was applied to determine the optimum ingredients for the preparation of meat analogs. The key ingredients namely protein, water, and polysaccharides concentrations can significantly affect the structure and final quality of meat analogs. Thus, the effect of protein ratio [mung bean protein isolate (MBPI): pumpkin protein isolate (PPI)], water, and MC concentrations to obtain a desired texture is important to explore. The selected independent variables were set at three levels (−1, 0, 1) with protein ratio (20:10, 15:15, and 10:20 of MBPI-PPI), Water (32, 37, and 42%), and MC (5, 6, and 7%). In total, 15 experimental runs identified as low (−1), medium (0), and high (1) including three central points were carried out to optimize the three independent variables as shown in Table 1. For this experiment, color, hardness, springiness, chewiness, moisture, and protein content of the meat analogs were used as dependent variables or response factors. Multiple linear regression analysis of the runs done in triplicate was performed to obtain the regression coefficients following a second-order polynomial model.
Where Y represents the dependent variables, β0 is the intercept, β1, β2, and β3 are the linear regression coefficient, β12, β13, and β23 represent the 2-way interactions, whereas β11, β22, and β33, represent the quadratic coefficients. The generated 3D surface plots from the polynomial equation were used to interpret the correlation between the dependent variables and each independent variable, i.e., protein, water, and MC.
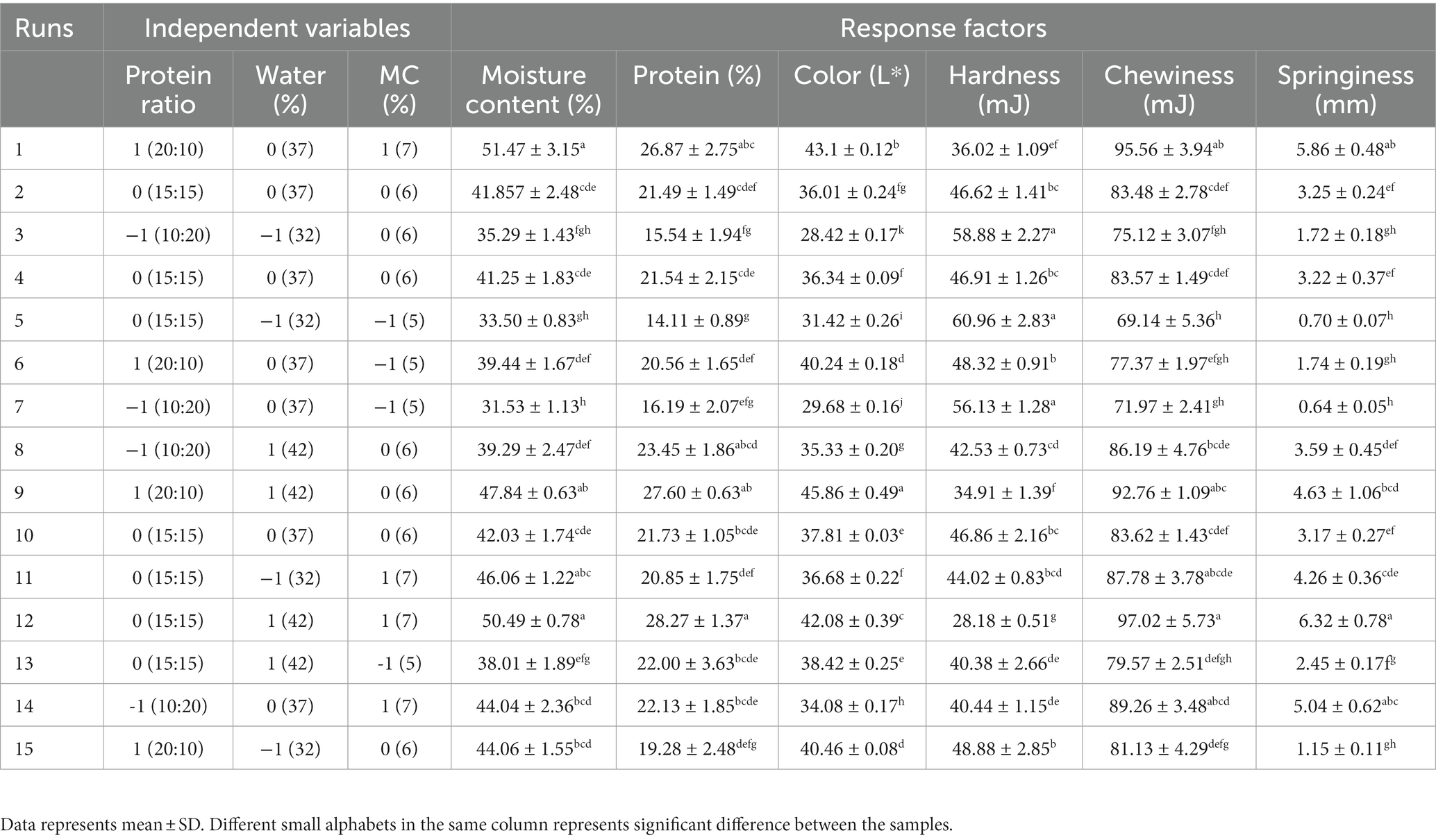
Table 1. Box–Behnken experimental design for optimization of meat analog and the output obtained in the form of different response factors.
2.4. Preparation of meat analog (MAs)
The MAs were prepared according to a modified protocol described by Yuliarti et al. (2021). Herein, each formulation of MA (100.0 g) contains ice-cold water, different MBPI-PPI ratios, potato starch, sunflower oil, calcium chloride, salt, baking powder, MC, and gum arabic in different combinations according to BBD (Table 1). The protein and MC emulsions were prepared separately in a food processor (Kenwood Multi-Functional 750 W, FDP03.COWH, China) for 3 min. The protein (MBPI and PPI)-based emulsion was prepared using proteins, baking powder, calcium chloride solution, salt, potato starch, and ice-cold water. Similarly, MC emulsion was prepared by mixing MC powder, soybean oil, and ice-cold water. Thereafter, protein and MC emulsions were combined and thoroughly homogenized for an additional 3 min to obtain a uniform emulsion. The obtained batter was then shaped into a mold with the dimensions: 4.0 cm × 3.0 cm × 2.5 cm (L × W × H) and afterward steamed at 100°C for 15 min. The analog was immediately frozen at −20°C for 48 h before further analysis.
2.5. Physicochemical properties of meat analogs (MAs)
2.5.1. Moisture content
The moisture content of all samples was determined using an oven-dry method. Briefly, 3.0 g of sample was sliced, transferred into pans, and placed in an oven at 103°C for at least 16 h until constant weight was attained. The percentage of initial moisture content in terms of wet basis (%MCinitial) was calculated after cooling in a desiccator using Eq. 5:
2.5.2. Total protein content
The total protein content was measured by the Kjeldahl method as described by AOAC International. The amount of total nitrogen in the raw materials was multiplied with a conversion factor of 6.25 to determine the total protein content.
2.5.3. Color
Color measurements of the interior cross-section from the analog were analyzed using a colorimeter (Konica Minolta CR-400, Tokyo, Japan). The color evaluation was expressed based on the Commission International de l’Eclairage (CIE) system and described as L*, a*, and b*. Measurements were taken at three differing points on the cross-section of each of the MA samples.
2.5.4. Texture profile analysis
The texture profile analysis (TPA) of MAs was determined using a texture analyzer (CT3, Brookfield Engineering Laboratories, Middleboro, USA) according to the protocol described by Yuliarti et al. (2021) with some modifications. Here an analog with dimensions 4.0 cm × 3.0 cm × 2.5 cm (L × W × H) was loaded onto the platform of a texture analyzer. The center of the analog was compressed twice to 40% from the original height using a cylindrical probe (diameter 7 mm) at a speed of 5.0 mms−1 at room temperature. TPA parameters including hardness, chewiness, and springiness were recorded using force vs. time plots.
2.6. Rheological properties
According to a previously described procedure, the viscoelasticity attribute was analyzed as per the method described by Zhu et al. (2021). A rheometer (HR-2, TA Instruments, Newcastle, United States) fitted with a parallel plate geometry (diameter: 40.0 mm) and a 1.0 mm gap was used to measure the rheological parameters at a temperature of 25°C. A spatula was used to carefully deposit 2.0 g of the MA sample on the bottom Peltier plate. Frequency sweeps (0.1–100 rad s−1) in the viscoelastic linear domain at a 1.0% strain were conducted to determine the rheological properties of the samples and the storage modulus G′ and loss modulus G′′ were recorded.
2.7. Microstructure
Scanning Electron Microscope (SEM) (JEOL scanning electron microscope, model: JSM-6010PLUS/LA, Tokyo, Japan) was used to determine the microstructure of the MAs using the method described by Yuliarti et al. (2021). Specifically, fresh analog was cut into small pieces (2–3 mm in thickness) and then solidified with liquid N2. Frozen samples were fixed with 2.5% glutaraldehyde in 0.2 M phosphate buffer, pH 7.2 for 12 h. Thereafter, the samples were rinsed with distilled water 3 times consecutively for 15 h followed by dehydration in a serial ethanol solution (50% for 15 min with 2 times, 70% for 15 min with 2 times, 80% for 15 min with 2 times, 90% for 15 min with 2 times, 100% for 30 min). The samples were placed in the vacuum chamber of SEM and images were recorded at a voltage of 20 kV at 100X magnification.
2.8. Statistical analysis
All experiments were carried out in triplicate and average values with standard deviation were reported. The data were subjected to one-way analysis of variance (ANOVA) using SPSS 24.0 software (SPSS INC., Chicago, IL, United States, 2002), and the mean values were compared using Tukey’s test (p < 0.05). Differences between the different meat analog samples were considered significant at p < 0.05. The RSM data was analyzed using design expert software (trial version 13.0, Stat Ease Inc., Minneapolis, United States). Analysis of variance (ANOVA) was applied to determine the linear regression, quadratic coefficients, and interactions. The coefficient of estimation of R2, the adjusted coefficient of determination (adjusted R2), and the predicted coefficient of determination (predicted R2) based on the polynomial equations were estimated at 95% (p < 0.05) significant levels.
3. Result and discussion
3.1. Protein functional properties
3.1.1. Emulsifying activity index (EAI)
EAI is often measured to determine the interfacial area emulsified per gram by an emulsifier (Jia et al., 2020). In the present study, the EAI of MBPI and PPI were measured as a function of pH (2.0, 4.0, 6.0, 8.0, and 10.0), and the results are shown in Figures 1A,B. Overall, the EAI of both proteins (MBPI and PPI) displayed similar magnitude as a function of pH, in which the intermediate EAI values were observed at pH 2.0 and 4.0, the lowest value at pH 6.0, and the highest values were recorded at pH 10 for both PPI and MBPI. However, the EAI values recorded for PPI at different pH were significantly lower compared to MBPI. For instance, the EAI of PPI at pH 2.0 and pH 4.0 were 7.23 and 7.7%, respectively– which decreased significantly at pH 6.0 but increased at pH 8.0 and 10.0, respectively.
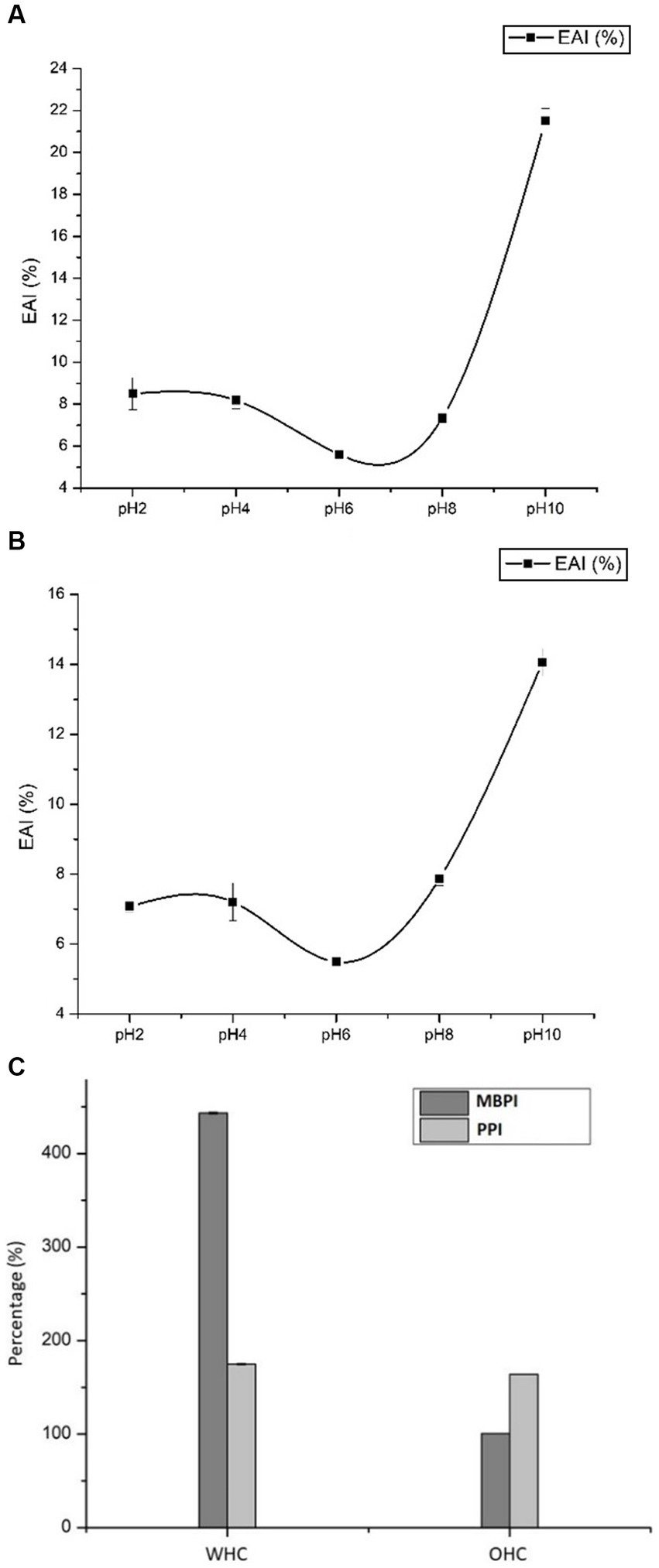
Figure 1. EAI of MBPI (Mung bean protein isolate) solutions (A) and PPI (Pumpkin protein isolate) solution (B) as a function of pH. (C) WHC and OHC of MBPI and PPI. Data were obtained in triplicates (n = 3) and represented in percentage (%). EAI, Emulsifying Activity Index; WHC, Water Holding Capacity; OHC, Oil Holding Capacity.
Overall, at extreme acidic and alkaline pH, better EAI was demonstrated, while poor EAI was shown at pH 6.0 suggesting that EAI of both MBPI and PPI were pH dependent. Similar results were observed earlier by Shevkani et al. (2015) for the EIA of kidney beans and field pea proteins. Notably, EAI was maximum at pH 10.0 in both substrates, which indicated that alkaline pH improved the emulsifying properties of legume proteins significantly. The highest EAI at pH 10.0 for isolated mung bean and soybean proteins was also reported by Samard and Ryu (2019), and for field pea isolates by Shevkani et al. (2015). As displayed in Figures 1A,B, EAI value considerably declined as the pH increased from 4.0 to 6.0, most likely due to a reduction in electrostatic repulsion between proteins because the pH was close to their isoelectric point (PI); (the pI of MBPI is 4.6 and for PPI is 5) (Zhang et al., 2009). Moreover, the protein aggregation is usually highest at pH near PI, which causes the development of large aggregates that requires more time for migration, thus, decreasing their ability to re-arrange and adsorb at the oil–water interface– resulting in low EAI (Lam and Nickerson, 2015).
It is not surprising that extremely acidic or alkaline pH substantially improved the EAI of MBPI and PPI because, at these pH values, the protein undergoes partial unfolding due to intramolecular repulsions between similar charges which in turn provide greater surface activity to these proteins (Jiang et al., 2018). Findings from this work were consistent with Tan et al. (2021), who showed that the EAI of soy protein isolates could be improved by extreme pH treatments. Such an increase in EAI is attributed to the induced structural changes, enhanced exposure of hydrophobic sites, and peptide chain flexibility initiated by alkaline pH. Noteworthy, substrates (MBPI and PPI) investigated in this study demonstrated significant EAI, making them potential ingredients for various food applications.
3.1.2. Water and oil holding capacity
The water-holding capacity (WHC) and oil-holding capacity (OHC) provide a measure of water/oil interactions with proteins and the water/oil retention capacity of proteins (Ge et al., 2021). Generally, WHC is associated with other functionalities like gelation, solubility, and emulsifying properties. Therefore, WHC could have a substantial impact on creating texture, flavor, and mouthfeel of the products (Ge et al., 2021). The WHC and OHC of MBPI and PPI are expressed as percentages (%), and the obtained data are presented in Figure 1C.
The highest WHC of 443.37% was observed in MBPI, which was almost 2.5 times higher than the WHC of PPI (174.80%). The protein hydrophobicity, conformation, amino acid composition, and the amount of protein present in the isolates could explain the observed differences in the WHC (Vinayashree and Vasu, 2021). Since, MBPIs consisted majorly of vicilin-type 8S, which have low molecular weight proteins and low surface hydrophobicity; thus, they might have displayed better WHC than PPIs that contained legumins-type 11S and 2S albumins. Additionally, vicilin conformation consists of a higher degree of unfolding and flexibility of polypeptide in the tertiary structure (Tang and Sun, 2011), allowing much greater surface area available for the protein-water interaction, and this might have improved the WHC of MBPI. Interestingly, the WHC value recorded for MBPI in this study was higher than pea protein (3.389 g water/g protein), and wheat proteins (1.376 g water/g protein), but less than that reported for soybean protein isolates (5.168 g water/g protein) according to Zhao et al. (2020).
The OHC results revealed that PPI exhibited a significantly higher value (163.93%) than that of MBPI (100.57%) as shown in Figure 1C. Interaction of oil molecules with more exposed hydrophobic groups on the PPI surface due to a conformational change of the protein, could be one possible reason for higher OHC of PPI. Miedzianka et al. (2021) have reported that the exposure of lipophilic groups in pumpkin protein during processing plays a crucial role in enhancing its OHC. Hence, we can postulate that exposure to the hydrophobic site of PPI facilitates the binding and/or interactions with oil molecules. The OHC of both, PPI and MBPI were consistent with those reported for soy, faba, and pea proteins with values ranging between 1.1–1.7 g/g (Ge et al., 2021). Overall, the WHC and OHC of MBPI and PPI indicated that these proteins can bind significant amounts of both water and oil.
3.2. The response of ingredient combinations on developed meat analogs
The effect of different ingredients combinations, protein ratio (X1), water % (X2), and MC % (X3) on response factors (Moisture content (MC), protein content (PC), color, hardness, springiness, and chewiness) of developed MAs are presented in Table 1. Non-linear trends in the responses as a function of ingredient combination were noticed with significant differences.
3.2.1. Changes In moisture content (MC)
As illustrated in Table 1, the moisture content values of different ingredient combinations of MAs were found to vary from 31.53 to 51.47%. The highest moisture content of 51.47% was reported in the MAs when high-level of X1 (20:10 of MBPI-PPI), medium level of X2 (37%), and high level of X3 (7 g) was used, while lowest moisture content was observed with low level of X1 (10:20 of MBPI-PPI), medium level of X2 (37%), and low level of X3 (5 g). A similar moisture content in the formulated MAs was reported by Chiang et al. (2019) soy protein-wheat gluten-based MAs.
Xia et al. (2023) studied the fibrous properties of yeast protein based MAs at different moisture and temperature and suggested the strengthening of fibrous structures at a temperature of 180°C with 55% moisture content. Based on the regression model, the moisture content of MAs in our study was linearly correlated to (Table 2) linear effects of protein ratio, water, and MC, which were found to be significant (p < 0.05). Conversely, interactive, and quadratic effects of ingredient combinations did not show a significant effect (p > 0.05). In the model, the coefficient of determination value (R2) presented a higher value than 0.997 which showed that the model was adequate. Also, the predicted R2 value (0.985) and adjusted R2 (0.994) were close to unity, suggesting the competence of the developed model to estimate the variation in the experimental test. The relationship between the moisture content of the meat analog and the coded value of the combination of the ingredients is given in the equation (Eq. 6).
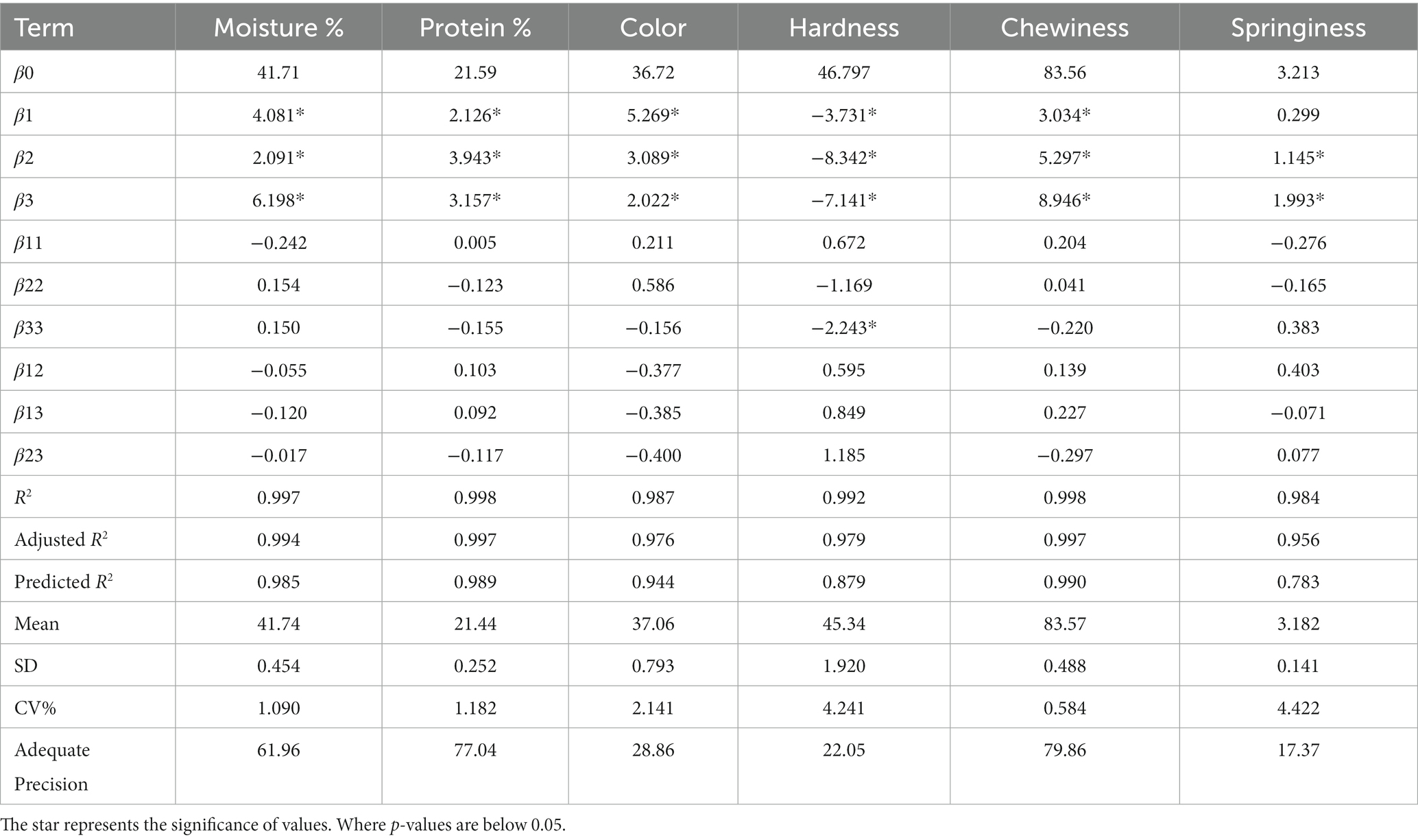
Table 2. Regression coefficients values estimated for ingredients combinations and responses of meat analog.
As shown in Eq. 6, the positive coefficient of all the linear terms of the independent variables indicated that they positively contributed to the moisture content of MAs. The Maximum positive coefficient (6.198) of X3 indicates that the amount of methylcellulose (MC) had a comparatively higher contribution to the moisture content than the protein ratio (X1) and the percentage of water (X2). Furthermore, the effects of ingredient combinations on moisture content are shown in Figures 2A–C. Notably, moisture content increased correspondingly with an increase in ingredient combinations (i.e., protein ratio, water, and MC). Ferawati et al. (2021) studied the high moisture MAs prepared by faba bean and yellow pea protein isolates and suggested a low moisture requirement of faba bean protein compared to yellow pea protein. It was noticed that the moisture content of MAs displayed a slight increase when the protein ratio and percentage of water were set to high levels while the percentage of MC was fixed at medium level (Figure 2A). However, further increment in the moisture content was found while maintaining medium levels of protein ratio or water percentage as illustrated in Figures 2B,C. This shows that when a high concentration of MC was used in MA formulation, then higher moisture content was retained in the MAs. This observation is attributable to the water-binding capacity of the MC, especially during the heating process. The water-binding capacity of the polysaccharides is due to the abundant hydroxyl groups that can form hydrogen bonds with water molecules (Dekkers et al., 2016).
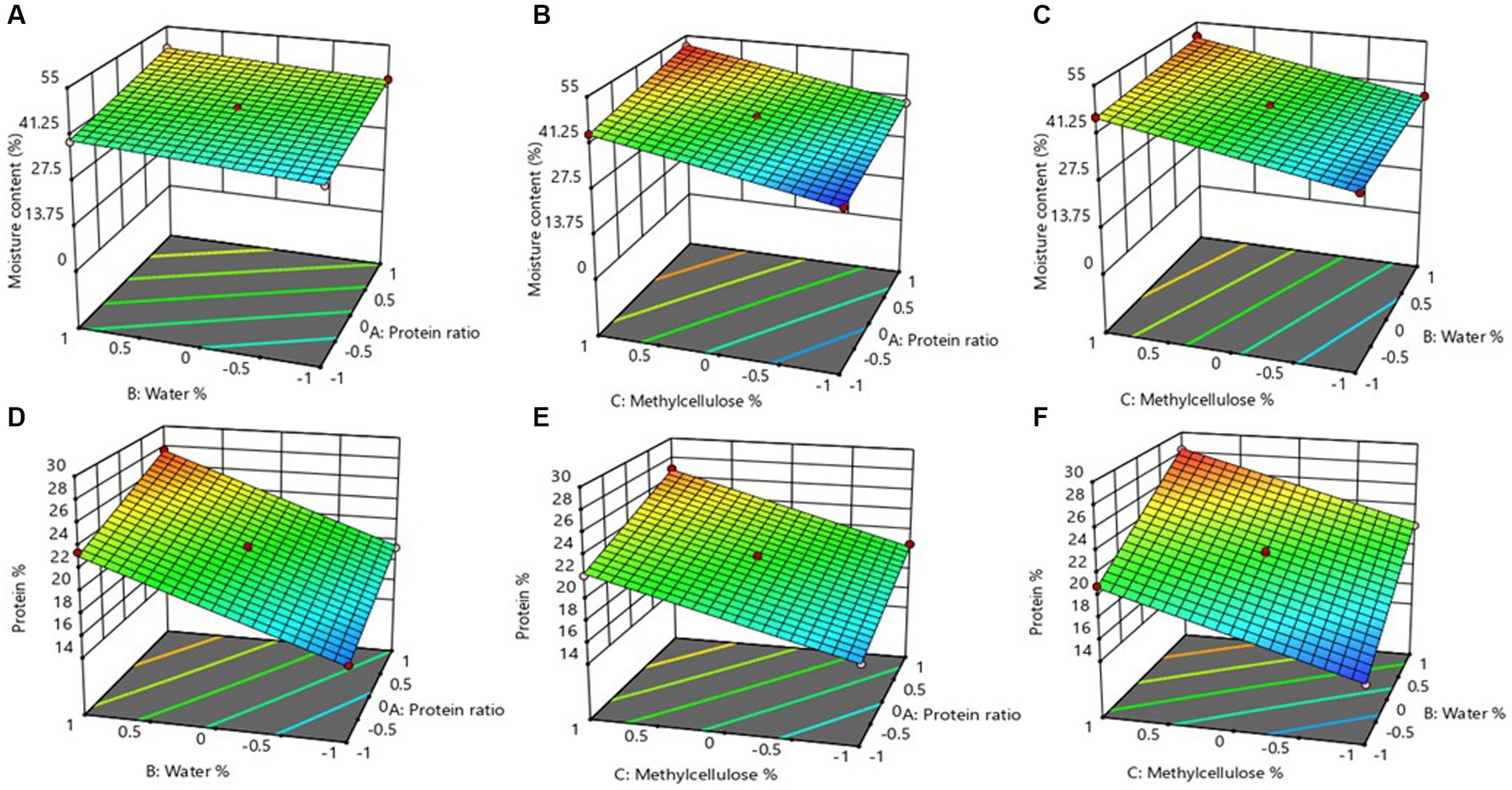
Figure 2. Response surface 3D plots showing the combined effect of water % and protein ratio (A), methylcellulose % and protein ratio (B), and methylcellulose % and water % (C) on the moisture content of meat analog. While the 3D plots displaying the combined effect of water % and protein ratio (D), methylcellulose % and protein ratio (E), and methylcellulose % and water % (F) on the final protein content % of the meat analog.
3.2.2. Protein content (PC)
Proteins are essential for giving MAs their characteristic texture, nutritional value, and organoleptic qualities (Kumar et al., 2022). The combination of different ingredients have an impact on the formation of MAs which depends on the source of protein and processing technique (Kyriakopoulou et al., 2021). Chiang et al. (2019) have reported the protein content of MAs extruded with soy protein-wheat gluten to be in the range of 25.38–26.76%. In this study the PC of MAs in relation to change in X1, X2, and X3 factors ranged from 14.11–28.27% (Table 1). The regression model showed that PC was significantly impacted (p < 0.05) by protein ratio, water percentage, and MC percentage. However, the interaction and quadratic effects of ingredient combinations were not statistically significant (p > 0.05) as displayed in Table 2. The model demonstrated a non-significant lack-of-fit along with a high coefficient of determination (R2 = 0.998) and predicted R2 of 0.997, which were practically in agreement with the adjusted coefficient of determination (R2 = 0.989), indicating the accuracy and adequacy of the model.
The regression equation for describing the correlation between PC and ingredients combination (coded) is given below (Eq. 7).
Herein, all three independent factors showed positive coefficients as evident in the equation above, which suggests the positive contribution of these three factors on the PC of MAs. The highest contribution was shown in the case of factor X2, followed by X3, and the lowest contribution on PC was shown by X1. Response surface plot (Figures 2D–F) shows that at medium-level water and high levels of the other two variables (i.e., protein ratio and percentage of MC), the PC of MAs displayed a declining trend. With the percentage of water set at a maximum level (42%), the PC experienced an increasing trend which can be easily observed in the Figure 2D. Based on the results, high PC was more pronounced in MAs formulated with ingredients combination containing 42% of water, regardless of the protein ratio. It is important to note that the PC for MAs observed in this study was higher than the median PC (14.0%) of all plant-based MAs, reviewed by Cutroneo et al. (2022). Therefore, the combination of the ingredients used in this study resulted in the MAs with sufficient PC, which is a crucial factor when formulating MAs.
3.2.3. Color
The Color attribute of MAs is an essential quality attribute since it mainly influences consumer’s perception and acceptance. Combinations of ingredients have an impact on the luminosity function of MAs, which affects how bright or dark the products are as well as how contrast or gradation effects that mimic meat are produced. The luminance function is one of the characteristics that may be utilized to define the color of MAs (De Angelis et al., 2020; Boukid, 2021). Therefore, instrumental color measurement was conducted to quantify the samples using a colorimeter. Purposely, L* values, a luminosity function was measured to reflect the lightness and/or darkness of the MAs (Table 1). The obtained value of the L* of MAs ranged from 28.42–45.86, the maximum value was observed with the combination of the high level of X1 (20:10 of MBPI-PPI), high level of X2 (42%), and a medium level of X2 (6 g), whereas minimum value was noted in a low level of X1 (10,20 of MBPI-PPI), low level of X2 (32%), and a medium level of X3 (6 g). The results generated from the analysis of variance for dependent variables are presented in Table 2. The L* was significantly affected linearly by protein ratio (p = 0.000), percentage of water (p = 0.000), and percentage of MC (p = 0.001). Moreover, there were no statistically significant interactive and quadratic effects between the independent variable (p > 0.05).
The results generated from the analysis of variance for dependent variables are presented in Table 2. It showed the model coefficients which confirmed the significance of the model (p < 0.05) and that the model is well fitted with a lack of fit p-values of 0.741. The non-significant lack of fit p-values indicated that the model effectively described the correlation between the independent variables (protein ratio, water percentage, and MC percentage) and the dependent variables. Moreover, the R2 of the model was 0.987, and the adjusted R2 of 0.976 were quite comparable to the predicted R2 = 0.944 generated for a model which further confirmed the significance of the model.
The following equation (Eq. 8) depicts the color (L*) response as influenced by the independent variables (X1, X2, and X3).
The independent variables show a linear positive relationship with L* values, according to the regression equation. A higher coefficient (5.269) of X1 indicates that L* depended mainly on the linear effect of variable X1 whereas X3 had a lower contribution. This indicates that the color parameters of formulated MAs may be primarily dependent on the plant protein source used for MAs preparation and other ingredients incorporated in the formulation.
The L* increased when higher levels of MBPI were incorporated into the PPI blend (20:10) as shown in Figures 3A,B. Conversely, blending an equal ratio of the two proteins significantly decreased the L*. This suggests that a higher fraction of MBPI could significantly increase the L* (lightness) of the MAs, which is attributable to the innate yellow color of the mung bean protein (Wen et al., 2022). Therefore, the color of the MAs can be tailored by adjusting the amount of MBPI. The values for L* reported in this study were lower than those reported by Chiang et al. (2019) and Yuliarti et al. (2021), who reported L* values above 55 in plant-based MAs. Moreover, the lower L* values observed indicate a reduction in luminosity; the development of dark color in MAs may be advantageous to emulate animal-based meat. In general, dark-brown MAs are often preferred over those with vivid colors (Cho et al., 2020). A study published by Ye et al. (2022) explained that the formation of brown pigments can mimic the color of cooked animal muscle (meat).
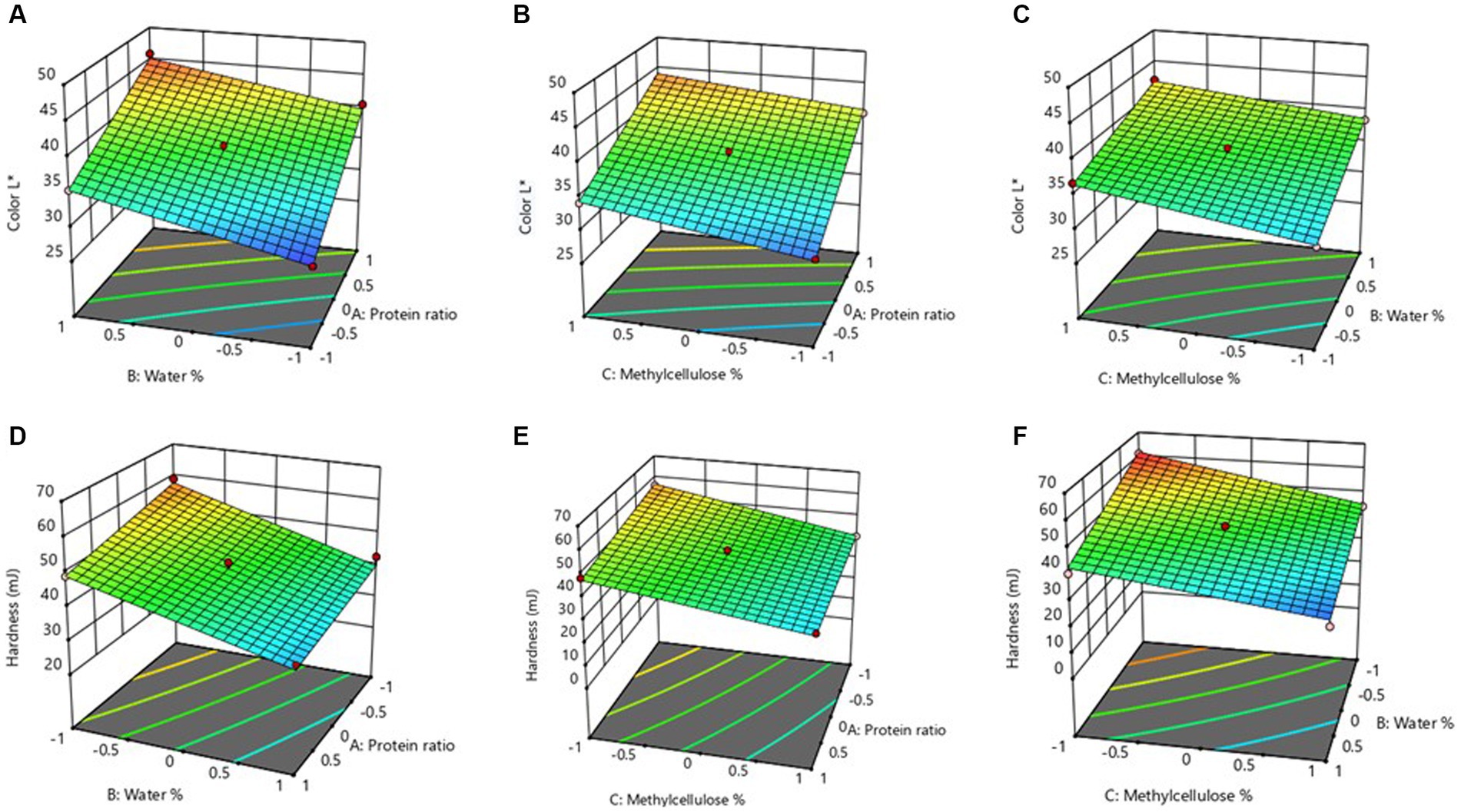
Figure 3. Response surface 3D plots showing the combined effect of water % and protein ratic (A), methylcellulose % and protein ratio (B), and methylcellulose % and water % (C) on the L* values of the meat analog. While the 3D plots displaying the combined effect of water % and protein ratio (D); methylcellulose % and protein ratio (E), and methylcellulose % and water % (F) on the hardness of the meat analog.
3.2.4. Texture
The texture is a vital quality indicator that provides more insight into the surface characteristics of MAs. The texture profile of MAs, which mimics the physical characteristics of meat, such as firmness, juiciness, chewiness, tenderness, and mouthfeel, is significantly influenced by the various ingredient combinations, such as proteins, polysaccharides, oils etc. (Godschalk-Broers et al., 2022). Accordingly, some characteristics of texture including hardness, chewiness, and springiness were examined by a texture analyzer to provide an adequate description of the texture profile of MAs in this study. As shown in Table 1, obtained values ranged from 28.18–60.96 mJ, 69.14–97.02 mJ, and 0.64–6.32 mm for hardness, chewiness, and springiness, respectively (Table 1). The maximum hardness (60.96 mJ) corresponded to the sample containing a medium level of X1, low level of X2, and X3 whereas the minimum hardness value (28.18 mJ) was obtained from an ingredient combination containing a medium level of X1, high level of X2 and X3. The coefficient of determination R2 and adjusted R2 were used to verify the robustness of the model. The hardness, chewiness, and springiness R2 were more than 0.90, which implied the model explained over 90% of all variations in the data. For hardness, significant linear effects (p < 0.05) of protein ratio, percentage of water, and percentage of MC were observed. The percentage of MC was found to have significant quadratic (p < 0.05) effects on hardness (Table 2).
The regression model (Eq. 9) describes the relationship between hardness and coded value of ingredients after neglecting the non-significant terms.
It is seen from Eq. 9 that the negative coefficient of linear terms of protein ratio (X1), percentage of water (X2), and percentage of MC (X3) had a negative influence on the hardness. A high coefficient of X2 (8.342) suggested that it had a maximum contribution to hardness. The negative coefficient of the quadratic term of X3 signified that their interaction was responsible for the decrease in the hardness of MAs. As the percentage of water decreased within the ingredient combinations, hardness increased and vice versa (Figures 3D–F). Previous findings have demonstrated that the addition of high amounts of water could disadvantageously decrease hardness (Chen et al., 2010). Response surface plots showed hardness increased by decreasing the water content (Figure 3D). Similarly, decreasing the amount of MC from 7 to 5 g and increasing the PPI ratio in the protein blends of MBPI-PPI increased the hardness of MAs when the water content was kept constant (Figures 3E,F). It is worth mentioning that reducing the water and the MC levels could make MAs brittle–and hence beneficial to the formation of the intended fibrous structure.
In terms of chewiness and springiness, maximum values of 97.03 mJ and 6.32 mm, respectively were recorded when the ingredient combination containing medium level of X1, high level of X2, and X3 was used. The protein ratio, percentage of water, and percentage of MC showed significant linear effects on chewiness (p < 0.05), whereas springiness was not significantly impacted by linear effects of protein, water, and MC ratio (p > 0.05) as displayed in Table 2. The protein ratio of equal blends of MBPI-PPI along with increased concentrations of water and MC significantly improved the chewiness (Figures 4A–C), and springiness (Figures 4D–F) of the MAs.
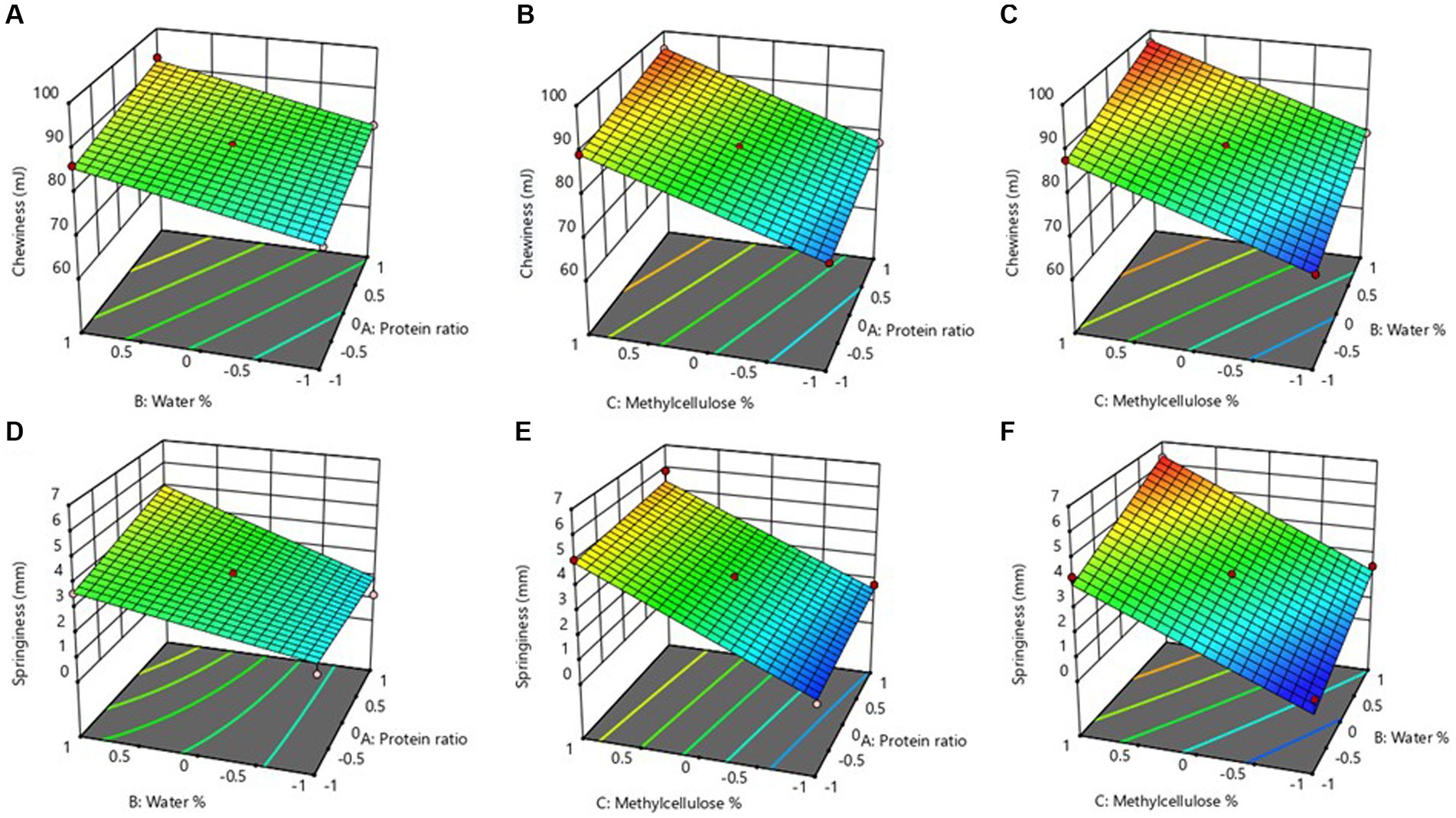
Figure 4. Response surface 3D plots showing the combined effect of water % and protein ratio (A), methylcellulose % and protein ratio (B), and methylcellose % and water % (C) on the Chewiness of the meat analog. While the 3D plots displaying the combined effect of water and protein ratio (D), methylcellulose 96 and protein ratio (E), and methylcellulose % and water % (F) on the Springiness of the meat analog.
The relationship between the ingredients combination, chewiness, and springiness of MAs are given in Eqs 10, 11, respectively.
The equations (Eqs 10, 11) describe the positive contribution of X1, X2, and X3 on chewiness and springiness as indicated by the positive coefficients presented. Besides, the coefficient of X3 demonstrated that its contribution is greatest on both texture attributes (chewiness and springiness). The effect of MA concentrations on chewiness and springiness was further in line with the previous results reported by Bakhsh et al. (2021) supporting the fact that increasing MC concentration could effectively improve the texture parameters of MAs. This was also supported by the study of Arora et al. (2017), where increasing the binding agents led to the formation of harder gels within the formulation, resulting in the improved texture of the mushroom-based sausage analog. In addition, the binding ability of different ingredients used in fabricating the MAs plays a vital role in the product’s final structure (Bakhsh et al., 2021).
Therefore, in this study, the amount of water and MC might be the two major ingredients that impacted the textural properties of MAs which imply that appropriate percentages of water and/or MC are necessary for the formation of the fibrous structure. Conversely, the protein ratio of MBPI-PPI showed limited impact on analog texture.
3.3. Optimizing the ingredients for the MAs
The responses were optimized using RSM to attain the MAs with desirable quality, based on the selected variables. Table 3 showed that according to the predicted values, all three independent variables should be set at the highest level to produce MAs with maximum protein content (30.62%), moisture content (53.95%), color L* (46.58), hardness (27.47 mJ), springiness (7.002 mm), and chewiness (100.9 mJ). The generated predicted values were comparable to the actual results with values of protein content (28.27%), moisture content (51.47%), color L* (45.86), hardness (28.18 mJ), springiness (6.317 mm), and chewiness (97.02 mJ), which verify the high reproducibility and reliability of all models evaluated in this study. Furthermore, the desirability function (DF) of optimized models is commonly used to validate the generated models (Mostafa et al., 2022). In this present study, all six models displayed a DF value of 1 which indicates an extremely desirable response and further validated the models.
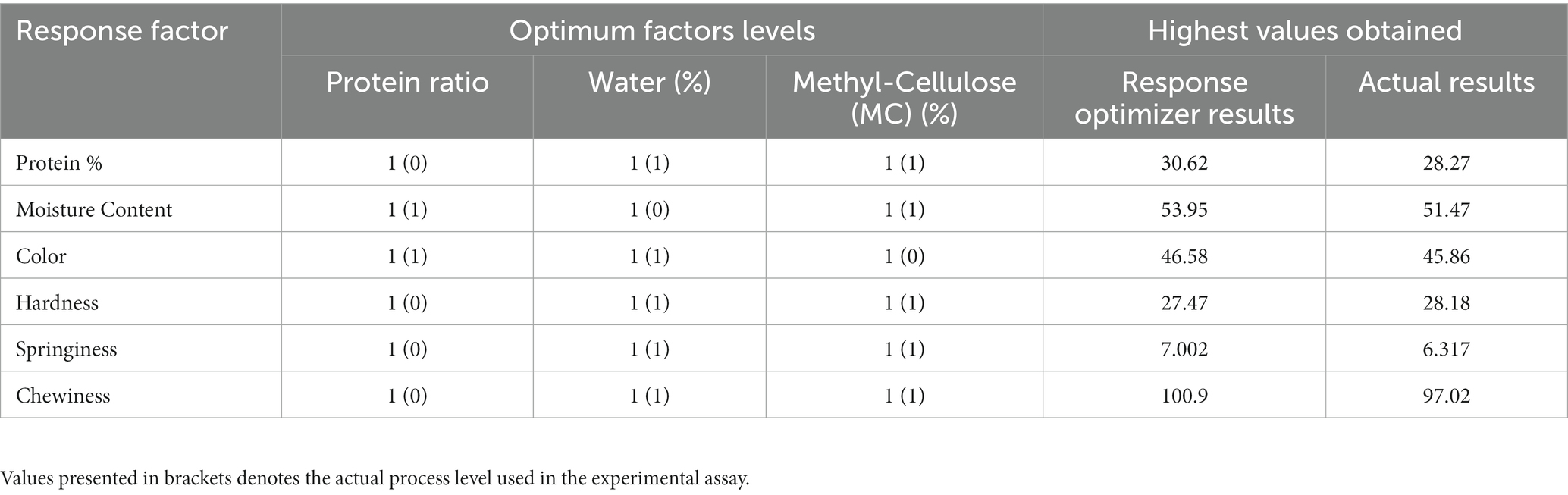
Table 3. Optimization of meat analog and validation of predicted and experimental values under optimum conditions.
3.4. Viscoelastic properties
The rheological properties of optimized MAs (i.e., ingredients combination of high-level protein ratio, high water content, and high MC content) were determined by frequency sweeps to obtain more information on the viscoelastic properties as a response to variation in frequency (Figures 5A,B). The loss modulus G” correlated to the viscous nature of the network and the storage modulus G’ showed elastic properties which are similar to solid-like characteristics (Yuliarti et al., 2021). Figure 5A illustrated that the G′ and G″ did not show any significant change at lower amplitude whereas at higher amplitude the G′ and G″ cross each other which caused change in the network because G″ dominated G′. Furthermore, frequency sweep measurements showed that both loss and storage moduli increased for the sample as the frequency increased (Figure 5B). Notably, G′ dominated over G″ throughout the experiential frequency range, suggesting the dominance of the elastic nature of the analog. Interestingly, this study has shown that the analog developed with higher ratios of MBPI to PPI, high water content, and MC content demonstrated less viscous, but a greater elastic response. This implies that the applied energy was stored in the interior network and not dissipated.
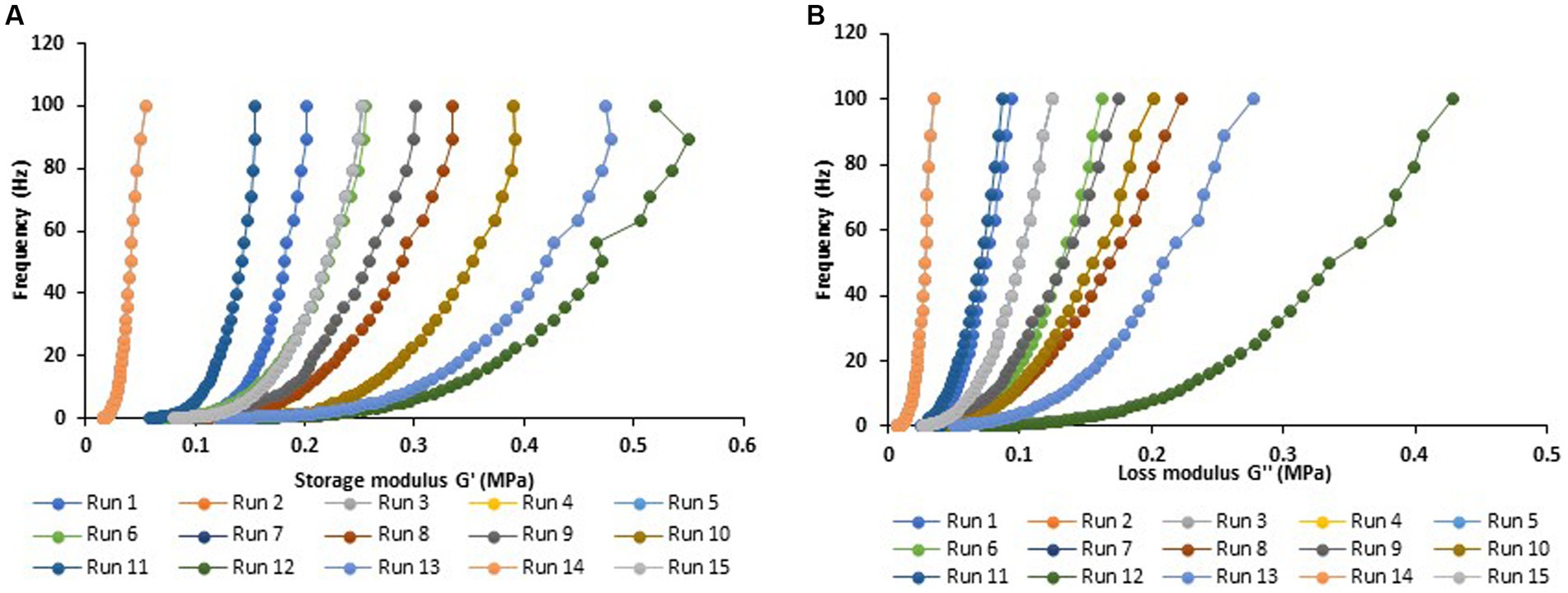
Figure 5. The viscoelastic measurements of MBPI + PPI meat analog with frequency sweeps from 0.1 to 100 rad s−1 and 1% linear domain (A). Storage modulus G′ showing the clastic nature of analog and (B). loss modulus G″ as function of the change in frequency (0 to 100 Hz) Run 1 to Run 15 are according to the Box—Behaken experimental design table (Table 1).
The incorporation of more MBPI fractions in the protein ratio formulation had resulted in increased moduli (G′ and G″), indicating increased protein gel strength, which would expedite a sufficient cross-link network. This indicates that MBPI could contribute more towards strengthening of the structure of the MAs, and it could be attributed to its high protein content which led to a higher ability to easily develop gel, thereby contributing to the strengthening of the analog structure. Similarly, Branch and Maria (2017) earlier showed that mung bean proteins can effortlessly improve gel at the least concentration compared with other proteins, e.g., soy proteins. On the other hand, the elastic behavior exhibited in the MAs can also be attributed to the gelling nature of MC which played an important role in creating an elastic compact network. Our findings infer that the viscoelasticity of MAs can be imparted by increasing the concentrations of MBPI, water, and MC ingredients, to create protein–protein, protein-water, and protein-polysaccharide interactions required to form the right elasticity, strength, and tight networks in the MAs.
3.5. Microstructure
The cross-section micrograph of MAs after freeze drying showed porous and fibrous structures as displayed in Figure 6. The cross-sectional area of the developed analog is shown in Figure 6A. Furthermore, to obtain the effects of ingredients combination on the fibrous structure of analog, SEM images were captured at 100x and 400x (Figures 6B,C) magnification. As observed in Figure 6B, the analog appeared very porous with a rough structure, most probably an aggregated network and some portion of the protein network slightly interconnected. This phenomenon might be explained by protein chains unfolding and aggregating during heating, which resulted in a three-dimensional network. Another possible phenomenon worth mentioning is that MC could form gels that entrap proteins, making proteins cross-link to form strong networks. Moreover, the protein network could be due to the interaction of proteins with polysaccharides mainly through hydrogen bonding (Ran et al., 2022). The incorporation of a high ratio of MBPI to PPI, and high concentrations of MC in the formulation contributed to the MA structure formation. Previous studies have proposed that the blending of two proteins could significantly enhance the formation of fibrous networks in MAs (Grabowska et al., 2014; Yuliarti et al., 2021). On the other hand, the network structure was loose and partly compact, which showed that the application of the heat-induced gelation technique alone was not sufficient to develop a tight, aligned, and more compact analog. Nonetheless, the network structure observed synchronizes with the viscoelastic results and further indicates the positive effects of mixing polysaccharides with proteins on the creation of structured plant-based MAs.
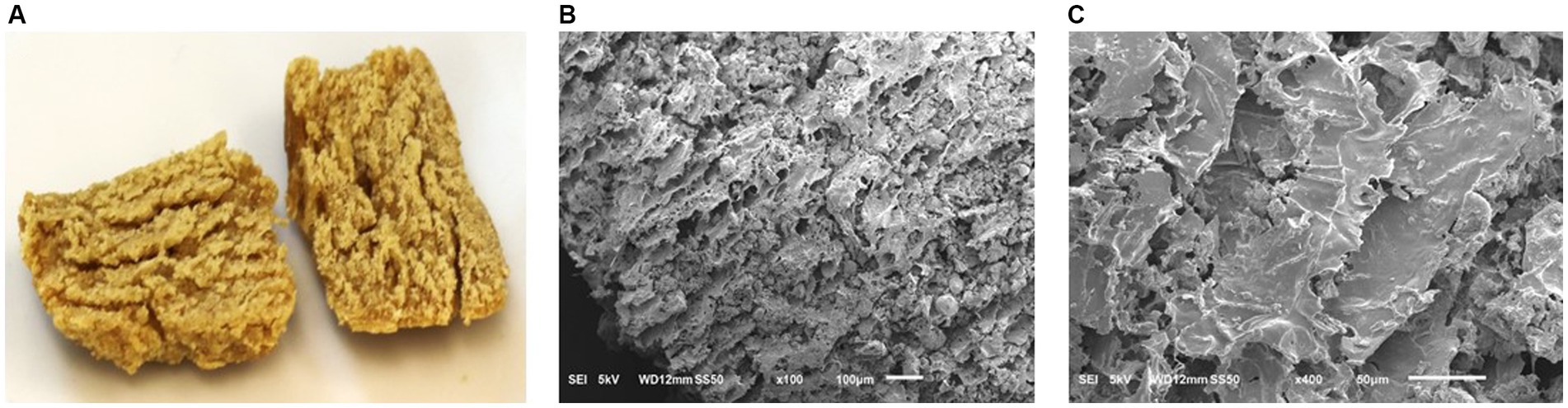
Figure 6. (A) Meat analog having MBPI-PPI in a 20:10 ratio, 42% of water, and 7% of MC. (B) SEM micrograph of analog showing porous and fibrous network at 100x. (C) Micrograph of analog at 400x showing sheet like structures.
4. Conclusion
This study investigated the potential of mungbean and pumpkin seed protein isolates as novel ingredients for the formation of plant-based MAs. Developed MAs from ingredients combinations of MBPI-PPI blends, different water content, and MC content using BBD were evaluated for their physicochemical parameters (moisture, protein content, color, texture, visco-elasticity, and microstructure). The results showed that ingredient levels significantly impacted the physicochemical and microstructural qualities of MAs. The ingredients combinations of MBPI-PPI in a 20:10 ratio (high level), 42% of water (high level), and 7% of MC (high level) demonstrated optimum conditions for developing plant-based analog with enhanced quality characteristics. The findings of this study showed that MBPI-PPI blends with polysaccharides played an important role to form an elastic and slightly compact network, to a certain extent. However, the heat-induced gelation technique was insufficient to create a fibrous and layered structure– that emulates the animal-based meat. Nevertheless, the incorporation of MBPI and MC contributed largely to the attainment of texture and visco-elasticity required for the fibrous structure in MAs. Thus, these ingredients could be considered as potential sources to produce plant-based MAs. This study clearly showed that a combination of MBPI: PPI, water, and MC formulated through heat-induced gelation can create MAs. However, further studies warrant attention for creating plant-based MAs that meet consumer acceptance.
Data availability statement
The data presented in the study are included in the article/supplementary material, further inquiries can be directed to the corresponding author.
Author contributions
MB: investigation, data collection, formal analysis, methodology, and writing-original draft. HM: data curation and data analysis. FA: formal analysis, investigation, and writing-review & editing. NS: writing-review & editing. SM: conceptualization, funding acquisition, supervision, project administration, and writing-reviewing & editing. All authors contributed to the article and approved the submitted version.
Acknowledgments
Authors are thankful to United Arab Emirates University (UAEU) for funding this project through UAEU-AUA (Asian University Alliance) grant (Fund code: 12F002).
Conflict of interest
The authors declare that the research was conducted in the absence of any commercial or financial relationships that could be construed as a potential conflict of interest.
Publisher’s note
All claims expressed in this article are solely those of the authors and do not necessarily represent those of their affiliated organizations, or those of the publisher, the editors and the reviewers. Any product that may be evaluated in this article, or claim that may be made by its manufacturer, is not guaranteed or endorsed by the publisher.
References
Arora, B., Kamal, S., and Sharma, V. (2017). Effect of binding agents on quality characteristics of mushroom based sausage analog. J. Food Process. Preserv. 41:e13134. doi: 10.1111/jfpp.13134
Bakhsh, A., Lee, S.-J., Lee, E.-Y., Sabikun, N., Hwang, Y.-H., and Joo, S. -T. (2021). A novel approach for tuning the physicochemical, textural, and sensory characteristics of plant-based meat analogs with different levels of methylcellulose concentration. Foods 10:560. doi: 10.3390/foods10030560
Batool, M., Ranjha, M. M. A. N., Roobab, U., Manzoor, M. F., Farooq, U., Nadeem, H. R., et al. (2022). Nutritional value, phytochemical potential, and therapeutic benefits of pumpkin (Cucurbita sp.). Plan. Theory 11:1394. doi: 10.3390/plants11111394
Boukid, F. (2021). Plant-based meat analogues: from niche to mainstream. Eur. Food Res. Technol. 247, 297–308. doi: 10.1007/s00217-020-03630-9
Branch, S., and Maria, S. (2017). Evaluation of the functional properties of mung bean protein isolate for development of textured vegetable protein. Int. Food Res. J. 24, 1595–1605.
Chen, F. L., Wei, Y. M., Zhang, B., and Ojokoh, A. O. (2010). System parameters and product properties response of soybean protein extruded at wide moisture range. J. Food Eng. 96, 208–213. doi: 10.1016/j.jfoodeng.2009.07.014
Chiang, J. H., Loveday, S. M., Hardacre, A. K., and Parker, M. E. (2019). Effects of soy protein to wheat gluten ratio on the physicochemical properties of extruded meat analogues. Food Struct. 19:100102. doi: 10.1016/j.foostr.2018.11.002
Cho, D. K., Lee, B., Oh, H., Lee, J. S., Kim, Y. S., and Choi, Y. M. (2020). Effect of searing process on quality characteristics and storage stability of sous-vide cooked pork patties. Foods 9:1011. doi: 10.3390/foods9081011
Cutroneo, S., Angelino, D., Tedeschi, T., Pellegrini, N., and Martini, D. (2022). Nutritional quality of meat analogues: results from the food labelling of Italian products (FLIP) project. Front. Nutr. 9:852831. doi: 10.3389/fnut.2022.852831
De Angelis, D., Kaleda, A., Pasqualone, A., Vaikma, H., Tamm, M., Tammik, M.-L., et al. (2020). Physicochemical and sensorial evaluation of meat analogues produced from dry-fractionated pea and oat proteins. Foods 9:1754. doi: 10.3390/foods9121754
Dekkers, B. L., Boom, R. M., and Van Der Goot, A. J. (2018). Structuring processes for meat analogues. Trends Food Sci. Technol. 81, 25–36. doi: 10.1016/j.tifs.2018.08.011
Dekkers, B. L., Nikiforidis, C. V., and Van Der Goot, A. J. (2016). Shear-induced fibrous structure formation from a pectin/SPI blend. Innovative Food Sci. Emerg. Technol. 36, 193–200. doi: 10.1016/j.ifset.2016.07.003
Du, H., Zhang, J., Wang, S., Manyande, A., and Wang, J. (2022). Effect of high-intensity ultrasonic treatment on the physicochemical, structural, rheological, behavioral, and foaming properties of pumpkin (Cucurbita moschata Duch.)-seed protein isolates. LWT 155:112952. doi: 10.1016/j.lwt.2021.112952
Ferawati, F., Zahari, I., Barman, M., Hefni, M., Ahlström, C., Witthöft, C., et al. (2021). High-moisture meat analogues produced from yellow pea and faba bean protein isolates/concentrate: effect of raw material composition and extrusion parameters on texture properties. Foods 10:843. doi: 10.3390/foods10040843
Ge, J., Sun, C.-X., Mata, A., Corke, H., Gan, R.-Y., and Fang, Y. (2021). Physicochemical and pH-dependent functional properties of proteins isolated from eight traditional Chinese beans. Food Hydrocoll. 112:106288. doi: 10.1016/j.foodhyd.2020.106288
Godschalk-Broers, L., Sala, G., and Scholten, E. (2022). Meat analogues: relating structure to texture and sensory perception. Foods 11:2227. doi: 10.3390/foods11152227
Grabowska, K. J., Tekidou, S., Boom, R. M., and Van Der Goot, A.-J. (2014). Shear structuring as a new method to make anisotropic structures from soy–gluten blends. Food Res. Int. 64, 743–751. doi: 10.1016/j.foodres.2014.08.010
Gu, J., Bk, A., Wu, H., Lu, P., Nawaz, M. A., Barrow, C. J., et al. (2022). Impact of processing and storage on protein digestibility and bioavailability of legumes. Food Rev. Intl. 39, 4697–4724. doi: 10.1080/87559129.2022.2039690
He, J., Evans, N. M., Liu, H., and Shao, S. (2020). A review of research on plant-based meat alternatives: driving forces, history, manufacturing, and consumer attitudes. Compr. Rev. Food Sci. Food Saf. 19, 2639–2656. doi: 10.1111/1541-4337.12610
Herz, E., Herz, L., Dreher, J., Gibis, M., Ray, J., Pibarot, P., et al. (2021). Influencing factors on the ability to assemble a complex meat analogue using a soy-protein-binder. Innovative Food Sci. Emerg. Technol. 73:102806. doi: 10.1016/j.ifset.2021.102806
Jia, C., Cao, D., Ji, S., Lin, W., Zhang, X., and Muhoza, B. (2020). Whey protein isolate conjugated with xylo-oligosaccharides via maillard reaction: characterization, antioxidant capacity, and application for lycopene microencapsulation. LWT 118:108837. doi: 10.1016/j.lwt.2019.108837
Jiang, J., Wang, Q., and Xiong, Y. L. (2018). A pH shift approach to the improvement of interfacial properties of plant seed proteins. Curr. Opin. Food Sci. 19, 50–56. doi: 10.1016/j.cofs.2018.01.002
Kumar, M., Tomar, M., Punia, S., Dhakane-Lad, J., Dhumal, S., Changan, S., et al. (2022). Plant-based proteins and their multifaceted industrial applications. LWT 154:112620. doi: 10.1016/j.lwt.2021.112620
Kumar, P., Sharma, N., Ahmed, M. A., Verma, A. K., Umaraw, P., Mehta, N., et al. (2022). Technological interventions in improving the functionality of proteins during processing of meat analogs. Front. Nutr. 9:1044024. doi: 10.1016/j.lwt.2021.112620
Kyriakopoulou, K., Keppler, J. K., and Van Der Goot, A. J. (2021). Functionality of ingredients and additives in plant-based meat analogues. Foods 10:600. doi: 10.3390/foods10030600
Lam, R. S. H., and Nickerson, M. T. (2015). The effect of pH and temperature pre-treatments on the physicochemical and emulsifying properties of whey protein isolate. LWT 60, 427–434. doi: 10.1016/j.lwt.2014.07.031
Lee, J.-S., Choi, I., and Han, J. (2022). Construction of rice protein-based meat analogues by extruding process: effect of substitution of soy protein with rice protein on dynamic energy, appearance, physicochemical, and textural properties of meat analogues. Food Res. Int. 161:111840. doi: 10.1016/j.foodres.2022.111840
Mcclements, D. J., and Grossmann, L. (2021). The science of plant-based foods: constructing next-generation meat, fish, milk, and egg analogs. Compr. Rev. Food Sci. Food Saf. 20, 4049–4100. doi: 10.1111/1541-4337.12771
Michelin, M., Marques, A. M., Pastrana, L. M., Teixeira, J. A., and Cerqueira, M. A. (2020). Carboxymethyl cellulose-based films: effect of organosolv lignin incorporation on physicochemical and antioxidant properties. J. Food Eng. 285:110107. doi: 10.1016/j.jfoodeng.2020.110107
Miedzianka, J., Zambrowicz, A., Zielińska-Dawidziak, M., Drożdż, W., and Nemś, A. (2021). Effect of acetylation on physicochemical and functional properties of commercial pumpkin protein concentrate. Molecules 26:1575. doi: 10.3390/molecules26061575
Moll, P., Salminen, H., Stadtmueller, L., Schmitt, C., and Weiss, J. (2023). Comparison of binding properties of a laccase-treated pea protein–sugar beet pectin mixture with methylcellulose in a bacon-type meat analogue. Foods 12:85. doi: 10.3390/foods12010085
Mostafa, H., Airouyuwa, J. O., and Maqsood, S. (2022). A novel strategy for producing nano-particles from date seeds and enhancing their phenolic content and antioxidant properties using ultrasound-assisted extraction: a multivariate based optimization study. Ultrason. Sonochem., 87:106017.
Nanta, P., Skolpap, W., and Kasemwong, K. (2021). Influence of hydrocolloids on the rheological and textural attributes of a gluten-free meat analog based on soy protein isolate. J. Food Process. Preserv. 45:e15244. doi: 10.1111/jfpp.15244
O'sullivan, J., Murray, B., Flynn, C., and Norton, I. (2016). The effect of ultrasound treatment on the structural, physical and emulsifying properties of animal and vegetable proteins. Food Hydrocoll. 53, 141–154. doi: 10.1016/j.foodhyd.2015.02.009
Ozturk, O. K., Salgado, A. M., Holding, D. R., Campanella, O. H., and Hamaker, B. R. (2023). Dispersion of zein into pea protein with alkaline agents imparts cohesive and viscoelastic properties for plant-based food analogues. Food Hydrocoll. 134:108044. doi: 10.1016/j.foodhyd.2022.108044
Palanisamy, M., Töpfl, S., Aganovic, K., and Berger, R. G. (2018). Influence of iota carrageenan addition on the properties of soya protein meat analogues. Lwt 87, 546–552. doi: 10.1016/j.lwt.2017.09.029
Pearce, K. N., and Kinsella, J. E. (1978). Emulsifying properties of proteins: evaluation of a turbidimetric technique. J. Agric. Food Chem. 26, 716–723.
Pirsa, S., and Hafezi, K. (2022). Hydrocolloids: structure, preparation method, and application in food industry. Food Chem. 399:133967. doi: 10.1016/j.foodchem.2022.133967
Ran, X., Lou, X., Zheng, H., Gu, Q., and Yang, H. (2022). Improving the texture and rheological qualities of a plant-based fishball analogue by using konjac glucomannan to enhance crosslinks with soy protein. Innovative Food Sci. Emerg. Technol. 75:102910. doi: 10.1016/j.ifset.2021.102910
Rehrah, D., Ahmedna, M., Goktepe, I., and Yu, J. (2009). Extrusion parameters and consumer acceptability of a peanut-based meat analogue. Int. J. Food Sci. Technol. 44, 2075–2084. doi: 10.1111/j.1365-2621.2009.02035.x
Samard, S., and Ryu, G.-H. (2019). Physicochemical and functional characteristics of plant protein-based meat analogs. J. Food Process. Preserv. 43:e14123. doi: 10.1111/jfpp.14123
Shevkani, K., Singh, N., Kaur, A., and Rana, J. C. (2015). Structural and functional characterization of kidney bean and field pea protein isolates: a comparative study. Food Hydrocoll. 43, 679–689. doi: 10.1016/j.foodhyd.2014.07.024
Shrestha, S., Van’t Hag, L., Haritos, V. S., and Dhital, S. (2023). Lentil and Mungbean protein isolates: processing, functional properties, and potential food applications. Food Hydrocoll. 135:108142. doi: 10.1016/j.foodhyd.2022.108142
Singh, M., Trivedi, N., Enamala, M. K., Kuppam, C., Parikh, P., Nikolova, M. P., et al. (2021). Plant-based meat analogue (PBMA) as a sustainable food: a concise review. Eur. Food Res. Technol. 247, 2499–2526. doi: 10.1007/s00217-021-03810-1
Taghian Dinani, S., Broekema, N. L., Boom, R., and Van Der Goot, A. J. (2023). Investigation potential of hydrocolloids in meat analogue preparation. Food Hydrocoll. 135:108199. doi: 10.1016/j.foodhyd.2022.108199
Tang, C.-H., and Sun, X. (2011). Structure–physicochemical function relationships of 7S globulins (vicilins) from red bean (Phaseolus angularis) with different polypeptide constituents. Food Hydrocoll. 25, 536–544. doi: 10.1016/j.foodhyd.2010.08.009
Tan, M., Xu, J., Gao, H., Yu, Z., Liang, J., Mu, D., et al. (2021). Effects of combined high hydrostatic pressure and pH-shifting pretreatment on the structure and emulsifying properties of soy protein isolates. J. Food Eng. 306:110622. doi: 10.1016/j.jfoodeng.2021.110622
Vinayashree, S., and Vasu, P. (2021). Biochemical, nutritional and functional properties of protein isolate and fractions from pumpkin (Cucurbita moschata var. Kashi Harit) seeds. Food Chem. 340:128177. doi: 10.1016/j.foodchem.2020.128177
Wen, Y., Kim, H. W., and Park, H. J. (2022). Effect of xylose on rheological, printing, color, texture, and microstructure characteristics of 3D-printable colorant-containing meat analogs based on mung bean protein. Food Res. Int. 160:111704. doi: 10.1016/j.foodres.2022.111704
Xia, S., Song, J., Ma, C., Hao, T., Hou, Y., Shen, S., et al. (2023). Effects of moisture content and processing temperature on the strength and orientation regulation of fibrous structures in meat analogues. Food Hydrocoll. 145:109113. doi: 10.1016/j.foodhyd.2023.109113
Ye, B., Chen, J., Ye, H., Zhang, Y., Yang, Q., Yu, H., et al. (2022). Development of a time–temperature indicator based on Maillard reaction for visually monitoring the freshness of mackerel. Food Chem. 373:131448. doi: 10.1016/j.foodchem.2021.131448
Yuliarti, O., Kiat Kovis, T. J., and Yi, N. J. (2021). Structuring the meat analogue by using plant-based derived composites. J. Food Eng. 288:110138. doi: 10.1016/j.jfoodeng.2020.110138
Zhang, T., Jiang, B., Mu, W., and Wang, Z. (2009). Emulsifying properties of chickpea protein isolates: influence of pH and NaCl. Food Hydrocoll. 23, 146–152. doi: 10.1016/j.foodhyd.2007.12.005
Zhao, F., Li, Y., Li, C., Ban, X., Cheng, L., Hong, Y., et al. (2021). Co-supported hydrocolloids improve the structure and texture quality of gluten-free bread. LWT 152:112248. doi: 10.1016/j.lwt.2021.112248
Zhao, H., Shen, C., Wu, Z., Zhang, Z., and Xu, C. (2020). Comparison of wheat, soybean, rice, and pea protein properties for effective applications in food products. J. Food Biochem. 44:e13157. doi: 10.1111/jfbc.13157
Keywords: plant-based meat analogs, plant proteins, physicochemical properties, texture, microstructure, viscoelasticity, mung bean, pumpkin proteins
Citation: Baig MA, Ajayi FF, Mostafa H, Sivapragasam N and Maqsood S (2023) Mungbean and pumpkin protein isolates as novel ingredients for the development of meat analogs using heat-induced gelation technique. Front. Sustain. Food Syst. 7:1243183. doi: 10.3389/fsufs.2023.1243183
Edited by:
Carla Cavallo, University of Naples Federico II, ItalyReviewed by:
Mehdi Mohammadian, University of Tehran, IranSara Cutroneo, University of Parma, Italy
Copyright © 2023 Baig, Ajayi, Mostafa, Sivapragasam and Maqsood. This is an open-access article distributed under the terms of the Creative Commons Attribution License (CC BY). The use, distribution or reproduction in other forums is permitted, provided the original author(s) and the copyright owner(s) are credited and that the original publication in this journal is cited, in accordance with accepted academic practice. No use, distribution or reproduction is permitted which does not comply with these terms.
*Correspondence: Sajid Maqsood, sajid.m@uaeu.ac.ae