- 1Research Institute on Terrestrial Ecosystems (IRET), National Research Council of Italy (CNR), Terni, Italy
- 2Department of Agricultural Sciences, University of Naples “Federico II”, Naples, Italy
- 3Italian Space Agency, Rome, Italy
Long-term space missions will require a self-sustaining food production system to meet the crew’s nutritional and health needs. For this purpose, plant-based food production systems with elevated resource efficiency are required, based on advanced agricultural technologies that produce phytonutrient-rich crops. In addition to the resource requirements for crop production on Earth, volume and time efficiency become essential factors to consider for food production in space. Microgreens represent a promising candidate for space farming as they have a high harvest index, short cultivation cycle, and high nutritional potential. However, the development of specific technical protocols for growing microgreens in space is essential since different agronomic inputs, such as substrates and fertigation, can modulate productivity, quality and resource efficiency of microgreens cultivation. The current work examines the effects of different substrates (coconut fiber and cellulose sponge) and nutrient solution (NS) management strategies (quarter strength Hoagland and half strength Hoagland/osmotic water) on the production of two species of microgreens [Raphanus sativus cv. Saxa 2 (Radish); Brassica oleracea var. capitata f. sabauda cv. Vertus (Savoy cabbage)]. The appraisal focused on (i) biomass production and quality, and (ii) sizing of space facilities devoted to the production of phytonutrients required for the astronauts’ wellbeing. In our study, the interaction among species, substrate and NS significantly affected the accumulation of fructose, sucrose, total soluble non-structural carbohydrates and nitrate as well as the daily production of total ascorbic acid and, in turn, the required microgreens serving to supply its adequate daily intake. Species-substrate interaction effects on fresh yield, dry yield, dry matter, anthocyanins, TPC, β-carotene and sulfate content as well as the cultivation surface required to produce the adequate daily intake of ascorbic acid (AscA) were assessed. Substrate-NS interaction modulated the anthocyanins, violaxanthin and sulfate contents independently of species. On the other hand, single factor effects were identified with respect to the accumulation of lutein, chlorophylls, glucose, and starch. Therefore, the management of microgreens cultivation in terms of NS and substrate is an effective tool to meet the phytochemical requirements of the crew.
1. Introduction
The future of manned space exploration missions, foreseen for the next decades, points far beyond the Earth’s orbit, toward the moon and Mars (Douglas et al., 2020). Long-term space missions will require complete crew autonomy, as regular resource resupply will become logistically and economically disadvantageous due to distance from Earth. Astronauts who rely on a space food system for extended periods of months or even years are particularly vulnerable to the consequences of nutrient deficiency or excess (Smith et al., 2014). To support the dietary requirements of the crew, it is crucial to design an efficient plant-based food production system developed on advanced agricultural technologies (Nguyen et al., 2022). Farming in space will face completely new environmental conditions like altered gravity, ionizing radiations, reduced atmospheric pressure, and high CO2 levels. Moreover, energy, volume and mass limitations will require the maximization of resources (light, water, and fertilizer) and influence payload design, which will, ultimately, impact the cost and feasibility of a mission (Wheeler, 2010; Douglas et al., 2020; Poulet et al., 2022). Several works investigated microgreens as a potential candidate for space farming since they are characterized by ease of cultivation, high-volume optimization potential, rich phytochemical composition and require little crew time investment (Kyriacou et al., 2016; Galieni et al., 2020; Zhang et al., 2021; Gupta et al., 2023; Izzo et al., 2023). Microgreens are a novel class of salad crops which have gained popularity for their large range of colors, peculiar tastes, and elevated nutraceutical properties (Bulgari et al., 2017). Microgreens are usually harvested between the complete expansion of the cotyledons and the appearance of the second true leaf, ensuring rapid and easy production cycles. The high availability of phytochemicals such as vitamins, minerals, polyphenols and glucosinolates highlights the health benefit potential of microgreens (Jambor et al., 2022). Thanks to their ease of cultivation and their positive properties for health, microgreens are now considered a functional food to diversify and enrich the nutrient content of human diet, especially in urban areas and in locations where fresh food availability is limited due to unfavorable climate context and technological backwardness (Singh et al., 2020). In the context of human space flight, they represent a ready-to-eat product with the potential to counteract astronauts diet deficiency and space-induced pathologies (Kyriacou et al., 2017); this is the case of certain phytochemicals, such as ascorbate, that are now considered functional radio mitigators that may be important in counteracting radiation damage in space (Mortazavi et al., 2015). A plethora of species can be consumed as a microgreen, and recently a rising literature is evaluating the productive and qualitative characteristics of increasingly different genotypes (De la Fuente et al., 2019; Kyriacou et al., 2019a,b; Xiao et al., 2019) even with specific reference to space farming (Izzo et al., 2023). Besides the species, microgreen production parameters and phytochemicals profiles can be heavily influenced by environmental and agronomical factors (Samuolienė et al., 2013; Kyriacou et al., 2020; Teng et al., 2022; Amitrano et al., 2023). To ensure that optimal yield and quality are achieved, it is crucial to consider the effect of these factors on a species-specific basis and make necessary optimizations. Further research is necessary to profit from controlled environment agriculture (CEA) through modifying growth environments in order to produce targeted phytonutrients in microgreens (Teng et al., 2022). Growth substrates, which are ideally derived from the available, renewable, and inexpensive source material, represent one of the main agronomical factors shaping production costs, yield, waste production, and quality attributes of microgreens farming (Kyriacou et al., 2020). There are two classes of available media for microgreen cultivation, which differ by their physical, chemical and biological characteristics: (1) organic, i.e., made of natural derived and biodegradable materials; and (2) inorganic, which are usually inert (Di Gioia et al., 2017a). The effects of cultivation media on the quantity and quality of the produce have been tested by several authors (Muchjajib et al., 2015; Di Gioia et al., 2017b; Bulgari et al., 2021). The management of the fertilization procedure is another important pre-harvest factor influencing the quality of the produce (Wang et al., 2008; Rouphael et al., 2018). Pre-fertilization of the growing media and/or post-emergence fertigation should be applied according to the species germination time and growth rate (Murphy and Pill, 2010). Generally, the common post-emergence NS are based on a modified Hoagland NS with half (50%) or quarter (25%) strength (Palmitessa et al., 2020; El-Nakhel et al., 2021; Keutgen et al., 2021). Although fertilization is essential to obtain satisfying productions (Murphy and Pill, 2010), several authors have shown that the introduction of moderate nutritional stress through mineral nutrient deprivation resulted in an enhanced phytochemical profile and greater quality of microgreens due to the activation of the secondary metabolism of the plant (Pannico et al., 2020; Kyriacou et al., 2021; Petropoulos et al., 2021). While there is a growing body of literature investigating how to efficiently produce microgreens on Earth systems, no space oriented ground-based research on microgreen productivity and quality has been conducted so far. In this paper, the effects of different substrates (coconut fiber and cellulosic sponge) and NS management (quarter-strength Hoagland and half-strength Hoagland/osmotic water) were tested on two different species of microgreens: Raphanus sativus cv. Saxa 2 and Brassica oleracea var. capitata f. Sabauda cv. Vertus. The aim of the work was to assess the effects of the interactions among species and growing conditions on (a) productivity and quality; (b) sizing of space facilities devoted to the production of nutraceuticals for the astronaut’s wellbeing.
2. Materials and methods
2.1. Plant material and experimental design
Based on the selection of Izzo et al. (2023), two microgreen species, radish (Raphanus sativus cv. Saxa 2) and savoy cabbage (Brassica oleracea var. capitata f. Sabauda cv. Vertus), both provided by Pagano Costantino & F.lli Srl (Scafati, Salerno, Italy), were sown on two different substrates: coconut fiber (Sisal Fiber, Imola, Italy) and cellulose sponge (Spontex SAS, Colombes, France). The sowing density was 32,500 and 42,500 seeds m−2 for radish and savoy cabbage, respectively. The experiment was carried out in a growth chamber (KBP-6395F, Termaks, Bergen, Norway) at the Department of Agricultural Sciences of the University of Naples ‘’Federico II’’ (Portici, Italy). The LED lighting system (K5 Series XL750, Kind LED, Santa Rosa, CA, United States) had a light spectrum in the range of 400–700 nm and an intensity of 300 ± 10 μmol m−2 s−1. Seed germination occurred in the dark, at 24°C and 100% relative humidity (RH). Once the microgreens emerged, the light was turned on (12 h photoperiod), and the growth chamber was set at 24/18 ± 2°C and RH 65 ± 5%. The microgreens were subjected to two different fertigation strategies that included (1) a quarter concentration of Hoagland’ NS (QS; EC: 0.4 ± 0.05 mS cm−1; pH: 6 ± 0.2) for the entire crop cycle as a control; (2) a Hoagland’s NS with half concentration (HS; EC: 0.8 ± 0.05 mS cm−1; pH: 6 ± 0.2) for the first half of the crop cycle and osmotic water for the remaining part (H2O; EC: 65.7 μS cm−1; pH:6.2), henceforth HS/H2O. The microgreens were distributed in the growth chamber according to a randomized trifactorial experimental design in which the factors were species [SP; radish and savoy cabbage], substrate [SU; coconut fiber (Coco) and cellulose sponge (Sponge)] and different management of the NS (QS and HS/H2O). Each experimental unit was replicated three times (n = 3; 24 experimental units). The radish and savoy cabbage microgreens were cut at the substrate level 14 and 16 days after sowing, respectively, weighed for fresh weight (FW) determination, freeze-dried and finely ground for subsequent analysis and for dry weight (DW) and dry matter percentage (DM) determination. An aliquot of the microgreens was immediately frozen at −80°C for qualitative analysis.
2.2. Ascorbic acid content
For the determination of total Ascorbic Acid (Tot. AscA), 10 mg of the frozen powder was extracted in an ice-cold glass–glass homogenizer with 2 mL of 3% Metaphosphoric acid (MPA) at 4°C. The mixtures were centrifuged at 16,000 × g for 5 min at 4°C. The supernatants were filtrated through a 0.2 μm (Whatman) PPII nylon filters then dehydro-ascorbate was reduced to AscA, using Tris (2-carboxyethyl) phosphine (TCEP) as a reducing agent. The TCEP was added to the final concentration of 5 mMol L−1 in the filtered extract, which was then incubated for 30 min at 25 ° C. After 30 min, the samples were injected into HPLC for the quantification of Tot. AscA. The chromatographic method used is that described in Chebrolu et al. (2012) with minimal changes. Tot. AscA was analyzed using an UltiMate 3,000 HPLC System ThermoScientific™ Dionex (Sunnyvale, CA, United States) coupled with a UV/VIS detector (ThermoScientific™ Dionex). The separation was performed using a Phenomenex Luna C18 column (250 mm × 4.6 mm i.d. and particle size 5 μm) and the run time was 15 min. The Tot. AscA peak was detected at 254 nm and the processing of the chromatographic peaks was performed using the software version Chromeleon 7.2 (ThermoScientific™ Dionex). The entire chromatographic separation was performed in an isocratic mobile phase consisting of 0.010 mol L−1 of KH2PO4, maintained at pH 2.8 and flow rate of 0.7 mL min−1 with an injection volume of 5 μL. The quantification was performed by means of a calibration curve of an AscA standard. All the reagents used are of a high degree of purity for HPLC analysis. Tot. AscA content was expressed as mg 100 g−1 of fresh weight (FW).
2.3. Total anthocyanins and total phenolic content
Total anthocyanins (Tot. Ant) were determined by extracting 10 mg of lyophilized powder in 2 mL of 1% HCl in methanol for 1 h at 65°C. The liquid extract was separated by centrifugation at 16,000 × g for 5 min. After centrifugation, the supernatant was separated, and the Tot. Ant content was quantified spectrophotometrically by measuring the absorbance at 530 nm and 657 nm. The reading at 657 nm allows the correction of the quantification of anthocyanins for any degradation products of chlorophylls. The phenolic component (TPC) was quantified according to the protocol described by Usenik et al. (2008) by extracting 10 mg of powder from the lyophilized samples in 2 mL of 100% methanol. After centrifugation at 16,000 × g for 5 min, the supernatant was recovered and used for the spectrophotometric quantification of polyphenols, determining the absorbance at 765 nm. The amount of total polyphenols was then calculated by relating the absorbance of each sample to the calibration line of gallic acid. Tot. Ant content and TPC were expressed as mg 100 g−1 FW.
2.4. Pigments content
Chlorophylls (a and b), β-carotene, lutein, neoxanthin and violaxanthin, were extracted from 10 mg of lyophilized microgreens powder with 2 mL 100% acetone at 4°C under dark conditions using a glass–glass homogenizer. The samples were centrifuged at 16,000 × g for 5 min at 4°C and filtered through a 0.2 μm nylon PPII syringe disposable filter; 15 μL of the clear extract was used to determine the concentration of pigments by an HPLC U3000 system (Dionex™ ICS-5000; Thermo Fisher Scientific, Waltham, MA, United States), equipped with a C18(2) LUNA (Phenomenex, Bologna, Italy) analytical column (5 μm, 250 mm × 4.6 mm) and a related guard column (Phenomenex, Bologna, Italy) maintained at 30°C. All separations were achieved isocratically using, from 0 to 4 min, a mobile phase composed of solution A: 1.75% water, 1.75% methanol, 1.75% dichloromethane, and 94.75% acetonitrile, and from 4.1 to 18 min a mobile phase composed of solution B: 50% acetonitrile and 50% diethyl acetate, with a final re-equilibration of 4 min with solution A. The flow rate was 1 mL min−1 for a total run time of 22 min. The autosampler was maintained at 4°C, the UV detector wavelength was set at 440 nm, and concentrations of all pigments were determined against standard curves (Žnidarčič et al., 2011). All the pigment contents were expressed as mg 100 g−1 FW.
2.5. Non-structural carbohydrates
Measurements of non-structural carbohydrates (NSCs) were performed using 10 mg samples of the microgreens powder obtained from the lyophilized material. The extraction was performed in 1 mL of 80% ethanol at 80°C for 45 min under continuous shaking conditions. The extract was centrifuged at 16,000 × g for 5 min, soluble sugars (glucose, fructose, and sucrose) were recovered in the supernatant, and starch was in the pellet. Soluble sugar determination, by spectrophotometric coupled enzymatic assay, was performed, as described in Scartazza et al. (2017). All sugar assays were performed in dual-wavelength mode (340–405 nm) in an Anthos plate reader (Anthos Labtec Instruments, Austria). The pellet, containing starch, was washed four times with a 50 mM NaAcetate buffer (pH 4.5) and then suspended and autoclaved at 120°C for 45 min in 1 mL of the same buffer. After autoclaving, the sample was incubated at 50°C for 1 h with amyloglucosidase (70 U) and α-amylase (4 U) to hydrolyze the starch to glucose. The glucose produced by starch hydrolysis was then measured as described before by spectrophotometric coupled enzymatic assay. All the NSC contents were expressed as mg 100 g−1 FW.
2.6. Anions content
The extraction of inorganic anions (nitrate, sulfate and chloride) was obtained using 10 mg of lyophilized microgreens powder and in water at 80°C for 45 min under continuous stirring. After extraction, the sample was centrifuged at 16,000 × g for 5 min and the supernatant was then recovered and filtered using a 0.2 μm nylon PPII syringe filter before injection into an ion chromatography system (DionexTM ICS-5000) equipped with a conductivity detector, an IonPac AS11-HC analytical column (4 × 250 mm) with associated guard and IonPac Anion Trap Column (ATC)-1 (Thermo Fisher Scientific, Waltham, MA, United States). The chromatographic system was coupled with an ERSTM 500 electrolytically regenerated suppressor to suppress unwanted ion interference in the assay. The runs were performed at a temperature of 30°C and a flow rate of 1 mL min−1. The mobile phase consists of a gradient of 100 mM NaOH between 1 and 15 mM over 24 min. The signal is integrated in micro-Siemens (μS). The eluents and standard solutions of inorganic anions were prepared using HPLC grade reagents (Merck KGaA, Darmstadt, Germany). Chromatographic system control, data acquisition and processing were performed by Chromeleon 7.2 software (ThermoScientific™ Dionex). Nitrate, sulfate and chloride content were expressed as mg 100 g−1 FW.
2.7. Daily production of total ascorbic acid, microgreens serving, and facilities cultivation surface size
The elaboration of the daily Tot. AscA production (DTAP) of the system per square meter was conducted based on the length of the growing period, the biomass productivity and the total ascorbate concentration of the microgreen produced. In addition, considering the average requirement (AR) set by EFSA Panel on Dietetic Products, Nutrition and Allergies (NDA), (2013) at 90 mg day−1 for an adult, it was possible to calculate the cultivation surface size (CSS) needed to produce the AR and the size of the serving that would apport the AR, for each of the factor’s combination. DTAP, Serving and CSS were expressed as mg m−2 day−1, grams and m2, respectively.
2.8. Statistical analysis
Statistical analysis was performed using IBM SPSS Statistics 20 (Chicago, IL, United States). All parameters of the experiments were subjected to the Multivariate analysis following the General Linear Model. Mean values were separated according to Tukey’s test with p = 0.05. All the parameters analyzed were subjected to Principal Component Analysis (PCA) using Minitab® 21.4 statistical software (Minitab LLC, State College, PA, United States).
3. Results
3.1. Production parameters
The species, the substrate, and their combination highly affected the yield (fresh and dry yield) and dry matter (SP × SU; Table 1). For both radish (the most productive species) and savoy cabbage, the cultivation in Coco substrate increased fresh and dry yield but reduced dry matter. Regardless of SP and SU, QS treatment increased fresh yield and decreased dry matter compared to HS/H2O throughout the crop cycle (Table 1). Although the SP × NS interaction did not result in significant differences in fresh and dry yields, the lowest dry matter values of the two species were obtained in the QS treatment (Table 1). Moreover, different NS management did not result in significant differences for microgreens grown in Coco for all the parameters shown in Table 1. Differentially, in the Sponge substrate, the use of QS NS, compared to HS/H2O, increased fresh yield by 10.46% and decreased dry matter by 6.43%.
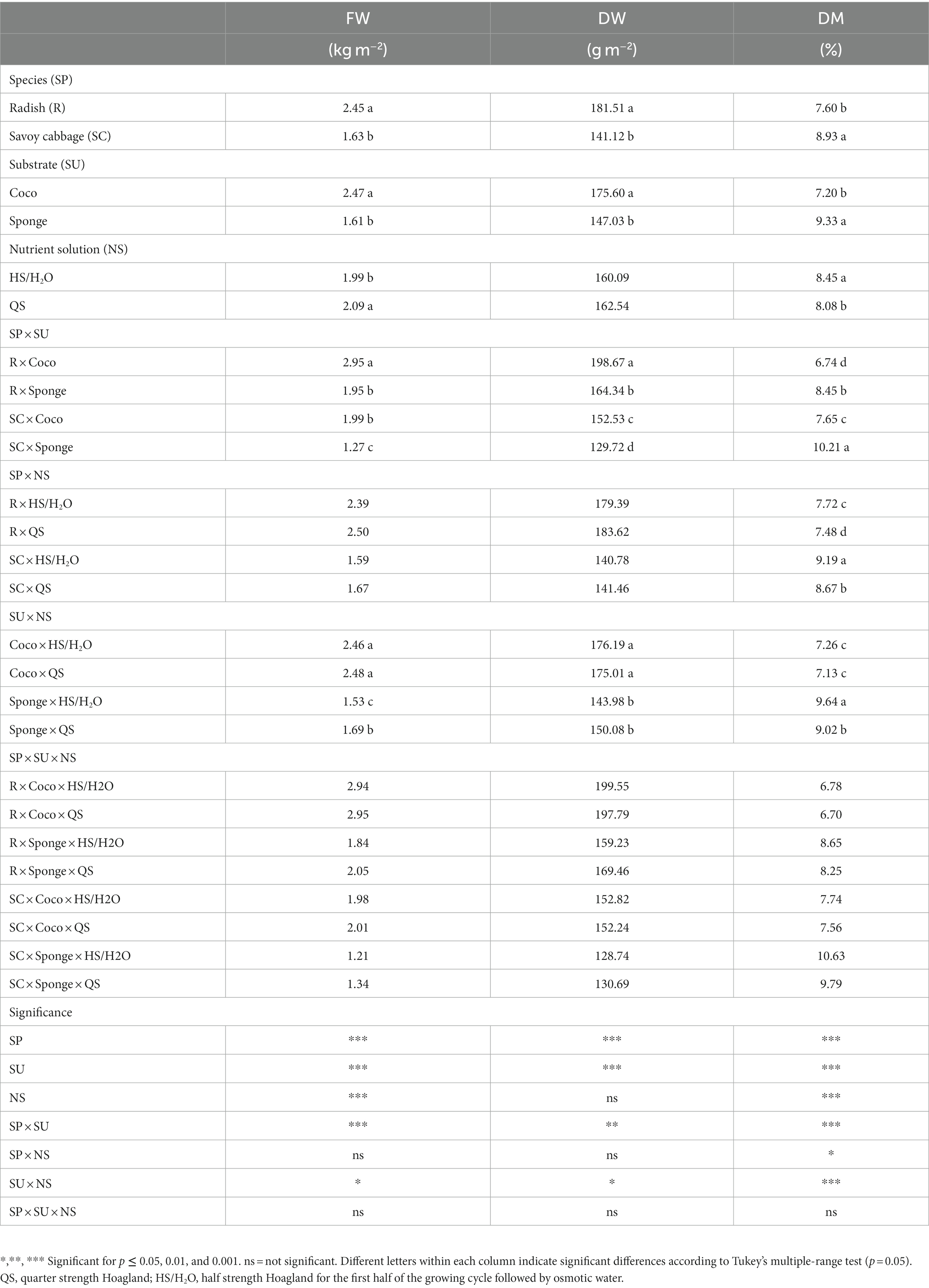
Table 1. Microgreens fresh weight (FW), dry weight (DW), and dry matter (DM) expressed in kg m−2, g m−2 and percentage (%), respectively.
3.2. Total ascorbic acid, total anthocyanins and total phenolic content
The values of Tot. AscA, Tot. Ant and TPC are shown in Table 2. Savoy cabbage accumulated 41.0% more Tot. AscA than radish (Table 2). The SU determined a significant effect for Tot. AscA, which was greater than 17.4% on Sponge compared to Coco. The NS and the interaction between the factors were not effective on modulating the Tot. AscA accumulation. Tot. Ant content was significantly influenced by the SP × SU and SU × NS interactions (Table 2). On both the substrates, radish showed higher Tot. Ant level than savoy cabbage. The use of the Sponge substrate resulted in an overall greater Tot. Ant content. NS significantly influenced the content of Tot. Ant only in combination with the Sponge substrate, where the HS/H2O solutions induced an increase of 19.0% in comparison to the QS (Table 2). The analysis conducted on the TPC showed significant interaction for the SP × SU combination. The SU had a dominant effect on the TPC: the low accumulating species, radish, cultivated on Sponge overcame savoy cabbage when it was cultivated on Coco (Table 2), while the use of Sponge resulted in an increment of 68.0 and 42.0% compared to Coco, respectively in savoy cabbage and radish. The NS factor did not significantly affect TPC.
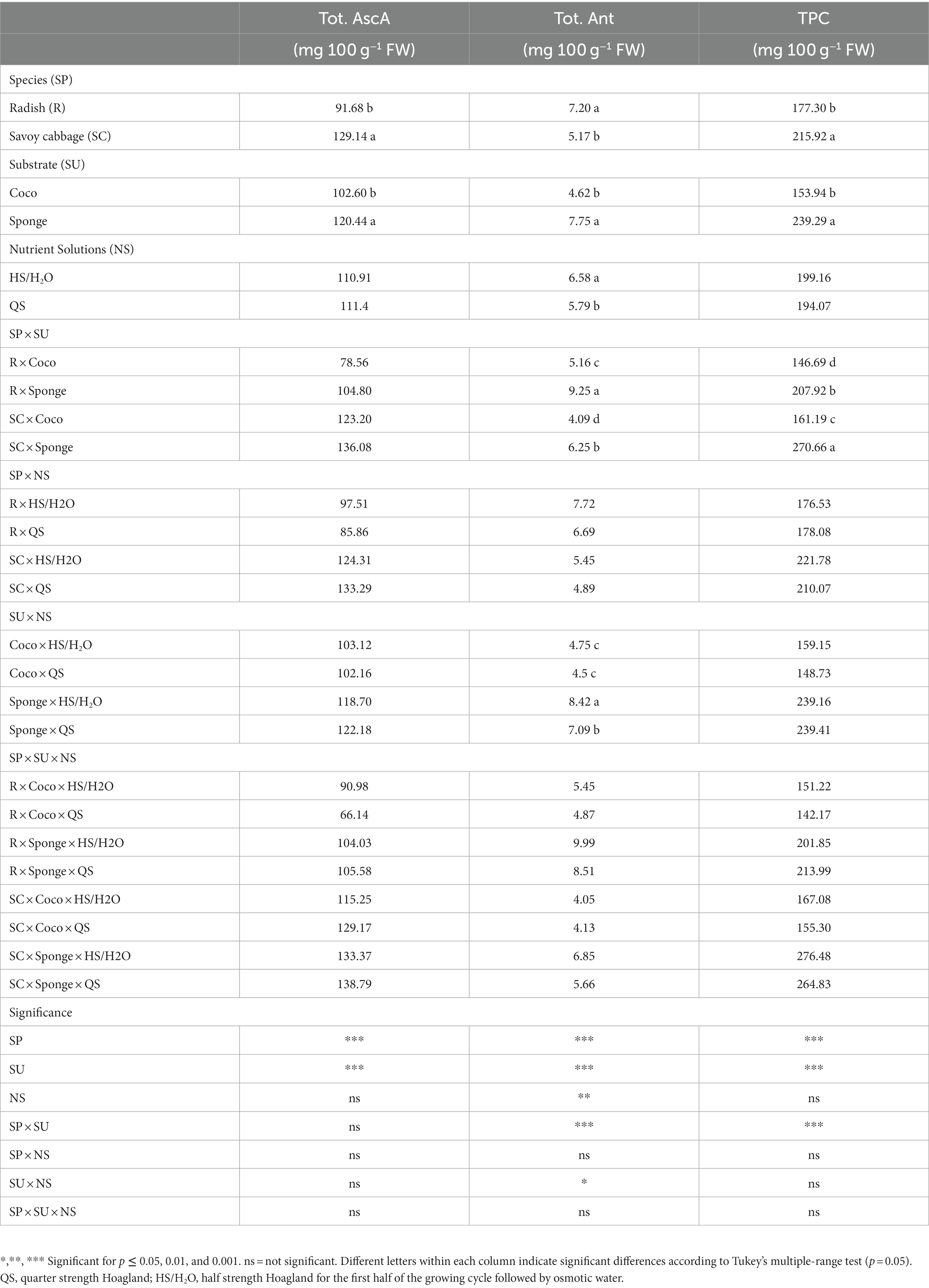
Table 2. Microgreens total AscA (Tot.AscA), total anthocyanins (Tot.Ant), and Total Phenolic Content (TPC) expressed in mg 100 g−1 fresh weight (FW).
3.3. Pigments
The contents of β-carotene and violaxanthin showed significant interaction for the SP × SU and SU × NS combinations, respectively (Table 3). The combination radish × Sponge showed a 35.0% higher content of β-carotene than the combination radish × Coco. The Sponge × HS/H2O combination showed a content of violaxanthin higher than that of the combination Coco × HS/H2O and Sponge × QS (Table 3). The other combinations did not show significant effects. The analysis of the other pigments showed significant differences between the two species tested for the content of lutein and chlorophylls (a and b), with the best performance in the accumulation of all three components in the case of savoy cabbage. Finally, no significant difference was found for the neoxanthin content.
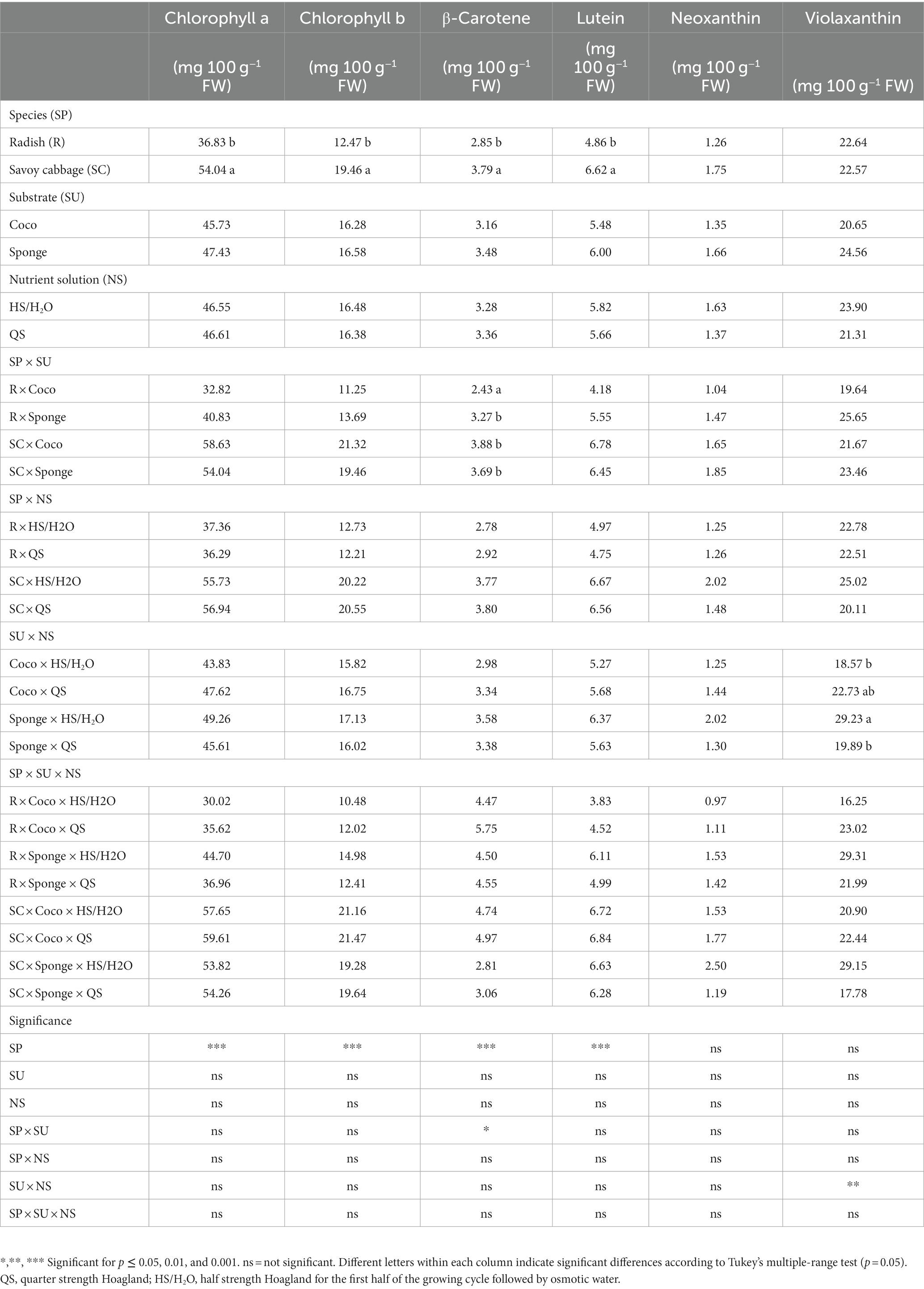
Table 3. Microgreens pigments (chlorophylls and carotenoids) expressed in mg 100 g−1 fresh weight (FW).
3.4. Non-structural carbohydrates
The contents of the non-structural carbohydrates (NSC) are reported in Table 4. The content of NSC was high and variable, with several differences likely due to either other factors and/or their partial or total interactions. Regardless of the experimental conditions, the most abundant NSC was glucose followed by starch, fructose, and sucrose (Table 4). Total soluble NSC (Tot. soluble) where the most relevant contributors to the total content of NSC in the microgreens, with some instances where the soluble NSC were close to represent 2.0% of fresh weight of the microgreens. The data highlighted the effect of the interaction SP × SU × NS on the content of fructose, sucrose and Tot. soluble. For these components, the modulation of the contents, due to the different nutritional solutions, is clearly visible in the case of the use of the Sponge substrate and savoy cabbage species. In the case of fructose and sucrose, the different substrates appear to have an accentuated modulation effect in the latter cultivar with higher quantities accumulated on Sponge (+66.0% in HS/H2O compared to QS). On the Tot. soluble, the differences due to the interactions of the factors determine different levels of accumulation for each combination of species and substrate, with the highest content found in savoy cabbage × Sponge and attributable to the interaction with the HS/H2O solution (Table 4). The total NSC content appears to be influenced by the SP × SU and SP × NS combinations. In neither of the two different combinations, however, does the interaction hide the greater concentration of NSC in savoy cabbage compared to radish. The SP × SU combination maintains this difference, while the use of Sponge almost a double increase in the NSC content for both species. The NS component interacted with the species, increasing the productivity in the treatment with HS/H2O only in the case of savoy cabbage. The glucose and starch content show no variability in response to the considered factors. Significant variations in glucose were detected between the two SP, SU, and NS, while differences in the starch content were induced only by the SP and SU (Table 4). The higher content of glucose and starch of the two species was found in the savoy cabbage cultivar while, as regards the evaluation of the performances between the two different substrates, the higher content was found for the samples grown on Sponge. Finally, HS/H2O NS determined significantly higher glucose levels than the QS.
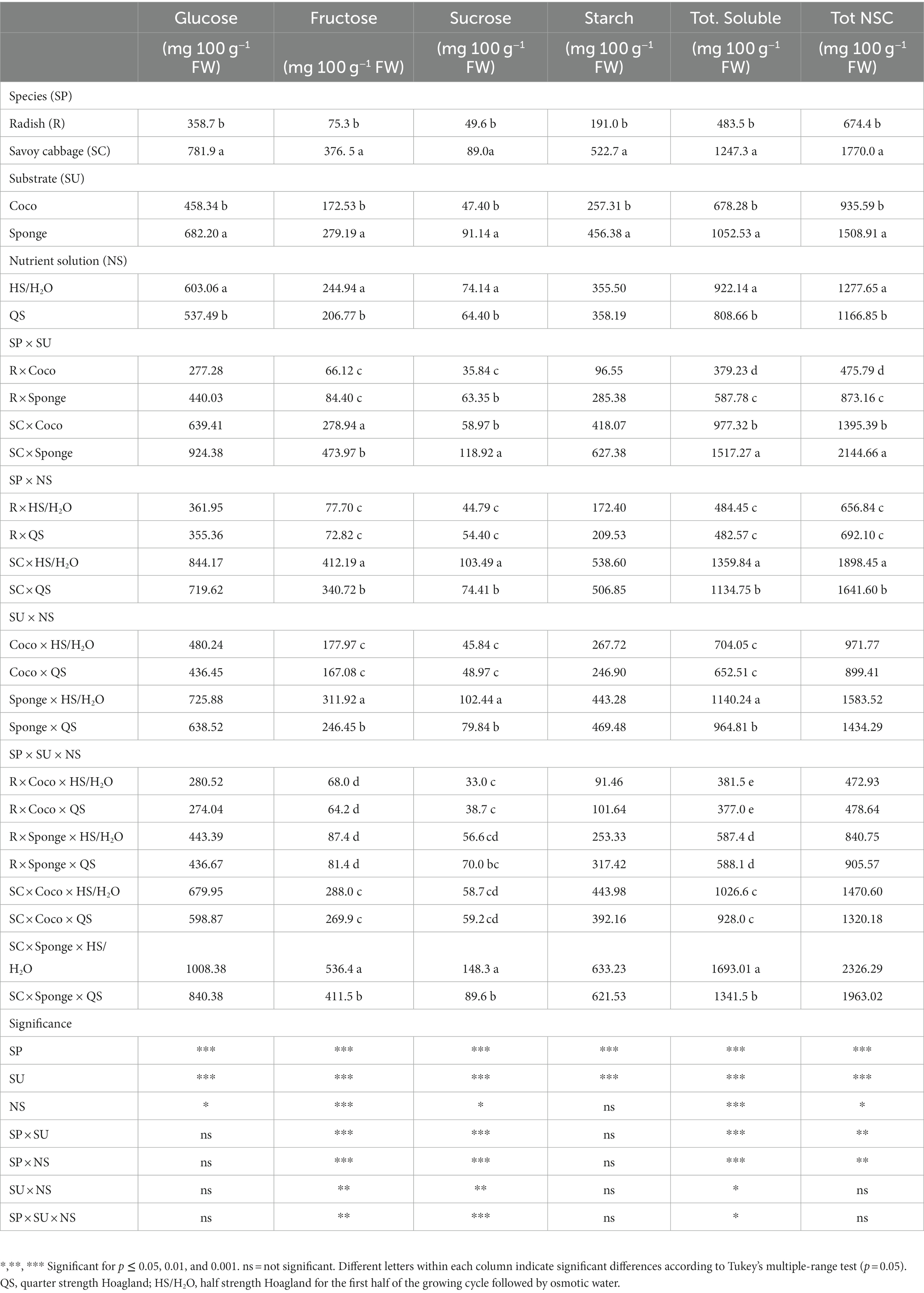
Table 4. Microgreens glucose, fructose, sucrose, starch, total soluble and total non-structural carbohydrates expressed in mg 100 g−1 fresh weight (FW).
3.5. Anions content
The nitrate, sulfate and chloride content are shown in Table 5. The nitrate content showed significant differences due to the interaction between the three factors analyzed, resulting in higher level in the combination between radish × Coco × QS. The lowest content was found in the combination savoy cabbage × Sponge with no differences between the two NS. The sulfate content was on average 22.3 mg 100 g−1 FW, ranging among 13.17 and 32.7 mg 100 g−1 FW in the combination’s radish × Coco and Sponge × HS/H2O, respectively, (Table 5). Sulfate content was influenced by the interaction between SU × NS with the effect of the different NS can be seen in the Sponge substrate where the QS result in lower content. Between the substrates higher levels of sulfate is accumulated with the use of Sponge. The range of chloride content varies from a minimum of 25.34 mg 100 g−1 FW in the combination radish × Coco × HS/H2O to a maximum of 53.15 mg 100 g−1 FW in the combination radish × Sponge x HS/H2O. The chloride content appears to be influenced by the SP × SU interaction with a greater accumulation in the combination of radish grown on Sponge, which presents an increase of 56.0% compared to the average of the other combinations, which have no accumulation difference for this compound (Table 5).
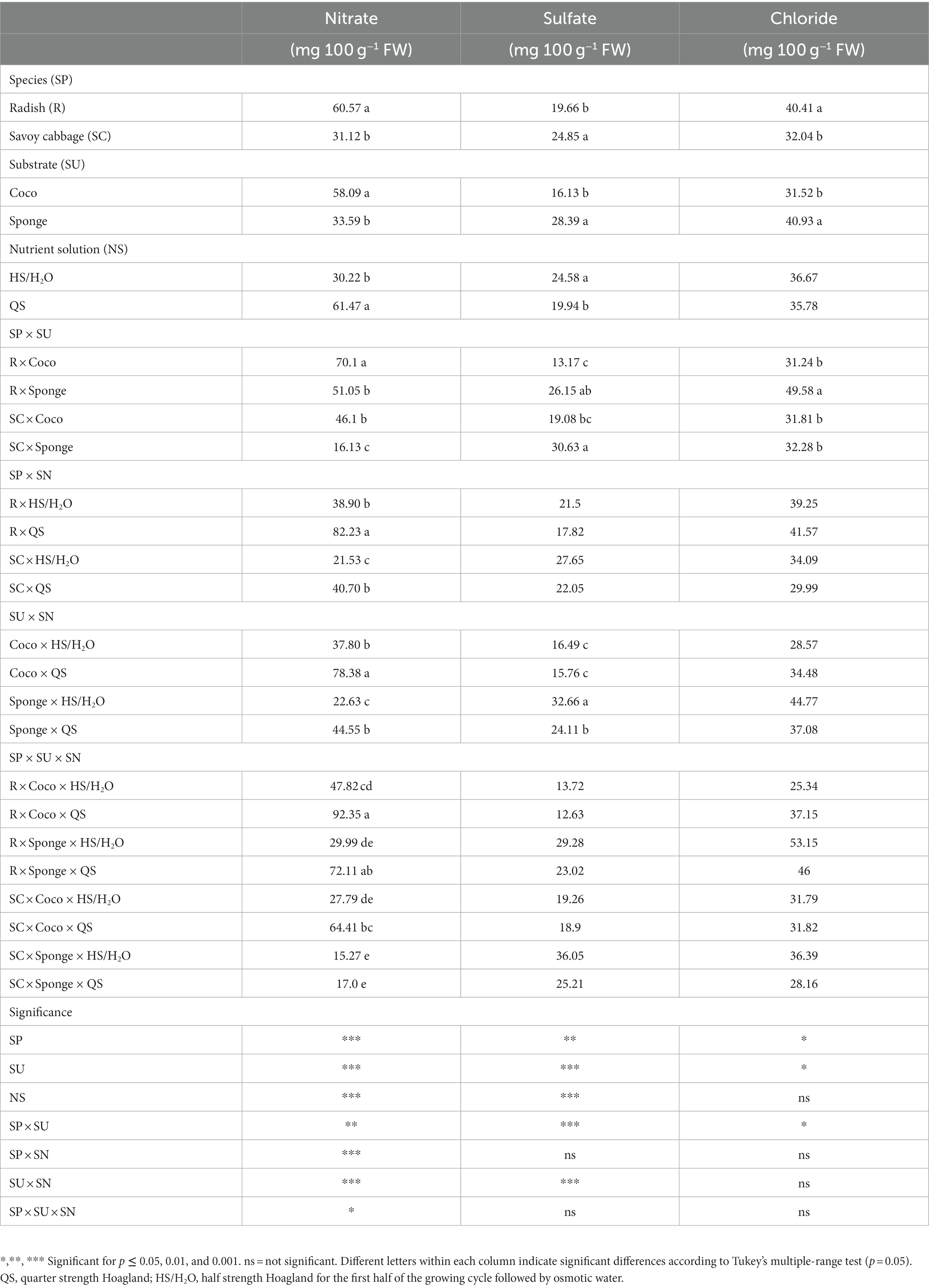
Table 5. Microgreens nitrate, sulfate and chloride content expressed in mg 100 g−1 fresh weight (FW).
3.6. Daily total ascorbic acid production, cultivation surface size and microgreens serving
The daily total ascorbic acid production (DTAP) in radish × Coco × HS/H2O was almost twice that of the combination savoy cabbage × Sponge × HS/H2O (Table 6). However, according to the statistical analysis, differences in the CSS are mainly attributable to the different interaction of SP and SU (p < 0.05). The minimal surface needed to produce 90 mg day−1 of ascorbate is achieved by the combination of radish × Coco, radish × Sponge and savoy cabbage × Coco with an average of 0.59 m2, while, in comparison, savoy cabbage × Sponge combination required a significative larger surface. The useful amount of fresh microgreen serving (Serving) for the intake of 90 mg of ascorbate is influenced by the interaction of all the three factors. According to Tukey’s test, the intake of the AR of ascorbate through the consumption of microgreens produced by the combination radish × Coco × QS required an amount of biomass 1.7 times higher than other tested combinations. The minimal serving useful to achieve the AR is 80 g.
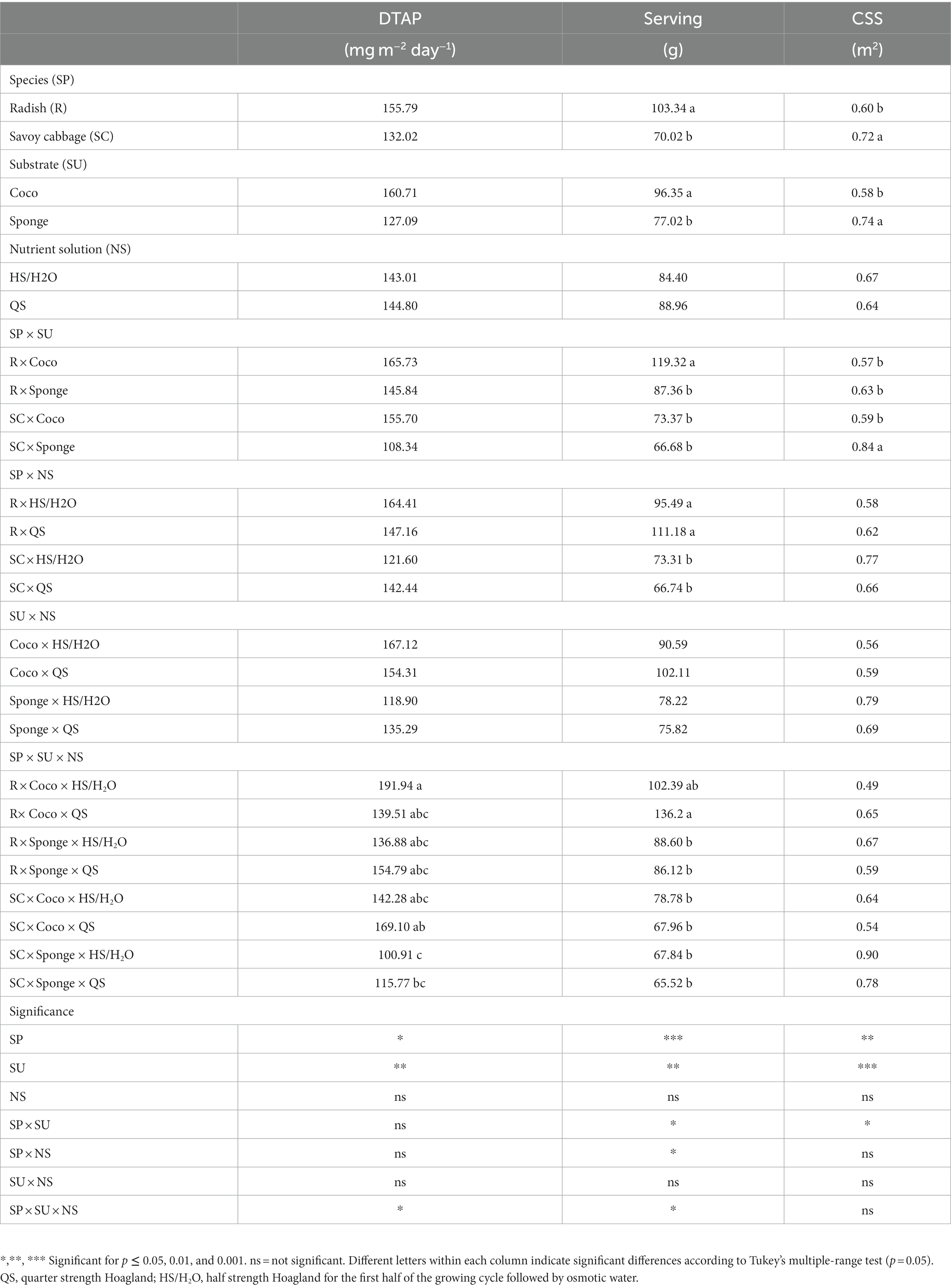
Table 6. Daily total ascorbate production (DTAP), fresh microgreens biomass to achieve the adequate daily intake of 90 mg of ascorbate (Serving) and the cultivation surface size to produce the AR of 90 mg of ascorbate (CSS) elaborated for each test factor expressed as mg m−2 day−1, g and m2, respectively.
3.7. Principal component analysis
Principal component analysis (PCA) has demonstrated to represent an adequate approach of collectively representing sample population divergence over multiple traits of productivity and quality in return for numerous cultivation factors (Kyriacou et al., 2020). In the current study, the PCA facilitated a brief view of the affinity among the microgreens species performance, their compositional variables and their daily total ascorbic acid production, cultivation surface size and microgreens serving (Figure 1). The species-dependent effects of the two substrates and nutrient solutions were highly visualized due to the good quality of the PCA loading and score plots as designated by the high percentage of the total variance (82.5%). The first two Principal Components (PCs) were correlated with eigenvalues greater than 1 (data not shown) and explained 82.5% of the cumulative variance, with PC1 accounting for 67.4% and PC2 for 15.1%. The biplot showed that PC1 was positively correlated with dry matter as biometric parameter and most of the quality attributes, including Tot. AscA, TPC, neoxanthin, lutein, β-carotene, glucose, fructose, sucrose, starch, Tot. soluble, Tot NSC, and CSS. PC1 was as well negatively correlated with two components of the biometric parameters (fresh and dry weight), nitrate, DAP and the serving. Moreover, PC2 was positively correlated with total Ant., violaxanthin, sulfate and chloride, while it was negatively correlated with chlorophylls a and b. In this controlled environment study, the score plot of the PCA integrated information on the bioactive profile of the two microgreens species subjected to two different nutrient solutions management and two different substrates: savoy cabbage was characterized by higher lutein, β-carotene, chlorophylls, total anthocyanins, total ascorbic acid, TPC, soluble sugars, total NSC, starch. Whereas, radish was superior in fresh and dry weight, DTAP, serving and nitrate. In particular, savoy cabbage cultivated on Sponge substrate and subjected to HS/H2O was positioned on the positive side of PC1 in the upper right quadrant of the PCA score plot as it delivered high quality microgreens (Figure 1). In addition, the same species cultivated on Sponge substrate and subjected to QS and cultivated on coco fiber with both nutrient solutions was positioned in the lower right quadrant. Radish microgreens grown on Sponge substrate with both nutrient solutions plus grown on coco fiber and subjected to QS were positioned in the upper left quadrant (Figure 1) and characterized by high serving, dry weight and chloride. Finally, the lower left quadrant depicted the treatment radish cultivated on Coco and subjected to HS/H2O. Thus, the effected PCA in this study illustrated a broad view of yield, quality traits and serving of radish and savoy microgreens.
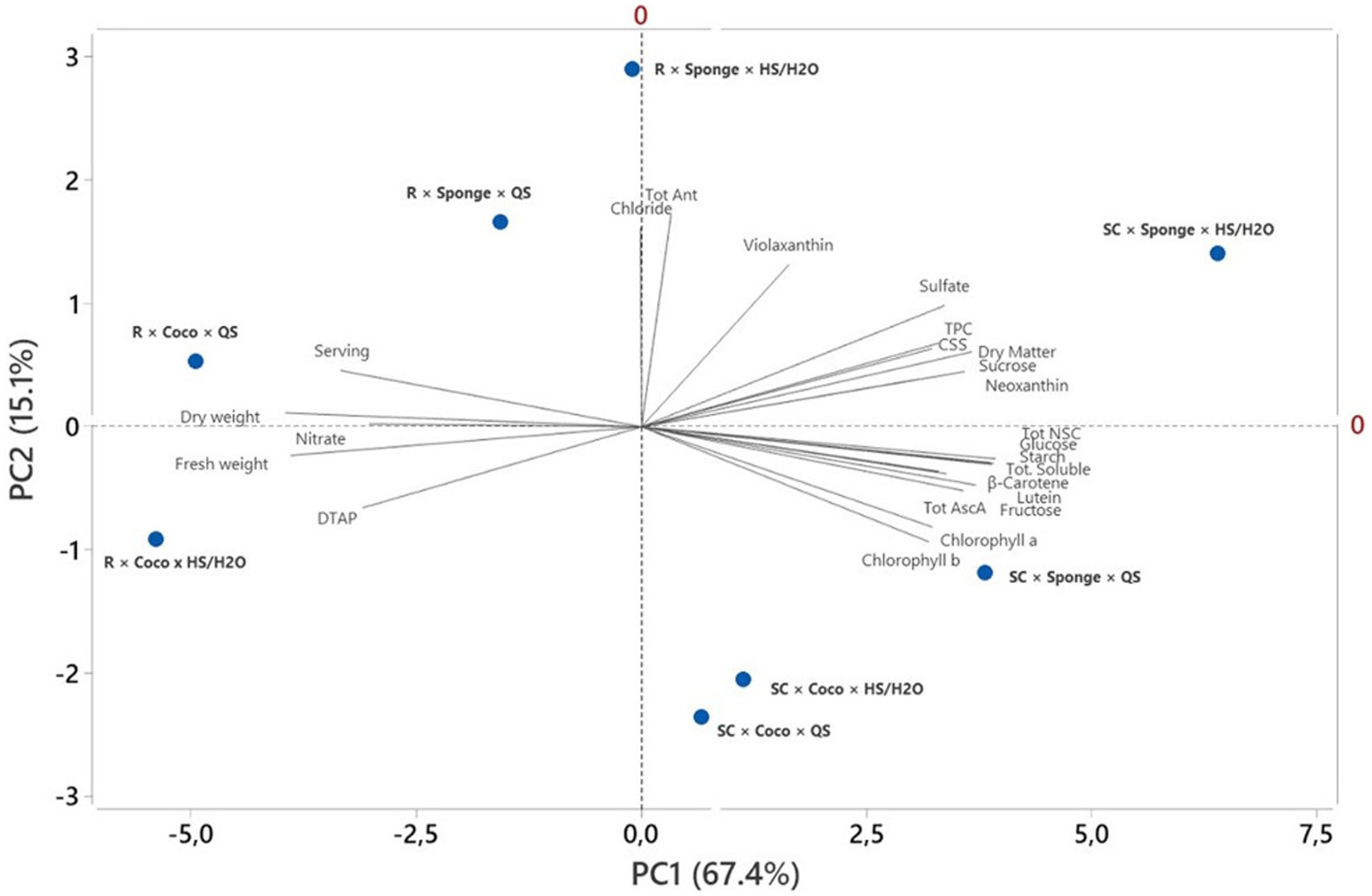
Figure 1. Principal component analysis loading plot of biomass parameter (fresh weight, dry weight and dry matter), quality parameters (Tot AscA, Tot Ant, TPC, violaxanthin, neoxanthin, lutein, chlorophyll a, chlorophyll b, β-carotene, glucose, fructose, sucrose, total soluble, total NSC, nitrate, sulfate and chloride), daily total ascorbate production (DTAP), microgreens serving to supply the AR of ascorbate (Serving) and the cultivation surface size for the production of ascorbate AR (CSS) of savoy cabbage (SC) and radish (R) microgreens grown on coconut fiber or cellulose sponge and supplied with quarter strength Hoagland nutrient solution (QS) or half strength Hoagland/osmotic water (HS/H2O).
4. Discussion
In order to optimize the yield and nutritional quality of microgreens, the choice of plant species is a critical factor (Kyriacou et al., 2019a,b; Caracciolo et al., 2020; Izzo et al., 2023). This is confirmed by the different production responses (fresh and dry yield) of the two microgreen species used, independently of the influence of the substrate and NS management. Interestingly, the yield obtained for radish (2.45 kg m−2), the most productive species in our experiment, was lower than what was recorded by Kyriacou et al. (2019a) for radish Sango (5.97 kg m−2) in a recent work in which 13 different species of microgreens grown on peat were characterized from a productive and phytochemical point of view. This significant difference is attributed to the harvest stage difference (cotyledons vs. two true leaves), in addition to the substrates used in this experiment vs. the peat moss-based substrate used for the screening. In a different study of Izzo et al. (2023), the same radish and savoy cabbage species were both cultivated on peat-based substrate and demonstrated higher yield. As reported by Kyriacou et al. (2020), the chemical and physical characteristics of the substrates significantly influenced the production response of microgreens. According to the authors’ hypothesis, the lower fresh yield obtained in our experiment could be attributed to a lower air capacity of Sponge and Coco, compared to peat. This characteristic, typical of coherent substrates characterized by a higher micropore content, would hinder root growth and justify the higher dry matter (8.26%, on average) obtained, compared to Kyriacou et al. (2019a,b; 4.10–6.05%). However, for both species of microgreens, Coco ensured a better response to production, confirming what was reported in part by Kyriacou et al. (2020). In contrast, dry matter percentage did not show the same trend as observed for fresh production, demonstrating that the species response to the substrate for this parameter is not uniform. Relative to the effect induced by the management of different NS, regardless of the cultivar, the mid-crop cycle replacement of the half-strength solution with osmotic water only (HS/H2O) resulted in significant differences in fresh yield compared to quarter-strength (QS) NS. While QS treated and HS/H2O treated delivered the same amount of nutrients, the difference in supply and deficiency (particularly of N and P) in the later growth stage (higher rooting and transpiration activity) could explain this result. Notably, a reduction in fresh production and an increase in dry matter were recorded only for microgreens grown on Sponge, again confirming the influence of the chemical and physical characteristics of the substrates on productivity.
Recently there has been a significant increase in research focusing on the nutritional aspects of microgreens, also oriented on the positive health outcomes that certain species can exert (De la Fuente et al., 2020, Li et al., 2021; Khattab et al., 2022). Hence, species selection can be also based on the type of phytonutrient needed to achieve a balanced diet and specific nutritional benefits. Although, we found that the content of certain phytochemicals, such as lutein and chlorophylls, are mainly determined by the plant genotype, the biochemical profile of microgreens can be influenced, in a species-specific way, by the cultivation regimen. A significant interaction between species and substrates on anthocyanins (Bulgari et al., 2021), polyphenols and β-carotene (Kyriacou et al., 2020) contents were already reported. These compounds have relevant nutraceutical functions since their antioxidant potentials can be helpful to counteract the detrimental effect of the space environment (De Micco et al., 2021; Gómez et al., 2021; Gonçalves et al., 2021). In our study, the interaction between species and substrates affected Tot. Ant., TPC, and β-carotene content, but also the accumulation of sulfate and chloride. Brassicaceae are the main contributors of dietary sulphate and 0.5 mg g−1 is considered a high concentration of sulfate in food and can negatively affect human health (Florin et al., 1991, 1993). Although different growing conditions in the experiment affected their content, the sulfate concentration in microgreens was consistently recorded as being lower than 0.5 mg g−1. EFSA has set a safe and adequate intake for chloride to 3.1 g day−1. The content in a 100 g serving of microgreens, obtained under the best chloride accumulator combination, would carry a very low dose of chloride, well below the daily threshold for an adult person, and would therefore not represent a threat for astronaut health.
Anthocyanins content strongly depends on the genetic background (Prinsi et al., 2019), but the modulation potential of environmental factors such as light regime and temperature is generally recognized (Rabino and Mancinelli, 1986). Our data show that mineral nutrition can be used to modulate anthocyanins content, and that this effect might be prone to interaction with other agronomical factors. In fact, we showed that the HS/H2O NS regime leads to an increase in anthocyanin content when combined with the cellulose sponge substrate, representing the first report on significant interaction between substrate and nutrition management on microgreens anthocyanins content. The accumulation of anthocyanins in vegetative tissues represents a general plant reaction to various stressors including nutrient deficiency (Stewart et al., 2001; Jiang et al., 2007; Yuan et al., 2009). In our work, the accumulation of anthocyanins is boosted through nutritional stress induced by nutrient deprivation, as already reported in other studies (El-Nakhel et al., 2019, 2021; Pannico et al., 2020). However, the role of the substrate in contributing to the accumulation of these compounds deserve more investigations. Notably, in the work by Bulgari et al. (2021), the anthocyanins content of red basil was enhanced by vermiculite use (pH 7–8), while this accumulation was lower in coconut fiber (pH 5.5–7). In our study, the cellulose Sponge substrate could be a potential contributor to the nutritional stress due to his alkaline pH (pH 8.49; Kyriacou et al., 2020). The favorable pH of coconut fiber (pH 5.34) could facilitate nutrient absorption, thus mitigating the nutritional stress induced by the HS/H20 nutrient solution. This, in turn, may lead to a less anthocyanins buildup when compared to the cellulosic sponge substrate.
HS/H2O NS also resulted in enhanced fructose and sucrose content when combined with the Sponge and savoy cabbage. The content of these soluble sugar could be an important post-harvest attribute influencing the shelf-life and the taste of the microgreens produce (Fallovo et al., 2009; Bulgari et al., 2021). Our results suggest that, depending on the specific species and substrate, NS management could represent a valuable tool to improve the quality of microgreens by increasing the fructose and sucrose content. The nitrate content is another important parameter for determining the quality of leafy vegetables (Colla et al., 2018). Nitrate intake might affect metabolism in humans, thereby producing a decrease in blood pressure (Kurtz et al., 2018). While this might be a positive effect on humans on Earth, it could be detrimental in space where low blood pressure is already induced by microgravity (Hughson et al., 2018). Although the level reached in microgreens for all the tested treatments is much lower than the threshold defined by the European Commission Regulation No 1258/2011, the use of different conditions can determine a different accumulation level of these compounds. In particular, the lower content was detected in combination with savoy cabbage and Sponge. Although in this last combination the different NS did not produce different results, in all the other combinations the sub-optimal nutrient conditions achieved through the use of HS/H2O treatments resulted in a lower accumulation of nitrate.
Nutrient deprivation strategies involve withholding or limiting the supply of nutrients to plants for a period of time before they are harvested to trigger the plant secondary metabolome and enhance crop quality (El-Nakhel et al., 2019). Significant increases in the content of total ascorbic acid, anthocyanins, and phenolic acids were already achieved through nutrient supply deprivation in lettuce microgreens, with a decrease also in the content of nitrates (Pannico et al., 2020). Our study found significant interactions between the factors that affect the accumulation of phytochemicals in the two tested species. This highlighted the importance of using different species for microgreens production in space and of scientifically planned production conditions for obtaining food products with various nutraceutical characteristics. On the other hand, the variability in the response of the two species to the growth conditions used underlines the need for precise control of environmental conditions and in-depth knowledge of how to manage them. While this may represent a potential complication, it can also be used to increase the system versatility, develop an expert system, or manage targeted phytonutrient production. As stated by Mortazavi et al. (2015), ascorbic acid (AscA) is a potential non-toxic and cost-effective radioprotector that might play an important role in counteracting radiation damage in space. Known as Vitamin C, AscA is a well-known antioxidant and the most widely used vitamin supplement worldwide. In addition to its antioxidant activity, AscA has been shown to have anti-atherogenic and anti-carcinogenic properties (Naidu, 2003). In the context of human space exploration, AscA can help to neutralize free radicals and protect cells from damage induced by ionizing radiation (Gómez et al., 2021). It is also involved in maintaining the immune system function, which is strongly affected during space travel (Crucian et al., 2018). AscA is a labile molecule, which can be easily damaged or destroyed by heat, light, and oxygen. It is, therefore, clear that the on-board production of fresh food containing elevated nutraceutical properties is necessary to provide an adequate intake of AscA. In this prospective, AscA could be considered a quality driving attribute on the basis of which the dimensions of closed microgreens cultivation system should be determined to achieve the AscA daily doses for astronauts. The EFSA recommended daily intake of vitamin C is 90 mg day−1 for adult men, and 75 mg day−1 for adult women. These recommendations are based on the average daily intake that is sufficient to meet the nutritional requirements and to prevent deficiency. The data indicate that microgreens have high concentrations of vitamin C, but the concentration can vary greatly depending on the trial and conditions tested. The efficiency of a production combination on phytonutrients, can be evaluated on the basis of two fundamental variables: (a) the concentration of one or several phytonutrients; (b) the productivity per day and unit area in one or several phytonutrients, which is determined by the previous parameter in combination with the productivity in biomass. A high concentration is important as it contributes to the nutrient productivity of the system and allows for easy daily consumption with a relatively small amount of food.
5. Conclusion
The current study comprises a novel report on how different substrates and nutrient solution management can impact the growth and the phytochemicals of radish and savoy cabbage microgreens. Our results indicate that cultivating on coco fiber, a higher fresh weight is obtained, while when applying NS/H2O total Ant. can be triggered, while Sponge substrate boost TPC. By using the right conditions, the daily recommended AscA intake can be achieved with 80 grams of fresh biomass. The tests conducted showed considerable variability in daily productivity and unit area and, for example, the cultivation of radish on Coco severely increased the fresh biomass required for the achievements of the AR. In our trial, less than 0.6 m2 of cultivation area could ensure the average daily dose when considering the optimal species and substrate combination (radish cultivated on both substrates and savoy cabbage on Coco). Greater area would be required in less optimal production conditions such as those provided by savoy cabbage and Sponge, but this choice could still be viable if other characteristics are targeted.
Data availability statement
The raw data supporting the conclusions of this article will be made available by the authors, without undue reservation.
Author contributions
YR, AB, and StP: supervision, conceptualization, resources, and coordination. StP, AB, and MB: funding acquisition. CN, MC, and LF run the experiments, the sampling, and the production parameters. GP, SM, and SiP run the biochemical analysis. GP worked on data curation and on the manuscript preparation. GP, MC, and LF worked on manuscript review and editing. All authors contributed to the article and approved the submitted version.
Funding
This research was conducted in the framework of the project “Systems and technologies for the production of microgreens in the Space ‘Microgreens x Microgravity’ -MICROx2” carried out with the collaboration and the coordination of the Italian Space Agency (ASI). Agreement n. 2021-2-HH.0.
Acknowledgments
The authors would like to thank Antonio Pannico, Luca Scognamiglio, and Giuseppe Galano for their technical help throughout the experiment.
Conflict of interest
The authors declare that the research was conducted in the absence of any commercial or financial relationships that could be construed as a potential conflict of interest.
Publisher’s note
All claims expressed in this article are solely those of the authors and do not necessarily represent those of their affiliated organizations, or those of the publisher, the editors and the reviewers. Any product that may be evaluated in this article, or claim that may be made by its manufacturer, is not guaranteed or endorsed by the publisher.
Supplementary material
The Supplementary material for this article can be found online at: https://www.frontiersin.org/articles/10.3389/fsufs.2023.1222914/full#supplementary-material
References
Amitrano, C., Paglialunga, G., Battistelli, A., De Micco, V., Del Bianco, M., Liuzzi, G., et al. (2023). Defining growth requirements of microgreens in space cultivation via biomass production, morpho-anatomical and nutritional traits analysis. Front. Plant Sci. 14:1190945. doi: 10.3389/fpls.2023.1190945
Bulgari, R., Baldi, A., Ferrante, A., and Lenzi, A. (2017). Yield and quality of basil, Swiss chard, and rocket microgreens grown in a hydroponic system. N. Z. J. Crop. Hortic. Sci. 45, 119–129. doi: 10.1080/01140671.2016.1259642
Bulgari, R., Negri, M., Santoro, P., and Ferrante, A. (2021). Quality evaluation of indoor-grown microgreens cultivated on three different substrates. Horticulturae 7:96. doi: 10.3390/horticulturae7050096
Caracciolo, F., El-Nakhel, C., Raimondo, M., Kyriacou, M. C., Cembalo, L., De Pascale, S., et al. (2020). Sensory attributes and consumer acceptability of 12 microgreens species. Agronomy 10:1043. doi: 10.3390/agronomy10071043
Chebrolu, K. K., Jayaprakasha, G. K., Yoo, K. S., Jifon, J. L., and Patil, B. S. (2012). An improved sample preparation method for quantification of ascorbic acid and dehydroascorbic acid by HPLC. LWT 47, 443–449. doi: 10.1016/j.lwt.2012.02.004
Colla, G., Kim, H. J., Kyriacou, M. C., and Rouphael, Y. (2018). Nitrate in fruits and vegetables. Sci. Hortic. 237, 221–238. doi: 10.1016/j.scienta.2018.04.016
Crucian, B. E., Choukèr, A., Simpson, R. J., Mehta, S., Marshall, G., Smith, S. M., et al. (2018). Immune system dysregulation during spaceflight: potential countermeasures for deep space exploration missions. Front. Immunol. 9:1437. doi: 10.3389/fimmu.2018.01437
De la Fuente, B., López-García, G., Máñez, V., Alegría, A., Barberá, R., and Cilla, A. (2019). Evaluation of the bioaccessibility of antioxidant bioactive compounds and minerals of four genotypes of Brassicaceae microgreens. Foods 8:250. doi: 10.3390/foods8070250
De la Fuente, B., López-García, G., Máñez, V., Alegría, A., Barberá, R., and Cilla, A. (2020). Antiproliferative effect of bioaccessible fractions of four Brassicaceae microgreens on human colon cancer cells linked to their phytochemical composition. Antioxidants 9:368. doi: 10.3390/antiox9050368
De Micco, V., Amitrano, C., Vitaglione, P., Ferracane, R., Pugliese, M., and Arena, C. (2021). Effect of light quality and ionising radiation on morphological and nutraceutical traits of sprouts for astronauts’ diet. Acta Astronautica. 185, 188–197. doi: 10.1016/j.actaastro.2021.05.007
Di Gioia, F., De Bellis, P., Mininni, C., Santamaria, P., and Serio, F. (2017a). Physicochemical, agronomical and microbiological evaluation of alternative growing media for the production of rapini (Brassica rapa L.) microgreens. J. Sci. Food Agric. 97, 1212–1219. doi: 10.1002/jsfa.7852
Di Gioia, F., Renna, M., and Santamaria, P. (2017b). Sprouts, microgreens and “baby leaf” vegetables. Minim. Process. Refrig. Fruits Veg. 403-432. doi: 10.1007/978-1-4939-7018-6
Douglas, G. L., Zwart, S. R., and Smith, S. M. (2020). Space food for thought: challenges and considerations for food and nutrition on exploration missions. J. Nutr. 150, 2242–2244. doi: 10.1093/jn/nxaa188
EFSA Panel on Dietetic Products, Nutrition and Allergies (NDA) (2013). Scientific opinion on dietary reference values for vitamin C. EFSA J. 11:3418. doi: 10.2903/j.efsa.2013.3418
El-Nakhel, C., Pannico, A., Graziani, G., Kyriacou, M. C., Gaspari, A., Ritieni, A., et al. (2021). Nutrient supplementation configures the bioactive profile and production characteristics of three Brassica L. microgreens species grown in peat-based media. Agronomy 11:346. doi: 10.3390/agronomy11020346
El-Nakhel, C., Pannico, A., Kyriacou, M. C., Giordano, M., De Pascale, S., and Rouphael, Y. (2019). Macronutrient deprivation eustress elicits differential secondary metabolites in red and green-pigmented butterhead lettuce grown in a closed soilless system. J. Sci. Food Agric. 99, 6962–6972. doi: 10.1002/jsfa.9985
Fallovo, C., Rouphael, Y., Cardarelli, M., Rea, E., Battistelli, A., and Colla, G. (2009). Yield and quality of leafy lettuce in response to nutrient solution composition and growing season. J. Food Agric. Environ 7, 456–462.
Florin, T., Neale, G., Gibson, G. R., Christl, S. U., and Cummings, J. H. (1991). Metabolism of dietary sulphate: absorption and excretion in humans. Gut 32, 766–773. doi: 10.1136/gut.32.7.766
Florin, T. H., Neale, G., Goretski, S., and Cummings, J. H. (1993). The sulfate content of foods and beverages. J. Food Compos. Anal. 6, 140–151. doi: 10.1006/jfca.1993.1016
Galieni, A., Falcinelli, B., Stagnari, F., Datti, A., and Benincasa, P. (2020). Sprouts and microgreens: trends, opportunities, and horizons for novel research. Agronomy 10:1424. doi: 10.3390/agronomy10091424
Gómez, X., Sanon, S., Zambrano, K., Asquel, S., Bassantes, M., Morales, J. E., et al. (2021). Key points for the development of antioxidant cocktails to prevent cellular stress and damage caused by reactive oxygen species (ROS) during manned space missions. npj Microgravity 7:35. doi: 10.1038/s41526-021-00162-8
Gonçalves, A. C., Nunes, A. R., Falcão, A., Alves, G., and Silva, L. R. (2021). Dietary effects of anthocyanins in human health: a comprehensive review. Pharmaceuticals 14:690. doi: 10.3390/ph14070690
Gupta, A., Sharma, T., Singh, S. P., Bhardwaj, A., Srivastava, D., and Kumar, R. (2023). Prospects of microgreens as budding living functional food: breeding and biofortification through OMICS and other approaches for nutritional security. Front. Genet. 14:1053810. doi: 10.3389/fgene.2023.1053810
Hughson, R. L., Helm, A., and Durante, M. (2018). Heart in space: effect of the extraterrestrial environment on the cardiovascular system. Nat. Rev. Cardiol. 15, 167–180. doi: 10.1038/nrcardio.2017.157
Izzo, L. G., El Nakhel, C., Rouphael, Y., Proietti, S., Paglialunga, G., Moscatello, S., et al. (2023). Applying productivity and phytonutrient profile criteria in modelling species selection of microgreens as space crops for astronaut consumption. Front. Plant Sci. 14:1210566. doi: 10.3389/fpls.2023.1210566
Jambor, T., Knizatova, N., Valkova, V., Tirpak, F., Greifova, H., Kovacik, A., et al. (2022). Microgreens as a fuctional component of the human diet: a review. J. Microbiol. Biotechnol. Food Sci. 12:e5870. doi: 10.55251/jmbfs.5870
Jiang, C., Gao, X., Liao, L., Harberd, N. P., and Fu, X. (2007). Phosphate starvation root architecture and anthocyanin accumulation responses are modulated by the gibberellin-DELLA signaling pathway in Arabidopsis. Plant Physiol. 145, 1460–1470. doi: 10.1104/pp.107.103788
Keutgen, N., Hausknecht, M., Tomaszewska-Sowa, M., and Keutgen, A. J. (2021). Nutritional and sensory quality of two types of cress microgreens depending on the mineral nutrition. Agronomy 11:1110. doi: 10.3390/agronomy11061110
Khattab, M. S., Aly, T. A., Mohamed, S. M., Naguib, A. M. M., AL-Farga, A., and Abdel-Rahim, E. A. (2022). Hordeum vulgare L. microgreen mitigates reproductive dysfunction and oxidative stress in streptozotocin-induced diabetes and aflatoxicosis in male rats. Food Sci. Nutr. 10, 3355–3367. doi: 10.1002/fsn3.2936
Kurtz, T. W., DiCarlo, S. E., Pravenec, M., and Morris, R. C. (2018). Functional foods for augmenting nitric oxide activity and reducing the risk for salt-induced hypertension and cardiovascular disease in Japan. J. Cardiol. 72, 42–49. doi: 10.1016/j.jjcc.2018.02.003
Kyriacou, M. C., De Pascale, S., Kyratzis, A., and Rouphael, Y. (2017). Microgreens as a component of space life support systems: a cornucopia of functional food. Front. Plant Sci. 8:1527. doi: 10.3389/fpls.2017.01587
Kyriacou, M. C., El-Nakhel, C., Graziani, G., Pannico, A., Soteriou, G. A., Giordano, M., et al. (2019a). Functional quality in novel food sources: genotypic variation in the nutritive and phytochemical composition of thirteen microgreens species. Food Chem. 277, 107–118. doi: 10.1016/j.foodchem.2018.10.098
Kyriacou, M. C., El-Nakhel, C., Pannico, A., Graziani, G., Soteriou, G. A., Giordano, M., et al. (2020). Phenolic constitution, phytochemical and macronutrient content in three species of microgreens as modulated by natural fiber and synthetic substrates. Antioxidants 9:252. doi: 10.3390/antiox9030252
Kyriacou, M. C., El-Nakhel, C., Pannico, A., Graziani, G., Soteriou, G. A., Giordano, M., et al. (2019b). Genotype-specific modulatory effects of select spectral bandwidths on the nutritive and phytochemical composition of microgreens. Front. Plant Sci. 10:1501. doi: 10.3389/fpls.2019.01501
Kyriacou, M. C., El-Nakhel, C., Soteriou, G. A., Graziani, G., Kyratzis, A., Antoniou, C., et al. (2021). Preharvest nutrient deprivation reconfigures nitrate, mineral, and phytochemical content of microgreens. Foods 10:1333. doi: 10.3390/foods10061333
Kyriacou, M. C., Rouphael, Y., Di Gioia, F., Kyratzis, A., Serio, F., Renna, M., et al. (2016). Micro-scale vegetable production and the rise of microgreens. Trends Food Sci. Technol. 57, 103–115. doi: 10.1016/j.tifs.2016.09.005
Li, X., Tian, S., Wang, Y., Liu, J., Wang, J., and Lu, Y. (2021). Broccoli microgreens juice reduces body weight by enhancing insulin sensitivity and modulating gut microbiota in high-fat diet-induced C57BL/6J obese mice. Eur. J. Nutr. 60, 3829–3839. doi: 10.1007/s00394-021-02553-9
Mortazavi, S. M. J., Foadi, M., Mozdarani, H., Haghani, M., and Mosleh, M. A. (2015). Future role of vitamin C in radiation mitigation and its possible applications in manned deep space missions: survival study and the measurement of cell viability. Int. J. Radiat. Res. 13, 55–60. doi: 10.1016/j.ejmp.2014.07.278
Muchjajib, U., Muchjajib, S., Suknikom, S., and Butsai, J. (2015). Evaluation of organic media alternatives for the production of microgreens in Thailand. Acta Hortic. 1102, 157–162. doi: 10.17660/ActaHortic.2015.1102.19
Murphy, C., and Pill, W. (2010). Cultural practices to speed the growth of microgreen arugula (roquette; Eruca vesicaria subsp. sativa). J. Hortic. Sci. Biotechnol. 85, 171–176. doi: 10.1080/14620316.2010.11512650
Naidu, K. A. (2003). Vitamin C in human health and disease is still a mystery? An overview. Nutr. J. 2, 1–10. doi: 10.1186/1475-2891-2-7
Nguyen, M., Knowling, M., Tran, N. N., Burgess, A., Fisk, I., Watt, M., et al. (2022). Space farming: horticulture systems on spacecraft and outlook to planetary space exploration. Plant Physiol. Biochem. 194, 708–721. doi: 10.1016/j.plaphy.2022.12.017
Palmitessa, O. D., Renna, M., Crupi, P., Lovece, A., Corbo, F., and Santamaria, P. (2020). Yield and quality characteristics of Brassica microgreens as affected by the NH4: NO3 molar ratio and strength of the nutrient solution. Foods 9:677. doi: 10.3390/foods9050677
Pannico, A., Graziani, G., El-Nakhel, C., Giordano, M., Ritieni, A., Kyriacou, M. C., et al. (2020). Nutritional stress suppresses nitrate content and positively impacts ascorbic acid concentration and phenolic acids profile of lettuce microgreens. Italus Hortus 27, 41–52. doi: 10.26353/j.itahort/2020.3.4152
Petropoulos, S. A., El-Nakhel, C., Graziani, G., Kyriacou, M. C., and Rouphael, Y. (2021). The effects of nutrient solution feeding regime on yield, mineral profile, and phytochemical composition of spinach microgreens. Horticulturae 7:162. doi: 10.3390/horticulturae7070162
Poulet, L., Engeling, K., Hatch, T., Stahl-Rommel, S., Velez Justiniano, Y. A., Castro-Wallace, S., et al. (2022). Large-scale crop production for the moon and Mars: current gaps and future perspectives. Front. Astron. Space Sci. 8:733944. doi: 10.3389/fspas.2021.733944
Prinsi, B., Morgutti, S., Negrini, N., Faoro, F., and Espen, L. (2019). Insight into composition of bioactive phenolic compounds in leaves and flowers of green and purple basil. Plan. Theory 9:22. doi: 10.3390/plants9010022
Rabino, I., and Mancinelli, A. L. (1986). Light, temperature, and anthocyanin production. Plant Physiol. 81, 922–924. doi: 10.1104/pp.81.3.922
Rouphael, Y., Kyriacou, M. C., Petropoulos, S. A., De Pascale, S., and Colla, G. (2018). Improving vegetable quality in controlled environments. Sci. Hortic. 234, 275–289. doi: 10.1016/j.scienta.2018.02.033
Samuolienė, G., Brazaitytė, A., Jankauskienė, J., Viršilė, A., Sirtautas, R., Novičkovas, A., et al. (2013). LED irradiance level affects growth and nutritional quality of Brassica microgreens. Cent. Eur. J. Biol. 8, 1241–1249. doi: 10.2478/s11535-013-0246-1
Scartazza, A., Moscatello, S., Gavrichkova, O., Buia, M. C., Lauteri, M., Battistelli, A., et al. (2017). Carbon and nitrogen allocation strategy in Posidonia oceanica is altered by seawater acidification. Sci. Total Environ. 607-608, 954–964. doi: 10.1016/j.scitotenv.2017.06.084
Singh, N., Rani, S., and Chaurasia, O. P. (2020). Vegetable microgreens farming in high-altitude region of trans-Himalayas to maintain nutritional diet of Indian troops. Proc. Natl Acad. Sci. India. Sect. B Biol. Sci. 90, 743–752. doi: 10.1007/s40011-019-01147-0
Smith, S. M., Zwart, S. R., and Heer, M. (2014). Human adaptation to space flight: the role of nutrition. United States. National Aeronautics and Space Administration, Lyndon B, Johnson Space Center.
Stewart, A. J., Chapman, W., Jenkins, G. I., Graham, I., Martin, T., and Crozier, A. (2001). The effect of nitrogen and phosphorus deficiency on flavonol accumulation in plant tissues. Plant Cell Environ. 24, 1189–1197. doi: 10.1046/j.1365-3040.2001.00768.x
Teng, Z., Luo, Y., Pearlstein, D. J., Wheeler, R. M., Johnson, C. M., Wang, Q., et al. (2022). Microgreens for home, commercial, and space farming: a comprehensive update of the Most recent developments. Annu. Rev. Food Sci. Technol. 14, 539–562. doi: 10.1146/annurev-food-060721-024636
Usenik, V., Fabčič, J., and Štampar, F. (2008). Sugars, organic acids, phenolic composition and antioxidant activity of sweet cherry (Prunus avium L.). Food Chem. 107, 185–192. doi: 10.1016/j.foodchem.2007.08.004
Wang, Z. H., Li, S. X., and Malhi, S. (2008). Effects of fertilization and other agronomic measures on nutritional quality of crops. J. Sci. Food Agric. 88, 7–23. doi: 10.1002/jsfa.3084
Wheeler, R. M. (2010). Plants for human life support in space: from Myers to Mars. Gravitation. Sp. Biol. 23, 25–35.
Xiao, Z., Rausch, S. R., Luo, Y., Sun, J., Yu, L., Wang, Q., et al. (2019). Microgreens of Brassicaceae: Genetic diversity of phytochemical concentrations and antioxidant capacity. Lwt. 101, 731–737. doi: 10.1016/j.lwt.2018.10.076
Yuan, Y., Chiu, L. W., and Li, L. (2009). Transcriptional regulation of anthocyanin biosynthesis in red cabbage. Planta 230, 1141–1153. doi: 10.1007/s00425-009-1013-4
Zhang, Y., Xiao, Z., Ager, E., Kong, L., and Tan, L. (2021). Nutritional quality and health benefits of microgreens, a crop of modern agriculture. J. Future Foods 1, 58–66. doi: 10.1016/j.jfutfo.2021.07.001
Keywords: growing media, nutrient deprivation, nutraceuticals, cotyledons, HPLC, carbohydrates, Brassicaceae, growing module
Citation: Paglialunga G, El Nakhel C, Proietti S, Moscatello S, Battistelli A, Formisano L, Ciriello M, Del Bianco M, De Pascale S and Rouphael Y (2023) Substrate and fertigation management modulate microgreens production, quality and resource efficiency. Front. Sustain. Food Syst. 7:1222914. doi: 10.3389/fsufs.2023.1222914
Edited by:
Ashish Rawson, National Institute of Food Technology Entrepreneurship and Management, IndiaReviewed by:
Wenjun Deng, Qingdao University, ChinaRajendra Kumar, Indian Agricultural Research Institute (ICAR), India
Copyright © 2023 Paglialunga, El Nakhel, Proietti, Moscatello, Battistelli, Formisano, Ciriello, Del Bianco, De Pascale and Rouphael. This is an open-access article distributed under the terms of the Creative Commons Attribution License (CC BY). The use, distribution or reproduction in other forums is permitted, provided the original author(s) and the copyright owner(s) are credited and that the original publication in this journal is cited, in accordance with accepted academic practice. No use, distribution or reproduction is permitted which does not comply with these terms.
*Correspondence: Alberto Battistelli, YWxiZXJ0by5iYXR0aXN0ZWxsaUBjbnIuaXQ=