- 1Bangladesh Wheat and Maize Research Institute, Dinajpur, Bangladesh
- 2Borlaug Institute for South Asia, Ludhiana, Punjab, India
- 3Département de Phytologie, Université Laval, Québec City, QC, Canada
- 4Department of Crop Improvement, Kumaraguru Institute of Agriculture, Erode, Tamil Nadu, India
- 5Department of Water Resources and Environmental Engineering, Faculty of Horticulture and Landscape Engineering, Slovak University of Agriculture, Nitra, Slovakia
- 6Institute of Plant and Environmental Sciences, Slovak University of Agriculture, Nitra, Slovakia
Crop failure is largely caused by various climate hazards, and among them, heat stress is the primary factor hindering crop production. The significant global loss of crop yield is primarily due to heat-related damage during the reproductive phase. Terminal heat stress has been well documented in wheat, causing morphophysiological alterations, biochemical disruptions, and reduction of genetic potential. The formation of shoots and roots, the effect on the double ridge stage, and early biomass in the vegetative stage are also impacted by heat stress. The final negative outcomes of heat stress include reduced grain number and weight, slower grain filling rate, reduced grain quality, and shorter grain filling duration. Plants have developed mechanisms to adapt to heat stress through modifications in their morphological or growth responses, physiological and biochemical pathways, and changes in enzyme reactions. Numerous heat tolerance genes have been identified in wheat, but the more extensive study is needed to increase heat tolerance in crops to satisfy the food demands of the world’s growing population. The global food policy needs to prioritize and promote additional joint research and the development of heat-tolerant wheat breeding to ensure the world’s food security.
1. Introduction
The rate at which the world’s population is expanding is 1.1% every year (The World Bank, 2019) which is faster than the experts’ predictions a few years ago (Yale University, 2020); hence the food consumption rate is also increasing. Agricultural research is being achieved quantum leaps in terms of commodity and non-commodity outputs to serve global food production. However, this achievement is always under pressure and becomes unstable due to several reasons. Climate change imposes significant uncertainty on crop production due to biotic agents or abiotic stresses. The significant climatic hazards are heats, droughts, salinity, floods, unexpected high or low rainfall, humidity, increasing windstorms and decreasing groundwater table and resources. These climate hazards hinder crop production, particularly in developing countries having fewer resources.
Heat is a crucial influence impacting agricultural output, making it one of the most important abiotic stresses. The term “heat stress” refers to temperatures that are higher than the critical threshold. Extreme heat stress is defined by the magnitude, duration, and pace of temperature increase. Threshold temperature varies from crop type, growing season, and crop growth stages. Heat tolerance is the capability of living beings to develop naturally or to produce economic yield at a temperature above the threshold. A significant cause of yield loss in agricultural production globally is heat-related reproductive tissue damage in various crops (Suzuki et al., 2012). A strong negative association between high seasonal temperature and crop yields, which is largely correlated with heat stresses, has been consistently reported by Akter and Rafiqul Islam (2017), Gammans et al. (2017), and Cui (2020). It’s possible that the floodplain regions of Asian countries like China, India, Bangladesh, Nepal, Iran, etc., may be hit the worst by global climate change (Braun et al., 2010). The data of The National Oceanic and Atmospheric Administration (2023) provided a global view of climate change over time, showing a growing portion of the Earth’s surface becoming warmer.1 It was revealed from Figure 1 that global warming is very prominent in all continents of the world. It was revealed that winter crops determine several yield contributing factors like the grain numbers, grain weight and grain size during this time (Poudel and Poudel, 2020).
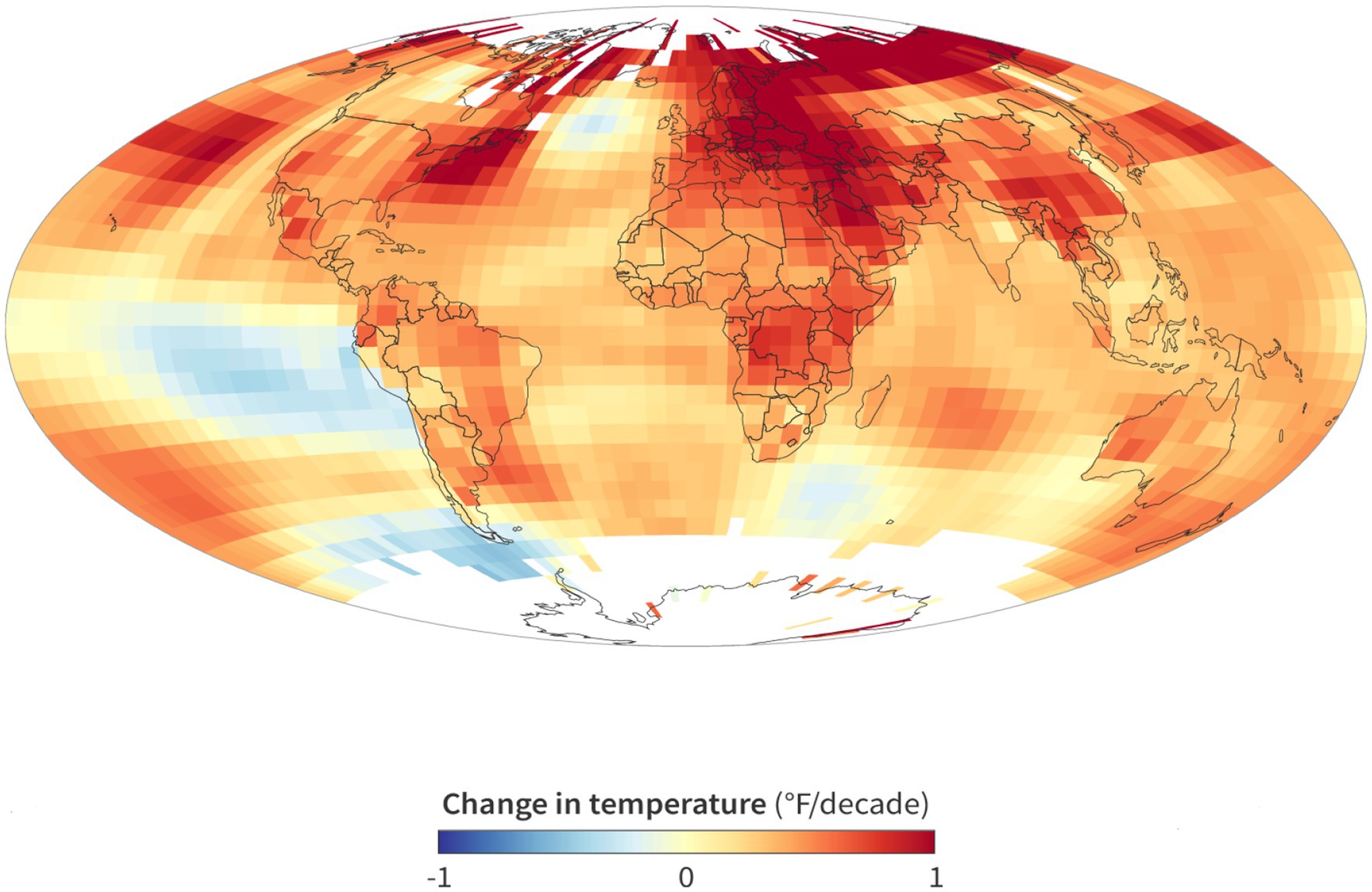
Figure 1. According to the map provided by NOAA Climate.gov, there is a discernible trend of rising global temperatures between the years 1993 and 2022, except for certain regions within the Southern Hemisphere oceans. https://www.climate.gov, Accessed on 28 April 2023.
Wheat is the prominent food grain globally which is grown in the winter season. The adverse effects of climate change on wheat production pose a danger to global food security, and sustained wheat output is anticipated to be increasingly negatively impacted by rising global temperatures (Tripathi et al., 2016). Heat stress has a negative impact on wheat output in various parts of the world. This is especially detrimental during the reproductive phase of the wheat plant (Mondal et al., 2013). It is essential that the implications of heat stress and potential means of enhancing heat tolerance for the profitability of wheat production in heat owing to global warming and alters in the extreme climate pattern be recognized. This is because heat stress and potential means of enhancing heat tolerance are directly related to the profitability of wheat production in heat. This is because these consequences must influence the performance of wheat production in heat.
Global wheat yield loss with the increase of each degree-Celsius temperature is 6.0 ± 2.9% (Zhao et al., 2017). The high seasonal climate in January and February affects the formation of the double ridge stage. The high temperature at the grain filling period is called terminal heat stress and the elevated temperature at sowing is called early heat stress. Wheat development as well as growth are facing a difficult constraint brought on by early and terminal heat shocks. A plant experiencing heat stress is the function of ambient temperature, agronomy and genetic factors that determine evaporative cooling potential (Braun et al., 2010). The majority of prior studies aimed to increase hexaploid wheat’s tolerance to heat stress by drawing on the variety present in its wild ancestors (Elbashir et al., 2017; Liu H. et al., 2019; Ma’arup et al., 2020; Poudel and Poudel, 2020; Elhadi et al., 2021; Itam et al., 2021, 2022; Ullah et al., 2021). Wild emmer wheat (T. turgidum ssp. dicoccoides) is considered a plausible resource due to its direct lineage to domesticated durum wheat (T. durum) and the A and B genomes of bread wheat (T. aestivum). The genetic introgression of wild emmer wheat for wheat improvement has been demonstrated to be feasible through the two lineages it possesses. Wild emmer wheat has been identified as a valuable resource for enhancing the resilience of wheat to environmental stressors (Peng J. et al., 2013; Tsujimoto et al., 2015; El Haddad et al., 2021) It is noteworthy that the inherent diversity of wild emmer wheat encompasses significant agronomic, physiological, and yield-related characteristics that are linked to the ability to withstand heat stress (Peng J. et al., 2013). The preservation of diversity in wild emmer wheat is essential for the enhancement and maintenance of wheat tolerance toward heat stress. Over the last decade, various omics methodologies have significantly transformed the manner in which biotechnologists and plant breeders explore the fundamental mechanisms of stress tolerance and cellular homeostasis (Yadav et al., 2022). In the upcoming sections, we will be discussing more on the specific heat stresses in wheat. When there is an increase in the average temperature throughout the winter, there is a major impact on wheat productivity and planting area. The effects of thermal stress on human well-being, especially food security, depend greatly on the combination of natural ecosystem stability and the approach taken by farmers and other stakeholders in the agricultural system. This review will also concentrate on basic heat stress in the development and growth of wheat, its morpho-physiological, biochemical, and molecular mechanisms, varietal improvement, or cultivation practices for heat stress management.
2. Impact of heat stress on wheat
Heat stress affects the whole development and growth system in plants, including phenological stages, morpho-physiological alteration, developmental process, biochemical reactions, and even genetic potentials to produce yield. Canopy humidity for evaporative cooling plays an important role in how plants respond to heat stress, and its intensity and duration may have a significant impact on how plants react (Braun et al., 2010). The temperature rise was directly related to a decrease in photosynthetic efficiency and ultimately a reduction in crop yield (Mathur et al., 2011). A computer modeling study on crop response revealed that global warming causes premature aging in wheat (Lobell et al., 2012). The high temperature of the day can damage the leaf photosynthesis components, reducing the rate of CO2 assimilation during the vegetative process compared to the optimal temperature setting. Heat stress inhibits the development of plants both throughout the plant growth and reproduction phases. Heat stress had a detrimental effect on the length of roots during the seedling stage, with notable differences observed among various wheat genotypes. Seedlings exhibiting heat tolerance demonstrated a lower reduction in root length compared to those that were susceptible. The wheat cultivars that exhibited tolerance during the seedling stage demonstrated a greater yield during the adult stage following exposure to heat treatment. It was found that heat stress had a detrimental effect on the length of roots during the seedling stage, with notable differences observed among various wheat genotypes. Wheat plants are susceptible to heat stress, which impedes growth, causes morpho-physiological changes in the plant, and eventually results in a large loss of yield and the heat stress can be caused by prolonged exposure to high temperatures (McClung and Davis, 2010; Grant et al., 2011). The response of plants to heat stress varies considerably with the degree and length of the temperature and the growth phases (Ruelland and Zachowski, 2010). The effects of high-temperature stress on growth and development may be seen in practically every developmental stage of the crop growth cycle (Cossani and Reynolds, 2012). Diagrammatic representation of the impact of heat stress on different major traits of wheat (Figure 2) and also discussed in different sub-headings below:
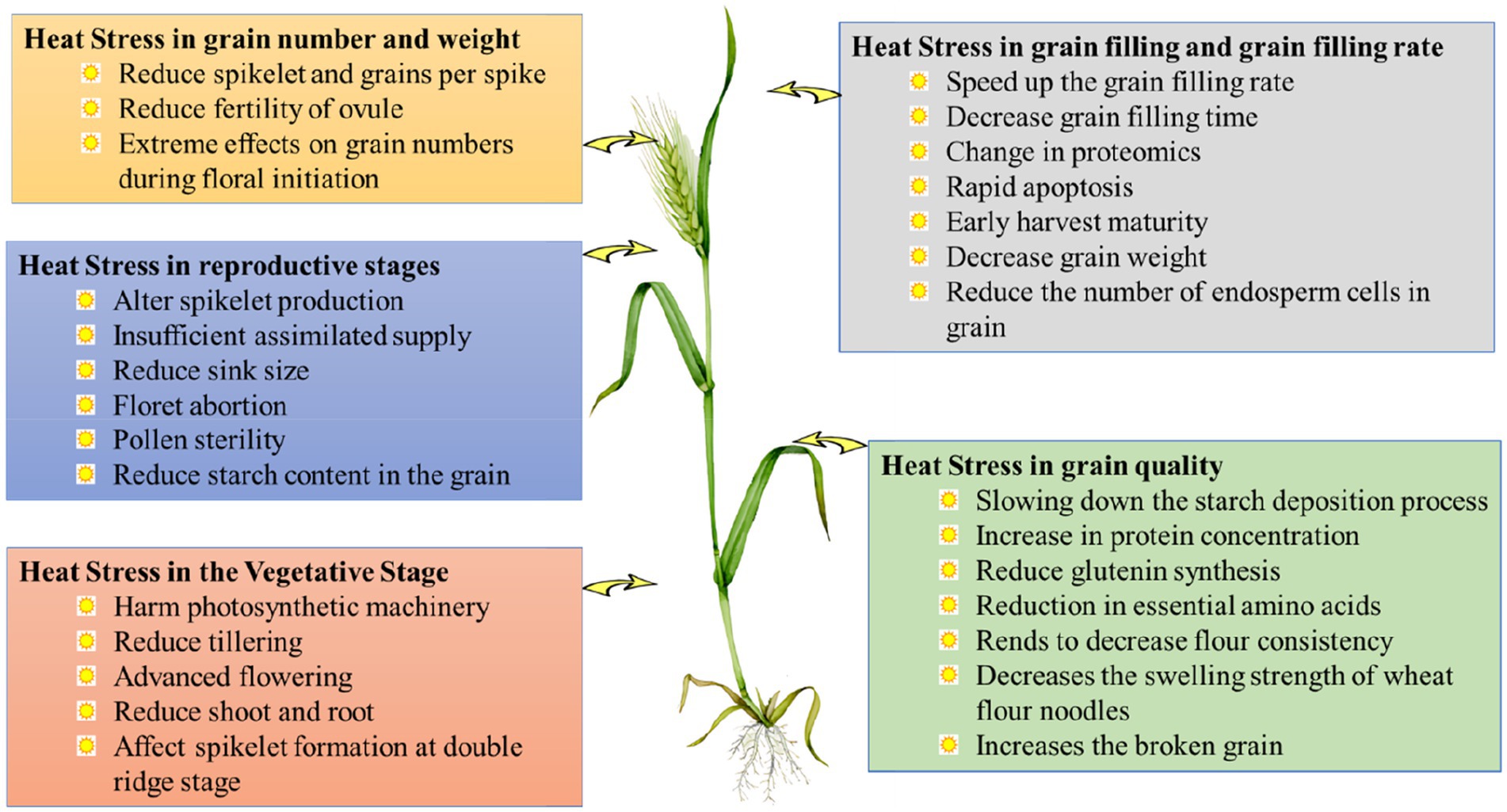
Figure 2. Diagrammatic representation of the impact of heat stress on different major traits of wheat.
2.1. Heat stress in the vegetative stage
The wheat plants’ periodic life cycle events are highly responsive to heat shock. Poor stand establishment results from high soil temperatures, which inhibit healthy wheat germination. High temperature affects vegetative growth and accumulation of shooting biomass. High-temperature harm photosynthetic machinery which shortens the vegetative period increases oxidative stress, reduces tillering, and advances flowering. High temperatures have a direct influence on the photosynthetic apparatus of wheat and other cereal crops, decreasing photosynthesis, photoassimilate generation, and shoot biomass production throughout the vegetative process (Wahid et al., 2007). The extent of damage will be determined by the developmental phase at which wheat plants are subjected to temperature extremes. After 7 days post germination, it has been demonstrated that heat treatment at 45°C for 2 hours may diminish the length of the shoots and roots, as well as the dry mass, chlorophyll content, and the membrane stability index (Gupta et al., 2013). For 24 h, heat treatment at 42°C prevented the growth of roots and the first leaves; it also caused a rise in reactive oxygen species and lipid peroxidation products in the development of coleoptile and seedling stage organs (Savicka and Škute, 2010). After the double ridge stage, heat stress had a more harmful influence on spikelet production than it had before (Frank and Bauer, 1997).
2.2. Heat stress in reproductive stages
Because of its direct effect, heat stress during the process of reproduction is more detrimental to a number of grains and dry matter production than heat stress during any other phase (Wollenweber et al., 2003). Several more studies have demonstrated that wheat is also likely to experience an increase in ‘terminal’ or end-of-season heat stress (Mitra and Bhatia, 2008; Semenov, 2009). The reproductive phases and growth of crops are considered the most vulnerable stages in cereals to high-temperature stress, which alters spikelet production and grain development due to insufficient assimilated supply, resulting in fewer spikelet/grains and reduced sink size (Barnabás et al., 2008; Shi et al., 2022). The effect of heat stress that eventually induces floret abortion at anthesis results in pollen sterility attributable to tissue dehydration, decreased CO2 assimilation and enhanced photorespiration (Wardlaw and Wrigley, 1994). For three consecutive days, when pollen mother cells divide, if wheat plants are exposed to 30°C, it significantly decreases grain set and grain yield (Saini and Aspinall, 1982). On the other hand, stigma and ovule development is accelerated by high temperatures reducing the duration of pollen and pollen tube development (Hedhly et al., 2009). For grain development, the optimal temperature varies between 12°C and 22°C (Farooq et al., 2011) and ample sterility is caused by temperatures above 30°C during floret formation (Saini et al., 1983). Photosynthesis boosts the supply’s rising temperature. Still, it may not fully compensate for starch deposition by the shortened duration, which leads to smaller grains and restricts yield (Andrew, 1987). Therefore, when temperatures escalate between anthesis and grain maturity, due to the reduced time to collect resources, grain yield is reduced. Day and night temperature variations between 28 and 37°C for about 10 to 20 days have been linked to having adverse effects. The filling and maturation times of grains are shortened, and their fresh weight, dry mass, protein, and starch content, and their size are all diminished, among other unfavorable outcomes. Ultimately, this leads to a reduction in yield (Hurkman et al., 2009).
2.3. Heat stress in grain number and weight
The number of grains is a major component of wheat yields. Temperature increases have been shown to hasten spike development while decreasing the number of spikelets and seeds formed per spike (Evans, 1978). In many places, heat stress is a substantial threat to wheat productivity when temperatures rise, particularly during the reproductive and grain-filling stages. Dry matter build-up and grain production are both negatively impacted by heat stress because of the negative effects on photosynthesis caused by metabolic constraints and oxidative damage to chloroplasts. When the temperature rises above 31°C just before anthesis, it leads to pollen sterility, which means that the pollen is unable to fertilize the ovules and produce viable seeds causing several pre-anthesis disruptions and subsequently affecting yield and yield components (Roychowdhury et al., 2023). This leads to a reduction in the total amount of grains produced since there are now fewer seeds being created. Following this, a lower total grain yield is achieved as a result of the decreased grain number (Fokar et al., 1998a). Elevated temperatures may have a substantial influence on wheat grain production, making it important for breeders to develop heat-tolerant varieties that can withstand such conditions. By reducing the sensitivity of the pollen to high temperatures, breeders can improve the grain yield of wheat and help ensure a stable food supply for the growing global population. Heat stress has extreme effects on grain numbers during floral initiation. For example, in the 30 days before anthesis, the number of grains per spike decreased by 4% for every 1°C (from 15 to 22°C) rise (Wheeler et al., 1996). At mid-anthesis, an increase in temperature of about 10°C induced a 40% decrease in grain number per spike. After being exposed to high temperatures of 30°C during the day and 20°C at night for 3 days, or 30°C for a single day, the number of grains produced by wheat plants was shown to decline. This decreased grain set was attributed to the high temperatures that were experienced (Saini and Aspinall, 1982).
2.4. Heat stress and grain development efficiency
Typically, extreme heat accelerates the grain-filling rate and shortens the grain-filling duration (Dias and Lidon, 2009). Increased temperature may accelerate phenological development, decrease grain filling time, change in proteomics, more rapid apoptosis, and achieve earlier harvest maturity and thus produce shriveled grain (Altenbach et al., 2003; Majoul-Haddad et al., 2013).
A study found that stress during the early phases of grain filling reduced the number of endosperm cells in the grain, leading to a decrease in grain weight (Nicolas et al., 1985). Endosperm cells play a critical role in grain filling as they provide the nutrients and energy necessary for the grain to grow and mature. When heat stress occurs during the early phases of grain filling, it can disrupt the normal development of the endosperm cells, leading to a decrease in their number. This reduction in the number of endosperm cells results in a reduction in grain weight as there are fewer cells available to contribute to grain growth. The research findings suggest that heat stress during the early phases of grain filling can have a significant impact on the weight and overall yield of wheat grains. This emphasizes the need of reducing heat stress to safeguard wheat quality and output. Starch synthesis, especially in the latter phases of grain filling, might be inhibited by insufficient grain assimilation resources (Blum, 1998). Compared to the daytime temperature, the night time temperature increase is more sensitive, has a negative effect on grain production by reducing filling time and crop yields. Temperatures between 20 and 23°C at night have delayed the grain filling period by 3 to 7 days (Prasad et al., 2008). Another study discovered a significant slowing of wheat grain filling when day/night temperature changes ranged from 32 to 22°C, as compared to 25 to 15°C (Song et al., 2015). During the grain-filling season, estimated grain yield losses in South Asia range from 6 to 10% per °C increase in temperature (Mondal et al., 2013). In the grain-filling stage, a day-night temperature swing of 34/26°C for 16 days raises leaf temperature, lowers leaf chlorophyll and photosystem-II maximum quantum production, and decreases the total grain output and the weight of individual grains (Pradhan and Prasad, 2015). High-temperature tension during post-anthesis causes leaf senescence. Research findings showed that during the grain-filling stage, a daily mean temperature of up to 30°C had a positive effect on the strength of the dough. However, when the temperature exceeded 30°C, the strength of the dough began to decline. In other words, there was a relationship between the daily mean temperature and the strength of the dough, with an optimal temperature range for maintaining strong dough (Irmak et al., 2008). Adapting some techniques, such as advancing the sowing date, adding additional nitrogen dose and irrigating at the grain filling point, help minimize the effect of heat stress by 7.5, 6.4, and 9%, respectively, in current heat-stressed scenarios in 2020 and 2050 (Dubey et al., 2020). In high temperatures, grain filling happens more quickly but for a shorter period of time. Research findings suggest that while elevated temperatures can cause a reduction in the grain growth rate, the number of grains is less affected. When the temperature exceeded 30°C, the grain filling rate did not increase enough to compensate for the shorter grain filling time, resulting in a decrease in total grain weight. This highlights the complex effects of elevated temperatures on grain filling and the limitations of this compensation mechanism in mitigating the negative impact of heat stress on wheat grain production (Sofield et al., 1977). According to the findings of other investigations, quick grain filling rates have not been able to compensate for the length of time grains take to fill when subjected to heat stress (Stone and Nicolas, 1995). Besides, length and grain growth rate are decreased by heat stress in genotypes (Viswanathan and Khanna-Chopra, 2001). Under ideal temperature circumstances, the decrease in grain filling time is counterbalanced by an increase in the grain filling rate. However, under high-temperature stress, compensatory correction does not take place, leading to a significant drop in grain weight. The essential explanations for reduced grain filling at high-temperatures are reduced leaf and spike photosynthesis and declined stem reserves remobilization One effective strategy for improving heat tolerance is to increase the rate at which grains fill and mature under high-temperature stress (Dias and Lidon, 2009). An improved grain filling rate can be achieved by optimizing the photosynthetic activity of the plant, which provides the energy required for grain growth and development. In addition, an improved grain filling rate can be achieved by optimizing the water relations in the grain, which is crucial for maintaining grain size and weight. Growing wheat with a higher grain filling rate is an important step toward making the crop more resilient to rising temperatures and assuring a steady supply of food in the face of a changing environment. It is conceivable to increase food security for millions of people throughout the world by employing this function to maximize grain filling in wheat types that are more resistant to high temperatures and yield more grain.
2.5. Heat stress in grain quality
The percentage of protein found in the grain and the average size of individual grains are the two most essential factors that determine the quality of wheat grain (Coles et al., 1997). Since it slows down the process of starch deposition, heat stress during grain filling can have a detrimental influence on the quality of the grain’s protein (Gooding et al., 2003). This statement highlights the adverse effect of high temperatures on the grain-filling stage and its impact on grain protein. The heat stress affects the grain’s ability to properly deposit starch, thereby altering the quality of the grain protein. Heat stress disrupts the balance of nitrogen and starch in wheat grains, leading to an increase in protein concentration. As the heat stress causes the grain to allocate more nitrogen toward the formation of protein, the resulting grains have a higher concentration of protein compared to those grown under normal temperature conditions (Stone and Nicolas, 1995). Heat stress applied during the initial stages of grain filling has been shown to result in a substantial increase in the protein content of wheat grains (Castro et al., 2007). Exposure to heat stress, however, reduces glutenin synthesis, while gliadin synthesis remains stable or increases (Majoul-Haddad et al., 2013). Heat stress can lead to a shift in the grain’s composition, causing an increase in protein levels but a reduction in essential amino acids. This change can also impact the sedimentation index, a measure of the grain’s protein quality (Dias et al., 2008). By reducing gluten strength-related parameters of lactic acid retention ability and mixograph peak time, heat tends to decrease flour consistency (Li Y. F. et al., 2013). During grain filling, high temperatures (>35°C) adversely affect the dough properties of wheat (Blumenthal et al., 1993). High-temperature stress also decreases the swelling strength of wheat flour noodles and increases the broken grain (Stone and Nicolas, 1996). A drop in starch content, which accounts for more than 65% of the dry weight of cereals, contributes to significant losses in yield (Rakszegi et al., 2006; Barnabás et al., 2008). Protein composition changes in a situation with a daily maximum temperature of more than 32°C, resulting in shriveled grains involving a higher proportion of bran and reducing the quality of wheat (Naeem et al., 2012).
3. Heat stress response and adaptation in wheat
Excessive heat can lead to cellular damage and cell death in plants, ultimately resulting in the breakdown of the plant’s structure and organization. Under intense heat stress, the cells of the plant become damaged and may die, leading to disruptions in the plant’s normal functioning. This can result in a loss of stability and order, causing the plant’s organization to deteriorate. The ultimate impact of this cellular damage and cell death is a reduction in the plant’s overall health and ability to produce a healthy crop. From the seedling stage to the maturing of the grain, heat stress can have an impact on production. However, it is most likely to reduce yields during the reproductive and maturing phases. Wheat can cope with heat stress either by escape, avoidance, or tolerance mechanism. In the escape process, the plant attempts to stop heat stress by completing its life cycle before stress pressure arrives. The avoidance mechanism is responsible for controlling the plant’s water status and limiting the amount of water lost through stomatal closure, stem and leaf waxiness, leaf rolling, changes in leaf orientation, leaf hairs, and the senescence of older leaves. The tolerance mechanism includes canopy cooling survival under low water potential, better photosynthesis to preserve the supply of assimilates, better efficiency of radiation usage, and extended time of grain filling at higher temperatures. Wheat responses are manifested at morphological, physiological, and biochemical levels and its adaptation to heat stress depends on how efficiently it responds (Akter and Rafiqul Islam, 2017). These responses are brought by a series of signaling cascades involving various transcription factors and signaling molecules which ultimately affect the transcriptome, proteome, and metabolome of the cell.
3.1. Morphological/growth responses
The number of seeds that germinate and the strength of the seedlings are both reduced when plants are subjected to heat stress (Hossain and Teixeira da Silva, 2012). The decreased efficiency of the endosperm is to blame for the lower rates of germination that occur when temperatures are extremely high. This is because a larger amount of carbon is used for respiration (Blum and Sinmena, 1994). Early maturing wheat genotypes avoid the severe effects of terminal heat stress. These genotypes exhibit rapid ground cover and accelerated grain-filling responses to minimize the impact of heat stress (Sharma et al., 2019). Wax deposition on the stem and leaf surface reduces plant dehydration by limiting the absorption of solar radiation. Leaf rolling provides heat tolerance as a beneficial trait by preserving the operation of photosystems I and II and efficient stomatal transpiration control (Sarieva et al., 2010). The number of productive tillers per plant and green leaf area substantially reduces due to heat stress (day/night temperature 30/25°C; Rahman et al., 2009). Plants accumulate lesser biomass under warm environments compared to optimum or cooler environments. There is a strong relationship between agronomic characteristics and yield under conditions of heat stress. These characteristics include but are not limited to, grains per spike, spikelet fertility, early ground cover, canopy temperature, thousand-grain weight, days until heading, days until maturity, and grain filling duration.
3.2. Physiological responses
Heat stress occurs when temperatures rise beyond the tolerance level of the plant, causing disruptions in its physiological processes and reducing the quality and quantity of grain produced (Hasanuzzaman et al., 2013). The physiological responses of plants to heat stress are complex and involve various physiological and biochemical pathways. Understanding these responses is critical for developing heat-tolerant wheat varieties and improving crop yields in regions prone to elevated temperatures. The following section will provide an overview of the physiological responses of heat stress in wheat and the mechanisms that plants use to cope with heat stress.
3.2.1. Photosynthesis in wheat or C4 crops
Plants rely on photosynthesis to flourish, however, this process can be inhibited by high temperatures. Heat stress during the vegetative and reproductive stages of wheat causes a sharp decline in the photosynthetic rate. Heat stress poses a risk to the thylakoids, contributing to chlorophyll degradation (Ristic et al., 2009). The electron transport activity of chloroplasts is substantially reduced during heat stress, followed by increased lipid peroxidation in thylakoids (Mishra and Singhal, 1992). Chlorophyll content can therefore be used as an index for assessing the heat tolerance of wheat. Stroma and thylakoid lamellae of the chloroplast are the sites where a heat-induced injury occurs affecting the carbon metabolism and photochemical reactions. When elevated temperatures suppress the current process of photosynthesis, transferring energy stored in the stem from previous photosynthesis to grain production can greatly improve grain filling (Blum, 1988). This stem reserve mobilization helps to keep the grain-filling process going even when current photosynthesis is hindered by heat stress. In simpler terms, utilizing the energy stored in the stem before flowering helps to maintain grain growth even when heat stress is reducing the plant’s ability to produce energy through photosynthesis. Elevated temperatures can take a toll on the chloroplast enzymes, rendering them ineffective and causing a drop in leaf photosynthesis. The cause of this inactivation is due to oxidative stress, which arises when temperatures rise and disrupt the delicate balance of the chloroplast system. This oxidative stress damages the chloroplast enzymes, making it difficult for them to function properly. As a result, the process of photosynthesis in the leaves slows down, leading to a decrease in overall photosynthesis. This reduction in photosynthesis can have a significant impact on the health and growth of the plant, ultimately reducing its yield and quality. The activity of Rubisco, the most abundant enzyme in the plant system and a key enzyme involved in photosynthesis, is reduced at high temperatures. In cool-season crops such as wheat, Photosystem-II is more susceptible to heat stress, while photosystem-I is relatively stable (Marutani et al., 2012; Mathur et al., 2014). At temperatures >40°C chlorophyll ‘a/b’ proteins of the ‘light-harvesting complex-II’ get dissociated from the photosystem-II (Iwai et al., 2006). The thylakoid membrane damage at high temperatures leads to the termination of photophosphorylation (Dias and Lidon, 2010). Wheat production under heat stress can be improved by selecting plants with a high normalized difference vegetation index (NDVI), an indirect indicator of chlorophyll content and biomass build-up (Liu C. et al., 2019). Leaf senescence is a distinctive heat injury symptom that occurs due to chlorophyll biosynthesis inhibition accompanied by vascular breakdown, membrane integrity loss and cellular homeostasis disturbance (Khanna-Chopra, 2012).
3.2.2. Respiration effect on heat stress
The effect on respiration of heat stress is not well known. As temperatures rise, the cost of respiration in plants also increases, eventually reaching a point where the rate of photosynthesis is unable to keep up with the energy demand (Levitt, 1980). At this point, the respiratory losses become too high, and the plant is unable to compensate, leading to a reduction in growth and overall health. In simpler terms, as temperatures increase, plants have to work harder to maintain their functions, and if this cost becomes too high, it will negatively affect their growth. This can be compared to a person who has to work harder to maintain their health in hot weather, and if this becomes too demanding, they will eventually tire and become exhausted. High-temperature stress induces mitochondrial production of oxygen radicals, and these organelles respond to stress tolerance by producing separate anti-stress proteins (Davidson and Schiestl, 2001). The combined effect of drought and high temperatures changed the mitochondrial ultrastructure of susceptible wheat cultivars in the leaves (Grigorova et al., 2012). In conditions of heat stress (daytime temperatures of 35°C and night-time temperatures of 25°C), the respiration rate in the flag leaf of wheat is much higher in susceptible varieties than it is in resistant types (Almeselmani et al., 2009).
3.2.3. Water status
When plants are subjected to high temperatures, the heat causes the tissues of the plants to lose moisture, which ultimately results in the plants being dehydrated. Because of this, the plant’s growth and development may be stunted, which will prevent it from realizing its full potential. Just like how we need to stay hydrated to perform our best, plants also rely on having enough water to thrive. When the heat causes the plant’s tissues to become dehydrated, it negatively impacts the plant’s overall health and growth. To retain maximum water potential during flowering, an upper limit of 31°C is required (Atkinson and Urwin, 2012). Water capacity and relative water content in leaves decrease with increasing leaf temperatures due to heat stress, which ultimately decreases photosynthetic ability (Farooq et al., 2011). Transpiration rate decreases during heat stress thus affecting plant growth. At high temperatures, there is an increase in the aquaporin activity, membrane fluidity, and membrane permeability, as well as a reduction in the water’s viscosity. This leads to an increase in the cell membrane’s hydraulic conductivity (Cochard et al., 2007; Martínez-Ballesta et al., 2009).
3.2.4. Stomatal conductance
When the temperature difference between the interior and exterior of a plant is higher, the plant is better able to control its temperature by allowing water to evaporate via its stomata. Evaporative cooling is the process that helps to reduce the temperature within the plant’s canopy, which in turn serves to protect the plant from the negative effects of heat stress. When the temperature is high everywhere around it, the plant cools itself down by exhaling water vapor via its stomata. This effectively lowers the temperature inside the plant’s canopy. The phenomenon is known as “Canopy Temperature Depression (CTD)” describes this drop in temperature. By utilizing evaporative cooling through stomatal conductance, plants are better equipped to cope with high temperatures and maintain optimal growing conditions. A strong association exists between CTD and wheat stomatal conductance in hot environments (Amani et al., 1996; Reynolds et al., 1998). Vapor pressure deficit between the canopy and the atmosphere as well as chemical signals generated in dehydrating roots regulate stomatal opening/closure, thus maintaining the water potential of the plant (Pirasteh-Anosheh et al., 2016). As a useful selection criterion for wheat yield under heat stress, stomatal conductance has been proposed and has a high heritability (Rebetzke et al., 2003; Condon et al., 2004).
3.3. Biochemical responses
Elevated temperatures can disrupt the normal functioning of biochemical processes, leading to significant reductions in crop yield. To cope with heat stress, plants have evolved complex mechanisms to regulate their biochemical responses and maintain metabolic homeostasis. Understanding these biochemical responses is crucial for the development of heat-tolerant crops and sustainable agriculture. In this context, it is important to examine the changes in the biochemical processes of wheat in response to heat stress, as well as the mechanisms involved in the regulation of these processes. When exposed to elevated temperatures, the normal metabolic activities of the plant can be disrupted, leading to a range of physiological responses. Some of the key biochemical responses of heat stress in wheat plants include:
3.3.1. Enzyme activities
The major photosynthetic enzyme Rubisco is heat sensitive and its activity declines sharply under high-temperature stress. Rubisco-activase which drives the activity of Rubisco also gets diminished under heat stress and this leads to a drop in carbon fixation. Additionally, at higher temperatures, the solubility of CO2 is substantially reduced in comparison to that of O2, which favors the oxygenation activity of Rubisco, ultimately leading to an increase in photorespiration and a reduction in the rate of photosynthesis (Lea and Leegood, 1999). Under conditions of extreme temperature stress, the endogenous levels of the enzyme rubisco-activase can be a determining factor in wheat yield (Ristic et al., 2009). When a conserved sequence that provides thermal stability to the enzyme in a heat-adapted species was mutated in wheat, the thermostability of wheat rubisco-activase was enhanced (Scafaro et al., 2019). Starch synthase is an important enzyme that plays a role in both the manufacture of endosperm starch and the filling of grains (SS). The activity of SS decreases at temperatures above 25°C which leads to impairment of starch accumulation eventually affecting grain filling (Keeling et al., 1993). SS is regulated at the transcriptional level during heat stress, where the level of transcripts for the enzyme decreases rapidly relative to other enzymes for starch biosynthesis (Hawker and Jenner, 1993). Temperatures that are higher than this range have a detrimental impact on the activity of soluble starch synthase (SSS), which ultimately leads to less starch being accumulated. The optimal temperature range for SSS is 20–25°C (Prakash et al., 2004). This impact can be reversed when the heat stress lasts for just a short amount of time; however, when the heat stress lasts for a longer period of time, there is a suppression of the enzyme activity that is almost impossible to reverse (Sumesh et al., 2008).
3.3.2. Membrane stability
The thermostability of the membrane is a valuable feature for selecting genotypes of heat-tolerant wheat (Blum et al., 2001). The genetic variability and heritability for membrane stability in wheat are high. (Shanahan et al., 1990; Fokar et al., 1998b). Heat stress affects the plasma membrane and leads to the leakage of solutes. The level of heat damage is dependent on the degree of unsaturation of the lipids in the membrane. Plants that can preserve their high-temperature membrane functionality can better withstand heat stress (Blum, 1988). A set of diverse wheat lines for cell membrane stability (CTS) was evaluated at heat stress conditions and concluded that CTS can efficiently discriminate heat-tolerant and susceptible lines and displayed a significant correlation with yield (Islam et al., 2017). Increased membrane stability index at elevated temperatures was observed in genotypes of heat-tolerant wheat due to an increase in antioxidant enzyme activity (Sairam et al., 1997).
3.3.3. Reactive oxygen species
Plants exposed to high-temperature stress secrete substantial amounts of reactive oxygen (ROS) species including superoxide radical (1O2−), hydrogen peroxide (H2O2), hydroxyl ion (OH-) and singlet oxygen (1O2) triggering oxidative stress. The major organelles that generate ROS during stress include chloroplast, mitochondria, and peroxisomes. Heat injury damages the cell membrane and protein which causes the liberation of ROS (Farooq et al., 2011). Increased ROS production results in the depolarization of cell membrane, lipid peroxidation, inactivation of many enzymes and programmed cell death (Blokhina, 2000; Mittler et al., 2011). Long-term (24 h) temperature that is really high (42°C) wheat seedlings exposed to stress showed elevated levels of superoxide ion and malondialdehyde content in their leaf tissue (Savicka and Škute, 2010).
3.3.4. Antioxidants
The antioxidant defense mechanism is accountable for maintaining the balance of reactive oxygen species (ROS) production and detoxification in plants. When exposed to stressors from the environment, these antioxidants scavenge excess ROS and guard against cellular damage. The antioxidant system in plants comprises enzymatic and non-enzymatic components (Caverzan et al., 2016). Enzyme components include superoxide dismutase (SOD), catalase (CAT), glutathione peroxidase (GPX), guaiacol peroxidase (POX), peroxiredoxin (Prxs), ascorbate-glutathione (AsAGSH) cycle enzymes such as ascorbate peroxidase (APX), monodehydroascorbate reductase (MDHAR), dehydroascorbate reductase (DHAR), and glutathione reductase (DHAR) (GR). Ascorbate (AsA), glutathione (GSH), tocopherol, carotenoids, and phenolic substances are nonenzymatic components that play a crucial role in cellular redox buffering. The conversion of ROS to O2 and water is responsible for superoxide dismutase, catalase and peroxidase systems. Superoxide dismutase is involved in the superoxide anion scavenging process (O2-). The scavenging of hydrogen peroxide (H2O2) is carried out by catalase and peroxidase. Antioxidant enzyme levels (catalase, guaiacol peroxidase and superoxide dismutase) increased significantly when wheat seedlings were exposed to short-term heat stress (45°C for 2 h) and were highly correlated with other heat tolerance traits (Gupta et al., 2013). Ascorbic acid is an antioxidant molecule that is water-soluble and plays a role in H2O2 enzymatic detoxification. Pre-anthesis treatment of the 400 mM ascorbic acid wheat cultivar increased heat resistance by controlling the activity of the APX and CATT antioxidant enzymes (Kumar et al., 2014).
3.3.5. Heat shock proteins
Heat shock proteins (HSPs) are a distinct class of proteins that are produced during high-temperature stress by plants. There are two types of HSPs: HSPs with high molecular weight (MW: 68 to 110kD) and HSPs with low molecular weight (MW) (MW: 15 to 27 kD). In all species, high molecular weight HSPs are contained, whereas low molecular weight HSPs are distributed abundantly in higher plants. Five big plant HSP families exist in the system viz., HSP100, HSP90, HSP70, HSP60, and minor HSPs (Gupta et al., 2010). The majority of plant-made HSPs are caused by heat stress and are referred to as molecular chaperones. By binding to them these proteins stabilize partially unfolded or denatured proteins and protect them from thermal aggregation (Vierling, 1991; Feder and Hofmann, 1999). After the stress is relieved, these proteins assist in re-folding of the folded proteins to recover them from stress. Some HSPs also participate in degrading harmful proteins generated from misfolding, denaturation or aggregation and aid in regulating cellular homeostasis (Wang et al., 2004). heat-tolerant wheat cultivars had high levels of HSP100 in the developing grains compared to heat susceptible cultivars under heat stress treatment (Sumesh et al., 2008).
Transgenic wheat encoding the elongation factor (Ef-Tu) of maize plastidial protein synthesis improved heat tolerance in wheat by decreasing thermal aggregation of leaf proteins, reducing heat damage to thylakoid membranes and raising the rate of CO2 fixation (Fu et al., 2008). Heat shock transcription factor TaHsfA6f over-expression in wheat up-regulated multiple HSPs and other heat stress defence proteins such as Golgi anti-apoptotic protein (GAAP) and the broad Rubisco-activase isoform (Xue et al., 2015). Under high-temperature conditions (37°C and 42°C), the TaHsp90 gene was expressed 7.6 times higher in the heat and drought-tolerant Indian wheat cultivar C−306 using subtractive hybridization suppression (SSH; Vishwakarma et al., 2018). HSP70 gene expression level varies temporally and spatially in heat-tolerant and heat-prone wheat cultivars at various stages of growth under heat stress (Kumar et al., 2016). The expression of HSP70 was found to be always higher in the tolerant cultivar compared to the susceptible cultivar at all the observed stages. HSP70 expression was also positively correlated with total antioxidant capacity and negatively correlated with cell membrane stability. In wheat genotypes, an SNP heat shock protein (HSP16.9) was found to contribute a 29.89% phenotypic difference in grain weight per spike between a heat-resistant and heat-susceptible protein (Garg et al., 2012). There are now 753 HSP genes known to exist in the wheat genome (Figure 3), including 169 TaSHSP, 273 TaHSP40, 95 TaHSP60, 114 TaHSP70, 18 TaHSP90, and 84 TaHSP100 (Kumar et al., 2020).
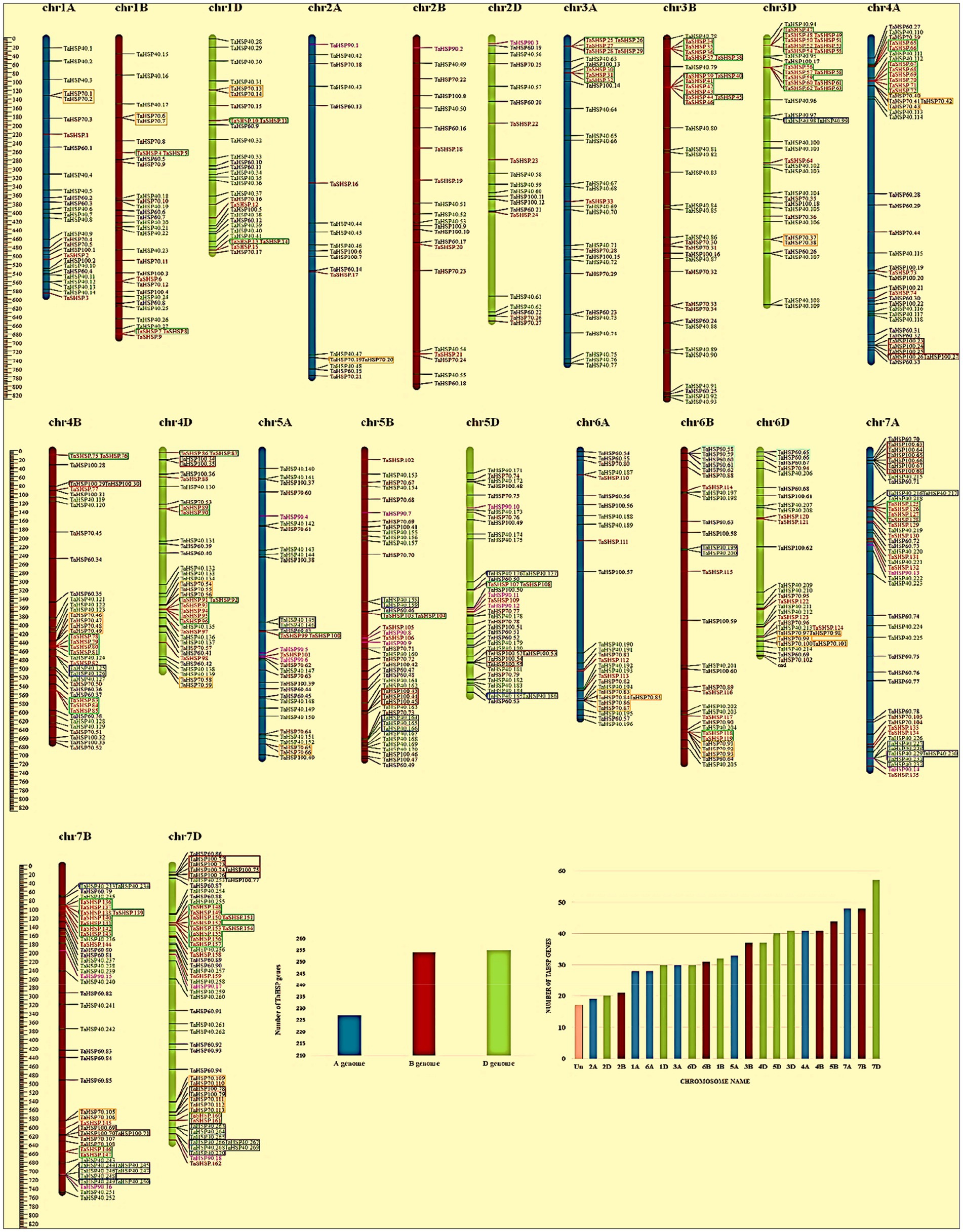
Figure 3. Wheat’s 21 chromosomes and three subgenomes HSP gene distribution. Boxed genes are tandem duplications. (A) Physical map of chromosomal distribution with gene names on the right and location on the left. HSP gene distribution on 21 chromosomes. (C) HSP gene distribution in three sub-genomes. Retrieved and modified from Kumar et al. (2020) with permission.
3.4. Heat stress on wheat disease
The future of world wheat production is threatened by a number of biotic constraints, one of the most important being disease. One research found that plant diseases were responsible for around 20% of the world’s total yield loss in major crops (Thind, 2012). The estimated actual yield losses of wheat caused by fungi and bacteria is about 10% and with viruses is 2% (Oerke, 2006). The major biotrophs are Blumeria graminis causing powdery mildew, Puccinia graminis causing stem rust, Puccinia treticina causing leaf rust, and Puccinia striiformis causing stripe rust to wheat production. Necrotrophic pathogens like Pyrenophora tritici-repentis causing tan spot, Mycosphaerella graminicola causing Septoria tritici leaf blotch, Phaeosphaeria nodorum causing Septoria nodorum blotch, Cochliobolus sativus causing spot blotch, and Fusarium graminearum and other Fusarium species causing Fusarium head or ear blight diseases of wheat. Many more fungal pathogens are causing soil-borne root rots of wheat (Duveiller et al., 2007). Common bunt caused by Tilletia caries and Karnal bunt caused by Tilletia indica can be of great importance in some regions to wheat production (Oerke, 2006; Bockus et al., 2010) summarized wheat pathogens including fungi, viruses, and bacteria as obstacles to wheat production.
Heat stress, especially high temperatures, makes the crop more susceptible to pathogen attack and difficult to combat. The development of plant and plant-pathogen interaction is highly influenced by both the rising temperature and the duration of its exposure. In a region relocation of warming climatic conditions due to changing climatic conditions causes several changes including synchronization of pathogen life-cycle stages, over-wintering, changes in polycyclic pathogens in wheat diseases generation number, and geographic distribution with increased risk of pathogen invasion (Chakraborty et al., 2000; Ghini et al., 2012; West et al., 2012) Global warming weakens a plant’s natural defense system because, at above-normal temperatures, decreases the production of a defense hormone called salicylic acid. Crop physiology and canopy architecture are both affected by varying weather conditions (Pangga et al., 2013), as well as disease levels and disease spread. Like above-ground leaf Pathogens, the growth, and prevalence of economically important soil-borne plant pathogens e.g., Alternaria, Fusarium, and Phoma species are also subjected to favor by warming (Delgado-Baquerizo et al., 2020). In addition, insects that feed on plants (Miedaner, 2018) make it easy to get an entry into mycotoxin-producing Fusarium species.
Temperature-sensitive genes in wheat L210 and L217 due to the temperature effect become susceptible to wheat leaf rust (Das et al., 2016). In European wheat stripe rust isolates higher aggressiveness against higher temperatures was reported by Mboup et al. (2012). It was reported by Walter et al. (2016) two isolates of yellow rust PstS1 and PstS2 in Western Australia and the South-Eastern USA were 3 days earlier and produced 370% more spores (area/day) at high temperatures (28/12°C) than the old races. Wheat cultivars having the gene Sr6 are resistant to stem rust growing at 18°C but at 27°C these cultivars become susceptible (Moerschbacher et al., 1989). Fusarium head blight incidence is estimated to increase at anthesis 10–16% by the 2050s and might be the risk of higher grain losses with mycotoxin contamination of grain because of the forecasted increase of temperatures in the United Kingdom, especially in southern England (Madgwick et al., 2011). Blumeria graminis f. sp. tritici, were found more resistant at the booting stage at 25°C until prior to inoculation (Desaint et al., 2021). Severe wheat yield loss in susceptible cultivars ranges up to 100% due to wheat blast disease as the result of the coincidence of growth stage with the warm temperature and wet years (Cruz and Valent, 2017; Singh et al., 2021). In Bangladesh, optimum planted wheat which faces minimum temperature at the heading stage had less blast severity but it was increased with the later sowing dates during the 2017–18 cropping season (BWMRI, 2018).
Resistance breeding to diseases of wheat under global climate change has several challenges. There is an increasing risk of diseases like leaf rust and fusarium head blight, re-emergence of the old pathogen as an example of stem rust including the emergence of a new or still unknown pathogen, and high adaptation of pathogens like P. striiformis, In the future, it is inevitable to adjustments into resistance breeding strategies with stability and durability of disease resistance under heat in long-term disease occurrence. Continuous delivery of appropriate and adapted wheat varieties to farmers to manage climate change-driven changes is unavoidable. A study found that breeding cultivars with better disease resistance are included in trials in nations with warmer temperatures that nevertheless reflect projected conditions in cooler places (Butterworth et al., 2010). Future research should reflect on multi-disease resistance genes (MDRs), i.e., genes or QTLs that confer resistance to multiple diseases. Manipulating the sowing date or alternations of heading and flowering dates have consequences on disease patterns in wheat, particularly for flower-infecting fungi such as Fusarium species (West et al., 2012).
4. Genetics of heat stress
Heat tolerance is a quantitative trait that is governed by the number of QTLs. Genetics under heat stress is being studied for the past three decades. Using Langdon CSLs (chromosome substitution lines), the first mapping investigation for heat tolerance was carried out in 1991. The genes responsible for heat tolerance were located on chromosomes 3A, 3B, 4A, 4B, and 6A, respectively, (Sun and Quick, 1991). Chromosomes 3A, 3B, and 3D were found to be correlated with heat tolerance in a wheat cultivar name Hope (Ruqiang et al., 1996). Increased heat tolerance was achieved in cultivar Hope by using CSLs between Chinese Spring and Hope (Ni et al., 2018). Chromosomes 3A and 3B harbored important genes regulating heat tolerance. To obtain comprehensive information on QTLs associated with heat tolerance in wheat, a thorough search was conducted using online databases, Google Scholar2 and PubMed.3 In addition, we utilized information available in WheatQTLdb.4 The identified QTLs were compiled and listed in Table 1.
Many QTLs regulating heat tolerance have consistently been located on chromosome 3B (Mason et al., 2010). A drought and heat-tolerant QTL, qDHY.3BL was mapped on Chr 3B in a ~ 1 Mbp interval which harbored 22 genes. Developments in quantitative genetics and molecular markers offered potential tools to identify QTLs influencing heat tolerance in wheat (Thomelin et al., 2016). Eight major QTL regions showing association with drought and heat tolerance located on 1B, 2B, 2D, 4A, 4B, 4D, 5A, and 7A chromosomes were recorded in a meta-QTL study, suggesting that fine-mapping would help locate the genes in these regions (Acuña-Galindo et al., 2015). With the availability of the published sequencing data progress in map-based cloning for heat-tolerance can be achieved for cloning major QTLs (Clavijo et al., 2017).
A heat-tolerant major QTL thermo-tolerance 1 (TT1) was located and cloned in Oryza glaberrima (African rice) which degrades the ubiquitinated proteins. The allele from heat-tolerant accession OgTT1 increases heat tolerance by eliminating the cytotoxic and degraded proteins contrary to heat sensitive-cultivar with OsTT1 allele. Overexpression of OgTT1 showed increased heat tolerance in oryza, Arabidopsis and Festuca elata (Li et al., 2015). Over-expression of OsMYB55 in maize increased heat and drought tolerance under stress conditions as indicated by high biomass and lesser leaf damage in the tolerant lines. Transcriptome analysis from RNA sequencing showed a constitutive upregulation of the genes involved in heat stress among the wild-type plants in OsMYB55 transgenic maize. In one of the three QTL regions, the candidate gene encoding HSc70 shows higher yields among the diploid potato population in a hybrid called Phureja-Tuberosumum (Casaretto et al., 2016; Trapero-Mozos et al., 2018). HSc70 expression was greater than others under moderate heat stress conditions, as seen with a greater tolerance to heat stress with enhanced yields among plants grown from stem cuttings.
Heat tolerance results from a combination of different genes that regulate adaptive characteristics such as enhanced photosynthetic activity, cool canopy, stomatal conductivity and improved pubescence, as most of these characteristics have been correlated with grain yield under heat stress conditions (Cossani and Reynolds, 2012). Leaf wax lowers heat uptake and reduces the leaf temperature (Ishag, 1994). Under conditions of limited water availability leaf waxiness showed an association with a cooler canopy (Richards et al., 1986) however, beneath the heat stress situation the influence of leaf wax in lowering the canopy temperature is not known. QTLs for flag leaf wax content were located on 1B, 3D and 5A chromosomes of which 1B and 5A harbored stable loci. Two QTLs for flag leaf waxes (QWax.tam09-1B and QWax.tam09-5A) showed a phenotypic variation of 9 and 12%, respectively, from 121 RIL populations generated from a cross between tolerant genotype ‘Halberd’ and susceptible genotype ‘Karl92’ were found to be located for heat tolerance (Mondal et al., 2015).
Plant survival, growth and reproduction are severely affected by heat, salinity, and other abiotic stress to which the plants respond by expressing stress-responsive genes that are regulated by heat stress transcription factors (HSFs) which in turn regulate the heat shock proteins (Hsps). HSF are TFs that bind to HSE cis components in the gene-promoting region and play a key role in plant tolerance against heat stress. Heat stress transcription factors have highly conserved regions however their notable diversity across plants shows their multiple functions and their incorporation into several stress-signaling and response networks. In Arabidopsis, ectopic expression of oryza HSFA2e and lily HSFA2 results in enhanced heat tolerance. Overexpression of soybean GmHSFA1 improved heat tolerance in soybean due to the activation of GmHsp70, GmHsp22 and GmHsps downstream genes (Zhu et al., 2006). Tomato HSFA1a is a unique and key regulator for acquired heat tolerance and is not replaced by other HSFs. HSFA1 subfamily is considered a key regulator of heat-stress response. However, the role of HSF genes is to be better understood to reduce the negative impact in transgenic plants. In tobacco, over-expression of VpHSF1 increased acquired heat tolerance but decreased basal heat tolerance and osmotic stress tolerance (Peng S. et al., 2013). SlHSFA3 led to an improvement in heat tolerance in Arabidopsis over tomato expression and showed a negative effect on seed germination under salinity stress (Li Z. et al., 2013). Since HSFs and molecular chaperones play a key role in cellular homeostasis, HSF modification can impede homeostasis, leading to pleiotropic and unwanted effects (Cabello et al., 2014). Much progress has been attained in characterizing the HSFAs class however, the biological function needs to be understood for HSFB and HSFC. The regulation factors of HSFS are to be clearly studied. Hence the regulatory mechanisms of these HSF genes are to be understood properly. They can be further integrated with several crop improvement projects through biotechnological methods.
Under conditions of stress, abscisic acid induces stomatal closure and activates stress-responsive genes. Improvements in molecular technologies, such as microarray and transcriptome analysis, led to the discovery of several stress-responsive genes. These genes not only produce enzymes and proteins for cellular defense, but also control signal transduction pathways and gene expression. In the leaves of heat-sensitive and heat-tolerant genotypes, i.e., Chinese Spring and TAM107 respectively, microarray analysis was used to match up gene expression assessment. After short and continued heat treatment, distinct gene expression patterns were established between the genotypes. The genes found included several significant factors responsible for different biological pathways that promoted the need for different wheat genotypes to decipher the genetics behind heat tolerance (Qin et al., 2008).
Genome-wide association study (GWAS) is a potential and proven approach to determine which genes are responsible for responding to heat stress because the process of heat tolerance is so complicated. GWAS has been used in wheat to dissect the complex genetic locus of heat tolerance (Tian et al., 2011). One hundred forty-eight MTAs with a phenotypic variation from 0.25 to 0.36 were identified in a study (El Hassouni et al., 2019). Grain number per spike reported the highest number of MTAs located over 10 loci, and harvest index over six loci. Under heat stress conditions a common region for grain yield, grain number per spike, harvest index and biomass were located on 6BS. A study on a double haploid population in bread wheat found a major QTL on 1A chromosome affecting grain number, grain weight per spike and spike per meter square (Heidari et al., 2011).
5. Strategies to be taken to combat heat stress
The discrepancy in environmental change has an ongoing impact on agriculture and food safety globally. The increasing frequency and intensity of heat stress events pose a major threat to global food security and the sustainability of wheat production. To mitigate the impact of heat stress on wheat, a range of strategies and practices must be implemented. These can include breeding for heat tolerance, proper irrigation and water management, soil management, mulching and shading, crop management practices, agronomic practices, the use of heat-tolerant cultivars, climate-smart agriculture, and research and development. By adopting these strategies, the resilience of wheat to heat stress can be improved, ensuring sustainable food production and food security for a growing global population. The following tactics can be taken as vital for crop adaptation in a heat-stress environment.
5.1. Agronomic and cultural management for adaptation to heat stress
By altering certain agronomic management techniques such as (i) conservation farming to minimize soil moisture loss (ii) using a balanced dose of fertilizers (iii) changing the sowing period and methods and (iv) using exogenous protectants to mitigate the impact of extreme heat, wheat can be better prepared to grow in warmer environments (Singh et al., 2011a). Mulching can be a good option for minimizing water loss owing to heat stress, especially in rainfed areas where water availability is a serious concern. Organic mulches help in maintaining soil moisture, improving plant growth and nitrogen use efficiency (Singh et al., 2011a). In the north-western plains zone of India, planting wheat in the presence of rice stubbles using zero tillage technique helps in conserving water, and soil nutrients, minimizing weed incidence, adapting to terminal heat stress and improves the overall health of the wheat crop (Erenstein and Laxmi, 2008). Delaying the wheat sowing beyond the recommended sowing time can expose the crop to heat stress during post-reproductive stages which ultimately affects the yield and grain quality. Therefore, late sowing of wheat varieties needs to be avoided at any cost. Planting cultivars having early maturity and long grain filling duration can avoid the effects of terminal heat stress (Al-Karaki, 2012).
For plants to thrive in the face of biotic and abiotic challenges, they must receive adequate and well-balanced amounts of fertilizers. A foliar spray of nutrients can help ameliorate the effects of heat stress. Potassium nitrate (KNO3) foliar spray at booting and grain filling stages increased grain yield in wheat by delaying senescence and improving carbohydrate transport and starch synthesis in the sink organs (Singh et al., 2011b). Orthophosphate (KH2PO4) used as a post-anthesis foliar spray reduced heat-induced leaf senescence and increased grain production (Dias and Lidon, 2010). The application of calcium chloride (CaCl2) enhanced the activities of antioxidant enzymes during heat stress in wheat. Calcium plays a role in stomatal regulation and guards chlorophyll against photo-oxidative damage which helps the plant in tackling heat stress (Dias et al., 2009). Supplementation with zinc during high-temperature conditions improves the plant’s responses by maintaining cell membrane stability, stabilizing chlorophyll fluorescence, improving water uptake efficiency and improving evaporative cooling (Peck and McDonald, 2010). An exogenous spray of magnesium in the form of magnesium sulphate (MgSO4.7H2O) during high-temperature stress improved heat tolerance by minimizing oxidative damage (Mengutay et al., 2013).
Recently, there is a rising demand for the use of osmoprotectants, growth regulators and signaling molecules as an exogenous therapy to reduce different abiotic stresses (Upreti and Sharma, 2016; Ahmed et al., 2019). These molecules reduce the effect of heat stress by activating various antioxidants that are responsible for alleviating oxidative damage. In wheat, the role of various growth protectants like arginine, salicylic acid, putrescine, ascorbic acid and α-tocopherol (vitamin E) in improving heat tolerance has been well elucidated. Treatment with salicylic acid increased heat tolerance in wheat by improving proline metabolism, reducing the formation of ethylene and improving photosynthesis under heat stress (Khan et al., 2013). By decreasing lipid peroxidation (malondialdehyde content), increasing proline biosynthesis and upregulating enzymatic antioxidants, melatonin (N-acetyl-5-methoxy tryptamine), a pleiotropic signaling molecule, enhanced heat stress tolerance in wheat (Buttar et al., 2020). By increasing the operation of antioxidant enzymes and proline biosynthesis, the exogenous application of polyamines (spermidine and spermine) reduced the impact of heat stress at the grain-filling stage in the heat-responsive wheat variety (Jing et al., 2020). In addition, there was a reduction in the malondialdehyde, soluble sugar and putrescine content. Plant growth regulators which are successfully utilized in other crops can also be tested for their effectiveness in wheat. The dosage and mode of the application need to be standardized for these growth protectants for their successful commercialization (Ratnakumar et al., 2016). The environmental toxicity assessment of these new-generation molecules must be carried out rigorously to confirm their safety.
5.2. Marker-assisted selection
Genetic divergence is a vital technique of breeding for new cultivars based on genetic resources (Raza et al., 2019). Landraces are a significant resource for genomic studies which contain larger genomic variance and are treasured for stress tolerance as it contains adaptable genetic resources to various climatic stresses (Lobell et al., 2011; Kumar et al., 2023). The urgent need for the hour is a genetic variation of heat tolerance in the case of wheat breeding, research and the use of new variants (Kayastha et al., 2023). High throughput MAS breeding can fast-track plant breeding with high productivity (Zafar et al., 2018). Heat tolerance is a much complex trait, which is governed by genotype and genotype × environment interaction effects (Tayade et al., 2018). In Mexican wheat landraces (>1,200) gathered from places with varying heat-stressed management areas, a group of superior accessions was detected, and a strong connection was observed between the amount of leaf chlorophyll and the thousand-grain weight (Ma et al., 2009). There are already certain heat-tolerant cultivars and lines established in Asia, and these can be utilized to cultivate new heat-resistant cultivars. A testified inherent foundation of germplasms from T. dicoccoides and T. monococcum that can be used to improve heat tolerance in bread wheat along with Aegilops speltoides, Ae. longissima and Ae. searsii because of capricious notches of heat tolerance were detected from them (Yoshida et al., 2002). However, only a small proportion of the recorded genomic variation in heat tolerance has been exploited due to restrictions on traditional breeding. GWAS and other QTL mapping related to heat stress tolerance characteristics can help to develop wheat cultivars that are ideal for high-temperature climate conditions (Paliwal et al., 2012). In general, QTLs perceived in environments known as constitutive QTLs could be used to build heat-tolerant landraces. On the other hand, QTLs detected solitary in explicit environments called adaptive QTLs, could be utilized for specific heat-stressed areas (Vargas et al., 2006; Collins et al., 2008).
5.3. Genome selection for heat tolerance crop improvement
Genomic selection (GS) was initially presented by Lande and Thompson (1990), but it was Meuwissen et al. (2001) that brought it to the public’s attention. Genomic selection is a modern approach to plant breeding that involves the use of DNA markers to predict the breeding values of individuals for different traits. Genomic selection are effective in promoting novel cultivars in many crops (Tayade et al., 2018). This technique has gained considerable attention in wheat breeding because of the complexity of the wheat genome and the need to develop improved varieties that can withstand various biotic and abiotic stresses. The development of high-density SNP arrays has facilitated the application of genomic selection in wheat breeding. The foremost gain of GS compared to MAS having minor effects alleles are also detected and utilized in the marker selection process (Cairns and Prasanna, 2018). GS is a helpful strategy for preparing novel breeding and advancing ground-breaking genomic evaluation marker-based models. Accessibility of high-throughput, lucrative genome-wide and scalable molecular markers suitable for a large population, in addition to or without the reference genome, is essential for the successful application of genomic selection in crops (Bhat et al., 2016). GS can be used as a pre-breeding device to aid in to detect of genomic resources with an advantageous variation for compound traits through predicting the breeding values of a specific population within the breeding population and offers new prospects to upsurge genetic gain of complex traits (Wang X. et al., 2018). GS has been applied in successful breeding programs in wheat (Song et al., 2017; Juliana et al., 2019a). The first use of genomic selection in wheat was reported in 2009 by the International Maize and Wheat Improvement Center (CIMMYT) in Mexico (Sun et al., 2020). Since then, it has been used by many researchers and breeders around the world to improve wheat varieties. They found that the accuracy of genomic selection increased with the size of the training population and the number of markers used. Since then, numerous studies have been conducted to evaluate the performance of genomic selection for different traits in wheat. In the past decade, numerous studies have investigated the potential of genomic selection in wheat, particularly for yield-related traits, disease resistance, and abiotic stress tolerance wheat (Song et al., 2017; Singh et al., 2019, 2022; Juliana et al., 2019a,b; Larkin et al., 2020; Dreisigacker et al., 2021; Mondal et al., 2021; Saini et al., 2022). These studies have shown promising results, indicating that genomic selection can enhance breeding efficiency and accelerate the development of improved wheat varieties. Their results showed that genomic selection could effectively predict wheat improvement. Utilization Genomic Selection for the breeding of wheat to adapt to heat-stressful environments is also employed in several breeding institutes, companies and universities (Mondal et al., 2021). Despite the promising results, several challenges still need to be addressed to fully realize the potential of genomic selection in wheat breeding. One of the major challenges is the lack of diversity in the training population, which can result in biased predictions. To overcome this challenge, efforts are being made to develop diverse training populations that capture the genetic variation in the target population. Another challenge is the high cost of genotyping, which can limit the adoption of genomic selection in small breeding programs. However, advances in genotyping technologies and the development of low-cost SNP arrays are expected to address this challenge in the near future.
5.4. Genome editing in wheat for heat tolerance
Wheat is a complex crop with a large genome, and many genes contribute to heat tolerance. As a reverse genetic tactic for targeting heat tolerance gene activity, genome-editing techniques such as transcription activator-like effector nucleases (TALENs), zinc finger nucleases (ZFNs), and clustered regularly interspaced short palindromic repeat (CRISPR) can also be used in addition to MAS. Compared to other genome-editing techniques, CRISPR-Cas9 has developed a powerful method for precise genome editing to study the pathways associated with heat stress and to increase thermotolerance in cropping systems (Nguyen et al., 2018; Biswal et al., 2019; Sun et al., 2022). The theory of CRISPR involves the design of a ~ 20 nucleotide RNA guide complementary to the gene of interest, while a Cas9 nuclease enzyme that cuts 3–4 bases next to the adjacent protospacer motif is repaired by homology-directed repair pathway or by non-homologous error-prone end-joining (Jiang and Doudna, 2017; Jaganathan et al., 2018). Using in silico and in vivo TILLING methods, four new small Hsp26 (sHsp26) alleles suitable for improving heat tolerance in durum wheat were identified (Comastri et al., 2018). Genome editing methods have unlocked new opportunities to instigate targeted editing of crop genomes involved in heat stress tolerance (Parmar et al., 2017; Haque et al., 2018). For example, stress-induced transcriptional factors from the AP2/ERF superfamily improved abiotic stress tolerance in crops based on CRISPR-Cas9 genome editing of ethylene response factors (ERFs; Debbarma et al., 2019).
Several reports describe the use of Agrobacterium-delivered CRISPR/Cas9 for wheat genome editing, despite the low transformation efficiency of this crop (Howells et al., 2018; Zhang et al., 2018; Okada et al., 2019; Zhang Z. et al., 2019). One of the primary targets for genome editing in wheat for heat tolerance is the Heat Shock Transcription Factor (HSTF) genes. HSTFs are a group of transcription factors that regulate the expression of heat shock proteins, which play a crucial role in protecting the plant from heat stress. By editing HSTF genes, scientists can increase the expression of heat shock proteins, which enhances the plant’s ability to tolerate heat stress. Another target for genome editing in wheat for heat tolerance is the Rubisco activase (RCA) gene. Rubisco is the key enzyme responsible for carbon fixation during photosynthesis. Under heat stress, the activity of Rubisco is reduced, leading to a decline in photosynthetic efficiency. RCA is a chaperone protein that activates Rubisco, and editing RCA genes can increase the expression of RCA and, therefore, enhance Rubisco activity under heat stress. Furthermore, genome editing can also be used to improve the wheat plant’s water use efficiency, which is closely linked to heat tolerance. By editing the genes that regulate stomatal opening and closing, researchers can control the plant’s water loss through transpiration, leading to more efficient use of water under heat-stress conditions. Target genes used in genome editing with their function to improve stress tolerance are presented in Table 2.
6. Global policy in breeding for heat tolerance and future prospects
Global policies on breeding for heat tolerance in wheat have been developed to address the challenges posed by global climate change, which is causing increasingly frequent and severe heat stress events. The followings are some of the current policies and future prospects in breeding for heat tolerance in wheat:
6.1. National and international research programs
National and international research programs have been established to develop heat-tolerant wheat varieties through breeding and genetic engineering. These programs aim to identify and characterize the genetic basis of heat tolerance and to develop and distribute heat-tolerant seed varieties to farmers. There is a growing trend toward international collaboration in wheat breeding, with countries working together to share their knowledge, expertise, and resources. This collaboration allows for the pooling of genetic diversity, which can be used to develop heat-tolerant wheat varieties.
6.2. Research and development
There is a significant emphasis on conducting research aimed at understanding the mechanisms underlying heat tolerance in wheat and developing new breeding strategies to improve the heat tolerance of wheat crops. Investment in biotechnology is being made to develop new technologies for the breeding and genetic engineering of heat-tolerant wheat. This includes the development of new tools for gene editing and the use of molecular markers to track the inheritance of heat-tolerant genes. The integration of new technologies, such as genomic selection, precision breeding, and phenotyping, is playing an increasingly important role in the development of heat-tolerant wheat varieties. These technologies allow for the rapid and efficient evaluation of large numbers of wheat varieties, making it possible to identify those with the highest levels of heat tolerance.
6.3. Adoption of sustainable farming practices
Global policies are promoting the adoption of sustainable farming practices, such as conservation tillage and cover cropping, which can help to mitigate the effects of heat stress on wheat crops. The adoption of sustainable farming practices can help to mitigate the effects of heat stress on crops and improve their resilience to high temperatures.
6.4. Partnerships between the public and private sector
Partnerships between the public and private sectors are being formed to facilitate the development and distribution of heat-tolerant wheat varieties. This collaboration helps to accelerate the pace of research and bring new varieties to market more quickly.
6.5. Funding to mitigate future challenges
There is an increasing amount of funding being allocated to studies aimed at making wheat more resilient to high temperatures. This funding is being provided by governments, non-profit organizations, and private companies, and in doing so, it is facilitating the spread of knowledge and the implementation of novel breeding procedures.
In the future, it is expected that these policies will continue to evolve and expand, as the need for heat-tolerant wheat varieties becomes more pressing. There may also be a greater focus on the development of new technologies and the integration of traditional and modern breeding techniques to achieve the goal of developing heat-tolerant wheat varieties. Additionally, there may be an increased emphasis on the deployment of these heat-tolerant varieties in regions that are most vulnerable to heat stress, such as developing countries in Africa and Asia. Wheat can strengthen food and nutrition security in many parts of the world, including places of the world where rising temperatures pose a threat, such as Sudan and Nigeria, as well as countries in South Asia. The lowest agricultural productivity in the world has been recorded in the African region. Most of the countries in this continent fullfil their rising food demand through overseas food imports. But these countries have the potential to increase their wheat production by improving policies to use their ready winter fellow land to produce wheat. According to research, new kinds of wheat that are both super-early and heat-tolerant have already been tested along the Senegal River basin, and these types are widely available through the CGIAR-WHEAT program (Sall et al., 2019).
An integrated effort is required for the development of climate-resilient agricultural output under extreme climatic circumstances and household farming systems. This includes the development of plant breeding and seed systems as well as production systems. This is achievable through the development of the commodity supply chain as well as the extension and training of farmers to implement heat-tolerant varieties. The use of heat-tolerant genotypes in a breeding program and developing commercial heat-tolerant varieties are being in priority for the last decade. South Asia, comprising Bangladesh, India, Nepal and Pakistan, is the largest region where nearly 40 million ha of wheat is grown by small farmers and it has been predicted that climate change has the highest impact on South Asian Agriculture. Nevertheless, there is no specific policy to work on heat stress tolerance wheat development. To address climate-smart agriculture, the Indian Council of Agricultural Research (ICAR) India initiated a fast-track variety release program and successfully release high yield potential verity namely DBW303 for the early sown condition of NWPZ. Similar tactics have been taken in several NARS-system in South Asia including Bangladesh Wheat and Maize Research Institute (BWMRI) in Bangladesh, Wheat Research Institute (WRI) in Pakistan, Nepal Agricultural Research Council (NARC) in Nepal are working on the heat-tolerant wheat development program. In the collaboration with International Maize and Wheat Improvement Center (CIMMYT) and other international research organizations, the NARS system of these countries is developing their skill and resources to do research combating heat stress.
The most recent directive issued by the government of Sudan about heat-tolerant wheat completely revamped food production in that country. The Technologies for African Agriculture Transformation (TAAT) program increased the use of technologies that have already been proven effective. These technologies included newly introduced varieties that are resistant to heat, drought, and disease; improved irrigation methods; the bed planting method of farming; control of rust (Puccinia graminis); highly automated growing and harvesting as well as compact milling systems. The National Agricultural Research System (NARS), in collaboration with the International Maize and Wheat Improvement Center (CIMMYT) and the International Center for Agricultural Research in Dry Areas (ICARDA), developed and released many high-yielding heat-tolerant wheat varieties to increase Sudan’s overall wheat production. This results in a robust production record of approximately 0.85 million tons of wheat, which is sufficient to meet almost 45% of the country’s demand for wheat. Between the years 2012 and 2016, Sudan only generated 24% of the country’s national wheat requirement, which means that the country is extremely dependent on imports totalling more than 1.5 million tons annually. Recent heat-tolerant varieties, such as Imam, Goumria, Zakia, Elnielain, and Bohaine, have a yield potential ranging from 5 to 8 t/ha (Evergreennews.com, 2019). The fast-track release of variety through national programs and scale-up of recent production technologies like the improved seed of climate-resilient, disease resistant and high-yielding varieties, engineered irrigation, improved agronomy and farm mechanization are the potential area where policy may play a significant role. Global policies and future prospects in breeding for heat tolerance in wheat aim to address the challenges posed by global climate change and ensure that farmers have access to heat-tolerant seed varieties that can help to maintain stable and sustainable food supplies. Overall, the prospects for breeding for heat tolerance in wheat are promising, with a growing commitment from governments, organizations, and the scientific community to address the challenges posed by global climate change.
7. Conclusion
Higher temperatures toward the end of the season, at the time of planting as well as short periods of heat shock, are the constraints of wheat production. Globally temperature is likely to rise with climate change, while fluctuations of the temperature may vary among regions. To prepare for adaptation to climate change, it is essential to segregate the effects of a specific aspect for influences on yield because fluctuations in diverse factors typically need different adaptation tactics. Approaches should specifically focus on the appropriate exploration of germplasm and the exploitation of novel alleles from the wild gene pool, due to the increasing demands for global food supply associated with the scathing pressure of population growth and climate change pathways. In addition, through physiological trait breeding, adaptive as well as morpho-physiological characteristics may be integrated into the high-yielding genotype. Even if multiple genes in wheat have been effectively engineered to enhance heat stress tolerance, their role in different conditions of heat stress and genomic structure is still to be explored. New high throughput phenomics techniques increase hope for difficult-to-measure module characters associated with heat tolerances. Because of all breeding methods, success depends on outstanding phenotypic characterization. When used with system biology techniques, new Omics-based applications could enormously improve conservative breeding to alleviate the tasks of heat stress and modernize the future of sustainable agriculture.
Author contributions
MF, UK, VT, PB, NK, KM, and AH gestated the concept and write-up of the overview. MF compiled and wrote the original draft. MF, AH, VB, and MB revised and edited the original draft. VB and MB received the financial support for the article. All authors carefully read and approved the article for submission to the journal.
Funding
This write-up of the article was completed through the financial support of the Slovak University of Agriculture, Slovak Republic, under the projects “APVV-20-0071.”
Acknowledgments
The authors extend their appreciation to the Slovak University of Agriculture, Slovak Republic, under the projects “APVV-20-0071” for supporting the study.
Conflict of interest
The authors declare that the research was conducted in the absence of any commercial or financial relationships that could be construed as a potential conflict of interest.
Publisher’s note
All claims expressed in this article are solely those of the authors and do not necessarily represent those of their affiliated organizations, or those of the publisher, the editors and the reviewers. Any product that may be evaluated in this article, or claim that may be made by its manufacturer, is not guaranteed or endorsed by the publisher.
Footnotes
1. ^https://www.climate.gov, Accessed on 28 April 2023.
2. ^https://scholar.google.com, Accessed on 27 May 2023.
3. ^https://pubmed.ncbi.nlm.nih.gov, Accessed on 27 May 2023.
4. ^http://www.wheatqtldb.net, Accessed on 27 May 2023.
References
Acuña-Galindo, M. A., Mason, R. E., Subramanian, N. K., and Hays, D. B. (2015). Meta-analysis of wheat QTL regions associated with adaptation to drought and heat stress. Crop Sci. 55, 477–492. doi: 10.2135/cropsci2013.11.0793
Ahmed, N., Zhang, Y., Li, K., Zhou, Y., Zhang, M., and Li, Z. (2019). Exogenous application of glycine betaine improved water use efficiency in winter wheat (Triticum aestivum L.) via modulating photosynthetic efficiency and antioxidative capacity under conventional and limited irrigation conditions. Crop J. 7, 635–650. doi: 10.1016/j.cj.2019.03.004
Akter, N., and Rafiqul Islam, M. (2017). Heat stress effects and management in wheat. A review. Agron. Sustain. Dev. 37:443. doi: 10.1007/s13593-017-0443-9
Al-Karaki, G. N. (2012). Phenological development-yield relationships in durum wheat cultivars under late-season high-temperature stress in a semiarid environment. ISRN Agron. 2012, 1–7. doi: 10.5402/2012/456856
Almeselmani, M., Deshmukh, P. S., and Sairam, R. K. (2009). High temperature stress tolerance in wheat genotypes: role of antioxidant defence enzymes. Acta Agron. Hungarica 57, 1–14. doi: 10.1556/AAgr.57.2009.1.1
Altenbach, S. B., DuPont, F. M., Kothari, K. M., Chan, R., Johnson, E. L., and Lieu, D. (2003). Temperature, water and fertilizer influence the timing of key events during grain development in a US spring wheat. J. Cereal Sci. 37, 9–20. doi: 10.1006/jcrs.2002.0483
Amani, I., Fischer, R. A., and Reynolds, M. P. (1996). Canopy temperature depression association with yield of irrigated spring wheat cultivars in a hot climate. J. Agron. Crop Sci. 176, 119–129. doi: 10.1111/j.1439-037X.1996.tb00454.x
Andrew, S. P. S. (1987). A mathematical model of root exploration and of grain fill with particular reference to winter wheat. Fertil. Res. 11, 267–281. doi: 10.1007/BF01063322
Atkinson, N. J., and Urwin, P. E. (2012). The interaction of plant biotic and abiotic stresses: from genes to the field. J. Exp. Bot. 63, 3523–3544. doi: 10.1093/jxb/ers100
Barnabás, B., Jäger, K., and Fehér, A. (2008). The effect of drought and heat stress on reproductive processes in cereals. Plant Cell Environ. 31, 11–38. doi: 10.1111/j.1365-3040.2007.01727.x
Bhat, J. A., Ali, S., Salgotra, R. K., Mir, Z. A., Dutta, S., Jadon, V., et al. (2016). Genomic selection in the era of next generation sequencing for complex traits in plant breeding. Front. Genet. 7:221. doi: 10.3389/fgene.2016.00221
Bhusal, N., Sarial, A. K., Sharma, P., and Sareen, S. (2017). Mapping QTLs for grain yield components in wheat under heat stress. PLoS One 12:e0189594. doi: 10.1371/journal.pone.0189594
Biswal, A. K., Mangrauthia, S. K., Reddy, M. R., and Yugandhar, P. (2019). CRISPR mediated genome engineering to develop climate smart rice: challenges and opportunities. Semin. Cell Dev. Biol. 96, 100–106. doi: 10.1016/j.semcdb.2019.04.005
Blokhina, O. (2000). Anoxia and oxidative stress: Lipid peroxidation, antioxidant status and mitochondrial functions in plants. Helsinki: University of Helsinki.
Blum, A. (1998). “Improving wheat grain filling under stress by stem reserve mobilisation,” in Euphytica. (Kluwer Academic Publishers), 77–83.
Blum, A., Klueva, N., and Nguyen, H. T. (2001). Wheat cellular thermotolerance is related to yield under heat stress. Euphytica 117, 117–123. doi: 10.1023/A:1004083305905
Blum, A., and Sinmena, B. (1994). Wheat seed endosperm utilization under heat stress and its relation to thermotolerance in the autotrophic plant. F. Crop. Res. 37, 185–191. doi: 10.1016/0378-4290(94)90097-3
Blumenthal, C. S., Barlow, E. W. R., and Wrigley, C. W. (1993). Growth environment and wheat quality: the effect of heat stress on dough properties and gluten proteins. J. Cereal Sci. 18, 3–21. doi: 10.1006/jcrs.1993.1030
Bockus, W. W., Bowden, R. L., Hunger, R. M., Morrill, W. L., Murray, T. D., and Smiley, R. W. (2010). Compendium of wheat diseases and pests. Saint Paul: American Phytopathological Society.
Braun, H. J., Atlin, G., and Payne, T. (2010). “Multi-location testing as a tool to identify plant response to global climate change” in Climate change and crop production. ed. M. P. Reynolds (Wallingford: CABI International), 115–138.
Buttar, Z. A., Wu, S. N., Arnao, M. B., Wang, C., Ullah, I., and Wang, C. (2020). Melatonin suppressed the heat stress-induced damage in wheat seedlings by modulating the antioxidant machinery. Plan. Theory 9, 1–17. doi: 10.3390/plants9070809
Butterworth, M. H., Semenov, M. A., Barnes, A., Moran, D., West, J. S., and Fitt, B. D. L. (2010). North–south divide: contrasting impacts of climate change on crop yields in Scotland and England. J. R. Soc. Interface 7, 123–130. doi: 10.1088/1755-1307/6/37/372051
BWMRI (2018). Annual research report. Nashipur, Dinajpur, Bangladesh Available at: www.bwmri.gov.bd
Cabello, J. V., Lodeyro, A. F., and Zurbriggen, M. D. (2014). Novel perspectives for the engineering of abiotic stress tolerance in plants. Curr. Opin. Biotechnol. 26, 62–70. doi: 10.1016/j.copbio.2013.09.011
Cairns, J. E., and Prasanna, B. M. (2018). Developing and deploying climate-resilient maize varieties in the developing world. Curr. Opin. Plant Biol. 45, 226–230. doi: 10.1016/j.pbi.2018.05.004
Casaretto, J. A., El-kereamy, A., Zeng, B., Stiegelmeyer, S. M., Chen, X., Bi, Y. M., et al. (2016). Expression of OsMYB55 in maize activates stress-responsive genes and enhances heat and drought tolerance. BMC Genomics 17, 312–315. doi: 10.1186/s12864-016-2659-5
Castro, M., Peterson, C. J., Rizza, M. D., Di Dellavalle, P., Vázquez, D., IbáÑez, V., et al. (2007). “Influence of heat stress on wheat grain characteristics and protein molecular weight distribution,” in Springer, Dordrecht, 365–371.
Caverzan, A., Casassola, A., and Patussi Brammer, S. (2016). “Reactive oxygen species and antioxidant enzymes involved in plant tolerance to stress,” in Abiotic and biotic stress in plants-recent advances and future perspectives. eds. A. K. Shanker and C. Shanker (InTech).
Chakraborty, S., Tiedemann, A. V., and Teng, P. S. (2000). Climate change: potential impact on plant diseases. Environ. Pollut. 108, 317–326. doi: 10.1016/S0269-7491(99)00210-9
Chen, Z., Ke, W., He, F., Chai, L., Cheng, X., Xu, H., et al. (2022). A single nucleotide deletion in the third exon of FT-D1 increases the spikelet number and delays heading date in wheat (Triticum aestivum L.). Plant Biotechnol. J. 20, 920–933. doi: 10.1111/pbi.13773
Clavijo, B. J., Venturini, L., Schudoma, C., Accinelli, G. G., Kaithakottil, G., Wright, J., et al. (2017). An improved assembly and annotation of the allohexaploid wheat genome identifies complete families of agronomic genes and provides genomic evidence for chromosomal translocations. Genome Res. 27, 885–896. doi: 10.1101/gr.217117.116
Cochard, H., Venisse, J. S., Barigah, T. S., Brunel, N., Herbette, S., Guilliot, A., et al. (2007). Putative role of aquaporins in variable hydraulic conductance of leaves in response to light. Plant Physiol. 143, 122–133. doi: 10.1104/pp.106.090092
Coles, G. D., Hartunian-Sowa, S. M., Jamieson, P. D., Hay, A. J., Atwell, W. A., and Fulcher, R. G. (1997). Environmentally-induced variation in starch and non-starch polysaccharide content in wheat. J. Cereal Sci. 26, 47–54. doi: 10.1006/jcrs.1996.0102
Collins, N. C., Tardieu, F., and Tuberosa, R. (2008). Quantitative trait loci and crop performance under abiotic stress: where do we stand? Plant Physiol. 147, 469–486. doi: 10.1104/pp.108.118117
Comastri, A., Janni, M., Simmonds, J., Uauy, C., Pignone, D., Nguyen, H. T., et al. (2018). Heat in wheat: exploit reverse genetic techniques to discover new alleles within the triticum durum shsp26 family. Front. Plant Sci. 9:1337. doi: 10.3389/fpls.2018.01337
Condon, A. G., Richards, R. A., Rebetzke, G. J., and Farquhar, G. D. (2004). Breeding for high water-use efficiency. J. Exp. Botany, 2447–2460. doi: 10.1093/jxb/erh277
Cossani, C. M., and Reynolds, M. P. (2012). Physiological traits for improving heat tolerance in wheat. Plant Physiol. 160, 1710–1718. doi: 10.1104/pp.112.207753
Cruz, C. D., and Valent, B. (2017). Wheat blast disease: danger on the move. Trop. Plant Pathol. 42, 210–222. doi: 10.1007/s40858-017-0159-z
Cui, X. (2020). Beyond yield response: weather shocks and crop abandonment. J. Assoc. Environ. Resour. Econ. 7, 901–932. doi: 10.1086/709859
Das, M. K., Bai, G., Mujeeb-Kazi, A., and Rajaram, S. (2016). Genetic diversity among synthetic hexaploid wheat accessions (Triticum aestivum) with resistance to several fungal diseases. Genet. Resour. Crop Evol. 63, 1285–1296. doi: 10.1007/s10722-015-0312-9
Davidson, J. F., and Schiestl, R. H. (2001). Mitochondrial respiratory Electron carriers are involved in oxidative stress during heat stress inSaccharomyces cerevisiae. Mol. Cell. Biol. 21, 8483–8489. doi: 10.1128/mcb.21.24.8483-8489.2001
Debbarma, J., Sarki, Y. N., Saikia, B., Boruah, H. P. D., Singha, D. L., and Chikkaputtaiah, C. (2019). Ethylene response factor (ERF) family proteins in abiotic stresses and CRISPR–Cas9 genome editing of ERFs for multiple abiotic stress tolerance in crop plants: a review. Mol. Biotechnol. 61, 153–172. doi: 10.1007/s12033-018-0144-x
Delgado-Baquerizo, M., Guerra, C. A., Cano-Díaz, C., Egidi, E., Wang, J.-T., Eisenhauer, N., et al. (2020). The proportion of soil-borne pathogens increases with warming at the global scale. Nat. Clim. Chang. 10, 550–554. doi: 10.1038/s41558-020-0759-3
Desaint, H., Aoun, N., Deslandes, L., Vailleau, F., Roux, F., and Berthomé, R. (2021). Fight hard or die trying: when plants face pathogens under heat stress. New Phytol. 229, 712–734. doi: 10.1111/nph.16965
Dias, A. S., Bagulho, A. S., and Lidon, F. C. (2008). Ultrastructure and biochemical traits of bread and durum wheat grains under heat stress. Brazilian J. Plant Physiol. 20, 323–333. doi: 10.1590/s1677-04202008000400008
Dias, A. S., and Lidon, F. C. (2009). Evaluation of grain filling rate and duration in bread and durum wheat, under heat stress after anthesis. J. Agron. Crop Sci. 195, 137–147. doi: 10.1111/j.1439-037X.2008.00347.x
Dias, A. S., and Lidon, F. C. (2010). Bread and durum wheat tolerance under heat stress: A synoptical overview. Emirates J. Food Agriculture 22, 412–436. doi: 10.9755/EJFA.V22I6.4660
Dias, A. S., Lidon, F. C., and Ramalho, J. C. (2009). I. Heat stress in Triticum: kinetics of ca and mg accumulation. Brazilian J. Plant Physiol. 21, 123–134. doi: 10.1590/S1677-04202009000200005
Dreisigacker, S., Crossa, J., Pérez-Rodríguez, P., Montesinos-L֯ópez, O. A., Rosyara, U., Juliana, P., et al. (2021). Implementation of genomic selection in the CIMMYT global wheat program, findings from the past 10 years. Crop Breed. Genet. Genom. 3:e210004. doi: 10.20900/cbgg20210005
Dubey, R., Pathak, H., Chakrabarti, B., Singh, S., Gupta, D. K., and Harit, R. C. (2020). Impact of terminal heat stress on wheat yield in India and options for adaptation. Agric. Syst. 181, 1–10. doi: 10.1016/j.agsy.2020.102826
Duveiller, E., Singh, R. P., and Nicol, J. M. (2007). The challenges of maintaining wheat productivity: pests, diseases, and potential epidemics. Euphytica 157, 417–430. doi: 10.1007/s10681-007-9380-z
El Haddad, N., Kabbaj, H., Zaïm, M., el Hassouni, K., Tidiane Sall, A., Azouz, M., et al. (2021). Crop wild relatives in durum wheat breeding: drift or thrift? Crop Sci. 61, 37–54. doi: 10.1002/csc2.20223
El Hassouni, K., Belkadi, B., Filali-Maltouf, A., Tidiane-Sall, A., al-Abdallat, A., Nachit, M., et al. (2019). Loci controlling adaptation to heat stress occurring at the reproductive stage in durum wheat. Agronomy 9, 1–20. doi: 10.3390/agronomy9080414
Elbashir, A. A. E., Gorafi, Y. S. A., Tahir, I. S. A., Elhashimi, A. M. A., Abdalla, M. G. A., and Tsujimoto, H. (2017). Genetic variation in heat tolerance-related traits in a population of wheat multiple synthetic derivatives. Breed. Sci. 67, 483–492. doi: 10.1270/jsbbs.17048
Elhadi, G. M. I., Kamal, N. M., Gorafi, Y. S. A., Yamasaki, Y., Ban, Y., Kato, K., et al. (2021). Novel loci for kernel hardness appeared as a response to heat and combined heat-drought conditions in wheat harboring Aegilops tauschii diversity. Agronomy 11. doi: 10.3390/agronomy11061061
Erenstein, O., and Laxmi, V. (2008). Zero tillage impacts in India’s rice-wheat systems: a review. Soil Tillage Res. 100, 1–14. doi: 10.1016/j.still.2008.05.001
Evans, L. T. (1978). The influence of irradiance before and after anthesis on grain yield and its components in microcrops of wheat grown in a constant daylength and temperature regime. F. Crop. Res. 1, 5–19. doi: 10.1016/0378-4290(78)90003-5
Evergreennews.com (2019). Scaling up of heat-tolerant wheat transforms food production, policy in Sudan–Ecogreen news. Available at: https://www.ecogreennews.com/scaling-up-of-heat-tolerant-wheat-transforms-food-production-policy-in-sudan/ (Accessed September 23, 2020)
Farooq, M., Bramley, H., Palta, J. A., and Siddique, K. H. M. (2011). Heat stress in wheat during reproductive and grain-filling phases. CRC. Crit. Rev. Plant Sci. 30, 491–507. doi: 10.1080/07352689.2011.615687
Feder, M. E., and Hofmann, G. E. (1999). Heat-shock proteins, molecular chaperones, and the stress response: evolutionary and ecological physiology. Annu. Rev. Physiol. 61, 243–282.
Fokar, M., Blum, A., and Nguyen, H. T. (1998a). Heat tolerance in spring wheat. II. Grain filling. Euphytica 104, 9–15. doi: 10.1023/A:1018322502271
Fokar, M., Nguyen, H. T., and Blum, A. (1998b). Heat tolerance in spring wheat. I. Estimating cellular thermotolerance and its heritability. Euphytica 104, 1–8. doi: 10.1023/A:1018346901363
Frank, A. B., and Bauer, A. (1997). Temperature effects prior to double ridge on apex development and Phyllochron in spring barley. Crop Sci. 37, 1527–1531. doi: 10.2135/cropsci1997.0011183X003700050019x
Fu, J., Momčilović, I., Clemente, T. E., Nersesian, N., Trick, H. N., and Ristic, Z. (2008). Heterologous expression of a plastid EF-Tu reduces protein thermal aggregation and enhances CO2 fixation in wheat (Triticum aestivum) following heat stress. Plant Mol. Biol. 68, 277–288. doi: 10.1007/s11103-008-9369-6
Gammans, M., Mérel, P., and Ortiz-Bobea, A. (2017). Negative impacts of climate change on cereal yields: statistical evidence from France. Environ. Res. Lett. 12:aa6b0c. doi: 10.1088/1748-9326/aa6b0c
Garg, D., Sareen, S., Dalal, S., Tiwari, R., and Singh, R. (2012). Heat shock protein based snp marker for terminal heat stress in wheat (Triticum aestivum L.). Aust. J. Crop. Sci. 6, 1516–1521.
Ghini, R., Hamada, E., Angelotti, F., Costa, L. B., and Bettiol, W. (2012). Research approaches, adaptation strategies, and knowledge gaps concerning the impacts of climate change on plant diseases. Trop. Plant Pathol. 37, 5–24. doi: 10.1590/S1982-56762012000100002
Gooding, M. J., Ellis, R. H., Shewry, P. R., and Schofield, J. D. (2003). Effects of restricted water availability and increased temperature on the grain filling, drying and quality of winter wheat. J. Cereal Sci. 37, 295–309. doi: 10.1006/jcrs.2002.0501
Grant, R. F., Kimball, B. A., Conley, M. M., White, J. W., Wall, G. W., and Ottman, M. J. (2011). Controlled warming effects on wheat growth and yield: field measurements and modeling. Agron. J. 103, 1742–1754. doi: 10.2134/agronj2011.0158
Grigorova, B., Vassileva, V., Klimchuk, D., Vaseva, I., Demirevska, K., and Feller, U. (2012). Drought, high temperature, and their combination affect ultrastructure of chloroplasts and mitochondria in wheat (Triticum aestivum L.) leaves. J. Plant Interact. 7, 204–213. doi: 10.1080/17429145.2011.654134
Guan, P., Lu, L., Jia, L., Kabir, M. R., Zhang, J., Lan, T., et al. (2018). Global QTL analysis identifies genomic regions on chromosomes 4A and 4B harboring stable loci for yield-related traits across different environments in wheat (Triticum aestivum L.). Front. Plant Sci. 9:529. doi: 10.3389/fpls.2018.00529
Gupta, N. K., Agarwal, S., Agarwal, V. P., Nathawat, N. S., Gupta, S., and Singh, G. (2013). Effect of short-term heat stress on growth, physiology and antioxidative defence system in wheat seedlings. Acta Physiol. Plant. 35, 1837–1842. doi: 10.1007/s11738-013-1221-1
Gupta, S. C., Sharma, A., Mishra, M., Mishra, R. K., and Chowdhuri, D. K. (2010). Heat shock proteins in toxicology: how close and how far? Life Sci. 86, 377–384. doi: 10.1016/j.lfs.2009.12.015
Haque, E., Taniguchi, H., Hassan, M. M., Bhowmik, P., Karim, M. R., Śmiech, M., et al. (2018). Application of CRISPR/Cas9 genome editing technology for the improvement of crops cultivated in tropical climates: recent progress, prospects, and challenges. Front. Plant Sci. 9:617. doi: 10.3389/fpls.2018.00617
Hasanuzzaman, M., Nahar, K., Alam, M. M., Roychowdhury, R., and Fujita, M. (2013). Physiological, biochemical, and molecular mechanisms of heat stress tolerance in plants. Int. J. Mol. Sci. 14, 9643–9684. doi: 10.3390/ijms14059643
Hassan, F. S. C., Solouki, M., Fakheri, B. A., Nezhad, N. M., and Masoudi, B. (2018). Mapping QTLs for physiological and biochemical traits related to grain yield under control and terminal heat stress conditions in bread wheat (Triticum aestivum L.). Physiol. Mol. Biol. Plants 24, 1231–1243. doi: 10.1007/s12298-018-0590-8
Hawker, J., and Jenner, C. (1993). High temperature affects the activity of enzymes in the committed pathway of starch synthesis in developing wheat endosperm. Funct. Plant Biol. 20:197. doi: 10.1071/pp9930197
Hedhly, A., Hormaza, J. I., and Herrero, M. (2009). Global warming and sexual plant reproduction. Trends Plant Sci. 14, 30–36. doi: 10.1016/j.tplants.2008.11.001
Heidari, B., Sayed-Tabatabaei, B. E., Saeidi, G., Kearsey, M., and Suenaga, K. (2011). Mapping QTL for grain yield, yield components, and spike features in a doubled haploid population of bread wheat. Genome 54, 517–527. doi: 10.1139/g11-017
Hossain, A., and Teixeira da Silva, J. A. (2012). Phenology, growth and yield of three wheat (Triticum aestivum L.) varieties as affected by high temperature stress. Not. Sci. Biol. 4, 97–109. doi: 10.15835/nsb437879
Howells, R. M., Craze, M., Bowden, S., and Wallington, E. J. (2018). Efficient generation of stable, heritable gene edits in wheat using CRISPR/Cas9. BMC Plant Biol. 18, 1–11. doi: 10.1186/S12870-018-1433-Z/FIGURES/2
Hu, X.-J., Chen, D., Lynne Mclntyre, C., Fernanda Dreccer, M., Zhang, Z.-B., Drenth, J., et al. (2018). Heat shock factor C2a serves as a proactive mechanism for heat protection in developing grains in wheat via an ABA-mediated regulatory pathway. Plant Cell Environ. 41, 79–98. doi: 10.1111/pce.12957
Hurkman, W. J., Vensel, W. H., Tanaka, C. K., Whitehand, L., and Altenbach, S. B. (2009). Effect of high temperature on albumin and globulin accumulation in the endosperm proteome of the developing wheat grain. J. Cereal Sci. 49, 12–23. doi: 10.1016/j.jcs.2008.06.014
Ibrahim, S., Saleem, B., Rehman, N., Zafar, S. A., Naeem, M. K., and Khan, M. R. (2022). CRISPR/Cas9 mediated disruption of inositol Pentakisphosphate 2-kinase 1 (TaIPK1) reduces phytic acid and improves iron and zinc accumulation in wheat grains. J. Adv. Res. 37, 33–41. doi: 10.1016/j.jare.2021.07.006
Irmak, S., Naeem, H. A., Lookhart, G. L., and MacRitchie, F. (2008). Effect of heat stress on wheat proteins during kernel development in wheat near-isogenic lines differing at Glu-D1. J. Cereal Sci. 48, 513–516. doi: 10.1016/j.jcs.2007.12.002
Ishag, H. M. (1994). “Genotype differences in heat stress in wheat in the irrigated Gezira scheme” in Wheat in heat stressed environments: Irrigated, dry areas and rice wheat cropping systems. eds. D. A. Saunders and G. P. Hettel (CIMMYT), 170–174.
Islam, A. U., Chhabra, A. K., Dhanda, S. S., and Munjal, R. (2017). Cell membrane stability-an important criterion for selection of heat tolerant genotypes in wheat (Triticum aestivum L.). J. Appl. Nat. Sci. 9, 1894–1900. doi: 10.31018/jans.v9i4.1458
Itam, M. O., Gorafi, Y. S. A., Tahir, I. S. A., and Tsujimoto, H. (2021). Genetic variation in drought resilience-related traits among wheat multiple synthetic derivative lines: insights for climate resilience breeding. Breed. Sci. 71, 435–443. doi: 10.1270/jsbbs.20162
Itam, M. O., Mega, R., Gorafi, Y. S. A., Yamasaki, Y., Tahir, I. S. A., Akashi, K., et al. (2022). Genomic analysis for heat and combined heat–drought resilience in bread wheat under field conditions. Theor. Appl. Genet. 135, 337–350. doi: 10.1007/s00122-021-03969-x
Iwai, M., Katayama, M., and Ikeuchi, M. (2006). Absence of the psbH gene product destabilizes the photosystem II complex and prevents association of the photosystem II-X protein in the thermophilic cyanobacterium Thermosynechococcus elongatus BP-1. Photosynth. Res. 87, 313–322. doi: 10.1007/s11120-005-9013-0
Jaganathan, D., Ramasamy, K., Sellamuthu, G., Jayabalan, S., and Venkataraman, G. (2018). CRISPR for crop improvement: an update review. Front. Plant Sci. 9:985. doi: 10.3389/fpls.2018.00985
Jiang, F., and Doudna, J. A. (2017). CRISPR–Cas9 structures and mechanisms. Annu. Rev. Biophys. 46, 505–529. doi: 10.1146/annurev-biophys-062215-010822
Jing, J., Guo, S., Li, Y., and Li, W. (2020). The alleviating effect of exogenous polyamines on heat stress susceptibility of different heat resistant wheat (Triticum aestivum L.) varieties. Sci. Rep. 10, 1–12. doi: 10.1038/s41598-020-64468-5
Juliana, P., Montesinos-López, O. A., Crossa, J., Mondal, S., González Pérez, L., Poland, J., et al. (2019a). Integrating genomic-enabled prediction and high-throughput phenotyping in breeding for climate-resilient bread wheat. Theor. Appl. Genet. 132, 177–194. doi: 10.1007/s00122-018-3206-3
Juliana, P., Poland, J., Huerta-Espino, J., Shrestha, S., Crossa, J., Crespo-Herrera, L., et al. (2019b). Improving grain yield, stress resilience and quality of bread wheat using large-scale genomics. Nat. Genet. 51, 1530–1539. doi: 10.1038/s41588-019-0496-6
Kayastha, P., KC, B., Pandey, B., Magar, B. R., Chand, H., Bhandari, J., et al. (2023). Molecular basis of heat stress tolerance in wheat. J. Agric. Appl. Biol. 4, 11–19. doi: 10.11594/jaab.04.01.02
Keeling, P. L., Bacon, P. J., and Holt, D. C. (1993). Elevated temperature reduces starch deposition in wheat endosperm by reducing the activity of soluble starch synthase. Planta 191, 342–348. doi: 10.1007/BF00195691
Khan, M. I. R., Iqbal, N., Masood, A., Per, T. S., and Khan, N. A. (2013). Salicylic acid alleviates adverse effects of heat stress on photosynthesis through changes in proline production and ethylene formation. Plant Signal. Behav. 8:26374. doi: 10.4161/psb.26374
Khanna-Chopra, R. (2012). Leaf senescence and abiotic stresses share reactive oxygen species-mediated chloroplast degradation. Protoplasma 249, 469–481. doi: 10.1007/s00709-011-0308-z
Kim, D., Alptekin, B., and Budak, H. (2018). CRISPR/Cas9 genome editing in wheat. Funct. Integr. Genomics 18, 31–41. doi: 10.1007/s10142-017-0572-x
Kumar, R. R., Goswami, S., Gadpayle, K. A., Singh, K., Sharma, S. K., Singh, G. P., et al. (2014). Ascorbic acid at pre-anthesis modulate the thermotolerance level of wheat (Triticum aestivum) pollen under heat stress. J. Plant Biochem. Biotechnol. 23, 293–306. doi: 10.1007/s13562-013-0214-x
Kumar, R. R., Goswami, S., Gupta, R., Verma, P., Singh, K., Singh, J. P., et al. (2016). The stress of suicide: temporal and spatial expression of putative heat shock protein 70 protect the cells from heat injury in wheat (Triticum aestivum). J. Plant Growth Regul. 35, 65–82. doi: 10.1007/s00344-015-9508-7
Kumar, A., Sharma, S., Chunduri, V., Kaur, A., Kaur, S., Malhotra, N., et al. (2020). Genome-wide identification and characterization of heat shock protein family reveals role in development and stress conditions in Triticum aestivum L. Sci. Rep. 10, 7858–7812. doi: 10.1038/s41598-020-64746-2
Kumar, R., Tripathi, G., Goyal, I., Sharma, J., Tiwari, R., Shimphrui, R., et al. (2023). Insights into genomic variations in rice Hsp100 genes across diverse rice accessions. Planta 257, 1–13. doi: 10.1007/S00425-023-04123-1/METRICS
Lande, R., and Thompson, R. (1990). Efficiency of marker-assisted selection in the improvement of quantitative traits. Genetics 124, 743–756. doi: 10.1093/genetics/124.3.743
Larkin, D. L., Holder, A. L., Mason, R. E., Moon, D. E., Brown-Guedira, G., Price, P. P., et al. (2020). Genome-wide analysis and prediction of fusarium head blight resistance in soft red winter wheat. Crop Sci. 60, 2882–2900. doi: 10.1002/csc2.20273
Lea, P., and Leegood, R. C. (1999). Plant biochemistry and molecular biology. Chichester: John Wiley & Sons.
Li, X. M., Chao, D. Y., Wu, Y., Huang, X., Chen, K., Cui, L. G., et al. (2015). Natural alleles of a proteasome α2 subunit gene contribute to thermotolerance and adaptation of African rice. Nat. Genet. 47, 827–833. doi: 10.1038/ng.3305
Li, L., Mao, X., Wang, J., Chang, X., Reynolds, M., and Jing, R. (2019). Genetic dissection of drought and heat-responsive agronomic traits in wheat. Plant Cell Environ. 42, 2540–2553. doi: 10.1111/pce.13577
Li, Y. F., Wu, Y., Hernandez-Espinosa, N., and Peña, R. J. (2013). Heat and drought stress on durum wheat: responses of genotypes, yield, and quality parameters. J. Cereal Sci. 57, 398–404. doi: 10.1016/j.jcs.2013.01.005
Li, Z., Zhang, L., Wang, A., Xu, X., and Li, J. (2013). Ectopic overexpression of SlHsfA3, a heat stress transcription factor from tomato, confers increased Thermotolerance and salt hypersensitivity in germination in transgenic Arabidopsis. PLoS One 8:e54880. doi: 10.1371/journal.pone.0054880
Liang, Z., Chen, K., Li, T., Zhang, Y., Wang, Y., Zhao, Q., et al. (2017). Efficient DNA-free genome editing of bread wheat using CRISPR/Cas9 ribonucleoprotein complexes. Nat. Commun. 8:14261. doi: 10.1038/ncomms14261
Lin, Q., Zong, Y., Xue, C., Wang, S., Jin, S., Zhu, Z., et al. (2020). Prime genome editing in rice and wheat. Nat. Biotechnol. 38, 582–585. doi: 10.1038/s41587-020-0455-x
Liu, H., Able, A. J., and Able, J. A. (2019). Genotypic performance of Australian durum under single and combined water-deficit and heat stress during reproduction. Sci. Rep. 9:14986. doi: 10.1038/s41598-019-49871-x
Liu, C., Pinto, F., Cossani, C. M., Sukumaran, S., and Reynolds, M. P. (2019). Spectral reflectance indices as proxies for yield potential and heat stress tolerance in spring wheat: heritability estimates and marker-trait associations. Front. Agric. Sci. Eng. 6, 296–308. doi: 10.15302/J-FASE-2019269
Lobell, D. B., Schlenker, W., and Costa-Roberts, J. (2011). Climate trends and global crop production since 1980. Science 333, 616–620. doi: 10.1126/science.1204531
Lobell, D. B., Sibley, A., and Ivan Ortiz-Monasterio, J. (2012). Extreme heat effects on wheat senescence in India. Nat. Clim. Chang. 2, 186–189. doi: 10.1038/nclimate1356
Ma, Y., Szostkiewicz, I., Korte, A., Moes, D., Yang, Y., Christmann, A., et al. (2009). Regulators of PP2C phosphatase activity function as abscisic acid sensors. Science 324, 1064–1068. doi: 10.1126/science.1172408
Ma’arup, R., Trethowan, R. M., Ahmed, N. U., Bramley, H., and Sharp, P. J. (2020). Emmer wheat (Triticum dicoccon Schrank) improves water use efficiency and yield of hexaploid bread wheat. Plant Sci. 295:110212. doi: 10.1016/j.plantsci.2019.110212
Madgwick, J. W., West, J. S., White, R. P., Semenov, M. A., Townsend, J. A., Turner, J. A., et al. (2011). Impacts of climate change on wheat anthesis and fusarium ear blight in the UK. Eur. J. Plant Pathol. 130, 117–131. doi: 10.1007/s10658-010-9739-1
Majoul-Haddad, T., Bancel, E., Martre, P., Triboi, E., and Branlard, G. (2013). Effect of short heat shocks applied during grain development on wheat (Triticum aestivum L.) grain proteome. J. Cereal Sci. 57, 486–495. doi: 10.1016/j.jcs.2013.02.003
Martínez-Ballesta, M. C., López-Pérez, L., Muries, B., Muñoz-Azcarate, O., and Carvajal, M. (2009). “Climate change and plant water balance: the role of Aquaporins – a review” in Climate change, intercropping, Pest control and beneficial microorganisms. ed. E. Lichtfouse (Dordrecht: Springer Netherlands), 71–89.
Marutani, Y., Yamauchi, Y., Kimura, Y., Mizutani, M., and Sugimoto, Y. (2012). Damage to photosystem II due to heat stress without light-driven electron flow: involvement of enhanced introduction of reducing power into thylakoid membranes. Planta 236, 753–761. doi: 10.1007/s00425-012-1647-5
Mason, R. E., Mondal, S., Beecher, F. W., Pacheco, A., Jampala, B., Ibrahim, A. M. H., et al. (2010). QTL associated with heat susceptibility index in wheat (Triticum aestivum L.) under short-term reproductive stage heat stress. Euphytica 174, 423–436. doi: 10.1007/s10681-010-0151-x
Mathur, S., Agrawal, D., and Jajoo, A. (2014). Photosynthesis: response to high temperature stress. J. Photochem. Photobiol. B Biol. 137, 116–126. doi: 10.1016/j.jphotobiol.2014.01.010
Mathur, S., Jajoo, A., Mehta, P., and Bharti, S. (2011). Analysis of elevated temperature-induced inhibition of photosystem II using chlorophyll a fluorescence induction kinetics in wheat leaves (Triticum aestivum). Plant Biol. 13, 1–6. doi: 10.1111/j.1438-8677.2009.00319.x
Maulana, F., Ayalew, H., Anderson, J. D., Kumssa, T. T., Huang, W., and Ma, X. F. (2018). Genome-wide association mapping of seedling heat tolerance in winter wheat. Front. Plant Sci. 9:1272. doi: 10.3389/FPLS.2018.01272/BIBTEX
Mboup, M., Bahri, B., Leconte, M., De Vallavieille-Pope, C., Kaltz, O., and Enjalbert, J. (2012). Genetic structure and local adaptation of European wheat yellow rust populations: the role of temperature-specific adaptation. Evol. Appl. 5, 341–352. doi: 10.1111/j.1752-4571.2011.00228.x
McClung, C. R., and Davis, S. J. (2010). Ambient thermometers in plants: from physiological outputs towards mechanisms of thermal sensing. Curr. Biol. 20, R1086–R1092. doi: 10.1016/j.cub.2010.10.035
Mengutay, M., Ceylan, Y., Kutman, U. B., and Cakmak, I. (2013). Adequate magnesium nutrition mitigates adverse effects of heat stress on maize and wheat. Plant Soil 368, 57–72. doi: 10.1007/s11104-013-1761-6
Meuwissen, T. H. E., Hayes, B. J., and Goddard, M. E. (2001). Prediction of total genetic value using genome-wide dense marker maps. Genetics 157, 1819–1829. doi: 10.1093/genetics/157.4.1819
Miedaner, T. (2018). Wo hat der Anbau seine Grenzen? [maize–where are the limits of cultivation? In German]. Innov. Mais 3, 22–25.
Mishra, R., Joshi, R. K., and Zhao, K. (2020). Base editing in crops: current advances, limitations and future implications. Plant Biotechnol. J. 18, 20–31. doi: 10.1111/pbi.13225
Mishra, R. K., and Singhal, G. S. (1992). Function of photosynthetic apparatus of intact wheat leaves under high light and heat stress and its relationship with peroxidation of thylakoid lipids. Plant Physiol. 98, 1–6. doi: 10.1104/pp.98.1.1
Mitra, R., and Bhatia, C. R. (2008). Bioenergetic cost of heat tolerance in wheat crop. Curr. Sci. 94, 1049–1053.
Mittler, R., Vanderauwera, S., Suzuki, N., Miller, G., Tognetti, V. B., Vandepoele, K., et al. (2011). ROS signaling: the new wave? Trends Plant Sci. 16, 300–309. doi: 10.1016/j.tplants.2011.03.007
Moerschbacher, B. M., Witte, U., Königs, D., and Reisener, H.-J. (1989). Changes in the level of enzyme activities involved in lignin biosynthesis during the temperature sensitive resistant response of wheat (Sr6) to stem rust (P6). Plant Sci. 65, 183–190.
Mohammadi, V. A., Zali, A. A., and Bihamta, M. R. (2008). Mapping QTLs for heat tolerance in wheat. J. Agric. Sci. Technol. 10, 261–267.
Mondal, S., Mason, R. E., Huggins, T., and Hays, D. B. (2015). QTL on wheat (Triticum aestivum L.) chromosomes 1B, 3D and 5A are associated with constitutive production of leaf cuticular wax and may contribute to lower leaf temperatures under heat stress. Euphytica 201, 123–130. doi: 10.1007/s10681-014-1193-2
Mondal, S., Sallam, A., Sehgal, D., Sukumaran, S., Farhad, M., Krishnan, J. N., et al. (2021). “Advances in breeding for abiotic stress tolerance in wheat” in Genomic designing for abiotic stress resistant cereal crops. ed. C. Kole (Basel: Springer Nature Switzerland), 71–103.
Mondal, S., Singh, R. P., Crossa, J., Huerta-Espino, J., Sharma, I., Chatrath, R., et al. (2013). Earliness in wheat: a key to adaptation under terminal and continual high temperature stress in South Asia. Field Crops Res. 151, 19–26. doi: 10.1016/j.fcr.2013.06.015
Naeem, H. A., Paulon, D., Irmak, S., and MacRitchie, F. (2012). Developmental and environmental effects on the assembly of glutenin polymers and the impact on grain quality of wheat. J. Cereal Sci. 56, 51–57. doi: 10.1016/j.jcs.2011.10.014
Nguyen, H.-C., Lin, K.-H., Ho, S.-L., Chiang, C.-M., and Yang, C.-M. (2018). Enhancing the abiotic stress tolerance of plants: from chemical treatment to biotechnological approaches. Physiol. Plant. 164, 452–466. doi: 10.1111/ppl.12812
Ni, Z., Li, H., Zhao, Y., Peng, H., Hu, Z., Xin, M., et al. (2018). Genetic improvement of heat tolerance in wheat: recent progress in understanding the underlying molecular mechanisms. Crop J. 6, 32–41. doi: 10.1016/j.cj.2017.09.005
Nicolas, M. E., Lambers, H., Simpson, R. J., and Dalling, M. J. (1985). Effect of drought on metabolism and partitioning of carbon in two wheat varieties differing in drought-tolerance. Ann. Bot. 55, 727–742. doi: 10.1093/oxfordjournals.aob.a086951
Oerke, E.-C. (2006). Crop losses to pests. J. Agric. Sci. 144, 31–43. doi: 10.1017/S0021859605005708
Okada, A., Arndell, T., Borisjuk, N., Sharma, N., Watson-Haigh, N. S., Tucker, E. J., et al. (2019). CRISPR/Cas9-mediated knockout of Ms1 enables the rapid generation of male-sterile hexaploid wheat lines for use in hybrid seed production. Plant Biotechnol. J. 17, 1905–1913. doi: 10.1111/PBI.13106
Paliwal, R., Röder, M. S., Kumar, U., Srivastava, J. P., and Joshi, A. K. (2012). QTL mapping of terminal heat tolerance in hexaploid wheat (T. aestivum L.). Theor. Appl. Genet. 125, 561–575. doi: 10.1007/s00122-012-1853-3
Pangga, I. B., Hanan, J., and Chakraborty, S. (2013). Climate change impacts on plant canopy architecture: implications for pest and pathogen management. Eur. J. Plant Pathol. 135, 595–610. doi: 10.1007/s10658-012-0118-y
Parmar, N., Singh, K. H., Sharma, D., Singh, L., Kumar, P., Nanjundan, J., et al. (2017). Genetic engineering strategies for biotic and abiotic stress tolerance and quality enhancement in horticultural crops: a comprehensive review. Biotech 7:239. doi: 10.1007/s13205-017-0870-y
Peck, A. W., and McDonald, G. K. (2010). Adequate zinc nutrition alleviates the adverse effects of heat stress in bread wheat. Plant Soil 337, 355–374. doi: 10.1007/s11104-010-0532-x
Peng, J., Sun, D., Peng, Y., and Nevo, E. (2013). Gene discovery in Triticum dicoccoides, the direct progenitor of cultivated wheats. Cereal Res. Commun. 41, 1–22. doi: 10.1556/CRC.2012.0030
Peng, S., Zhu, Z., Zhao, K., Shi, J., Yang, Y., He, M., et al. (2013). A novel heat shock transcription factor, VpHsf1, from Chinese wild Vitis pseudoreticulata is involved in biotic and abiotic stresses. Plant Mol. Biol. Report. 31, 240–247. doi: 10.1007/s11105-012-0463-1
Pirasteh-Anosheh, H., Saed-Moucheshi, A., Pakniyat, H., and Pessarakli, M. (2016). “Stomatal responses to drought stress” in Water Stress and Crop Plants: A Sustainable Approach. ed. P. Ahmad (Chichester, UK: John Wiley & Sons, Ltd), 24–40.
Poudel, P. B., and Poudel, M. R. (2020). Heat stress effects and tolerance in wheat: a review. J. Biol. Today’s World 9, 1–6.
Pradhan, G. P., and Prasad, P. V. V. (2015). Evaluation of wheat chromosome translocation lines for high temperature stress tolerance at grain filling stage. PLoS One 10:e0116620. doi: 10.1371/journal.pone.0116620
Prakash, P., Sharma-Natu, P., and Ghildiyal, M. C. (2004). Effect of different temperature on starch synthase activity in excised grains of wheat cultivars. Indian J. Exp. Biol. 42, 227–230.
Prasad, P. V. V., Staggenborg, S. A., and Ristic, Z. (2008). “Impacts of drought and/or heat stress on physiological, developmental, growth, and yield processes of crop plants” in Responses of crops to limited water: Understanding and modeling water stress effects on plant growth processes. eds. L. Ahuja, V. Reddy, S. Saseendran, and Q. Yu (Madison: American Society of Agronomy, Inc.), 301–355.
Qin, D., Wu, H., Peng, H., Yao, Y., Ni, Z., Li, Z., et al. (2008). Heat stress-responsive transcriptome analysis in heat susceptible and tolerant wheat (Triticum aestivum L.) by using wheat genome Array. BMC Genomics 9, 1–19. doi: 10.1186/1471-2164-9-432
Rahman, M. A., Chikushi, J., Yoshida, S., and Karim, A. J. M. (2009). Growth and yield components of wheat genotypes exposed to high temperature stress under control environment. Bangladesh J. Agric. Res. 34, 361–372. doi: 10.3329/bjar.v34i3.3961
Rakszegi, M., Láng, L., and Bedő, Z. (2006). Importance of starch properties in quality oriented wheat breeding. Cereal Res. Commun. 34, 637–640. doi: 10.1556/crc.34.2006.1.159
Ratnakumar, P., Khan, M. I. R., Minhas, P. S., Farooq, M. A., Sultana, R., Per, T. S., et al. (2016). Can plant bio-regulators minimize crop productivity losses caused by drought, salinity and heat stress? An integrated review. J. Appl. Bot. Food Qual. 89, 113–125. doi: 10.5073/JABFQ.2016.089.014
Raza, A., Mehmood, S. S., Ashraf, F., and Khan, R. S. A. (2019). Genetic diversity analysis of Brassica species using PCR-based SSR markers. Gesunde Pflanz. 71, 1–7. doi: 10.1007/s10343-018-0435-y
Rebetzke, G. J., Condon, A. G., Richards, R. A., and Farquhar, G. D. (2003). Gene action for leaf conductance in three wheat crosses. Aust. J. Agric. Res. 54, 381–387. doi: 10.1071/AR02151
Reynolds, M. P., Singh, R. P., Ibrahim, A., Ageeb, O. A. A., Larqué-Saavedra, A., and Quick, J. S. (1998). “Evaluating physiological traits to complement empirical selection for wheat in warm environments,” in Euphytica. (Kluwer Academic Publishers), Vol 100, 85–94.
Richards, R., Rawson, H., and Johnson, D. (1986). Glaucousness in wheat: its development and effect on water-use efficiency, gas exchange and photosynthetic tissue temperatures*. Funct. Plant Biol. 13:465. doi: 10.1071/pp9860465
Ristic, Z., Momilović, I., Bukovnik, U., Prasad, P. V. V., Fu, J., Deridder, B. P., et al. (2009). Rubisco activase and wheat productivity under heat-stress conditions. J. Exp. Bot. 60, 4003–4014. doi: 10.1093/jxb/erp241
Roychowdhury, R., Zilberman, O., Chandrasekhar, K., Curzon, A. Y., Nashef, K., Abbo, S., et al. (2023). Pre-anthesis spike growth dynamics and its association to yield components among elite bread wheat cultivars (Triticum aestivum L. spp.) under Mediterranean climate. Crop. Res. 298:108948. doi: 10.1016/j.fcr.2023.108948
Ruelland, E., and Zachowski, A. (2010). How plants sense temperature. Environ. Exp. Bot. 69, 225–232. doi: 10.1016/j.envexpbot.2010.05.011
Ruqiang, X., Qixin, S., and Shuzhen, Z. (1996). Chromosomal location of genes for heat tolerance as measured by membrane thermostability of common wheat cv. Hope. Yi chuan = Hered. 18, 1—3. Available at: https://europepmc.org/article/cba/298013 (Accessed December 1, 2020)
Saini, H. S., and Aspinall, D. (1982). Abnormal sporogenesis in wheat (Triticum aestivum L.) induced by short periods of high temperature. Ann. Bot. 49, 835–846. doi: 10.1093/oxfordjournals.aob.a086310
Saini, D. K., Chopra, Y., Singh, J., Sandhu, K. S., Kumar, A., Bazzer, S., et al. (2022). Comprehensive evaluation of mapping complex traits in wheat using genome-wide association studies. Mol. Breed. 42:1272. doi: 10.1007/s11032-021-01272-7
Saini, H. S., Sedgley, M., and Aspinall, D. (1983). Effect of heat stress during floral development on pollen tube growth and ovary anatomy in wheat (Triticum aestivum L.). Aust. J. Plant Physiol. 10, 137–144. doi: 10.1071/PP9830137
Sairam, R. K., Deshmukh, P. S., and Shukla, D. S. (1997). Tolerance of drought and temperature stress in relation to increased antioxidant enzyme activity in wheat. J. Agron. Crop Sci. 178, 171–178. doi: 10.1111/j.1439-037X.1997.tb00486.x
Sall, A. T., Chiari, T., Legesse, W., Seid-Ahmed, K., Ortiz, R., Van Ginkel, M., et al. (2019). Durum wheat (Triticum durum Desf.): origin, cultivation and potential expansion in sub-saharan Africa. Agronomy 9:263. doi: 10.3390/agronomy9050263
Sangwan, S., Munjal, R., Ram, K., and Kumar, N. (2019). QTL mapping for morphological and physiological traits in RILs of spring wheat population of WH1021 X WH711. J. Environ. Biol. 40, 674–682. doi: 10.22438/jeb/40/4/MRN-1002
Sarieva, G. E., Kenzhebaeva, S. S., and Lichtenthaler, H. K. (2010). Adaptation potential of photosynthesis in wheat cultivars with a capability of leaf rolling under high temperature conditions. Russ. J. Plant Physiol. 57, 28–36. doi: 10.1134/S1021443710010048
Savicka, M., and Škute, N. (2010). Effects of high temperature on malondialdehyde content, superoxide production and growth changes in wheat seedlings (Triticum aestivum L.) Aukštos temperatūros poveikis kviečių (Triticum aestivum L.). Ekologija 56, 26–33. doi: 10.2478/v10055-010-0004-x
Scafaro, A. P., Bautsoens, N., Boer, B.Den, Van Rie, J., and Gallé, A. (2019). A conserved sequence from heat-adapted species improves rubisco activase thermostability in wheat. Plant Physiol. 181, 43–54. doi: 10.1104/pp.19.00425.
Semenov, M. A. (2009). Impacts of climate change on wheat in England and Wales. J. R. Soc. Interface 6, 343–350. doi: 10.1098/rsif.2008.0285
Shan, Q., Wang, Y., Li, J., and Gao, C. (2014). Genome editing in rice and wheat using the CRISPR/Cas system. Nat. Protoc. 9, 2395–2410. doi: 10.1038/nprot.2014.157
Shanahan, J. F., Edwards, I. B., Quick, J. S., and Fenwick, J. R. (1990). Membrane Thermostability and heat tolerance of spring wheat. Crop Sci. 30:247. doi: 10.2135/cropsci1990.0011183x0003000020001x
Sharma, D., Singh, R., Tiwari, R., Kumar, R., and Gupta, V. K. (2019). “Wheat responses and tolerance to terminal heat stress: a review” in Wheat Production in Changing Environments. eds. M. Hasanuzzaman, K. Nahar, and Md. A. Hossain (Singapore: Springer), 149–173.
Shi, W., Yang, J., Kumar, R., Zhang, X., Impa, S. M., Xiao, G., et al. (2022). Heat stress during gametogenesis irreversibly damages female reproductive organ in Rice. Rice 15, 1–18. doi: 10.1186/S12284-022-00578-0/FIGURES/11
Shirdelmoghanloo, H., Taylor, J. D., Lohraseb, I., Rabie, H., Brien, C., Timmins, A., et al. (2016). A QTL on the short arm of wheat (Triticum aestivum L.) chromosome 3B affects the stability of grain weight in plants exposed to a brief heat shock early in grain filling. BMC Plant Biol. 16, 1–15. doi: 10.1186/s12870-016-0784-6
Singh, P. K., Gahtyari, N. C., Roy, C., Roy, K. K., He, X., Tembo, B., et al. (2021). Wheat blast: a disease spreading by intercontinental jumps and its management strategies. Front. Plant Sci. 12:710707. doi: 10.3389/fpls.2021.710707
Singh, R. P., Juliana, P., Huerta-Espino, J., Govindan, V., Crespo-Herrera, L. A., Mondal, S., et al. (2022). “Achieving genetic gains in practice BT – wheat improvement: food security in a changing climate” in Achieving genetic gains in practice. eds. M. P. Reynolds and H.-J. Braun (Cham: Springer International Publishing), 97–123.
Singh, A., Singh, D., and Gill, B. (2011a). “Planting time, methods, and practices to reduce the deleterious effects of high-temperature on wheat” in The proceedings of international conference on preparing agriculture for climate change (Ludhiana, Punjab: Punjab Agricultural University), 338–339.
Singh, A., Singh, D., Kang, J. S., and Aggarwal, N. (2011b). Management practices to mitigate the impact of high temperature on wheat: a review. IIOAB J. 2, 11–22.
Singh, D., Wang, X., Kumar, U., Gao, L., Noor, M., Imtiaz, M., et al. (2019). High-throughput phenotyping enabled genetic dissection of crop lodging in wheat. Front. Plant Sci. 10:143. doi: 10.3389/fpls.2019.00394
Sofield, I., Evans, L., Cook, M., and Wardlaw, I. (1977). Factors influencing the rate and duration of grain filling in wheat. Funct. Plant Biol. 4:785. doi: 10.1071/pp9770785
Song, J., Carver, B. F., Powers, C., Yan, L., Klápště, J., El-Kassaby, Y. A., et al. (2017). Practical application of genomic selection in a doubled-haploid winter wheat breeding program. Mol. Breed. 37:117. doi: 10.1007/s11032-017-0715-8
Song, W. F., Zhao, L. J., Zhang, X. M., Zhang, Y. M., Li, J. L., Zhang, L. L., et al. (2015). Effect of timing of heat stress during grain filling in two wheat varieties under moderate and very high temperature. Indian J. Genet. Plant Breed. 75, 121–124. doi: 10.5958/0975-6906.2015.00018.8
Stone, P. J., and Nicolas, M. E. (1995). Comparison of sudden heat stress with gradual exposure to high temperature during grain filling in two wheat varieties differing in heat tolerance. I. Grain growth. Aust. J. Plant Physiol. 22, 935–944. doi: 10.1071/PP9950935
Stone, P. J., and Nicolas, M. E. (1996). Effect of timing of heat stress during grain filling on two wheat varieties differing in heat tolerance. II. Fractional protein accumulation. Aust. J. Plant Physiol. 23, 739–749. doi: 10.1071/PP9960739
Sumesh, K. V., Sharma-Natu, P., and Ghildiyal, M. C. (2008). Starch synthase activity and heat shock protein in relation to thermal tolerance of developing wheat grains. Biol. Plant. 52, 749–753. doi: 10.1007/s10535-008-0145-x
Sun, J., Khan, M., Amir, R., and Gul, A. (2020). Chapter 23 – genomic selection in wheat breeding. Cambridge, MA: Academic Press), 321–330.
Sun, Q. X., and Quick, J. S. (1991). Chromosomal locations of genes for heat tolerance in tetraploid wheat. Cereal Res. Commun. 19, 431–437.
Sun, L., Wen, J., Peng, H., Yao, Y., Hu, Z., Ni, Z., et al. (2022). The genetic and molecular basis for improving heat stress tolerance in wheat. aBIOTECH 3, 25–39. doi: 10.1007/s42994-021-00064-z
Suzuki, N., Koussevitzky, S., Mittler, R., and Miller, G. (2012). ROS and redox signalling in the response of plants to abiotic stress. Plant Cell Environ. 35, 259–270. doi: 10.1111/j.1365-3040.2011.02336.x
Talukder, S. K., Babar, M. A., Vijayalakshmi, K., Poland, J., Prasad, P. V. V., Bowden, R., et al. (2014). Mapping QTL for the traits associated with heat tolerance in wheat (Triticum aestivuml.). BMC Genet. 15:19. doi: 10.1186/s12863-014-0097-4
Tayade, R., Nguyen, T. D., Oh, S. A., Hwang, Y. S., Yoon, I. S., Deshmuk, R., et al. (2018). Effective strategies for enhancing tolerance to high-temperature stress in rice during the reproductive and ripening stages. Plant Breed. Biotechnol. 6, 1–18. doi: 10.9787/PBB.2018.6.1.1
Telfer, P., Edwards, J., Norman, A., Bennett, D., Smith, A., Able, J. A., et al. (2021). Genetic analysis of wheat (Triticum aestivum) adaptation to heat stress. Theor. Appl. Genet. 134, 1387–1407. doi: 10.1007/S00122-021-03778-2/METRICS
The National Oceanic and Atmospheric Administration (2023). Temperature – global yearly, difference from average | NOAA Climate.gov. Available at: https://www.climate.gov/maps-data/data-snapshots/data-source/temperature-global-yearly-difference-average (Accessed April 28, 2023)
The World Bank (2019). Population growth (annual%). Available at: https://data.worldbank.org/indicator/SP.POP.GROW (Accessed September 5, 2020)
Thind, T. S. (2012). Presidential address-fungicides in crop health security–the road ahead. Indian Phytopathol. 65, 109–115.
Thomelin, P. M. L., Bonneau, J., Taylor, J. D., Choulet, F., Sourdille, P., Langridge, P., et al. (2016). Positional cloning of a QTL, qDHY.3BL, on chromosome 3BL for drought and heat tolerance in bread wheat. In proceedings of the plant and animal genome conference (PAGXXIV) (San Diego, USA), np. Available at: https://hal.archives-ouvertes.fr/hal-02340444 (Accessed December 1, 2020)
Tian, F., Bradbury, P. J., Brown, P. J., Hung, H., Sun, Q., Flint-Garcia, S., et al. (2011). Genome-wide association study of leaf architecture in the maize nested association mapping population. Nat. Genet. 43, 159–162. doi: 10.1038/ng.746
Trapero-Mozos, A., Morris, W. L., Ducreux, L. J. M. M., McLean, K., Stephens, J., Torrance, L., et al. (2018). Engineering heat tolerance in potato by temperature-dependent expression of a specific allele of Heat-Shock Cognate 70. Plant Biotechnol. J. 16, 197–207. doi: 10.1111/pbi.12760
Tripathi, A., Tripathi, D. K., Chauhan, D. K., Kumar, N., and Singh, G. S. (2016). Paradigms of climate change impacts on some major food sources of the world: a review on current knowledge and future prospects. Agric. Ecosyst. Environ. 216, 356–373. doi: 10.1016/j.agee.2015.09.034
Tsujimoto, H., Sohail, Q., Matsuoka, Y., Ogihara, Y., Takumi, S., and Handa, H. (2015). Advances in wheat genetics: From genome to field. Proceedings of the 12th international wheat genetics symposium.
Ullah, S., Randhawa, I. A. S., and Trethowan, R. (2021). Genome-wide association study of multiple traits linked to heat tolerance in emmer-derived hexaploid wheat genotypes. Mol. Breed. 41:29. doi: 10.1007/s11032-021-01222-3
Upreti, K. K., and Sharma, M. (2016). “Role of plant growth regulators in abiotic stress tolerance” in Abiotic Stress Physiology of Horticultural Crops. eds. N. K. Srinivasa Rao, K. S. Shivashankara, and R. H. Laxman (New Delhi, India: Springer), 19–46.
Vargas, M., Van Eeuwijk, F. A., Crossa, J., and Ribaut, J. M. (2006). Mapping QTLs and QTL x environment interaction for CIMMYT maize drought stress program using factorial regression and partial least squares methods. Theor. Appl. Genet. 112, 1009–1023. doi: 10.1007/s00122-005-0204-z
Vierling, E. (1991). The roles of heat shock proteins in plants. Annu. Rev. Plant Physiol. Plant Mol. Biol. 42, 579–620. doi: 10.1146/annurev.pp.42.060191.003051
Vishwakarma, H., Junaid, A., Manjhi, J., Singh, G. P., Gaikwad, K., and Padaria, J. C. (2018). Heat stress transcripts, differential expression, and profiling of heat stress tolerant gene TaHsp90 in Indian wheat (Triticum aestivum L.) cv C306. PLoS One 13:e0198293. doi: 10.1371/journal.pone.0198293
Viswanathan, C., and Khanna-Chopra, R. (2001). Effect of heat stress on grain growth, starch synthesis and protein synthesis in grains of wheat (Triticum aestivum L.) varieties differing in grain weight stability. J. Agron. Crop Sci. 186, 1–7. doi: 10.1046/j.1439-037X.2001.00432.x
Wahid, A., Gelani, S., Ashraf, M., and Foolad, M. R. (2007). Heat tolerance in plants: an overview. Environ. Exp. Bot. 61, 199–223. doi: 10.1016/j.envexpbot.2007.05.011
Walter, S., Ali, S., Kemen, E., Nazari, K., Bahri, B. A., Enjalbert, J., et al. (2016). Molecular markers for tracking the origin and worldwide distribution of invasive strains of Puccinia striiformis. Ecol. Evol. 6, 2790–2804. doi: 10.1002/ece3.2069
Wang, X., Guan, P., Xin, M., Wang, Y., Chen, X., Zhao, A., et al. (2021). Genome-wide association study identifies QTL for thousand grain weight in winter wheat under normal-and late-sown stressed environments. Theor. Appl. Genet. 134, 143–157. doi: 10.1007/S00122-020-03687-W/METRICS
Wang, B., Huang, J., Zhang, M., Wang, Y., Wang, H., Ma, Y., et al. (2020). Carbon dots enable efficient delivery of functional DNA in plants. ACS Appl. Bio Mater. 3, 8857–8864. doi: 10.1021/acsabm.0c01170
Wang, W., Pan, Q., He, F., Akhunova, A., Chao, S., Trick, H., et al. (2018). Transgenerational CRISPR-Cas9 activity facilitates multiplex gene editing in allopolyploid wheat. Cris. J. 1, 65–74. doi: 10.1089/crispr.2017.0010
Wang, W., Vinocur, B., Shoseyov, O., and Altman, A. (2004). Role of plant heat-shock proteins and molecular chaperones in the abiotic stress response. Trends Plant Sci. 9, 244–252. doi: 10.1016/j.tplants.2004.03.006
Wang, X., Xu, Y., Hu, Z., and Xu, C. (2018). Genomic selection methods for crop improvement: current status and prospects. Crop J. 6, 330–340. doi: 10.1016/j.cj.2018.03.001
Wardlaw, I., and Wrigley, C. (1994). Heat tolerance in temperate cereals: an overview. Funct. Plant Biol. 21:695. doi: 10.1071/pp9940695
West, J. S., Townsend, J. A., Stevens, M., and Fitt, B. D. L. (2012). Comparative biology of different plant pathogens to estimate effects of climate change on crop diseases in Europe. Eur. J. Plant Pathol. 133, 315–331. doi: 10.1007/s10658-011-9932-x
Wheeler, T. R., Batts, G. R., Ellis, R. H., Hadley, P., and Morison, J. I. L. (1996). Growth and yield of winter wheat (Triticum aestivum) crops in response to CO2 and temperature. J. Agric. Sci. 127, 37–48. doi: 10.1017/s0021859600077352
Wollenweber, B., Porter, J. R., and Schellberg, J. (2003). Lack of interaction between extreme high-temperature events at vegetative and reproductive growth stages in wheat. J. Agron. Crop Sci. 189, 142–150. doi: 10.1046/j.1439-037X.2003.00025.x
Xue, G.-P., Drenth, J., and McIntyre, C. L. (2015). TaHsfA6f is a transcriptional activator that regulates a suite of heat stress protection genes in wheat (Triticum aestivum L.) including previously unknown Hsf targets. J. Exp. Bot. 66, 1025–1039. doi: 10.1093/jxb/eru462
Yadav, M. R., Choudhary, M., Singh, J., Lal, M. K., Jha, P. K., Udawat, P., et al. (2022). Impacts, tolerance, adaptation, and mitigation of heat stress on wheat under changing climates. Int. J. Mol. Sci. 23:2838. doi: 10.3390/IJMS23052838
Yale University (2020). World population: 2020 overview. YaleGlobal online. Available at: https://yaleglobal.yale.edu/content/world-population-2020-overview (Accessed September 6, 2020)
Yang, J., Sears, R. G., Gill, B. S., and Paulsen, G. M. (2002). Quantitative and molecular characterization of heat tolerance in hexaploid wheat. Euphytica 126, 275–282. doi: 10.1023/A:1016350509689
Yoshida, R., Hobo, T., Ichimura, K., Mizoguchi, T., Takahashi, F., Aronso, J., et al. (2002). ABA-activated SnRK2 protein kinase is required for dehydration stress signaling in Arabidopsis. Plant Cell Physiol. 43, 1473–1483. doi: 10.1093/pcp/pcf188
Zafar, S. A., Hameed, A., Nawaz, M. A., Ma, W., Noor, M. A., Hussain, M., et al. (2018). Mechanisms and molecular approaches for heat tolerance in rice (Oryza sativa L.) under climate change scenario. J. Integr. Agric. 17, 726–738. doi: 10.1016/S2095-3119(17)61718-0
Zhai, H., Jiang, C., Zhao, Y., Yang, S., Li, Y., Yan, K., et al. (2021). Wheat heat tolerance is impaired by heightened deletions in the distal end of 4AL chromosomal arm. Plant Biotechnol. J. 19, 1038–1051. doi: 10.1111/pbi.13529
Zhang, L., Geng, X., Zhang, H., Zhou, C., Zhao, A., Wang, F., et al. (2017). Isolation and characterization of heat-responsive gene TaGASR1 from wheat (Triticum aestivum L.). J. Plant Biol. 60, 57–65. doi: 10.1007/s12374-016-0484-7
Zhang, Z., Hua, L., Gupta, A., Tricoli, D., Edwards, K. J., Yang, B., et al. (2019). Development of an Agrobacterium-delivered CRISPR/Cas9 system for wheat genome editing. Plant Biotechnol. J. 17, 1623–1635. doi: 10.1111/PBI.13088
Zhang, Y., Liang, Z., Zong, Y., Wang, Y., Liu, J., Chen, K., et al. (2016). Efficient and transgene-free genome editing in wheat through transient expression of CRISPR/Cas9 DNA or RNA. Nat. Commun. 7:12617. doi: 10.1038/ncomms12617
Zhang, R., Liu, J., Chai, Z., Chen, S., Bai, Y., Zong, Y., et al. (2019). Generation of herbicide tolerance traits and a new selectable marker in wheat using base editing. Nat. Plants 5, 480–485. doi: 10.1038/s41477-019-0405-0
Zhang, Y., Massel, K., Godwin, I. D., and Gao, C. (2018). Applications and potential of genome editing in crop improvement. Genome Biol. 19:210. doi: 10.1186/s13059-018-1586-y
Zhang, H., Zhang, J., Lang, Z., Botella, J. R., and Zhu, J.-K. (2017). Genome editing—principles and applications for functional genomics research and crop improvement. CRC. Crit. Rev. Plant Sci. 36, 291–309. doi: 10.1080/07352689.2017.1402989
Zhao, C., Liu, B., Piao, S., Wang, X., Lobell, D. B., Huang, Y., et al. (2017). Temperature increase reduces global yields of major crops in four independent estimates. Proc. Natl. Acad. Sci. U. S. A. 114, 9326–9331. doi: 10.1073/pnas.1701762114
Keywords: wheat, heat stress, changing climate, food security, current era
Citation: Farhad M, Kumar U, Tomar V, Bhati PK, Krishnan J. N, Kishowar-E-Mustarin, Barek V, Brestic M and Hossain A (2023) Heat stress in wheat: a global challenge to feed billions in the current era of the changing climate. Front. Sustain. Food Syst. 7:1203721. doi: 10.3389/fsufs.2023.1203721
Edited by:
Taku Tsusaka, Ostrom, ThailandReviewed by:
Ritesh Kumar, Boyce Thompson Institute (BTI), United StatesAalok Shiv, Indian Institute of Sugarcane Research (ICAR), India
Rajib Roychowdhury, Agricultural Research Organization, Volcani Center, Israel
Copyright © 2023 Farhad, Kumar, Tomar, Bhati, Krishnan J., Kishowar-E-Mustarin, Barek, Brestic and Hossain. This is an open-access article distributed under the terms of the Creative Commons Attribution License (CC BY). The use, distribution or reproduction in other forums is permitted, provided the original author(s) and the copyright owner(s) are credited and that the original publication in this journal is cited, in accordance with accepted academic practice. No use, distribution or reproduction is permitted which does not comply with these terms.
*Correspondence: Md. Farhad, bWQuZmFyaGFkQGJ3bXJpLmdvdi5iZA==; Akbar Hossain, YWtiYXJob3NzYWlud3JjQGdtYWlsLmNvbQ==