- 1College of Agriculture, Northeast Agricultural University, Harbin, China
- 2Heilongjiang Agricultural Engineering Vocational College, Harbin, China
- 3Lab of Functional Genomics and Bioinformatics, Institute of Crop Science, Chinese Academy of Agricultural Sciences, Beijing, China
Soybean is an important grain and oil crop cultivated worldwide. Mepiquat chloride (DPC), a plant growth regulator, is widely used in cotton planting; however, its application in soybean remains rarely studied. Herein, we explored DPC regulation in soybeans using morphology, physiology, and proteomics analyses. Morphological and physiological analyses showed that DPC significantly reduced the plant height and shoot dry weight, promoted the growth of lateral roots in a specific concentration range, increased the activities of the leaf protective enzymes (superoxide dismutase and catalase), and decreased and increased malondialdehyde and total flavonoid contents, respectively. Proteomic analysis of leaves treated with 100 mg/L DPC revealed that multiple proteins related to plant growth and stress resistance were regulated after treatment. The key proteins involved in photosynthesis and cell wall elongation in the two varieties of soybean were significantly downregulated and those related to promoting lateral root growth and stress resistance were significantly upregulated. Overall, DPC inhibited shoot growth and photosynthesis in soybean but promoted lateral root growth, enhanced protective enzyme activity, and improved the ability of plants to resist abiotic stress. This study preliminarily determined the suitable DPC concentration for soybean spraying and provided a theoretical basis for its rational application.
1. Introduction
Soybean (Glycine max (Linn.) Merr.), an important food and economic crop cultivated worldwide (Manan et al., 2017) has a protein content of more than 40% and provides eight essential amino acids that cannot be synthesized by the human body (Li et al., 2018; Zhang et al., 2020). In China, one of the earliest countries to plant soybeans, the development of the soybean cultivation industry has been restricted owing to planting risks and benefits (Mallory et al., 2015). China’s soybean demand is expected to reach 112.92 million tons in 2021, of which 85.48% will come from imports. Under the double cycle era, it is crucial to improve soybean productivity, strengthen domestic self-sufficiency, and guarantee soybean supply.
Mepiquat chloride (1,1-dimethylpiperidium chloride, DPC) is a white, odorless, stable chemical compound. As a new type of plant growth regulator, it can be absorbed by leaves and quickly transmitted to other parts of the plant, with the advantages of low toxicity and residue (MertoĞLu et al., 2019). Cell elongation is hampered by inhibiting the biosynthesis of gibberellin in plants (Wang et al., 2014), which shortens internodes and renders a compact plant type. Mepiquat chloride, which balances vegetative and reproductive growth and promotes lateral root growth, was first applied to cotton (Chen et al., 2020). In recent years, DPC has been applied to crops such as corn (Kamran et al., 2018), sunflower (Tomáš et al., 2018), and castor (Souza-Schlick et al., 2018). It has achieved good results in regulating plant morphology; however, systematic studies on the application of DPC in soybeans remain limited. Previously, we explored the regulatory mechanism of DPC in soybean growth at transcriptional and metabolic levels and found that at the transcriptional level, the number of downregulated genes was higher than that of the upregulated genes. The downregulated genes were mainly related to photosynthesis and cell wall elongation, which led to the inhibition of soybean growth. Metabolomics analysis found that approximately 60% of the differential metabolites were related to the synthesis of flavonoids, and the content was predominantly upregulated. As an essential secondary metabolite in plants, flavonoids can help plants resist the damage caused by pests and diseases and promote plants’ metabolic activities. Moreover, several studies have shown that flavonoids can enhance the ability of plants to resist adversity (Song et al., 2016; Waseem et al., 2019; Benitez et al., 2021).
Soybeans are highly susceptible to different stresses during growth (Hossain et al., 2013), such as drought and saline-alkali stress. Zou et al. (2020) showed that different degrees of drought reduced soybean yield by 20%–50%. Plants have developed a series of mechanisms to resist long-term adversity. As an important vegetative organ of plants, the root system helps plants absorb nutrients and water they need from the soil. Good root status is the basis for high and stable yield of soybean. Recent studies have shown that DPC can enhance the ability of soybean to resist adversity by increasing the activity of antioxidant enzymes (Wang et al., 2022a). Luo et al. (2010) showed that the use of DPC treatment enhanced the cold resistance of sweet pepper seedlings, which was manifested by the enhancement of superoxide dismutase and catalase activities, effectively removing excess reactive oxygen species in the body, thereby reducing the damage caused by stress. Ashrafi et al. (2015) showed that the malondialdehyde (MDA) content was maintained at a low level under good growth conditions but markedly increased when the plant was subjected to stress.
Photosynthesis is a fundamental process in plant growth and development. Currently, research on the DPC regulation of plant photosynthesis is mainly limited to the physiological level, with limited studies at the molecular level. The results of previous studies remain controversial in that some studies have shown that DPC enhances plant photosynthesis (Gonias et al., 2012) while others that it inhibits photosynthesis (Tung et al., 2018). Proteomics, a core component of scientific research in the post-genomic era, can compare differences in protein expression in plants under different conditions. Therefore, proteomic analysis is helpful to effectively assess the effect of DPC on the soybean photosynthetic system.
As the executors of plant physiological functions, proteins can respond to changes in the external environment. Therefore, in this study, we used the Heinong44 (HN44) and Heinong65 (HN65) soybean varieties as experimental materials. The DPC solution was sprayed at the seedling stage to explore the growth status of soybean shoots and roots through morphological and physiological experiments, and the appropriate concentration of DPC was screened. Simultaneously, tandem mass tag-quantitative proteomics analysis was used to identify differentially expressed proteins, and bioinformatics analysis was used to explore changes in the soybean leaf proteome after DPC treatment. Our study is essential for understanding the mechanism by which DPC regulates soybean growth, and these findings will provide a theoretical basis for the rational application of DPC in soybeans.
2. Materials and methods
2.1. Plant materials and treatments
Soybean varieties HN44 and HN65 were obtained from the Heilongjiang Academy of Agricultural Sciences, DPC was from Hebei Guoxin Nuonong Biotechnology company. The test treatment was done using the sand culture method. A barrel with a diameter of 0.30 m and a height of 0.35 m was used. The bottom of the barrel had three 1 cm diameter holes. Seeds of the same size, with no pests or diseases, were selected, and eight seeds were sowed in each pot, after the emergence of each pot to protect three seedlings. Before the true leaves were completely unfolded, the plant was watered once a day with 500 mL water, and after spreading the leaves, 500 mL Hoagland nutrient solution was supplied. When the soybean grew to the V3 stage, the DPC solution at concentrations of 50, 100, 200, and 400 mg/L was sprayed evenly on the leaf surface, and the control group was sprayed with water (CK). Subsequently, sampling was performed every 3 days for a total of four times, and at least three biological replicates were maintained for each sampling. Proteomic analysis was performed on day 7 of treatment.
2.2. Determination of morphological indexes
Soybean plants sprayed with 50, 100, 200, and 400 mg/L DPC were sampled on days 3, 6, 9, and 12. The total root length and number of root tips were measured using a plant root scanner (RHIZOScan).
2.3. Determination of physiological indicators
The absorbance at 560 nm was measured using the nitrogen blue tetrazolium method, and the superoxide dismutase (SOD) activity was calculated (Wang et al., 2022b). Fresh leaves were taken out in an ultralow temperature refrigerator at −80°C. 0.1 g fresh leaves were weighed and placed in a precooling bowl. 1 mL precooling extraction medium was added to grind the homogenate on the ice bath. After centrifugation at 4°C and 10,000 rpm for 15 min, the supernatant was the crude extract of superoxide dismutase (SOD). Adding 0.05 mol/L PBS, (PH7.8) 1.5 mL, 130 mM methionine (Met) 0.3 mL, NBT 0.3 mL, EDTA-Na2 0.3 mL, riboflavin 0.3 mL SOD extract 0.1 mL added distilled water 0.5 mL in transparent glass tube. The test tube was placed under 4,000 LX fluorescent lamp for photochemical reaction for 20 min, and the temperature was controlled between 25°C and 35°C. Cover the tube with a cloth mask to terminate the reaction. Control tube in dark disposal zero, with distilled water instead of enzyme solution as a control. The absorbance of the reaction solution was measured at 560 nm.
Notes: the total SOD activity was expressed as fresh weight enzyme unit per gram (u/g FW); A0, light absorbance of the tube; A, sample tube absorbance; V, sample total volume (3.3 mL); Vt, determination of enzyme dosage (0.1 mL); W, sample fresh weight; the protein content unit is mg/g.
Furthermore, the guaiacol colorimetric method was used to measure the absorbance at 470 nm to calculate the catalase (POD) activity (Wang and Huang, 2015). The preparation of crude enzyme solution was the same as SOD, reagent preparation: 0.2 mol/L phosphate buffer (pH 6.0): A mother liquor (Na2HPO4) 123 mL and B mother liquor (NaH2PO4) 877 mL were mixed. 1,000 mL PBS (0.2 M, pH 6.0); Preparation of reaction mixture: 200 mL PBS (0.2 M, pH 6.0), 0.076 mL guaiacol (2-Methoxyphenol) was dissolved by heating and stirring, and 0.112 mL 30% H2O2 was added after cooling. After mixing, it was stored in the refrigerator for further use sample determination: take 3 mL reaction solution and add 30 μL enzyme solution, PBS as control, and then determine the OD470 value (measured for 40 s).
Notes: where ΔA470, the change of absorbance in reaction time; W, is sample fresh weight (g); T, is the reaction time (min); Vt, is the total volume of the reaction solution (3.3 mL); V, enzymatic liquid volume (0.03 mL) for determination.
The absorbance at 510 nm was measured using the NaNO2-Al (NO3)3-NaOH colorimetric method to calculate total flavonoid content (Kedrina-Okutan et al., 2019). Take 0.1 g of fresh leaves, grind and add 1.5 mL 60% ethanol, shake at 60°C for two hours. Centrifuged at 25°C, 12,000 rpm for 10 min, and the supernatant was taken for testing.
Notes: ΔA, A−ACK; W, is sample fresh weight (g); V1, the volume of the sample in the reaction; V, volume of the extract.
Moreover, the absorbance at 532 and 600 nm was measured using the thiobarbituric acid method to calculate MDA content (Li, 2010). Take 0.1 g fresh leaves, add 0.1 mL prepared extract, grind into homogenate, centrifuge at 4°C × 12,000 rpm for 10 min, and take the supernatant to be tested.
2.4. Proteomics sample processing
Lysate (1.5% SDS/100 mM Tris-Cl) was added to the sample, the supernatant was centrifuged after complete grinding, and protein precipitation was obtained by acetone precipitation. After re-dissolution with 8 M urea/100 mM Tris-Cl, Dithiothreitol (DTT) was added and incubated at 37°C for 1 h. Subsequently, iodoacetamide (IAA) was added, and the alkylation reaction was performed at room temperature and dark to block the thiol group,the protein concentration was determined using the Bradford method. Simultaneously, the same amount of sample was labeled with tandem mass tag. After the labeled samples were mixed in equal amounts, Sep-Pak C18 was used for desalination. After vacuum drying, the mixed samples were separated using high-pH reversed-phase chromatography and stored at −80°C. Preparing for machine testing.
2.5. Mass spectrometry detection
Mass spectrometry data were collected using an Orbitrap Exploris 480 mass spectrometer in series with an EASY-nLC 1,200 liquid phase liquid chromatography-mass spectrometry system. The peptide sample was dissolved in a loading buffer, inhaled by the autosampler, and combined with an analytical column (75 μm × 25 cm, C18, 19 μm, 100 Å) for separation. An analytical gradient was established using two mobile phases (mobile phase A: 0.1% formic acid and mobile phase B: 0.1% formic acid, 80% ACN). The flow rate of the liquid phase was set to 300 nL/min. Mass spectrometry data were collected in DDA mode, and each scan cycle contained one MS full scan (R = 60 K, AGC = 300%, max IT = 20 ms, scan range = 350–1,500 m/z) and 20 subsequent MS/MS scans (R = 15 K, AGC = 100%, max IT = auto, cycle time = 2 s). The higher energy collision dissociation was set to 35%. The screening window of the quadrupole was set to 1.2 Da, and the dynamic exclusion time for repeated ion collection was set to 35 s.
2.6. Data analysis
Mass spectrometry data were retrieved using MaxQuant (V1.6.6) software, and the data retrieval algorithm was Andromeda. The UniProt. Glycine_max.20190126. Fasta Proteome Reference Database was used for retrieval. The screening conditions for differential proteins were p < 0.05 and Fold Change <0.83 or > 1.2. Combining the literature and UniProt1 to determine the biological functions of the differentially expressed proteins, proteins were classified according to Gene Ontology (GO) annotation and Kyoto Encyclopedia of Genes and Genomes (KEGG) metabolic pathways.2 The STRING online database3 and Cytoscape v3.8.2 software were used for correlation construction. Cytoscape MCODE and CytoNCA plug-ins were used to search for cluster subnetworks and perform differential protein analysis. SPSS was used for data analysis, and Origin2021 was used for plotting.
3. Result
3.1. Effects of different concentrations of DPC on morphological indexes of soybean
Plant height and shoot dry weight of soybean decreased with increasing spraying concentration. The total root length and root tip number first increased, reached a maximum at 100 mg/L, and then decreased with the increase in spraying concentration. After 12 days of treatment with 100 mg/L DPC, the plant height of HN44 and HN65 decreased by 9.38% and 7.01%, respectively. In addition, the dry weight of above-ground parts decreased by 11.78% and 10.21%, respectively; the number of root tips increased by 32.07% and 38.38%, respectively; and the total root length increased by 30.72% and 33.36%, respectively. In summary, the above-ground changes in HN44 after DPC treatment were higher than those in HN65, and DPC promoted the root growth of HN65 (Figure 1).
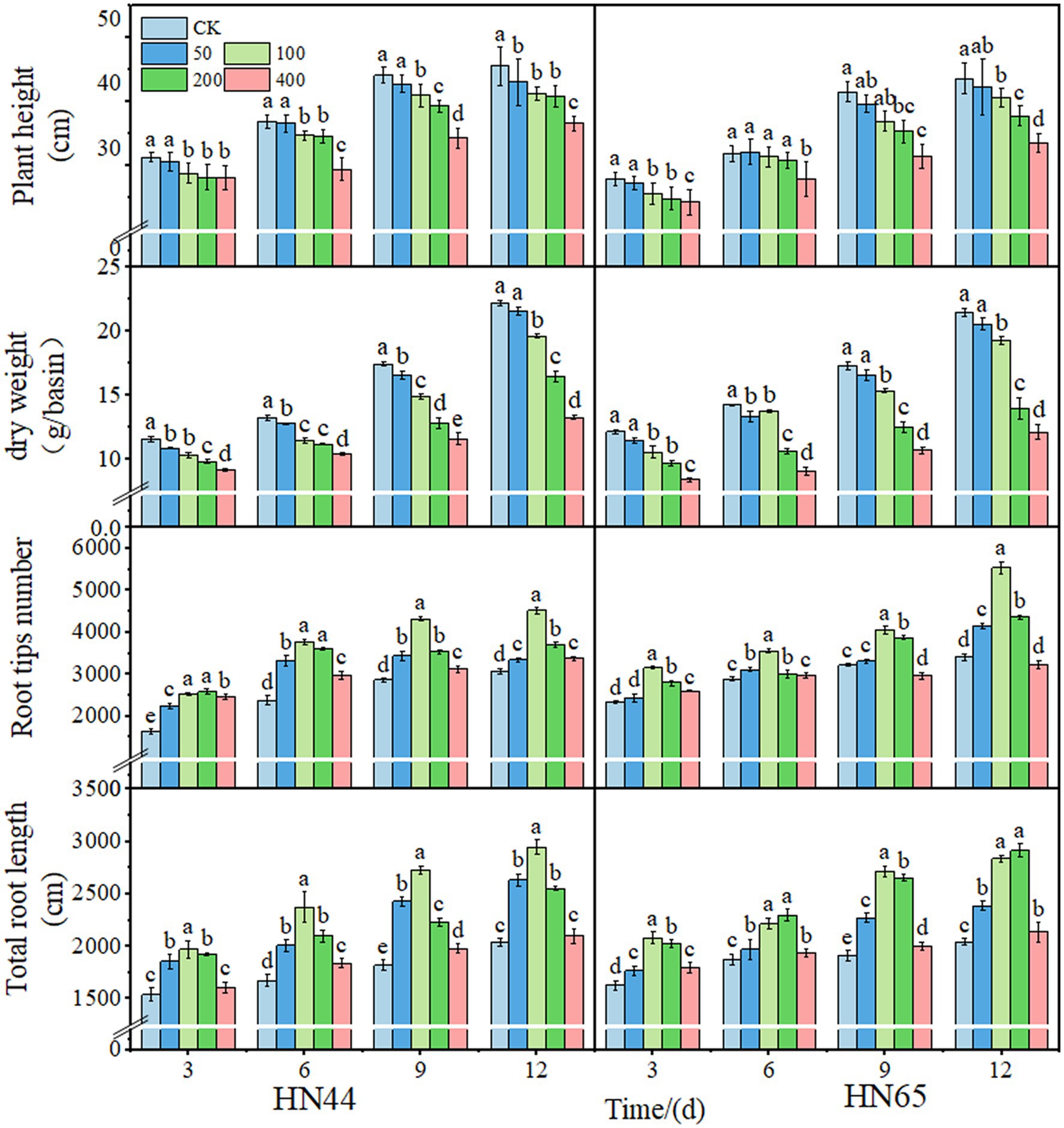
Figure 1. Effects of different concentrations of mepiquat chloride (DPC) on morphological indexes of two soybean varieties (HN44 and HN65). CK indicates the control group.
3.2. Effects of different concentrations of DPC on physiological indexes of soybean
With increased DPC concentration, SOD, POD activity, and total flavonoid content increased and decreased. Generally, the protective enzyme activity of the two soybean varieties reached a maximum at the 100 mg/L DPC treatment. Compared to those of the CK, after DPC treatment, the SOD activities of HN44 and HN65 increased by 15.26% and 27.27%, respectively. The POD activity reached a maximum at nine days after 100 mg/L DPC treatment. Moreover, the POD activities of HN44 and HN65 increased by 53.52% and 39.85%, respectively, after DPC treatment compared to those of CK. The total flavonoid content reached a maximum at 9 days after 100 mg/L DPC treatment and increased by 41.80% and 31.61% in HN44 and HN65, respectively, compared to that in CK. With an increase in DPC concentration, MDA content decreased and then increased and was the lowest at the 100 mg/L DPC treatment in the two soybean varieties. The MDA content of HN44 and HN65 decreased by 27.34% and 21.18%, respectively, compared with that of CK (Figure 2).
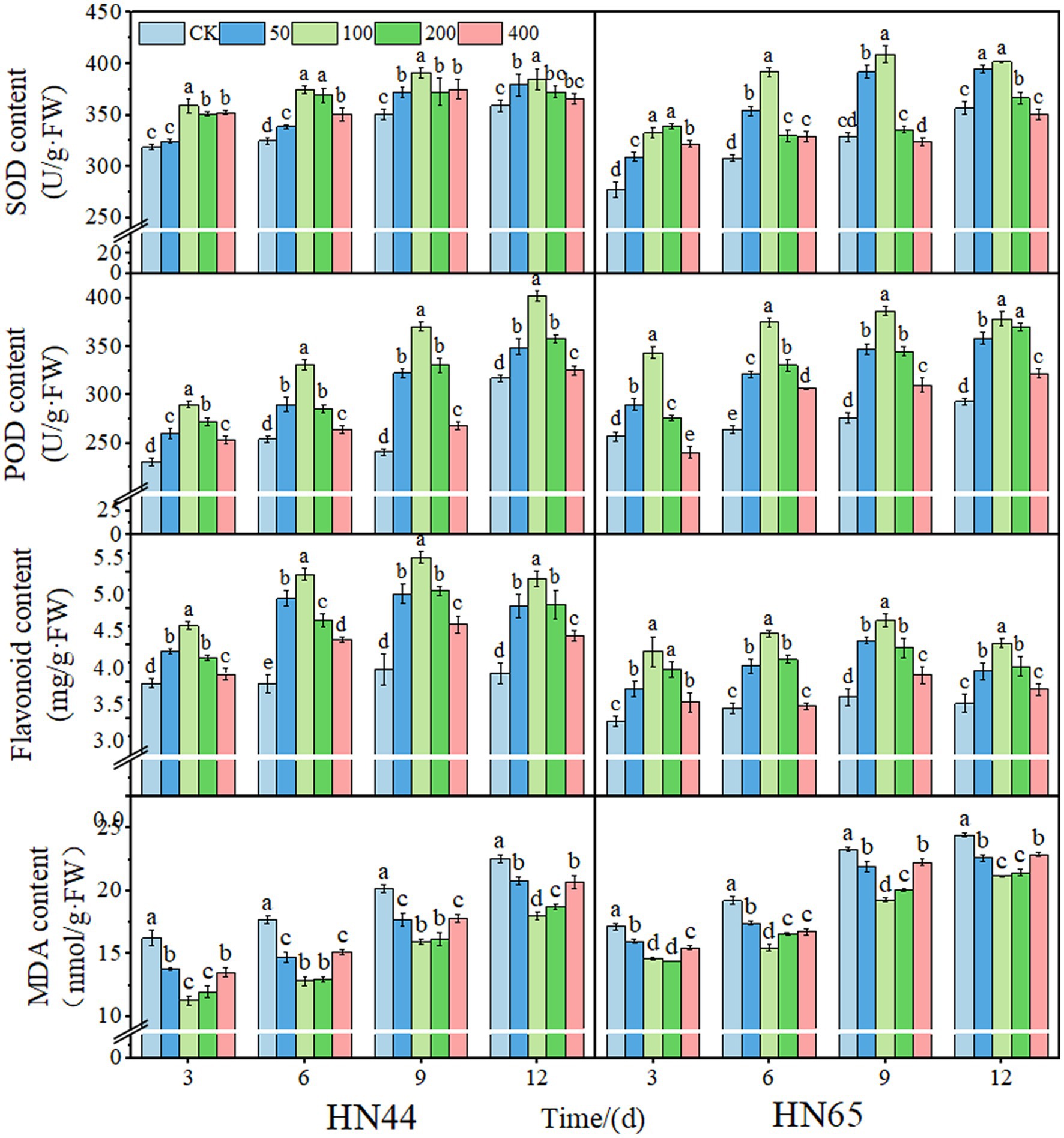
Figure 2. Effects of different concentrations of mepiquat chloride (DPC) on the physiological activity of soybean leaves of two varieties (HN44 and HN65). SOD, superoxide dismutase; POD, catalase; MDA, malondialdehyde.
3.3. Quantitative proteomics results
Based on the results of previous morphological and physiological indicators, we selected 100 mg/L DPC-treated soybean leaves for proteomic analysis to further explore the regulatory mechanism of DPC in soybean. A total of 10,223 proteins were detected in soybean leaves. Based on the difference threshold, 55 and 74 differentially expressed proteins were identified in the HN44 and HN65 soybean varieties, respectively. HN44 included 19 upregulated and 26 downregulated proteins, and HN65 included 51 upregulated and 23 downregulated proteins (Figure 3A). The distribution of differentially expressed proteins between the two treatment pairs was visualized using a Venn diagram. A total of nine differentially expressed proteins were found between the two groups simultaneously (Figure 3B). These nine expressed proteins were mainly related to flavonoid synthesis, antioxidant enzyme regulation, and photosynthesis and may be the response proteins of DPC in soybean. The cellular localization of differentially expressed proteins in the two varieties of soybean is shown in Figures 3C,D. Most of the differentially expressed proteins involved four parts: chloroplast, cytoplasm, nucleus, and extracellular matrix.
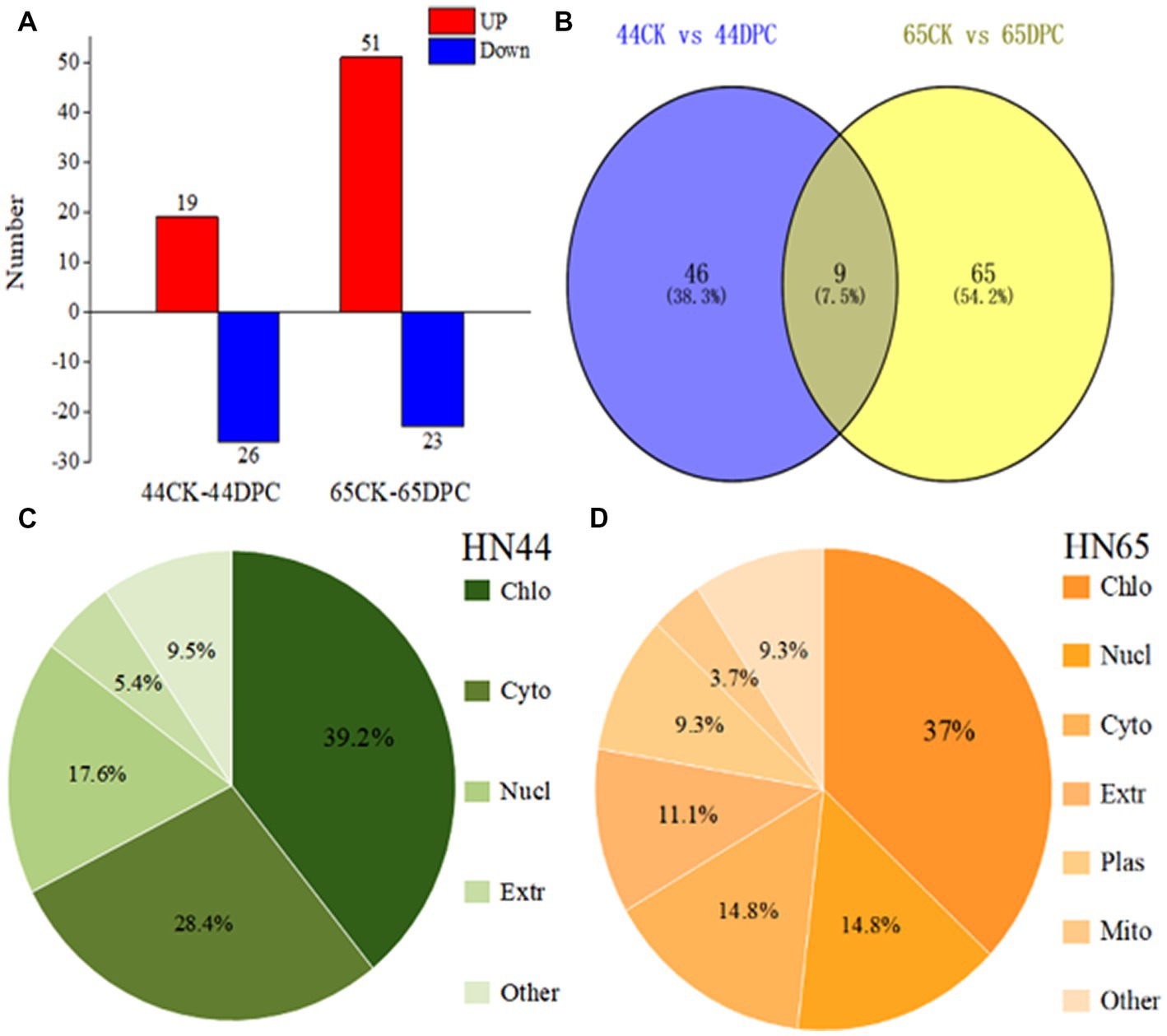
Figure 3. Proteome analysis of two soybean varieties (HN44 and HN65) after mepiquat chloride (DPC) treatment. (A) The number of upregulated (red) and downregulated (blue) proteins after HN44 and HN65 soybean varieties were treated; (B) Venn diagram of HN44 and HN65 differential proteins; (C) Subcellular localization of HN44 differential protein; (D) Subcellular localization of HN65 differential protein.
3.4. Go annotation analysis of differential proteins
GO annotation was performed on 55 and 74 differentially expressed proteins in HN44 and HN65, respectively, and the proteins were divided into three parts: biological process (BP), cellular component (CC), and molecular function (MF).
The GO enrichment of differential proteins after spraying HN44 variety with DPC is shown in Figure 4. In the BP process, differential proteins are mainly involved in the photosynthetic process and cell wall tissues; both are downregulated proteins. In the CC process, the differentially expressed proteins were mainly enriched in five parts: the thylakoid membrane, cell membrane, chloroplast, cell wall, and nucleus, among which the differentially expressed proteins involved in the cell membrane and nucleus regulation were mainly positively regulated. In the MF process, most differentially expressed proteins were related to oxidoreductase activity, copper ion binding, and electron carrier activity. Figure 5 shows the GO enrichment of the differentially expressed proteins after spraying DPC onto HN65. In the BP process, the differential proteins were mainly involved in photosynthetic processes, protein-chromosome connection processes, lipid biosynthesis, redox, and carbohydrate metabolism processes. The first two processes are generally downregulated, and the last three are upregulated proteins. In the CC process, the enrichment position of the differential proteins was consistent with that of HN44. In the MF process, differential proteins were enriched in metal ion binding, organic binding, 4 iron, 4 sulfur cluster binding, FMN binding, and oxidoreductase activity.
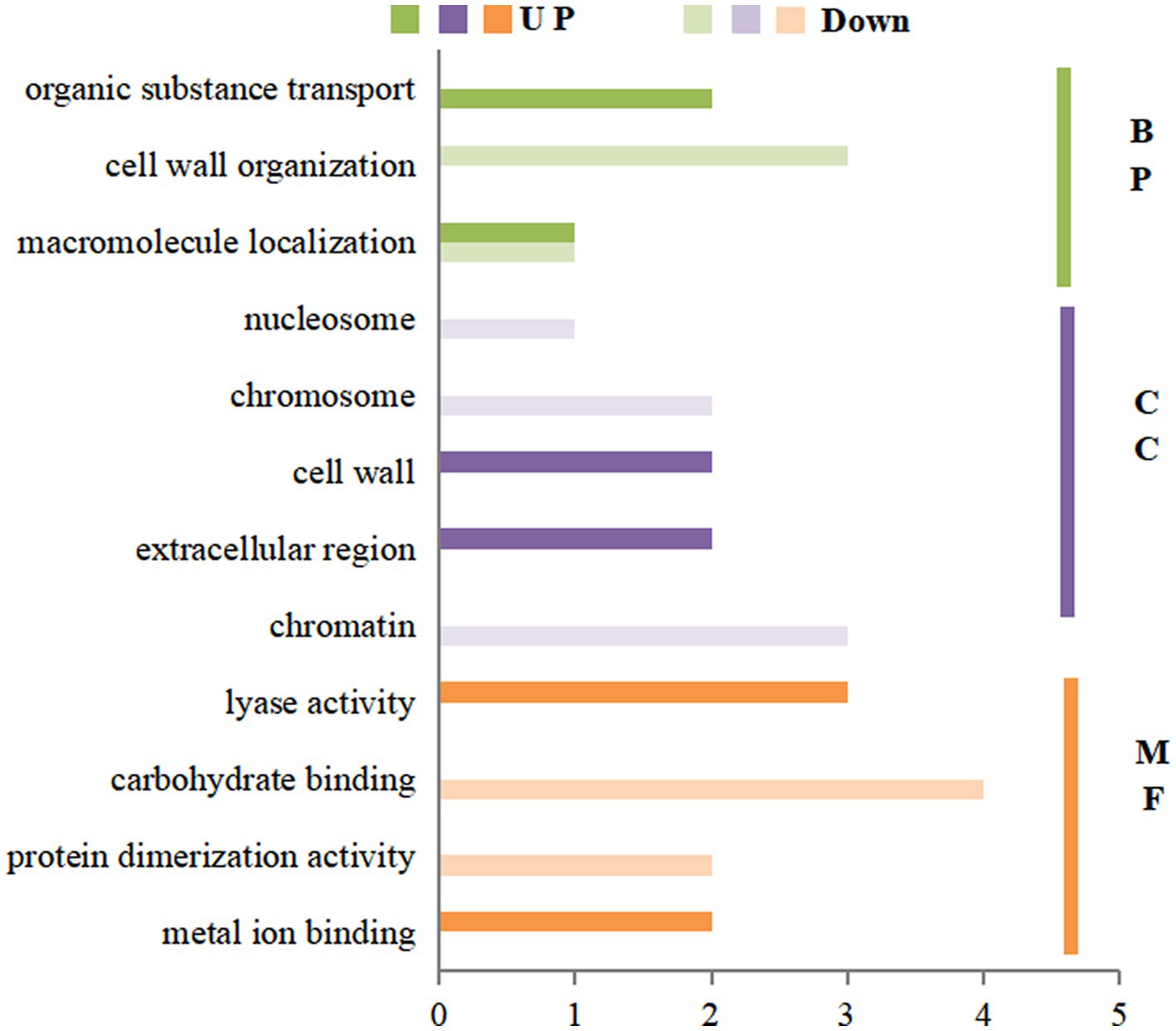
Figure 4. Gene ontology (GO) annotation analysis of differential proteins after spraying mepiquat chloride (DPC) on the HN44 soybean variety. BP, biological process; CC, cellular component; MF, molecular function.
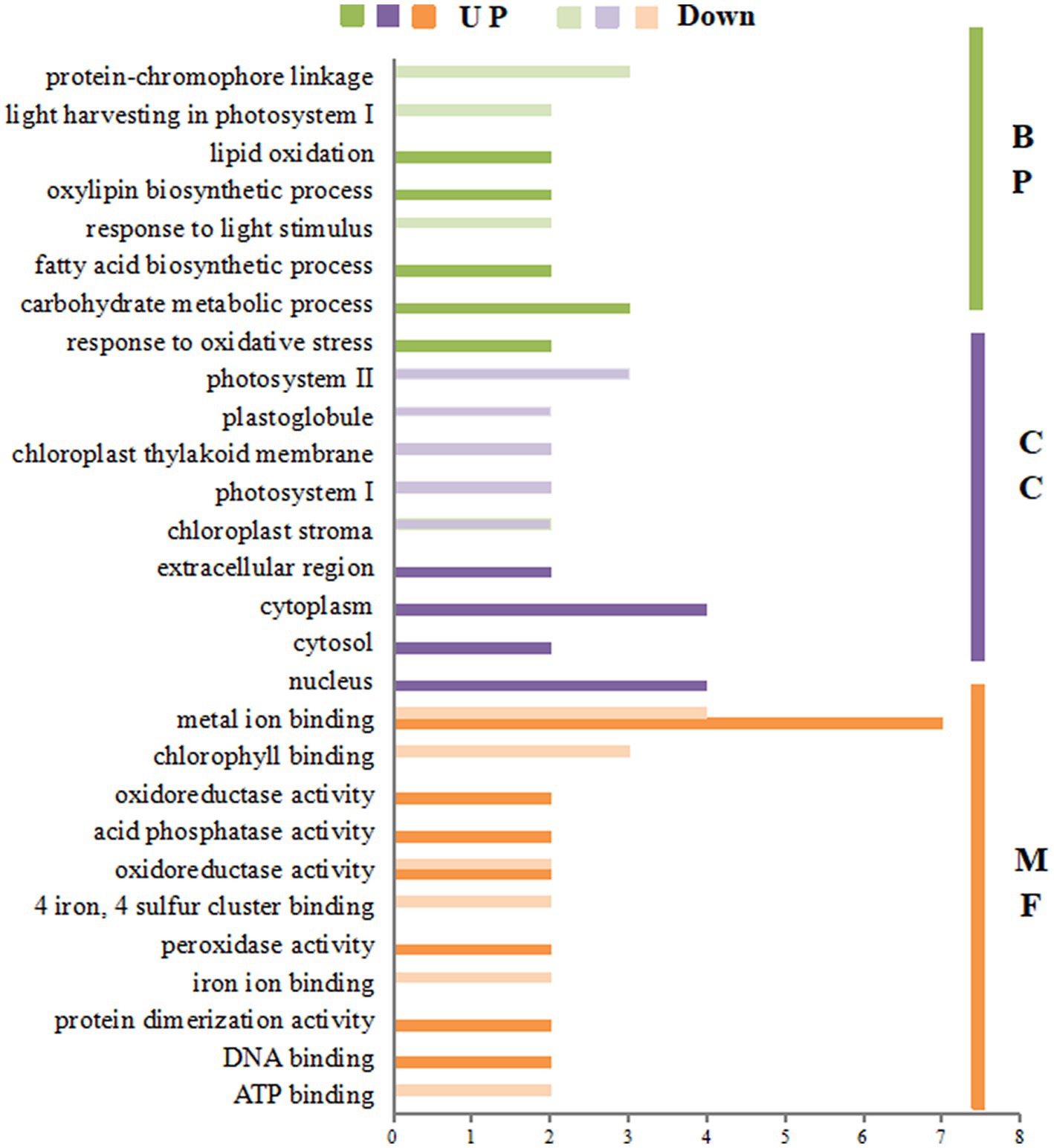
Figure 5. Gene ontology (GO) annotation analysis of differential proteins after spraying mepiquat chloride (DPC) on the HN65 soybean variety. BP, biological process; CC, cellular component; MF, molecular function.
The changes in protein levels in HN44 and HN65 varieties were not the same after DPC treatment. Similarly, the proteins related to the nucleus and whole cell membrane components of HN44 and HN65 were significantly upregulated after treatment. However, the photosynthesis of both varieties was inhibited, and the differential proteins related to chloroplasts were significantly reduced. However, we found that HN65 was more sensitive to DPC than HN44, and more proteins were affected in vivo, mainly reflected in the enhancement of lipid and carbohydrate synthesis and metabolism and an increase in oxidoreductase activity.
3.5. KEGG enrichment and K-means analysis
KEGG enrichment analysis of differentially expressed proteins indicated that HN44 and HN65 were enriched in three and seven pathways, respectively, as shown in Figure 6. In HN44, the main enriched pathways included metabolic pathways, photosynthesis, and biosynthesis of secondary metabolites. The metabolic pathways involved were mainly the C4-dicarboxylic acid cycle, amino acid biosynthesis, and thiamine. The differential proteins of HN65 were primarily enriched in the biosynthesis of secondary metabolites, metabolic pathways, photosynthesis-antenna proteins, linoleic acid metabolism, nitrogen metabolism, and glutathione metabolism. Among them, the metabolic pathways mainly involved the tricarboxylic acid (TCA) cycle, C4-dicarboxylic acid cycle, amino acid biosynthesis, jasmonic acid, lignin, and flavonoid biosynthesis. It was standardized and centralized to explore further the changing trend of the relative content of differential proteins between different treatments, and subsequently, K-means analysis was performed. Six clusters (K = 6) showed dynamic changes in proteins between treatments (Figure 6).
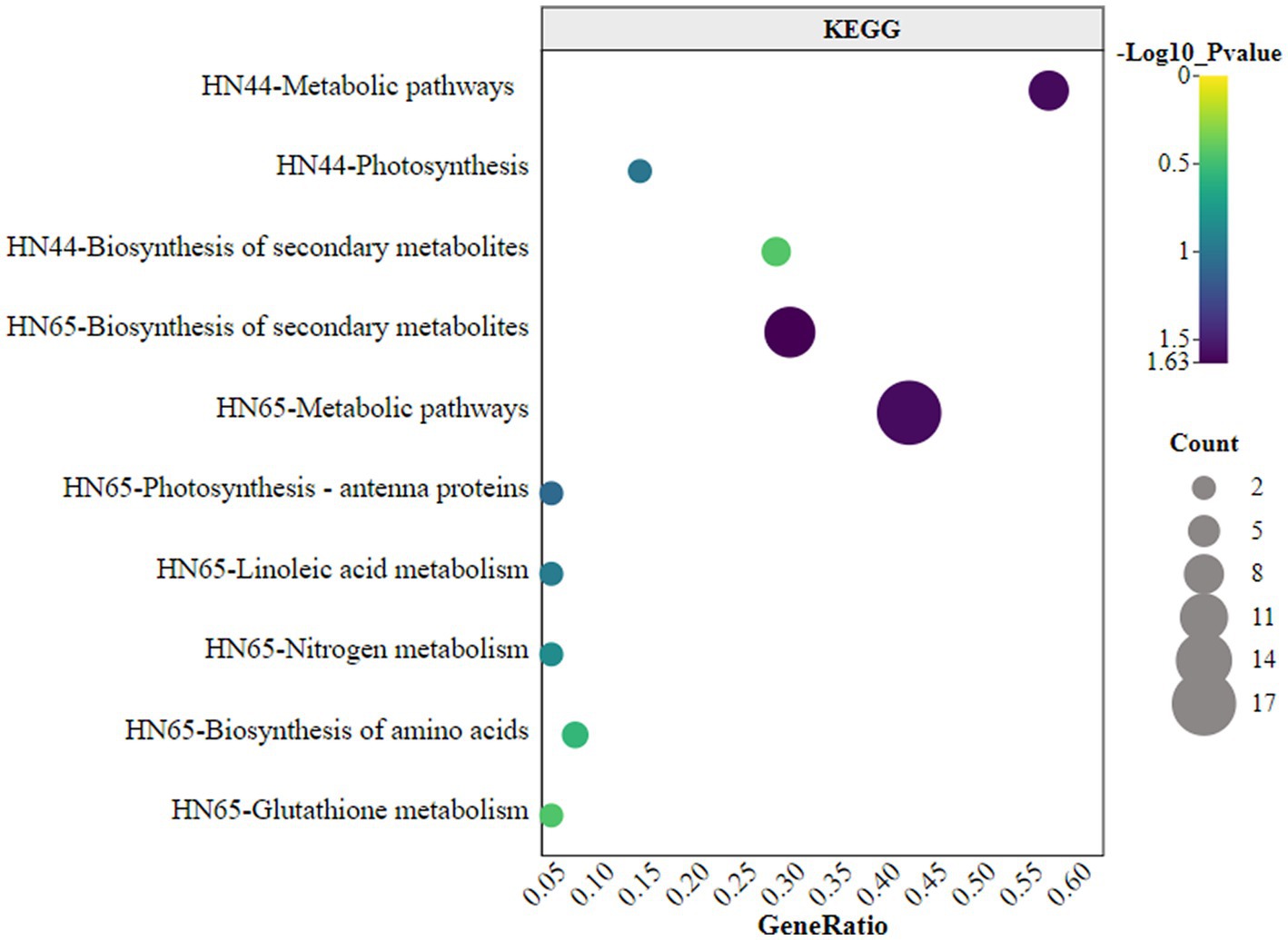
Figure 6. Kyoto Encyclopedia of Genes and Genomes (KEGG) enrichment analysis of differential proteins.
The first group showed the largest number of differentially expressed proteins, and the two varieties showed a decrease in the content of differentially expressed proteins after treatment, followed by the second group, with a low decrease in HN44 and an increase in HN65. The other four groups showed an increasing trend simultaneously; however, some differences in this increase were observed. Based on the K-means clustering analysis, we divided the differential proteins into three categories (simultaneous decrease, simultaneous increase, and one variety increase and another decrease) for KEGG enrichment analysis (Figure 7). K-means analysis showed that most proteins were downregulated in both varieties simultaneously. As shown in Figure 7A, the downregulated proteins were mainly involved in the TCA cycle, photorespiration, C4-dicarboxylic acid cycle, and amino acid metabolism. Figure 7B indicates downregulation in HN44 cells and upregulation in HN65 cells. The remaining four groups of differentially expressed proteins showed different degrees of increase in the two varieties, mainly related to β-glucosidase, lignin synthesis, and starch and sucrose metabolism.
3.6. Differential protein protein–protein interaction (PPI) network annotation
To obtain the network connection between the two varieties of differentially expressed proteins, we imported the data into the STRING database and Cytoscape software to construct its PPI network (Figure 8). For HN44 and HN65, 19 and 18 network nodes and 45 and 34 edges were obtained, respectively. CytoNCA betweenness centrality analysis was performed on nodes. The BC value was proportional to the node size. The larger the node, the higher the betweenness centrality of the protein in the PPI. PPI was analyzed using MCODE, and the cluster with the highest score was divided into HN44 and HN65. The HN44 cluster included eight proteins with a score of 6.286, with C6TBU3 as the seed node, mainly related to transmembrane transport and ATP synthesis. The HN65 cluster included five proteins with a score of 5, with A0A0R0KBX5 as the seed node, mainly related to antioxidant enzyme activity.
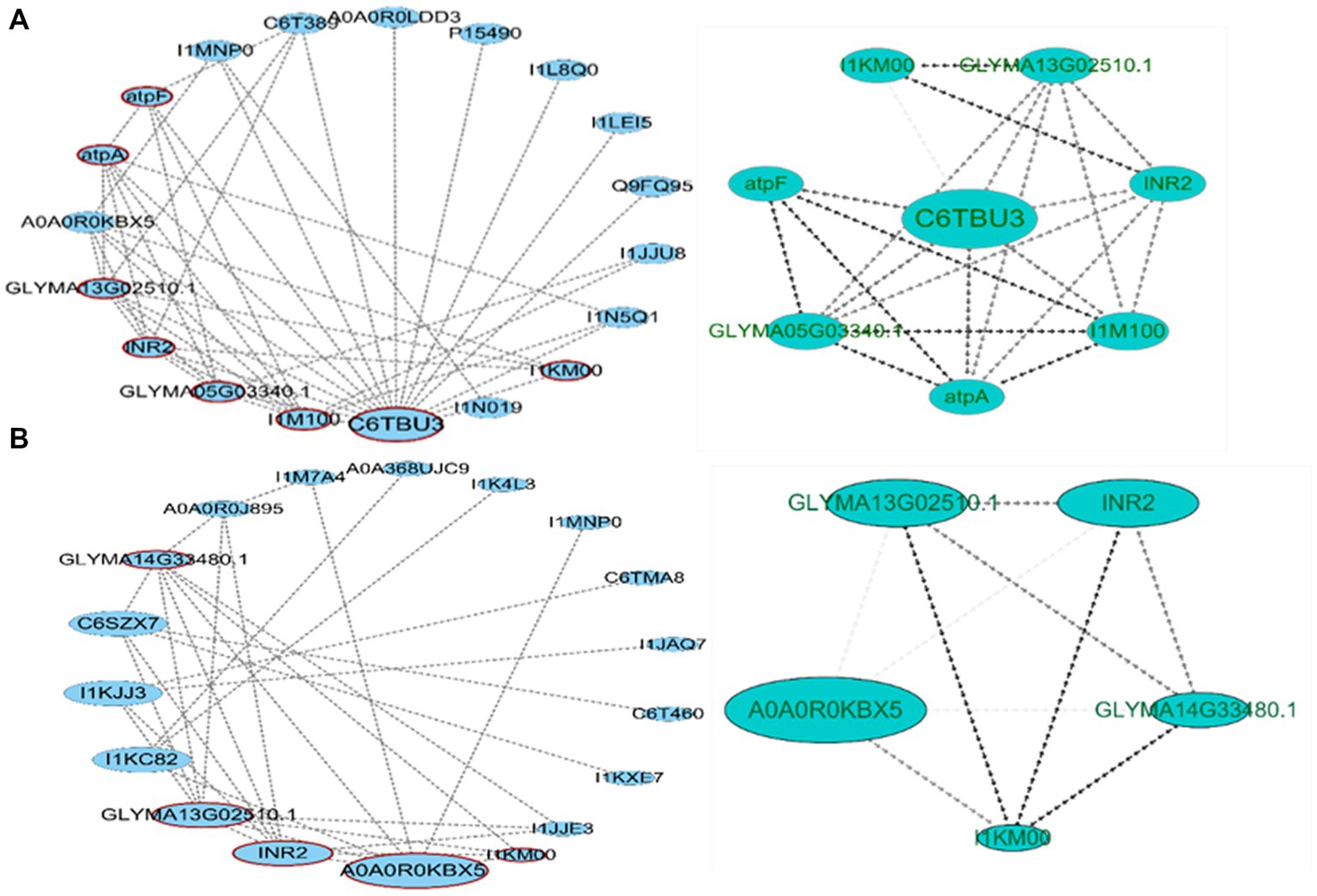
Figure 8. Protein–protein interaction (PPI) network analysis of differential proteins. (A) PPI network construction of HN44 differential proteins and high clustering obtained by MCODE analysis. (B) PPI network construction of HN65 differential proteins and high clustering obtained by MCODE analysis.
3.7. Mechanism analysis of DPC regulating soybean
The previous experimental results (Wang et al., 2022a) were combined with the physiology and proteomics results of this experiment to explore the regulation of soybean growth by DPC (Figure 9). Approximately 70% of the differentially expressed genes were downregulated at the transcriptional level, manifested by inhibiting the photosynthetic system and cell wall elongation. We believe that this is an important reason why DPC inhibited soybean growth. Approximately 75% of the differential metabolites, mainly flavonoids, lignans, and coumarins, at the metabolic level were upregulated. Among them, flavonoids accounted for more than half of the upregulated differential metabolites, indicating that DPC can improve the ability of soybean to resist adversity by enhancing the content of secondary metabolites in the body. At the protein level, we found that the protein xyloglucan endotransglucosidase and pectin acetyl esterase were significantly downregulated, inhibiting the cell wall’s elongation. In addition, proteins related to the photosystem and the electron transfer of the b6-f complex were significantly downregulated, which inhibited photosynthesis, but at the same time, several proteins related to root growth and stress resistance were significantly upregulated.
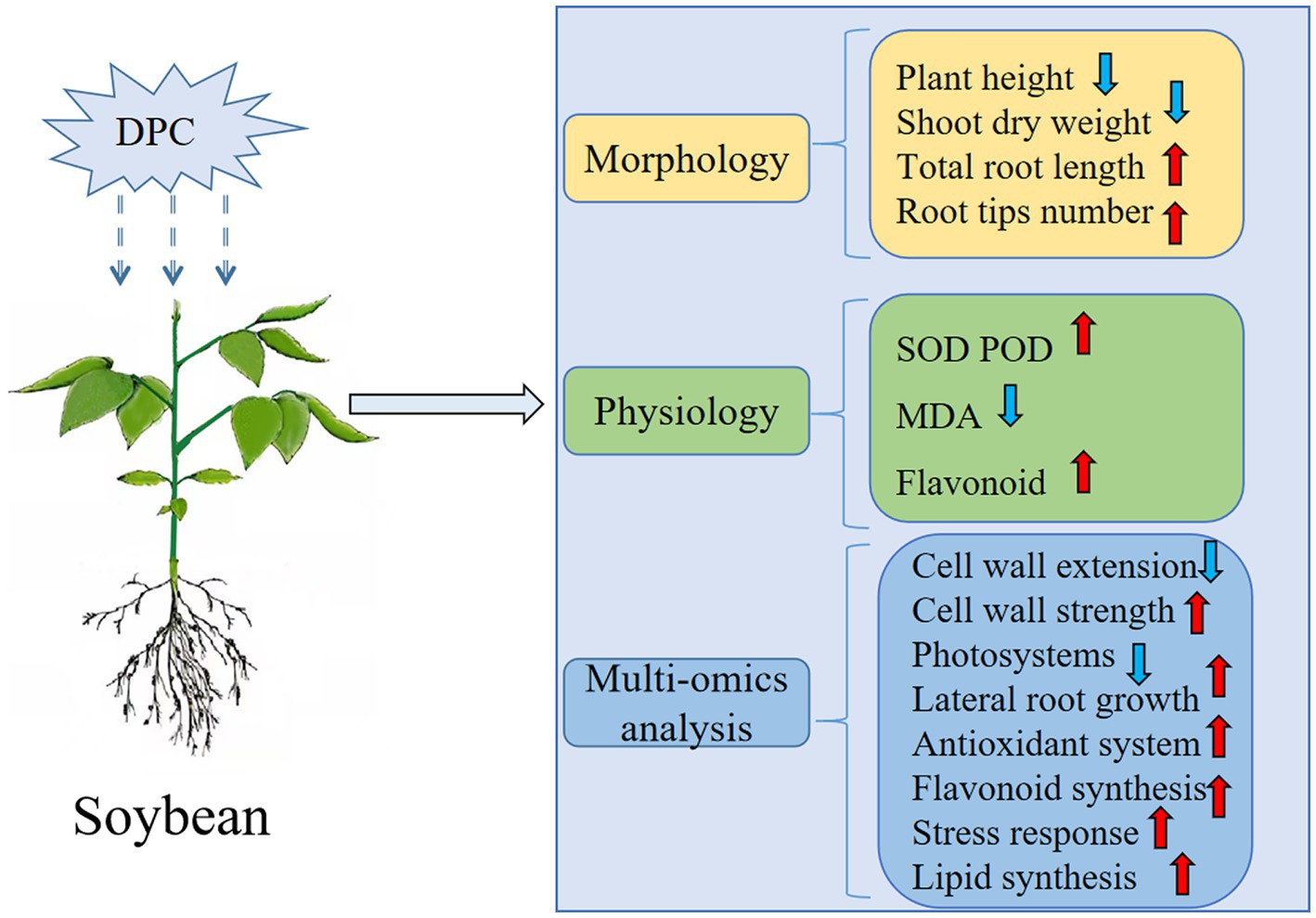
Figure 9. Summary of mepiquat chloride (DPC) regulation mechanism on soybeans. SOD, superoxide dismutase; POD, catalase; MDA, malondialdehyde. (The red arrow is rising, and the blue arrow is falling).
4. Discussion
Mepiquat chloride has been widely used as a growth retardant in cotton and maize (Spitzer et al., 2016; Echer and Rosolem, 2017); however, its application in soybeans remains scarcely studied. In this study, the regulatory mechanism of DPC in soybean was comprehensively analyzed by spraying DPC at the seedling stage. Mepiquat chloride delayed the shoot growth of soybean, which indicated that plant height and shoot dry weight decreased, root growth was promoted, and total root length and the number of root tips increased. Juscelina et al. (2020) treated Eucalyptus with DPC, and the results showed that the root dry weight increased after treatment. The internal water use efficiency (WUEi) and SPAD index were improved, and the growth promotion effect was significant. In addition, DPC enhanced the stress resistance of plants, which was manifested by an increase in SOD and POD activities, a decrease in MDA content, an increase in flavonoid content, and considerable upregulation of differential proteins involved in plant disease resistance and defense response.
As a mild plant growth retardant, DPC can reduce plant height and adjust crop canopy structure (Tung et al., 2020). This study indicated that DPC-treated soybean varieties showed significantly reduced plant height compared to the control. Spitzer et al. found that the activities of key enzymes in the early synthesis of gibberellin, ent-copalyl diphosphate synthase, and ent-kaurene synthase were inhibited by DPC treatment, and the plant height of maize was significantly reduced (Spitzer et al., 2016), which was consistent with the results of the present study. Proteomics results showed that xyloglucan endotransglucosylase (A0A445LKH0) and pectin acetylesterase (A0A445F2P8), which are related to cell wall construction, were significantly downregulated. Xyloglucan endotransglucosylase (A0A445LKH0) is a critical cell wall-modifying enzyme. It plays a vital role in relaxing cell walls and promoting cell elongation. The significant downregulation of this differential protein is an important reason for the decrease in plant height. In our previous experiments, the transcriptome results showed that the differential genes in the process of xyloglucan and hemicellulose metabolism were significantly downregulated, which was consistent with the results of the protein and morphological experiments.
As one of the three major vegetative organs, the root system helps plants absorb soil nutrients, and good root status is the basis for a high and stable soybean yield (Cheng et al., 2021). In this study, with the increase in DPC concentration, the total root length and root tip number of soybean first increased and then decreased, and the two varieties generally reached a maximum of 100 mg/L. Differential protein analysis showed that A0A445K4L3 and A0A445G8Y3 proteins that promote lateral root development were significantly upregulated. Wu et al. (2019) found that DPC promotes the formation of lateral cotton roots, which is consistent with the results of this experiment. Reducing plant height is conducive to constructing good plant types to lay a theoretical foundation for the rational close planting of soybean (Liang et al., 2020). Good root conditions are essential to resist abiotic stresses such as drought (Boguszewska-Mańkowska et al., 2019).
In addition, critical protective enzymes in plants, such as SOD and POD, can enhance the adaptability of plants to the external environment, and their activity is closely related to plant resistance. This study reported that DPC significantly increased the activity of SOD and POD in soybean leaves, and the increase in protective enzyme content helped maintain the balance between free radical production and scavenging. The content of protective enzymes in soybean leaves was the highest at 100 mg/L, indicating that free radicals were removed more effectively or promptly at this concentration, thereby promoting physiological metabolic function and improving the ability of soybean to resist adversity. Malondialdehyde was used to measure the degree of membrane lipid peroxidation during plant growth. When MDA levels are low, the plant metabolism is strong, which is conducive to accumulating assimilation products. Marius et al. (2015) showed that MDA accumulation is inversely proportional to the protective ability of plant tissues within a specific range. Our results showed that the MDA content of the soybean leaves decreased significantly after being sprayed with DPC. As an important secondary metabolite, total flavonoids have antioxidant activities and play an essential role in plant stress and pest resistance. In a previous experiment, we found that flavonoids accounted for approximately 60% of the differential metabolites. Therefore, we determined the change in the flavonoid content in soybean leaves by measuring the total flavonoid content. The results showed that DPC treatment significantly increased the total flavonoid content, consistent with the results of the multi-omics study. Horbowicz et al. (2021) found that the accumulation of flavonoids can slow the senescence rate of leaves and maintain plants in a good growth state.
The application of DPC can regulate photosynthetic processes in crops. Gonias et al. (2012) showed that applying DPC can increase the net photosynthetic and CO2 exchange rates of cotton leaves and affect the distribution of photosynthetic assimilates. In contrast, studies have shown that DPC treatment reduces cotton’s net photosynthetic and transpiration rates (Tung et al., 2018, 2019). In this experiment, the proteins related to the photosynthesis of HN44 and HN65 were significantly downregulated, including proteins involved in the electron transfer of P700 and cytochrome b6-f complex in photosystem I (A0A445K3L3 and A0A445KUC7), proteins related to the light capture complex (I1JJU8, I1JJU8, C6TD73, and A0A445L7Y5), and photosystem II central protein (A0A445KLU5 and Q2PMT8). Simultaneously, the ATP synthesis-related proteins I1MNP0, I1M100, A0A368UJC9, A0A445KND3, I1MNP0, and A0A0B2PVR1 were also significantly downregulated. A previous transcriptome analysis found that differential genes related to the photosystem and porphyrin synthesis were significantly downregulated. Photosynthesis is considered the most important and limiting factor in plants. Based on the results of the multi-omics experiments, significant inhibition of photosynthesis at the protein level was observed in soybean after DPC treatment. However, it needs to be further verified through physiological experiments, which is also one of the limitations of the present study.
In plants, phenylpropanoids are compounds composed of one or more C6-C3 units connected by benzene rings and three straight-bond carbons (Anguraj et al., 2015). Various metabolites, including lignin and flavonoids, play crucial roles in plant growth and development. The most significant function of lignin is to enhance the mechanical strength of plant cell walls and promote the formation of xylem vessels, which is of great significance in improving crop stem strength and lodging resistance (Dong and Lin, 2021). In this experiment, the related proteins A0A445M496, A0A0R0LDD3, A0A445JCJ8, A0A445KH18, and I1L2L1 enhanced the mechanical strength of the soybean cell wall after DPC treatment and were significantly upregulated, which was of great significance for reducing the crop lodging rate under high-density planting conditions. The proteins P26987, P15490, I1K6M9, A0A445KUT8, C6T1D7, and A0A445K9K5 are related to the enhancement of plant stress resistance and were significantly upregulated. A0A445K9K5 (chalcone-flavonone isomerase family protein) is involved in flavonoid biosynthesis in plants. Flavonoids are secondary metabolites, and studies have shown that flavonoids can improve the resistance of plants to abiotic stress (Bian et al., 2020; Ma et al., 2020). Wang et al. (2022b) reported that DPC could significantly increase the content of flavonoids in soybean by DPC treatment, which is of great significance in resisting abiotic stress.
5. Conclusion
The morphological and physiological indices of soybean showed significant dynamic changes after treatment with different concentrations of DPC, which inhibited soybean elongation and shoot dry weight, promoted root growth, increased SOD and POD activities involved in stress resistance, increased total flavonoid content, and decreased the membrane lipid peroxidation product MDA content. We found that DPC regulated the distribution of assimilates by affecting photosynthesis in soybean, promoting the transfer of assimilates to roots, and was beneficial to root growth. An increase in the content of protective enzymes and secondary metabolites involved in stress resistance is conducive to soybean resistance to adverse environments during growth. In conclusion, the results of this study showed that 100 mg/L DPC treatment could improve the ability of soybeans to resist adversity at the seedling stage, which has important practical significance for production practice.
Data availability statement
The raw data were uploaded to the iProX proteomics mass spectrometry data storage platform, numbered PXD040486 (http://proteomecentral.proteomexchange.org/cgi/GetDataset?ID=PXD040486).
Author contributions
YT and XL: software. ZQ and XZ: formal analysis. SS: writing—original draft preparation. JL and SD: writing—review and editing. All authors contributed to the article and approved the submitted version.
Funding
This work was supported by the National Key R&D Program of China (Grant No. 2020YFD1000902-02) and funded by the Natural Science Foundation of Heilongjiang Province of China (Grant No. LH2021C023).
Conflict of interest
The authors declare that the research was conducted in the absence of any commercial or financial relationships that could be construed as a potential conflict of interest.
Publisher’s note
All claims expressed in this article are solely those of the authors and do not necessarily represent those of their affiliated organizations, or those of the publisher, the editors and the reviewers. Any product that may be evaluated in this article, or claim that may be made by its manufacturer, is not guaranteed or endorsed by the publisher.
Footnotes
References
Anguraj, V. A. K., Sukumaran, A., Li, X., and Dhaubhadel, S. (2015). Soybean isoflavonoids: role of GmMYB176 interactome and 14-3-3 proteins. Phytochem. Rev. 15, 391–403. doi: 10.1007/s11101-015-9431-3
Ashrafi, E., Razmjoo, J., Zahedi, M., and Pessarakli, M. (2015). Screening Alfalfa for Salt Tolerance Based on Lipid Peroxidation and Antioxidant Enzymes. Agronomy Journal. 107, 167–173.
Benitez, J. J., Gonzalez Moreno, A., Guzman-Puyol, S., Heredia-Guerrero, J. A., Heredia, A., and Dominguez, E. (2021). The response of tomato fruit cuticle membranes against heat and light. Front. Plant Sci. 12:807723. doi: 10.3389/fpls.2021.807723
Bian, X. H., Li, W., Niu, C. F., Wei, W., Hu, Y., Han, J. Q., et al. (2020). A class B heat shock factor selected for during soybean domestication contributes to salt tolerance by promoting flavonoid biosynthesis. New Phytol. 225, 268–283. doi: 10.1111/nph.16104
Boguszewska-Mańkowska, D., Zarzyńska, K., and Nosalewicz, A. (2019). Drought differentially affects root system size and architecture of potato cultivars with differing drought tolerance. Am. J. Potato Res. 97, 54–62. doi: 10.1007/s12230-019-09755-2
Chen, J., Yang, X., Geng, J., Wang, Y., Liu, Q., Zhang, H., et al. (2020). Controlled-release potassium chloride containing mepiquat chloride improved bioavailability of soil potassium and growth of cotton plants. Arch. Agron. Soil Sci. 67, 1901–1915. doi: 10.1080/03650340.2020.1817902
Cheng, Y., Wang, X., Cao, L., Ji, J., Liu, T., and Duan, K. (2021). Highly efficient Agrobacterium rhizogenes-mediated hairy root transformation for gene functional and gene editing analysis in soybean. Plant Methods 17:73. doi: 10.1186/s13007-021-00778-7
Dong, N. Q., and Lin, H. X. (2021). Contribution of phenylpropanoid metabolism to plant development and plant-environment interactions. J. Integr. Plant Biol. 63, 180–209. doi: 10.1111/jipb.13054
Echer, F. R., and Rosolem, C. A. (2017). Plant growth regulation: a method for fine-tuning mepiquat chloride rates in cotton1. Pesqui. Agropecu. Trop. 47, 286–295. doi: 10.1590/1983-40632016v4745540
Gonias, E. D., Oosterhuis, D. M., and Bibi, A. C. (2012). Cotton radiation use efficiency response to plant growth regulators. J. Agric. Sci. 150, 595–602. doi: 10.1017/s0021859611000803
Horbowicz, M., Wiczkowski, W., Goraj-Koniarska, J., Miyamoto, K., Ueda, J., and Saniewski, M. (2021). Effect of methyl jasmonate on the terpene trilactones, flavonoids, and phenolic acids in Ginkgo biloba L. leaves: relevance to leaf senescence. Molecules 26:4682. doi: 10.3390/molecules26154682
Hossain, Z., Khatoon, A., and Komatsu, S. (2013). Soybean proteomics for unraveling abiotic stress response mechanism. J. Proteome Res. 12, 4670–4684. doi: 10.1021/pr400604b
Juscelina, A. S., Sylvana, N. M., Paulo, A. T., Paula, A. S. R., Luan, S. O., and Otavio, C. C. (2020). Morphophysiological changes by mepiquat chloride application in Eucalyptus clones. Trees 35, 189–198. doi: 10.1007/s00468-020-02021-7
Kamran, M., Ahmad, I., Wang, H., Wu, X., Xu, J., Liu, T., et al. (2018). Mepiquat chloride application increases lodging resistance of maize by enhancing stem physical strength and lignin biosynthesis. Field Crop Res. 224, 148–159. doi: 10.1016/j.fcr.2018.05.011
Kedrina-Okutan, O., Novello, V., Hoffmann, T., Hadersdorfer, J., Schneider, A., Schwab, W., et al. (2019). Polyphenolic diversity in Vitis sp. leaves. Sci. Hortic. 256:108569. doi: 10.1016/j.scienta.2019.108569
Li, H. S. The principles and techniques of plant physiological andbiochemical experiments. (2010). Beijing: High Educ Press. 260–261.
Li, X., Rehman, S. U., Yamaguchi, H., Hitachi, K., Tsuchida, K., Yamaguchi, T., et al. (2018). Proteomic analysis of the effect of plant-derived smoke on soybean during recovery from flooding stress. J. Proteome 181, 238–248. doi: 10.1016/j.jprot.2018.04.031
Liang, F. B., Yang, C. X., Sui, L. L., Xu, S. Z., Yao, H. S., and Zhang, W. F. (2020). Flumetralin and dimethyl piperidinium chloride alter light distribution in cotton canopies by optimizing the spatial configuration of leaves and bolls. J. Integr. Agric. 19, 1777–1788. doi: 10.1016/s2095-3119(19)62792-9
Luo, L., Xu, F., Hong, S., Weng, H., Duan, L., and Li, Z. (2010). Inducing effects of mepiquat chloride on the chilling resistance of sweet pepper (Capsicum annuum) seedlings. Chin. J. Pestic. Sci. 12, 142–148. doi: 10.3969/j.issn.1008-7303
Ma, S., Lv, L., Meng, C., Zhang, C., and Li, Y. (2020). Integrative analysis of the metabolome and transcriptome of Sorghum bicolor reveals dynamic changes in flavonoids accumulation under saline-alkali stress. J. Agric. Food Chem. 68, 14781–14789. doi: 10.1021/acs.jafc.0c06249
Mallory, M. L., Zhao, W., and Irwin, S. H. (2015). The cost of post-harvest forward contracting in corn and soybeans. Agribusiness 31, 47–62. doi: 10.1002/agr.21388
Manan, S., Ahmad, M. Z., Zhang, G., Chen, B., Haq, B. U., Yang, J., et al. (2017). Soybean LEC2 regulates subsets of genes involved in controlling the biosynthesis and catabolism of seed storage substances and seed development. Front. Plant Sci. 8:1604. doi: 10.3389/fpls.2017.01604
Marius, A. D. V., Liu, L., Mekawy, A. M. M., Ueda, A., Nagaoka, T., and Saneoka, H. (2015). Effect of salt stress on Na accumulation, antioxidant enzyme activities and activity of cell wall peroxidase of huckleberry (Solanum scabrum) and eggplant (Solanum melongena). Int. J. Agric. Biol. 17, 1149–1156. doi: 10.17957/IJAB/15.0052
MertoĞLu, K., EvrenosoĞLu, Y., and Polat, M. (2019). Combined effects of ethephon and mepiquat chloride on late blooming, fruit set, and phytochemical characteristics of black diamond plum. Turk. J. Agric. For. 43, 544–553. doi: 10.3906/tar-1811-65
Song, Q., Cao, W. L., Jiang, H., Zhang, A. H., and Meng, X. C. (2016). H2O2 improves quality of radix scutellariae through anti-oxidant effect. Pharmacogn. Mag. 12, 84–90. doi: 10.4103/0973-1296.176063
Souza-Schlick, G. D., Soratto, R. P., Fernandes, A. M., and Martins, J. D. L. (2018). Mepiquat chloride effects on castor growth and yield: spraying time, rate, and management. Crop Sci. 58, 880–891. doi: 10.2135/cropsci2017.07.0420
Spitzer, T., Míša, P., Bílovský, J., and Kazda, J. (2016). Management of maize stand height using growth regulators. Plant Prot. Sci. 51, 223–230. doi: 10.17221/105/2014-pps
Tomáš, S., Jan, B., and Jan, K. (2018). Effect of using selected growth regulators to reduce sunflower stand height. Plant Soil Environ. 64, 324–329. doi: 10.17221/213/2018-pse
Tung, S. A., Huang, Y., Ali, S., Hafeez, A., Shah, A. N., Ma, X., et al. (2019). Mepiquat chloride effects on potassium acquisition and functional leaf physiology as well as lint yield in highly dense late-sown cotton. Ind. Crop. Prod. 129, 142–155. doi: 10.1016/j.indcrop.2018.11.056
Tung, S. A., Huang, Y., Ali, S., Hafeez, A., Shah, A. N., Song, X., et al. (2018). Mepiquat chloride application does not favor leaf photosynthesis and carbohydrate metabolism as well as lint yield in late-planted cotton at high plant density. Field Crop Res. 221, 108–118. doi: 10.1016/j.fcr.2018.02.027
Tung, S. A., Huang, Y., Hafeez, A., Ali, S., Liu, A., Chattha, M. S., et al. (2020). Morpho-physiological effects and molecular mode of action of mepiquat chloride application in cotton: a review. J. Soil Sci. Plant Nutr. 20, 2073–2086. doi: 10.1007/s42729-020-00276-0
Wang, X. K., and Huang, J. L. (2015). Plant physiological and biochemical test principle and technology. Beijing: High Educ Press. 282–283.
Wang, X. Y., Jiang, J. X., Wu, Z. H., Li, X. M., and Dong, S. K. (2022a). Relationship between soil moisture content and superoxide dismutase activity in soybean leaves in Northeast China. J. Biobaased Mater. Bioenergy 15, 799–804. doi: 10.1166/jbmb.2021.2148
Wang, L., Mu, C., Du, M., Chen, Y., Tian, X., Zhang, M., et al. (2014). The effect of mepiquat chloride on elongation of cotton (Gossypium hirsutum L.) internode is associated with low concentration of gibberellic acid. Plant Sci. 225, 15–23. doi: 10.1016/j.plantsci.2014.05.005
Wang, X. Y., Zhou, Q., Wang, X., Song, S., Liu, J., and Dong, S. (2022b). Mepiquat chloride inhibits soybean growth but improves drought resistance. Front. Plant Sci. 13:982415. doi: 10.3389/fpls.2022.982415
Waseem, M., Rong, X., and Li, Z. (2019). Dissecting the role of a basic helix-loop-helix transcription factor, SlbHLH22, under salt and drought stresses in transgenic Solanum lycopersicum L. Front. Plant Sci. 10:734. doi: 10.3389/fpls.2019.00734
Wu, Q., Du, M., Wu, J., Wang, N., Wang, B., Li, F., et al. (2019). Mepiquat chloride promotes cotton lateral root formation by modulating plant hormone homeostasis. BMC Plant Biol. 19:573. doi: 10.1186/s12870-019-2176-1
Zhang, Q., Yang, W., Liu, W., Qin, W., Chen, H., Wu, D., et al. (2020). Nutritional evaluation of whole soybean curd made from different soybean materials based on amino acid profiles. Food Qual. Saf. 4, 41–50. doi: 10.1093/fqsafe/fyaa011
Keywords: soybean, growth, physiology, proteomics, resilience, morphology, mepiquat chloride
Citation: Song S, Li X, Tian Y, Zhou X, Qu Z, Liu J and Dong S (2023) Physiology and proteomics analyses reveal the regulatory mechanism of mepiquat chloride in soybean. Front. Sustain. Food Syst. 7:1188159. doi: 10.3389/fsufs.2023.1188159
Edited by:
Vuk M. Maksimović, University of Belgrade, SerbiaReviewed by:
Arti Bartwal, Indian Council of Agricultural Research (ICAR), IndiaTengxiang Lian, South China Agricultural University, China
Copyright © 2023 Song, Li, Tian, Zhou, Qu, Liu and Dong. This is an open-access article distributed under the terms of the Creative Commons Attribution License (CC BY). The use, distribution or reproduction in other forums is permitted, provided the original author(s) and the copyright owner(s) are credited and that the original publication in this journal is cited, in accordance with accepted academic practice. No use, distribution or reproduction is permitted which does not comply with these terms.
*Correspondence: Shoukun Dong, c2hvdWt1bmRvbmdAMTYzLmNvbQ==