- Department of Entomology, University of Kentucky, Lexington, KY, United States
As consumer demand and grower interest for pasture-raised poultry grow, more research is needed to understand the ecological consequences of the integration of pasture-raised poultry on agroecosystems. Poultry could have profound and complex net effects on arthropod communities given their high density per area, broad omnivory, and high manure deposition. Further, some studies suggest poultry may aid in the suppression of agricultural pests in integrated systems. Yet, unlike wild birds, pasture-raised poultry have received little attention in the field of agroecological net effects. Across 2 years, we examined how an absence (control- cover crop only), low- [9.51 m2 (102.4 ft.2) of pasture per broiler] and high-densities [4.76 m2 (51.2 ft.2) of pasture per broiler] of broilers impacted cover crop biomass, ground-dwelling arthropods, and plant-dwelling arthropods in a rotationally grazed mixed-cover crop system. High- and low-density poultry treatments had 7.8-fold and 3.5-fold less cover crop biomass compared to the control treatment after 1–3 days of access, respectively. Despite the depletion of cover crops, there were substantial positive effects on ground-dwelling arthropods. Most striking was the impact on house fly larvae where high-density poultry treatments had ~1,432-fold more house fly larvae relative to the control treatments. Dung beetle, spider, and rove beetle mean relative abundances increased 47-, 2.4-, and 3.5-fold, respectively, from the control treatment to the high-density poultry treatment. In contrast, the mean relative abundances of plant-dwelling arthropod orders Coleoptera, Hemiptera, and Hymenoptera were 4-, 5-, and 3.6-fold higher, respectively, in the control treatment relative to the high-density poultry treatment. Overall, these results suggest that pasture-raised poultry may promote the abundance of ground-dwelling arthropods through bottom-up mechanisms by depositing fecal material. However, poultry decreased the abundance of plant-dwelling arthropods, likely by destroying their habitat and food resources (via consumption and trampling of cover crop) and direct consumption of arthropods. While the integration of poultry into crop rotations is thought to benefit crop yield through nutrient deposition in the form of manure, this study suggests it may also stimulate the soil and ground-foraging arthropod food webs. This study is the first to evaluate the impacts of pastured poultry to arthropod communities in a mixed-cover crop system.
1. Introduction
Once common across North America, integrated crop-livestock systems have greatly declined as specialization and agricultural intensification have come to characterize the agricultural landscape over the last century (Dimitri et al., 2005; Naylor et al., 2005; Hilimire, 2011). Prior to the industrialization and specialization of agriculture, the functioning of agroecosystems heavily relied on the complexity, diversity, and synergy conferred by crop-livestock integrated systems to produce food and fiber, fertilize soil, and to power farm machinery (Russelle et al., 2007; Hilimire, 2011). Despite these benefits, crop and livestock systems became ecologically disintegrated and spatially disconnected as specialization was facilitated by the wide availability of manmade inputs such as synthetic fertilizer and the mechanization of farm equipment (Clark, 2004). Further, specialization has been historically incentivized through policies that minimized risks for specific crops, catalyzing farmers to shift from diverse integrated farms to farms consisting of homogenized crops, with the average number of commodities produced by individual farms dropping from five commodities per farm in 1990 to less than two crops per farm in 2002 (Dimitri et al., 2005; Hilimire, 2011).
Recently, however, there has been a renewed interest in integrated crop-livestock systems and the ecological benefits that they may confer to agroecosystems (Hilimire, 2011; Sossidou et al., 2011; Elkhoraibi et al., 2017). In particular, there has been an increased interest in pasture-raised poultry over the last decade, defined by the American Pastured Poultry Producers Association (APPPA) as operations in which poultry have continuous access to pasture and are moved to fresh pasture regularly (Rothrock et al., 2019; American Pastured Poultry Producers Association., 2022). While the number of pasture-raised poultry operations in the United States is not tracked and reported in the Census of Agriculture conducted by the USDA National Agriculture Statistics Service (USDA NASS, 2017), there has been an increase in small to medium-sized poultry operations in the United States within the last decade (USDA NASS, 2007, 2017). This re-emerging interest in pasture-raised poultry is driven by potential ecological benefits such as increased soil quality, control of weeds, and manure deposition as fertilizer as well as prospects of improved meat and egg quality and growing consumer interest alike (Sossidou et al., 2011; Elkhoraibi et al., 2017; Rothrock et al., 2019).
Like wild birds, it is often hypothesized that pasture-raised poultry will exert top-down forces on arthropod communities and have the potential to consume insect pests, though this has rarely been quantified. While there have been studies devoted to investigating the net effects of wild birds on arthropod communities (e.g., Mooney et al., 2010 and references therein), there has been scant attention on investigating the net effects of pasture-raised poultry on arthropod communities in agroecosystems. Studies on the impacts of wild birds in agriculture show that wild birds can impact arthropod communities primarily through two pathways. First, insect-eating birds can consume pest insects in agroecosystems, providing ecosystem services of pest suppression to a given agroecosystem. For example, wild birds provide pest suppression services in coffee by reducing coffee berry borer beetle [Hypothenemus hampei (Ferrari)] activity by ~50% in Costa Rica (Karp et al., 2013). Second, wild birds can act as intraguild predators by consuming natural enemy arthropods, essentially providing an ecosystem disservice by disrupting pest control services provided by insect predators. For example, Grass et al. (2017) found that wild bird activity disrupted biological control of aphid pests in cereal crops when insect-feeding birds consumed natural enemies of aphids. When birds were excluded from the cereal crops, arthropod natural enemy abundance was greater and aphid densities were lower (Grass et al., 2017). Overall, from the literature on the net effects of wild birds on arthropod communities in agroecosystems we can glean that wild birds typically exert top-down forces on arthropod communities (Maas et al., 2013; Díaz-Siefer et al., 2021). Thus, it can be expected that chickens and other insect-eating poultry would interact with arthropod communities in a similar way. Indeed, a small study that investigated the role of free-range chickens and geese as biological control agents of insect and weed pests associated with an intercropping of apples and potatoes found, through dissections of chicken digestive crops, that chickens consumed a variety of insects including beneficial (dung beetles and ground beetles) insects and pest insects such as Japanese Beetles, which were found in 75% of dissected digestive crops (Clark and Gage, 1996). Further, geese were able to maintain weed cover to <10% across the season through consistent grazing. However, chickens or geese did not have an impact on yield (Clark and Gage, 1996).
Yet, there are key differences between wild birds and pasture-raised poultry that may yield differences on their net effects to agroecosystems, especially through bottom-up forces. Pasture-raised poultry are large birds, in high densities, and confined to small areas within agroecosystems, likely concentrating their consumptive effects of arthropods and plants and intensifying their deposition of manure. On the contrary, wild birds are highly mobile and likely to interact with agroecosystems at a landscape scale (Gonthier et al., 2014). Specifically, the prolonged persistence of pasture-raised poultry on the same patch of vegetation may mean that poultry will likely deposit high quantities of feces, and their associated nutrients, to the agroecosystem, likely at much greater densities than wild birds. A typical chicken layer, for example, is estimated to produce 58.97 kg (130 lb.) of fresh manure per year, with a flock of 1,000 hens estimated to produce 58,967 kg (65 tons) of fresh manure per year, though these estimates vary depending on the type of poultry (chickens, turkeys, etc.) and whether the poultry are broilers, layers, breeders, etc. (McCall, 1980; Chastain et al., 2001). Although the literature on the impact of pasture-raised poultry manure deposition on arthropod communities is scarce, studies focusing on how applying animal manure as fertilizer impacts arthropod communities show that such applications can in some instances reduce abundances of insect crop pests (Brown and Tworkoski, 2004) and in some instances increase activity of arthropod generalist predators (Rowen et al., 2019). Thus, in addition to top-down effects of arthropod consumption that are typical for avian predators such as arthropod-eating birds, pastured-raised poultry may exert bottom-up effects through manure deposition. Additionally, in contrast to wild birds, pasture-raised poultry may produce additional top-down effects by reducing plant biomass through vegetative consumption and trampling.
Across 2 years, we investigated how the addition of pasture-raised poultry at varying densities impacted cover crop biomass and the abundances of plant- and ground-dwelling arthropods relative to control plots in an organic mixed-cover crop system. Here we hypothesized that poultry, given their wide diet breadth and their documented role in consuming insects, would exhibit strong top-down effects on cover crop biomass and arthropod communities. We predicted that low- and high-density poultry treatments would have reduced cover crop biomass and decreased arthropod abundances relative to the control treatment. As pastured poultry operations continue to grow across the United States, it remains critical to investigate potential ecological impacts that pastured poultry activity may have on agroecosystems and to evaluate how these impacts may stray from our predictions which are largely based on net effects studies that focus on wild bird activity.
2. Materials and methods
This study was conducted under the approval and guidance of the Institutional Animal Care and Use Committee (IACUC) at the University of Kentucky under #2020-3446.
2.1. Experimental setup
This experiment was conducted in the fall of 2020 and 2021 during the fall poultry integration phase of a larger project that examined various aspects of integrating pasture-raised poultry (as defined by APPPA; see intro) within a vegetable crop rotation system. The basic rotation sequence for this larger experiment was as follows: spring vegetable crop, summer cover crop, fall integrated poultry treatments established on the summer cover crop, and finally winter cover crop. The three integrated rotational treatments were: (1) no poultry, (2) low-density poultry [9.51 m2 (102.4 ft.2) of pasture per broiler], or (3) high-density [4.76 m2 (51.2 ft.2) of pasture per broiler] poultry in the fall rotational segments (Supplementary Figure S1). The low-density treatment approximates Pasture Raised Certification standard densities [10.03 m2 (108 ft.2) of pasture per broiler; Certified Humane® 2014]. Given that this study focused on the short-term ecological impacts of poultry on arthropod communities in the fall of 2020 and 2021, the methodological description will focus on summer cover crop and fall poultry integration phases. For a more detailed description of field preparation throughout the rotational sequence, see the Supplementary material.
The initial experimental setup in March of 2020 utilized two experimental fields [each ~82.3 m (270 ft.) long by 15.24 m (50 ft.) wide] within the certified organic section of the University of Kentucky Horticulture Research Farm. Four blocks of three 9.75 m (32 ft.) by 9.75 m (32 ft.) plots across the two fields (n = 12 plots) were centered and separated by 3.05 m (10 ft.) between each plot. Within each block, each plot was randomly assigned to one of the three fall integrated poultry treatments. In the spring of 2020, before poultry integration began, baseline arthropod sampling was collected.
In the summer of 2020 and 2021, all plots were mowed, drip tape was removed, and fields were spaded and cultivated. On June 10th, 2020, a cover crop mixture [buckwheat 44.83 kg/ha (40 lb./acre), cowpea 44.83 kg/ha (40 lb./acre), and teff 13.45 kg/ha (12 lb./acre)] was drill seeded across all plots. On June 28th of 2021, a cover crop mixture [teff 50.44 kg/ha (45 lb./acre), crimson clover 36.99 kg/ha (33 lb./acre), annual rye grass 35.31 kg/ha (31.5 lb./acre)] was broadcast seeded across all plots. Changes in seeding methods and densities between years were made to better establish a dense cover crop within a short time period and changes to cover crop mixture between years was primarily due to the cowpea being very tall in 2020, making it challenging to move poultry pens (see Supplementary material for details). Summer cover crops were mowed prior to the integration of poultry to facilitate the movement of chicken tractor hoop pens (“chicken tractors” hereafter; Figure 1; Skelton et al., 2012).
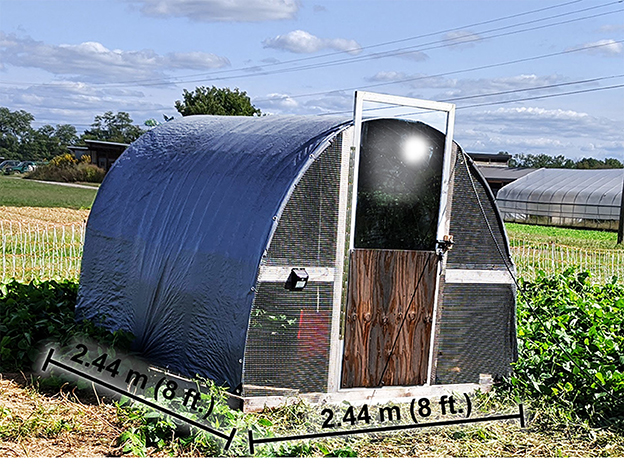
Figure 1. Chicken tractor. Chicken tractor with base dimensions of 2.44 m by 2.44 m (8 ft. by 8 ft.).
2.2. Pastured poultry integration
Poultry were brooded to 3 weeks of age (see Supplementary material for brooder management) before being integrated into experimental plots at the following densities: no poultry (n = 0), low density poultry (n = 10 in 2020, n = 12 in 2021) and high-density poultry (n = 20 in 2020, n = 22 in 2021). The number of chickens in the poultry density treatments differed across years because in 2021 additional poultry were needed for an additional experiment by a colleague. We used the Red Ranger breed in 2020 and the Cornish Rock Cross breed in 2021. The change in breed was made to better reflect the type of pastured-poultry operations in this region in which Cornish Rock Cross are typically used. Within each experimental plot (except the no poultry treatment), poultry were housed in chicken tractors, floorless movable pens that allow chickens to interact with the vegetation that they are placed on while the structures simultaneously provide protection from predators. Chicken tractors were constructed following Skelton et al. (2012). The chicken tractors consisted of a frame base of dimensions 2.44 m x 2.44 m (8 ft. x 8 ft.) with cattle panel looping from one side of the base to the other to give the tractor a hooped structure, and welded wire to exclude predators (Figure 1). Each tractor was equipped with a door, allowing for easy entrance by chicken caretakers. Tarps were placed over each chicken tractor to provide chickens with protection from flying predators and rain. All chicken tractors were moved to the next adjacent position within experimental plots on the same day and received the same time duration within each position. However, the chicken tractors were moved to the next adjacent position every few days for the first 2 weeks of the experiment and roughly every day for the remainder of the experiment (Figure 2).
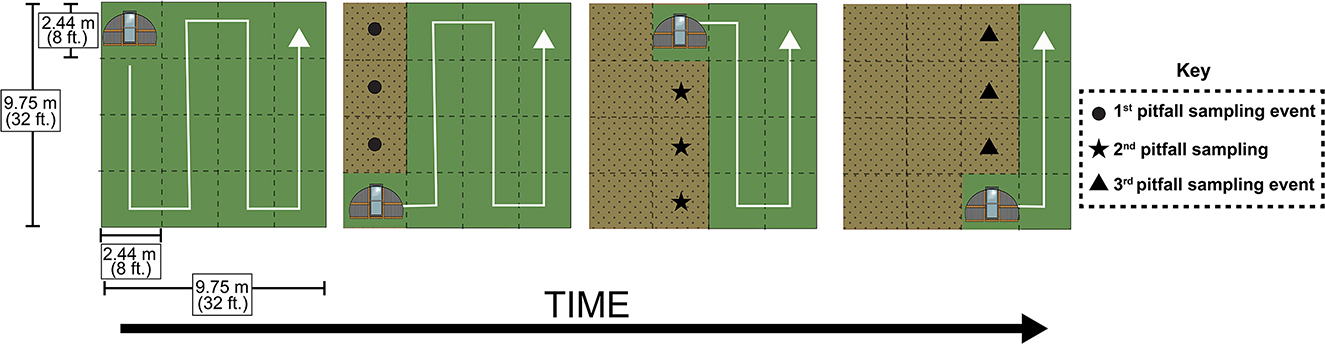
Figure 2. Tractor movement and pitfall sampling scheme. Tractor movement across a poultry treatment plot. This graphic depicts the movement of a chicken tractor across the duration of the experiment within a 9.75 m by 9.75 m (32 ft. by 32 ft.) plot. White arrows depict the direction in which the tractors move, with tractors moving into adjacent 2.44 m by 2.44 m (8 ft. by 8 ft.) positions across time. Green spaces depict cover crop that has yet to be reached by chicken tractors while brown stippled spaces are meant to depict spaces that have already been occupied by chicken tractors. Circle, star, and triangle shapes represent the arrangement of pitfall traps across three sampling events following chicken tractor movements.
The perimeter of the entire experimental setup was bordered by an electric fence to prevent entrance from predators. For added protection, each plot containing poultry was enclosed with an additional electric fence. Two solar-powered electric fence chargers were used to power the electric fence, with one charger dedicated to powering the perimeter fence located immediately outside of the experimental field and the other charger located in the non-cover cropped corridor. The same layout was followed for both years of the experiment.
2.3. Cover crop and biomass cover
To measure the effect of pasture-raised poultry on cover crop biomass and percent vegetative cover we collected biomass samples and conducted visual assessments of percent cover of each 2.44 m by 2.44 m (8 ft. by 8 ft.) chicken tractor position. In order to avoid edge effects, we focused biomass sampling efforts to the central 1.22 m2 (4 ft.2) area within the 2.44 m2 (8 ft.2) area that had previously been occupied by a chicken tractor (i.e., the dimensions of the base of the chicken tractors). Within this 1.22 m2 (4 ft.2) area we randomly sampled a 0.305 m2 (1 ft.2) quadrat of biomass per plot. All vegetation that was rooted within the quadrat was collected, placed in paper bags, and dried in a drying oven for ~48–72 h or until completely dry. In 2020, there were six biomass samples collected per plot (2020 total = 72 biomass samples) and in 2021 there were nine biomass samples collected per plot (2021 total = 108 biomass samples). To account for spatial and pseudo replication, we averaged biomass samples that were collected from the same plot for each year. Percent vegetative cover was assessed through visual observation and was recorded for each 2.44 m2 (8 ft.2) area that had previously been occupied by a chicken tractor.
2.4. Arthropod sampling
In order to assess the impact of poultry presence on arthropod communities, we used pitfall traps and sweep-nets to collect ground-dwelling and plant-dwelling arthropods, respectively. Pitfall traps were deployed for seven days, and sweep-net sampling took place during the afternoon on days in which pitfalls were deployed. Pitfall traps consisted of a plastic cup and a removable funnel, with the cup depth being ~10.8 cm (4.25 in.) (https://www.carolina.com/entomology/pitfall-trap-pk-10/654131.pr). Pitfalls were placed flush with the ground, allowing for arthropods to easily walk or fall into trap. Pitfall solution was 10% NaCl and a few drops of unscented dish detergent to break surface tension, a common solution used across studies that use pitfalls (Hohbein and Conway, 2018). Plastic covers made of plastic disposable plates were secured over each pitfall with landscape staples to prevent rainwater from contaminating traps. Following the first three tractor movements, we immediately deployed three pitfalls in the center of each of the 2.44 m2 (8 ft.2) spaces that were previously occupied by a chicken tractor in each plot. We repeated this sampling strategy an additional two times per year for a total of three collecting events per year, resulting in a total of nine pitfall samples per plot each year (see Figure 2 for sampling scheme). In total, 216 pitfall traps were deployed across the 2 years. Following pitfall trap collection, the samples were transferred from the NaCl solution to 70% EtOH until samples could be sorted. Sweep-net sampling consisted of 30 sweeps per plot per collecting event using a standard 15-inch diameter sweep-net (BioQuip Products, Rancho Dominguez, CA 90220, USA) as the collector walked up and down a 2.44 m by 7.32 m (8 ft. by 24 ft.) area within the plot. This 2.44 m by 7.32 m (8 ft. by 24 ft.) area is meant to represent an area previously occupied by a chicken tractor across three movements. Sweep-net samples were stored in a standard freezer until sorting and identification could take place. In order to account for spatial and temporal pseudo replication, we aggregated arthropod abundances by averaging abundances from samples collected within the same plot for each year. Additionally, analyses were limited to common taxa found within the collected samples (at least 100 individuals across both years resulting in the exclusion of pseudoscorpions and millipedes from pitfall analysis and the exclusion of spiders, Lepidoptera, and Orthoptera from sweep-net analysis).
2.5. Functional groups
In order to determine whether the addition of poultry to a cover crop system impacted functional arthropod groups we adopted the following guild classifications: natural enemies, known crop pests, predatory hemipterans, and herbivorous hemipterans. The composition of these groups varied by collection method (pitfall vs. sweep net) as these different methods collected different insect types, but functional trait identity remained consistent for insects that were collected by both methods. For pitfall samples, the natural enemies group included spiders (Class: Arachnida; Order: Araneae), ground beetles (Coleoptera: Carabidae), minute pirate bugs (Hemiptera: Anthocoridae), big eyed bugs (Hemiptera: Geocoridae), damsel bugs (Hemiptera: Nabidae), lady beetles (Coleoptera: Coccinellidae), and wasps (including both predators and parasitoids). The sweep net natural enemies group included spiders, minute pirate bugs, big eyed bugs, damsel bugs, lady beetles, soldier beetles (Coleoptera: Cantharidae), predatory stink bugs (Coleoptera: Pentatomidae), and wasps. The known crop pest group for pitfall samples consisted of any identifiable pest of common fruit and vegetable crops, including: cucumber beetles (Diabrotica undecimpunctata Mannerheim and Acalymma vittatum (Fabricius)), bean leaf beetle (Cerotoma trifurcata (Forster)), flea beetles (Coleoptera: Chrysomelidae), aphids (Hemiptera: Aphidae), corn earworm (Helicoverpa zea (Boddie)), weevils (Coleoptera: Curculionidae), green June beetle (Cotini nitida (Linnaeus)), pigweed flea beetle (Disonycha glabrata (Fabricius)), squash bugs (Anasa tristis (De Geer)), armyworms (Spodoptera spp.), and the tarnished plant bug (Lygus lineolaris (Palisot de Beauvois)). The sweep net samples known crop pest group included cucumber beetle, bean leaf beetle, flea beetles, pigweed flea beetle, aphids, weevils, corn earworm, false chinch bugs (Nysius spp.), tarnished plant bug, and non-predaceous stinkbugs. The predatory hemipteran group for pitfall samples consisted of minute pirate bugs, big eyed bugs, and damsel bugs. The predatory hemipteran group for sweep net samples consisted of minute pirate bugs, big eyed bugs, damsel bugs, and predatory stinkbugs. The herbivorous hemipterans group consisted of hemipterans that were not recognized as major agricultural pests including plant hoppers (Hemiptera: Cicadellidae), tree hoppers (Hemiptera: Membracidae), milkweed bugs (Hemiptera: Lygaeidae), and other hemipterans for the pitfall samples and leaf hoppers, tree hoppers, spittle bugs (Hemiptera: Cercopidae), plant bugs (Hemiptera: Miridae), and other hemipterans for sweep net samples.
2.6. Statistical analysis
All analyses were performed in R Studio version 4.2.1 (R Core Team 2022). Linear mixed effects models (LMM) were fit to each of the response variables using the “lmer” function in the package “lme4” with block and year as random effects and poultry density treatment as a fixed effect (Bates et al., 2019). If the residuals of the model were not normal, as tested by the Shapiro-Wilk Test, we applied a log (X+1) or square root transformation to meet assumptions of Gaussian distribution. In order to account for false discovery rates associated with multiple comparisons, we adjusted p-values from our models using the function “p.adjust” from the package “stats” with a Holm-Bonferroni correction (R Core Team, 2021). Post hoc tests were performed using the function “emmeans” with a Tukey adjustment from the package “emmeans” to determine significant pairwise comparisons (Lenth et al., 2019). Non-metric multidimensional scaling (NMDS) was used to visualize differences in composition between poultry and control treatments of pitfall and sweep net samples at the order level using the function “metaMDS” with a Bray-Curtis dissimilarity calculation from the “vegan” package (Oksanen et al., 2020). In order to determine whether composition of pitfalls and sweep net samples differed across treatments at the order level we performed permutational multivariate analysis of variance (PERMANOVA) with the “adonis” function from the “vegan” package. Post hoc pairwise comparisons were conducted by using the function “pairwise.adonis” from the package “pairwiseAdonis” and p-values reported from this analysis are adjusted with a Holm-Bonferroni correction (Martinez Arbizu, 2020). As mentioned throughout the methods section, some changes were made between 2020 and 2021. Specifically, seeding method (drill vs. broadcast seeding), cover crop composition, and poultry breed changed across the two experimental years. Given these differences and how they might impact abundance of ground- and plant-dwelling arthropods we ran additional models in which year, treatment, and an interaction between year and treatment were included as fixed effects. Despite all these differences between years, the impact of poultry integration on the arthropod community was relatively consistent (See Supplementary Tables S7–S9 and Supplementary Figures S3–SS5 for model output and graphs).
3. Results
3.1. Cover crop biomass and percent cover
Poultry integration significantly reduced cover crop biomass (g) (F2, 20 = 51.306, p < 0.001). A Tukey post hoc test (Tukey, 1977) revealed that the high-density poultry treatment (mean ± SE; 3.42 g ± 0.825 g) had less biomass than the low-density poultry treatment (5.83 g ± 0.852 g; p=0.021), and the no poultry control treatment (37.2 g ± 8.74 g; p < 0.001). There was also significantly less biomass in the low-density poultry treatment relative to the control (p < 0.001). Percent cover of cover crops also varied by treatment (F2, 21 = 254.78, p < 0.001). A Tukey post hoc test revealed that the high-density poultry treatment (14.9 ± 2.73) had significantly lower percent cover relative to the low-density poultry treatment (25.4 ± 3.93; p=0.041), and the control (96.7 ± 0.594; p < 0.001). The low-density poultry treatment also had significantly less cover than the control (p < 0.001).
3.2. Arthropod abundance
In total, across the 2 years, 52,692 arthropods of varying life stages were captured in the pitfall traps, with the greatest abundances being from the order Diptera (22,756) of which 11,069 were house fly larvae, followed by the order Coleoptera with 12,496 individuals, and order Hymenoptera with 8,500 individuals. A total of 5,507 arthropods were collected from sweep net samples, with the greatest abundances being from the order Diptera with 4,178 individuals, followed by order Hemiptera with 805 individuals, and Hymenoptera with 229 individuals.
Further, prior to the addition of poultry to the experimental field in fall of 2020, arthropod abundances were sampled via sweep-net and pitfall samples, following the collection methods outlined above, during the spring of 2020 when broccoli was in rotation to assess the baseline arthropod community. This was to assess whether there were any pre-existing differences in arthropod abundances in the areas to be used as experimental plots. Analysis of these samples, at the order level, found no differences in relative abundance of either plant-dwelling or ground-dwelling arthropods in the areas that were to be used as experimental plots during the fall poultry rotation See Supplementary Tables S1–S4 for details on the abundances of these baseline samples.
3.3. Ground-dwelling arthropods
Poultry density treatments had a significant effect on the relative abundances of 15 of the 20 insect taxa and functional groups that we examined, with exceptions being centipedes (Class Chilopoda), pill bugs (Order: Isopoda), ground beetles, and herbivorous hemipterans (Table 1).
3.3.1. Orders
At the order level, it was revealed that poultry density treatments had a significant effect on relative abundances of Coleoptera, earwigs (Order: Dermaptera), Diptera, Hemiptera, Hymenoptera, Lepidoptera, Orthoptera, Psocodea, and Spiders (Table 1). The orders Coleoptera, Dermaptera, Diptera, Hemiptera, Hymenoptera, and Spiders followed a similar trend in which there were greater abundances of these orders in the high-density poultry treatment relative to control treatment and greater abundance in low-density poultry treatment relative to the control treatment but there was no difference between high-and low-density treatments (Figures 3A–E, I; see Supplementary Table S5 for post hoc pairwise comparisons and associated p-values). The orders Lepidoptera and Psocodea shared the same trend in which the abundance of these orders was greater in high-density poultry treatment relative to control, but there were no differences in abundance between low-density and control treatment or between high-density and low-density treatments (Figures 3F, H and Supplementary Table S5). Orthoptera, in contrast to the other orders, had a greater abundance in the control treatment relative to high-density treatment and greater abundance in control treatment relative to low-density treatment but there was no difference between high-density and low-density treatments (Figure 3G and Supplementary Table S5).
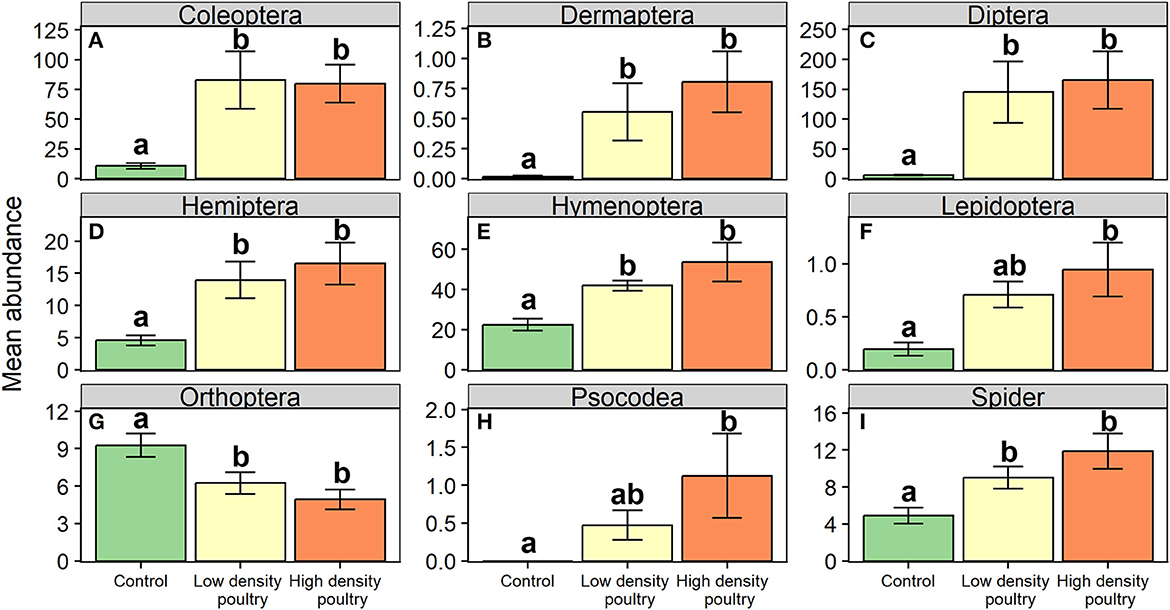
Figure 3. Ground-dwelling arthropod orders. Mean abundances of ground-dwelling arthropod orders (A–I). Bars represent standard error bars while letters above bars indicate significant differences (p < 0.05) between treatments per Tukey HSD post hoc (see Supplementary Table S5 for Tukey HSD post hoc results).
3.3.2. Other taxa
Below the order level, we found that poultry density treatment had a significant effect on the abundance of ants, dung beetles (Coleoptera: Scarabaeidae), house fly larvae, and rove beetles (Coleoptera: Staphylinidae) (Table 1). Ant, house fly larvae, and rove beetle abundances were greater in high-density poultry treatment relative to control and greater in low-density poultry treatment relative to control, but we found no differences in abundance between high-density and low-density poultry treatments (Figures 4A, C, D and Supplementary Table S5). Ant, house fly larvae, and rove beetle abundances were 43.3-, 1432-, and 3.5-fold greater in high-density relative to control treatments, respectively. Dung beetle abundance was 43.3 times greater in high-density poultry treatment relative to control treatment and was greater in high-density poultry relative to low-density poultry treatments, but we found no difference between low-density poultry treatment and control treatment (Figure 4B and Supplementary Table S5).
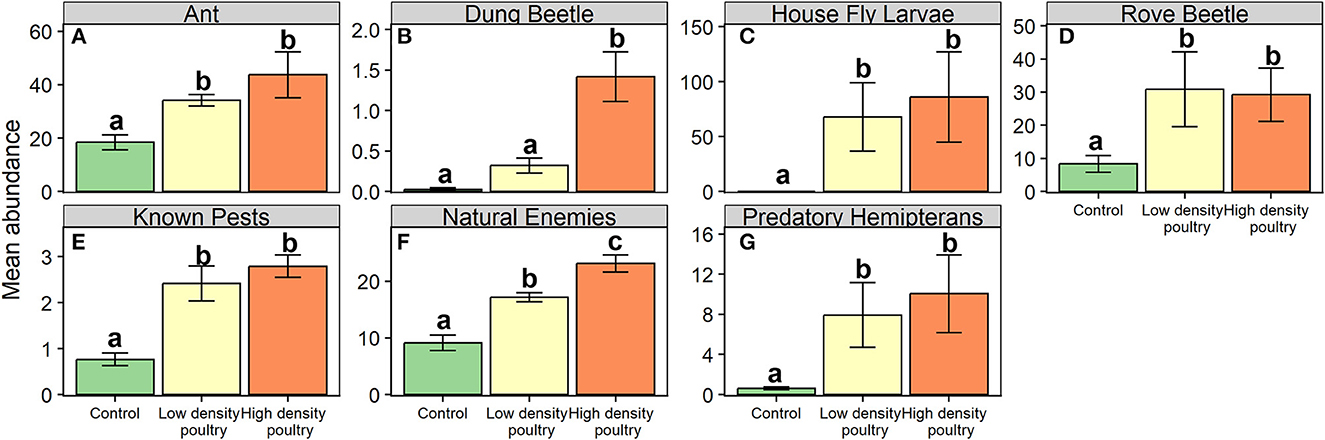
Figure 4. Ground-dwelling arthropod taxa of interest and functional groups. Mean abundances of ground-dwelling arthropod taxons (A–D) and functional groups (E–G). Bars represent standard error bars while letters above bars indicate significant differences (p < 0.05) between treatments per Tukey HSD post hoc test (see Supplementary Table S5 for Tukey HSD post hoc results).
3.3.3. Functional groups
We found that poultry density treatment had a significant effect on the relative abundance of known crop pests, natural enemies, and predatory hemipterans functional groups (Table 1). Known crop pests and predatory hemipterans had greater abundances in high-density poultry relative to control treatment greater abundances in low-density poultry treatment relative and control treatment but we found no difference between high- and low-density poultry treatments (Figures 4E, G and Supplementary Table S5). We found greater abundances of natural enemies in high-density poultry treatment relative to control treatment and relative to low-density poultry treatment, and greater abundances in low-density poultry treatment relative control treatment (Figure 4F and Supplementary Table S5).
3.4. Plant-dwelling arthropods
At the ordinal level, poultry density treatment had a significant effect on the abundance of plant-dwelling Coleoptera, Hemiptera, and Hymenoptera but not Diptera (Table 2). Coleoptera and Hemiptera abundances were greater in the control treatment relative to the high-density treatment and greater in control treatment relative to low-density treatment but there was no difference between high-density and low-density treatments (i, ii in Figure 5A and Supplementary Table S6). Coleoptera and Hemiptera abundances were 4.1- and 5.1-fold greater in the control treatment relative to the high-density poultry treatment, respectively. Hymenoptera had 3.6-fold greater abundance in control treatment relative to high-density and Hymenoptera abundance was greater in control treatment relative to low-density treatment and between low- and high-density poultry treatments (iii in Figure 5A and Supplementary Table S6).
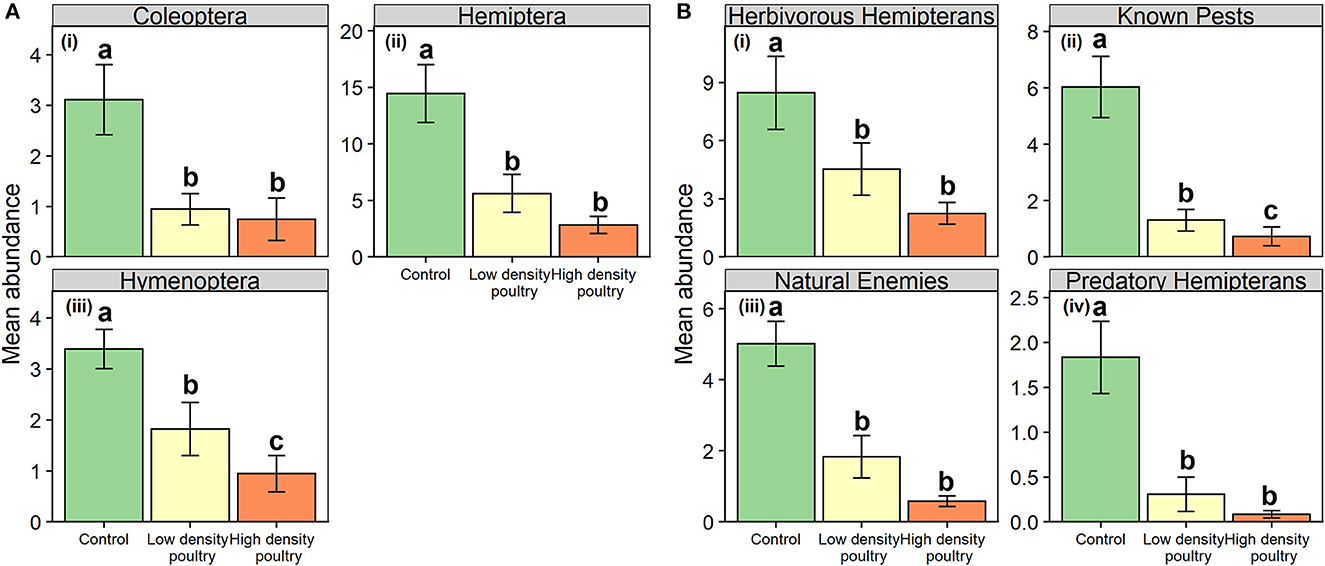
Figure 5. Plant dwelling-arthropod orders and functional groups. Mean abundances of plant-dwelling arthropod orders (A) and functional groups (B). Bars represent standard error bars while letters above bars indicate significant differences (p < 0.05) between treatments per Tukey HSD post hoc (see Supplementary Table S6 for Tukey HSD post hoc results).
Poultry density treatments had a significant effect on plant-dwelling arthropod abundances of herbivorous hemipteran, known crop pest, natural enemy, and predatory hemipteran functional groups (Table 2). Abundances of herbivorous hemipterans, natural enemies, and predatory hemipterans were greater in control treatment relative to high-density and low-density treatments, but no differences were observed between high- and low-density poultry treatments (i, iii-iv in Figure 5B and Supplementary Table S6). Herbivorous hemipteran, natural enemy, and predatory hemipteran abundances were 3.8-, 8.6-, and 22.9-fold times greater in control treatment relative to high-density poultry treatment, respectively. Known crop pest abundance was 8.1-fold greater in control treatment relative to high-density and was greater in control treatment relative to low-density treatments (ii in Figure 5B and Supplementary Table S6). Further, known crop pest abundance was greater in the low-density poultry treatment relative to the high-density poultry treatment.
3.5. Community composition
The community composition of ground-dwelling arthropods, at the order level, varied with treatment (F-value = 11.6, R2= 0.52, p = 0.001; Figure 6A). A post hoc analysis showed significant pairwise differences between high-density poultry treatment and control treatment (p = 0.003) and significant pairwise differences between low-density poultry treatment and control treatment (p = 0.003) but not between low-density and high-density treatments (p = 0.461). For plant-dwelling arthropods, we found no differences in community composition, at the order level, across treatments (F-value = 2.07; R2 = 0.16; p = 0.091; Figure 6B).
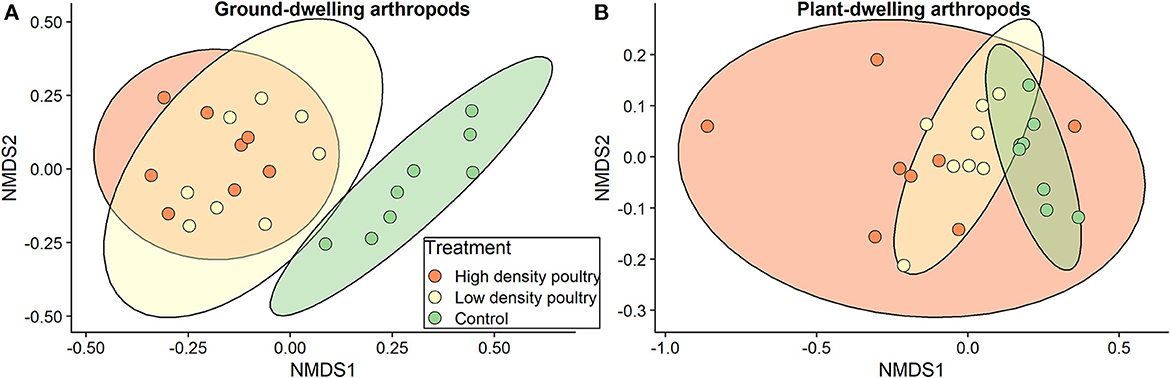
Figure 6. Composition between treatments. NMDS plots for (A) ground-dwelling and (B) plant-dwelling arthropod communities at the order level. Number of reduced dimensions for both was k = 2. Stress = 0.105 for (A) and stress=0.056 for (B).
4. Discussion
This is one of the few studies that has investigated how the addition of poultry to an agroecosystem impacted the abundance and community composition of arthropods. We hypothesized that poultry would exhibit strong negative effects on arthropods given observations that poultry consume arthropods and trample and consume vegetation. Indeed, this study found strong negative impacts of integration on cover crop biomass and percent vegetative cover. However, integration of poultry to a mixed-cover crop system had a positive relationship on the abundance of several ground-dwelling arthropods, suggesting that poultry may promote ground-dwelling arthropod communities. Conversely, we observed a negative relationship between the abundance of several plant-dwelling arthropods and the addition of poultry to a cover-crop system, suggesting that poultry may reduce plant-dwelling arthropod communities. Potential mechanisms for these observed relationships can best be described as bottom-up and top-down effects for ground dwelling-arthropods and plant-dwelling arthropods, respectively (Hunter and Price, 1992).
4.1. Top-down effects
While the main drivers of these relationships remain unknown, it is likely that top-down effects by poultry on plant-dwelling arthropods are driven by chicken activity that results in the destruction of plant-dwelling arthropod habitat (i.e., plant stems and leaves) via trampling and consumption of plant material (Figures 7A–C). Poultry reduced cover crop biomass by 72 and 87% and in the low- and high-density poultry treatments relative to the control, respectively. This phenomenon is in contrast to observed relationships between wild birds and vegetation, as the presence of wild birds has been shown to increase plant biomass in addition to reducing leaf damage and plant morality in both agricultural and natural systems by consuming herbivorous arthropods (Mäntylä et al., 2011). There is also a possibility that poultry may be consuming plant-dwelling arthropods (Figures 7A, D, E). However, this likely only contributes a small fraction to the observed reduction in plant-dwelling arthropod abundance given the drastic reduction in plant cover that was observed across the study and the lack of insect foraging behavior displayed by the chickens. Likewise, consumption of ground-dwelling arthropods is likely also occurring (Figures 7A, D, F), although the effect of this consumption is greatly outweighed by the positive relationship between chicken fecal deposition and ground-dwelling arthropod abundance.
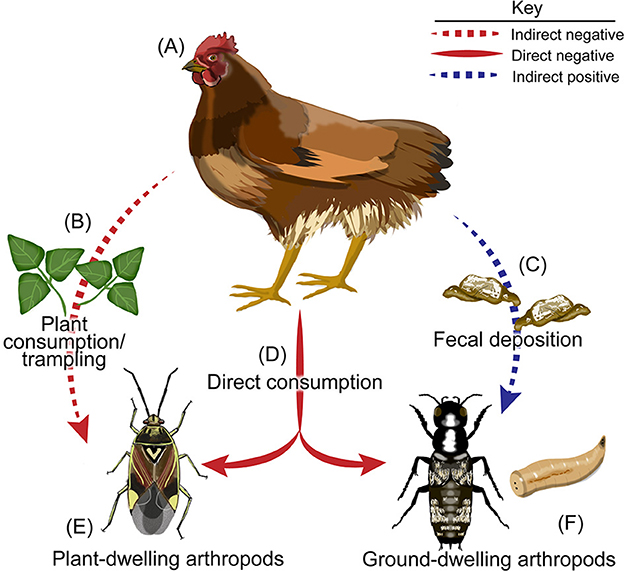
Figure 7. Potential pathways in which poultry may impact arthropod communities. A diagram showing the potential indirect and direct pathways in which pasture-raised poultry may impact arthropod communities. Poultry such as (A) chickens may (B) consume or trample plant habitat of (E) plant-dwelling arthropods, thereby reducing their local populations indirectly. (A) Chickens may also alter arthropod communities via their (C) fecal deposition which may increase the abundance of (F) ground-dwelling arthropods. Finally, (A) chickens may also (D) directly consume both plant-dwelling and ground-dwelling arthropods. Illustrations by K Garcia.
Future studies should compare how arthropod abundance changes in response to vegetation removal or destruction in the absence of poultry (e.g., by mowing, artificial trampling) to better estimate how much chicken insect foraging contributes to changes in arthropod communities. Additionally, it is also possible that the chicken tractors themselves contributed to decreases in plant-dwelling arthropods relative to control treatments (without tractors). The movement of these tractors, the short-term shading (1–3 days), and even the increased human activity (to care for poultry) near tractors may have impacted the arthropods and cover crops. Additionally, future studies should aim to establish perennial forages that are better suited for the destructive nature of pastured poultry and are more typical of pastured-poultry operations. Indeed, extension specialists suggest that forages for pastured poultry should consist of plant species that are tolerant to scratching and biting, have large leaf to stem ratios, and can recover from grazing and trampling (Jacob et al., 2017). This study utilized annual cover crops established after spring vegetable production to address over-arching questions related to the integration of poultry into vegetable rotations. However, a clear challenge to this short-term rotational integration was the establishment of an annual cover crop capable of withstanding poultry integration, evidenced by the drastic reductions in cover crop biomass. The rotational plan that was implemented across this project was designed to capture replication of experiment plots in order to replicate the impact of poultry integration on arthropods, cover crops, and crops as part of a larger project (Supplementary Figure S1). For this reason, we had rapid rotations of spring vegetables, summer cover crops, fall poultry, and winter cover crops. Many farmers that integrate poultry often utilize perennial pastures that are in place for a year or more before transition vegetable crops. While this research does not exactly represent these longer-term integration strategies, it is perhaps even more impressive that this short-term rotational integrated system found such strong changes in the arthropod communities, given the high-level of disturbance.
Whatever top-down effects poultry may be having on plant-dwelling arthropods, either directly or indirectly, appear to be impacting both beneficial (i.e., natural enemies) and crop pest insects; the relative abundances of both of these functional groups were greater in the control treatments relative to the poultry treatments (Figure 5B), suggesting that poultry activity comparably affects these functional groups in this cover-crop system. Similarly, plant-dwelling herbivorous hemipteran and predatory hemipteran abundances were also greater in the control treatment relative to high- and low-density poultry treatments. Thus, in this cover crop system, the addition of poultry non-discriminately negatively impacts the relative abundances of both beneficial and crop pest insects. Conversely, ground-dwelling natural enemies and known pests both increased with the addition of poultry (Figures 4E–G). Thus, it remains unclear whether natural enemies that are being promoted by poultry are providing sufficient, if any, pest suppression. Future studies should determine whether increased populations of ground-dwelling natural enemies (via addition of poultry) are providing pest suppression of insect pests through ecological approaches such as sentinel prey experiments which are often used to measure biological control activity by predators (Chisholm et al., 2014). Additionally, future research should quantitatively investigate whether poultry are consuming plant-dwelling arthropods and vegetation, such as with the use of DNA metabarcoding-based diet analysis (Crisol-Martinez et al., 2016; Mata et al., 2021). If pasture-raised poultry are indeed found to be consuming insects at significant rates, this might be beneficial to farmers as past research has shown that adding insect meal to poultry diet can improve growth performance (Benzertiha et al., 2020), nutrient digestibility and immune function (Elahi et al., 2022), and gut health (Biasato et al., 2018; Józefiak et al., 2020).
4.2. Bottom-up effects
Despite the evidence of top-down effects on plant-dwelling arthropod communities, the bottom-up effects on ground-dwelling arthropods were most staggering. Most impressive of all was the impact of poultry on house fly larvae (Figure 4C). Indeed, of the 11,069 house fly larvae that we collected in our pitfall traps across both years, only four were collected from control treatment plots. Despite these sharp differences in abundance between poultry density treatments, it should not come as a surprise since house flies are considered to be major pests of animal husbandry operations including poultry farms where they consume foodstuffs and wastes (Axtell, 1999; Malik et al., 2007). House fly larvae are known to be consumed by a variety of insects, including some hister beetles (Coleoptera: Histeridae) and the larvae of other flies (Malik et al., 2007). Indeed, the predatory functional groups: ants, rove beetles, spiders, predatory hemipterans, and natural enemies had greater relative abundances in poultry plots relative to control plots. It is plausible that these predators recruited to poultry plots to take advantage of dipteran prey. Additionally, the close proximity of research plots may have facilitated some mobile coprophagous insects being lured from control plots to experimental plots, thus inflating the observed differences. House flies may be so highly mobile that this would not play a role. Other less mobile groups may have moved from control plots to experimental plots. Ultimately, changes in relative abundance of arthropods reflects local population growth and colonization of plots to target resources, and it is likely that this phenomenon would be observed regardless of plot placement.
To our knowledge no other study has documented the bottom-up impacts of pasture-raised poultry manure deposition on arthropod communities. However, studies have investigated how applying poultry manure as fertilizer, sans poultry, impacts arthropods. Brown and Tworkoski (2004), for example, found that the application of composted chicken manure resulted in increased arthropod predators, less herbivores, and reduced abundances of key apple pests in apple orchards (Brown and Tworkoski, 2004). Additionally, a systematic review by Rowen et al. (2019) on fertility management for insect pest control found that manure fertilizer increased generalist predator activity in 6 of 13 studies. It is theorized that manure and other detritus increases populations of decomposer arthropods, whose populations can then assist in sustaining generalist predator populations (Halaj and Wise, 2002; Rowen et al., 2019). Our study appears to support this hypothesis, as we observed increased abundance of fly larvae and predatory groups (spiders, rove beetles, natural enemies, and predatory hemipterans).
In addition to potentially sustaining generalist predator populations, house fly larvae can aid in decomposition and nutrient cycling, and have in some instances been used to convert raw poultry manure to fertilizer (Calvert et al., 1970). However, given the documented role of house flies as vectors of food-borne pathogens to fresh produce including the transmission of Escherichia coli O157:H7 to spinach (Wasala et al., 2013) and the transmission of E. coli O157:H7 and Salmonella enterica to lettuce (Pace et al., 2017), special consideration should be given to the spatiotemporal separation of pastured poultry and fresh produce. Future studies should aim to investigate what spatial configurations between pastured poultry and fresh produce pose the least threats to food safety and what the role of house flies may be in heightening such risks. Further, when incorporating livestock and their raw manure into any crop rotational system, there are temporal aspects to consider. For example, USDA's National Organic Program standards call for a 120-day interval between the application of raw manure and harvest of crops whose edible portions come into contact with soil and a 90-day interval for crops whose edible portions do not come into contact with ground (USDA AMS NOP, 2023). Anecdotally, we observed large masses of maggots in residual poultry food that was exposed to rain after tractors had moved in the experimental rotation. Thus, it is possible that discarded poultry feed also impacted the abundance of house fly larvae.
Despite the large effect sizes observed between treatments, it remains unclear whether these changes in arthropod communities will persist over time. In this study, insect collection was done shortly after poultry had been occupying the collection areas in a 3-week time period. Thus, these results only highlight short-term effects on arthropod communities by poultry. It should be noted that within this study's rotational system fields were mowed and tilled immediately after broilers reached market weight and were processed which would act as a major disturbance to both the ground and vegetative arthropod community. Thus, it remains unclear what longer-term net effects of pastured-poultry activity could have on arthropod communities the following spring when vegetables are planted, and whether the effects of some interactions (Figure 7) would have longer-term effects than others (i.e., would the reduction of plant-dwelling arthropods persist longer than the increased abundance of ground-dwelling arthropods or vice versa).
Additionally, both the short-term nature of this study and our anticipation of strong top-down impacts on arthropod communities were similar to expectations of wild bird exclusion studies, although our results revealed additional bottom-up effects. Studies of the net effects of wild birds also show contrasting impacts via direct consumption of crops and indirect benefits to crops through trophic cascades (Pejchar et al., 2018). In some instances, birds can provide protection to crops by consuming insect pests. However, in some instances birds can constrain pest control services by consuming arthropod natural enemies (Martin et al., 2013). For the most part, studies that focus on the net effects of birds on agroecosystems focus on top-down effects such as predation of insects or crops. However, outside of agroecosystems, studies show that some gregarious bird species can have bottom-up effects as well. Seabirds, for example, typically forage across a vast marine area and then deposit marine nutrients (guano, carcasses, food scraps, reproduction byproducts) on their nesting islands (Kolb et al., 2012). The nesting activity of seabirds can also result in disturbances to the local environment (Kolb et al., 2012). For example, nesting great cormorants (Phalacrocorax carbo L.) in the Stockholm archipelago of Sweden were found to negatively impact plant species richness, percent vegetative cover, and plant species composition (Kolb et al., 2012). In regard to arthropod communities, Kolb et al. (2012) found varying responses for abundance of functional groups with herbivorous coleopteran abundance decreasing in islands with nesting cormorants but with fungivorous coleopteran and scavenging coleopteran abundances increasing (Kolb et al., 2012). These results from wild gregarious bird studies align with our findings, in which we found that there were not only top-down forces on plant vegetation and arthropods associated with vegetation, but also bottom-up forces on other arthropod groups.
5. Conclusion
This study revealed the drastic changes that can occur in arthropod community structure when prompted by the addition of pasture-raised poultry to a crop rotation. While it is thought that the addition of poultry to a crop rotation system may bolster yield through nutrients delivered from fecal deposits, this study shows that poultry activity may also stimulate ground-dwelling arthropods through bottom-up ecological mechanisms. However, this study also found that the addition of poultry decreased the abundance of plant-dwelling arthropods. The implications that these changes in arthropod abundance may mean for both short- and long-term biological control remain to be explored. Nonetheless, this study has contributed to our understanding of how poultry may impact arthropod communities.
Data availability statement
The raw data supporting the conclusions of this article will be made available by the authors, without undue reservation.
Ethics statement
The animal study was reviewed and approved by Institutional Animal Care and Use Committee at the University of Kentucky.
Author contributions
DG developed the methodology. KG, VH, KT, and DG collected the data. KG and DG analyzed the data. KG wrote the initial manuscript. DG and JD supervised the project. All authors contributed to the article and approved the submitted version.
Funding
This project was supported by OREI Grant #2019-51300-30244 awarded to DG, National Science Foundation Graduate Research Fellowship (Grant No. 1839289) to KG, USDA Hatch (KY008079) grant to DG.
Acknowledgments
Carrying out this project would not have been possible without the guidance and assistance from various people across the years. We are deeply grateful to Nat Colten for constructing the moveable chicken tractors that provided shelter for our chickens, and to Neil Wilson, Jay Tucker, and Steve Diver for their help and guidance in setting up various aspects of the experimental field at the UK Horticulture Research Farm. We also thank Ryan Kuesel and Katie Fiske for their assistance with various aspects of the project including chicken care, Alexis Gauger, Sara Jones, and Susanne Deeb for their assistance in sorting insect samples, Dr. Eric Chapman for his aid in insect identification, and Dr. Mark Williams for his guidance throughout the project. We are grateful to Mac Stone for his advice on raising pastured poultry and for allowing us to tour Elmwood Stock Farm to witness integrated farming in action. We thank Dr. Tony Pescatore and Dr. Lynne Cassone for their expertise and guidance on chicken health throughout the project and Rebecca Florence from the Office of the Attending Veterinarian at the University of Kentucky for their assistance and guidance in preparing and submitting animal use protocols. We thank Dr. Muntazir Mushtaq, Dr. Sean Clark, Dr. Kathleen Hilimire, Dr. Matthew Jones, Dr. Boyd Mori, Dr. Kathleen Delate, and Karenna Petersen for comments on earlier versions of this manuscript. Lastly, we are especially grateful to Delia Scott Hicks for her monumental role in getting this experiment off the ground.
Conflict of interest
The authors declare that the research was conducted in the absence of any commercial or financial relationships that could be construed as a potential conflict of interest.
Publisher's note
All claims expressed in this article are solely those of the authors and do not necessarily represent those of their affiliated organizations, or those of the publisher, the editors and the reviewers. Any product that may be evaluated in this article, or claim that may be made by its manufacturer, is not guaranteed or endorsed by the publisher.
Supplementary material
The Supplementary Material for this article can be found online at: https://www.frontiersin.org/articles/10.3389/fsufs.2023.1162753/full#supplementary-material
References
American Pastured Poultry Producers Association. (2022). Is Pastured Poultry Organic? Available online at: https://apppa.org/Is-Pastured-Poultry-Organic (accessed August 25, 2022).
Axtell, R. C. (1999). Poultry integrated pest management: status and future. Int. Pest Manag. Rev. 4, 53–73. doi: 10.1023/A:1009637116897
Bates, D., Maechler, M., Bolker, B., and Walker, S. (2019). Fitting linear mixed-effects models using lme4. J. Statist. Softw. 67, 1–48. doi: 10.18637/jss.v067.i01
Benzertiha, A., Kierończyk, B., Kołodziejski, P., Pruszyńska–Oszmałek, E., Rawski, M., Józefiak, D., et al. (2020). Tenebrio molitor and Zophobas morio full-fat meals as functional feed additives affect broiler chickens' growth performance and immune system traits. Poult. Sci. 99, 196–206. doi: 10.3382/ps/pez450
Biasato, I., Gasco, L., De Marco, M., Renna, M., Rotolo, L., Dabbou, S., et al. (2018). Yellow mealworm larvae (Tenebrio molitor) inclusion in diets for male broiler chickens: effects on growth performance, gut morphology, and histological findings. Poult. Sci. 97, 540–548. doi: 10.3382/ps/pex308
Brown, M., and Tworkoski, T. (2004). Pest management benefits of compost mulch in apple orchards. Agric. Ecosyst. Environ. 103, 465–472. doi: 10.1016/j.agee.2003.11.006
Calvert, C., Morgan, N., and Martin, R. (1970). House fly larvae: Biodegradation of hen excreta to useful products. Poult. Sci. 49, 588–589. doi: 10.3382/ps.0490588
Chastain, J. P., Camberato, J. J., and Skewes, P. (2001). Poultry manure production and nutrient content. In: Confined Animal Manure Managers Certification Program Manual: Poultry Version. Clemson, SC: Clemson University Extension.
Chisholm, P. J., Gardiner, M. M., Moon, E. G., and Crowder, D. W. (2014). Tools and techniques for investigating impacts of habitat complexity on biological control. Biol. Control 75, 48–57. doi: 10.1016/j.biocontrol.2014.02.003
Clark, E. A. (2004). Benefits of re-integrating livestock and forages in crop production systems. J. Crop Improv. 12, 405–436. doi: 10.1300/J411v12n01_06
Clark, M. S., and Gage, S. H. (1996). Effects of free-range chickens and geese on insect pests and weeds in an agroecosystem. Am. J. Alt. Agri. 11, 39–47. doi: 10.1017/S0889189300006718
Crisol-Martinez, E., Moreno-Moyano, L. T., Wormington, K. R., Brown, P. H., and Stanley, D. (2016). Using next-generation sequencing to contrast the diet and explore pest-reduction services of sympatric bird species in macadamia orchards in Australia. PLoS ONE 11. doi: 10.1371/journal.pone.0150159
Díaz-Siefer, P., Olmos-Moya, N., Fontúrbel, F. E., Lavandero, B., Pozo, R. A., and Celis-Diez, J. L. (2021). Bird-mediated effects of pest control services on crop productivity: a global synthesis. J. Pest Sci. 1–10. doi: 10.1007/s10340-021-01438-4
Dimitri, C., Effland, A., and Conklin, N. C. (2005). The 20th Century Transformation of US Agriculture and Farm Policy. Economic Research Service (USDA).
Elahi, U., Xu, C.-C., Wang, J., Lin, J., Wu, S.-G., Zhang, H.-J., et al. (2022). Insect meal as a feed ingredient for poultry. Anim. Biosci. 35, 332. doi: 10.5713/ab.21.0435
Elkhoraibi, C., Pitesky, M., Dailey, N., and Niemeier, D. (2017). Operational challenges and opportunities in pastured poultry operations in the United States. Poult. Sci. 96, 1648–1650. doi: 10.3382/ps/pew448
Gonthier, D. J., Ennis, K. K., Farinas, S., Hsieh, H.-Y., Iverson, A. L., Batáry, P., et al. (2014). Biodiversity conservation in agriculture requires a multi-scale approach. Proc. R. Soc. B Biol. Sci. 281, 20141358. doi: 10.1098/rspb.2014.1358
Grass, I., Lehmann, K., Thies, C., and Tscharntke, T. (2017). Insectivorous birds disrupt biological control of cereal aphids. Ecology 98, 1583–1590. doi: 10.1002/ecy.1814
Halaj, J., and Wise, D. H. (2002). Impact of a detrital subsidy on trophic cascades in a terrestrial grazing food web. Ecology 83, 3141–3151. doi: 10.1890/0012-9658(2002)0833141:IOADSO2.0.CO;2
Hilimire, K. (2011). Integrated crop/livestock agriculture in the United States: a review. J. Sustain. Agric. 35, 376–393. doi: 10.1080/10440046.2011.562042
Hohbein, R. R., and Conway, C. J. (2018). Pitfall traps: a review of methods for estimating arthropod abundance. Wildl. Soc. Bull. 42, 597–606. doi: 10.1002/wsb.928
Hunter, M. D., and Price, P. W. (1992). Playing chutes and ladders: heterogeneity and the relative roles of bottom-up and top-down forces in natural communities. Ecology 724–732. doi: 10.2307/1940152
Jacob, J., Pescatore, T., and Smith, R. (2017). Pastured Poultry. Lexington: Universiry of Kentucky.
Józefiak, A., Benzertiha, A., Kierończyk, B., Łukomska, A., Wesołowska, I., and Rawski, M. (2020). Improvement of cecal commensal microbiome following the insect additive into chicken diet. Animals 10, 577. doi: 10.3390/ani10040577
Karp, D. S., Mendenhall, C. D., Sand,í, R. F., Chaumont, N., Ehrlich, P. R., Hadly, E. A., et al. (2013). Forest bolsters bird abundance, pest control and coffee yield. Ecol. Lett. 16, 1339–1347. doi: 10.1111/ele.12173
Kolb, G. S., Jerling, L., Essenberg, C., Palmborg, C., and Hambäck, P. A. (2012). The impact of nesting cormorants on plant and arthropod diversity. Ecography 35, 726–740. doi: 10.1111/j.1600-0587.2011.06808.x
Lenth, R., Singmann, H., Love, J., Buerkner, P., and Herve, M. (2019). Package ‘emmeans'. Available online at: https://CRAN.R-project.org/package=emmeans
Maas, B., Clough, Y., and Tscharntke, T. (2013). Bats and birds increase crop yield in tropical agroforestry landscapes. Ecol. Lett. 16, 1480–1487. doi: 10.1111/ele.12194
Malik, A., Singh, N., and Satya, S. (2007). House fly (Musca domestica): a review of control strategies for a challenging pest. J Environ. Sci. Health B 42, 453–469. doi: 10.1080/03601230701316481
Mäntylä, E., Klemola, T., and Laaksonen, T. (2011). Birds help plants: a meta-analysis of top-down trophic cascades caused by avian predators. Oecologia 165, 143–151. doi: 10.1007/s00442-010-1774-2
Martin, E. A., Reineking, B., Seo, B., and Steffan-Dewenter, I. (2013). Natural enemy interactions constrain pest control in complex agricultural landscapes. Proc. Nat. Acad. Sci. 110, 5534–5539. doi: 10.1073/pnas.1215725110
Martinez Arbizu, P. (2020). Package “pairwiseAdonis”: Pairwise Multilevel Comparison Using Adonis. Available online at: https://github.com/pmartinezarbizu/pairwiseAdonis
Mata, V. A., da Silva, L. P., Veríssimo, J., Horta, P., Raposeira, H., McCracken, G. F., et al. (2021). Combining DNA metabarcoding and ecological networks to inform conservation biocontrol by small vertebrate predators. Ecol. Appl. 31, e02457. doi: 10.1002/eap.2457
McCall, W. W. (1980). Chicken Manure. Hawaii Cooperative Extension Service, College of Tropical Agriculture and Human Resources, University of Hawaii, Hawaii, Unites States.
Mooney, K. A., Gruner, D. S., Barber, N. A., Van Bael, S. A., Philpott, S. M., and Greenberg, R. (2010). Interactions among predators and the cascading effects of vertebrate insectivores on arthropod communities and plants. Proc. Nat. Acad. Sci. 107, 7335–7340. doi: 10.1073/pnas.1001934107
Naylor, R., Steinfeld, H., Falcon, W., Galloway, J., Smil, V., Bradford, E., et al. (2005). Losing the links between livestock and land. Science. 310, 1621–1622. doi: 10.1126/science.1117856
Oksanen, J., Blanchet, F. G., Friendly, M., Kindt, R., Legendre, P., McGlinn, D., et al (2020). Vegan: Community Ecology Package. R Package Version 2.5-6. 2019. Available online at: https://CRAN.R-project.org/package=vegan
Pace, R. C., Talley, J. L., Crippen, T. L., and Wayadande, A. C. (2017). Filth fly transmission of Escherichia coli O157: H7 and Salmonella enterica to lettuce, Lactuca sativa. Ann. Entomol. Soc. Am. 110, 83–89. doi: 10.1093/aesa/saw092
Pejchar, L., Clough, Y., Ekroos, J., Nicholas, K. A., Olsson, O., Ram, D., et al. (2018). Net effects of birds in agroecosystems. Bioscience 68, 896–904. doi: 10.1093/biosci/biy104
R Core Team. (2021). R: A Language and Environment for Statistical Computing. R Foundation for Statistical Computing, Vienna, Austria. Available online at: https://www.R-project.org/
Rothrock, M. J. Jr, Gibson, K. E., Micciche, A. C., and Ricke, S. C. (2019). Pastured poultry production in the united states: strategies to balance system sustainability and environmental impact. Front. Sustain. Food Syst. 3, 74. doi: 10.3389/fsufs.2019.00074
Rowen, E., Tooker, J. F., and Blubaugh, C. K. (2019). Managing fertility with animal waste to promote arthropod pest suppression. Biol. Control 134, 130–140. doi: 10.1016/j.biocontrol.2019.04.012
Russelle, M. P., Entz, M. H., and Franzluebbers, A. J. (2007). Reconsidering integrated crop–livestock systems in North America. Agron. J. 99, 325–334. doi: 10.2134/agronj2006.0139
Skelton, S., Jacob, J., and Pescatore, T. (2012). Making a Hoop Pen for Pasture Poultry. Lexington, KY: Cooperative Extension Service, University of Kentucky College of Agriculture.
Sossidou, E., Dal Bosco, A., Elson, H., and Fontes, C. (2011). Pasture-based systems for poultry production: implications and perspectives. World's Poul. Sci. J. 67, 47–58. doi: 10.1017/S0043933911000043
USDA AMS NOP (2023). United States Department of Agriculture- Agricultural Marketing Service, National Organic Program. 5006: Processed Animal Manures in Organic Crop Production. Available online at: https://www.ams.usda.gov/rules-regulations/organic/handbook/5006 (accessed April 14, 2023).
USDA NASS (2007). Census of Agriculture. Available online at: www.nass.usda.gov/AgCensus
USDA NASS (2017). Census of Agriculture. Available online at: www.nass.usda.gov/AgCensus
Keywords: pasture-raised poultry, ground-dwelling arthropods, chicken manure, crop-livestock integration, top-down, bottom-up, net effects
Citation: Garcia K, Halmos V, Thongjued K, Dupuis JR and Gonthier DJ (2023) Net effects of pasture-raised poultry on arthropod communities driven by top-down and bottom-up forces in a mixed-cover crop system. Front. Sustain. Food Syst. 7:1162753. doi: 10.3389/fsufs.2023.1162753
Received: 10 February 2023; Accepted: 24 April 2023;
Published: 12 May 2023.
Edited by:
Raghavendra Singh, Indian Institute of Pulses Research (ICAR), IndiaReviewed by:
Muntazir Mushtaq, Shoolini University, IndiaSean Clark, Berea College, United States
Kathleen Hilimire, Fort Lewis College, United States
Matthew Jones, Cascade Agroecology, United States
Copyright © 2023 Garcia, Halmos, Thongjued, Dupuis and Gonthier. This is an open-access article distributed under the terms of the Creative Commons Attribution License (CC BY). The use, distribution or reproduction in other forums is permitted, provided the original author(s) and the copyright owner(s) are credited and that the original publication in this journal is cited, in accordance with accepted academic practice. No use, distribution or reproduction is permitted which does not comply with these terms.
*Correspondence: Karina Garcia, a2FyaW5hX2dhcmNpYSYjeDAwMDQwO3VreS5lZHU=
†Present address: Karina Garcia, Department of Corporate Responsibility, Handsome Brook Farms, New York, NY, United States