- 1Cardiovascular Center, Taichung Veterans General Hospital, Taichung, Taiwan
- 2School of Food, Jiangsu Food and Pharmaceutical Science College, Huai'an, China
- 3Department of Food and Beverage Management, Chung-Jen Junior College of Nursing, Health Sciences and Management, Chia-Yi, Taiwan
- 4Department of Food Science and Technology, National Chung-Hsing University, Taicuhng, Taiwan
- 5Department of Food and Nutrition, Providence University, Taichung, Taiwan
The post-mortem rigid of farmed rainbow trout (Oncorhynchus mykiss) affects the texture of the meat and might even be risky for microorganisms, undermining the popularity of frozen filets among consumers. This study investigated the importance of different conditions (0°C, 4°C, and 25°C for 0–4 days) and physicochemical characteristics of rainbow trout filet storage on fish’s characteristics, freshness, quality, and shelf-life. Results showed that the fish easily underwent rigor mortis and resolution of rigor mortis when stored at 25°C. However, rigor mortis was more likely to occur under storage at 0°C than at 4°C [with 100 kV/m high voltage electrostatic fields (HVEF)] due to low-temperature stimulation, but resolution of rigor mortis began to appear after 24 h of storage at either temperature. The protein solubility and gel strength of fish stored at 25°C continued to decrease due to thermal denaturation, and those of fish stored at 4°C and 0°C also showed a decrease owing to rigor mortis and then an increase when rigor mortis disappeared. The K values increased rapidly at 25°C and reached 61% at 14 h. Under storage at 4°C and 0°C, the K values only slightly increased before resolution of rigor mortis. However, the K-values did not exceed 40% for 4 days post-resolution of rigor mortis. Differential Scanning Calorimetry analysis and tissue sectioning showed that protein denaturation and separation between muscle fibers persisted during storage at various temperatures, most notably at 25°C. It is important to note that storing at 0°C for 96 h did not result in any significant changes in the molecular protein composition. Moreover, the muscle tissue remained in excellent condition compared to storage at 25°C and 4°C. Additionally, there was no significant difference in the total viable count (TVC) and psychrophilic bacteria between storage at 0°C and 4°C, considered safe for food consumption. These promising findings are conveyed in a circular economy based on the food industry, particularly adequate raw materials, stable prices, measures to reduce food loss and waste, and contributing to developing efficient, diverse, and sustainable food processing systems.
Highlights
• Constant low-temperature preservation is required to maintain the freshness of fish.
• Storage at 4°C with HVEF treatment may delay 12 h for reaching maximum rigor mortis.
• Preserved fish are also suitable for food processing.
1. Introduction
Rainbow trout (Oncorhynchus mykiss) has progressively gained a place in the market as an economically valuable species. It has been characterized as a fatty fish and is one of the most popular fish for farming (Rezaei and Hosseini, 2008; Barrientos et al., 2019; Bienkiewicz et al., 2019). Compared with wild (Pacific species) and cultured (Atlantic species) salmon, its prices are lower and its flesh color, mouthfeel, and intramuscular fat layer are substantially different (Fox et al., 2018). It has been cultivated for artificial farming in many countries and regions (Genç and Diler, 2019; Yin C. et al., 2022). Aquaculture farms have been established for local supplies to ensure the stability and safety of the raw material source for the food industry-related supply chain. In addition, rainbow trout have been widely used for fresh service distribution and as raw material for the food and catering industries. For Chinese dishes, it is suitable for steaming, deep-frying, and grilling, and for Western dishes, it is sold as ready-to-eat food (appetizers, salads, or sandwiches) in the form of smoked fish filets and frozen products. To date, rainbow trout is most commonly used by the catering industry as a substitute for salmon in preparing Japanese cuisine primarily to reduce the raw material cost; raw fish forms are typically sliced filets for direct consumption called sashimi (Xiong et al., 2021). In light of this situation, the U.S. Food and Drug Administration has explicitly stated that rainbow trout should not be labeled salmon on food packaging. Meanwhile, the European Union has established general rules to prevent food fraud. As stated in EU Regulation 1379/2013, correctly labeling species using scientific and commercial species names is mandatory (Monteiro et al., 2021).
The freshness of fishery products depends on the product’s value, excluding the difference in fish species (Freitas et al., 2020; Li H. et al., 2022). Compared with livestock meat, fish tissues have fewer connective tissues, softer muscle tissues, and stronger self-digestion, making them prone to muscle protein hydrolysis and spoilage (Listrat et al., 2016; Prabhakar et al., 2020; Cheng H. et al., 2023; Hui et al., 2023; Ozogul et al., 2023). Therefore, preserving fish freshness is a typical application of low-temperature refrigeration (Freitas et al., 2020; Du et al., 2022). The muscles can retain good freshness during the post-mortem rigor mortis process, while the resolution of rigor mortis, the muscles soften, and the freshness decline (Warner, 2016). Thus, a remarkable relationship exists between freshness and the state of muscle protein (Yu et al., 2022).
In several surveys, it was found an “ambivalence” while analyzing the consumers’ preference for fish properties; most of them seem to prefer wild fish to farmed fish, locally originated fish to imported fish, fresh fish to frozen fish, whole fish to processed fish, but the same consumers have opposite situations in terms of convenience, accessibility, low price, and environmental protection (Carlucci et al., 2015; Lopes and de Freitas, 2023; Pascoe et al., 2023; Rodriguez-Salvador and Calvo Dopico, 2023; Yuan et al., 2023). Despite that, frozen, canned fish products and ready-to-eat fresh fish filets are considered very suitable products (perfect for emerging markets) while most consumers tend to save the time and effort required to prepare food and eat fish, in addition to the perception that fresh fish products are considered challenging to prepare, which is higher among young people than elderly people, while people living in coastal areas prefer fresh fish (Carlucci et al., 2015). Rainbow trout are usually sold alive in Taiwan, but death frequently occurs during shipping caused of unsuitable conditions; sold on ice is less favored by consumers, presumably due to the post-mortem rigid storage affecting the meat quality. Therefore, knowing of the physical and chemical indices of the finished product is important for the sensory characteristics and shelf life. This study investigated the correlation among the changes in muscle protein solubility, water retention, enthalpy, K- value, TVBN- value, and bacterial count under different storage temperatures (where HVEF was used at 4°C). The findings will serve as a reference for the selection of storage conditions for fish.
2. Materials and methods
2.1. Materials
Cultured rainbow trout (Oncorhynchus mykiss) specimens, each weighing about 600–700 g was purchased from a local market (Taichung, Taiwan). After slaughtering and eviscerating, the fish were rinsed with tap water, packed in polystyrene boxes, covered with ice, and carried back to the laboratory within 30 min. The semi-dressed fish were cleaned, dried with towels, and individually placed in a PVC plastic bag and sealed. Finally, the fish were randomly grouped and stored at 0°C, 4°C, and 25°C and sampled for physiochemical property determination at different storage times (0, 6, 12, 24, 36, 48, 72, and 96 h). In particular, the 4°C group was stored in a refrigerator (75% relative humidity) with the 100 kV/m high-voltage electrostatic field (HVEF) by an output voltage of 50 kV DC electrostatic generator (SC-PME50, Cosmic International Co. Ltd., New Taipei City, Taiwan). The storage protocols and conditions were followed as described by Hsieh et al. (2011) with some modification. All analytical grade reagents were purchased from Sigma-Aldrich® (Merck KGaA, Darmstadt, Germany).
2.2. Rigor mortis index
The fish rigor index was measured, following the method of Kiessling et al. (2006) and Le et al. (2020). Each fish was placed on the platform’s edge at 1/2 length to determine the length of normal sagging (tail drop) and sagging during the rigor phase. The rigor index was then calculated using the following equation:
where, D0 is the length of normal sagging before the rigid phase, and D is the length of sagging in the rigor phase.
2.3. Determination of protein concentration and solubility
The solubility of the proteins in the samples was determined as described by Mohsenpour et al. (2023) with some modifications. In brief, 40 g of NaOH and 1 g of glycerin were added to 200 mL of distilled water. In another beaker, 2 g of CuSO4-5H2O was added to 200 mL of distilled water. Eventually, the two solutions were mixed, added with distilled water to a volume of 500 mL, and named Biuret reagent 1. Biuret reagent 2 was prepared by thoroughly mixing 40 g of NaOH and 1 g of glycerin with distilled water, followed by adding distilled water to a volume of 500 mL. The sample was separated into two tubes with 2 mL each, followed by the addition of 2 mL of Biuret 1 and 2, respectively, and then reacted at room temperature for 45 min. Absorbance at 550 mm was measured by a spectrophotometer (U-2000, Hitachi Ltd., Tokyo, Japan) and calculated by the following equation:
where, Ia is the absorbance of Biuret reagent 1 at 550 nm, Ib is the absorbance of the blank of Biuret reagent 1 at 550 nm, IIa is the absorbance of Biuret reagent 2 at 550 nm, IIb is the absorbance of the blank of Biuret reagent 2 at 550 nm, and F2 is the calibration coefficient for protein concentration.
Albumin fraction V was prepared by adding 10 mM tris–HCl buffer to 20 mg/mL as standard and then diluting to different concentrations. Biuret method and Kjeldahl were used for determination. The correlation and correction coefficients between the two methods were obtained and applied to the above Biuret method equation.
Water-soluble protein was extracted in accordance with the method of Sarma et al. (2000). The sample was homogenized for 3 min with 5 g of sample and 95 g of distilled water. Salt-soluble protein extract was performed as described by Eady et al. (2014). The sample was homogenized with 95 mL of 5% NaCl (pH regulated to 7.2 with 0.02 M NaHCO) and 5 g of the sample for 3 min. Both homogenates were centrifuged at 4,500 × g for 15 min. The supernatants were added with distilled water and 0.02 M NaHCO to a volume of 100 mL. Protein concentrations were determined as described in the previous section. Total protein solubility, namely, the sum of water-soluble and salt-soluble protein, was expressed in g/100 g of fish meat.
2.4. Preparation of surimi gelatin and determination of gel strength
Surimi gel was prepared following the method described by Huang et al. (2022). The fish meat was adjusted to 80% water, minced at 4°C for 1 min, added with 2.5% NaCl, and poured into a steel hollow cylindrical container (inner diameter and height both 13 mm), which was sealed, placed in a hot water bath at 90°C for 20 min, cooled for 15 min in an ice bath, and allowed to stand at room temperature for 30 min. Finally, a surimi gel sample was obtained. The measurement was performed at room temperature (about 25°C) with a tissue analyzer (CR-200D, Sun Scientific Co., Ltd., Japan) under the following conditions: using Model 1 and a spherical plunger (No. 2) of 5 mm diameter, loading table was raised at a speed of 150 mm/min, and the penetration level was 90% of the sample height. Meanwhile, the gel strength (g × mm) was obtained as breaking force × breaking strain.
2.5. Water holding capability
The WHC was determined using the method reported by Rybicka et al. (2022). In brief, 5 g of sample was wrapped with four sheets of Whatman grade 4 filter paper (150 mm circle, Whatman International Ltd., Giles, Buckinghamshire, United Kingdom), placed in a centrifuge tube, and centrifuged at 3,000 × g for 30 min at 4°C. The filter paper was removed, excess water from the sample’s surface was aspirated, and the sample was weighed. The WHC was calculated with the following equation:
2.6. Determination of K value, adenosine 5′-triphosphate (ATP), and its related compounds
The K value, ATP, and its related compounds were determined following the method described by Kuda et al. (2008) and Yin M. et al. (2022) with some modifications. In brief, 5 g of fish meat was homogenized with 25 mL of 6% perchloric acid (PCA) at 4°C for 3 min, followed by centrifugation at 3000 × g at 4°C for 10 min. NaOH was used to regulate the pH to 6.5–6.8, and 20 mL of supernatant was filtered through a 0.45 μm membrane in an ice bath for 30 min. In the end, the solution was added with deionized water to a volume of 50 mL and then frozen (−70°C) until use. A Hitachi Model L5000 high-performance liquid chromatography (HPLC) with a Might RP-18 column (5 μm, 250–4.6 mm end-capped, Kanto Chemical Co., Inc. Tokyo, Japan) was used. The mobile phase was 0.06 M K2HPO4–0.04 M KH2PO4 (pH 4.0), and the flow rate was 1 m/min. The detector was a UV detector at a wavelength of 254 mm. The sample injection volume was 10 μL. The concentration of each product was calculated according to the relationship between the retention time, concentration, and peak area of the standard product. The percentage of H × R (inosine) and Hx (hypoxanthine) to the total amount of ATP-related compounds was determined. A low K value indicates the good freshness of the sample. The freshness index K value was calculated using the following equation:
2.7. Determination of protein molecular composition with sodium dodecyl sulfate-polyacrylamide gel electrophoresis (SDS-PAGE)
Protein composition was analyzed following the method described by Bradford (1976). In brief, 0.2 g of fish sample was added to 5 mL of 62.5 mM Tris–HCl (pH 6. 8) solution (containing 8 M urea, 1% SDS, 1% 2-mercaptoethanol, 2 mM EDTA and 0.5 mM phenylmethylsulfonyl fluoride) and dissolved at room temperature (25°C) for 24 h. The sample was centrifuged at 900 g for 5 min, and 1 mL of the supernatant was added with two drops of tracking dye and heated at 100°C for 5 min to form the sample solution, which was then stored at −20°C until use. Each lane was loaded with 5 μL of the sample, followed by the chamber buffer, and the voltage was set at 80 V. The protein standards were myosin of rabbit skeletal muscle (200.0 kDa), β-galactosidase of E. co1i (116.3 kDa), phosphorylase B of rabbit muscle (97.4 kDa), serum albumin of bovine (66.2 kDa), ovalbumin of hen egg white (45.0 kDa), carbonic anhydrase of bovine (31.0 kDa), trypsin inhibitor of soybean (21.5 kDa), lysozyme of hen egg white (14.4 kDa), and aprotinin of bovine pancrease (6.5 kDa).
2.8. Determination of enthalpy (∆H) change of actin and myosin in muscle
The method of Huang et al. (2022) and Nikoo et al. (2019) were adopted. In brief, 30–35 mg of the sample was loaded into a stainless-steel crucible. For the control group, the same amount of sample was added with 23–27 mg of distilled water [flesh fish’s water content of 73–77% (Cheng Y.-T. et al., 2023)]. The enthalpy change of actin and myosin in muscle was measured with a Differential Scanning Calorimetry (DSC92, KEP Technologies, Mougins- Sophia Antipolis, France) at a temperature increase rate of 5°C/min in the range of 8°C–100°C.
2.9. Determination of total viable count (TVC) and psychrophilic bacteria
TVC and psychrophilic bacteria contents were performed using the Cheng Y.-T. et al. (2023) and ISO (2019) methods, respectively. In brief, 10 g of fish meat was homogenized with 90 mL of sterile saline in stomacher® 400 Circulator Lab Blender (Seward Ltd., United Kingdom) at low and high speeds for 2 min each, followed by the addition of 1 mL of the homogenate to 9 mL of sterile saline, serial dilution, and dispersion of 1 mL of dilution into culture dishes. Following the pour plate count method, 15 mL of psychrophilic plate count agar was poured and incubated at 37 and 7°C for 2 and 10 days, and the results were expressed as log CFU/g fish meat.
2.10. Determination of pH
In brief, 5 g of fish meat and 45 mL of distilled water were homogenized for 2 min at 2,000 × g in a homogenizer (Brinkmann Polytron Pt-3000, Kinematica AG, Switzerland) and then measured by a pH meter (MP220, Mettler Toledo, Columbus, United States).
2.11. Texture analysis
The texture of the sample was analyzed using the method described by Li Q. et al. (2022) with some modifications. The sample was cut into cubes of approximately 1.5 cm and measured for breaking stress (g), breaking strain (cm), and breaking strength (g × cm) of rainbow trout meat by a texture analyzer TA-XT2 (Stable Micro Systems Ltd., United Kingdom). The P/30C conical probe determined the conditions with 90% penetration of the sample at a speed of 2 mm/s.
2.12. Observation of tissue microstructure
The samples were cut into cubes (2 cm3) and dipped in neutral formalin solution (10%, pH 7.0) at 4°C for 6 h, followed by paraffin embedding and cut into 4 μm thick sections of tissue slicing. The sliced samples were observed and photographed under a light microscope (Model: AFX-IIA, Nikon group Co. Tokyo, Japan) at a magnification of 10 × 10.
2.13. Statistical analysis
All the data in this study are presented using the mean ± standard deviation (SD), with three replicates for each condition (n = 3). The statistical analysis system (version 9.4, SAS Institute Inc., Cary, NC, United States) was used to analyze the differences between the means of each condition with a one-way ANOVA. Then, Duncan’s new multiple-range test was used to compare the significant differences among each group (p < 0.05).
3. Results and discussion
3.1. Rigor mortis characteristics of rainbow trout and quality changes during storage
3.1.1. Rigor mortis index
The occurrence of rigor mortis was characterized by the initiation of a rigor mortis process caused by biochemically advanced ATP depletion (below 2 μM). This test is a prerequisite for accurately controlling the quality of commercially available fish by determining adverse indicators (Delbarre-Ladrat et al., 2006). Improper treatment of different fish species in the pre-slaughter period possibly leads to the rapid deterioration of meat quality, decrease in muscle pH, WHC loss, protein denaturation, lipid oxidation, and increase in microbiota (Chytiri et al., 2004). The effects of storage temperature on the rigor mortis index of rainbow trout are shown in Figure 1A. Under 25°C storages, the maximum rigor mortis was obtained at 2.5 h, followed by the end of rigor mortis at 3.5 h. Only about 25% of rigor was available at 14 h. Under 4°C HVEF storage, the fish reached maximum rigor mortis between 12- and 24 h; resolution of rigor mortis release started after 24 h, and the degree of rigor was only about 43% after 96 h, and there were significant differences between all groups (p < 0.05). Under 0°C storage, the fish reached maximum rigor mortis in 6 h and lasted until 24 h, resolution of rigor mortis started afterward, and the degree of rigor mortis was about 57% after 96 h (p < 0.05). The above results showed that the rigor mortis occurred significantly faster at 25°C storage, while 4°C and 0°C declined after 36 h. This phenomenon can be attributed to the increased Mg-ATPase activity in myofibrils at 25°C, which contributed to the loss of sarcoplasmic reticulum (SR) function and resulted in the release of calcium ions that induced rigor mortis (Watabe et al., 1990). The fish stored at 0°C experienced the cold contraction effect, and the SR lost its function and released calcium ions, leading to rigor mortis. Notably, the 4°C group with HVEF storage obtained similar rigor mortis results as 0°C storage in a non-freezing condition (p < 0.05). The higher the storage temperature, the faster the rate of discharge of rigor mortis in the fish due to the release and activation of endogenous enzymes in the muscles that induce muscle tenderization (Moeller et al., 1977; Asghar and Bhatti, 1988). The meat of the rainbow trout slaughtered and fasted for 5 days had higher post-mortem pH at 0 h and earlier post-mortem rigor mortis compared with the trout that fasted for 3–4 days (Bermejo-Poza et al., 2017).
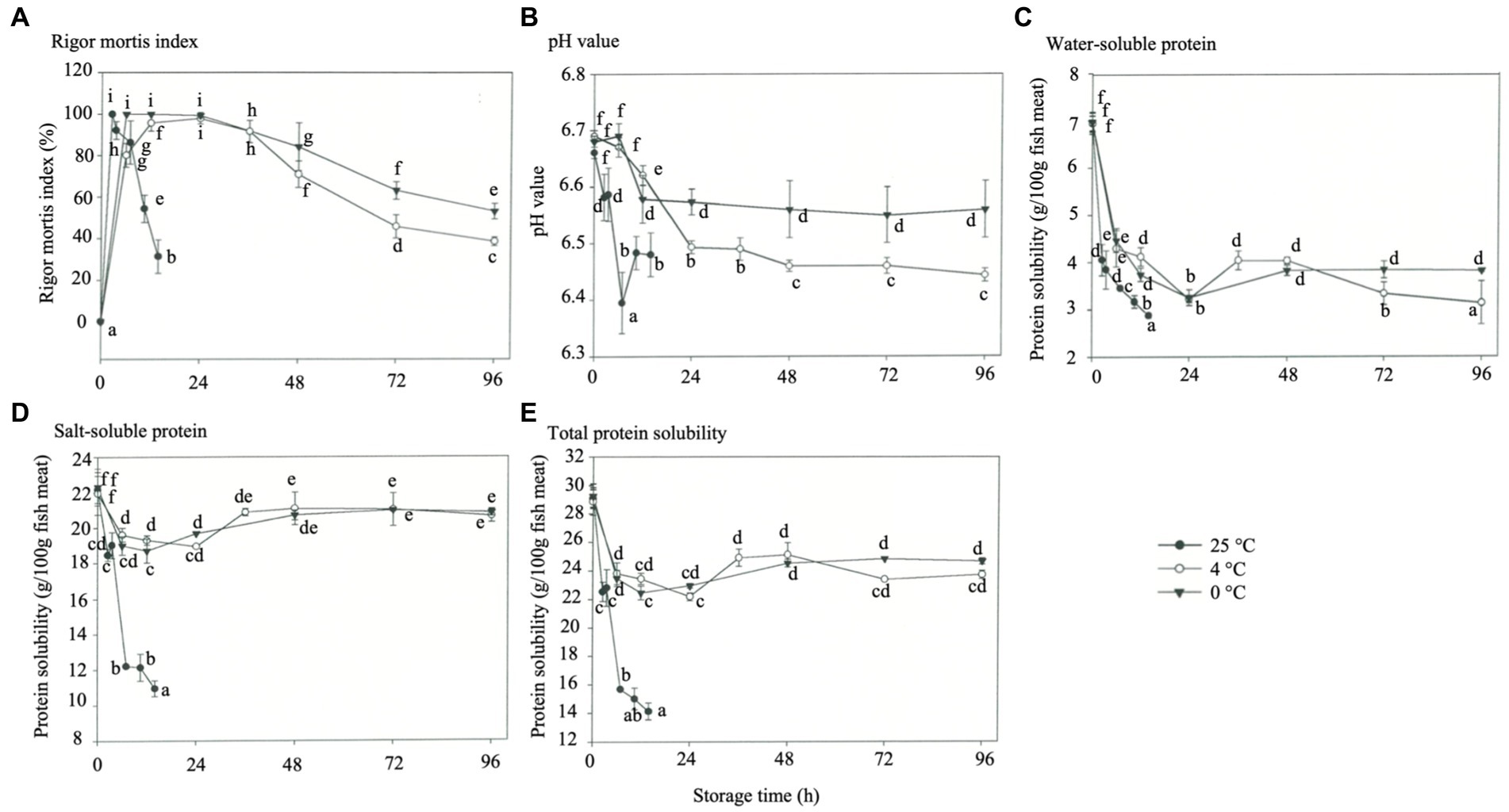
Figure 1. Effects of different storage temperatures on the physicochemical properties of rainbow trout. (A) Rigor mortis index. (B) pH value. (C) Water soluble protein. (D) Salt-soluble protein. (E) Total protein solubility.
3.2. Changes in pH
The effect of storage temperature on the pH of rainbow trout muscle is shown in Figure 1B. The pH of the fresh fish muscle was about 6.67. In the absence of oxygen, the glycogen in muscles would undergo anaerobic glycolysis, and lactic acid would have decreased the pH after the death of the fish. Meanwhile, hydrogen ions might have been generated from ATP decomposition (Kumar et al., 2012). The decrease in fish pH at each storage temperature occurred at or after the maximum post-mortem rigor mortis, and the reduction was significant at high storage temperatures. Okuma and Abe (1992) reported that muscle glycogen content decreased to the lowest level during maximum rigor mortis, and the produced lactic acid reduced the pH. In addition, a pH increase was observed at 25°C storage for 10.5 h, which was assumed to result from microbial multiplication, muscle protein hydrolysis, and amine generation during ATP hydrolysis. Under storage at 0°C and 4°C, the pH decreased at 16-24 h, while the pH of the 4°C group was kept at pH 6.5 after 24 h with similar results to the 0°C group (p < 0.05). This also implies that HVEF can maintain pH stability to avoid a rapid drop in pH while achieving the same results as the 0°C storage, which this study’s findings were consistent with those of Hsieh et al. (2011). Moreover, the pH decrease during storage was probably related either to the breakdown of glycogen, creatine phosphate, ATP, and lactic acid or the dissolution of carbon dioxide in fish muscles; while the carbon dioxide could be absorbed through the formation of carbonic acid and cause acidification (Ekonomou et al., 2020). Meanwhile, the subsequent increase in pH during storage was probably attributed to the growth of lactic acid bacteria and the production of volatile base substances (ammonia, trimethylamine, and other biogenic amines) by microbial or endogenous enzymes due to protein degradation (Jemmi and Keusch, 1992; Hsieh et al., 2011; Ko et al., 2016).
3.3. Effects of storage temperatures on protein solubility
One of the most common methods for assessing protein denaturation and aggregation is determining protein solubility (Mohsenpour et al., 2023). The effect of storage temperature on water-soluble protein (Figure 1C), salt-soluble protein (Figure 1D), and total protein solubility (Figure 1E) of rainbow trout was examined. Proteolysis represents an essential biochemical phenomenon in post-mortem fish and is fundamental in developing the tenderness and flavor of processed products (Delbarre-Ladrat et al., 2006). In this study, the water-soluble protein showed a dramatic decrease in solubility at the maximum post-mortem rigor mortis less than three storage temperatures (Figure 1C). When rigor mortis began to disappear, the solubility of water-soluble proteins increased only under 25°C storage (p < 0.05). This phenomenon can be attributed to muscle contraction during rigor mortis. Because the ribosomes (composed of many ribosomal protein subunits) in myofibroblasts are subjected to mechanical damage, cold stress, free radical attack, and other factors during refrigeration, these proteins are degraded, dissociated, or oxidized, while leads to the formation of differentially abundant proteins (including myosin-binding proteins, filamins, actinin, troponin, and muscle-restricted coiled-coil protein) (Shui et al., 2022). With the release of muscle rigidity, the water-soluble protein solubility increased again because of the weakening of muscle tissues. Under 4 days of storage, the solubility of the protein decreased again. During storage, the muscles’ free fatty acids and lipid oxides react hydrophobically with the protein surface, forming a hydrophobic zone that decreases the solubility (Giovambattista et al., 2008).
The solubility of salt-soluble proteins showed a decreasing trend during maximum rigor mortis under all three storage temperatures (Figure 1D). This phenomenon was presumably caused by forming a large molecular actin complex with myosin precipitated during centrifugation, leading to a decrease in solubility. However, the solubility of salt-soluble proteins showed a rebound at the initial stage of released rigor mortis, and this behavior can be attributed to the weakening of inter-actin bonding. Nevertheless, a slight decrease in solubility was observed at 4 days of storage, probably due to the initialization of protein denaturation. The solubility of salt-soluble proteins decreased under storage at 25°C while the muscles were unstiffened due to the denaturation of some proteins at this temperature. The solubility of total proteins decreased continuously under storage at 25°C, and that under 4°C and 0°C decreased during rigor mortis but increased at the beginning of resolution of rigor mortis (Figure 1E) (p < 0.05). However, this parameter decreased when the storage time increased, and the proteins denatured slightly, which was mainly evident under 4°C storage. Monovalent cations (such as Na+ and K+) have a decisive inhibitory effect on proteases (myostatin, dipeptidase, and aminopeptidase) that maintain muscle structure and preserve texture. Alternatively, transglutaminase activity was increased in NaCl, improving muscle firmness, cohesion, and elasticity in the product (Gomes et al., 2021). Some studies showed that high-voltage electric fields reduced the solubility of proteins in samples by affecting the structure of proteins (secondary and tertiary) or by degrading hydrogen bonds (Mohsenpour et al., 2023).
3.4. Gel strength properties
The gel was formed by the dissolution and dispersion of the actin of myofibril by salt, then hydrated in actin and denatured by heating, forming a 3D reticular gel (Levitsky et al., 2008). The effects of storage temperature on the gel breaking stress (Figure 2A), breaking strain (Figure 2B), and gel strength (Figure 2C) of rainbow trout gels were determined, and the results represented the hardness and elasticity of the gels. These two factors collectively indicate the strength of the gel. The gel-breaking stress, breaking strain, and gel strength decreased in all three storage temperatures when the muscles were rigid (Figures 2A–C), probably due to the rigid bonds formed by the muscle fibers. As a result, the actin and myosin were less soluble, which caused a poor gel structure with heating. The lowest gel strength was observed during the maximum rigor of rainbow trout stored at 25°C (p < 0.05), which was assumed to be caused by the synergistic effect of muscle rigor and thermal denaturation. For rainbow trout, the gel-breaking stress, breaking strain, and gel strength decreased slightly at three storage temperatures when the muscles started to unstiffen. This phenomenon can be attributed to the severe rigidity bonding between the myofibers and the weakening of muscle tissue during storage (Chan et al., 2022). Notably, HVEF treatment avoided degradation of proteins (myosin and actin) in the filets (Xie et al., 2021), and Qian et al. (2022) reported that treatment with 10 and 15 kV/m slowed the deterioration of texture properties (hardness and elasticity) during refrigeration, presumably related to protease activity inhibition.
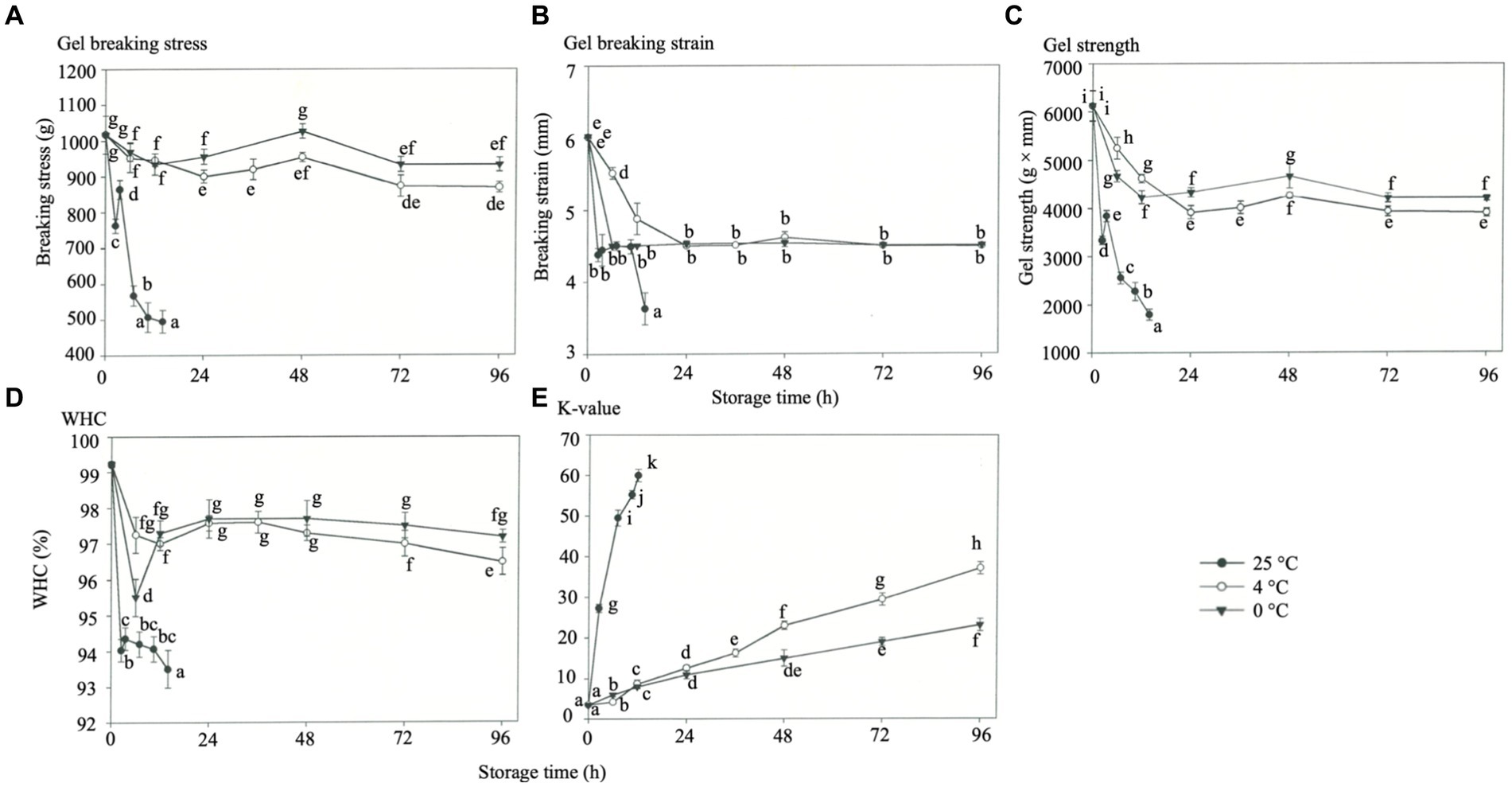
Figure 2. Effects of different storage temperatures on the gel strength, water holding capacity (WHC), and K-value of rainbow trout muscles. (A) Gel breaking stress. (B) Gel breaking strain. (C) Gel strength. (D) WHC. (E) K-value.
Moreover, a slight increase in actin and myosin solubility was observed in the post-rigor period. The gel breaking stress, breaking strain, and gel strength decreased again at the end of rigor mortis, possibly due to endogenous enzymes and storage temperature causing the gradual denaturation of muscle proteins. The higher the storage temperature, the more evident is the phenomenon.
3.5. Water-holding capacity
The water content supports a range of biochemical, microbiological, and physical reactions that affect fish’s sensory, nutritional, and functional properties during processing and storage. Thereby, the WHC serves as an indicator to assess the quality of fish (Chan et al., 2022; Mohsenpour et al., 2023). The effects of storage temperature on the WHC of rainbow trout were observed (Figure 2D). The results showed that the WHC of rainbow trout muscle decreased during rigor mortis at all three storage temperatures. The most significant decrease in WHC was observed at 25°C and 0°C due to heat and cold shrinkage (p < 0.05). When rigidification was resolved, the WHC at 25°C continued to decrease. By contrast, the WHC at 0°C and 4°C regained stability, except for the decrease caused by protein denaturation within 48 h (p < 0.05). In addition, Huang et al. (2020) reported improved WHC of catfish filets with HVEF treated during storage at 4°C, related to smaller overall gaps between muscle fibers. The principal fluid loss in fatty fish, such as salmon and rainbow trout, mainly comprises water because the WHC prevents fluid and potential nutrient loss while increasing product yield throughout the entire value chain, thereby improving quality (Ofstad et al., 1995). For producers, a high WHC results in low drip loss and great protein functionality and profitability, which can be translated to a good appearance, juiciness, and texture (Chan et al., 2022).
3.6. K-value and content of ATP with its related compounds
K-value determines the freshness and spoilage of aquatic products according to the variation of nucleotides in post-mortem fish (Prabhakar et al., 2020). Notably, less than 20% K-values would be considered “sashimi” quality, while values from 20 to 60% would be acceptable, while 60% represents an unacceptable threshold (Hsieh et al., 2011). The effect of storage temperature on the K value of rainbow trout was determined (Figure 2E), and the K-value of fresh rainbow trout was around 4.5%. The maximum K-value of rigor mortis was about 12% for 24 h storage at 4°C and 0°C, respectively, while increasing to 26.5% for storage at 25°C. Regardless of the storage temperature, the K-value of rainbow trout showed a significant increase at the end of rigor mortis. However, the K-value of rainbow trout increased to 61% when stored at 35°C after 14 h, reaching the level of spoilage, and increased to 23 and 37% when stored for 4 days at 0°C and 4°C, respectively (p < 0.05), while this study’s results agreed with Hsieh et al. (2011). Despite treatment with the higher HVEF (300–900 kV/m), the fish’s K-value deteriorated after the fourth day (Ko et al., 2016). Interestingly, the high HVEF (600–900 kV/m) treatment on fish filets has been reported to affect the metabolic reactions with reduced ATP degradation, protein denaturation, microbial growth, and oxidative degradation, which effectively extended the shelf life of fish filets at 4°C for at least 8 days (Ko et al., 2016). The above results showed that the K-value of rainbow trout increased slowly during rigor mortis when stored at 4°C and 0°C. A possible increase in endogenous enzymes caused by the weakening of muscle tissues enhanced nucleotide breakdown at the end of rigor mortis (Nie et al., 2022). The K-value showed a relatively significant increase after the fish reached maximum rigor mortis (Okuma and Abe, 1992). When stored at 25°C, the rainbow trout showed a linear, rapid increase in muscle status and K-value due to microbial growth, thermal denaturation, and endogenous enzyme activation.
Watabe et al. (1989) showed that the ATP content of fish at maximum post-mortem rigor mortis typically decreased to less than 1 μmol/g, and ADP and AMP showed a consistent decreasing trend. Moreover, IMP accumulated to the highest level at maximum rigor mortis in all the fish stored at 25°C. The ATP and ADP of fish disappeared rapidly, and IMP accumulated mainly within a short period post-mortem (Prabhakar et al., 2020). IMP contributes significantly to the taste of aquatic products (Sarower et al., 2012). The ATP content of rainbow trout decreased significantly at the initial stage at all three storage temperatures (Figures 3A–F). This study showed that rainbow trout stored at low temperatures of 0°C and 4°C had better taste during post-mortem rigor mortis than fish stored at other temperatures (p < 0.05). Therefore, applying an electric field to filets may influence their metabolic reactions and reduce the degradation of ATP (Ko et al., 2016). However, the IMP content decreased with the end of rigor mortis over 24 h of storage, and the content of spoilage factors inosine and hypoxanthine rapidly increased, indicating that the degree of freshness decreased quickly. Okuma and Abe (1992) reported that IP started degradation at maximum post-mortem rigor mortis with inosine and hypoxanthine (Hx) formation. When the rainbow trout was stored at 25°C, the content of inosine and hypoxanthine increased rapidly and hence was the main reason for the rapid decrease in freshness.
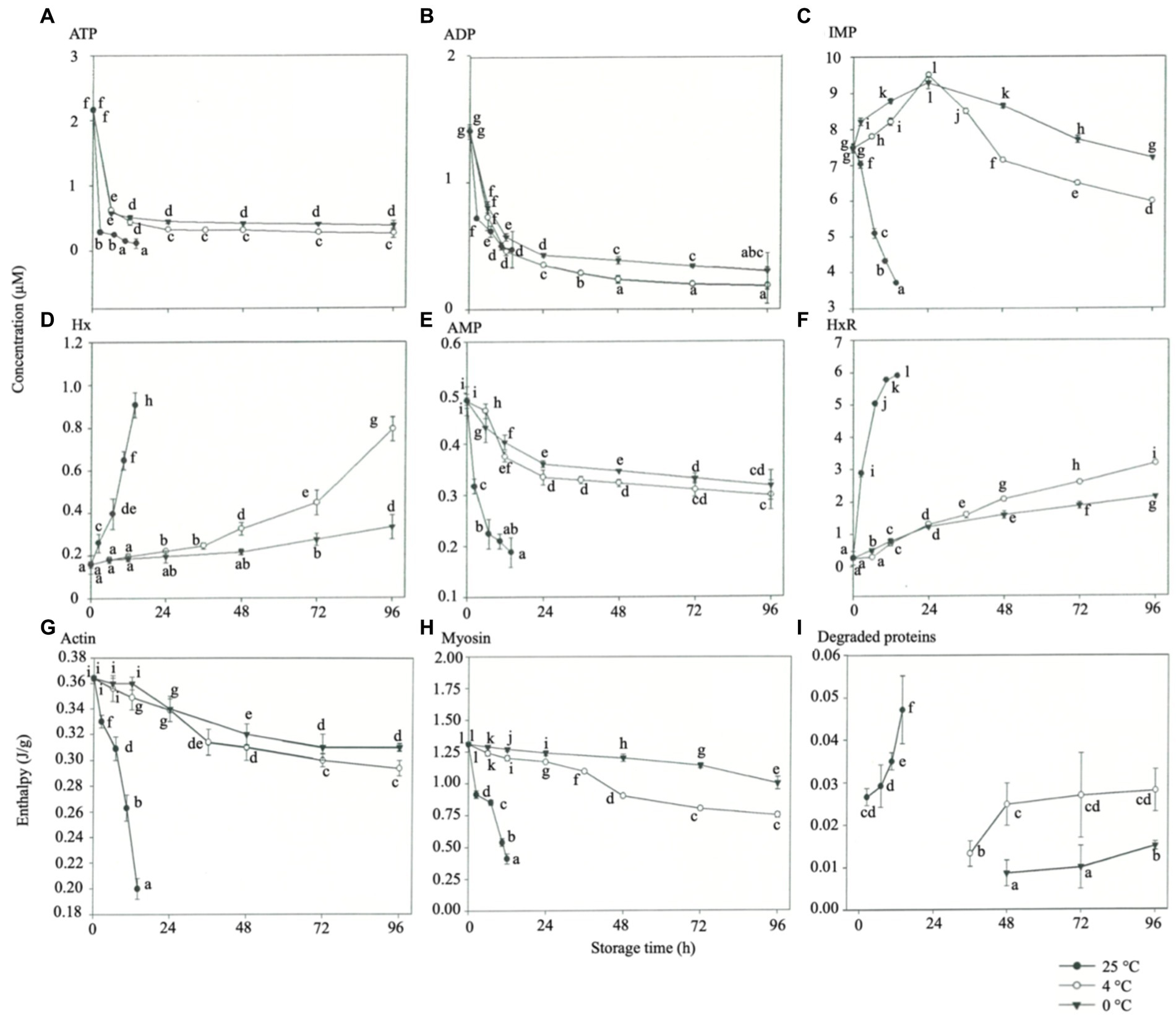
Figure 3. Effects of different storage temperatures on the content of ATP-related compounds and enthalpy of various proteins in rainbow trout. (A) ATP. (B) ADP. (C) IMP. (D) Hx. (E) AMP. (F) HxR. (G) Actin. (H) Mysoin. (I) Degraded proteins.
3.7. The enthalpy changes in muscle
The DSC-measured enthalpy values express the net content of the protein structure (Minikel, 2014; LibreTexts, 2023). The majority of water in the muscle resides in the spaces of the muscle fibers (thin and thick filament interstices), so myosin and actin are associated with the WHC of the fish, the texture of the fish products, and the functional properties of the surimi (Offer and Trinick, 1983; Nikoo et al., 2019). The effects of storage temperature on the enthalpies of actin (Figure 3G), myosin (Figure 3H), and degraded proteins (Figure 3I) of rainbow trout muscle were observed. The thermal denaturation temperatures of actin and myosin were 75°C and 53°C, respectively, which gradually increased to 85°C and 63°C, respectively (p < 0.05), while the rainbow trout was in post-mortem rigor mortis. Regardless of the storage temperature for the rainbow trout, the heat absorption wave similar to that of degraded proteins appeared at 42°C. This finding may be attributed to the formed rigidity of the muscle fibers as a potent complex of actin and myosin following the increase of storage time and the arrival of multiple heat absorption peaks between 20°C and 50°C presumably due to the thermal denaturation and enzymatic hydrolysis of the proteins into small molecule proteins. The enthalpy of actin and myosin in the rainbow trout decreased smoothly during rigor mortis at 4°C and 0°C storage (p < 0.05). It was significantly reduced at the end of rigor mortis, with the most apparent protein denaturation occurring at 4°C storage and a rapid decrease in the enthalpy of actin and myosin at 25°C storages (p < 0.05). Based on the above results, the proteins are less likely to be denatured during rigor mortis, and protein denaturation would occur significantly as rigor mortis progressed. In addition, the degradation of proteins occurred at 2.5, 36, and 48 h under storage at 25°C, 4°C, and 0°C, respectively, and the heat absorption peaks of proteins increased with the storage temperature (Figure 3I) (p < 0.05). The possible reason might be the increased activity of endogenous enzymes in rainbow trout at high temperatures, resulting in pronounced protein hydrolysis (Moeller et al., 1977). Kaneniwa et al. (2000) and Kaneniwa et al. (2004) showed that the enzymatic hydrolysis of muscle proteins in carp and rainbow trout occurred more significantly under storage at 20°C than at 0°C.
3.8. Protein molecular composition via SDS-PAGE
The protein compositions of rainbow trout stored at 25°C (Figure 4A), 4°C (Figure 4B), and 0°C (Figure 4C) were analyzed. The results showed that the molecular weight of rainbow trout’s 66 kDa protein belt decreased after storage at 25°C for 14 h. The molecular weights of 37.9, 35.6, 33.3, 19.5, and 6.5 kDa protein belts also decreased after 10.5 h. The most significant disappearance was observed for the 6.5 kDa protein. Furthermore, the molecular weights of 66, 37.9, 35.6, and 33.3 kDa protein belts decreased after storage at 4°C for 96 h. At 72 h, the molecular weights of 19.5 and 6.5 kDa protein belts decreased, and the rate of the disappearance of 6.5 kDa proteins was the most significant. No significant changes in either protein belt were observed for storage at 0°C for 96 h. Bauchart et al. (2007) reported few protein hydrolyses in rainbow trout muscle, remarkably preserved at 0°C, which agreed with this study. Therefore, it is possible that the degeneration of the myofibrillar proteins was caused by the rigor mortis of the rainbow trout, in which the protein contents were best maintained at 0°C, followed by 4°C during preservation. However, the 30 kDa protein formed by troponin-T degradation in post-mortem muscle during storage has been reported (Ho et al., 1994; Gagaoua et al., 2021). Moreover, several studies have reported that the myofibrillar proteins degraded to myosin heavy chain (MHC) (∼200 kDa), which gradually degraded or even disappeared with time, where the protein belts of 66 kDa and 33.3 kDa were probably the degradation products of MHC (Ge et al., 2018). In addition, the other proteins were para myosin (∼100 KDa), tropomyosin (37.9 KDa), troponin-T (35.6 kDa), and Myosin Light Chain (MLC ∼ 17 KDa) (Wang et al., 2011; Li et al., 2017), which were also consistent with this study. A similar phenomenon was observed for stored goat muscle in which MHC, troponin T, desmin, and actin were lost by the hydrolysis by endogenous enzyme in the muscles (Xiao et al., 2022). The disappearance of protein belts may be attributed to the hydrolysis of endogenous proteolytic enzymes in muscles, which was consistent with the findings of Alarcón et al. (2001) and Schreurs et al. (1995). Likewise, under refrigerated conditions, the dissociation and fragmentation of myofibrillar proteins occurred in muscle tissue resulting from endogenous cathepsin, calpain, several serine proteases, and bacterial growth (Ge et al., 2018; Xie et al., 2019; Shui et al., 2022). Alternatively, the high protein denaturation at high temperatures, even at low pH, induced high enzymatic hydrolysis activity (Shenouda, 1980; Xiong, 1997; Nielsen and Jørgensen, 2004; Quevedo et al., 2021). Incidentally, Zhang et al. (2021) have also reported that fresh ground pork at high salt (2 M KCl) raises the WHC of myofibrils such that the degeneration of myofibrillar proteins during freezing has not reproduced; in contrast, treatment with low pH and high salt decreased WHC; it increased the hydrophobicity of the surface, indicating that myofibrillar protein denaturation has occurred by a mechanism similar to freeze-thawing.
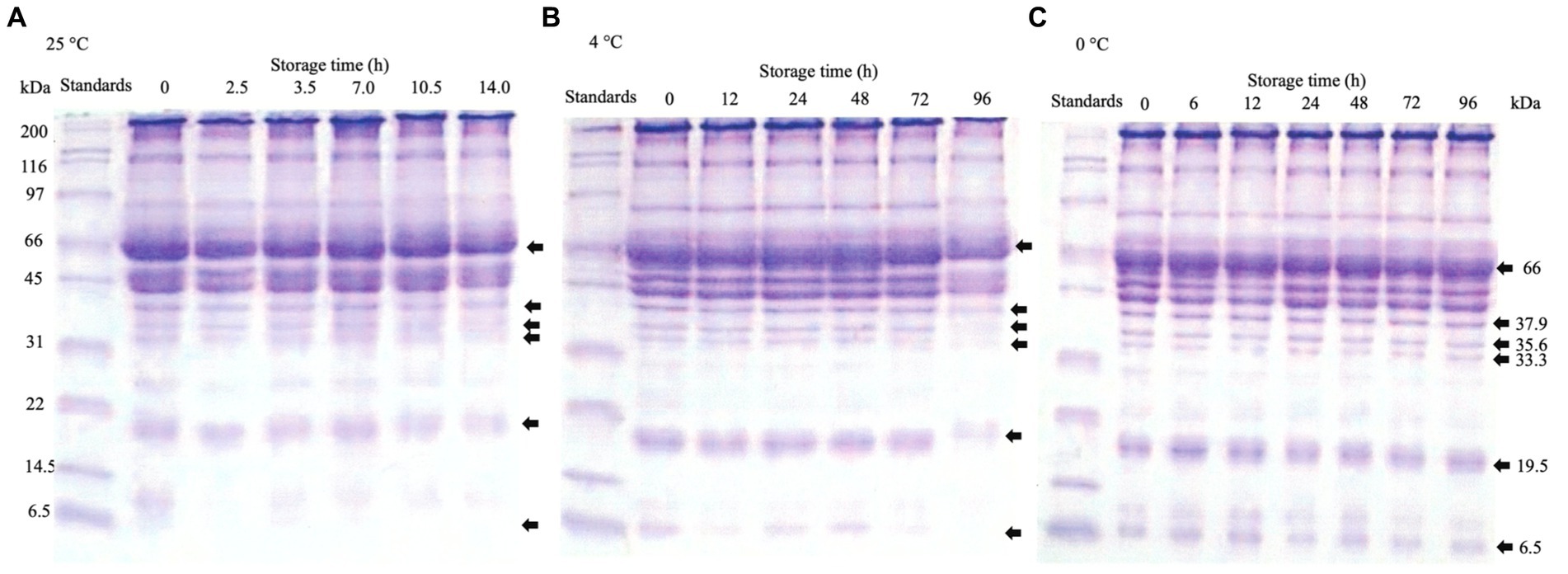
Figure 4. Effects of different storage temperatures on the SDS-PAGE analysis of rainbow trout proteins. (A) 25°C. (B) 4°C. (C) 0°C.
3.9. Observation of rainbow trout tissue sections
The tissue sections of rainbow trout during storage at 25°C (Figure 5A), 4°C (Figure 5B), and 0°C (Figure 5C) were observed. The fresh tissues were intact under storage at 25°C (0 h), while the gaps between the muscle bundles appeared after 2.5 h (post-mortem rigor mortis); subsequently, after 7 h (the end of rigor mortis), the gaps widened and increased, causing the separation of connections between the muscle bundles (Figure 5A). The rainbow trout showed small gaps in the muscle fibers when stored at 4°C for 24 h (rigor mortis stage). At the end of rigor mortis stage, the gaps increased gradually. After 96 h, the muscle fibers partially separated (Figure 5B), consistent with the above results (Figure 5A). The fish stored at 0°C for 24 h (rigor mortis phase) showed minimal gaps in the muscle fibers compared with those stored at 25°C and 4°C (Figure 5C). According to the report by Huang et al. (2020), the muscle fiber gap in catfish filets decreased significantly after undergoing HVEF treatment for 15 min and being stored at 4°C for 0, 3, and 7 days, while the discovery differs from this study, possibly due to differences in fish species, treatment intensity, and duration. Moreover, at the end of rigor mortis, the gaps in muscle fibers increased with storage time. Nevertheless, the muscle tissue integrity was significantly better when stored at 0°C than at 25°C and 4°C. The above results showed that the muscle trout in rigor mortis maintained its muscle tissues’ integrity except for those stored at 25°C. However, spaces between the muscle fibers began to appear, which might be caused by the cavitation effect (Ma et al., 2021). With the end of rigor mortis, the separation of the muscle fibers became evident. The increase in muscle fiber’s interstitial spaces was mainly associated with disrupting the Z-lines and related structures (Delbarre-Ladrat et al., 2006). The degradation of connective tissues in fish muscles occurs during storage, and the hypothesized primary cause of muscle fiber interstices and muscle tenderization is the action of endogenous muscle enzymes, which causes the degradation of fish structural proteins (Hansen et al., 1996; Paludan-Müller et al., 1998; Nielsen and Jørgensen, 2004). Therefore, the muscular endogenous enzymes begin operating as soon as the fish dies. In addition, the end of rigor mortis promotes the action of endogenous enzymes with increased muscle tissue disintegration and denaturation (Warner, 2016). Hence, preservation temperature should be an important factor.
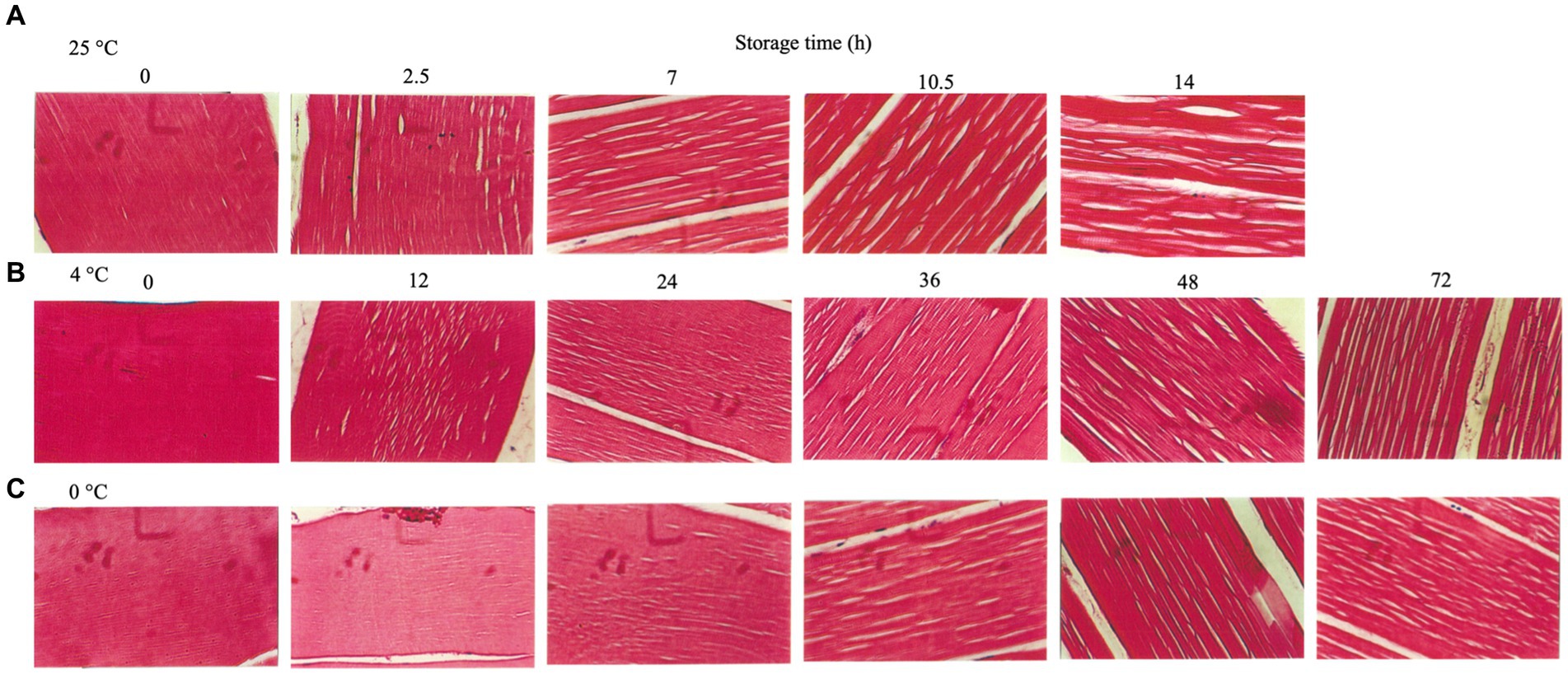
Figure 5. Muscle tissue sections of rainbow trout stored under different temperatures. (A) 25°C. (B) 4°C. (C) 0°C.
3.10. Effects of storage temperature on the TVC and psychrophilic bacteria
The shelf life may be reduced by 50% when the storage temperature increases from 0°C to 4°C because bacterial growth, which causes fish spoilage, is significantly affected by the storage temperature (Lorentzen et al., 2020). Moreover, Genç and Diler (2019) reported the predicted shelf life of rainbow trout with different storage temperatures of 2°C, 10°C, and 20°C as 8–15.7 days, 2.3–4.5 days, and 1–1.8 days, respectively, while the actual shelf life shortened rapidly. The effect of storage temperature on the TVC (Figure 6A) and psychrophilic bacteria (Figure 6B). TVC of fresh fish (0 h) was about 3.2 log CFU/g. Under 25°C storage, this value increased sharply with the storage time, reaching 6.45 log CFU/g after 14 h (p < 0.05). TVC increased slightly for samples stored at 4°C and 0°C for 96 h, with recorded readings of 3.56 and 3.40 log CFU/g, respectively (Figure 6A). During storage for 24 h, psychrophilic bacteria exhibited outstanding proliferation in the fraction compared to 0°C, at 4°C, with a significant difference (p < 0.05). However, the proliferation of psychrophilic bacteria in rainbow trout showed an increasing trend after 36 h of storage at 4°C, with 3.72 and 4.13 log CFU/g at 48 and 96 h, respectively (p < 0.05) (Figure 6B), implying a significant difference at 36–96 h (p < 0.05). HVEF treatment cannot wholly inhibit microorganisms, but it effectively reduces the growth rate of microorganisms during the shelf life of fish filets (Hsieh et al., 2011; Ko et al., 2016). Compared with medium- and high-temperature bacteria, psychrophilic bacteria are sensitive to temperature change.
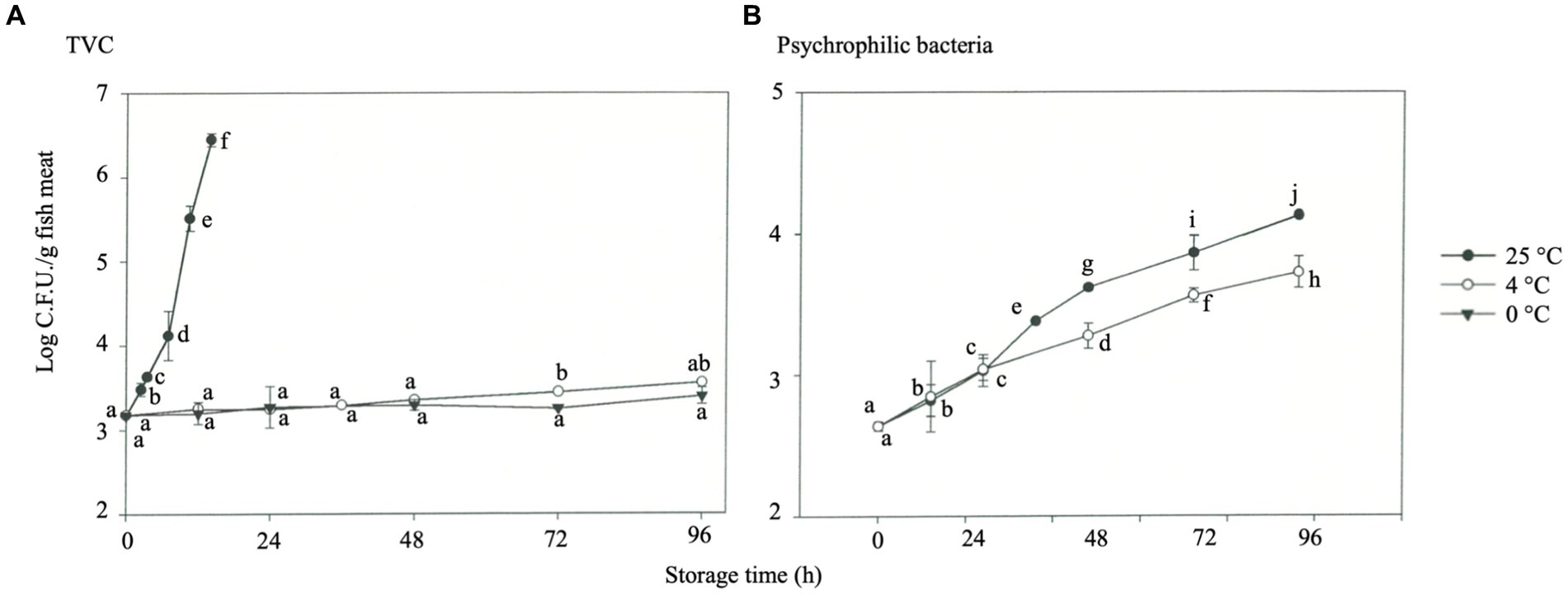
Figure 6. Microbial growth in rainbow trout stored under different temperatures. (A) TVC. (B) Psychrophilic bacteria.
Chytiri et al. (2004) have been indicated that the mesophilic counts of fileted and uncut rainbow trout were over 7 log CFU/cm2 after 10 and 18 days of ice storage. Meanwhile, more biotic bacteria were found in fileted fish during storage than in whole unshelled trout, probably due to the cross-contamination of trout samples during fileting; similar results were reported by Tavakoli et al. (2018). Temperature fluctuations also accelerate the loss of polyunsaturated fatty acids and increase unpleasant odors associated with water migration (Yu et al., 2020). In practice, this realization is difficult to achieve because the cold chain in transportation operations between the producer and the final consumer is regularly interrupted (Skawińska and Zalewski, 2022). Hence, attention should be paid to the temperature change during the low-temperature storage of rainbow trout.
4. Conclusion
This study found that rainbow trout stored at different temperatures underwent post-mortem rigor mortis and resolution of rigor mortis at different rates, leading to changes in rainbow trout muscles. The rainbow trout specimens stored at 25°C and 0°C were more susceptible to rigor mortis than those stored at 4°C due to shrinkage effects (heat and cold). In addition, both 4°C (treated with 100 kV/m HVEF) and 0°C storage only effectively inhibited the growth of TVC and psychrophilic bacteria. However, the post-mortem rigor mortis, which occurred under 4°C and 0°C storage, caused the degradation of the total protein solubility and WHC of the fish, serving as a warranty for good freshness and quality. Moreover, the endogenous enzymatic activity of rainbow trout at 25°C was higher than that at 4°C and 0°C storage, resulting in easy resolution of rigor mortis accompanied by microbial growth, while spoilage also occurred within 14 h at 25°C storages. By contrast, rainbow trout’s freshness and hygienic quality were within the acceptable range when stored at 4°C (with HVEF at 100 kV/m) and 0°C for 4 days. This study revealed rainbow trout’s physicochemical and microbiology after storage at different temperatures and treated methods. These findings provide researchers and producers with valuable information for scheduling production schedules for stable products, extending food shelf-life through processing (e.g., smoked or frozen after conditioning), and expanding the opportunities for aquaculture development.
Data availability statement
The original contributions presented in the study are included in the article/supplementary material, further inquiries can be directed to the corresponding authors.
Author contributions
Y-TC, P-HH, W-CL, S-CC, P-MW, W-CK, and P-HL: conceptualization. P-HL: data curation. P-HH, W-CL, S-CC, P-MW, and P-HL: formal analysis and investigation. Y-TC and W-CK: funding acquisition. P-HH, W-CL, P-MW, and P-HL: methodology and validation. Y-TC and W-CK: project administration. Y-TC, W-CL, S-CC, and P-HL: resources. Y-TC, P-HH, W-CL, S-CC, P-MW, and P-HL: software. W-CK: supervision and visualization. P-HH and P-HL: writing – original draft and writing – review & editing. All authors contributed to the article and approved the submitted version.
Funding
This research was financially supported by Taichung Veterans General Hospital and by Rong Sing Medical Foundation, Taiwan. This research was also supported by grants provided by from the Ministry of Science and Technology (MOST 111-2313-B-126-002) in Taiwan.
Conflict of interest
The authors declare that the research was conducted in the absence of any commercial or financial relationships that could be construed as a potential conflict of interest.
Publisher’s note
All claims expressed in this article are solely those of the authors and do not necessarily represent those of their affiliated organizations, or those of the publisher, the editors and the reviewers. Any product that may be evaluated in this article, or claim that may be made by its manufacturer, is not guaranteed or endorsed by the publisher.
References
Alarcón, F. J., Moyano, F. J., and Díaz, M. (2001). Use of SDS-page in the assessment of protein hydrolysis by fish digestive enzymes. Aquac. Int. 9, 255–267. doi: 10.1023/A:1016809014922
Asghar, A., and Bhatti, A. R. (1988). “Endogenous proteolytic enzymes in skeletal muscle: their significance in muscle physiology and during postmortem aging events in carcasses” in Advances in Food Research. eds. C. O. Chichester, E. M. Mrak, and B. S. Schweigert, vol. 31 (Cambridge, MA: Academic Press), 343–451.
Barrientos, C., Tapia, J., Bertrán, C., Peña-Cortés, F., Hauenstein, E., Fierro, P., et al. (2019). Is eating wild rainbow trout safe? The effects of different land-uses on heavy metals content in Chile. Environ. Pollut. 254:112995. doi: 10.1016/j.envpol.2019.112995
Bauchart, C., Chambon, C., Mirand, P. P., Savary-Auzeloux, I., Rémond, D., and Morzel, M. (2007). Peptides in rainbow trout (Oncorhynchus mykiss) muscle subjected to ice storage and cooking. Food Chem. 100, 1566–1572. doi: 10.1016/j.foodchem.2005.12.023
Bermejo-Poza, R., De la Fuente, J., Pérez, C., González de Chavarri, E., Diaz, M. T., Torrent, F., et al. (2017). Determination of optimal degree days of fasting before slaughter in rainbow trout (Oncorhynchus mykiss). Aquaculture 473, 272–277. doi: 10.1016/j.aquaculture.2017.01.036
Bienkiewicz, G., Tokarczyk, G., Czerniejewska-Surma, B., and Suryn, J. (2019). Changes in the EPA and DHA content and lipids quality parameters of rainbow trout (Oncorhynchus mykiss, Walbaum) and carp (Cyprinus carpio, L.) at individual stages of hot smoking. Heliyon 5:e02964. doi: 10.1016/j.heliyon.2019.e02964
Bradford, M. M. (1976). A rapid and sensitive method for the quantitation of microgram quantities of protein utilizing the principle of protein-dye binding. Anal. Biochem. 72, 248–254. doi: 10.1016/0003-2697(76)90527-3
Carlucci, D., Nocella, G., De Devitiis, B., Viscecchia, R., Bimbo, F., and Nardone, G. (2015). Consumer purchasing behaviour towards fish and seafood products. Patterns and insights from a sample of international studies. Appetite 84, 212–227. doi: 10.1016/j.appet.2014.10.008
Chan, S. S., Roth, B., Jessen, F., Jakobsen, A. N., and Lerfall, J. (2022). Water holding properties of Atlantic salmon. Compr. Rev. Food Sci. Food Saf. 21, 477–498. doi: 10.1111/1541-4337.12871
Cheng, Y.-T., Huang, P.-H., Lu, W.-C., Chu, S.-C., Wang, P.-M., Ko, W.-C., et al. (2023). Creating added-value filet product from rainbow trout (Oncorhynchus mykiss) by salting and smoking method: physicochemical and textural attributes. Front. Sustain. Food Syst. 7:112844. doi: 10.3389/fsufs.2023.1153862
Cheng, H., Wang, J., and Xie, J. (2023). Progress on odor deterioration of aquatic products: characteristic volatile compounds, analysis methods, and formation mechanisms. Food Biosci. 53:102666. doi: 10.1016/j.fbio.2023.102666
Chytiri, S., Chouliara, I., Savvaidis, I. N., and Kontominas, M. G. (2004). Microbiological, chemical and sensory assessment of iced whole and filleted aquacultured rainbow trout. Food Microbiol. 21, 157–165. doi: 10.1016/S0740-0020(03)00059-5
Delbarre-Ladrat, C., Chéret, R., Taylor, R., and Verrez-Bagnis, V. (2006). Trends in postmortem aging in fish: understanding of proteolysis and disorganization of the myofibrillar structure. Crit. Rev. Food Sci. Nutr. 46, 409–421. doi: 10.1080/10408390591000929
Du, X., Wang, B., Li, H., Liu, H., Shi, S., Feng, J., et al. (2022). Research progress on quality deterioration mechanism and control technology of frozen muscle foods. Compr. Rev. Food Sci. Food Saf. 21, 4812–4846. doi: 10.1111/1541-4337.13040
Eady, M., Samuel, D., and Bowker, B. (2014). Effect of pH and postmortem aging on protein extraction from broiler breast muscle. Poult. Sci. 93, 1825–1833. doi: 10.3382/ps.2013-03619
Ekonomou, S. I., Bulut, S., Karatzas, K. A. G., and Boziaris, I. S. (2020). Inactivation of Listeria monocytogenes in raw and hot smoked trout fillets by high hydrostatic pressure processing combined with liquid smoke and freezing. Innovative Food Sci. Emerg. Technol. 64:102427. doi: 10.1016/j.ifset.2020.102427
Fox, M., Mitchell, M., Dean, M., Elliott, C., and Campbell, K. (2018). The seafood supply chain from a fraudulent perspective. Food Secur. 10, 939–963. doi: 10.1007/s12571-018-0826-z
Freitas, J., Vaz-Pires, P., and Câmara, J. S. (2020). From aquaculture production to consumption: freshness, safety, traceability and authentication, the four pillars of quality. Aquaculture 518:734857. doi: 10.1016/j.aquaculture.2019.734857
Gagaoua, M., Terlouw, E. M. C., Mullen, A. M., Franco, D., Warner, R. D., Lorenzo, J. M., et al. (2021). Molecular signatures of beef tenderness: underlying mechanisms based on integromics of protein biomarkers from multi-platform proteomics studies. Meat Sci. 172:108311. doi: 10.1016/j.meatsci.2020.108311
Ge, L., Xu, Y., Xia, W., and Jiang, Q. (2018). Synergistic action of cathepsin B, L, D and calpain in disassembly and degradation of myofibrillar protein of grass carp. Food Res. Int. 109, 481–488. doi: 10.1016/j.foodres.2018.04.067
Genç, I. Y., and Diler, A. (2019). Development of shelf life prediction model in rainbow trout stored at different temperatures. J. Aquat. Food Prod. Technol. 28, 1027–1036. doi: 10.1080/10498850.2019.1682734
Giovambattista, N., Lopez, C. F., Rossky, P. J., and Debenedetti, P. G. (2008). Hydrophobicity of protein surfaces: separating geometry from chemistry. Proc. Natl. Acad. Sci. 105, 2274–2279. doi: 10.1073/pnas.0708088105
Gomes, M. D. S. A., Kato, L. S., Carvalho, A. P. A. D., Almeida, A. E. C. C. D., and Conte-Junior, C. A. (2021). Sodium replacement on fish meat products – A systematic review of microbiological, physicochemical and sensory effects. Trends Food Sci. Technol. 118, 639–657. doi: 10.1016/j.tifs.2021.10.028
Hansen, L. T., Gill, T., Røntved, S. D., and Huss, H. H. (1996). Importance of autolysis and microbiological activity on quality of cold-smoked salmon. Food Res. Int. 29, 181–188. doi: 10.1016/0963-9969(96)00003-8
Ho, C. Y., Stromer, M. H., and Robson, R. M. (1994). Identification of the 30 kDa polypeptide in post mortem skeletal muscle as a degradation product of troponin-T. Biochimie 76, 369–375. doi: 10.1016/0300-9084(94)90110-4
Hsieh, C.-W., Lai, C.-H., Lee, C.-H., and Ko, W.-C. (2011). Effects of high-voltage electrostatic fields on the quality of tilapia meat during refrigeration. J. Food Sci. 76, M312–M317. doi: 10.1111/j.1750-3841.2011.02218.x
Huang, P.-H., Chiu, C.-S., Lu, W.-C., and Li, P.-H. (2022). Effect of compositions on physicochemical properties and rheological behavior of gelatinized adzuki-bean cake (yokan). LWT 168:113870. doi: 10.1016/j.lwt.2022.113870
Huang, H., Sun, W., Xiong, G., Shi, L., Jiao, C., Wu, W., et al. (2020). Effects of HVEF treatment on microbial communities and physicochemical properties of catfish fillets during chilled storage. LWT 131:109667. doi: 10.1016/j.lwt.2020.109667
Hui, X., Wan, Y., Dong, H., Peng, J., Wu, W., Yang, X., et al. (2023). A promising insight into the inhibition of lipid oxidation, protein degradation and biogenic amine accumulation in postmortem fish: functional glazing layers of modified bio-polymer. LWT 177:114575. doi: 10.1016/j.lwt.2023.114575
ISO . (2019). ISO 17410:2019(en) Microbiology of the food chain — Horizontal method for the enumeration of psychrotrophic microorganisms. In Vol. ISO 17410:2019 : International Organization for Standardization. Geneva
Jemmi, T., and Keusch, A. (1992). Behavior of Listeria monocytogenes during processing and storage of experimentally contaminated hot-smoked trout. Int. J. Food Microbiol. 15, 339–346. doi: 10.1016/0168-1605(92)90067-D
Kaneniwa, M., Miao, S., Yuan, C., Lida, H., and Fukuda, Y. (2000). Lipid components and enzymatic hydrolysis of lipids in muscle of Chinese freshwater fish. J. Am. Oil Chem. Soc. 77:825. doi: 10.1007/s11746-000-0132-3
Kaneniwa, M., Yokoyama, M., Murata, Y., and Kuwahara, R. (2004). Enzymatic hydrolysis of lipids in muscle of fish and shellfish during cold storage. Adv. Exp. Med. Biol. 542, 113–119. doi: 10.1007/978-1-4419-9090-7_6
Kiessling, A., Helge Stien, L., Torslett, Ø., Suontama, J., and Slinde, E. (2006). Effect of pre- and post-mortem temperature on rigor in Atlantic salmon muscle as measured by four different techniques. Aquaculture 259, 390–402. doi: 10.1016/j.aquaculture.2005.11.008
Ko, W.-C., Yang, S.-Y., Chang, C.-K., and Hsieh, C.-W. (2016). Effects of adjustable parallel high voltage electrostatic field on the freshness of tilapia (Orechromis niloticus) during refrigeration. LWT Food Sci. Technol. 66, 151–157. doi: 10.1016/j.lwt.2015.10.019
Kuda, T., Fujita, M., Goto, H., and Yano, T. (2008). Effects of retort conditions on ATP-related compounds in pouched fish muscle. LWT Food Sci. Technol. 41, 469–473. doi: 10.1016/j.lwt.2007.02.018
Kumar, A., Kumar, S., Sharma, B. D., Mendiratta, S. K., and Patel, A. K. (2012). Functional meat and meat products: an overview. J. Adv. Vet. Res. 2, 313–325. doi: 10.1533/9780857092557.3.512
Le, T. T., Nguyen, H. T., and Pham, M. A. (2020). Rigor mortis development and effects of filleting conditions on the quality of Tra catfish (Pangasius hypophthalmus) fillets. J. Food Sci. Technol. 57, 1320–1330. doi: 10.1007/s13197-019-04166-x
Levitsky, D. I., Pivovarova, A. V., Mikhailova, V. V., and Nikolaeva, O. P. (2008). Thermal unfolding and aggregation of actin. FEBS J. 275, 4280–4295. doi: 10.1111/j.1742-4658.2008.06569.x
Li, H., Chen, Y., Li, M., Huang, J., Zu, X., Liao, T., et al. (2022). Effects of temporary rearing with organic selenium on the muscle flavor and texture properties of largemouth bass (Micropterus salmonides). Food Chem. 397:133747. doi: 10.1016/j.foodchem.2022.133747
Li, Q., Li, H.-T., Bai, Y.-P., Zhu, K.-R., and Huang, P.-H. (2022). Effect of thermal treatment on the physicochemical, ultrastructural, and antioxidant characteristics of Euryale ferox seeds and flour. Foods 11:2404. doi: 10.3390/foods11162404
Li, Q., Zhang, L., Lu, H., Song, S., and Luo, Y. (2017). Comparison of postmortem changes in ATP-related compounds, protein degradation and endogenous enzyme activity of white muscle and dark muscle from common carp (Cyprinus carpio) stored at 4°C. LWT 78, 317–324. doi: 10.1016/j.lwt.2016.12.035
LibreTexts . (2023). BiologyI module 4.4: Tertiary structure and protein stability. Available at: https://bio.libretexts.org/Under_Construction/OLI/Biochemistry/Unit_2%3A_Biochemistry/Module_4%3A_Protein_Structure/Module_4.4%3A_Tertiary_Structure_and_Protein_Stability
Listrat, A., Lebret, B., Louveau, I., Astruc, T., Bonnet, M., Lefaucheur, L., et al. (2016). How muscle structure and composition influence meat and flesh quality. Sci. World J. 2016:3182746. doi: 10.1155/2016/3182746
Lopes, I. G., and de Freitas, T. M. (2023). Fish consumption in Brazil: state of the art and effects of the COVID-19 pandemic. Aquaculture 574:739615. doi: 10.1016/j.aquaculture.2023.739615
Lorentzen, G., Ageeva, T. N., Heide, M., and Esaiassen, M. (2020). Temperature fluctuations in processing and distribution: effect on the shelf life of fresh cod fillets (Gadus morhua L.). Food Control 112:107102. doi: 10.1016/j.foodcont.2020.107102
Ma, X., Mei, J., and Xie, J. (2021). Effects of multi-frequency ultrasound on the freezing rates, quality properties and structural characteristics of cultured large yellow croaker (Larimichthys crocea). Ultrason. Sonochem. 76:105657. doi: 10.1016/j.ultsonch.2021.105657
Minikel, E. V. (2014). Molecular biology 02: 'Thermodynamics of protein folding'. Available at: http://www.cureffi.org/2014/09/05/molecular-biology-02/
Moeller, P., Fields, P., Dutson, T., Landmann, W., and Carpenter, Z. (1977). High temperature effects on lysosomal enzyme distribution and fragmentation of bovine muscle. J. Food Sci. 42, 510–512. doi: 10.1111/j.1365-2621.1977.tb01534.x
Mohsenpour, M., Nourani, M., and Enteshary, R. (2023). Effect of thawing under an alternating magnetic field on rainbow trout (Oncorhynchus mykiss) fillet characteristics. Food Chem. 402:134255. doi: 10.1016/j.foodchem.2022.134255
Monteiro, C. S., Deconinck, D., Eljasik, P., Sobczak, M., Derycke, S., Panicz, R., et al. (2021). A fast HRMA tool to authenticate eight salmonid species in commercial food products. Food Chem. Toxicol. 156:112440. doi: 10.1016/j.fct.2021.112440
Nie, X., Zhang, R., Cheng, L., Zhu, W., Li, S., and Chen, X. (2022). Mechanisms underlying the deterioration of fish quality after harvest and methods of preservation. Food Control 135:108805. doi: 10.1016/j.foodcont.2021.108805
Nielsen, M. K., and Jørgensen, B. M. (2004). Quantitative relationship between trimethylamine oxide aldolase activity and formaldehyde accumulation in white muscle from gadiform fish during frozen storage. J. Agric. Food Chem. 52, 3814–3822. doi: 10.1021/jf035169l
Nikoo, M., Benjakul, S., Ahmadi Gavlighi, H., Xu, X., and Regenstein, J. M. (2019). Hydrolysates from rainbow trout (Oncorhynchus mykiss) processing by-products: properties when added to fish mince with different freeze-thaw cycles. Food Biosci. 30:100418. doi: 10.1016/j.fbio.2019.100418
Offer, G., and Trinick, J. (1983). On the mechanism of water holding in meat: the swelling and shrinking of myofibrils. Meat Sci. 8, 245–281. doi: 10.1016/0309-1740(83)90013-X
Ofstad, R., Kidman, S., Myklebust, R., Olsen, R. L., and Hermansson, A.-M. (1995). Liquid-holding capacity and structural changes in comminuted salmon (Salmo salar) muscle as influenced by pH, salt and temperature. LWT Food Sci. Technol. 28, 329–339. doi: 10.1016/S0023-6438(95)94599-7
Okuma, E., and Abe, H. (1992). Major buffering constituents in animal muscle. Comp. Biochem. Physiol. Comp. Physiol. 102, 37–41. doi: 10.1016/0300-9629(92)90008-E
Ozogul, F., Durmuş, M., Kosker, A. R., Özkütük, A. S., Kuley, E., Yazgan, H., et al. (2023). The impact of marine and terrestrial based extracts on the freshness quality of modified atmosphere packed sea bass fillets. Food Biosci. 53:102545. doi: 10.1016/j.fbio.2023.102545
Paludan-Müller, C., Dalgaard, P., Huss, H. H., and Gram, L. (1998). Evaluation of the role of Carnobacterium piscicola in spoilage of vacuum- and modified-atmosphere-packed cold-smoked salmon stored at 5°C. Int. J. Food Microbiol. 39, 155–166. doi: 10.1016/S0168-1605(97)00133-5
Pascoe, S., Paredes, S., and Coglan, L. (2023). Do “local” markets offer new opportunities to Australian seafood producers? Fish. Res. 263:106691. doi: 10.1016/j.fishres.2023.106691
Prabhakar, P. K., Vatsa, S., Srivastav, P. P., and Pathak, S. S. (2020). A comprehensive review on freshness of fish and assessment: analytical methods and recent innovations. Food Res. Int. 133:109157. doi: 10.1016/j.foodres.2020.109157
Qian, C., Pan, H., Shao, H., Yu, Q., Lou, Y., and Li, Y. (2022). Effects of HVEF treatment on the physicochemical properties and bacterial communities of Larimichthys crocea fillets during refrigerated storage. Int. J. Food Sci. Technol. 57, 7691–7700. doi: 10.1111/ijfs.16115
Quevedo, M., Karbstein, H. P., and Emin, M. A. (2021). Influence of thermomechanical treatment and pH on the denaturation kinetics of highly concentrated whey protein isolate. J. Food Eng. 292:110294. doi: 10.1016/j.jfoodeng.2020.110294
Rezaei, M., and Hosseini, S. F. (2008). Quality assessment of farmed rainbow trout (Oncorhynchus mykiss) during chilled storage. J. Food Sci. 73, H93–H96. doi: 10.1111/j.1750-3841.2008.00792.x
Rodriguez-Salvador, B., and Calvo Dopico, D. (2023). Differentiating fish products: consumers’ preferences for origin and traceability. Fish. Res. 262:106682. doi: 10.1016/j.fishres.2023.106682
Rybicka, I., Silva, M., Gonçalves, A., Oliveira, H., Marques, A., Fernandes, M. J., et al. (2022). The development of smoked mackerel with reduced sodium content. Foods 11:349. doi: 10.3390/foods11030349
Sarma, J., Vidya Sagar Reddy, G., and Srikar, L. N. (2000). Effect of frozen storage on lipids and functional properties of proteins of dressed Indian oil sardine (Sardinella longiceps). Food Res. Int. 33, 815–820. doi: 10.1016/S0963-9969(00)00077-6
Sarower, G., Hasanuzzaman, A., Biswas, B., and Abe, H. (2012). Taste producing components in fish and fisheries products: A review. Int. J. Food Ferment. Technol. 2, 113–121.
Schreurs, F. J. G., Van Der Heide, D., Leenstra, F. R., and De Wit, W. (1995). Endogenous proteolytic enzymes in chicken muscles. Differences among dtrains with different growth rates and protein efficiencies. Poult. Sci. 74, 523–537. doi: 10.3382/ps.0740523
Shenouda, S. Y. K. (1980). “Theories of protein denaturation during frozen storage of fish flesh” in Advances in Food Research. eds. C. O. Chichesters, E. M. Mrak, and G. F. Stewart, vol. 26 (Cambridge, MA: Academic Press), 275–311.
Shui, S., Yan, H., Tu, C., Benjakul, S., Aubourg, S. P., and Zhang, B. (2022). Cold-induced denaturation of muscle proteins in hairtail (Trichiurus lepturus) during storage: physicochemical and label-free based proteomics analyses. Food Chem. X 16:100479. doi: 10.1016/j.fochx.2022.100479
Skawińska, E., and Zalewski, R. I. (2022). Economic impact of temperature control during food transportation— a COVID-19 perspective. Foods 11:467. doi: 10.3390/foods11030467
Tavakoli, S., Naseri, M., Abedi, E., and Imani, A. (2018). Shelf-life enhancement of whole rainbow trout (Oncorhynchus mykiss) treated with Reshgak ice coverage. Food Sci. Nutr. 6, 953–961. doi: 10.1002/fsn3.636
Wang, P. A., Vang, B., Pedersen, A. M., Martinez, I., and Olsen, R. L. (2011). Post-mortem degradation of myosin heavy chain in intact fish muscle: effects of pH and enzyme inhibitors. Food Chem. 124, 1090–1095. doi: 10.1016/j.foodchem.2010.07.093
Warner, R. (2016). “Meat: conversion of muscle into meat” in Encyclopedia of Food and Health. eds. B. Caballero, P. M. Finglas, and F. Toldrá (Cambridge, MA: Academic Press), 677–684.
Watabe, S., Hwang, G. C., Ushio, H., Yamanaka, H., Hatae, K., and Hashimoto, K. (1990). Acceleration of physicochemical change in carp muscle by washing in either chilled or heated water. J. Food Sci. 55, 674–677. doi: 10.1111/j.1365-2621.1990.tb05204.x
Watabe, S., Ushio, H., Iwamoto, M., Kamal, M., Ioka, H., and Hashimoto, K. (1989). Rigor-mortis progress of sardine and mackerel in association with ATP degradation and lactate accumulation. Nippon Suisan Gakkaishi 55, 1833–1839. doi: 10.2331/suisan.55.1833
Xiao, Y., Fu, S., Jiao, Y., Zhang, R., and Liu, Y. (2022). Study on the changes of goat meat quality and the expression of 17 quality-related genes within 48 h of postmortem aging. Food Res. Int. 158:111506. doi: 10.1016/j.foodres.2022.111506
Xie, Y., Chen, B., Guo, J., Nie, W., Zhou, H., Li, P., et al. (2021). Effects of low voltage electrostatic field on the microstructural damage and protein structural changes in prepared beef steak during the freezing process. Meat Sci. 179:108527. doi: 10.1016/j.meatsci.2021.108527
Xie, J., Wang, Z., Wang, S., and Qian, Y.-F. (2019). Textural and quality changes of hairtail fillets (Trichiurus haumela) related with water distribution during simulated cold chain logistics. Food Sci. Technol. Int. 26, 291–299. doi: 10.1177/1082013219888306
Xiong, Y. L. (1997). “Protein denaturation and functionality losses” in Quality in Frozen Food. eds. M. C. Erickson and Y.-C. Hung (New York, NY: Springer US), 111–140.
Xiong, X., Huang, M., Xu, W., Li, Y., Cao, M., and Xiong, X. (2021). Using real time fluorescence loop-mediated isothermal amplification for rapid species authentication of Atlantic salmon (Salmo salar). J. Food Compos. Anal. 95:103659. doi: 10.1016/j.jfca.2020.103659
Yin, M., Matsuoka, R., Yanagisawa, T., Xi, Y., Zhang, L., and Wang, X. (2022). Effect of different drying methods on free amino acid and flavor nucleotides of scallop (patinopecten yessoensis) adductor muscle. Food Chem. 396:133620. doi: 10.1016/j.foodchem.2022.133620
Yin, C., Wang, J., Qian, J., Xiong, K., and Zhang, M. (2022). Quality changes of rainbow trout stored under different packaging conditions and mathematical modeling for predicting the shelf life. Food Packag. Shelf Life 32:100824. doi: 10.1016/j.fpsl.2022.100824
Yu, Y., Tang, M., Dai, H., Feng, X., Ma, L., and Zhang, Y. (2022). Dominating roles of protein conformation and water migration in fish muscle quality: the effect of freshness and heating process. Food Chem. 388:132881. doi: 10.1016/j.foodchem.2022.132881
Yu, Y.-J., Yang, S.-P., Lin, T., Qian, Y.-F., Xie, J., and Hu, C. (2020). Effect of cold chain logistic interruptions on lipid oxidation and volatile organic compounds of Salmon (Salmo salar) and their correlations with water dynamics. Front. Nutr. 7:155. doi: 10.3389/fnut.2020.00155
Yuan, B., Cui, Y., Liu, W., Sheng, W., Xu, H., and Yang, L. (2023). Consumer preferences for oyster trait attributes in China: A choice experiment. Aquaculture 571:739471. doi: 10.1016/j.aquaculture.2023.739471
Keywords: rainbow trout, freshness, rigor mortis, high voltage electrostatic field (HVEF), K value, protein denaturation
Citation: Cheng Y-T, Huang P-H, Lu W-C, Chu S-C, Wang P-M, Ko W-C and Li P-H (2023) Physicochemical properties of rainbow trout (Oncorhynchus mykiss) filet treated with high-voltage electrostatic field under different storage temperatures. Front. Sustain. Food Syst. 7:1158953. doi: 10.3389/fsufs.2023.1158953
Edited by:
Dandan Ren, Dalian Ocean University, ChinaReviewed by:
Eduardo Esteves, University of Algarve, PortugalShi Wenzheng, Shanghai Ocean University, China
Copyright © 2023 Cheng, Huang, Lu, Chu, Wang, Ko and Li. This is an open-access article distributed under the terms of the Creative Commons Attribution License (CC BY). The use, distribution or reproduction in other forums is permitted, provided the original author(s) and the copyright owner(s) are credited and that the original publication in this journal is cited, in accordance with accepted academic practice. No use, distribution or reproduction is permitted which does not comply with these terms.
*Correspondence: Wen-Chien Lu, m104046@cjc.edu.tw; Wen-Chien Ko, wenchienko@nchu.edu.tw; Po-Hsien Li, pohsien0105@pu.edu.tw
†These authors have contributed equally to this work