- 1Department of Chemical Engineering, University of Granada, Granada, Spain
- 2Department of Soil Science and Agricultural Chemistry, University of Granada, Granada, Spain
Iron biofortification has been of main interest for tackling iron deficiency anemia, one of the highest prevalence among micronutrient deficiencies (hidden hunger) in developing countries. This study investigated the effect of activated spent coffee grounds (SCG) and its hydrochars at three temperatures (160°C, 180°C, 200°C) as bio-chelates to level up the iron content of lettuce. Four bio-chelates (ASCG-Fe, AH160-Fe, AH180-Fe and AH200-Fe) were obtained by activation and Fe-functionalization. A pot trial was conducted at doses of 0.2% of the bio-chelates on lettuce with two controls: soil without biofortifying agents (control) and a commercial chelate (control-Fe). Outcomes showed no significant differences (p < 0.05) in soil properties nor in plant growth and morphology, indicating absence of phytotoxicity. All bio-chelates enhanced iron content of plants between 41% (AH200-Fe) and 150% (AH160-Fe) compared to control. The best biofortification effect performed by AH160-Fe was similar to control-Fe (169%), also in terms of soil–plant efficiency both products showed the same transfer factor of 0.07. A proportional impact (up to 150%) was seen on the contribution to the recommended daily intake (RDI). Moreover, higher contents of Mn (29%) and Cu (133%) was evidenced in lettuce with the application of ASCG-Fe and AH180-Fe. These findings suggest activated SCG hydrochars, better than SCG, at small (sub-toxic) doses can successfully achieve agronomic iron biofortification.
1. Introduction
Malnutrition caused by vitamin and mineral deficiency in humans is called “hidden hunger” which approximately 2 billion people in the world are estimated to suffer from Szerement et al. (2022). Iron deficiency is the main cause of iron deficiency anemia. This is the most important nutritional deficiency due to its high prevalence worldwide, mainly affecting people from low-and middle-income countries (WHO, 2020). Among the strategies that have been addressed to mitigate this problem, agronomic biofortification is the most promising so far for its relatively easy application, low cost, effectiveness and almost no adverse effect on crop yield (Szerement et al., 2022). Agricultural soils do not usually have iron deficiency, but this element is not available to be used by the plant. High content of carbonates, as well as high pH of soil, are some of the factors that restrict the bioavailability of this micronutrient; for this reason, foliar application has been the most studied agronomic biofortification technique in recent years (Zulfiqar et al., 2020; Pal et al., 2021; Preciado-Rangel et al., 2022). However, despite the drawbacks, soil application remains a challenge that continues to arouse interest. The use of siderophore-producing microorganisms (Patel et al., 2018), as well as bio-chelates of carbonaceous materials from agro-industrial waste (Cervera-Mata et al., 2021a) are new alternatives explored for soil application to mobilize unavailable iron and/or provide more assimilable amount of it.
Spent coffee grounds (SCG) hydrochars are second-generation by-products resulting from the hydrothermal carbonization (HTC) of SCG, a waste from the agri-food industry that is produced in quantities of approx. 15.33 million tons per year throughout the world (Kamil et al., 2019). In order to revalue this waste within a circular economy framework both, SCG and its hydrochars, have recently been characterized and assessed as an organic soil amendment (Cervera-Mata et al., 2018, 2020, 2021b). On top of that, their application as agricultural bio-chelates can spark new interest to sustainably address micronutrient deficiencies in crops since SCG are proven to mobilize mineral elements in the soil (Cervera-Mata et al., 2020). Hydrochars are known to have higher content of polyphenols and melanoidins than SCG (Cervera-Mata et al., 2021b) what makes this residue a material with probably greater potential to adsorb minerals due to the so-called “chelating capacity” attributed to these compounds (Takenaka et al., 2005; Rufián-Henares and de La Cueva, 2009). An effective ability of antioxidant compounds to form bonds with iron ions has been evidenced because of the presence of their functional groups that perform metal binding (Gulcin and Alwasel, 2022). However, the limitation of plant growth and therefore crop yield reported with the use of SCG and its hydrochars (Hardgrove and Livesley, 2016; Cervera-Mata et al., 2018) are still a challenge to tackle. These negative effects have been related to the high content of compounds classified as toxic such as polyphenols, along with high doses of the bio-products used in the soil (Cervera-Mata et al., 2018, 2020, 2021b).
Carbonaceous materials derived from different procedures are rarely used right after the biomass conversion, in that way, there are various post-treatments proposed in literature to obtain activated carbons (ACs) (Adan-Mas et al., 2021). Activation, considering both type processes: physical or chemical, aims to add more functionality to particle surfaces through the modification or improvement of their chemical reactivity (Yokota et al., 2022). Chemical activation with NaOH has been previously used in SCG for energetic purposes (Chiu and Lin, 2019), however, it is a procedure that may be promising for several applications. The enhancement of metal ion adsorption capacity (Hu et al., 2012), the low energy requirements and the use of non-toxic reagents (Yokota et al., 2022) make this technique an important tool for obtaining agricultural bio-chelates from SCG and SCG hydrochars.
There is a research gap whether SCG hydrochars can also act as agricultural bio-chelates as previously proven with SCG (Cervera-Mata et al., 2021a). Consequently, this study offers a possible new alternative for: (1) SCG revalorization through a cascade of processes (HTC, activation, functionalization), and (2) crop iron biofortification through smart bioinorganic fertilizers. Based on the above, the main objective of this study was to investigate the in vitro effect of activated SCG and SCG hydrochars, obtained at three different temperatures, at low doses, on the enrichment of the iron content of lettuce (biofortification) compared to controls without any biofortifying agent and a commercial chelate. In addition, to assess the efficiency of these bio-products as non-phytotoxic bio-chelates into the soil–plant system. Finally, the content of other micronutrients was determined in order to explore possible variations derived from the mineral mobilization caused by the assayed bio-products. The interest of this study lies in the large amount of SCG generated worldwide and in the difficulties for its traditional use as soil organic amendment due to its high phytotoxicity; it is a paradigm shift in the reuse of this type of biowaste.
2. Materials and methods
2.1. Raw materials
SCG were obtained from the cafeteria of the Faculty of Pharmacy (University of Granada). SCG were spread out on a flat surface and dried at room temperature. Physical, chemical and physicochemical properties of SCG were previously characterized by our research group: C 47.03%, N 1.92%, H 7.66%, fixed carbon 13.89%, volatile matter 85.02%, ash 1.20%, C/N 24, pH 5.76, electrical conductivity (EC25) 2.22 dS/m, polyphenols 77 mg GAE/g (Cervera-Mata et al., 2021b). The soil used in the cultivation assay was collected from the arable layer (0–20 cm) of the Vega of Granada (37°14′7.1″N, 3°45′40.7″W), typical of the Mediterranean climate. The soil assayed had the following properties: coarse fragments (CF) 2%, clay 58.0%, silt 29.9%, pH 8.2, EC25 1.3, OC 1.36%, carbonates as CaCO3 39%, C/N 13, total N 0.11% (Cervera-Mata et al., 2018). Soil was air-dried and sieved (5 mm). The assay was carried out with 30-day-old lettuce (Lactuca sativa var. longifolia) of the “Little Duende” variety from a commercial greenhouse (Saliplant S.L., Granada, Spain).
2.2. Hydrochars production by HTC
The HTC was carried out in a 1-L reactor (Highpreactor™ BR-300, Berghof Ltd., Germany) where 30 g of SCG and deionized water at solid:water ratio of 1:10 (w/w) were loaded. The temperature was then set to 160°C, 180°C, and 200°C (heating rate of 15–20 min) for each experimental run, for 1 h at 300 rpm. The hydrochars obtained (H160, H180, and H200) were recovered by vacuum filtration after the reactor cooled down, and dried in an oven at 60°C overnight.
2.3. Bio-products activation
A suspension of 40 g of bio-product (SCG/hydrochars) in 400 ml of NaOH 0.1 M was put under 40°C at 450 rpm for 1 h on a hotplate. Temperature was controlled with a thermometer and a funnel was placed on top of the flask for recirculation of condensed water. After cooldown of all activated samples, pH was settled to ~7 approx. With HCl 1 N, centrifuged at 3000 rpm and decanted. The solid residues were dried in an oven at 60°C for 48 h and stored.
2.4. Bio-products Fe-functionalization
The activated SCG and hydrochars (ASCG, AH160, AH180, AH200) were functionalized with Fe to bio-chelates following a modified procedure described by Morikawa and Saigusa (2008). The reagent employed was Iron (II) sulfate heptahydrate FeSO4 • 7H2O (MW 278.01) from Sigma (St. Louis, MO). In each case, 40 g of bio-product, 10 g of the reagent and 400 ml of deionized water were used. The mixture was prepared and stored under dark conditions, room temperature and 120 rpm stirring for 24 h. The mixtures were centrifuged (2,500 rpm, 10 min, 20°C) and decanted. The precipitate was washed 3 times with 250 ml of deionized water using the same centrifugal method and then decanted. The functionalized products were dried in an oven at 50°C for 24 h and stored. The Fe content of the resulting bio-chelates was determined by mineralization of 0.2 g of bio-product with HNO3 (65%) and H2O2 at 185°C for 20 min in a microwave digestor (Multiwave 5,000 with Rotor 24HVT50, Anton Paar). Mineral ions in previous extracts were measured using a PerkinElmer® Avio500 inductively coupled plasma–optical emission spectrometer (ICP-OES; Waltham, MA, United States).
2.5. Experimental design
A fixed amount of 0.7 g of each activated bio-chelate was used to obtain a total of 400 g of the soil-bio-chelate mixture. For each soil microcosm case, a triple-15 fertilizer was added twice: day 0 and day 20 of the assay, in quantities of 0.338 g/kg soil to provide N: 50 mg/kg, P: 22 mg/kg, K: 42 mg/kg. The commercial chelate iron ethylenediamine-N, N′-bis (EDDHA-Fe, 6% w/w) (Trade Corporation International, S.A.U) at a concentration of 10 mg/kg, as well as soil without any other product added but NPK were set to be general controlling agents. A total of 6 experimental treatments performed in quadruplicate were set up (Table 1, abbreviations used from here on for each treatment).
The soil mixtures were transferred to PVC pots of 300 ml capacity closed with a double mesh of fiberglass at the base to avoid the loss of fine particles and transplanted with the baby lettuce (average fresh weight of 1.67 ± 0.16 g). The pots were incubated in a growth chamber under controlled conditions with a relative humidity of 50–60%, temperature of 22/18°C (day/night) and 12/12-h photoperiod. The water content of soil was maintained between field capacity and permanent wilting point, according to weighting requirements throughout the assay. Plants were harvested after 40 days.
2.6. Analytical procedures
After cultivation, the whole edible part of lettuce (leaves) was harvested. The samples were weighted (total fresh weight, TFW), subsequently washed with distilled water, dried at 60°C overnight, weighted again (total dry weight, TDW) and stored. Mineralization of plant material was carried out applying the same procedure used for the bio-chelates with HNO3 and H2O2 in a microwave digestor. Soil pH was measured in 1:2.5 (w/w) soil–water suspension and electrical conductivity at 25°C (EC25) measured in 1:5 (w/w) soil–water suspension. Soil available micronutrients were extracted by the method of Lindsay and Norvell (1978). Total Fe content, as well as Zn, Mn, and Cu, from soil and plant extracts were determined by an ICP-OES instrument as described before.
2.7. Efficiency evaluation
To evaluate the efficiency of the bio-chelates, different parameters were calculated. According to Zhao et al. (2019), the Fe utilization efficiency (UE) for lettuce with respect to the total amount of Fe added to soil was calculated:
Also, Fe available reserve efficiency (ARE) in soil was calculated:
Finally, according to Almendros et al. (2013), we calculated the transfer factor (TF) to relate the parameters determined in soil and plant:
2.8. Statistical analysis
One-way analysis of variance (ANOVA) for statistical differences among treatments was assessed using the Tukey test. The significance level was set at 95% (p < 0.05). Statistical analysis was carried out using SPSS 26.0 for Windows (IBM SPSS Inc., New York, United States).
3. Results
3.1. Fe content in the bio-chelates
The final Fe content of the bio-chelates was: ASCG-Fe: 11547.5 ppm, AH160-Fe: 12829.1 ppm, AH180-Fe: 16149.3 ppm, AH200-Fe: 19453.5 ppm.
3.2. Effects on soil
The application of bio-chelates did not show a significant influence on any of the soil properties analyzed (Table 2). pH and EC25 are maintained, as well as the available content of micronutrients.
3.3. Morphology and development of the edible part of lettuce
The physical appearance of the plants was similar at the end of the 40 days of cultivation (Figure 1). No visual differences were found in terms of volume, size, or color intensity of the edible part among the treatments. All lettuces had a homogeneous development from day 0 of sowing to harvest. No signs of phytotoxicity were found. TFW and TDW values were also not significantly affected by the addition of the bio-chelates (Figure 2). All plants achieved an average TFW of 28.28 ± 1.39 g and an average TDW of 2.18 ± 0.19 g.
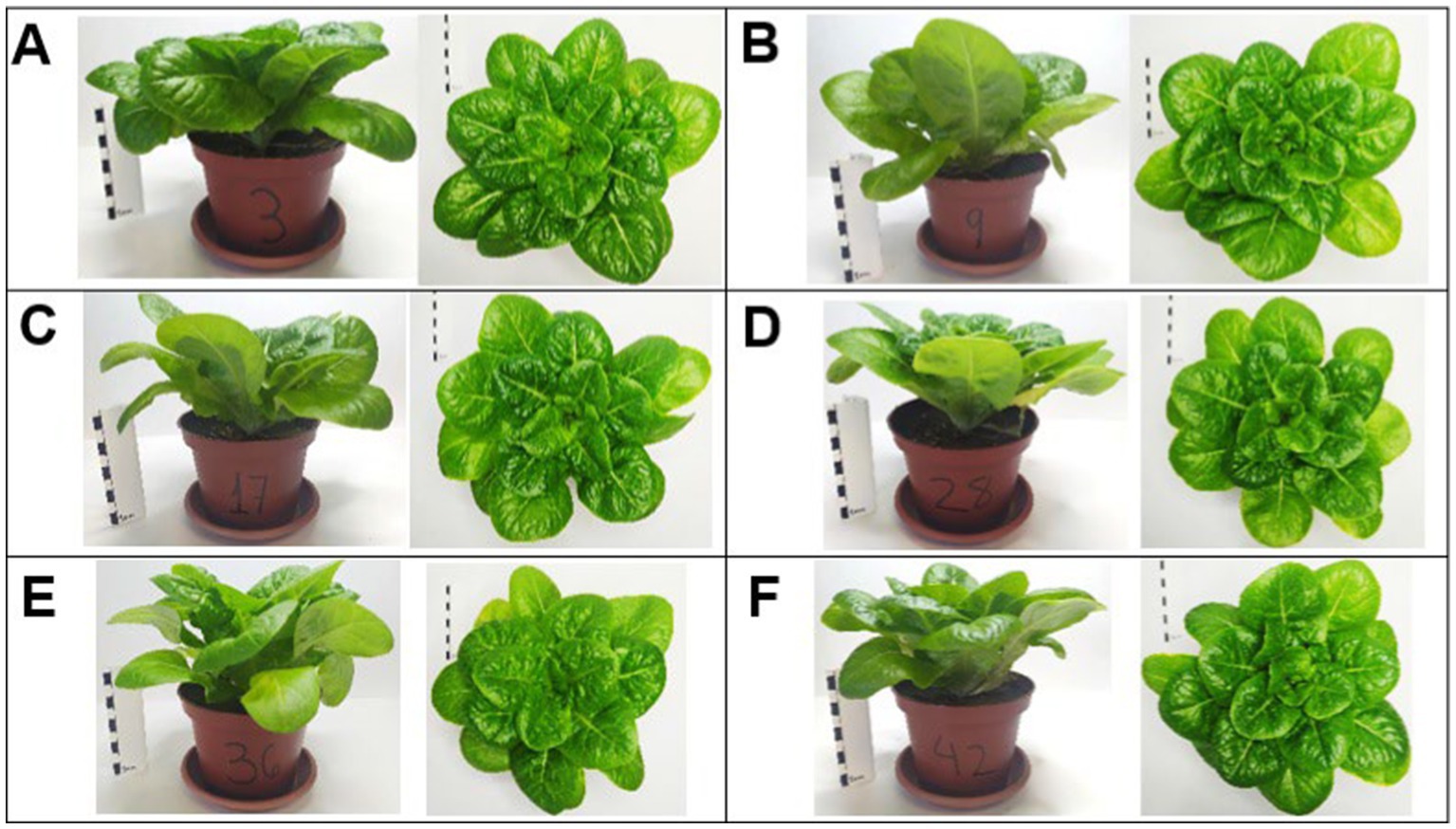
Figure 1. Lettuce images, axial, and zenithal planes, of every treatment (Table 1) after 40 days of cultivation. (A) control; (B) control-Fe; (C) ASCG-Fe; (D) AH160-Fe; (E) AH180-Fe; (F) AH200-Fe.
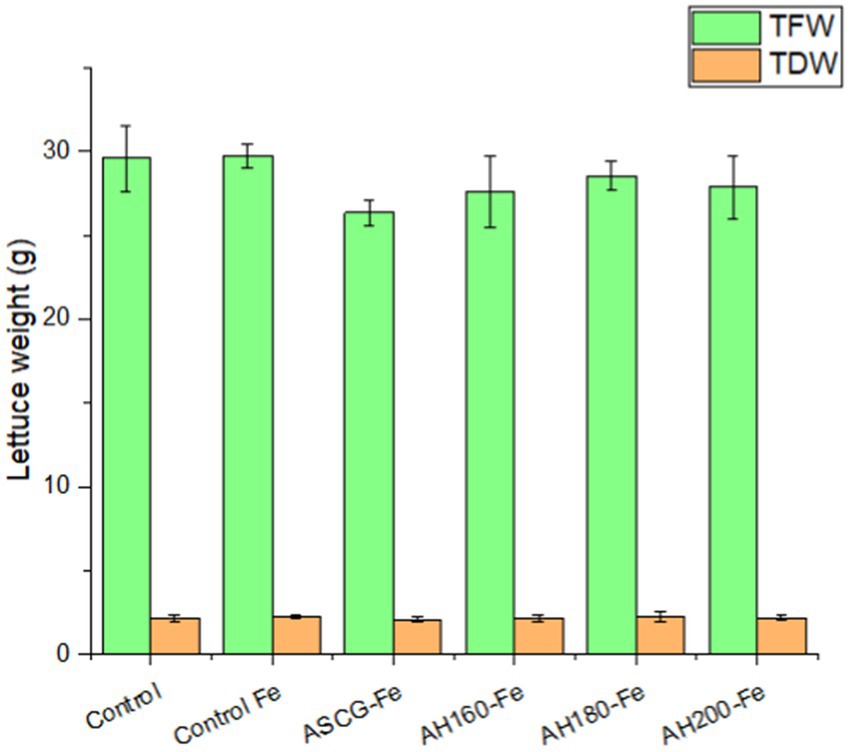
Figure 2. Total fresh weight (TFW) and total dry weight (TDW) of lettuce from different treatments after 40 days of cultivation. The bars represent the standard deviations of the means (N = 4). The absence of letters indicates absence of statistically significant differences (p < 0.05).
3.4. Fe biofortification
Plant Fe content varies significantly (p < 0.05, Figure 3). Three groups are differentiated according to Fe content: low (0.23 mg/100 g FW), medium (~0.52 mg/100 g FW) and high (~0.85 mg/100 g FW). Only plants of the control belong to the first group. The second group is made up by the plants grown with AH200-Fe and ASCG-Fe. These show an increase of this micronutrient compared to control, but this is not as high as control-Fe. Finally, the group with the highest Fe content values. In this last group are lettuce grown with the bio-chelates AH180-Fe and AH160-Fe, and control-Fe. These treatments produced lettuce with three-fold Fe content more than the control.
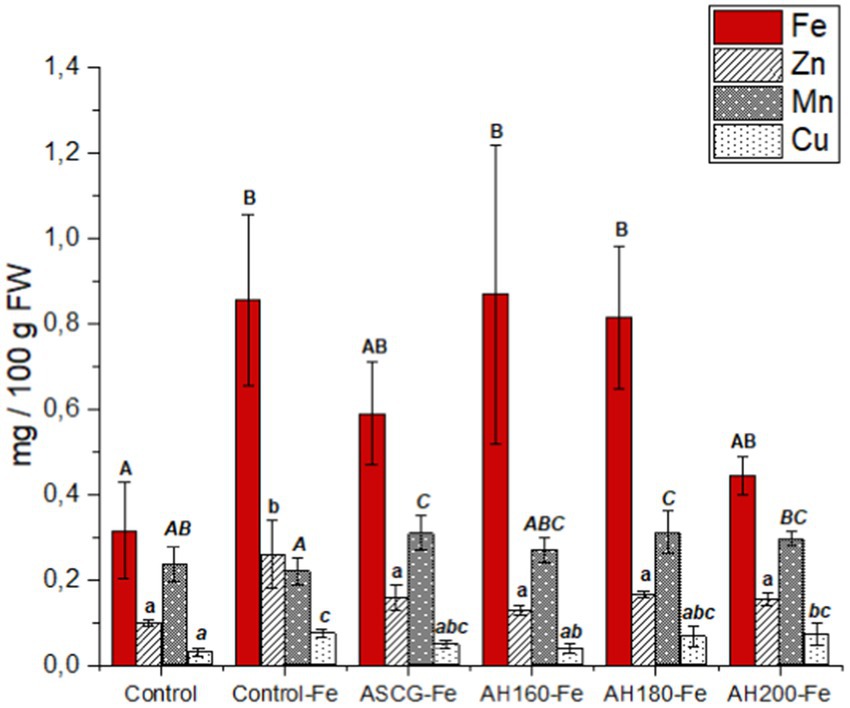
Figure 3. Fe and other micronutrients contents in the edible part of lettuce in the different treatments. The bars represent the standard deviations of the means (N = 4). Different capital letters indicate statistically significant differences (p < 0.05) in Fe content, lower-case letters in Zn content, capital italic letters in Mn and lower-case italic letters in Cu. FW: fresh weight.
3.5. Other micronutrients content evolution
Figure 3 shows that none of the bio-chelates assayed has significant influence on the Zn content, but they do have on Mn and Cu contents. All bio-chelates caused an increase of Mn up to almost 30% and of Cu up to 133%, with respect to the control. Lettuces treated with AH180-Fe exhibit the greatest increase of these micronutrients, on the contrary, those treated with AH160-Fe show the least variations.
4. Discussion
4.1. Plant performance
The application of the previously activated bio-chelates did not negatively affect the normal development of the plants (Figure 1). This result is remarkable since the use of SCG and SCG hydrochars has been associated with phytotoxic effects (Hardgrove and Livesley, 2016). Cervera-Mata et al. (2018) found an evident biomass limitation in lettuce grown with SCG at doses of 2.5 and 10%, pointing out that the degree of phytotoxicity present in plants is proportional to the dose used. The same was reported for SCG hydrochars (H175, H185) at even lower doses, from 1% (Cervera-Mata et al., 2021b) and finally for the application of SCG as bio-chelates in doses of 0.5% (Cervera-Mata et al., 2021a). In this work, the dose of 0.2% used, the lowest ever tested for these bio-products, has shown favorable results in terms of phytotoxicity control, as well as soil properties shifts (Table 2). The amount of bio-chelates supplied to the crop was sufficient for the proposed biofortification objective (Figure 3), which will be discussed later, mainly due to the amount of Fe contained in each bio-product tested (see method). However, the absence of phytotoxicity that is clearly seen not only in the morphological aspect of lettuces (Figure 1) but also in the uniformity of the TFW and TDW (Figure 2), can be attributed both to the low dose applied and, possibly, to the decrease of polyphenols present in the bio-chelates. According to Wilson and Novak (2009), activation with NaOH leads to the solubilization of a variety of organic compounds that, in the case of these bio-products, could include polyphenols. This fact might open new possibilities for the use of these residues in the agricultural field, therefore future studies are necessary.
4.2. Iron biofortification and implications over other micronutrients contents
All the bio-chelates tested increased Fe contents in the plant with respect to the control, but only AH160-Fe and AH180-Fe showed to equal the biofortification effect produced by control-Fe, 150–171% more Fe than the control (Figure 3). Other soil-applied products for Fe fortification report increases of 30% for the case of melanoidins functionalized with Fe (Mel-Fe) in lettuce (Cervera-Mata et al., 2021a) and 340% using Pseudomonas cultures in mungbean (Patel et al., 2018). However, the latter could present limitations for the application in extensive crops due to the requirements of production, maintenance, and viability of the living organisms.
To better understand the dynamics of iron in this study, it is essential to mention that the degree of biofortification obtained (Figure 3) does not seem to be related to the Fe content of each bio-chelate used (see results section). Table 3 shows exclusively the distribution of Fe supplied by the biofortifying agents between plant and soil —the former as Fe content and the latter as available Fe content— both understood as “usable Fe,” and the rest of Fe which is not available. The usable Fe was uniform in every treatment since no statistical differences were found neither in plant nor soil between treatments; however, there is a great difference when comparing this usable Fe with the total Fe added by the bio-chelates. Thus, for example, AH200-Fe supplies the biggest amount of Fe but only 3.4% represents usable Fe. In contrast, AH160-Fe has 2.5 times more usable Fe (8.6%) than AH200-Fe, despite supplying less Fe (Table 3). According to Rousseau et al. (2019), iron bioaccessibility and bioavailability can be negatively affected by polyphenols due to, paradoxically, the great affinity of these compounds for this mineral. Although this affinity is beneficial when designing bio-chelates for mineral binding (Gulcin and Alwasel, 2022), the formation of particularly iron-polyphenol complexes can be a problem if the micronutrient is not released into the medium in sufficient quantity to be used by organisms (Cianciosi et al., 2022). This limiting effect of polyphenols has already been addressed for iron fortification purposes of foods by exploring encapsulation methods or the use of competing complexing agents to ensure the bioavailability of iron (McGee and Diosady, 2018). Consequently, the content of polyphenols in the activated bio-products again gain importance for future analyzes to confirm the above-mentioned hypothesis since, although the load of polyphenols may have been reduced after activation, the existence of a remaining content could still influence the availability of this element in bio-chelates. In the same way, as one portion of non-available Fe can still be absorbed in the particles, another portion can be fixed in the soil as oxides of Fe affecting the mineral bioavailability (Adriano, 2001).
The analysis of usable Fe from the bio-chelates agrees with the efficiency parameters also shown in Table 3. Considering the UE, plants seem to have less capacity to use Fe from AH200-Fe and greater capacity from AH160-Fe, which is also in line with the biofortification results found (Figure 3). Like the UE, the values of ARE in the soil and TF of the system fit this variation pattern, thus evaluating the efficiency of each of the bio-chelates as follows: AH160-Fe > AH180-Fe > ASCG-Fe > AH200-Fe. However, regardless of the available amount of this micronutrient found in the soil (Table 2), the remaining Fe contained in the particles (total content, Figure 3) could be released into the medium over time and have a residual effect in the next cycles of cultivation depending on the composition and type of soil (Maqueda et al., 2015). In addition, it is worth mentioning that all bio-chelates, except AH200-Fe, outperform control-Fe in terms of UE and ARE. On the other hand, the TF is the same for AH160-Fe, AH180-Fe and control-Fe, which exhibit the highest values among the tested biofortifying agents (0.07). Cervera-Mata et al. (2021a) reported a similar TF for SCG melanoidins (Mel-Fe) and a slightly lower TF for SCG-Fe (approx. 0.05). This parameter is a good indicator of the quality of the crop, especially due to the problem of Fe immobilization in calcareous soils (Maqueda et al., 2015).
Another important parameter that can be influenced by the use of these bio-chelates is the availability of other micronutrients in the soil and its consequent variation in plant content. The available content of Zn, Mn and Cu in the soil did not show any variation due to the effect of the bio-chelates (Table 2), but significant differences (p < 0.05) were observed in the plant content (Figure 3). Only Mn (29%) and Cu (133%) were increased in lettuce. Several statements exist in the literature on this topic. Kasongo et al. (2013) reported a decrease in Cu, Zn, Mn and Fe with the addition of SCG in doses of 5, 10 and 20 t/ha in Italian ryegrass crops, both in plants and soil. On the contrary, Cervera-Mata et al. (2019) have registered increases in micronutrients in lettuce proportional to the addition of 1–10% SCG, especially V, Co, Mn and Zn, alleging the ability of SCG to mobilize not only essential elements but also toxic ones. SCG hydrochars also seem to increase the Zn and Cu contents both in plant and soil, as reported by Cervera-Mata et al. (2020) when testing the addition of H180 as an agricultural amendment at a dose of 7.5%. In this study, the results in plant and soil differ from those reported so far. Since NPK was added to the soil along with the bio-product in all the aforementioned tests, the difference in results may rely on the type of soil used. Having said that, the decrease reported by Kasongo et al. (2013) was evidenced in an acid soil (pH: 5.2) while the increases reported partially in this study and totally in those carried out by Cervera-Mata et al. (2019, 2020) were on an alkaline-neutral soil (pH: 7–8). The study by Cruz et al. (2014) in which a tendency to reduction was also seen, not only in micronutrients but also in macronutrients, in lettuce grown with SCG at different doses (2.5–20%), was carried out in an acid soil (pH in CaCl2: 5–6), which strengthens this hypothesis. Therefore, the increase or decrease of mineral elements in plants seems to be conditioned by the pH of the medium. According to Adriano (2001), the sorption of metal ions in the soil is positively influenced by the increase of pH of the soil. Zn and Mn, for instance, are more mobile at pHs between 7–8, while Cu at slightly lower pHs, between 6–7. The author also adds the effect of the complexation of metal ions with organic matter, which helps increase its bioavailability. The combination of these two factors could explain the results found in this study, especially the mobilization of Cu.
4.3. Nutritional aspects
In order to have a complementary perspective of the use of bio-chelates of SCG and its hydrochars in plant biofortification, it is necessary to dig into the nutritional aspects that will finally have a direct impact on human health. Table 4 summarizes the dietary contribution of Fe by lettuce of each treatment obtained in this work.
Although literature reports a standard Fe content of 0.2 mg per serving of lettuce (NutritionValue.org, 2022), the micronutrient content can vary according to the variety of the plant. In this study, it was found a Fe content of 0.110 mg per serving for Lactuca sativa var. longifolia. Taking into account an average weight per serving of 35 g of lettuce (NutritionValue.org, 2022), there is a clear increase of the Fe contribution to the recommended daily intake (RDI) for women and men (NutritionValue.org, 2022; U.S. Department of Health and Human Services, 2022) when lettuce is cultivated with biofortifying agents (Table 4). The intake per serving increases up to 0.305 mg Fe in lettuce grown with bio-chelates compared to 0.110 mg Fe of conventional lettuce (control). This is also directly reflected in the contribution of RDI as all treatments managed to double and even triple this percentage. Interest relies on AH160-Fe and AH180-Fe since these bio-chelates not only show the highest contribution to RDI but seem to behave the same as the commercial chelate (control-Fe), which triples the values of the control. This fact could be of particular relevance for the prevalence of iron-deficiency anemia in the world population, especially in women (33%) and children (42%) (WHO, 2020).
5. Conclusion
Activated SCG and SCG hydrochars are a potential material for agronomic biofortification at doses of 0.2%. This study shows that, especially AH160-Fe, has similar biofortification capacity to that of a commercial chelate; followed by AH180-Fe, ASCG-Fe and finally AH200-Fe. These results bring about lettuce with three times more Fe content than non-biofortified lettuce and, in turn, into a proportional increase in the percentage of the RDI in men and women. The biofortification efficiency of these bio-chelates is not conditioned by their total Fe content, however other characteristics of the bio-products acquired after activation could be involved. None of the assayed products modified soil properties nor limited plant growth, yet they do mobilize and facilitate the uptake of elements such as Mn and Cu. Finally, the application of these bio-chelates could possibly maintain the status of available Fe in the soil for subsequent crops through later microbial mineralization. For this, a toxicity study for soil microbiota may be recommended after the application of bio-chelates to guarantee total safety.
Data availability statement
The original contributions presented in the study are included in the article, further inquiries can be directed to the corresponding author.
Author contributions
GD and AF-A formulated the idea for this article. LL-R conducted laboratory experimental studies and wrote the first draft of the manuscript. GD, AF-A, LL-R, and JF-B contributed to the trials design, methodology, and data interpretation. JF-B provided analytical and statistical support. AF-A managed funding acquisition. JF-B, GD, and AF-A reviewed and edited the manuscript. All authors have read and approved the final manuscript.
Funding
This work was supported by projects PY20_00585 and RDPTC-2018 (AT17_6096_OTRI UGR) from Andalusian Ministry of Economic Transformation, Knowledge, Industry and Universities and FEDER.
Acknowledgments
This research was carried out as a part of a PhD dissertation by the first author, which is being developed within the context of the “Chemistry Programme” at the University of Granada.
Conflict of interest
The authors declare that the research was conducted in the absence of any commercial or financial relationships that could be construed as a potential conflict of interest.
Publisher’s note
All claims expressed in this article are solely those of the authors and do not necessarily represent those of their affiliated organizations, or those of the publisher, the editors and the reviewers. Any product that may be evaluated in this article, or claim that may be made by its manufacturer, is not guaranteed or endorsed by the publisher.
References
Adan-Mas, A., Alcaraz, L., Arévalo-Cid, P., López-Gómez, F. A., and Montemor, F. (2021). Coffee-derived activated carbon from second biowaste for supercapacitor applications. Waste Manag. 120, 280–289. doi: 10.1016/j.wasman.2020.11.043
Almendros, P., Gonzalez, D., and Alvarez, J. M. (2013). Long-term bioavailability effects of synthesized zinc chelates fertilizers on the yield and quality of a flax (Linum usitatissimum L.) crop. Plant Soil 368, 251–265. doi: 10.1007/s11104-012-1502-2
Cervera-Mata, A., Fernández-Arteaga, A., Navarro-Alarcón, M., Hinojosa, D., Pastoriza, S., Delgado, G., et al. (2021a). Spent coffee grounds as a source of smart biochelates to increase Fe and Zn levels in lettuces. J. Clean. Prod. 328:129548. doi: 10.1016/j.jclepro.2021.129548
Cervera-Mata, A., Lara, L., Fernández-Arteaga, A., Ángel Rufián-Henares, J., and Delgado, G. (2021b). Washed hydrochar from spent coffee grounds: a second generation of coffee residues. Eval. Organ. Amend. Waste Manag. 120, 322–329. doi: 10.1016/j.wasman.2020.11.041
Cervera-Mata, A., Navarro-Alarcón, M., Delgado, G., Pastoriza, S., Montilla-Gómez, J., Llopis, J., et al. (2019). Spent coffee grounds improve the nutritional value in elements of lettuce (Lactuca sativa L.) and are an ecological alternative to inorganic fertilizers. Food Chem. 282, 1–8. doi: 10.1016/j.foodchem.2018.12.101
Cervera-Mata, A., Navarro-Alarcón, M., Rufián-Henares, J. Á., Pastoriza, S., Montilla-Gómez, J., and Delgado, G. (2020). Phytotoxicity and chelating capacity of spent coffee grounds: two contrasting faces in its use as soil organic amendment. Sci. Total Environ. 717:137247. doi: 10.1016/j.scitotenv.2020.137247
Cervera-Mata, A., Pastoriza, S., Rufián-Henares, J. Á., Párraga, J., Martín-García, J. M., and Delgado, G. (2018). Impact of spent coffee grounds as organic amendment on soil fertility and lettuce growth in two Mediterranean agricultural soils. Arch. Agron. Soil Sci. 64, 790–804. doi: 10.1080/03650340.2017.1387651
Chiu, Y. H., and Lin, L. Y. (2019). Effect of activating agents for producing activated carbon using a facile one-step synthesis with waste coffee grounds for symmetric supercapacitors. J. Taiwan Inst. Chem. Eng. 101, 177–185. doi: 10.1016/j.jtice.2019.04.050
Cianciosi, D., Forbes-Hernández, T. Y., Regolo, L., Alvarez-Suarez, J. M., Navarro-Hortal, M. D., Xiao, J., et al. (2022). The reciprocal interaction between polyphenols and other dietary compounds: impact on bioavailability, antioxidant capacity and other physico-chemical and nutritional parameters. Food Chem. 375:131904. doi: 10.1016/j.foodchem.2021.131904
Cruz, R., Morais, S., Mendes, E., Pereira, J. A., Baptista, P., and Casal, S. (2014). Improvement of vegetables elemental quality by espresso coffee residues. Food Chem. 148, 294–299. doi: 10.1016/j.foodchem.2013.10.059
Gulcin, İ., and Alwasel, S. H. (2022). Metal ions, metal Chelators and metal chelating assay as antioxidant method. Processes 10:132. doi: 10.3390/pr10010132
Hardgrove, S. J., and Livesley, S. J. (2016). Applying spent coffee grounds directly to urban agriculture soils greatly reduces plant growth. Urban For. Urban Green. 18, 1–8. doi: 10.1016/j.ufug.2016.02.015
Hu, J. L., He, X. W., Wang, C. R., Li, J. W., and Zhang, C. H. (2012). Cadmium adsorption characteristic of alkali modified sewage sludge. Bioresour. Technol. 121, 25–30. doi: 10.1016/j.biortech.2012.06.100
Kamil, M., Ramadan, K. M., Awad, O. I., Ibrahim, T. K., Inayat, A., and Ma, X. (2019). Environmental impacts of biodiesel production from waste spent coffee grounds and its implementation in a compression ignition engine. Sci. Total Environ. 675, 13–30. doi: 10.1016/j.scitotenv.2019.04.156
Kasongo, R. K., Verdoodt, A., Kanyankogote, P., Baert, G., and van Ranst, E. (2013). Response of Italian ryegrass (Lolium multiflorum lam.) to coffee waste application on a humid tropical sandy soil. Soil Use Manag. 29, 22–29. doi: 10.1111/sum.12006
Lindsay, W. L., and Norvell, W. A. (1978). Development of a DTPA soil test for zinc, iron, manganese, and copper. Soil Sci. Soc. Am. J. 42, 421–428. doi: 10.2136/sssaj1978.03615995004200030009x
Maqueda, C., Morillo, E., Lopez, R., Undabeytia, T., and Cabrera, F. (2015). Influence of organic amendments on Fe, cu, Mn, and Zn availability and clay minerals of different soils. Arch. Agron. Soil Sci. 61, 599–613. doi: 10.1080/03650340.2014.946409
McGee, E. J. T., and Diosady, L. L. (2018). Prevention of iron-polyphenol complex formation by chelation in black tea. LWT 89, 756–762. doi: 10.1016/j.lwt.2017.11.041
Morikawa, C. K., and Saigusa, M. (2008). Recycling coffee and tea wastes to increase plant available Fe in alkaline soils. Plant Soil 304, 249–255. doi: 10.1007/s11104-008-9544-1
NutritionValue.org (2022). Available at: https://www.nutritionvalue.org/Lettuce%2C_raw_75113000_nutritional_value.html (accessed 15 August 2022).
Pal, V., Singh, G., and Salwinder,, &, Dhaliwal, S.. (2021). A new approach in agronomic biofortification for improving zinc and iron content in chickpea (Cicer arietinum L.) grain with simultaneous foliar application of zinc Sulphate, ferrous Sulphate and urea. J. Soil Sci. Plant Nutr. 21, 883–896. doi: 10.1007/s42729-021-00408-0/Published
Patel, P., Trivedi, G., and Saraf, M. (2018). Iron biofortification in mungbean using siderophore producing plant growth promoting bacteria. Environ. Sustain. 1, 357–365. doi: 10.1007/s42398-018-00031-3
Preciado-Rangel, P., Valenzuela-García, A. A., Pérez-García, L. A., González-Salas, U., Ortiz-Díaz, S. A., Buendía-García, A., et al. (2022). Foliar biofortification with iron improves nutraceutical quality and antioxidant capacity in lettuce. Terra Latinoamericana 40, 1–7. doi: 10.28940/terra.v40i0.1060
Rousseau, S., Kyomugasho, C., Celus, M., Hendrickx, M. E. G., and Grauwet, T. (2019). Barriers impairing mineral bioaccessibility and bioavailability in plant-based foods and the perspectives for food processing. Crit. Rev. Food Sci. Nutr. 60, 826–843. doi: 10.1080/10408398.2018.1552243
Rufián-Henares, J. A., and de La Cueva, S. P. (2009). Antimicrobial activity of coffee melanoidins - a study of their metal-chelating properties. J. Agric. Food Chem. 57, 432–438. doi: 10.1021/jf8027842
Szerement, J., Szatanik-Kloc, A., Mokrzycki, J., and Mierzwa-Hersztek, M. (2022). Agronomic biofortification with se, Zn, and Fe: an effective strategy to enhance crop nutritional quality and stress defense—a review. J. Soil Sci. Plant Nutr. 22, 1129–1159. doi: 10.1007/s42729-021-00719-2
Takenaka, M., Sato, N., Asakawa, H., Wen, X., Murata, M., and Homma, S. (2005). Characterization of a metal-chelating substance in coffee. Biosci. Biotechnol. Biochem. 69, 26–30. doi: 10.1271/bbb.69.26
U.S. Department of Health and Human Services (2022). National Institutes of Health (NHI). U.S. Department of Health and Human Services. Available at: https://ods.od.nih.gov/factsheets/Iron-HealthProfessional/#h2 (accessed August 15, 2022).
WHO (2020). World Health Organization. Geneva: WHO Press. Available at: https://www.who.int/news/item/20-04-2020-who-guidance-helps-detect-iron-deficiency-and-protect-brain-development (accessed August 15, 2022).
Wilson, C. A., and Novak, J. T. (2009). Hydrolysis of macromolecular components of primary and secondary wastewater sludge by thermal hydrolytic pretreatment. Water Res. 43, 4489–4498. doi: 10.1016/j.watres.2009.07.022
Yokota, S., Nishimoto, A., and Kondo, T. (2022). Alkali-activation of cellulose nanofibrils to facilitate surface chemical modification under aqueous conditions. J. Wood Sci. 68:14. doi: 10.1186/s10086-022-02022-9
Zhao, A., Yang, S., Wang, B., and Tian, X. (2019). Effects of ZnSO4 and Zn-EDTA applied by broadcasting or by banding on soil Zn fractions and Zn uptake by wheat (Triticum aestivum L.) under greenhouse conditions. J. Plant Nutr. Soil Sci. 182, 307–317. doi: 10.1002/jpln.201800341
Keywords: bio-chelates, circular economy, bio-residue, valorization, iron deficiency, micronutrients
Citation: Lara-Ramos L, Fernández-Bayo J, Delgado G and Fernández-Arteaga A (2023) Agronomic iron-biofortification by activated hydrochars of spent coffee grounds. Front. Sustain. Food Syst. 7:1092306. doi: 10.3389/fsufs.2023.1092306
Edited by:
Maryke T. Labuschagne, University of the Free State, South AfricaReviewed by:
Amita Shakya, Amity University, Chhattisgarh, IndiaRanjeet Kumar Mishra, Ramaiah Institute of Technology, India
Copyright © 2023 Lara-Ramos, Fernández-Bayo, Delgado and Fernández-Arteaga. This is an open-access article distributed under the terms of the Creative Commons Attribution License (CC BY). The use, distribution or reproduction in other forums is permitted, provided the original author(s) and the copyright owner(s) are credited and that the original publication in this journal is cited, in accordance with accepted academic practice. No use, distribution or reproduction is permitted which does not comply with these terms.
*Correspondence: Leslie Lara-Ramos, bGxhcmFAY29ycmVvLnVnci5lcw==