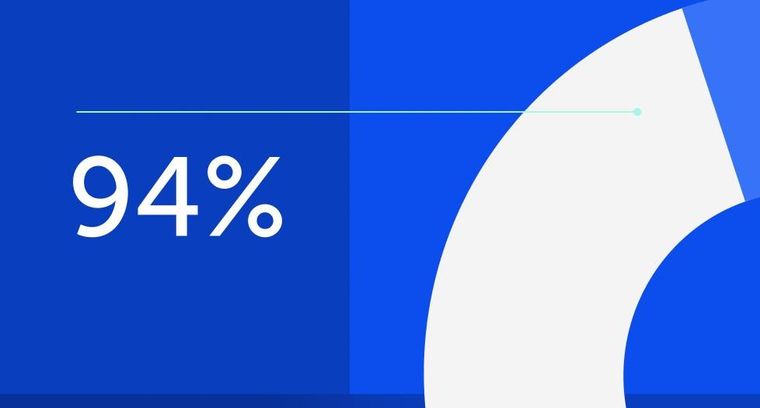
94% of researchers rate our articles as excellent or good
Learn more about the work of our research integrity team to safeguard the quality of each article we publish.
Find out more
ORIGINAL RESEARCH article
Front. Sustain. Food Syst., 16 February 2023
Sec. Sustainable Food Processing
Volume 7 - 2023 | https://doi.org/10.3389/fsufs.2023.1053013
This article is part of the Research TopicSustainable Food Systems in Ibero-AmericaView all 5 articles
Introduction: Pectinolytic enzymes are of great importance for the clarification process of “Vinho Verde” wine must, contributing to the reduction of haze development. During the last decade, a growing body of knowledge has been established about the effects of electric fields on the activation of important food enzymes. However, the influence of electrical parameters on catalytic activity is enzyme-dependent and should be evaluated on a case-by-case basis. This work describes, for the first time, the effects of electric fields and electrical frequency on the activity of pectinase (PEC) in the accelerated clarification of “Vinho Verde” must.
Method: Moderate electric fields (MEF) with intensities below 20 V/cm and at electrical frequencies ranging from 50 to 20 kHz were applied at temperatures between 15 and 35°C. Enzymatic activity was measured for 25 min, and the initial rate of reaction was determined by the coefficient of the linear plot of galacturonic acid (GAL) production as a function of time.
Results: The results show that electrical frequency can increase enzymatic activity depending on temperature conditions; at 20°C and with electrical frequencies of 2 and 20 kHz, enzymatic activity increased by up to 40 and 20%, respectively, when compared with the control sample (without the application of MEF). Temperature dependence was evaluated through the Arrhenius equation, showing that energy of activation (Ea) can be reduced from 9.2 to 6.6 kJ/mol at sub-optimal temperatures for PEC activity when MEF is applied.
Discussion: Electrical parameters, when combined with temperatures below 20°C, reduced pectin concentration in “Vinho Verde” wine must by up to 42% of its initial content. This emergent treatment can be integrated in relevant environmental conditions, presenting an opportunity to increase enzyme efficiency even in low-temperature conditions, which favors the winemaking process.
The use of oenological enzymes supports pre-fermentative operations (such as color and juice extraction) and post-fermentation stages related to wine stabilization, such as filtration and clarification during the winemaking process (Espejo, 2021). Particularly, pectinolytic enzymes are of great importance in the winemaking process by degrading pectin, a complex heteropolysaccharide composed of a backbone rich in α-1,4 linked d-galacturonic acid, which is present in plant cell walls (Espejo, 2021; Reichembach and de Lúcia Oliveira Petkowicz, 2021). Removing pectic materials during the winemaking process has critical industrial relevance since it can decisively contribute to reducing haze occurrence and improving wine clarity and also because of its color stability, which drives consumer choices.
The winemaking process conducted at a low temperature (<20°C) has been attracting interest as it provides an opportunity to offer unique organoleptic properties; e.g., it can contribute to leveraging the fruitiness and herbaceous aromas of white wine by retaining its fruity acetate esters (Deed et al., 2017; Shi et al., 2022). In addition, the clarification of wine or wine must when performed at a low temperature can prevent spoilage, maintain organoleptic stability and nutritional properties, and contribute to energy savings (Naga Padma et al., 2011; Bezus et al., 2022). In this context, the bioprospection for cold-active PEC, or strategies to improve catalytic activity in low temperatures has enormous potential in the wine industry. This is made even more critical by the fact that commercial oenological PECs are often mesophilic and introduce poor efficiency to the process (Merín and de Ambrosini, 2015). Recently, several works have exploited the potential of treatments based on the application of MEF to change the biological activity of food enzymes. MEF treatments include the application of electric fields of intensity below 1000 V/cm (typically between 1 and 20 V/cm), using alternating currents with a varying electrical frequency (i.e., from 50 to 20 kHz). In this process, temperature can be controlled by the ohmic heating effect (Samaranayake and Sastry, 2016b; Durham and Sastry, 2020). Castro et al. (2004) compared inactivation kinetics under conventional and ohmic heating of several enzymes, such as polyphenoloxidase, lipoxygenase, PEC, alkaline phosphatase, and p-galactosidase; in this study, electric fields of 90 V/cm were applied under low electrical frequency regimes (50 Hz) and identical heating conditions (at temperatures ranging from 60 to 90°C). It was observed that electric fields only promoted enhanced inactivation in the cases of lipoxygenase and polyphenoloxidase (Castro et al., 2004). More recently, controlled low-frequency treatments (≤60 Hz) at 0.4 V/cm, combined with the optimal enzyme temperature (65°C) significantly increased the inactivation of pectin methylesterase activity in tomato homogenate (Samaranayake and Sastry, 2016b); these results were, however, dependent on the electrical frequency applied; at higher electrical frequencies (in the range of kHz), the inactivation effects were negligible. MEF treatments were also reported to increase cellulase activity below its optimal working temperature, enhancing the hydrolytic process (Durham and Sastry, 2020); in this case, a low electrical frequency (i.e., 50 Hz) and an increasing electric field intensity contributed to an increase in enzymatic activity. These latter results corroborate a previous work from the same research group where α-amylase activity was also enhanced in the frequency range of 1–60 Hz, contrary to what was observed in high-frequency regimes (Samaranayake and Sastry, 2018). Overall, previous conclusions point out that MEF treatments have different outcomes depending on the type of experimental conditions and the enzyme type. Nevertheless, it seems obvious that enzyme activity appears to be most affected (either positively or negatively) when treatments at low electrical frequencies (≤60 Hz) are applied. It has been suggested that non-thermal effects due to low electrical frequencies may be linked with molecular displacements of the same order of the intermolecular distance in water (Samaranayake and Sastry, 2016b). Some authors have also proposed that electric fields may also affect the prosthetic metal ions within the structure of certain enzymes (Castro et al., 2004; Makroo et al., 2022). Thermodynamically, it is possible that the effects of MEF may be associated with the ions that exist around the enzyme molecules. The collision of these ions with the water molecules existing in the medium may contribute to a change in enzymatic activity (Brochier et al., 2019). Recently, it was hypothesized that MEF may result in an electrophoretic motion similar to the ones that result in a slight increase in effective temperature (Durham and Sastry, 2020). Another explanation for the inactivation of enzyme activity by the MEF may be due to the electrochemical reactions that may occur. These reactions can result in production-reactive compounds that can interact with enzymes, thus causing changes in the conformational structure of their active site (Brochier et al., 2019). Undoubtedly, there is an opportunity to modulate enzymatic activity through MEF processing and the results should not be generalized. This work describes, for the first time, the effects of MEF on oenological PEC activity under non-optimal temperature conditions (<20°C) in the clarification of Portuguese “Vinho Verde” must (VVM), which can be literally translated as “green wine” must.
All chemicals were of analytical grade and purchased from the following sources: Fisher Scientific, (Lisbon, Portugal); Merck Life Science S.L., (Algés, Portugal); Sigma Aldrich Co., (St. Louis, MO, USA); and Panreac AppliChem, (Barcelona, Spain).
White grapes of different varieties (Alvarinho, Arinto, Loureiro, and Trajadura) were harvested in a demarcated region, from different vineyards located in northwestern Portugal (Quinta das Arcas 41.200933645225476, −8.454296443159473; Quinta de Agralongo N 41.61624532010636, W−8.393731159791397; Quinta dos Ingleses 41.27910467211141, −8.233217844459455). White must was collected just after the stemming and crushing of the grapes. Immediate refrigeration was applied to avoid oxidation and spontaneous fermentation. Before the experiments took place, must was decanted and filtered using a cheesecloth to remove any large particles.
VVM was characterized by pH (pH meter, HI 2210, Hanna Instruments, Italy) and electrical conductivity (Conductivity meter, LF538, WTW, Germany) measurements were performed at room temperature (~23°C). The presence of reducing sugars was evaluated spectrophotometrically through 3,5-Dinitrosalicylic acid (DNS) reagent (98%, PanReac AppliChem, Barcelona, Spain) and the pectin content was determined gravimetrically, as described in the following sections.
DNS reagent was prepared as described elsewhere (Coughlan and Moloney, 1988; Saqib and Whitney, 2011) with slight modifications. About 1 g of DNS was added to ~25 mL of distilled water at 80°C, followed by the addition of 30 g of sodium tartrate and 20 mL of sodium hydroxide at 2 mol/L (pellets for analysis EMSURE, Merck Life Science S.L., Algés, Portugal). The solutions were gently stirred until the reagents were dissolved. The final volume was made up to 100 mL with distilled water. A test tube containing 0.5 mL of DNS solution and 0.5 mL of the test sample was placed in boiling water for 5 min. The test tube was quickly cooled down by adding 5 mL of cold water to the mixture. The absorbance of VVM and control samples (distilled water) were read at 540 nm using a microplate reader (Synergy HT, BioTek Instruments, Inc., Winooski, VT, U.S.A.) at room temperature. The reducing sugar concentration was determined against a linear regression by correlating the absorbance of glucose standard solutions in the concentration range between 0.4 and 3.2 g/L. The coefficient of the correlation was >0.99 and the data were expressed as reducing carbohydrates (g/L).
Pectin was precipitated using acidified ethanol, as described in literature (Diez et al., 2010; Uçan et al., 2014; Dorado et al., 2021). About 4 mL of WM were added to 8 mL of acidified ethanol (99% ethanol; absolute, Fisher Scientific, Lagoas Park, Portugal) and to 1% pure hydrochloric acid at 37% (Sigma Aldrich Co., St. Louis, MO, USA). The mixture was settled for 5 min at room temperature and then centrifuged (centrifuge, Mikro EBA 20, Hettich, Germany) at 1960 g for 5 min. The resultant pellet was dried at 50°C for 24 h and then weighed. Pectin yields were calculated by dividing the pellet mass (after drying) by the total mass sample, then multiplying the result by 100.
For the enzymatic hydrolysis of pectin, an oenological PEC was kindly supplied by Bioenno (Valongo, Portugal), isolated from Aspergillus niger fermentation (Bioeno, 2022). This enzyme is reported to have maximum activity at a pH of between 2.8 and 3.5, allowing a fulminant depectinization of the must and enhancing the aromatic expression of the fruit. PEC activity was determined by measuring the initial rate of reaction under different MEF conditions and expressed as micromoles of GAL formed per minute. A unit PEC was considered the amount of enzyme required to efficiently produce 1 micromole of GAL per minute; thus, the activity of the PEC was expressed in units of activity per milliliter.
The effects of MEF were first evaluated in a must simulant solution (MS) as a substrate to allow proper control of the physical and chemical environment, avoiding the presence of interfering substances such as sulfites and alcohol, as well as to overcome issues related to the seasonality and variability of fresh wine must. The MS consisted of a citrate buffer solution (0.05 mol/L, at pH of 3.5) with 2.5 g/L of pectin (from citrus peel, Sigma Aldrich Co., St. Louis, MO, USA). This substrate was later replaced by VVM to assess the effects of MEF treatment conditions that were most effective.
An enzymatic reaction was triggered in jacketed glass reactors over a stirring plate, connected to a temperature-controlled water bath (Julabo HE-4 Seelbach, Germany). MS was placed in the glass reactors to which a solution of 10 g/L of PEC was added to achieve a PEC concentration of 0.3 g/L in a total working volume of 20 mL. To monitor the kinetics of the enzymatic reaction, aliquots of 0.1 mL were taken at different time intervals, i.e., from 0 min (when the enzyme is added to the substrate) up to 25 min. An assay blank, corresponding to a sample of the substrate, was also performed. PEC-catalyzed reactions were generated at temperatures ranging from 15 to 35°C.
MEF treatments were also performed in the same glass reactors but with two stainless steel electrodes placed one at each end of the reactor. The electrodes were at a distance of 3.5 cm from each other and connected to a power generation system consisting of a signal generator (1–25 MHz and 1–10 V; Agilent 33220 A, Penang, Malaysia) and a power amplifier (Precision Power Amplifier 4505, NF Corporation, Japan). Temperature profiles were collected over time, using a type K thermocouple (Omega 709) connected to a data logger (National Instruments, USB−9161). The kinetics of the enzymatic reaction was followed as described in PEC-catalyzed initial reaction rates section but, in this case, under different electrical conditions, by changing the electrical frequency from 20 to 20 kHz and the electric field from 0 to 14 V/cm.
GAL resulting from PEC-catalyzed reactions was determined by DNS (Miller, 1959) and the 2-Cyanoacetamide method (Jacob et al., 2008; Ninga et al., 2021) with some modifications. Both methods are widely employed to follow pectin degradation and determine enzyme activity (Jacob et al., 2008; Oumer and Abate, 2018; Ahmed et al., 2021). DNS assay was performed as described previously (PEC activity section) and GAL concentration was determined against a linear regression, which correlated with the absorbance of GAL standard solutions in the concentration range between 0.5 and 1 g/L. For the analysis of enzymatic activity, through the 2-Cyanoacetamide method, about 0.3 mL of the collected sample (MS and PEC enzyme) were mixed with 0.3 mL of 2-Cyanoacetamide aqueous solution (99%, Sigma Aldrich Co., St. Louis, MO, USA) (at 1%) and 0.6 mL borate buffer (Borate Buffered Saline, Sigma Aldrich Co., St. Louis, MO, USA) (0.1 mol/L at pH 9) and placed in a bath at 100°C for 5 min. Samples were further cooled in an ice bath and sample absorbance was measured in a quartz microplate at 274 nm.
All measurements were made at least in triplicate and all data were shown as mean ± standard deviation or error. Statistical analysis and data modeling were performed using Origin software for Windows (OriginPro 2016, b.9.3.266).
PEC reaction rates at different temperatures were first determined in the MS substrate. This allowed for the avoidance of seasonality issues of VVM collected samples, as well as issues caused by its compositional variability and microbiological instability (for example, due to the occurrence of spontaneous fermentation). Through this experimental approach, it was possible to identify, with high reproducibility, the optimal temperature interval conditions for PEC activity, which could be further applied in VVM samples. The physical and chemical proximal characterization of VVM was important in tuning the MS properties, such as pH and electrical conductivity.
Despite there being little published information regarding the physical and chemical characteristics of VVM, the results of the proximal characterization were within the range expected and were highly comparable to the values observed in other types of grape musts (Loira et al., 2018; Bañuelos et al., 2020). VVM presented an acidic character and a relatively high content of fermentable sugars, as shown in Table 1.
The electrical conductivity value of VVM was well above 0.5 mS/cm, making it a good electrical conductor for MEF application and enabling the ohmic heating effect to take place when needed (Dar et al., 2020). This preliminary characterization allowed for the preparation of an MS solution that was highly comparable to the VVM composition, presenting a pH of 3.5, a pectin concentration of 2.5 g/L, and an electrical conductivity of 2.57 mS/cm (at 25°C). The absence of reducing sugars in MS allowed the PEC activity to be followed using DNS methods without any kind of interference.
Any given enzyme-catalyzed reaction should initially follow a linear relationship before the depletion of substrates, which allows for the calculation of its initial velocity (Bisswanger, 2014). Preliminary results have shown that under the established experimental conditions, the initial velocity (υ0) of pectin hydrolysis can be obtained from the slope of the linear part of the curve during the first 25 min of the reaction. Both methods—DNS and 2-Cyanoacetamide—allowed GAL quantification with highly comparable results; for example, at 20 °C, the υ0 obtained for DNS and 2-Cyanoacetamide were 0.098 μmol/min (R2 = 0.977) and 0.103 μmol/min (R2 = 0.967), respectively. DNS was chosen to proceed with the quantification of GAL due to its ease of operation. Figure 1 shows the linear relationship of GAL production as a function of time at different temperatures and under the effects of MEF. This linear relationship presents high correlation coefficients ranging from 0.968 to 0.999. In all sets of experiments, MEF was applied at 7 V/cm with an electrical frequency of 20 kHz, allowing the evaluation of the effects of increasing temperatures on enzymatic activity.
Figure 1. Linear relationship of GAL formation as a function of time at different temperatures and under MEF effects.
The effects of MEF treatment on the pectin hydrolysis reaction rate showed a temperature-dependent behavior. For example, υ0 for MEF treatments at 15 and 20°C were 29 and 21% higher, respectively, than the ones observed in the control assays (p < 0.05), where MEF was not applied. While at temperatures ≥ 25 °C, the υ0 values of MEF were ~10% lower than the corresponding controls (p < 0.05). For MEF and control treatments, the rate of chemical reactions increased with increasing temperatures, and the onset of enzyme deactivation was observed at temperatures near 35°C. For better interpretation and discussion of these results, PEC activity was then modeled considering the MEF treatments performed.
The results obtained under different temperature conditions did not allow the use of the traditional Arrhenius equation to describe catalytic behavior. Under the chosen experimental conditions, enzyme deactivation or denaturation was a relevant factor, and an additional term needed to be considered to better predict enzymatic activity decay (Santos et al., 2007). To better describe the effects of temperature on enzyme activity, it was considered in terms of a mathematical model described by Wojcik and Milek (Wojcik and Miłek, 2016) with a slight modification, which takes into consideration the energy of activation (Ea). The dimensionless enzyme activity (α) was calculated using the following (Equation 1):
where υT corresponds to the reaction rate at a given temperature, while υmax is the maximum value of the average reaction rate observed experimentally. Equation 2 was then used to express α as a function of reaction temperature (T)
where Topt, Ea, and β correspond to optimal temperature, energy of activation, and a derived parameter, respectively. The energy of deactivation (Ed) was derived from Equation 3 (Wojcik and Miłek, 2016).
Figure 2 shows experimental and fitted data of the PEC activity as a function of temperature under the control and MEF conditions, as described previously (see Initial reaction rates of PEC section). The high correlation coefficients (R2 > 0.97) confirm that the proposed model (Wojcik and Miłek, 2016) was well fitted to the experimental data. The main objective of this study is to understand the effect of MEF at room temperature (between 20 and 25°C). The deactivation temperature of PEC (Ed) was determined but should be interpreted with some care due to the lack of experimental points in the range of denaturation temperatures. Nonetheless, the experimental conditions allowed for accurate modeling, taking into account the temperature dependence and the onset of enzyme denaturation. Table 2 shows estimated parameters derived from Equation 2 and traditional Arrhenius linearization. Estimated parameters gave a clear indication that MEF contributed to reducing Ea and to accelerating enzymatic reactions at lower temperatures when compared with the control assays, thus confirming higher υ0 observed at temperatures of 15 and 20°C. Despite the scarce availability of data regarding MEF treatments, Zhang et al. (2017) reported similar results with a pulsed electric field (PEF) treatment obtaining Ea values of 96.01 ± 1.30 and 90.94 ± 2.57 kJ/mol for the untreated and PEF-treated PEC, respectively, thus demonstrating that PEF treatment can significantly lower the reaction's energy barrier. Conversely, thermal stability seems to be most affected by the presence of MEF considering the lower values of Topt and Ed when compared with the control treatments. Interestingly, these two events—high enzymatic activity and less thermal stability induced by MEF—have been recently reported. A larger body of knowledge has been established regarding the non-thermal effects of MEF in several enzymes by combining experimental approaches with molecular dynamics simulations (Samaranayake and Sastry, 2016b, 2018). Sastry and Durham (Durham and Sastry, 2020) have concluded that MEF enhances enzymatic hydrolysis of cellulose at 30 and 40°C, which are below the considered optimal enzyme temperature (i.e., 50°C).
Figure 2. PEC activity variation as a function of temperature: experimental data and fitting to a mathematical model of Equation 2.
The latter authors have concluded that MEF treatments, due to the induced electrophoretic motion, have an effect that is similar to a slight increase in medium temperature, which may accelerate enzyme activity below optimal temperature or increase the loss of stability near optimal temperature. This evidence supports the obtained results in this study, considering that MEF induces higher reaction rates at temperatures below 25°C, while the loss of activity was verified between 25 and 35°C when compared with control assays. This was also corroborated by the lower Ea, Ed, and Topt estimated under MEF when compared with control assays. It is noteworthy that all enzymatic assays were performed under mild temperature conditions (≤35°C), revealing the importance of MEF effects on the observed outcomes. This study produces, for the first time, a characterization of PEC activity in wine must under MEF, which makes the comparison of results difficult. Most published literature describes the optimal temperature for PEC, which differs depending on the type of PEC and intended application. For cold-active enzymes, the optimal temperature can be widely distributed between 5 and 90°C, but a higher frequency is observed between 30 and 40°C, particularly for the clarification of fruit juices (Sandri et al., 2011; Santiago et al., 2016), which compares very well with the estimated optimal temperatures obtained in this work. To get a better insight into the effects of MEF on PEC activity, the influence of applying different electric field strengths and electrical frequencies was evaluated at 20 and 30°C. This range of temperatures covers a transition behavior of PEC activity under the influence of MEF.
Interestingly, Figure 3 shows that the reaction rate at different temperatures is also dependent on the electric field and electrical frequency applied. At 30°C, increasing electric fields and electrical frequencies resulted in the loss of PEC activity, while the opposite was observed at 20°C (p < 0.05). It has been previously reported that the combination of a low electric field (0.4 V/cm) and lower electrical frequencies ≤ 60 Hz can contribute to the inactivation of pectin methylesterase (PME) at reaction temperatures of 60°C, while the effects observed at the same temperature but with the application of higher electrical frequencies (up to 1 MHz) were considered negligible (Samaranayake and Sastry, 2016b).
Figure 3. PEC activity under different electric fields and electrical frequencies at 20°C (black squares) and 30°C (gray circles), with varying electrical frequencies (with a constant electric field of 7 V/cm) and varying electric fields (with a constant electrical frequency of 25 kHz). Control assays correspond to 0 V/cm and 0 Hz, and the standard error was <7% for all results.
In addition, the catalytic activity of PME was also dependent on the electric field applied in combination with the reaction temperature: electric fields up to 10 V/cm allowed for the increased activity of PME at temperatures near 70°C, but enhanced inactivation was observed at higher temperatures when compared with the corresponding control assay (Samaranayake and Sastry, 2016a).
Overall, the results from this study show that the efficacy of enzyme activity under MEF is strictly dependent on three factors: (i) reaction temperature; (ii) electric field; and (iii) electric frequency. Other authors have stated that the kinetics of enzymatic reactions are improved by low-intensity electric fields that promote the mild polarization of enzymes, revealing the enzyme's active site to substrates. On the other hand, high-intensity electric fields frequently inactivate enzymes by largely destroying their conformations (Huang et al., 2012). In this sense, a study by Ohshima et al. (2007) states that six different types of enzymes had a 20% increase in activity when exposed to a PEF (12–13 kV/cm) with a short decaying time, which is in line with the results obtained in this work, despite different electric field magnitudes.
Given the existing literature reports and the results of this study, it would be interesting to develop experimental designs aiming a combination of MEF experimental variables toward a fine-tune control of enzymatic activity for the intended applications. Additionally, the effects of other variables, such as type of electric waveform, current density, and electrochemical reactions are still not well understood, and all of them may play a crucial role in the observed effects. Regarding electrical frequency, the impact of electrolysis is still scarce. However, the frequency dependence of the production of free radicals, which are highly reactive at the surface of the electrodes, and their interaction within the molecular structure of enzymes may affect its stability (Ammam and Fransaer, 2011). Electrochemical reactions are usually fostered in lower frequency regimes in combination with high electrical conductivity of the medium, the high electric field applied, and consequently, high reaction temperatures. Another challenge is driven by the fact that it is difficult to isolate non-thermal effects given that MEF application generates an ohmic heating effect depending on the electrical conductivity of the medium, the intensity of the applied electric field, and the treatment time. In this work, enzyme activity assays were performed under cold and mild temperature conditions (from 15 to 35°C), which have a minimal ohmic heating effect, and the experimental artifacts promoted by heating kinetics or conduction and convection heating transfer mechanisms; these are particularly relevant when high temperatures are needed.
Knowing that the removal of pectin in low temperatures can contribute to microbiological stability and add interesting organoleptic features to “Vinho Verde” wine (Merín and de Ambrosini, 2015), the results regarding PEC activity at 20°C under MEF application were highly satisfactory since they enabled the attainment of almost 50% of maximum PEC activity; maximum PEC activity was only observed at a temperature of 35°C without the application of MEF. To corroborate and validate previous results, enzymatic treatments were then assisted by MEF (i.e., at 7 V/cm, 20 kHz, and 20°C) using a fresh VVM sample collected just after the grape stemming and crushing steps in the vineyards. The pectin contents were determined gravimetrically after the MEF treatment, as described in VVM samples section. Figure 4 shows the effects of MEF on the pectin removal from a treated VVM (sample with PEC and treated with MEF) benchmarked with positive control (with PEC but without MEF application) and an untreated sample or negative control (without PEC and MEF treatment). The results obtained confirm that MEF can significantly enhance the PEC activity in wine must (p < 0.05), with a reduction of 42% of the initial content of pectin at 20°C. It is, however, noteworthy that without the application of MEF, it was only reduced by 10%. This difference was even visible to the naked eye, contributing to a reduction in the turbidity of the must samples. These results suggest that MEF may be easily used to assist the winemaking process below room temperature, improving the product's quality without additives or post-production clarification methods.
Figure 4. Pectin concentration of Vinho Verde must samples just before the addition of PEC (untreated) and after PEC treatment at 20°C, without MEF application (PEC sample) and assisted by MEF at 7 V/cm and 20 kHz (PEC+MEF sample). Different letters correspond to the statistical difference (p < 0.05) between treatments.
For the first time, MEF technology was applied in the winemaking process of “Vinho Verde”. The results from this study reveal that MEF can support pectin hydrolysis below the optimal temperature range for PEC by reducing its Ea and decreasing the initial pectin content by 42%. This offers winemakers an interesting opportunity to develop enzymatic operations at cold temperatures, reducing energy consumption and improving the organoleptic quality of their developed wines. The mechanisms behind this behavior are underlined in published literature and linked with induced electrophoretic motion that can contribute to an effect similar to an increase in temperature medium. From the results, it is also possible to conclude that a comprehensive understanding is needed given that the enzymatic behavior was not only temperature-dependent but also highly influenced by other electrical variables, such as electric field intensity and electrical frequency, even when the ohmic heating effect was absent or minimal. A fine-tune control of electrical, thermal, and chemical variables in synergy with the use of cold-active PEC may cause interesting outcomes in the optimization of the enzymatic operations needed for the winemaking process. Given the low intensity of the electric fields applied, the MEF technology can be supported by a renewable source of energy, presenting an element of environmental sustainability.
The raw data supporting the conclusions of this article will be made available by the authors, without undue reservation.
All authors listed have made a substantial, direct, and intellectual contribution to the work and approved it for publication.
This study was supported by the Portuguese Foundation for Science and Technology (FCT) under the scope of the strategic funding of UIDB/04469/2020 unit, LABBELS- Associate Laboratory in Biotechnology, Bioengineering and Microelectromechanical Systems, LA/P/0029/2020, and funded by BIOECONORTE (ref. NORTE-01-0145-FEDER-000070) with a funding body from the Norte Portugal Regional Coordination and Development Commission (CCDR-N), NORTE2020, Portugal 2020 and ESIF - European Structural and Investment Funds.
RP acknowledges FCT for the Assistant Research contract obtained under CEEC Individual 2017.
The authors declare that the research was conducted in the absence of any commercial or financial relationships that could be construed as a potential conflict of interest.
All claims expressed in this article are solely those of the authors and do not necessarily represent those of their affiliated organizations, or those of the publisher, the editors and the reviewers. Any product that may be evaluated in this article, or claim that may be made by its manufacturer, is not guaranteed or endorsed by the publisher.
Ahmed, T., Rana, M. R., Zzaman, W., Ara, R., and Aziz, M. G. (2021). Optimization of substrate composition for pectinase production from Satkara (Citrus macroptera) peel using Aspergillus niger-ATCC 1640 in solid-state fermentation. Heliyon 7, e08133. doi: 10.1016/j.heliyon.2021.e08133
Ammam, M., and Fransaer, J. (2011). Effects of AC-electrolysis on the enzymatic activity of glucose oxidase. Electroanalysis 23, 755–763. doi: 10.1002/elan.201000598
Bañuelos, M. A., Loira, I., Guamis, B., Escott, C., Del Fresno, J. M., Codina-Torrella, I., et al. (2020). White wine processing by UHPH without SO2. Elimination of microbial populations and effect in oxidative enzymes, colloidal stability and sensory quality. Food Chem. 332. doi: 10.1016/j.foodchem.2020.127417
Bezus, B., Cavello, I., Contreras-Esquivel, J. C., and Cavalitto, S. (2022). Pectinases produced by extremophilic yeasts: from cold environments to the food industry. Value-Addition. Food Prod. Process. Through Enzym. Technol. 437–452. doi: 10.1016/B978-0-323-89929-1.00010-X
Bioeno (2022). Available online at: http://www.bioeno.pt/docs/W-COMPACT-LIQUID-FT.pdf (accessed July 17, 2022).
Brochier, B., Mercali, G. D., and Marczak, L. D. F. (2019). Effect of moderate electric field on peroxidase activity, phenolic compounds and color during ohmic heating of sugarcane juice. J. Food Process. Preserv. 43, e14254. doi: 10.1111/jfpp.14254
Castro, I., Macedo, B., Teixeira, J. A., and Vicente, A. A. (2004). The effect of electric field on important food-processing enzymes: comparison of inactivation kinetics under conventional and ohmic heating. J. Food Sci. 69, C696–C701. doi: 10.1111/j.1365-2621.2004.tb09918.x
Coughlan, M. P., and Moloney, A. P. (1988). Isolation of 1,4-β-d-glucan 4-glucanohydrolases of Talaromyces emersonii. Methods Enzymol. 160, 363–368. doi: 10.1016/0076-6879(88)60140-6
Dar, A. H., Shams, R., Rizvi, Q. E. H., and Majid, I. (2020). “Microwave and ohmic heating of fresh cut fruits and vegetable products,” in Fresh-Cut Fruits and Vegetables, ed M. W. Siddiqui (Academic Press), 295–337. doi: 10.1016/B978-0-12-816184-5.00013-6-2
Deed, R. C., Fedrizzi, B., and Gardner, R. C. (2017). Influence of fermentation temperature, yeast strain, and grape juice on the aroma chemistry and sensory profile of sauvignon Blanc wines. J. Agric. Food Chem. 65, 8902–12. doi: 10.1021/acs.jafc.7b03229
Diez, L., Guadalupe, Z., Ayestarán, B., and Ruiz-Larrea, F. (2010). Effect of yeast mannoproteins and grape polysaccharides on the growth of wine lactic acid and acetic acid bacteria. J. Agric. Food Chem. 58, 7731–7739. doi: 10.1021/jf100199n
Dorado, C., Cameron, R. G., Manthey, J. A., and Ferguson, K. L. (2021). Bench scale batch steam explosion of Florida red and white grapefruit juice processing residues. Futur. Foods 3, 100020. doi: 10.1016/j.fufo.2021.100020
Durham, E. K., and Sastry, S. K. (2020). Moderate electric field treatment enhances enzymatic hydrolysis of cellulose at below-optimal temperatures. Enzyme Microb. Technol. 142, 109678. doi: 10.1016/j.enzmictec.2020.109678
Espejo, F. (2021). Role of commercial enzymes in wine production: a critical review of recent research. J. Food Sci. Technol. 58, 9. doi: 10.1007/s13197-020-04489-0
Huang, K., Tian, H., Gai, L., and Wang, J. (2012). A review of kinetic models for inactivating microorganisms and enzymes by pulsed electric field processing. J. Food Eng. 111, 191–207. doi: 10.1016/j.jfoodeng.2012.02.007
Jacob, N., Niladevi, K. N., Anisha, G. S., and Prema, P. (2008). Hydrolysis of pectin: An enzymatic approach and its application in banana fiber processing. Microbiol. Res. 163, 538–544. doi: 10.1016/j.micres.2006.07.016
Loira, I., Morata, A., Bañuelos, M. A., Puig-Pujol, A., Guamis, B., González, C., et al. (2018). Use of Ultra-High Pressure Homogenization processing in winemaking: control of microbial populations in grape musts and effects in sensory quality. Innov. Food Sci. Emerg. Technol. 50, 50–56. doi: 10.1016/j.ifset.2018.10.005
Makroo, H. A., Srivastava, B., and Jabeen, A. (2022). Influence of mild electric field (MEF) on polyphenol oxidase and quality attributes of pineapple juice during ohmic heating. LWT 156, 113021. doi: 10.1016/j.lwt.2021.113021
Merín, M. G., and de Ambrosini, V. I. M. (2015). Highly cold-active pectinases under wine-like conditions from non-Saccharomyces yeasts for enzymatic production during winemaking. Lett. Appl. Microbiol. 60, 467–474. doi: 10.1111/lam.12390
Miller, G. L. (1959). Use of dinitrosalicylic acid reagent for determination of reducing sugar. Anal. Chem. 31, 426–428. doi: 10.1021/ac60147a030
Naga Padma, P., Anuradha, K., and Reddy, G. (2011). Pectinolytic yeast isolates for cold-active polygalacturonase production. Innov. Food Sci. Emerg. Technol. 12, 178–181. doi: 10.1016/j.ifset.2011.02.001
Ninga, K. A., Desobgo, Z. S. C., De, S., and Nso, E. J. (2021). Pectinase hydrolysis of guava pulp: effect on the physicochemical characteristics of its juice. Heliyon 7, e08141. doi: 10.1016/j.heliyon.2021.e08141
Ohshima, T., Tamura, T., and Sato, M. (2007). Influence of pulsed electric field on various enzyme activities. J. Electrostat. 65, 156–161. doi: 10.1016/j.elstat.2006.07.005
Oumer, O. J., and Abate, D. (2018). Screening and molecular identification of pectinase producing microbes from coffee pulp. Biomed Res. Int. 65, 156–161. doi: 10.1155/2018/2961767
Reichembach, L. H., and de Lúcia Oliveira Petkowicz, C. (2021). Pectins from alternative sources and uses beyond sweets and jellies: an overview. Food Hydrocoll. 118, 106824. doi: 10.1016/j.foodhyd.2021.106824
Samaranayake, C. P., and Sastry, S. K. (2016a). Effect of moderate electric fields on inactivation kinetics of pectin methylesterase in tomatoes: the roles of electric field strength and temperature. J. Food Eng. 186, 17–26. doi: 10.1016/j.jfoodeng.2016.04.006
Samaranayake, C. P., and Sastry, S. K. (2016b). Effects of controlled-frequency moderate electric fields on pectin methylesterase and polygalacturonase activities in tomato homogenate. Food Chem. 199, 265–272. doi: 10.1016/j.foodchem.2015.12.010
Samaranayake, C. P., and Sastry, S. K. (2018). In-situ activity of α-amylase in the presence of controlled-frequency moderate electric fields. LWT Food Sci. Technol. 90, 448–454. doi: 10.1016/j.lwt.2017.12.053
Sandri, I. G., Fontana, R. C., Barfknecht, D. M., and da Silveira, M. M. (2011). Clarification of fruit juices by fungal pectinases. LWT Food Sci. Technol. 44, 2217–2222. doi: 10.1016/j.lwt.2011.02.008
Santiago, M., Ramírez-Sarmiento, C. A., Zamora, R. A., and Parra, L. P. (2016). Discovery, molecular mechanisms, and industrial applications of cold-active enzymes. Front. Microbiol. 7, 1408. doi: 10.3389/fmicb.2016.01408
Santos, A. M. P., Oliveira, M. G., and Maugeri, F. (2007). Modelling thermal stability and activity of free and immobilized enzymes as a novel tool for enzyme reactor design. Bioresour. Technol. 98, 3142–3148. doi: 10.1016/j.biortech.2006.10.035
Saqib, A. A. N., and Whitney, P. J. (2011). Differential behaviour of the dinitrosalicylic acid (DNS) reagent toward mono- and di-saccharide sugars. Biomass Bioenergy 35, 4748–4750. doi: 10.1016/j.biombioe.2011.09.013
Shi, X., Liu, Y., Ma, Q., Wang, J., Luo, J., Suo, R., et al. (2022). Effects of low temperature on the dynamics of volatile compounds and their correlation with the microbial succession during the fermentation of Longyan wine. LWT 154, 112661. doi: 10.1016/j.lwt.2021.112661
Uçan, F., Akyildiz, A., and Agçam, E. (2014). Effects of different enzymes and concentrations in the production of clarified lemon juice. J. Food Process. 2014, 1–14. doi: 10.1155/2014/215854
Wojcik, M., and Miłek, J. (2016). A new method to determine optimum temperature and activation energies for enzymatic reactions. Bioprocess Biosyst. Eng. 39, 1319–1323. doi: 10.1007/s00449-016-1596-7
Keywords: electrical frequency, optimal temperature enzymatic activity, energy of activation, pectinase, “Vinho Verde” wine must, clarification
Citation: Queirós M, Pereira G, Leite AC, Leal R, Rodrigues R, Teixeira JA and Pereira RN (2023) Tunning pectinase activity under the effects of electric fields in the enhanced clarification of wine must. Front. Sustain. Food Syst. 7:1053013. doi: 10.3389/fsufs.2023.1053013
Received: 24 September 2022; Accepted: 02 January 2023;
Published: 16 February 2023.
Edited by:
Ana Isabel Bourbon, International Iberian Nanotechnology Laboratory (INL), PortugalReviewed by:
Sunil C. K., Indian Institute of Food Processing Technology, IndiaCopyright © 2023 Queirós, Pereira, Leite, Leal, Rodrigues, Teixeira and Pereira. This is an open-access article distributed under the terms of the Creative Commons Attribution License (CC BY). The use, distribution or reproduction in other forums is permitted, provided the original author(s) and the copyright owner(s) are credited and that the original publication in this journal is cited, in accordance with accepted academic practice. No use, distribution or reproduction is permitted which does not comply with these terms.
*Correspondence: Ricardo N. Pereira, cnBlcmVpcmFAZGViLnVtaW5oby5wdA==
†These authors have contributed equally to this work
Disclaimer: All claims expressed in this article are solely those of the authors and do not necessarily represent those of their affiliated organizations, or those of the publisher, the editors and the reviewers. Any product that may be evaluated in this article or claim that may be made by its manufacturer is not guaranteed or endorsed by the publisher.
Research integrity at Frontiers
Learn more about the work of our research integrity team to safeguard the quality of each article we publish.