- 1Institut National pour l'Etude et la Recherche Agronomiques, Mulungu Research Station, Bukavu, South-Kivu, Democratic Republic of Congo
- 2The Alliance of Bioversity and CIAT, Bukavu, Democratic Republic of Congo
- 3The Alliance of Bioversity and CIAT, Kampala, Uganda
- 4The Alliance of Bioversity and CIAT, Addis Ababa, Ethiopia
- 5Department of Agronomy, University of Kisangani, Kisangani, Democratic Republic of Congo
- 6Department of Sciences, University of Kisangani, Kisangani, Democratic Republic of Congo
Land degradation is a major obstacle to agricultural development in Africa, where it's accentuated by poor agricultural practices and climate change effects. Restoration of degraded lands is crucial to prevent incursions into virgin and marginal lands. A field experiment was carried out over a four-year period on two degraded sites, to assess and compare the effect of the common practices of: (i) burning crop residues and weeds by resource-poor farmers (T1) and (ii) burning crop residues and weeds followed with application of manure and/or NPK as external inputs (T2) by resource-endowed farmers with (iii) an innovative agroecological package (T3) on soil physical, chemical and biological attributes, and crop yields. T3 consisted of crop rotation and/ intercropping with Mucuna pruriens cover crop, grasses (Pennisetum purpureum and Setaria sphacelate) and shrubs (Calliandra calothyrsus, and Leucaena diversifolia), and the application of manure and NPK. A randomized complete block design with 8 and 10 blocks, with each package appearing once in each block, was used respectively, at Mulungu and Mushinga. The agroecological package significantly improved soil and plant parameters compared to the common practices at both sites. The average plot-level aboveground biomass was significantly lower (p < 0.001) in T1 (11.3–17.4 t/ha) and T2 (10.1–21.9) than in T3 (39.9–60.4 t/ha). Similar trends were observed for bean and maize grain and banana bunch yields. When T1 is compared to T3, mean yields increased 2.6 times (848 kg/ha against 327 kg/ha), 2.8 times (2,201 kg/ha against 792 kg/ha) and 1.5 times (7.4 t/ha against 5.0 t/ha) for bean grains, maize grain, and banana bunches, respectively. Improvements were also observed for soil physical, biological, and chemical properties. A decrease in soil temperature; and increases in soil porosity, earthworm density/m2 (1,932 against 0), nodules/bean plant (28 against 21) and root length density (65 against 15.5 cm) were observed in T3. T3 (compared to T1), had a decrease in acidity and Al3+; an increase in soil organic matter, K+, Ca+, and aboveground carbon stock (26.5 t/ha against 5.6 t/ha). The innovative agroecological package is thus an approach that can be used to effectively restore degraded and abandoned farmlands.
1. Introduction
Over two billion hectares of arable land is degraded world over (Akpo et al., 2016; Ouedraogo et al., 2017; Kim et al., 2021), accounting for over 20% of the world's land, with nearly a third of this land located in Africa (Roose, 2018). Land degradation is a major obstacle to agricultural development in Africa, negatively affecting the production and provision of ecosystem goods and services, and livelihoods (WHO, 2022). Through emission of greenhouse gases and reducing the ability of landscapes to uptake carbon, land degradation also contributes to climate change (Olsson et al., 2019).
Land degradation has been accelerated by the combined pressure arising from agricultural production, deforestation, urbanization and harsh climatic conditions such as droughts (IUCN, 2015; Hermans and McLeman, 2021; WHO, 2022). Agricultural practices which reduce soil carbon content, often lead to changes in soil structure, disruptions in soil microbial activity, alterations in the provision of water and nutrients to crops, contributing to land degradation and lower crop productivity (Mayer et al., 2019; Zhu et al., 2021).
In the highlands of the Great Lakes Region (GLR) of Africa, the average population density reaches 400 inhabitants per km2, putting high pressure on available arable lands (Bahige et al., 2018). In this region, cultivated land, especially on slopes, is increasingly eroded due to intensive cultivation methods, deforestation, and sub-optimal management practices, all contributing to massive runoff. These activities constitute major causes of land degradation and loss of arable land (Olsson et al., 2019) and result in reduced soil fertility, lower yields, and a reduced quality of agro-pastoral products.
Reclamation of degraded lands while at the same time using the productive lands sustainably is crucial for the prevention of expansion into forested and marginal lands. Agroecological practices have been shown to have a good potential for restoring lands and ensuring their sustainable use (Blanco-Canqui et al., 2015; Pyame et al., 2016; Gambart et al., 2020; Blomme et al., 2022). Agroecological intensification integrates ecological principles into farm and system management to improve agricultural performance while minimizing the impact of agriculture on the environment by reducing reliance on external inputs (Wezel et al., 2014). Cover cropping, agroforestry, intercropping, mulching, soil and water conservation, and efficient use of fertilizers are some of the common agroecological practices (Wezel et al., 2014; Gambart et al., 2020; Tsufac et al., 2021). Pyame et al. (2016) reported an agroforestry-grass fallow system referred to as the “Green Carpet”, which included intercropping, cover crops, the application of compost and no tillage, to have a high potential to improve soils on steep slopes, ensuring the creation/establishment of substantial carbon sinks and the rehabilitation of severely degraded land, while at the same time improving overall plot/field productivity. Agroforestry in such a system improves soil moisture and temperature, soil structure, soil nutrient recycling, soil erosion control and soil organic matter levels (Gliessman, 2015; Tsufac et al., 2021). Intercropping offers multiple services including improved light and nutrient use efficiency, better nutrient recycling especially when deep and shallow rooted crop are intercropped, weed suppression, improved soil micro-climate (temperature and moisture), reduced soil erosion, pest and disease suppression and higher total yields (Gliessman, 2015; Blomme et al., 2018, 2022). Cover cropping, a form of intercropping involves the integration of soil covering crops, often legumes, offering similar services as for intercropping, but often higher levels of weed suppression, soil erosion control, soil temperature and moisture remediation and improvement of soil structure (Gliessman, 2015; Blomme et al., 2022). Minimum tillage reduces soil organic carbon breakdown, improves carbon sequestration and soil fertility (Fiorini et al., 2020) whereas compost application improves carbon and nutrient stocks and soil physical, chemical and biological properties (Maris et al., 2021). These practices when deployed in combination can potentially support the restoration of degraded farms.
This study explored a modification of the “Green Carpet” approach (Pyame et al., 2016) for the restoration of degraded and abandoned farmlands in eastern DR Congo. The study specifically assessed, over a 4-year restoration period, the effects of an innovative agroecological package on (i) total biomass production and the overall productivity of a bean-maize-banana intercropping system; (ii) the chemical, physical and biological properties of the soil, and (iii) carbon sequestration in aboveground plant biomass. The agroecological package consisted of the application of small doses of inorganic fertilizer at trial establishment, readily available grasses and shrubs planted as hedges for the entire study period, mucuna planted as cover crop during the fallow season, and application of mulch and/or compost from the grass, hedge and mucuna cuttings.
2. Materials and methods
2.1. Site description
Field experiments were established on highly degraded and abandoned farmlands in two agroecological zones in South Kivu province, Democratic Republic of Congo (DR Congo). The first site is located at the INERA Mulungu Research Station (02° 19' 907” S, 028° 46' 540” E, 1,825 masl) in the territory of Kabare, while the second site is in the territory of Walungu, groupement of Mushinga (02° 45' 592” S, 028° 39' 591” E, 1,592 masl) (Supplementary Figure 1).
The soils at both INERA-Mulungu and Mushinga are Ferralsols (Heri-Kazi and Bielders, 2020). Variation in clay content is limited across the soil profile, except in the B horizon where it can range from 6 to 83%. The silt content varies from 1 to 23%. Ferralsols are desaturated and acidic, with low cation exchange capacity values: 7.5 cmol+ kg−1 on average in the A horizon and only 3.0 cmol+ kg−1 in B horizon. Their pH generally varies between 4.0 and 5.5. Most Ferralsols have base saturation rates below 50% with a large proportion of exchangeable aluminum (Luíz et al., 2002).
The Mushinga experimental site was located on a modestly sloping (1 to 3%) plot with poorer soils and was severely degraded. Cinchona trees (Cinchona officinalis) had been grown commercially on this site for over 50 years. Over this period, trees had been regularly harvested, by cutting off stems above soil level and stem cuttings were subsequently exported off field for Quinine extraction from the bark. No external inputs had been applied on these Cinchona fields, potentially resulting in a net export of nutrients. This aspect, coupled to the sloppy terrain and the poor soil type eventually resulted in the abandonment of the Cinchona tree plantation. The soils, at onset of the experiments, had a very low pH and base concentration (P, K, Ca, and Mg) (Table 1). The Mulungu experimental site was located on a steep slope (7–9%). Multiple years of crop production without specific efforts to control soil erosion resulted in severe soil degradation and low yields, and ultimately the abandonment of the land. The soils in Mulungu had a low soil carbon and nitrogen content (Table 1). The soil characteristics of these two sites are described in Ntamwira et al. (2017).

Table 1. Soil characteristics from soil samples collected in 2016 at the Mulungu and Mushinga experimental field sites.
2.2. Treatments
The field experiments were conducted over a 5-year period. A randomized complete block design with 8 and 10 blocks, respectively, at Mulungu and Mushinga was used. The blocks were arranged along the slope to minimize spatial variation [within blocks] in e.g., soil fertility and drainage due to the slope. Each block was divided into three plots and three treatments/ land management packages (two packages mimicking farmers practice and a novel agroecological package) randomized separately within each block, each management package appearing once in each block. A spacing of 3 and 1 m was left between the blocks and plots/treatments within blocks, respectively, to minimize shading and drifting of soil amendments. Within each of the treatment plot and at different time periods three crop spp., beans (Phaseolus vulgaris), maize (Zea mays) and banana (Musa spp.) were planted either as sole crops or jointly as intercrops, as detailed in the subsequent sections below and Table 2. The land management practices served as the independent variables while the performance of the crops; and soil chemical and bio-physical properties under these packages served as the dependent variables.
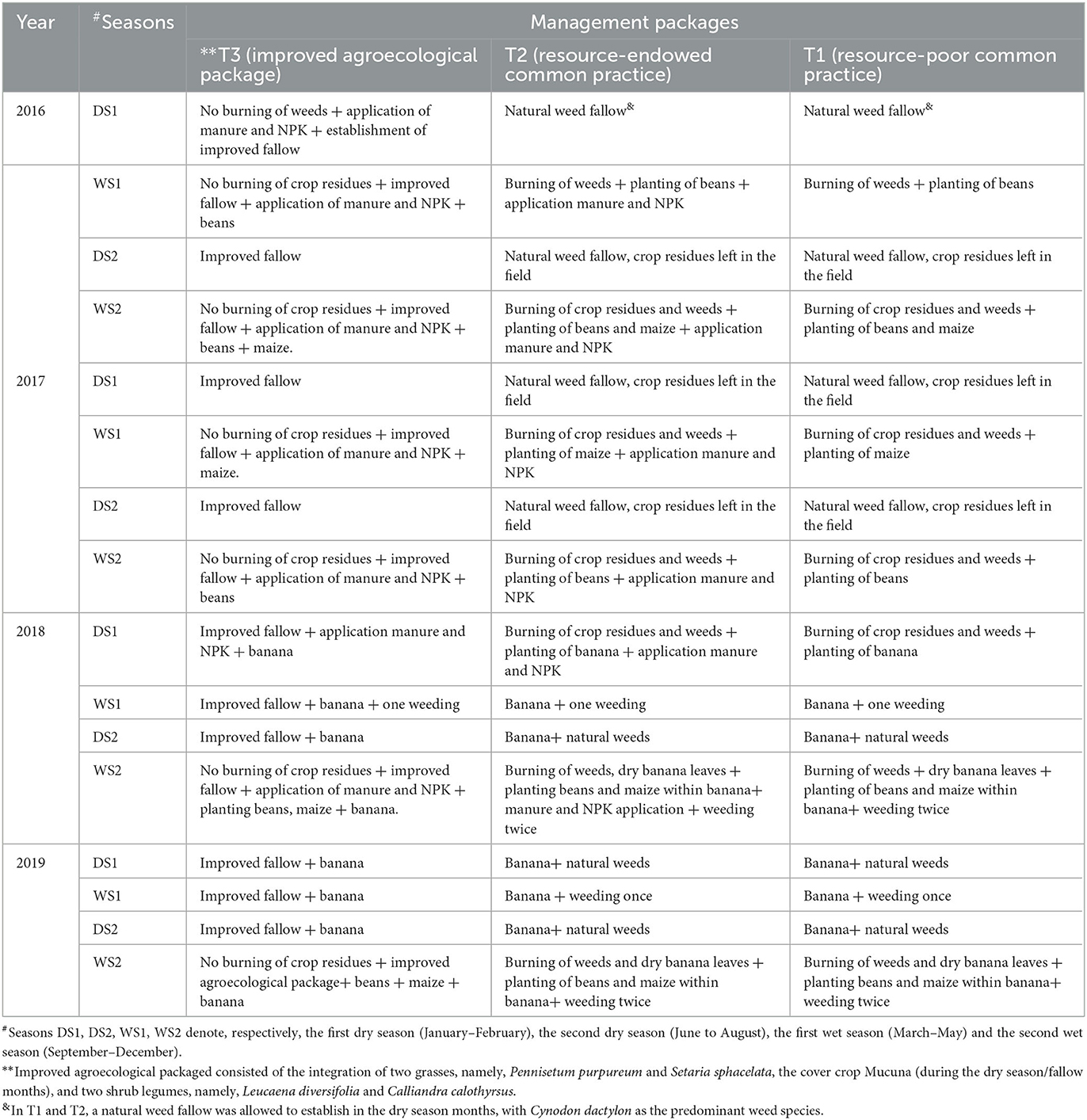
Table 2. Description of the management packages established over a four-year period at Mushinga and Mulungu, in South Kivu Province, Democratic Republic of Congo.
The first management package (T1) consisted of the burning of crop residues and weeds, a natural weed fallow, and no chemical fertilizer (NPK) or manure application (Table 2). This package mimicked the resource-poor farmer's practice, that dominates in the study landscape. Cynodon dactylon was the predominant weed species in the natural weed fallow that established in the dry season months of January to March and June to September.
The second management package (T2) combined the burning of weeds and crop residues, a weed fallow, and the application of NPK (17:17:17) and manure at planting from year 1 to year 3 (T2). This mimicked the practices of resource-endowed farmers who have livestock to produce manure and/or can afford chemical fertilizers for their crops. Like T1, a natural weed fallow with Cynodon dactylon as the predominant weed species was allowed to establish in the dry season months.
The third (T3) was a novel agroecological package that included i) the application of a combination of manure and chemical fertilizer in the first three years (Y1 to Y3), ii) a mixture of leguminous shrubs (Calliandra calothyrsus, and Leucaena diversifolia) and grasses (Pennisetum purpureum and Setaria sphacelata) planted as hedges and in relay with the food crops (Figure 1); and iii) a Mucuna pruriens cover crop only established in the dry season months (January–March and June–September) instead of a weed fallow. The shrubs and grasses used in T3 were selected according to their performance and biomass production from field trials carried out by Ntamwira et al. (2019) in eastern DR Congo. In these trials, yield of Pennisetum sp. (29.3 t/ha) and Setaria sp. (19.5 t/ha) outperformed Brachiaria sp. (13.8 t/ha) and Tripsacum sp. (13.8 t/ha) while Calliandra sp. (11.0 t/ha) and Leucaena diversifolia (8 t/ha) outperformed Leucaena leucocephala (3 t/ha) and Albizia chinensis (2 t/ha). Manure and NPK were crucial for enabling the grass and shrub species to pick up/ establish in T3. In both T2 and T3, composted cow dung manure at a rate of 20 t DM ha−1 and micro-doses of NPK (17:17:17) fertilizer at 50 kg ha−1 were applied annually in the planting holes of all newly established crops, during the first 3 years.
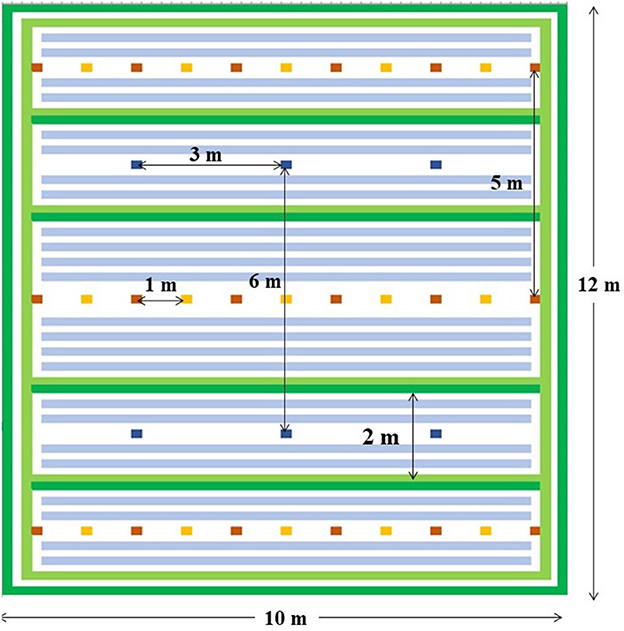
Figure 1. Field layout of the plot with the agroecological package, T3 a green bands represent the grasses (Pennisetum and Setaria), orange and red cells represent the shrubs (Calliandra and Leucena), light blue-gray bands represent mucuna lines (in dry season months only), while dark blue cells represent banana or plantain mats. Only banana or plantain mats are present in T1 and T2 (natural weed fallow with burning, with or without the application of manure and NPK).
The grasses and shrub trees in T3 were established in January 2016 using the residual soil moisture from the main rainy season (September–December). The two grass species were planted in two parallel rows, 25 cm apart, with each grass species occupying a row. The two parallel rows were planted as a hedge around the plot and in alleys spaced at 2 m apart within the whole plot to increase overall plot biomass, maximize erosion control and water infiltration (Figure 1). Within the rows, the grasses were spaced 25 cm apart. The two shrub species were planted at a spacing of 1m within rows and 5 m between rows of the shrubs. Plants of the two shrub species were alternated across a line. A total of 33 plants for both shrub species were planted per plot (this comprised 3 lines of 11 plants), resulting in a total of 330 plants across the entire experimental field at Mushinga and 264 across the plots in Mulungu.
The grasses (within plot and hedge) were cut at the beginning of each annual cropping season, when the land was being prepared for bean and maize sowing. Thus, the first cutting of grasses in T3 occurred in March 2016, at 30 cm aboveground, just before sowing the beans. During the annual crop growing season, grasses were cut every 2 weeks to reduce shade for annual crops and to provide mulch. The grass cuttings were retained within T3 as mulch material. Cutting of shrubs (50 cm aboveground) in T3 started at 2 years after trial establishment and was done at the onset of the annual cropping season. A large number of stakes were obtained from the first pruning of the shrubs and used to support the climbing beans, while the remaining shrub biomass and subsequent biomass from shrub pruning were applied as mulch within the T3 plots.
In T3, Mucuna sp. was planted at trial establishment (i.e., Jan. 2016), and subsequently (from year 1 to year 4), at 1 month before the harvest of annual crops (i.e., in May and December) and allowed to grow during the subsequent dry season months. Mucuna was planted across the whole T3 plots between the grass and shrub alleys at a plant spacing of 0.5 x 1 m.
In the 4th year, compost generated from materials within T3 was applied in September instead of manure and NPK, at a rate of 20 tha−1. The materials for composting comprised of cuttings/ pruning's of Pennisetum sp. and Setaria sp. grasses, the leguminous shrubs, and crop residues following crop harvest at the end of May 2019. The materials for compost were heaped in the alleys between the T3 plots and a thin layer of topsoil sourced from within the T3 plot was used to cover the compost heaps. The materials were then composted over a three-month period before use in September.
Each block measured 32 m by 12 while the management packages were assigned to plots measuring 10 x 12 m (Figure 1). The number of replications at INERA-Mulungu were limited by the size of the degraded farmlands. For all three management packages, half of the blocks (i.e., 5 at Mushinga and 4 at Mulungu) were cropped during each growing season, while the other half were left under fallow to maximize soil fertility restoration. For example, in Y3, beans and maize were sown under banana in half of the plots, while the other plots with banana remained under fallow. During the subsequent cropping season, the part that was fallowed was brought under cultivation, while the part that was cropped returned to fallow. Minimum tillage to sow seeds was applied in package T3, whereas the soil was tilled with a hand hoe to remove the weeds in T1 and T2.
Due to the poor state of the soils at both experimental locations at establishment of the experiments, only the bean crop which has a lower demand for nutrients, compared to the maize and banana crops, was introduced in the first season of the trials. The maize crop was introduced as an intercrop to beans in the second annual cropping season of year 1 (i.e., September), 9 months after trial establishment. It was presumed that the fallow and legume crop would have improved the soil conditions to support the maize crop. Across the three land management treatments, to prevent buildup of bean diseases, only maize was planted at 15 months after trial initiation (March of year 2). The banana crop which has the highest demand for nutrients was introduced as a third intercrop in the third year (September 2019), as gradual improvements in performance of the component intercrops, indicating improvement in soil fertility, was observed. Beans were planted at a spacing of 25 x 50 cm, while maize had a spacing of 50 x 100 cm.
For the banana crop, a wide spacing of 3 x 6 m was used to minimize competition with beans and maize, or for T3 with the Mucuna cover crop, the grasses/shrub hedges, and annual crops. This resulted in six Musa plants per 120 m2 plot. In each banana planting hole, 40 kg of decomposed cow dung manure and 100 g of NPK was applied at planting for treatments T2 and T3. At establishment of the banana crop in T3, the lines of grasses per plot were halved (resulting in a new spacing of 200 cm x 25 cm between the grasses) to minimize competition between banana and the grasses and annual crops (beans and maize). The planting lines of grasses, shrubs, and food crops were perpendicular to the slope, to limit the occurrence of erosion. A summary of the detailed treatment combinations over a period of 4 years is provided in Table 2.
2.3. Data collection
The collected data comprised, aboveground biomass yield for the grasses, mucuna, shrubs and crops; legume grain yield, banana bunch weight, legume root nodulation, soil earthworm population, root length density and soil physical and chemical properties.
Fresh aboveground biomass was only measured during or at the end of the last wet season (September-December) of the fourth year of the experiment. For the grasses, shrubs, Mucuna and weeds, fresh samples were cut and weighed to obtain the fresh biomass yield per plot. 500 g of fresh biomass weight per species was subsequently dried in an oven at 105°C for 48 h, to determine the total dry biomass. The dry matter yield per hectare and per species was obtained through extrapolation of plot yields.
Grasses were cut on two rows in the middle of the plot between two banana plants, over a 1 m length in two different locations per plot of 120 m2. Shrub species samples were cut from a 10 m long row in the middle of the plot. For mucuna and weeds, the aboveground biomass samples were collected from an area of 1 x 1 m, and from three randomly selected locations within each plot.
Bean aboveground biomass was assessed when at least 50% of the plants had formed pods. All bean plants in a 1 m2 section located in the middle of each treatment plot, totaling 120 bean plants, were harvested, and weighed to determine the fresh aboveground biomass weight. A 500 g sub-sample was subsequently dried in an oven at 105°C for 48 h, to determine the total (dry) biomass. Dry matter yield per hectare was obtained through extrapolation of plot aboveground biomass yields.
For maize, plants were cut at soil level at harvest time, on a 10 m row per variety, in rows located in the center of a plot. A total of 126 maize plants were hence assessed per plot. Dry biomass yields were determined as described above. For the banana crop, plant biomass was assessed at bunch harvest. Bunch harvesting for T3 was completed in the fourth year, while harvests in T1 and T2 plots were completed in the fifth year. The banana pseudostem without bunch, the leaves, and bunch were weighed separately at bunch harvest to determine fresh biomass weights. A total of 48 and 60 banana plants were harvested and assessed per management package at Mulungu and Mushinga, respectively. Dry matter yield was determined as described above.
Bean grain yields were assessed at the center of each plot in a sub-plot of 10 x 3 m comprising 24, 10 m-long rows. A total of 1,968 bean plants were hence assessed per plot. The yield of dry bean seeds per hectare was obtained by extrapolation of the plot yield. With regards to maize grain yield, six middle lines out of the 12 lines, containing a total of 228 maize plants, were assessed per treatment plot. To determine banana bunch yields, 48 and 60 banana plants per treatment combination were used at Mulungu and Mushinga, respectively. Crop yields per plot were extrapolated to obtain yields per ha, using a total of 556 Musa spp. plants per ha.
The assessed soil physical traits included soil texture, soil moisture, bulk density coupled with soil porosity and soil surface temperature. To determine the moisture content and bulk density of the soil, soil samples were taken in each plot at two locations in the middle of the plot. These soil samples were collected during the dry season (August) from the 0–5 cm, 10–15 cm and 15–20 cm soil layers. This period represents a time when water availability for plants is limited. A cylinder of 5 cm in diameter and 5 cm in height was used. Six samples were taken from the middle of each plot giving a total of 48 and 60 samples for Mulungu and Mushinga, respectively. Soil samples were weighed to determine the fresh weight and were subsequently oven dried at a temperature of 105 C, until a constant weight was obtained. The particle size density was obtained by dividing the dry mass of the sample (the constant weight obtained after drying in the oven (dry soil) by the volume of the Koppeky cylinder (100 cm3). The porosity value was deducted from the soil density. The following equation (GLOBE, 2014) was used:
Where P is the porosity, Bd is the bulk density and Pd is the particle density of the sample.
The soil temperature was measured at 5 cm soil depth during the dry season using a Hotbred thermometer with a height of 30 cm graduated from −5° to 65°C. Soil temperature was assessed at three points (with 3 m distance between individual points) in the center of each plot, giving a total of 24 measurements per management package at Mulungu and 30 at Mushinga.
Earthworms (Lumbricus terrestris) presence and numbers were manually assessed during the raining season (between November and December) of the fourth year (2019) of the experiment by exploring a 25 x 25 x 30 cm earth block as proposed by Ruiz and Lavelle (2008). First, a 20 cm wide strip was cleared around the entire perimeter of a block, and soil was subsequently removed from this perimeter up to 30 cm depth. The block was then assessed from top to bottom starting with the organic layer above the soil surface, the 0–10 cm soil layer, the 10–20 cm soil layer and finally the 20–30 cm soil layer. For each section/layer, the soil was carefully disintegrated by hand or using a knife and all the earthworms were collected and counted. Two locations were sampled per plot, and a total of 64 and 80 samples were collected per management package at Mulungu and Mushinga, respectively. The number of individual earthworms per 1 m2 of soil was calculated for each location and layer/depth.
Root length density was assessed just next to the grass species in T3. Eight soil-root samples were collected at 10 cm from a line of each grass species, per repetition, in the center of each plot using the Koppéky cylinder of 100 cm3 volume up to a soil depth of 5 cm. In T3, most roots belonged to the grass species. In T1 and T2, soil-root samples were collected in the middle of a plot using the same methods as applied in T3. All soil-root samples were collected in Y4 (December 2019). Per management package, a total of 64 and 80 soil-root samples were collected at Mulungu and Mushinga, respectively. These roots were sorted and weighed (dry weight) at the soil laboratory of the INERA Mulungu Research Station. The mean values from the samples were then extrapolated to 1 m2 of soil.
Bean root nodulation was assessed at flowering stage. This represents that physiological stage when the number and weight of nodules is highest (Van Schoonhoven and Pastor-Corrales, 1992). This evaluation consisted of randomly harvesting 20 bean plants for per treatment plot and counting the active root nodules per plant.
Soil chemical characteristics were determined at trial establishment and 4 years after trial establishment to determine treatment effects on the various soil characteristics. Soil chemical analysis for soil pH, C, OM, P Mg, Ca, K, Al3+ and total N was carried out according to the methods of Pauwels et al. (1992). Soil carbon was determined by the Walkley-Black method, i.e., the oxidation of the organic matter by a mixture of potassium dichromate and sulphuric acid. Nitrogen was determined by colorimetry in sulphuric acid solution and selenium using spectrophotometer. Soil pH was measured from a 1:2.5 soil: water extract. Exchangeable cations, K, Ca and Mg were extracted from the soil using 1M ammonium acetate buffered at pH7. The extraction of K was done on a flame photometer, while Ca and Mg were determined on atomic absorption spectrophotometer. Phosphorus was analyzed by colorimetry using dried Potassium dihydrogen phosphate (KH2P04) dissolve in deionized H20 then dilute to 500ml. Exchangeable Aluminum Al3+ was extracted with 1 mol L-1 KCl solution in deionized H20.
The determination of the carbon stock was carried out as described by Kiaire et al. (2013). This consisted of the quantification of accumulated carbon in the aboveground biomass of (i) grasses cut at 30 cm from the ground, (ii) shrubs cut at 50 cm from the ground for the different fractions of the shrub (stems, branches, leaves, flowers, and fruits) and (iii) mucuna aboveground biomass for T3, while accumulated carbon in the aboveground biomass of weeds was assessed in T1 and T2. The aboveground sequestered carbon was derived from the aboveground dry biomass (AGB) values, on the assumption that 50% of dry biomass is made up of carbon (Lal, 2010; Kiaire et al., 2013).
Data analysis was carried out using the statistical software R version 4.0.2 (R Core Team, 2020). The significance level was established at a p < 0.05. To determine the effects of the management packages on observed parameters a one-way ANOVA was used. This analysis was followed by a post-hoc Tukey test for multiple comparisons of means.
3. Results
3.1. System aboveground biomass
The aboveground biomass (AGB) of the crops, weeds, grasses, shrubs and the overall total varied significantly between the management treatments at both sites (p < 0.001). The total and crop (banana, beans and maize) biomass was consistently and significantly higher in the agroecological package T3, with the least biomass yield obtained under T1 and T2 (Figure 2; Table 3). For example, total AGB varied between 17.4 and 60.4 t/ha at Mulungu and 11.1 and 39.9 t/ha at Mushinga whereas banana AGB varied between 7.8 to 14.4 at Mulungu and 0.4 to 5.8 t/ha at Mushinga in T3 and T1, respectively (Table 3). The addition of manure and NPK coupled with burning of weeds and crop residues in T2 only significantly (5% LSD) improved banana and bean AGB yields at Mushinga when compared to T1 where weeds and crop residues were burnt with no addition of manure and NPK (Table 3). In package T3, the grasses (66% to 79% of biomass), banana (14% and 24%), and shrubs (1.2 to 13%), were respectively, the major contributors to the total biomass yield. In contrast, the banana crop and weeds accounted for a greater portion (90–100%) of the total biomass yield in packages T1 and T2. Weed volumes in the T1 and T2 plots were not significantly different (at 5% LSD) at both sites but were significantly higher (p < 0.001) than in the T3 plots. In T3, zero weed biomass was recorded in both sites at the end of the dry season (i.e., end of August), when mucuna was totally covering the soil surface. in the T3 plots (Table 3).
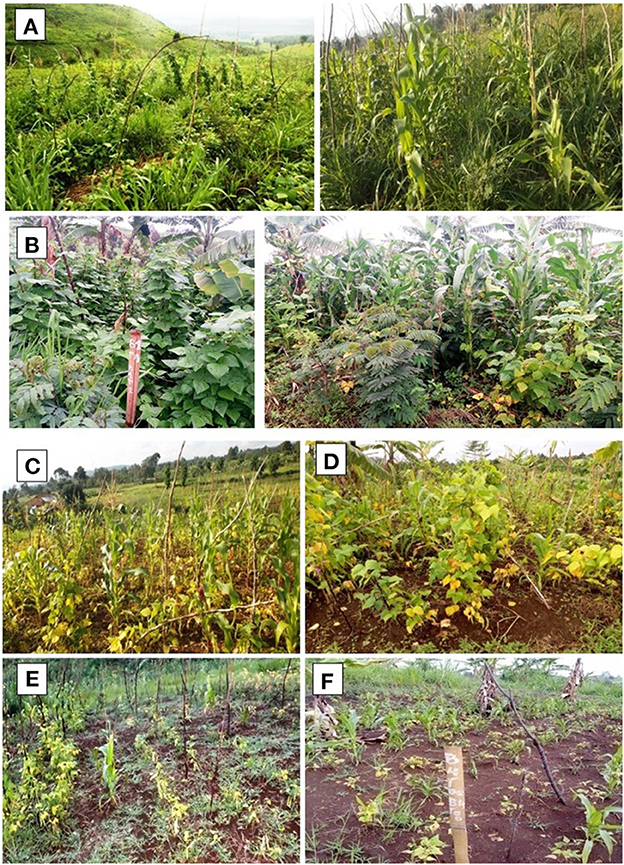
Figure 2. T3: The field with the modified agroecological package during the first year (A) and the 4th year (B) after trial establishment in Mulungu, T2: burn practices with NPK and manure application during the first year (C) and 4th year (D) and T1: burn practices without NPK and manure application during the first year (E) and 4th year (F).
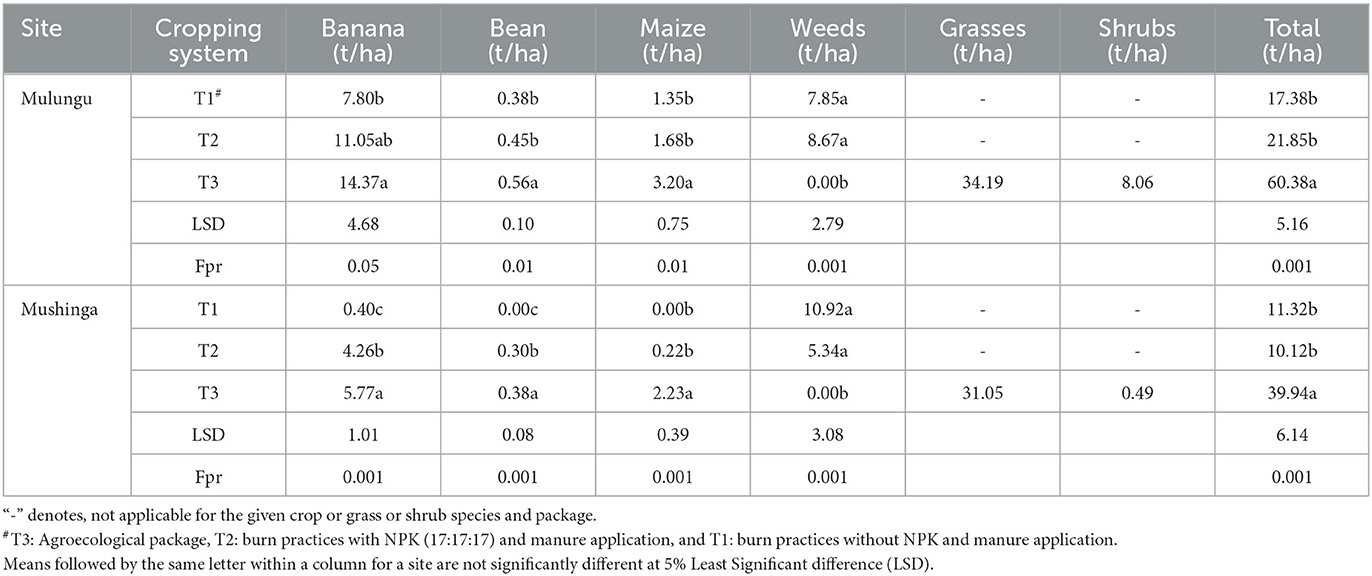
Table 3. Mean aboveground biomass yield (dry weight basis) for different crops, weeds, grasses and shrubs and total biomass yield for three land management packages at two experimental sites in eastern Democratic Republic of Congo assessed after 4 years of experimentation.
3.2. Bean and maize grain, and banana bunch yields in year four
Significant differences (p < 0.001) were observed in bean and maize grain yields between the management packages in both study sites. Within both sites, yields were significantly higher in the T3 innovative agroecological package compared to T1 and T2 (Figure 3). Significant differences were only observed between T1 and T2 at the heavily degraded Mushinga site.
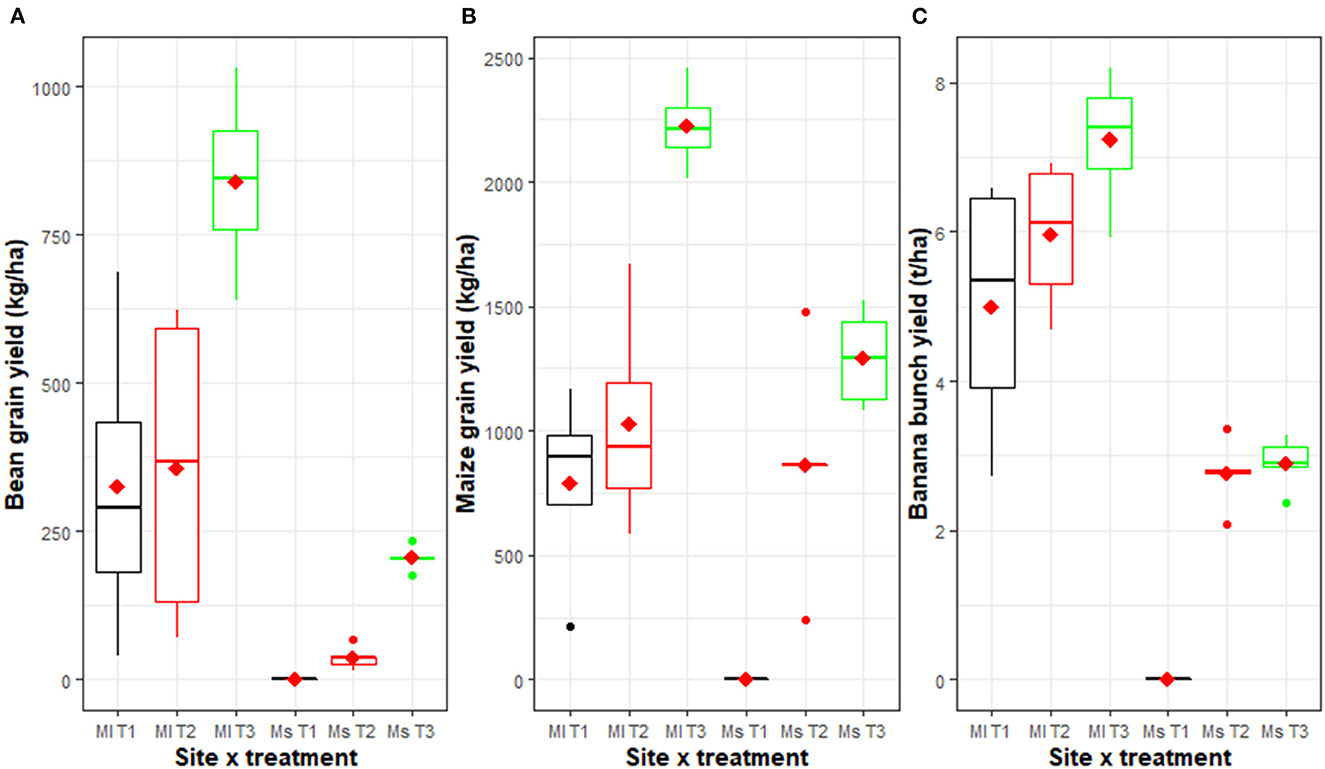
Figure 3. Bean (A) and maize (B) grain yields, and banana bunch yields (C) assessed after 4 years of experimentation under three different management practices and in two sites [Mulungu (Ml) and Mushinga (Ms)]. The management practices included T3: an improved agroforestry practice in which grasses, shrubs, mucuna cover crop, NPK and manures had been integrated, T2: burning of weeds and crop residues with NPK and manure application, and T1: burning of weeds and crop residues without NPK and manure application. The horizontal line within each box is the median, while the diamond within the box is the mean. The lower and upper boundaries of the boxes are respectively, the 25th and 75th percentile; the bars/whiskers below and above the box are the 10th and 90th percentile and points beyond 10th and 90th percentiles are outliers. Significant differences (p < 0.001) were observed between packages within each site.
The agroecological package T3 significantly improved banana yields (P < 0.001) in both study sites (Figure 3). At Mulungu, average Musa (beer banana and plantain combined) yields, varied from 6.2 t/ha to 8.5 t/ha, 4.7 to 7.2 t/ha and 3.9 to 6.1 t/ha for the T3, T2 and T1 plots, respectively. At Mushinga, banana yields ranged from 2.5 to 3.4 t/ha, 1.9 to 2.8 t/ha and 0 t/ha for T3, T2, and T1, respectively. T1 had the lowest bunch yields, while T2 had intermediate yields.
3.3. Effect of the agroecological package on soil physical properties
Mean soil temperature varied significantly (p < 0.001) between packages at both sites (Figure 4). A lower mean soil temperature was recorded in T3 (21.9–23.0°C), while the highest (27.2–27.4 °C) was found under T1. Only slight and non-significant reductions (at 5% LSD) in temperature were observed when manure and inorganic fertilizers were added in addition to burning weeds and crop residues. A mean reduction of 5.2°C at Mulungu and 4.4°C at Mushinga was recorded under T3 in comparison with the common practice of burning weeds and crop residues without application of manure and inorganic fertilizers (Figure 4A).
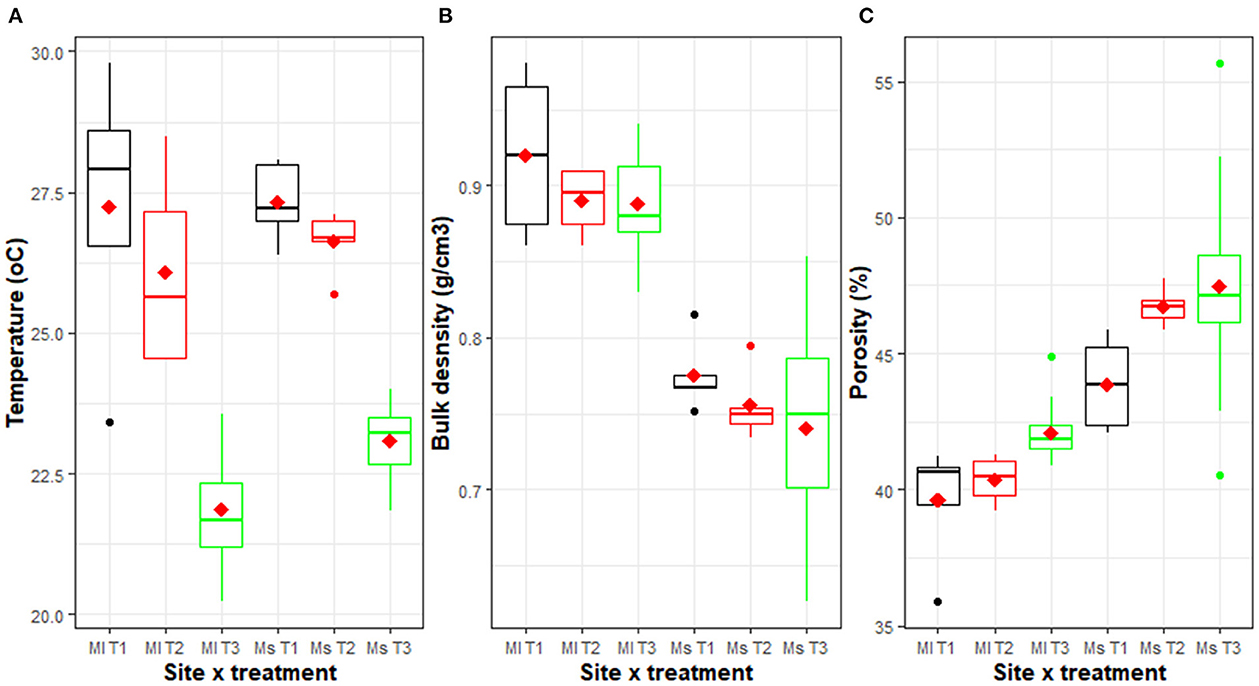
Figure 4. Soil temperature (A), bulk density (B), and porosity (0–20 cm soil layer) (C) across three management packages (T3, T2 and T1) and two sites [Mulungu (Ml) and Mushinga (Ms)], measured 4 years after trial establishment. The management practices included, T3: an improved agroforestry practice in which grasses, shrubs and mucuna cover crop had been integrated, T2: burning of weeds and crop residues with NPK and manure application, and T1: burning of weeds and crop residues without NPK and manure application. The horizontal line within each box is the median, while the diamond shaped symbol within the box is the mean. The lower and upper boundaries of the boxes are respectively, the 25th and 75th percentile; the bars/whiskers below and above the b ox are the 10th and 90th percentile and points beyond 10th and 90th percentiles are outliers.
Though not significantly different (p > 0.05), soil bulk density values at both sites were lower under T3 compared to T2 or T1 (Figure 4B). For the soils of Mulungu, soil bulk density was 0.89 g/cm3 for T3 and 0.92 g/cm3 for T1, while at Mushinga it was 0.74 g/cm3 for T3 and 0.78 g/cm3 for T1. Bulk density improvements were also observed with the addition of the manure and inorganic fertilizer in T2 even though weed and crop residues had been burnt (Figure 4B).
T3 had higher levels of soil porosity compared to the T1 and T2, with significant (p = 0.01) and non-significant (p = 0.059) differences at Mulungu and Mushinga, respectively (Figure 4C). Soil porosity varied from 39.6% in the T1 to 42.1% under T3 in Mulungu, and from 43.8% under T1 to 47.6% under T3 at Mushinga. In Mushinga, soil porosity was profoundly increased by addition of manure and NPK (T2) compared to the common practice of only burning the weed and crop residues.
3.4. Soil biological properties as affected by the agroecological package
3.4.1. Earthworm populations
At both sites, a significantly higher (p < 0.001) average number of earthworms was observed in T3 compared to the T1 and T2 packages in which weeds and crop residuals were periodically burnt. A mean of 1,932 earthworms/m2 was observed in T3 compared to only 133 earthworms/m2 in T1 at Mulungu (Figure 5A). At Mushinga, a mean of 84 earthworms/m2 was observed in T3 compared to 0 earthworms/ m2 in T1 at Mushinga. At Mulungu, the application of manure and NPK (T2) coupled with burning of weeds and crop residues also had significantly higher (p < 0.001) number of earthworms.
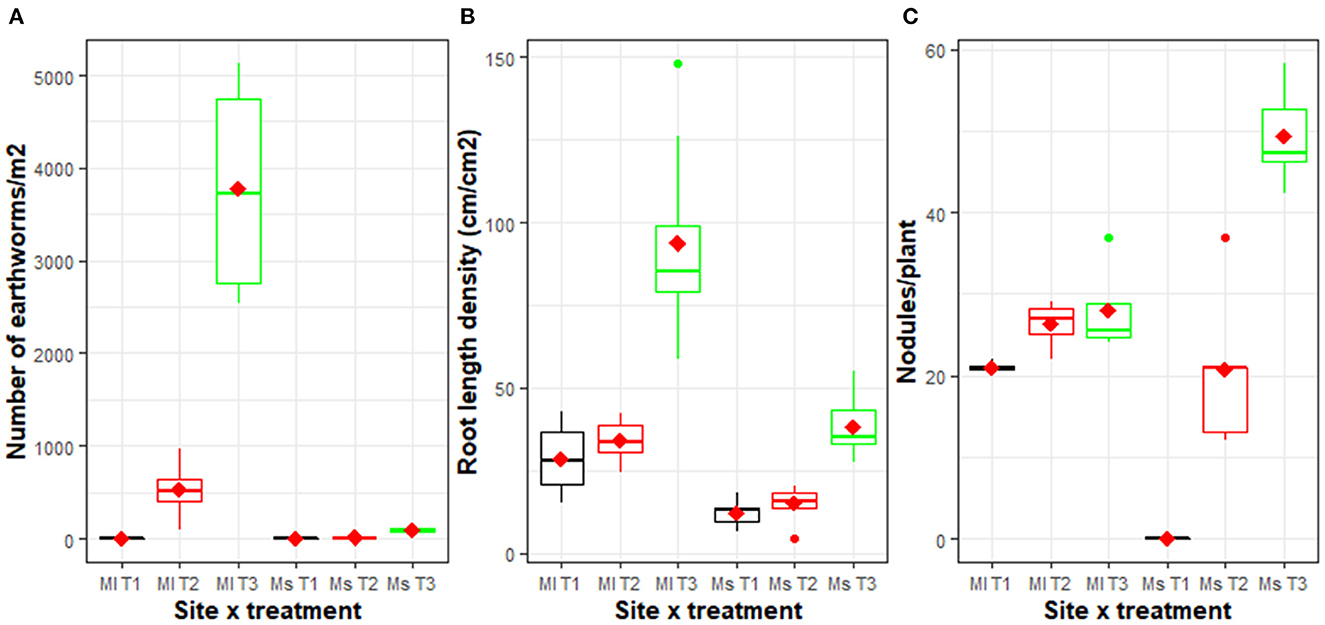
Figure 5. Number of earthworms/m2 (A), aggregated root length density (cm/cm2) (B), and the number of root nodules per bean plant (C) in three management packages across two sites [Mulungu (Ml) and Mushinga (Ms)] measured after 4 years of experimentation. Root length was measured in the 0–50 cm soil layer while earthworm populations were determined in the 0–30 cm soil layer. The management practices included T3: an innovative agroecological package in which grasses, shrubs and mucuna cover crop had been integrated; T2: burning of weeds and crop residues with NPK and manure application; and T1: burning of weeds and crop residues without NPK and manure application. The horizontal line within each box is the median, while the diamond shaped symbol within the box is the mean. The lower and upper boundaries of the boxes are respectively, the 25th and 75th percentile; the bars/whiskers below and above the box are the 10th and 90th percentile and points beyond 10th and 90th percentiles are outliers.
3.4.2. Root length density
Across the two sites, total root length density (RLD) of all plant species (grasses, shrubs, mucuna, weeds and crops) measured in the topsoil layer (0–50 cm depth) was significantly higher (p < 0.001) in T3 package compared to T1 and T2. For example, RLD was 91 cm/m2 at Mulungu and 39 cm/m2 at Mushinga in the T3 plots compared to 29 cm/m2 at Mulungu and 12 cm/m2 at Mushinga for T1 (Figure 5B). The application of manure and NPK in T2 did not significantly improve RLD compared to T1 (Figure 5B).
3.4.3. Bean root nodulation
The agroecological package, T3 relative to T1 (burning of weeds and crop residues) significantly (p< 0.001) improved root nodulation in beans, especially at Mushinga (Figure 5C). Mean number of root nodules were 28 and 49 for T3 compared with 21 and 0 for T1 at Mulungu and Mushinga, respectively. The application of manure and NPK in T2 also significantly (5% LSD) improved root nodulation in beans, compared to T1. At Mulungu, no significant difference (5% LSD) was however observed in number of root nodules between the T3 and T2 packages (Figure 5C).
3.5. Soil chemical proprieties
The three management packages differently impacted the soil chemical attributes across the two sites. Relative to the soil chemical composition at onset of the trials, soil N, P and Al3+ declined while soil pH, OM, C, K, Ca, and Mg had increased in the T3 plots at Mulungu in the fourth year of the experiment (Table 4). Significant changes (p < 0.05) were observed for OM. C, P, K, Mg and Al, though T3 also outperformed T1 and T2 for soil N and P levels. Soil Al3+ in the T3 declined to zero while soil pH only increased. At Mushinga, soil pH, K and Ca increased significantly (p < 0.05) under T3, while significant declines occurred for Mg and Al3+ under T3. Declines though non-significant (p> 0.05 or 5% LSD) occurred for C, N, OM, and P under T3 at Mushinga (Table 4). For all chemical properties, T3 outperformed packages T1 and T2 (Table 4).
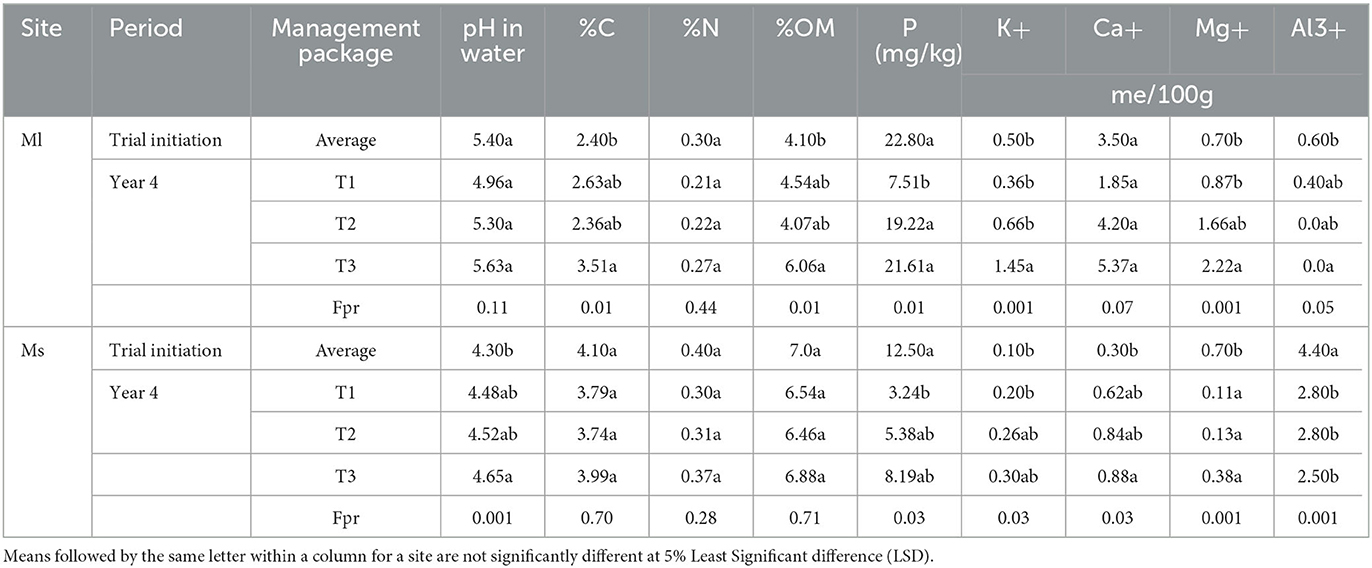
Table 4. Soil chemical properties at trial initiation and during the fourth year of trial at Mulungu (Ml) and Mushinga (Ms) sites in eastern Democratic Republic of Congo.
3.6. Aboveground plant biomass carbon stocks as influenced by the agroecological package
The agroecological package (T3) significantly (p < 0.001) increased the amount of sequestered organic carbon (OC) in both sites compared to T2 and T1. The amount of sequestered organic carbon in the T3 plots was 21.4 and 31.5 t/ha in Mushinga and Mulungu, respectively, compared with 7.4 and 7.8 t/ha in T2 and 4.3 and 6.8 t/ha in T1 (Figure 6). The differences in the amount of sequestered OC between T2 and T1 were not significant at 5% LSD.
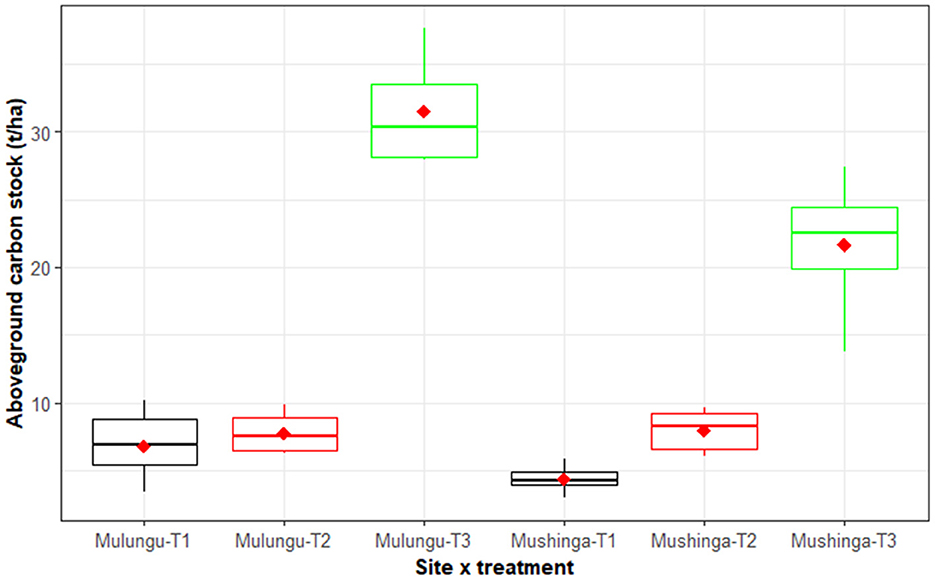
Figure 6. Mean organic carbon stocked in aboveground biomass for three management practices across two sites (Mushinga and Mulungu), assessed after 4 years of experimentation. The management practices included T3: an agroecological package integrating grasses, shrubs and mucuna cover crop, T2: burning of crop residues and weeds with NPK and manure application, and T1: burning of crop residues and weeds without NPK and manure application. The horizontal line within each box is the median, while the diamond shaped symbol within the box is the mean. The lower and upper boundaries of the boxes are, respectively, the 25th and 75th percentile; the bars/whiskers below and above the box are the 10th and 90th percentile and points beyond 10th and 90th percentiles are outliers.
4. Discussion
4.1. Effect of the agroecological package on total biomass of the agrosystem in year 4
The high total biomass yield under T3 can be attributed to grasses, shrubs and mucuna that established well in the poor soil conditions. The grasses, shrubs and mucuna cover crop also provided a continuous cover protecting the soils against erosion and improved soil physical and chemical properties (c.f. Figures 4, 5). This is supported by the fact that crop (banana, beans, maize) biomass yields under this package were more superior than the controls. Pyame et al. (2016) reported the high soil moisture and organic matter content in a similar agroecological package in DR Congo to boost plant growth. Soleymani et al. (2016) and Nyambo et al. (2020) reported crop residue retention and the establishment of cover crops to contribute to the improvement of various soil properties and crop yields.
The suppression of weeds in the T3 package can be mainly attributed to the munuca crop cover that smothered weeds. The shrubs and grasses also offer shading that could have also contributed to weed suppression. Weed biomass suppression in banana-mucuna intercropped plots in Eastern of DR Congo was also reported by Blomme et al. (2022). This is further supported by the fact that weed biomass was significantly higher in the T1 and T2 plots that were left open for a natural weed fallow to establish.
4.2. Bean grain, maize grain and banana bunch yields in the fourth year
Higher bean and maize grain yields observed in T3 can be attributed to multiple factors including a higher level of soil nutrients (Table 3) and better soil physical (c.f. Figure 4) and biological (c.f. Figure 5) properties. Package T3 followed by T2 generally had a higher concentration of N, P, and the basic cations, especially K that are crucial for optimal bean growth and yield. Several studies have reported soil N, P and K to be crucial for the growth and yield of both the bean and maize crop (Drevon et al., 2015). Soils in T1 and T2 were also more acidic. Acidity can limit the availability of some soil nutrients. For example, acidity has been reported to reduce the availability of soil P and coupled with low soil fertility has been reported to limit bean yields when weed and crop residues are burned at the onset of a cropping season (Thung, 1990). The T3 package improved soil moisture content, soil porosity, and earthworm population, collectively creating suitable conditions for the growth of crops. Agroecological practices such as minimum tillage, fallowing and ensuring a permanent ground cover have been reported to improve physical, chemical, and biological soil conditions for improved crop growth (Pyame et al., 2016; Das et al., 2019; Nyambo et al., 2020). According to Page et al. (2019) and Xiao et al. (2019) minimum tillage as practiced in T3, most often leads to higher yields compared to conventional tillage-based agriculture.
Banana yield under T1 at Mushinga was 0 t/ha possibly due to the highly degraded soils and soil acidity (especially Aluminum toxicity) that persisted over the 4 years of the trials (c.f. Table 4). Banana yields, respectively, increased across sites with the application of manure and NPK (T2), and in the agroecological package (T3). Soil nutrients can be replenished in the soil through organic matter and manure (Dhed'a et al., 2011). Large amounts of biomass were returned to the soil in T3 through the decomposition of the roots, litter and cuttings/pruning of the grasses, shrubs, and mucuna, stimulating the rooting of the banana plant, enhancing mineral absorption, and ultimately increasing banana yields. The components of this package helped to replenish the needed soil nutrients for banana growth and development. Banana uptakes a large amount of nutrients from the soil, especially K, N, and P (Nyombi et al., 2010; Taulya, 2013). Similar positive effects on banana growth and yield after mulch application or cover crop integration were reported by Dhed'a et al. (2011) and Das et al. (2019).
4.3. Soil physical and biological properties in year 4
4.3.1. Soil temperature
The average temperatures recorded during the dry season under agroecological package were significantly lower (about 4.4–5.2°C lower) than those under common practices of burning weeds and crop residues (T1 and T2, c.f. Figure 4). This can be attributed to the shading effect of the high and permanent soil cover arising from the mulch and shade provided by the mucuna cover crop, grasses, and shrubs. These cover crops could have thus helped to reduce direct solar irradiation, moisture loss through evaporation thus moderating soil temperature. These results are consistent with previous research that have shown improved ground cover to lower soil temperature (Das et al., 2019; Mubvumba et al., 2021). These studies reported ground covers to decrease soil temperature by reducing water loss through increased infiltration, reduced soil surface runoff and evaporation. In a similar study, the integration of agroforestry trees and cover crops reduced soil temperature by 8.4°C (Pyame et al., 2016). The slight drop in soil temperature with application of manure and inorganic fertilizers, despite the burning of weed and crop residues, can be attributed to improved crop growth arising from an improved availability of nutrients.
4.3.2. Soil bulk density
The higher clay and loam content at Mulungu, resulted in a more compact soil. Soil bulk density negatively correlates with soil pore space, while soil compaction decreases the number of large soil pores (Correa et al., 2019; Jalal et al., 2021). In the current study, the agroecological package had a 3.3–5.1% lower soil bulk density at the end of the fourth year across the study sites compared to the common practice of burning weeds and crop residues without fertilizer application (T1). A reduction in soil bulk density has been reported in similar studies integrating strips of grasses and shrubs within cropping systems (Pyame et al., 2016). The improved activity of soil macro-and micro fauna, stimulated by the agroecological practices could have also played a secondary role to plant roots and organic matter in improving the soil bulk density in T3. In this study, for example, the earth worm populations increased by 1,353% under the agroecological package, potentially improving breakdown of organic materials, nutrient recycling, and soil porosity and aeration due to their tunneling activities. Several studies have similarly reported earthworms to improve soil bulk density (Blanchart et al., 1999).
4.3.3. Soil porosity
Soil porosity (0–20 cm soil layer) in the T3 plots was 6.3 and 8.7% higher than the T1 plots at, respectively, Mulungu and Mushinga (Figure 4). This can be attributed to (i) increased root biomass in the soil, (ii) increased soil organic matter arising from decomposed roots and mulch from the intercrop components, and (iii) the improved population and activity of soil fauna, especially earthworms as demonstrated above. Conservation practices such as minimum tillage, the use of cover crops, the installation of hedges and grass bands have been reported to increase the organic matter content of the soil and ultimately soil porosity (Ouedraogo et al., 2017; Pheap et al., 2019). Mondal et al. (2019) also reported direct sowing of annual crops (e.g., maize or beans) under cover crop mulch without disturbing the soil through tillage improved soil porosity. Compared to T1 (burning of weeds and crop residues without manure and fertilizer), soil porosity increased by 2% to 6.6% when manure and NPK were applied following weed and crop burning, possibly through improved crop root mass due to improved nutrient availability and soil organic matter. Semida et al. (2014) reported a decrease in soil bulk density and a corresponding increase in soil porosity through the application of organo-mineral fertilizers.
4.3.4. The number of earthworms
The earthworm population increased by 522% when only manure and NPK were applied, whereas it increased by a staggering 3,779% in the agroecological package plots [T3] in Mulungu. The increased earthworm population can be attributed to the increased plant biomass in the soil and soil surface, coupled with the improved soil physical and chemical properties. Das et al. (2019) reported the differences in earthworm numbers per surface unit area to be linked to variations in soil ecological properties such as % soil cover, soil temperature, soil moisture content, % soil organic matter and soil porosity. In the current study, all these attributes were improved in T3 due to the agroecological practices. Higher densities of earthworms under plant cover were also reported by Teutscherová et al. (2021). Minimum tillage was practiced in the current trial, thus preventing a disruption of the soil structure. Various authors have also reported an increase in earthworm numbers when zero or minimum tillage is practiced instead of conventional tillage (Pyame et al., 2016; Fiorini et al., 2020). In contrast to the agroforestry practice in T3, burning of crop residues and weeds, in addition to depriving the earthworms of the needed organic material for food, the fires could have potentially killed some earthworms in the topsoil layers in the vicinity of burning weeds/ crop residues.
4.3.5. Total root length density
A significantly larger (p < 0.001) root length density was observed in the agroecological package, T3. This is attributed to the high root mass from the grasses. The grass P. purpureum and S. sphacelata were observed to contribute the most to root length density in the current study. P. purpureum has been reported to have a high potential for root production, to the point of forming a hairy mat with very important physical and especially biological properties in the restoration of severely degraded soils (Pyame et al., 2016). Das et al. (2019) also observed a higher volume of roots in plots with cover crops and cover crop residues compared to bare plots.
4.3.6. Bean root nodulation
Root nodulation was improved by the application of manure and NPK and the agroecological package relative to the where weeds and crop residues were burnt without addition of manure and NPK. These improvements in root nodulation can be attributed to the improved availability of carbon and nutrients to support nodulation coupled with the improvement in soil physical and chemical properties over the 4-year period due to manure and NPK application in T2, and the effect of grasses, hedges, and cover crop in addition to the nutrients supplied in T3. Soil moisture, soil temperature and nutrition have been shown to affect root nodulation in legume crops (Deak et al., 2019; Kasper et al., 2019; Ntamwira et al., 2021; Blomme et al., 2022).
4.4. Impact of agroecological practices on soil chemical properties
Soils at both experimental sites were degraded at the onset of the experiments. The soils at the two sites were poor in soil organic matter (SOM) (< 8%). A profound increment in SOM only occurred in T3. This can be attributed to increase in below and aboveground biomass from the grasses, shrubs and mucuna cover crop and the mulch they supply in the agroecological package. The cover from this practice potentially also minimized the direct solar irradiation thus slowing the breaking down of soil organic matter (Blanco-Canqui et al., 2015). Soil chemical properties such as organic matter and carbon content are reported to depend on cropping systems, plant species and land topography (Mayer et al., 2019; Xie et al., 2021). Mulching and no-till as practiced in the study also increase soil organic matter in the soil (Wang et al., 2019; D'Hose et al., 2020).
The soil pH was low and varied between 4.3 and 5.5 (c.f. Table 2) at onset of trial, indicating an advanced stage of degradation of these soils. This could have been exacerbated by the high content of exchangeable aluminum (0.6–4.4 meg/100 g of soil). Al3+ is responsible for increasing the concentration of H+ in soil thus, soil acidity (Toufiq, 2012). The profound improvements under the agroecological package could be attributed to the higher buildup of soil organic matter. Soil organic matter has been reported to improve the buffering capacity, thus cation exchange capacity of soils (Jiang et al., 2018). This is supported by the fact that, high declines in the exchangeable Al3+ of between 43 and 100% occurred in the agroecological package. Wouteres (1991) reported organic matter to contribute to neutralization of exchangeable aluminum in soils through forming complexes between aluminum and di- and tri-carboxylated acids, thus improving the fertility of soils. Declines in Al3+, albeit low, also occurred for controls, possibly due to either the effect of organic manure application and/ or crops established in the plots.
Though the initial total nitrogen (< 5%) levels were already low, further declines were observed possibly due to increased crop uptake and exposure of soil carbon to direct solar irradiation thus an increased volatilization of N. However, the agroecological package caused a high N accumulation, possibly due to N fixation from the mucuna cover crop and the shrub species. The high SOM and above ground cover, also helps in fixing N and minimizing N loss through volatilization. Similar trends to N were observed for soil phosphorus (<24 mg kg−1).
Profound improvements occurred for K and, Mg, Ca in the agroecological package, possibly due to better retention caused by the higher SOM and recycling of soil nutrients by the shrub and grass species. The decrease in most nutrients in the controls can be attributed to the fact that the micro doses used could not cover the nutrient requirements of all crops coupled to the continuous burning of organic residues.
These results illustrate that agroecological practices have a high potential for restoring degraded soils. However, these soil assessments were conducted after a four-year experimentation, a period that is short given changes is soil conditions can be subtle and slow. Thus, pronounced recoveries could have been realized in soil properties if the experiment had been allowed to run for a much longer time duration.
4.5. Aboveground plant biomass carbon stocks in year 4
A total of 78 to 80% more carbon was sequestered in the agroecological package (T3) compared with the common practice of burning weeds and crop residues (T1). Numerous studies have shown similar agroecological practices to promote carbon sequestration from the atmosphere into the soil-plant system (Ntamwira et al., 2018; Rahmati et al., 2020; Sanaullah et al., 2020). This agroecological package sequesters carbon unlike the slash-and-burn systems that release carbon into the atmosphere. Organo-mineral fertilizers did not significantly increase carbon sequestration. This suggests that application of organo-fertilizers may need to be supported with practices that build up soil organic matter for them to effectively sequester adequate carbon stocks. These results are in line with observations made by Béghin-Tanneau et al. (2019) who stated that carbon sequestration depends on fertilizer type and soil fertility management practices.
5. Conclusion
The results of this study highlight the significant effects of applied agroecological practices (split application of inorganic fertilizers at start, minimum tillage, intercrop with grasses and shrubs, a mucuna cover crop fallow, and integration of cuttings from grasses, shrubs, mucuna and crops as mulch and/ or compost) on restoration of soil health, carbon sequestration and production in degraded fields. The agroecological package improved soil physical properties (e.g., soil temperature, porosity, bulk density), soil chemical properties (e.g., Al3+, OM and K) and soil biological properties (earthworm population, and plant root density) and the performance of crops relative to the controls. The package also improved carbon sequestration relative to the common practice of burning weeds and crop residues with or without the application of inorganic inputs. Two fields with different characteristics and levels of degradation were used suggesting that the packages could have a wide application. Residue burning or removal, as is often practiced by farmers in eastern DR Congo, during field management/sowing was observed to be detrimental to soil health and ultimately crop production, thus should be discouraged. It is however important to note that the observed changes due to the agroecological package were cumulative and require a long-time duration. This latter information is crucial to minimize the risk of discouragement when using these approaches. The integration of livestock in this system to benefit from the biomass generated from the shrubs and grasses and at the same time to supply manure and meet other nutritional and income needs, could further improve the package.
Data availability contributions
The raw data supporting the conclusions of this article will be made available by the authors, upon request, without undue reservation.
Author contributions
JN conceived and developed the research concept, set up the field trials, collected laboratory and field data, conducted data analysis, result interpretation, and manuscript preparation and editing. WO and GB contributed to conducting data analysis, result interpretation, and manuscript preparation and editing. AL, DM, and BD contributed to conceptualization of field experiments and supervision of the study. WO, GB, and AL contributed to resource mobilization. All authors approved the submitted version.
Funding
This study was supported by funds from the Harvestplus and ECABREN project, the Belgian Directorate-General for Development Cooperation and Humanitarian Aid contribution to the CIALCA project and the OneCGIAR Regional Initiative for West and Central Africa.
Acknowledgments
We are grateful for the financial support from the Alliance of Bioversity International and CIAT through the Harvestplus and ECABREN Project, the CIALCA project funded by the Belgian Directorate-General for Development Cooperation and Humanitarian Aid, and the OneCGIAR Regional Initiative for West and Central Africa. Sincere gratitude and appreciation are extended to the team from the Institut National pour l'Etude et la Recherche Agronomiques (INERA-Mulungu) for helping with data collection.
Conflict of interest
The authors declare that the research was conducted in the absence of any commercial or financial relationships that could be construed as a potential conflict of interest.
Publisher's note
All claims expressed in this article are solely those of the authors and do not necessarily represent those of their affiliated organizations, or those of the publisher, the editors and the reviewers. Any product that may be evaluated in this article, or claim that may be made by its manufacturer, is not guaranteed or endorsed by the publisher.
Supplementary material
The Supplementary Material for this article can be found online at: https://www.frontiersin.org/articles/10.3389/fsufs.2023.1017341/full#supplementary-material
References
Akpo, M. A., Saidou, A., Balogoun, I., Yabi, I., and et Bio bigou, L. B. (2016). Evaluation de la performance des pratiques de gestion de la fertilité des sols dans le bassin de la rivière Okpara au Benin. Eur. Sci. J. 12, 1857–7881. doi: 10.19044/esj.2016.v12n33p370
Bahige, M. F., Fikiri, Z. J., and Baloka, K. (2018). Aménagement d'une zone tampon autour du Parc National de Kahuzi-Biega en haute altitude (Nord-Est) à Bugorhe et Irambi-Katana, dans le Territoire de Kabare, Sud-Kivu, RD Congo. Int. J. Innov. Appl. Stud. 23, 523–540.
Béghin-Tanneau, R., Guérin, F., Guiresse, M., Kleiber, D., and Scheiner, J. D. (2019). Carbon sequestration in soil amended with anaerobic digested matter. Soil Till. Res. 192, 87–94. doi: 10.1016/j.still.2019.04.024
Blanchart, E., Albrecht, A., Alegre, J., Duboisset, A., Gilot, C., Pashanasi, B., et al. (1999). Effects of earthworms on soil structure and physical properties. Earthworm manage tropic. Agroecosys. 5, 149–171.
Blanco-Canqui, H., Shaver, T. M., Lindquist, J. L., Shapiro, C. A., Elmore, R. W., Francis, C. A., et al. (2015). Cover crops and ecosystem services: Insights from studies in temperate soils. Agron. J. 107, 2449–2474. doi: 10.2134/agronj15.0086
Blomme, G., Ntamwira, J., and Ocimati, W. (2022). Mucuna pruriens, Crotalaria juncea, and chickpea (Cicer arietinum) have the potential for improving productivity of banana-based systems in Eastern Democratic Republic of Congo. Legume Sci. 4, e145. doi: 10.1002/leg3.145
Blomme, G., Ocimati, W., Groot, J. C. J., Ntamwira, J., Bahati, L., Kantungeko, D., et al. (2018). Agroecological integration of shade- and drought-tolerant food/feed crops for year-round productivity in banana-based systems under rain-fed conditions in Central Africa. Acta Hortic. 1196, 41–54. doi: 10.17660/ActaHortic.2018.1196.5
Correa, J., Postma, J. A., Watt, M., and Wojciechowski, T. (2019). Soil compaction and the architectural plasticity of root systems. J. Exp. Bot. 70, 6019–6034. doi: 10.1093/jxb/erz383
Das, A., Layek, J., Ramkrushna, G. I., Rangappa, K., Lal, R., Ghosh, P. K., et al. (2019). Effects of tillage and rice residue management practices on lentil root architecture, productivity and soil properties in India's Lower Himalayas. Soil Till. Res. 194, 104313. doi: 10.1016/j.still.2019.104313
Deak, E. A., Martin, T. N., Fipke, G. M., Stecca, J. D. L., Tabaldi, L. A., Nunes, U. R., et al. (2019). Effects of soil temperature and moisture on biological nitrogen fixation in soybean crop. Aust. J. Crop Sci. 13, 1327–1334. doi: 10.21475/ajcs.19.13.08.p1739
Dhed'a, D. B., Moango, M. A., and et Swennen, R. (2011). “La culture des bananiers et bananiers plantains en R.D.”, in Congo. Support didactique. Kinshasa: Edition Saint Paul Afrique. p. 82.
D'Hose, T., Debode, J., De Tender, C., Ruysschaert, G., and Vandecasteele, B. (2020). Has compost with biochar applied during the process added value over biochar or compost for increasing soil quality in an arable cropping system?. Appl. Soil Eco. 156, 103706. doi: 10.1016/j.apsoil.2020.103706
Drevon, J. J., Abadie, J., Alkama, N., Andriamananjara, A., Amenc, L., Bargaz, A., et al. (2015). Phosphorus use efficiency for N2 fixation in the rhizobial symbiosis with legumes. Biological Nitrogen Fixation. Hoboken: Wiley. 455–464. doi: 10.1002/9781119053095.ch46
Fiorini, A., Boselli, R., Maris, S. C., Santelli, S., Ardenti, F., Capra, F., et al. (2020). May conservation tillage enhance soil C and N accumulation without decreasing yield in intensive irrigated croplands? Results from an eight-year maize monoculture. Agri. Ecosys. Environ. 296, 106926. doi: 10.1016/j.agee.2020.106926
Gambart, C., Swennen, R., Blomme, G., Groot, J. C., Remans, R., and Ocimati, W. (2020). Impact and opportunities of agroecological intensification strategies on farm performance: A case study of banana-based systems in central and south-western Uganda. Front. Sustain. Food Syst. 4, 87. doi: 10.3389/fsufs.2020.00087
Gliessman, S. R. (2015). Agroecology: The Ecology of Sustainable Food Systems, Third edit. ed. Oxfordshire, United Kingdom: Taylor & Francis. doi: 10.1201/b17881
GLOBE (2014). Soil Particle Density Protocol. p. 10. Available online at: https://www.globe.gov/documents/352961/08b00ef4-5344-4c7c-85ff-b840b291e309 (accessed 24 December, 2022).
Heri-Kazi, B. A., and Bielders, L. C. (2020). Dégradation des terres cultivées au Sud-Kivu, R.D. Congo : perceptions paysannes et caractéristiques des exploitations agricoles. Biotechnol. Agron. Soc. Environ. 24, 99–116. doi: 10.25518/1780-4507.18544
Hermans, K., and McLeman, R. (2021). Climate change, drought, land degradation and migration: exploring the linkages. Curr. Opin.Environ. Sustain. 50, 236–244. doi: 10.1016/j.cosust.2021.04.013
IUCN (2015). Land Degradation and Climate Change: The Multiple Benefits of Sustainable Land Management in the Drylands. IUCN Issues Brief. Available online at: https://www.iucn.org/sites/default/files/2022-07/land_degradation_issues_brief_cop21_031215.pdf (accessed 18 December, 2022).
Jalal, D. J. W., Stevens, B. W., Iversen, M. U., Sainju, M. B., and Allen, L. (2021). Soil cone index and bulk density of a sandy loam under no-till and conventional tillage in a corn-soybean rotation. Soil Till. Res. 206, 104842. doi: 10.1016/j.still.2020.104842
Jiang, J., Wang, Y., Yu, M., Cao, N., and Yan, J. (2018). Soil organic matter is important for acid buffering and reducing aluminum leaching from acidic forest soils. Chem. Geol. 501, 86–94. doi: 10.1016/j.chemgeo.2018.10.009
Kasper, S., Christoffersen, B., Soti, P., and Racelis, A. (2019). Abiotic and biotic limitations to nodulation by leguminous cover crops in South Texas. Agric. 9, 209. doi: 10.3390/agriculture9100209
Kiaire, M., Sibiri, J. O., Sarr, B., and Belem, M. (2013). “Guide de Mesure et de Suivi du Carbone dans le système sol-végétation des formations forestières et agroforestières en Afrique de l'ouest,” in Alliance Mondiale contre le Changement Climatique (Afrique de l'ouest: AMCC/GCCA). p. 46. Available online at: http://www.laboress-afrique.org/ressources/assets/docP/Document_N0804.pdf
Kim, N., Riggins, C. W., Rodríguez-Zas, S., Zabaloy, M. C., and Villamil, M. B. (2021). Long-term residue removal under tillage decreases amoA-nitrifiers and stimulates nirS-denitrifier groups in the soil. Appl. Soil Ecol. 157, 103730. doi: 10.1016/j.apsoil.2020.103730
Lal, R. (2010). Crop Residue and Soil C. Carbon Management and Sequestration Center. Ohio, USA: The Ohio State University. p. 14.
Luíz, C. B., Brossard, M., Leprun, J. C., and Bruand, A. (2002). Mise en valeur des Ferralsols de la région du Cerrado au Brésil et évolution de leurs propriétés physiques: une étude bibliographique. Etude et Gestion des Sols, Association Française pour l'Etude des Sols 9, 83–104.
Maris, S. C., Fiorini, A., Boselli, R., Santelli, S., and Tabaglio, V. (2021). Cover crops, compost, and conversion to grassland to increase soil C and N stock in intensive agrosystems. Nutr. Cycling Agroecosyst. 119, 83–101. doi: 10.1007/s10705-020-10110-9
Mayer, S., Kühnel, A., Burmeister, J., Kögel-Knabner, I., and Wiesmeier, M. (2019). Controlling factors of organic carbon stocks in agricultural topsoils and subsoils of Bavaria. Soil Till. Res. 193, 22–32. doi: 10.1016/j.still.2019.04.021
Mondal, S., Chakraborty, D., Das, T. K., Shrivastava, M., Mishra, A. K., Bandyopadhyay, K. K., et al. (2019). Conservation agriculture had a strong impact on the sub-surface soil strength and root growth in wheat after a 7-year transition period. Soil Till. Res. 195, 104385. doi: 10.1016/j.still.2019.104385
Mubvumba, P., DeLaune, P. B., and Hons, F. M. (2021). Soil water dynamics under a warm-season cover crop mixture in continuous wheat. Soil Tillage Res. 206, 104823. doi: 10.1016/j.still.2020.104823
Ntamwira, B. J., Mirindi, C. T., Pyame, M. L. D., Dhed'a, D. B., Mariam, B. E., Moango, M. A., et al. (2017). Évaluation agronomique des variétés de haricot volubile riches en micronutriments dans un système intégé d'Agroforesterie sur deux sols contrastés à l'Est de la RD Congo. J. Appl. Biosci. 114, 11368–11387. doi: 10.4314/jab.v114i1.10
Ntamwira, B. J., Pyame, M. L. D., Kanyenga, L. A., Bembeleza, Z. E., Katunga, M. D., and Dhed'a, D. B. (2019). Aboveground biomass production of different species of shrub and gramineae on two contrasting soil in Eastern Democratic Republic of Congo. Int. J. Innov. Appl. Studies 27, 445–455.
Ntamwira, B. J., Pyame, M. L. D., Kanyenga, L. A., and Dhed'a, D. B. (2018). The effects of different combinations of herbaceous and shrubs and microdose of fertilizer on bean and maize yields, soil proprieties and carbon sequestration on two degraded soils in the highland of South Kivu, Eastern of DR Congo. Int. J. Innov. Appl. Stud. 23, 275–284.
Ntamwira, J., Ocimati, W., Kearsley, E., Safari, N., Bahati, L., Amini, D., et al. (2021). The integration of shade-sensitive annual crops in Musa spp. plantations in South Kivu, Democratic Republic of Congo. Agron. 11, 368. doi: 10.3390/agronomy11020368
Nyambo, P., Chiduza, C., and et Araya, T. (2020). Carbon input and maize productivity as influenced by tillage, crop rotation, residue management and biochar in a semiarid region in South Africa. Agron. 10, 1–16. doi: 10.3390/agronomy10050705
Nyombi, K., Van Asten, P. J., Corbeels, M., Taulya, G., Leffelaar, P. A., and Giller, K. E. (2010). Mineral fertilizer response and nutrient use effciencies of East African highland banana (Musa spp., AAA-EAHB, cv. Kisansa). Field Crops Res. 117, 38–50. doi: 10.1016/j.fcr.2010.01.011
Olsson, L., Barbosa, H., Bhadwal, S., Cowie, A., Delusca, K., Flores-Renteria, D., et al. (2019). “Land degradation: IPCC special report on climate change, desertification, land 5 degradation, sustainable land management, food security, and 6 greenhouse gas fluxes in terrestrial ecosystems,” in IPCC Special Report on Climate Change, Desertification, Land 5 Degradation, Sustainable Land Management, Food Security, and 6 Greenhouse Gas Fluxes in Terrestrial Ecosystems. Geneva: Intergovernmental Panel on Climate Change (IPCC). p. 1.
Ouedraogo, J., Senou, I., Youl, S., Ouedraogo, E., Sedogo, M. P., and et Nacro, H. B. (2017). Effet de la macrofaune et des modes de gestionde la fertilité sur le carbone d'un lixisol au Burkina faso. Agron. Afri. 29, 257–267.
Page, K. L., Dang, Y. P., Dalal, R. C., Reeves, S., Thomas, G., Wang, W., et al. (2019). Changes in soil water storage with no-tillage and crop residue retention on a Vertisol: impact on productivity and profitability over a 50 year period. Soil Till. Res. 194,104319. doi: 10.1016/j.still.2019.104319
Pauwels, J., Van Ranst, E., Verloo, M., Mvondo, Z. A., Pauwels, J., Van Ranst, E., et al. (1992). Manuel De Laboratoire De Pédologie - Méthodes D'analyses De Sols Et De Plantes; Equipment Et Gestion Des Stocks De Verrerie Et De Produits Chimiques. Bruxelles, Belgium: Publications Agricoles Nr. 28, A.G.C.D. p. 180.
Pheap, S., Lefèvre, C., Thoumazeau, A., Leng, V., Boulakia, S., Koy, R., et al. (2019). Multi-functional assessment of soil health under conservation agriculture in Cambodia Soil Till. Res. 194, 104349. doi: 10.1016/j.still.2019.104349
Pyame, M. L. D., Geert, B., and Mate, M. J. (2016). “Culture en assiettes sous tapis vert: réhabilitater sol, forêt et climat,” in Editions Universitaires europpéennees (Beau Bassin: Editions Universitaires Europpéennees). p. 140.
R Core Team (2020). “R: A language and environment for statistical computing,” R Foundation for Statistical Computing. Available online at: https://www.R-project.org/ (accessed 12 July, 2022).
Rahmati, M., Eskandari, I., Kouselou, M., Feiziasl, V., Mahdavinia, G. R., Aliasgharzad, N., et al. (2020). Changes in soil organic carbon fractions and residence time five years after implementing conventional and conservation tillage practices. Soil Till. Res. 202, 104632. doi: 10.1016/j.still.2020.104632
Roose, E. (2018). Restauration de la productivité de sols tropicaux et méditerranéens, contribution à l'agroécologie. Marseille: IRD Éditions. p. 712. doi: 10.4000/books.irdeditions.24108
Ruiz, N., and Lavelle, P. (2008). Soil Macrofauna Field Manual: Technical Level. Rome: Food and Agriculture Organization of the United Nations. p. 101.
Sanaullah, M., Usman, M., Wakeel, A., Cheema, S. A., Ashraf, I., and Farooq, M. (2020). Terrestrial ecosystem functioning affected by agricultural management systems: a review. Soil Till. Res. 196, 104446. doi: 10.1016/j.still.2019.104464
Semida, W. M., Abd El-Mageed, T. A., and Howladar, S. M. (2014). A novel organo-mineral fertilizer can alleviate negative effects of salinity stress for eggplant production on reclaimed saline calcareous soil. Acta Hortic. 1034, 493–499. doi: 10.17660/ActaHortic.2014.1034.61
Soleymani, A., Hesam, S. M., and et Khoshkharam, M. (2016). The impact of barley residue management and tillage on forage maize. Rom. Agric. Res. 33, 161–167.
Taulya, G. (2013). East African highland bananas (Musa spp. AAA-EA) ‘worry' more about potassium deficiency than drought stress. Field Crops Res. 151, 45–55. doi: 10.1016/j.fcr.2013.07.010
Teutscherová, N., Vázquez, E., Sotelo, M., Villegas, D., Velásquez, N., Baquero, D., et al. (2021). Intensive short-duration rotational grazing is associated with improved soil quality within one year after establishment in Colombia. Appl. Soil Ecol. 159, 103835. doi: 10.1016/j.apsoil.2020.103835
Thung, M. (1990). “Phosphorus: A limiting nutrient in bean (Phaseolus vulgaris L.) production in Latin America and field screening for efficiency and response,” in Genetic Aspects of Plant Mineral Nutrition, eds N. El Bassam, M. Dambroth, and B. C. Loughman (Dordrecht: Springer), 501–521.
Toufiq, M. I. (2012). Effect of Al compounds on soil pH and bioavailability of Al in two acid soils. Turk. J. Agric. For. 36, 720−728. doi: 10.3906/tar-1109-26
Tsufac, A. R., Awazi, N. P., and Yerima, B. P. K. (2021). Characterization of agroforestry systems and their effectiveness in soil fertility enhancement in the Southwest region of Cameroon. Curr. Res. Environ. Sustain. 3, 100024. doi: 10.1016/j.crsust.2020.100024
Van Schoonhoven, A., and Pastor-Corrales, M. A. (1992). Système standard pour l'évaluation du germoplasme du haricot, CIAT (Centro International de Agricultura Tropical). Publication du CIAT N°207. 49p.
Wang, H., Xu, J., Liu, X., Zhang, D., Li, L., Li, W., et al. (2019). Effects of long-term application of organic fertilizer on improving organic matter content and retarding acidity in red soil from China. Soil Till. Res. 195, 104382. doi: 10.1016/j.still.2019.104382
Wezel, A., Casagrande, M., Celette, F., Vian, J. F., Ferrer, A., and Peign,é, J. (2014). Agroecological practices for sustainable agriculture. A review. Agron. Sustain. Dev. 34, 1–20. doi: 10.1007/s13593-013-0180-7
WHO (2022). Climate change: Land degradation and desertification. Available online at: https://www.who.int/news-room/questions-and-answers/item/climate-change-land-degradation-and-desertification (accessed on 18 December, 2022).
Wouteres, J. (1991). Gestion de la matière organique dans les sols tropicaux. Tropicultura, Notes Techniques. 9, 81–85.
Xiao, L., Zhao, R., and Kuhn, N. J. (2019). Straw mulching is more important than no tillage in yield improvement on the Chinese Loess Plateau. Soil Till. Res. 194, 104314. doi: 10.1016/j.still.2019.104314
Xie, E., Zhang, Y., Huang, B., Zhao, Y., Shi, X., Hu, W., et al. (2021). Spatiotemporal variations in soil organic carbon and their drivers in southeastern China during 1981–2011; Soil Till. Res. 205, 104763. doi: 10.1016/j.still.2020.104763
Keywords: agroecology, grasses, improved fallow, mucuna, organic carbon, shrubs, soil degradation
Citation: Ntamwira J, Ocimati W, Blomme G, Lubobo AK, Mwarabu Lolonga Pyame D and Dhed'a Djailo B (2023) Innovative agroecological practices can restore degraded farmlands and revive crop yields. Front. Sustain. Food Syst. 7:1017341. doi: 10.3389/fsufs.2023.1017341
Received: 11 August 2022; Accepted: 16 February 2023;
Published: 10 March 2023.
Edited by:
Luuk Fleskens, Wageningen University and Research, NetherlandsReviewed by:
Robert B. Zougmoré, Agriculture and Food Security (CCAFS), MaliRoger R. B. Leakey, International Tree Foundation, United Kingdom
Copyright © 2023 Ntamwira, Ocimati, Blomme, Lubobo, Mwarabu Lolonga Pyame and Dhed'a Djailo. This is an open-access article distributed under the terms of the Creative Commons Attribution License (CC BY). The use, distribution or reproduction in other forums is permitted, provided the original author(s) and the copyright owner(s) are credited and that the original publication in this journal is cited, in accordance with accepted academic practice. No use, distribution or reproduction is permitted which does not comply with these terms.
*Correspondence: Walter Ocimati, dy5vY2ltYXRpQGNnaWFyLm9yZw==