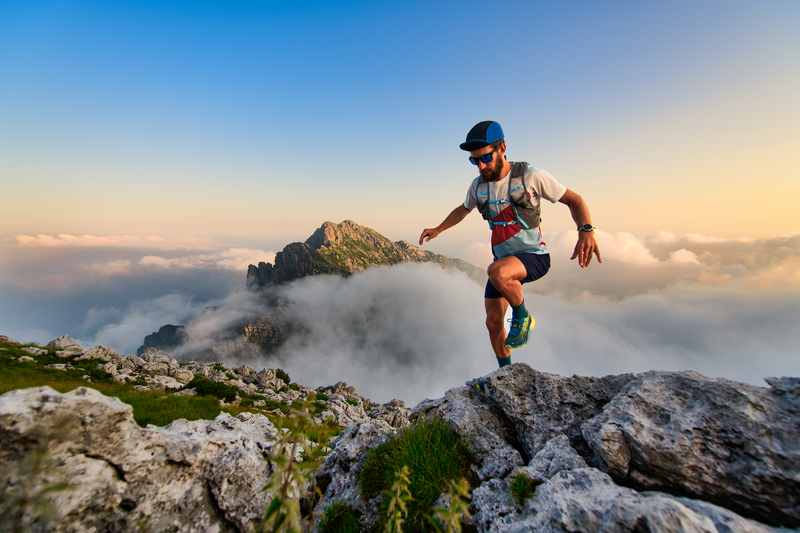
95% of researchers rate our articles as excellent or good
Learn more about the work of our research integrity team to safeguard the quality of each article we publish.
Find out more
REVIEW article
Front. Sustain. Food Syst. , 25 August 2022
Sec. Sustainable Food Processing
Volume 6 - 2022 | https://doi.org/10.3389/fsufs.2022.969503
Tenderness could measure the eating quality of meat. The mechanism of muscle tenderization is becoming more and more critical in the past decade. Since the transforming of muscle into edible meat requires a complex physiological and biochemical process, the related tenderization of meat can be beneficial to improving the meat quality. As a non-thermal processing technology with energy-saving, environmental protection, and intense penetration, ultrasonic treatment has been widely used in the tenderizing process of meat products. In this paper, the principle of meat tenderization, the ultrasonic technology, and the application of ultrasonic technology in meat tenderization is summarized. The effect of ultrasonic technology on the tenderization of meat products is discussed from different perspectives (muscle fibers and connective tissue properties).
Demand for high-quality meat, especially red meat, is expected to increase (Mullen et al., 2017), and food quality is paramount when consumers choose meat. For meat consumers, quality characteristics refer to appearance (color and marbling) and palatability (tenderness, juiciness, flavor) (Aaslyng and Meinert, 2017; Kim et al., 2018). Palatability characteristics are among the essential factors affecting consumers' meat purchasing decisions. Tenderness is one of the most crucial indexes affecting the edible quality of meat products (Liu et al., 2016). It is also the most variable of all meat characteristics (Ma and Kim, 2020) and is influenced by many biological and environmental factors (Bhat et al., 2018a). Therefore, tenderness is an essential factor affecting market prices. The meat industry has developed many techniques to improve the texture of high-quality cuts and the tenderness of low-quality muscles.
The meat tenderness can be affected by many factors before/after slaughter. The “toughness” is determined by the characteristics of the muscle, especially the amount and type of its connective tissue (collagen) (Veiseth et al., 2004). The muscle characteristics depend on factors such as animal species, age, nutritional status, sex, and muscle type (Bao et al., 2021). Two critical factors determining final tenderness after slaughter are the sarcomere length when the muscle enters a state of stiffness (Rhee et al., 2004) and the time and extent of aging (Koohmaraie and Geesink, 2006). Latorre et al. (2019) have studied that the meat tenderness is extremely unstable due to the muscle discontinuous change after slaughter, which reduces the eating quality of the meat. In addition, proteomic technology has found candidate proteins associated with changes in meat tenderness, among which the degradation of myofibrillar proteins is regarded as the critical factor (Purslow, 2018).
Research on post-mortem interventions for meat tenderization needs to be pushed forward. Emerging technology seems to be the best option to solve the above problems. Ultrasound provides an alternative to traditional food processing methods and is considered a promising new environmentally friendly technology (Jadhav et al., 2021). Ultrasound is a sound wave with a frequency above 20 kHz (Caraveo-Suarez et al., 2022). It is a new way to control, improve, and speed up processing without compromising food quality (Li et al., 2019). In recent years, some studies have indicated the effect of high-intensity ultrasonic (HIU) on fresh meat processing, including the mass transfer or marinating (Gómez-Salazar et al., 2018; Xiong et al., 2020), meat tenderizing (Peña-Gonzalez et al., 2019; Shi et al., 2020), freezing, and thawing (Sun et al., 2019; Astráin-Redín et al., 2021a), etc. Chemat and Ashokkumar (2017) emphasized that the primary purpose of ultrasound research is to study and analyze the desirable and undesirable degradation caused by the ultrasound of food products. Therefore, it is crucial to understand the ultrasound properties and the effects of ultrasound on muscle. Because it will add a significant benefit to its future applications and is particularly important for research in the meat industry. This review explores the principles of ultrasound treatment and the effects of ultrasound on meat tenderness. In addition, this paper proposes the tenderization mechanisms of ultrasound.
Meat tenderization is a complex process with several related sub-mechanisms. Post-slaughter degradation of the myofibrillar proteins directly affects meat tenderness (Madhusankha and Thilakarathna, 2021). Degradation of collagen, reduction in the diameter of muscle fibers bundles, changes in the sarcomere length during rigor mortis, and the chemical and structural changes that occur during aging are different mechanisms aiming at tenderization. The extreme shortening of sarcomere and hydrolysis of myofibril-related proteins during rigidity make an enzymatic intervention necessary. In this section, we review some studies about proteases and their involvement in the tenderization process.
Some researchers believe in the primary role of the calpain system (Koohmaraie and Geesink, 2006). This section reviews the mechanism of action of calprotease on meat tenderness. The calpain system is the mechanism that has been widely studied to affect meat tenderness (Koohmaraie and Geesink, 2006). Apart from the sarcoplasmic calcium ions concentration and calpastatin to calpain ratio, several environmental factors, such as the temperature, pH, and oxidation, have been indicated to affect the activity of calpains (Piatkov et al., 2014). The calpain system includes the isoforms of several proteolytic enzymes, such as calpain and calpastatin (Bhat et al., 2018b). The μ-calpain and m-calpain need different calcium ions concentrations for their activation. While m-calpain needs 400 to 800 μM calcium ions for half of the total activity, μ-calpain requires just 3 to 50 μM calcium ions for half of the maximum activity (Goll et al., 2003). Since the activation of m-calpain is limited by the extremely high concentration of calcium ions, μ-calpain is widely used in meat tenderization (Camou et al., 2007).
The inhibition of the activity of μ-calpain and m-calpain by calpastatin is also a calcium-dependent event as calpain-calpastatin binding requires calcium (Bhat et al., 2018b). Calpastatin inhibits calpain by avoiding calpain proteolytic activation, membrane binding, and the expression of catalytic activity (Lonergan et al., 2010). These results were later confirmed (Geesink et al., 2006). The high levels of calpastatin found in the hypertrophic muscles of lambs are associated with postmortem protein hydrolysis and the tenderness of meat quality (Cruzen et al., 2014).
Calpain can degrade essential proteins in myofibrils, but Chang et al. (2020) indicated that calpain had different activation speeds due to the other fiber-type compositions. Calpain degrades the filamentous structure connecting adjacent myofibrils at the level of each Z-disc and the binding force between I-band and Z-line (Taylor et al., 1995), indicating that calpain destroys myofibrils. The ordered structure of muscle fibers and the integrity between muscle fibers and surrounding muscle fibers are destroyed by calprotease hydrolysis (Uytterhaegen and Demeyer, 1994). The combination of calpain and myofibril has the activity of degrading desmin. Desmin is an intermediate filament connecting myofibrils in muscle cells, mainly located in the Z-band. It can destroy the Z-line and achieve the effect of tenderization (Lyu and Ertbjerg, 2021). The degradation of tropomyosin binding subunit Tn-T and the weaken the filament structure can improve tenderness (Whipple and Koohmaraie, 1992).
Cathepsins generally designate peptidases in the lysosomes and are primarily active at acidic pH (Sentandreu et al., 2002). The role of cathepsins in post-mortem tenderization is controversial, primarily because they are found in the lysosomes, limiting substrate accessibility (Kaur et al., 2021). Due to the decline in pH and temperature throughout the post-mortem storage, the membranes of the lysosomes rupture and cause the release of cathepsins into the cytosol (Sentandreu et al., 2002). Cathepsin B, D, H, and L are the most abundant in muscle fibers, and they have been claimed to be involved in the degradation of proteins during post-mortem aging. Some evidence on fast glycolytic correlated the low pH with an enhanced release of cathepsins B and L from the lysosomes. The myofibrillar electrophoretic degradation pattern showed enhanced fiber proteolysis on fast glycolytic compared to slow glycolytic muscles (O'Halloran et al., 1997). The results from a study based on the comparison between post-mortem fish and meat suggest that the leading protagonists of sea bass white muscle degradation are cathepsins B and L, with the calpains having a secondary role, while in meat, the two classes act synergistically. Moreover, the authors suggest that after a few hours' meat pH reaches the optimal value for the activity of cathepsin D (Chéret et al., 2007). However, a study by Hopkins and Thompson (2001) reported that the inhibition of cathepsins B and L was not found to affect meat tenderness. Cathepsin D has remained active only within a narrow pH and temperature range (Zeece et al., 1986), suggesting that this enzyme might not play a significant role in the post-mortem tenderization process.
Sentandreu et al. (2002) first proposed the hypothesis that the caspases system is involved in proteolysis and muscle tenderization during post-mortem maturation. Caspases-mediated apoptosis is a new mechanism that affects meat tenderness. Previous studies have shown that proteins related to meat tenderization are degraded under the action of the calcium-activating enzymes. However, some proteins related to tenderness were still degraded under the condition that calcium activating enzyme could not function, which may be related to Caspases. Zhang et al. (2020) found that lysosomal dysfunction mediated by iron increased mitochondrial membrane permeability and decreased mitochondrial membrane potential, thereby enhancing caspase-9/-3 activation, and indicated that lysosomal iron contributes to post-mortem meat tenderization through the lysosomal-mitochondrial dysfunction-induced apoptosis pathway. Wang et al. (2017) used cyclosporin A to suppress the activities of caspase-9 and caspase-3. And they found that the apoptosis rate, myofibril fragmentation index, and Ca2+ level of the yak meat during post-mortem decreased, while muscle shear force increased at the same time. Another study demonstrated that some oxidized myofibrillar proteins are more receptive to cleavage using both calpains and caspase 3, which showed a synergic action (Chen et al., 2015). In addition, Xiong et al. (2012) analyzed the potential contribution of mechanical disruption by ultrasonics and endogenous proteolytic enzymes on the tenderisation of hen muscle. These authors demonstrated that degradation of muscle proteins should not be exclusively attributed to the calpain system, and the effector caspase-3 may be involved in post-mortem muscle protein proteolysis.
Currently, a multi-mechanism has been widely proposed to work together for meat maturity (Figure 1). The myofibril-bound calpain maintains the proteolytic activity and degraded desmin when re-activated with calcium ions (Lyu and Ertbjerg, 2021). Apoptosis enzymes are mainly activated in the early post-mortem and can participate in the limited degradation of some myofibril proteins in postmortem tenderization (Huang et al., 2011). S-nitrosylation of the cysteine residues of L-calpain can reduce the activity of L-calpain, affecting the auto solubility and catalytic ability (Liu et al., 2016). At the same time, some studies have found the mechanism of interaction and mutual influence between the caspases and calpain systems. When the endoplasmic reticulum calcium ions balance is disrupted, calpain can activate caspase-12, which activates pro-apoptotic proteins, inducing mitochondrial volume increase and content copy sharing (Smuder et al., 2010). Protein nitrosylation can also modify the calcium release channel and affect its release rate, causing muscle contraction to varying degrees. Enzymes involved in glycolysis (such as phosphofructokinase) are modified by nitrosylation, affecting the rate of post-mortem pH decline and the end-point pH, which in turn affects the maturation quality of meat (Liu et al., 2016). Further studies found that anti-apoptotic activity was positively associated with increased collagen toughness, suggesting that (anti/-) apoptosis could be involved in the post-mortem proteolysis system and meat tenderness (Cramer et al., 2018). In addition, protein phosphorylation has an impact on muscle maturation.
Ultrasound is an emerging technology with great application potential in the food field. It is a sound wave whose frequency exceeds the human audible limit (Lin et al., 2021). The speed of ultrasonic pulse depends on the acoustic properties of the medium (Kasaai, 2013). The mechanical energy is then transferred to the medium. Part of the energy is lost by conversion to heat, and another part produces cavitation. Ultrasound can be classified as a non-thermal process, but mechanical friction generates heat due to mechanical vibrations during propagation, increasing the temperature by 1 to 10°C (Zhang and Abatzoglou, 2020). The ultrasound apparatus consists of three main parts: a generator, a transducer or converter, and a probe or horn (Gómez-Salazar et al., 2021). Ultrasound is a type of vibrational energy produced by a transducer that converts electrical energy into sound energy, resulting in a phenomenon called cavitation (Linares and Rojas, 2022). Ultrasound is divided into HIU (low frequency) (> 10 W) and low intensity (high frequency) (LIU) (< 1 W) according to the intensity of ultrasound (Li et al., 2019). HIU is mainly used for food processing. Because it physically, chemically, and mechanically alters food to improve heat and mass transfer. HIU is applied to meat products to measure the composition of the meat product and improves mass transfer and diffusion throughout the development. It also imparts some desirable technical properties to meat products, such as texture (tenderness) (Domínguez et al., 2019).
Ultrasound and acoustic intensity can induce acoustic cavitation and shock wave effects by adjusting the frequency (Ma et al., 2021a). The acoustic waves with a constant ultrasound frequency produce acoustic cavitation. When a liquid is exposed to ultrasonic waves, liquid rupture occurs in the form of tiny vapor-filled bubbles. The formation of bubbles and subsequent collapse is called cavitation (Czechowska-Biskup et al., 2005). Among the non-thermal mechanisms, cavitation is generated by good energy ultrasound, starting with nucleation, followed by the growth and collapse of microscopic bubbles (Umego et al., 2021). When the ultrasonic intensity exceeds 10 W/cm2, transient acoustic cavitation occurs. They have one or more acoustic cycles and produce pressure changes and molecular bond breakage within a short period, resulting in a rapid increase in temperature (Lorimer and Mason, 1987). Steady acoustic cavitation is the process by which tiny air bubbles form in a region of the sound field centered on a liquid (via impurities). The transient acoustic cavitation is due to the inability of the fluid to withstand higher pressures during wave expansion. When this happens, a large amount of acoustic energy is released in minimal amounts (Flint and Suslick, 1991). The collapse of the cavitation bubble releases energy that generates localized high temperature and pressure differentials. Thus, the boundary layer is disturbed, and the resistance is significantly reduced. At the same time, the collapse locally emits concentrated acoustic energy, forming a sizeable local gradient, which is very conducive to the formation of micro-streams (Alarcon-Rojo et al., 2019).
It is widely believed that the “sponge effect” is mainly responsible for the internal mass transfer enhancement by ultrasound (Astráin-Redín et al., 2021b). First, the enhancement resulting from ultrasound technology is a direct effect (inertial flow and “sponge effect”). Then, microchannels are formed from water activity to enhance the mass transfer. This explains why the enhancement of the hydration process after a certain treatment time is higher than in the early stage. Few studies on ultrasound-assisted grain hydration have confirmed this (Ghafoor et al., 2014; Patero and Augusto, 2015), and the hydration curves have become increasingly diverse over time. When ultrasound propagates through a solid medium, the sound waves cause a series of rapid and continuous compression and rarefaction, resulting in a series of rapid alternating contractions and expansions of the material, like repeated extrusion sponging. The “sponge effect” helps to keep the microchannels for moisture movement unobstructed and promotes moisture migration in solid (Yao et al., 2009).
Ultrasound-assisted tenderization originally refers to the explosion of small explosives in water to generate oscillating waves (Wang et al., 2018a). The meat is forced to vibrate in this environment. The interaction forces between meat proteins are changed by oscillating waves, improving the tenderness of the meat Jayasooriya et al. (2007). Tenderness is now often enhanced by using the “acoustic coupling” of low-frequency ultrasound (20 to 100 kHz) to force the meat to vibrate. The acoustic waves are affected by pressure and displacement, showing compressions (high-pressure area) and rarefactions (low-pressure area) (Wen et al., 2019). Ultrasound produces mechanical effects, heat effects, and cavitation effects. The cavitation effect plays a primary role in the tenderization process of meat products (Figure 2).
Figure 2. Diagrammatic sketch of the impact of ultrasound on meat (Alarcon-Rojo et al., 2019).
Tenderness is the primary quality attribute of meat (Ardeshiri et al., 2019). However, meat tenderness is considered a multifactorial sensory attribute (Gagaoua et al., 2019). Because it is the result of a complex, interrelated pathway, also related to factors such as the amount of fatty and connective tissue, the length of the sarcomere, and the ratio of proteolytic systems/endogenous inhibitors (Gagaoua et al., 2021). Studies have shown that the tenderization reduces the contraction of muscle fibers and affects myofibril integrity or the division of connective tissue, resulting in the amelioration of muscle tissue tenderness (Tantamacharik et al., 2018). Therefore, understanding the muscle structure can better study the effect of ultrasound technology on meat tenderization.
The whole muscle is surrounded by the epimysium, a layer of connective tissue. The sarcolemma is covered by another connective tissue called the endomysium. The fibers are collected into fiber bundles, in which the third connective tissue (perimysium) wraps the fiber bundle. Within the fiber cell, the smallest contractile elements (sarcomeres) are assembled into a long thread of myofibril with a diameter of 1 pm (Tornberg, 1996). Each myofibril is composed of functionally repeating segments. The smallest contractile unit in a muscle cell is the sarcomere, with the Z-line as the boundary at both ends. The degree to which actin and myosin overlap affects the degree of muscle contraction and, ultimately, the tenderness of the meat (Locker and Hagyard, 1963). Usually, the muscle is covered by a protective sheath of epimysium that helps protect the muscle from friction with other muscles and bones. This process continues at the end of the muscle, where it combines with other connective tissue to form a tendon that connects the muscle to the bone (Sikes and Tobin, 2021). Collagen is the major protein in the connective tissue around the endomysium. The content and solubility of collagen affect the toughness of meat (Bailey, 1972). Therefore, despite its complex structure, meat can be simply thought of as a system composed of two main protein components: muscle fibers and intramuscular connective tissue (IMCT). The myofibrillar structure is responsible for muscle contraction, and the connective tissue holds the muscle fibers together and connects the muscle to the skeletal framework.
An ultrasound is a high-frequency elastic wave propagating through a medium. Cavitation occurs when the wave energy reaches a threshold (Ma et al., 2021b). The propagation of acoustic waves between positive and negative pressure causes a series of non-linear processes, such as expansion, compression, collapse, and oscillation of tiny bubbles (Alarcon-Rojo et al., 2019). Cavitation zones created by ultrasound in liquid media can lead to extremely high temperatures and pressure (Bian et al., 2022), resulting in the generation of free radicals (Figure 3). These phenomena lead to changes in meat properties, microstructure, and molecular reactions, resulting in tender meat products. The mechanism of ultrasound treatment of meat tenderization is divided into three aspects. Violently change in the mechanical vibration of the medium particles is caused by the difference in the displacement and acceleration of the medium ions during ultrasound propagation (Zou et al., 2019). Various physical effects associated with cavitation can lead to structural changes in meat by disrupting weak intermolecular forces. HIU causes physical damage to meat tissue through cavitation, increases protein decomposition activity, and accelerates mass transfer. The results of these studies showed significant improvements in myofibrillar protein breakdown and improved meat tenderness. The collapse of cavitation bubbles produces strong hydrodynamic shear forces, turbulence, high temperature (5,000 K), and high pressure (100 MPa) (Wen et al., 2019). These physical effects can damage the cell membrane, and result in physicochemical damage to proteins. The ability of ultrasound to disrupt cell membranes has been investigated. Ultrasound can directly increase meat tenderness by weakening muscle tissue or proteolysis by releasing lysosomal cathepsins and, or intracellular Ca2+ (Chang et al., 2015). In addition, the “sponge effect” produced by ultrasound enhances mass transfer in assisting marinade tenderizing.
Ultrasound can be divided into two categories according to the difference in frequency and power: i) High-frequency and low intensity (> 1 MHz, > 1 W/cm2); ii) Low frequency and high-intensity (20~100 kHz, 10~1,000 W/cm2) (Alarcon-Rojo et al., 2019). Among them, the ultrasound of low-frequency and high-intensity is primarily used in meat tenderization. At present, it is generally believed that the frequency, power, and treatment time of ultrasound have a significant influence on the improvement of tenderness (Table 1).
Table 1. Findings of various studies elucidating the effect of ultrasound on the tenderness of different muscles.
Smith et al. (1991) believed that low-frequency and short-time ultrasound treatment significantly improved meat tenderness. Exposure of semitendinosus specimens to HIU for 2 and 4 min resulted in a significant decrease in shear force. The intense cavitation produced by low-frequency ultrasound could mechanically damage the muscle fibers, activate the calpains and cathepsins, and degrade the proteins.
Ultrasound power also affects the meat tenderness through the strength of the cavitation effect. Zou et al. (2018a) showed that the pressure loss and shear force decreased with the increasing ultrasound power (0, 400, 600, 800, and 1,000 W, frequency of 20 kHz). The 400 W treated sample had a better tenderization effect compared to the control group, due to the increased space between the myofibrils and better gelation was obtained, resulting in the tenderness of the spiced beef rising with the increase of ultrasound power. Meat tenderness is positively correlated with time within a reasonable sonication time. Therefore, the Ultrasound-assisted tenderization time can reasonably control in combination with frequency and power. However, too long a treatment time will reduce the protease activity and inhibits the protein decomposition, which can lead to a decrease in meat tenderness.
In addition, the study of beef myofibrillar proteins showed that physicochemical properties of myofibrillar proteins change with the increase of the ultrasonic strength and the extension of the treatment time (Kang et al., 2016a; Amiri et al., 2018). However, Zou et al. (2018b) believed that the solubility and surface hydrophobicity of chicken actomyosin could be significantly improved when the ultrasonic intensity was in the range of 1.15 to 2.36 W/cm2. But the high ultrasonic power of 11.43 W/cm2 could lead to protein aggregation. Therefore, in practical application, it is necessary to set the ultrasound treatment parameters according to the inherent characteristics of the protein. Otherwise, the ultrasound intensity is too large, or the treatment time is too long, which may cause excessive protein denaturation and aggregation.
Most authors accept that ultrasound can improve meat tenderness and reduce marinating time without affecting other quality parameters (Wang et al., 2018b). The effect of ultrasound on tenderness is mainly due to mechanical rupture of myofibrillar protein structures (Stadnik et al., 2008), disruption of collagen macromolecules, and migration of proteins and other compounds. Therefore, studying muscle fibers at different levels can provide a better understanding of the mechanisms by which new techniques improve meat tenderness (Kahraman et al., 2011).
The ultrasound cavitation effect produces bubbles. The microjet caused by the implosion of the bubbles causes physical damage to the object's surface (Bian et al., 2022), which can disrupt the muscle fibers and increase the gap among muscle fibers. At the same time, ultrasound can also produce a strong shearing effect, causing muscle fibers protein damage (Figure 4A), which further promotes the cleavage and dissolution of sarcoplasmic and myofibrillar proteins to a higher degree, thereby improving tenderness (Xiong et al., 2020). More profound studies showed that microstructural changes included z- and m-lines separation (Kang et al., 2016b; Yeung et al., 2017; Zou et al., 2018a), desmin and troponin-N degradation (Kang et al., 2017), changes in secondary structures of myofibrillar proteins (Amiri et al., 2018), and myosin denaturation (Xue et al., 2018). Wang et al. (2018b) used the ultrasound treatment (20 kHz) at an intensity of 25 W. cm−2 for 20 or 40 min to treat the beef semitendinosus (ST) muscle samples. The treated samples showed significantly low shear. Zou et al. (2018a) reported that ultrasound-treated meats disrupted the integrity of myosin and actin. In addition, the ultrasound effect also showed an increase in free actin and a decrease in actomyosin ATPase activity during storage.
Figure 4. Mechanism of the effects of ultrasound technology on myofibril (A), collagen fibers (B), myofibrillar protein (C), and collagen (D) of meat.
Ultrasound significantly affects collagen properties (Chang et al., 2012), especially thermal properties, but does not affect insoluble collagen content. The effect of ultrasound on collagen solubility during cooking was also found when meat was sonicated in a 40 kHz, 1,500 W bath (Chang et al., 2015). Although it has been reported that ultrasound does not affect shear force (Jørgensen et al., 2008; McDonnell et al., 2014), ultrasound appears to play an essential role in meat tenderness. The perimysium and endomysium are the essential structures of connective tissue. The micro-jet, micro-turbulence, and high temperature and pressure generated by ultrasound cavitation led to cracks in the endomysium and perimysium, disrupting the physical structure of collagen fibers and causing collagen fibers to be arranged disorderly and loosely (Figure 4B). However, the improvement of tenderness is achieved by reducing connective tissue content and solubility, especially collagen (Kim et al., 2013). Gonzalez-Gonzalez et al. (2020) collagen was significantly reduced in HIU-treated samples compared to untreated samples (Figure 4D). They found that HIU application was an excellent comprehensive treatment for improving the tenderization of the three muscles.
The effect of ultrasound on pH also indirectly affects meat tenderness. Ultrasound effect on pH has been analyzed in various studies and conditions. Peña-Gonzalez et al. (Peña-Gonzalez et al., 2019) pointed out that HIU of the meat after storage caused a higher final pH. Higher pH is beneficial for liquid retention (Huff-Lonergan and Lonergan, 2005). Several authors have reported an increase in the pH of pale, soft, exudative (PSE) poultry meat (Vanderhout et al., 2022) and uncured dry-fermented beef (Wójciak et al., 2019) after ultrasound treatment. Ultrasound cavitation may lead to the release of proteolytic enzymes and deaminase, increasing the availability of amino acids and basic amines (Huang et al., 2017) and reducing acidic protein groups (Amiri et al., 2018). Another mechanism of ultrasound-induced increase in meat pH could be the release of ions from the cellular structure into the cytoplasm, or changes in protein structure may lead to changes in the functional localization of ions (Alarcon-Rojo et al., 2019).
WHC is mainly determined by the water outside the myofibrillar lattice and the muscle cell (Bertram et al., 2002). The more water the muscle fibers hold, the more tender the meat. Ojha et al. (2017) found that the raised intake of curing solution caused by the ultrasonic treatment could lead to an increased electrostatic repulsion within myofibrils, thereby causing water mobility and osmotic dehydration. Cao et al. (2021) indicated an increase in WHC after a brief (20 min) sonication of chicken breast, and the opposite effect occurred when the ultrasound time exceeded. In addition, ultrasound can increase the strength of muscle fibers to bind water (Figure 4C). But some authors reported that the ultrasound at 40 kHz and 110 W application increased rabbit meat hardness and reduced WHC (Li et al., 2019). The cooking loss increased when beef semitendinosus was sonicated several times (10, 20, 30,40, 50, or 60 min) at 40 kHz and 1500 W (Chang et al., 2015). The reason is the increase in the myofibril gap caused by the prolonged ultrasound radiation time. Therefore, the WHC of the meat is improved by reducing the cooking loss of the meat (Li et al., 2018). The variability observed in the literature may cause by differences in ultrasound methods. The authors used different time points and intensities, which prevented direct comparisons.
The texture profile analysis parameters of cooked meat are highly correlated with consumer perceptions of tenderness (Shi et al., 2022). Vandenberghe-Descamps et al. (2018) found that improvements in meat tenderness and juiciness contributed to improving mouthfeel. Peña-Gonzalez et al. (2019) proved that the hardness of sonicated samples showed significantly deceased. Chang et al. (2012) reported high-power sonication had a significant effect on bovine m. semitendinosus textural properties. Ultrasound induces changes in macromolecules such as enzymes, which remain active in the post-mortem period and can cause changes in the physical properties of the meat. Therefore, the effects of acoustic cavitation on meat tissue can promote enzymatic reactions and compromise cellular integrity (Lian et al., 2013). It has been reported that the distance between myofibrils increases, the toughness changes, and the tenderness of spiced beef increases with increasing ultrasound power (Zou et al., 2018a). But prolonged or high-intensity sonication can cause undesired protein oxidation, leading to toughness in the meat (Alarcon-Rojo et al., 2015).
The lysosomes release the cathepsin and calpain owing to the disruption of ultrasound, which could increase the protein solubility (Xiong et al., 2020). Ouali et al. (2013) reported a biomarker of meat tenderness. They found that meat tenderness begins immediately after animal death with the onset of apoptosis followed by the cooperative action of endogenous proteolytic systems. And they reviewed significant predictors of meat tenderness, such as caspases, serpins, annexins, galectins, and peroxiredoxins, all associated with the apoptotic process. Finally, they highlighted the role of mitochondria, which plays a crucial role in the initiation and development of apoptosis. Ultrasound has been reported to increase enzymatic activity, mainly due to various effects, such as an enhancement of mass transfer by micro mixing, enhanced enzyme release by cell disruption, and stimulated biochemical reactions within cellular tissues to increase the production of specific enzymes (Yu et al., 2013). Additionally, the higher pH value in ultrasound-treated meat, to some extent, maintained the calpain activity for continuous tenderization (Shi et al., 2022). Therefore, applying ultrasound can change the properties of meat and induce apoptosis through cavitation, which is the most critical acoustic-mechanical effect that can damage mitochondria and cause tenderization.
Ultrasound has a positive effect on meat quality (Luo et al., 2021). However, it has been reported that the technology affects the sensory and physicochemical properties of the meat. HIU applied by probe or bath (2.39, 6.23, 11.32, and 20.96 W/cm2 or 40 kHz, 11 W/cm2, respectively) increases carbonyl formation and TBARS values in beef (Peña-González et al., 2017) and pork (de Lima Alves et al., 2018). Lipid oxidation has no direct negative effect on meat texture. But lipid oxidation can accelerate protein oxidation by producing highly aggressive free radicals (peroxides, hexanal, propanal, malondialdehyde, etc.) (Barroug et al., 2021). Changes caused by protein oxidation can affect physical and chemical properties, such as solubility, hydrophobicity, WHC, meat tenderness, and gel function (Zhang et al., 2013). For example, calpain oxidation may arrest its proteolytic activity (Rowe et al., 2004). When proteins in meat are oxidized, disulfide crosslinks are often created, and protein aggregates are formed. The physical forces created by these chemical structures make the meat tougher (Zhang et al., 2013).
Another degradation effect of ultrasound in related material meat is through the effect of biaxially oriented polypropylene film on oxygen permeability, solubility, and diffusion coefficient (Ščetar et al., 2019). Therefore, this effect should be considered when ultrasonication polypropylene-packed meat.
In recent years, the industry has focused on improving the food quality and tenderness of meat by external proteases such as papain, bromelain, and ficin (Kim and Joo, 2020). However, it is imperative to effectively control the activity of enzymes and increase the diffusion of enzymes in the meat to avoid adverse effects on meat quality, umami, color, and other qualities due to excessive activity (Bhat et al., 2018a). Although the use of exogenous proteases in meat tenderization has been extensively studied, developing new approaches to improve enzyme distribution has received little attention (Barekat and Soltanizadeh, 2018). The ultrasound-assisted enzymatic technique has been widely used recently in changing the structure and properties of food proteins and improving substrate solubility and hydrolysis sites to make enzymes more accessible (Barekat and Soltanizadeh, 2019; Dong et al., 2019).
The enzyme treatments reduce the muscle shear force and WHC. In the ultrasonic-assisted enzyme treatment, the ultrasonic wave can promote the migration and uniform distribution of water in the meat, which improves the water retention of the meat. At the same time, ultrasonic cavitation and physical shearing will destroy myofibrils and increase the degree of enzymatic tenderization (Cao et al., 2021). Ultrasound-assisted papain treatment increases beef tenderness by improving the proliferation of papain and disrupting muscle integrity (Barekat and Soltanizadeh, 2018). Pan et al. (2021) showed that the ultrasonic-assisted papain treatment with/without fermentation method had the best tenderization effect. In addition, Zhao et al. (2018) showed that ultrasound combined with bromelain treatment had a significant impact on the tenderization of duck meat. In the phenomenon of cavitation, highly active compounds such as radicals are formed inside the bubbles, and the collapse of the bubbles provides a condition for chemical reactions (Mason et al., 1996; Torres et al., 2008). Therefore, the radicals generated in the enzyme solution can react with the monomers (amino acids) of the enzyme, increasing the active site of the enzyme. But increasing the duration of ultrasound may cause many changes in the structure of the enzyme, especially in its active area, which reduces the enzyme's activity (Yu et al., 2014). Mehrabani et al. (2022) found that ultrasound decreased enzymatic activity when the time increased from 20 to 30 min. The study by Yu et al. (2014) found that the long-time application of ultrasonic waves for 30 or 60 min would change the spatial structure of the enzyme, thereby reducing its activity. In addition, Mehrabani et al. (2022) also indicated that the result of ultrasound-assisted enzymatic tenderization may be a synergistic effect between endogenous protease and exogenous protease.
In summary, one of the main challenges in using these enzymes is to counteract the effects of hyper tenderization (Jun-Hui et al., 2020). In addition, the effect of sonication time on enzyme activity should be considered to determine the optimal tenderization effect.
The chemical tenderization process focused on salt or non-phosphate additives to modify the meat's physicochemical properties (e.g., water-holding capacity, WHC) (Shen et al., 2016). Not only can the addition of sodium chloride improve the flavor and tenderness of the meat, but it improves the binding properties of meat by enhancing the solubility of myofibrillar proteins (González-González et al., 2017; Inguglia et al., 2018; Gómez-Salazar et al., 2021).
During chemical tenderization, the water flows from the meat to the brine, and the salt diffuses from the brine to the meat are two main types of mass transfer (Cárcel et al., 2007). The mechanism of tenderization caused by salting is shown in Figure 5. Salt can lead to uneven salt distribution and long curing times. It has been reported that ultrasound applications in meat curing can result in structural changes to muscle tissues and fibers and affect the color and texture of salted muscles by regulating salt diffusion (Wang et al., 2018b). At present, ultrasound is used to enhance mass transfer (Inguglia et al., 2018; Visy et al., 2021). An accelerated mass transfer of brined muscles assisted by ultrasonically irradiated liquids is mainly attributed to its “acoustic cavitation” effect, essentially the growth and collapse of vapor microbubbles in the dispersed medium (Pan et al., 2022). The ultrasonic treatment causes the air bubbles to form and bump into the tissue, leading to microinjections of saline into the sample, which helps explain the increase in sodium chloride content in ultrasonically treated meat (Ozuna et al., 2013). Finally, the application of sodium chloride, moisture, and ultrasound promotes the change in meat, which could be confirmed by observing the microstructure. Xiong et al. (2020) have reported that ultrasound could promote the separation of muscle membrane by altering protein structure, enhancing the marinade uptake and tenderness, and reducing cooking losses. Therefore, many studies in recent years have attempted to clarify that ultrasonic waves can cause cell membrane rupture and increase the tenderness of the meat.
Figure 5. Diagrammatic sketch of salt tenderization: Step 1 involves dissociation of actomyosin, and step 2 involves dissociation of myosin from the thick filament. Salt impacts both constraints whereas phosphate lowers the concentration of Sodium chloride for maximum myosin extraction from >1.0 to 0.4 mol/L, primarily by weakening actomyosin interaction.
Power ultrasound has been shown to have a positive impact on the mass transfer process. In general, the application parameters of ultrasound in processed meat should be closely related to curing conditions and muscle type(Gómez-Salazar et al., 2018). Ultrasonic treatment below 300 W for 120 min can effectively improve the tenderness and WHC of cured beef. But few studies focus on how different ultrasonic treatment frequencies affect the marinating time and meat quality, such as poultry texture and secondary lipid oxidation (Tan et al., 2018).
In recent years, the utilization of amino acids in meat and meat products has attracted more and more attention from meat researchers. Numerous references reported that amino acids could significantly improve the functional properties of the myofibrillar protein (MP) and improve WHC, texture, color, and other quality properties of meat products (Zhu et al., 2018). Among them, the successful application of basic amino acids has received increasing attention due to its superior performance in compensating for sensory defects of non-chlorinated salt-based meat products (da Silva et al., 2020; Vidal et al., 2020). Shi et al. (2022) indicated that ultrasound-assisted L-histidine marination meat exhibited the highest WHC. It is essential to ensure maximum tenderness and juiciness in edible products. The appropriate concentration of L-histidine solution also improves the microstructure and texture of protein gel from meat (Wang et al., 2020). Ultrasound disrupted cell membranes and tissues and facilitated the penetration of L-histidine solutions (Zou et al., 2020). Furthermore, ultrasound-assisted L-histidine treatment is an effective method to improve WHC by altering moisture distribution and mobility (Guo et al., 2021).
In addition to amino acids, polysaccharides have also shown promising effects in improving muscle texture. Polysaccharides are effective fat substitutes due to their ability to reduce water mobility. In some studies, the meat products that added polysaccharides (especially dietary fibers) showed lower cooking loss and higher strength of gel properties (Goudoulas and Germann, 2017; Zhuang et al., 2020). Sodium alginate (SA, a kind of polysaccharide) extracted from seaweed can enhance the gelling, viscosification, and stabilization properties of muscle protein (Zhou et al., 2014), and change the WHC of myosin gel and meat tissue (Yao et al., 2018). Potassium alginate (PA) has the potential to improve muscle WHC by modifying the physicochemical characteristics of proteins or tissue, thereby contributing to improving meat texture (Pietrasik and Janz, 2010). Alginate forms higher strength gel or insoluble polymer through crosslinks with calcium ions, creating a water barrier that reduces muscle shrinkage during heat-induced denaturation (Shi et al., 2020). Therefore, PA can potentially promote the tenderness and WHC of meat products by optimizing the gel properties (Vilar et al., 2020). But the polysaccharides are mostly used in minced meat, so the ultrasound-assisted potassium alginate treatment could generate a complete synergistic effect to promote meat quality (Shi et al., 2020).
Salt tenderization, exogenous enzymes, aging, and other technical interventions used in meat industry could effectively improve meat tenderness. However, the use of these methods has certain limitations and disadvantages. Emerging tenderization techniques, such as ultrasound systems, provide advantages over the applied methods. The optimal utilization of emerging tenderization technologies requires continuous adjustment for different carcass muscles, different markets (food service industry, fresh products, export products), and different consumer group preferences. Therefore, more research to optimize process parameters is needed for different muscles and cuts before some of these new methods can find commercial applications in the meat industry. The current combinations of traditional and emerging methods could be used to achieve the tender effects of “1+1>2.” For example, combining ultrasonic waves and excipients could change the weakness of traditional tenderization technology, such as instability and poor online application. But it is undeniable that further research and optimization of process parameters are needed to maximize the effect of the emerging coupled tenderization technology. Therefore, Future research in this area should focus on overcoming the challenges posed by the shortcomings of such new technologies.
YD and JM conceptualized the idea. YD prepared all figures using the TIFF format. YD, HZ, and JX wrote the review. CS and JX revised the manuscript. All authors contributed to the article and approved the submitted version.
This research was financially supported by China Agriculture Research System of MOF and MARA (CARS-47), and the Shanghai Science and Technology Commission Public Service Platform Construction Project (20DZ2292200 and 19DZ2284000).
The authors declare that the research was conducted in the absence of any commercial or financial relationships that could be construed as a potential conflict of interest.
All claims expressed in this article are solely those of the authors and do not necessarily represent those of their affiliated organizations, or those of the publisher, the editors and the reviewers. Any product that may be evaluated in this article, or claim that may be made by its manufacturer, is not guaranteed or endorsed by the publisher.
Aaslyng, M. D., and Meinert, L. (2017). Meat flavour in pork and beef–From animal to meal. Meat Sci. 132, 112–117. doi: 10.1016/j.meatsci.2017.04.012
Alarcon-Rojo, A. D., Carrillo-Lopez, L. M., Reyes-Villagrana, R., Huerta-Jiménez, M., and Garcia-Galicia, I. A. (2019). Ultrasound and meat quality: a review. Ultrason. Sonochem. 55, 369–382. doi: 10.1016/j.ultsonch.2018.09.016
Alarcon-Rojo, A. D., Janacua, H., Rodriguez, J. C., Paniwnyk, L., and Mason, T. J. (2015). Power ultrasound in meat processing. Meat Sci. 107, 86–93. doi: 10.1016/j.meatsci.2015.04.015
Amiri, A., Sharifian, P., and Soltanizadeh, N. (2018). Application of ultrasound treatment for improving the physicochemical, functional and rheological properties of myofibrillar proteins. Int. J. Biol. Macromol. 111, 139–147. doi: 10.1016/j.ijbiomac.2017.12.167
Ardeshiri, A., Sampson, S., and Swait, J. (2019). Seasonality effects on consumers' preferences over quality attributes of different beef products. Meat Sci. 157, 107868. doi: 10.1016/j.meatsci.2019.06.004
Astráin-Redín, L., Abad, J., Rieder, A., Kirkhus, B., Raso, J., Cebrián, G., et al. (2021a). Direct contact ultrasound assisted freezing of chicken breast samples. Ultrason. Sonochem. 70, 105319. doi: 10.1016/j.ultsonch.2020.105319
Astráin-Redín, L., Alejandre, M., Raso, J., Cebrián, G., and Álvarez, I. (2021b). Direct contact ultrasound in food processing: impact on food quality. Front. Nut. 8, 633070. doi: 10.3389/fnut.2021.633070
Bailey, A. J. (1972). The basis of meat texture. J. Sci. Food Agr. 23, 995–1007. doi: 10.1002/jsfa.2740230811
Bao, G., Liu, X., Wang, J., Hu, J., Shi, B., Li, S., et al. (2021). Effects of slaughter age on myosin heavy chain isoforms, muscle fibers, fatty acids, and meat quality in Longissimus thoracis muscle of tibetan sheep. Front. Vet. Sci. 8, 689589. doi: 10.3389/fvets.2021.689589
Barekat, S., and Soltanizadeh, N. (2017). Improvement of meat tenderness by simultaneous application of high-intensity ultrasonic radiation and papain treatment. Innov. Food. Sci. Emerg. Technol. 39, 223–229. doi: 10.1016/j.ifset.2016.12.009
Barekat, S., and Soltanizadeh, N. (2018). Effects of Ultrasound on Microstructure and Enzyme Penetration in Beef Longissimus Lumborum Muscle. Food Bioprocess. Technol. 11, 680–693. doi: 10.1007/s11947-017-2043-8
Barekat, S., and Soltanizadeh, N. (2019). Application of high-intensity ultrasonic radiation coupled with papain treatment to modify functional properties of beef Longissimus lumborum. J. Food Sci. Technol. 56, 224–232. doi: 10.1007/s13197-018-3479-1
Barroug, S., Chaple, S., and Bourke, P. (2021). Combination of natural compounds with novel-thermal technologies for poultry products: a review. Front. Nutr. 8, 6628723. doi: 10.3389/fnut.2021.628723
Bertram, H. C., Purslow, P. P., and Andersen, H. J. (2002). Relationship between meat structure, water mobility, and distribution: a low-field nuclear magnetic resonance study. Agric. Food Chem. 50, 824–829. doi: 10.1021/jf010738f
Bhat, Z. F., Morton, J. D., Mason, S. L., and Bekhit, A. E. D. A. (2018a). Applied and emerging methods for meat tenderization: a comparative perspective. Compr. Rev. Food Sci. Food Saf. 17, 841–859. doi: 10.1111/1541-4337.12356
Bhat, Z. F., Morton, J. D., Mason, S. L., and Bekhit, A. E-D. A. (2018b). Role of calpain system in meat tenderness: a review. Food Sci. Hum. Wellness. 7, 196–204. doi: 10.1016/j.fshw.2018.08.002
Bian, C., Cheng, H., Yu, H., Mei, J., and Xie, J. (2022). Effect of multi-frequency ultrasound assisted thawing on the quality of large yellow croaker (Larimichthys crocea). Ultrason. Sonochem. 82, 105907. doi: 10.1016/j.ultsonch.2021.105907
Camou, J. P., Marchello, J. A., Thompson, V. F., Mares, S. W., and Goll, D. E. (2007). Effect of postmortem storage on activity of μ-and m-calpain in five bovine muscles. J. Anim. Sci. 85, 2670–2681. doi: 10.2527/jas.2007-0164
Cao, C., Xiao, Z., Tong, H., Tao, X., Gu, D., Wu, Y., et al. (2021). Effect of ultrasound-assisted enzyme treatment on the quality of chicken breast meat. Food Biioprod. Process. 125, 193–203. doi: 10.1016/j.fbp.2020.11.005
Caraveo-Suarez, R. O., Garcia-Galicia, I. A., Santellano-Estrada, E., Carrillo-Lopez, L. M., Huerta-Jimenez, M., Alarcon-Rojo, A. D., et al. (2022). Integrated multivariate analysis as a tool to evaluate effects of ultrasound on beef quality. J. Food Process Eng. e14112. doi: 10.1111/jfpe.14112
Cárcel, J., Benedito, J., Bon, J., and Mulet, A. (2007). High intensity ultrasound effects on meat brining. Meat Sci. 76, 611–619. doi: 10.1016/j.meatsci.2007.01.022
Chang, H., and Wong, R. (2012). Textural and biochemical properties of cobia (Rachycentron canadum) sashimi tenderised with the ultrasonic water bath. Food Chem. 132, 1340–1345. doi: 10.1016/j.foodchem.2011.11.116
Chang, H. J., Wang, Q., Tang, C. H., and Zhou, G. H. (2015). Effects of ultrasound treatment on connective tissue collagen and meat quality of beef semitendinosus muscle. J. Food Quality. 38, 256–267. doi: 10.1111/jfq.12141
Chang, H. J., Xu, X. L., Zhou, G. H., Li, C. B., and Huang, M. (2012). Effects of characteristics changes of collagen on meat physicochemical properties of beef semitendinosus muscle during ultrasonic processing. Food Bioprocess Technol. 5, 285–297. doi: 10.1007/s11947-009-0269-9
Chang, Y. S., Wu, S. Y., Stromer, M. H., and Chou, R. R. (2020). Calpain activation and proteolysis in postmortem goose muscles. Anim. Sci. J. 91, e13423. doi: 10.1111/asj.13423
Chemat, F., and Ashokkumar, M. (2017). Preface: Ultrasound in the processing of liquid foods, beverages and alcoholic drinks. Ultrason. Sonochem. 38, 753. doi: 10.1016/j.ultsonch.2017.01.041
Chen, L., Feng, X. C., Zhang, Y., Liu, X., Zhang, W., Li, C., et al. (2015). Effects of ultrasonic processing on caspase-3, calpain expression and myofibrillar structure of chicken during post-mortem ageing. Food Chem. 177, 280–287. doi: 10.1016/j.foodchem.2014.11.064
Chéret, R., Delbarre-Ladrat, C., de Lamballerie-Anton, M., and Verrez-Bagnis. (2007). Calpain and cathepsin activities in post mortem fish and meat muscles. Food Chem. 101, 1474–1479. doi: 10.1016/j.foodchem.2006.04.023
Cramer, T., Penick, M. L., Waddell, J. N., Bidwell, C. A., and Kim, B. A. (2018). new insight into meat toughness of callipyge lamb loins-the relevance of anti-apoptotic systems to decreased proteolysis. Meat Sci. 140, 66–71. doi: 10.1016/j.meatsci.2018.03.002
Cruzen, S. M., Paulino, P. V., Lonergan, S. M., and Huff-Lonergan, E. (2014). Postmortem proteolysis in three muscles from growing and mature beef cattle. Meat Sci. 96, 854–861. doi: 10.1016/j.meatsci.2013.09.021
Czechowska-Biskup, R., Rokita, B., Lotfy, S., Ulanski, P., and Rosiak, J. M. (2005). Degradation of chitosan and starch by 360-kHz ultrasound. Carbohyd. Polym. 60, 175–184. doi: 10.1016/j.carbpol.2004.12.001
da Silva, S. L., Lorenzo, J. M., Machado, J. M., Manfio, M., Cichoski, A. J., Fries, L. L. M., et al. (2020). Application of arginine and histidine to improve the technological and sensory properties of low-fat and low-sodium bologna-type sausages produced with high levels of KCl. Meat Sci. 159, 107939. doi: 10.1016/j.meatsci.2019.107939
de Lima Alves, L., da Silva, M. S., Flores, D. R. M., Athayde, D. R., Ruviaro, A. R., Da Silva Brum, D., et al. (2018). Effect of ultrasound on the physicochemical and microbiological characteristics of Italian salami. Food Res. Int. 106, 363–373. doi: 10.1016/j.foodres.2017.12.074
Domínguez, R., Pateiro, M., Gagaoua, M., Barba, F. J., Zhang, W., Lorenzo, J. M. A., et al. (2019). A comprehensive review on lipid oxidation in meat and meat products. Antioxidants. 8, 429. doi: 10.3390/antiox8100429
Dong, Z. Y., Li, M. Y., Tian, G., Zhang, T. H., Ren, H., Quek, S. Y., et al. (2019). Effects of ultrasonic pretreatment on the structure and functionality of chicken bone protein prepared by enzymatic method. Food Chem. 299, 125103. doi: 10.1016/j.foodchem.2019.125103
Du, X., Li, H., Nuerjiang, M., Shi, S., Kong, B., Liu, Q., et al. (2021). Application of ultrasound treatment in chicken gizzards tenderization: effects on muscle fiber and connective tissue. Ultrason. Sonochem. 79, 105786. doi: 10.1016/j.ultsonch.2021.105786
Flint, E. B., and Suslick, K. S. (1991). The temperature of cavitation. Science. 253, 1397–1399. doi: 10.1126/science.253.5026.1397
Gagaoua, M., Picard, B., and Monteils, V. (2019). Assessment of cattle inter-individual cluster variability: the potential of continuum data from the farm-to-fork for ultimate beef tenderness management. J. Sci. Food Agric. 99, 4129–4141. doi: 10.1002/jsfa.9643
Gagaoua, M., Terlouw, E. M. C., Mullen, A. M., Franco, D., Warner, R. D., Lorenzo, J. M., et al. (2021). Molecular signatures of beef tenderness: Underlying mechanisms based on integromics of protein biomarkers from multi-platform proteomics studies. Meat Sci. 172, 108311. doi: 10.1016/j.meatsci.2020.108311
Geesink, G. H., Kuchay, S., Chishti, A. H., and Koohmaraie, M. (2006). μ-Calpain is essential for postmortem proteolysis of muscle proteins. J. Anim. Sci. 84, 2834–2840. doi: 10.2527/jas.2006-122
Ghafoor, M., Misra, N. N., Mahadevan, K., and Tiwari, B. K. (2014). Ultrasound assisted hydration of navy beans (Phaseolus vulgaris). Ultrason. Sonochem. 21, 409–414. doi: 10.1016/j.ultsonch.2013.05.016
Goll, D. E., Thompson, V. F., Li, H., Wei, W., and Cong, J. (2003). The calpain system. Physiol. Rev. 83, 731–801. doi: 10.1152/physrev.00029.2002
Gómez-Salazar, J. A., Galván-Navarro, A., Lorenzo, J. M., and Sosa-Morales, M. E. (2021). Ultrasound effect on salt reduction in meat products: a review. Curr. Opin. Food Sci. 38, 71–78. doi: 10.1016/j.cofs.2020.10.030
Gómez-Salazar, J. A., Ochoa-Montes, D. A., Cerón-García, A., Ozuna, C., and Sosa-Morales, M. E. (2018). Effect of acid marination assisted by power ultrasound on the quality of rabbit meat. J. Food Quality. 2018, 1–6. doi: 10.1155/2018/5754930
Gonzalez-Gonzalez, L., Alarcon-Rojo, A. D., Carrillo-Lopez, L. M., Garcia-Galicia, I. A., Huerta-Jimenez, M., Paniwnyk, L., et al. (2020). Does ultrasound equally improve the quality of beef? An insight into longissimus lumborum, infraspinatus and cleidooccipitalis. Meat Sci. 160, 107963. doi: 10.1016/j.meatsci.2019.107963
González-González, L., Luna-Rodríguez, L., Carrillo-López, L. M., Alarcón-Rojo, A. D., García-Galicia, I., Reyes-Villagrana, R., et al. (2017). Ultrasound as an alternative to conventional marination: acceptability and mass transfer. J. Food Quality. 2017, 1–8. doi: 10.1155/2017/8675720
Goudoulas, T. B., and Germann, N. (2017). Phase transition kinetics and rheology of gelatin-alginate mixtures. Food Hydrocoll. 66, 49–60. doi: 10.1016/j.foodhyd.2016.12.018
Guo, X., Gao, F., Zhang, Y., Peng, Z., and Jamali, M. A. (2021). Effect of l-histidine and l-lysine on the properties of oil-in-water emulsions stabilized by porcine myofibrillar proteins at low/high ionic strength. LWT. 141, 110883. doi: 10.1016/j.lwt.2021.110883
Hopkins, D. L., and Thompson, J. M. (2001). Inhibition of protease activity. Part 1. The effect on tenderness and indicators of proteolysis in ovine muscle. Meat Sci. 59, 175–185. doi: 10.1016/S0309-1740(01)00068-7
Hu, J., Ge, S., Huang, C., Cheung, P. C. K., Lin, L., Zhang, Y., et al. (2018). Tenderization effect of whelk meat using ultrasonic treatment. Food Sci. Nutr. 6, 1848–1857. doi: 10.1002/fsn3.686
Hu, Y., Yu, H., Dong, K., Yang, S., and Ye, X. (2014). Chen. Analysis of the tenderisation of jumbo squid (Dosidicus gigas) meat by ultrasonic treatment using response surface methodology. Food Chem. 160, 219–225. doi: 10.1016/j.foodchem.2014.01.085
Huang, F., Huang, M., Zhou, G., Xu, X., and Xue, M. (2011). In vitro proteolysis of myofibrillar proteins from beef skeletal muscle by caspase-3 and caspase-6. J. Agric. Food Chem. 59, 9658–9663. doi: 10.1021/jf202129r
Huang, M., Bao, J., Hallström, B. M., Petranovic, D., and Nielsen, J. (2017). Efficient protein production by yeast requires global tuning of metabolism. Nat. Commun. 8, 1–12. doi: 10.1038/s41467-017-00999-2
Huff-Lonergan, E., and Lonergan, S. M. (2005). Mechanisms of water-holding capacity of meat: the role of postmortem biochemical and structural changes. Meat Sci. 71, 194–204. doi: 10.1016/j.meatsci.2005.04.022
Inguglia, E. S., Zhang, Z., Burgess, C., Kerry, J. P., and Tiwari, B. K. (2018). Influence of extrinsic operational parameters on salt diffusion during ultrasound assisted meat curing. Ultrasonics. 83, 164–170. doi: 10.1016/j.ultras.2017.03.017
Jadhav, H. B., Annapure, U. S., and Deshmukh, R. R. (2021). Non-thermal technologies for food processing. Front. Nutr. 8, 657090. doi: 10.3389/fnut.2021.657090
Jayasooriya, S. D., Torley, P. J., D'arcy, B. R., and Bhandari, B. R. (2007). Effect of high power ultrasound and ageing on the physical properties of bovine Semitendinosus and Longissimus muscles. Meat Sci. 75, 628–639. doi: 10.1016/j.meatsci.2006.09.010
Jiménez Muñoz, L. M., Díaz, I. S., Rohner, C. S., Ramirez, G. C., and Ambrosio, A. F. (2017). Effectiveness of high power ultrasound for surimi-based preparation of lionfish (Pterois volitans) patties by textural, sensory and shape preference. J. Culin. Sci. Technol. 17, 89–102. doi: 10.1080/15428052.2017.1404538
Jørgensen, A. S., Christensen, M., and Ertbjerg, P. (2008). Marination with kiwifruit powder followed by power ultrasound tenderizes porcine M. Biceps Femoris. Paper presented at the 54th International Congress of Meat Science and Technology. doi: 10.1002/jsfa.3633
Jun-Hui, X., Hui-Juan, C., Bin, Z., and Hui, Y. (2020). The mechanistic effect of bromelain and papain on tenderization in jumbo squid (Dosidicus gigas) muscle. Food Res. Int. 131, 108991. doi: 10.1016/j.foodres.2020.108991
Kahraman, T., Bayraktaroglu, A. G., Vural, A., Issa, G., and Ergun, E. (2011). Electron microscopy of contractile bands and quality characteristics in high-voltage electrical stimulation broiler breast meat. Poult. Sci. 90, 486–490. doi: 10.3382/ps.2010-01096
Kang, D., Gao, X., Ge, Q., Zhou, G., and Zhang, W. (2017). Effects of ultrasound on the beef structure and water distribution during curing through protein degradation and modification. Ultrason. Sonochem. 38, 317–325. doi: 10.1016/j.ultsonch.2017.03.026
Kang, D., Wang, A., Zhou, G., Zhang, W., Xu, S., Guo, G., et al. (2016b). Power ultrasonic on mass transport of beef: Effects of ultrasound intensity and NaCl concentration. Innov. Food Sci. Emerg. Technol. 35, 36–44. doi: 10.1016/j.ifset.2016.03.009
Kang, D., Zou, Y., Cheng, Y., Xing, L., Zhou, G., Zhang, W., et al. (2016a). Effects of power ultrasound on oxidation and structure of beef proteins during curing processing. Ultrason. Sonochem. 33, 47–53. doi: 10.1016/j.ultsonch.2016.04.024
Kasaai, M. R. (2013). Input power-mechanism relationship for ultrasonic irradiation: Food and polymer applications. Nat. Sci. 5, 14–22. doi: 10.4236/ns.2013.58A2003
Kaur, L., Hui, S. X., Morton, J. D., Kaur, R., Chian, F. M., Boland, M., et al. (2021). Endogenous proteolytic systems and meat tenderness: Influence of post-mortem storage and processing. Food Sci. Anim. Resour. 41, 589–607. doi: 10.5851/kosfa.2021.e27
Kim, D. S., and Joo, N. (2020). Texture characteristics of horse meat for the elderly based on the enzyme treatment. Food Sci. Anim. Resour. 40, 74. doi: 10.5851/kosfa.2019.e86
Kim, H. W., Choi, Y. S., Choi, J. H., Kim, H. Y., Lee, M. A., Hwang, K. E., et al. (2013). Tenderization effect of soy sauce on beef M. biceps femoris. Food Chem. 139, 597–603. doi: 10.1016/j.foodchem.2013.01.050
Kim, Y. H. B., Ma, D., Setyabrata, D., Farouk, M. M., Lonergan, S. M., Huff-Lonergan, E., et al. (2018). Understanding postmortem biochemical processes and post-harvest aging factors to develop novel smart-aging strategies. Meat Sci. 144, 74–90. doi: 10.1016/j.meatsci.2018.04.031
Koohmaraie, M., and Geesink, G. H. (2006). Contribution of postmortem muscle biochemistry to the delivery of consistent meat quality with particular focus on the calpain system. Meat Sci. 74, 34–43. doi: 10.1016/j.meatsci.2006.04.025
Latorre, M. E., Palacio, M. I., Velázquez, D. E., and Purslow, P. P. (2019). Specific effects on strength and heat stability of intramuscular connective tissue during long time low temperature cooking. Meat Sci. 153, 109–116. doi: 10.1016/j.meatsci.2019.03.016
Li, K., Kang, Z. L., Zou, Y. F., Xu, X. L., and Zhou, G. H. (2015). Effect of ultrasound treatment on functional properties of reduced-salt chicken breast meat batter. J. Food Sci. Technol. 52, 2622–2633. doi: 10.1007/s13197-014-1356-0
Li, X., Wang, Y., Sun, Y. Y., Pan, D. D., and Cao, J. X. (2018). The effect of ultrasound treatments on the tenderizing pathway of goose meat during conditioning. Poult. Sci. 97, 2957–2965. doi: 10.3382/ps/pey143
Li, X., Wang, Z., and Xia, H. (2019). Ultrasound reversible response nanocarrier based on sodium alginate modified mesoporous silica nanoparticles. Front. Chem. 7, 00059. doi: 10.3389/fchem.2019.00059
Lian, T., Wang, L., and Liu, Y. A. (2013). New insight into the role of calpains in post-mortem meat tenderization in domestic animals: a review. Asian-Australas J. Anim. Sci. 26, 443. doi: 10.5713/ajas.2012.12365
Lin, D., Zhang, Q., Xiao, L., Huang, Y., Yang, Z., Wu, Z., et al. (2021). Effects of ultrasound on functional properties, structure and glycation properties of proteins: a review. Crit. Rev. Food Sci. 61, 2471–2481. doi: 10.1080/10408398.2020.1778632
Linares, G., and Rojas, M. L. (2022). Ultrasound-assisted extraction of natural pigments from food processing by-products: a review. Front. Nut. 9, 891462. doi: 10.3389/fnut.2022.891462
Liu, R., Li, Y., Wang, M., Zhou, G., and Zhang, W. (2016). Effect of Protein S-Nitrosylation on Autolysis and Catalytic Ability of Mu-Calpain. Food Chem. 213, 470–477. doi: 10.1016/j.foodchem.2016.06.104
Locker, R., and Hagyard, C. J. A. (1963). cold shortening effect in beef muscles. J. Sci. Food Agr. 14, 787–793. doi: 10.1002/jsfa.2740141103
Lonergan, E. H., Zhang, W., and Lonergan, S. M. (2010). Biochemistry of postmortem muscle—Lessons on mechanisms of meat tenderization. Meat Sci. 86, 184–195. doi: 10.1016/j.meatsci.2010.05.004
Lorimer, J. P., and Mason, T. J. (1987). Sonochemistry. Part 1-the physical aspects. Chem. Soc. Rev. 16, 239–274. doi: 10.1039/CS9871600239
Luo, M., Shan, K., Zhang, M., Ke, W., Zhao, D., Nian, Y., et al. (2021). Application of ultrasound treatment for improving the quality of infant meat puree. Ultrason. Sonochem. 80, 105831. doi: 10.1016/j.ultsonch.2021.105831
Lyu, J., and Ertbjerg, P. (2021). Ca2+-induced binding of calpain-2 to myofibrils: Preliminary results in pork longissimus thoracis muscle supporting a role on myofibrillar protein degradation. Meat Sci. 172, 108364. doi: 10.1016/j.meatsci.2020.108364
Ma, D., and Kim, Y. H. B. (2020). Proteolytic changes of myofibrillar and small heat shock proteins in different bovine muscles during aging: Their relevance to tenderness and water-holding capacity. Meat Sci. 163, 108090. doi: 10.1016/j.meatsci.2020.108090
Ma, X., Mei, J., and Xie, J. (2021b). Mechanism of ultrasound assisted nucleation during freezing and its application in food freezing process. Int. J. Food Prop. 24, 68–88. doi: 10.1080/10942912.2020.1858862
Ma, X., Yang, D., Qiu, W., Mei, J., and Xie, J. (2021a). Influence of multifrequency ultrasound-assisted freezing on the flavour attributes and myofibrillar protein characteristics of cultured large yellow croaker (Larimichthys crocea). Front. Nut. 8, 779546. doi: 10.3389/fnut.2021.779546
Madhusankha, G. D. M. P., and Thilakarathna, R. C. N. (2021). Meat tenderization mechanism and the impact of plant exogenous proteases: a review. Arab. J. Chem. 14, 102967. doi: 10.1016/j.arabjc.2020.102967
Mason, T. J., Paniwnyk, L., and Lorimer, J. P. (1996). The uses of ultrasound in food technology. Ultrason. Sonochem. 3, S253–S60. doi: 10.1016/S1350-4177(96)00034-X
McDonnell, C. K., Allen, P., Morin, C., and Lyng, J. G. (2014). The effect of ultrasonic salting on protein and water–protein interactions in meat. Food Chem. 147, 245–251. doi: 10.1016/j.foodchem.2013.09.125
Mehrabani, A., Javan, A. J., Hesarinejad, M. A., Mahdavi, A., and Parsaeimehr, M. (2022). The combined effect of ultrasound treatment and leek (Allium ampeloprasum) extract on the quality properties of beef. Food Biosci. 47, 101622. doi: 10.1016/j.fbio.2022.101622
Mullen, A. M., Álvarez, C., Zeugolis, D. I., Henchion, M., O'Neill, E., Drummond, L., et al. (2017). Alternative uses for co-products: Harnessing the potential of valuable compounds from meat processing chains. Meat Sci. 132, 90–98. doi: 10.1016/j.meatsci.2017.04.243
O'Halloran, G. R., Troy, D. J., Buckley, D. J., and Reville, W. J. (1997). The role of endogenous proteases in the tenderisation of fast glycolysing muscle. Meat Sci. 47, 187–210. doi: 10.1016/S0309-1740(97)00046-6
Ojha, K. S., Keenan, D. F., Bright, A., Kerry, J. P., and Tiwari, B. (2016). Ultrasound assisted diffusion of sodium salt replacer and effect on physicochemical properties of pork meat. Int. J. Food Sci. Tech. 51, 37–45. doi: 10.1111/ijfs.13001
Ojha, K. S., Kerry, J. P., and Tiwari, B. K. (2017). Investigating the influence of ultrasound pre-treatment on drying kinetics and moisture migration measurement in Lactobacillus sakei cultured and uncultured beef jerky. LWT. 81, 42–49. doi: 10.1016/j.lwt.2017.03.011
Ouali, A., Gagaoua, M., Boudida, Y., Becila, S., Boudjellal, A., Herrera-Mendez, C. H., et al. (2013). Biomarkers of meat tenderness: present knowledge and perspectives in regards to our current understanding of the mechanisms involved. Meat Sci. 95, 854–870. doi: 10.1016/j.meatsci.2013.05.010
Ozuna, C., Puig, A., García-Pérez, J. V., Mulet, A., and Cárcel, J. A. (2013). Influence of high intensity ultrasound application on mass transport, microstructure and textural properties of pork meat (Longissimus dorsi) brined at different NaCl concentrations. J. Food Eng. 119, 84–93. doi: 10.1016/j.jfoodeng.2013.05.016
Pan, J., Li, C., Liu, X., He, L., Zhang, M., Huang, S., et al. (2022). A multivariate insight into the organoleptic properties of porcine muscle by ultrasound-assisted brining: Protein oxidation, water state and microstructure. LWT. 159, 113136. doi: 10.1016/j.lwt.2022.113136
Pan, Z., Li, H., Lei, M., Ai, Z., Wu, Y., Huang, Z., et al. (2021). Effect of ultrasound combined with papain treatment on tenderness of chicken breast. FST. 42, 162–7. doi: 10.13386/j.issn1002-0306.2020080036
Patero, T., and Augusto, P. E. D. (2015). Ultrasound (US) enhances the hydration of sorghum (Sorghum bicolor) grains. Ultrason. Sonochem. 23, 11–15. doi: 10.1016/j.ultsonch.2014.10.021
Peña-Gonzalez, E., Alarcon-Rojo, A. D., Garcia-Galicia, I., Carrillo-Lopez, L., and Huerta-Jimenez, M. (2019). Ultrasound as a potential process to tenderize beef: Sensory and technological parameters. Ultrason. Sonochem. 53, 134–141. doi: 10.1016/j.ultsonch.2018.12.045
Peña-González, E. M., Alarcón-Rojo, A. D., Rentería, A., García, I., Santellano, E., Quintero, A., et al. (2017). Quality and sensory profile of ultrasound-treated beef. Ital J. Food Sci. 29. doi: 10.14674/1120-1770/ijfs.v604
Piatkov, K. I., Oh, J. H., Liu, Y., and Varshavsky, A. (2014). Calpain-generated natural protein fragments as short-lived substrates of the N-end rule pathway. Proc. Natl. Acad. Sci. USA. 111, E817–E826. doi: 10.1073/pnas.1401639111
Pietrasik, Z., and Janz, J. A. M. (2010). Utilization of pea flour, starch-rich and fiber-rich fractions in low fat bologna. Food Res. Int. 43, 602–608. doi: 10.1016/j.foodres.2009.07.017
Pohlman, F. W., Dikeman, M. E., Zayas, J. F., and Unruh, J. A. (1997). Effects of ultrasound and convection cooking to different end point temperatures on cooking characteristics, shear force and sensory properties, composition, and microscopic morphology of beef longissimus and pectoralis muscles. J. Anim. Sci. 75, 386–401. doi: 10.2527/1997.752386x
Purslow, P. P. (2018). Contribution of collagen and connective tissue to cooked meat toughness; some paradigms reviewed. Meat Sci. 144, 127–134. doi: 10.1016/j.meatsci.2018.03.026
Rhee, M. S., Wheeler, T. L., and Shackelford, S. D. (2004). Koohmaraie. Variation in palatability and biochemical traits within and among eleven beef muscles. J. Anim. Sci. 82, 534–550. doi: 10.2527/2004.822534x
Rowe, L. J., Maddock, K. R., Lonergan, S. M., and Huff-Lonergan, E. (2004). Influence of early postmortem protein oxidation on beef quality. J. Anim. Sci. 82, 785–793. doi: 10.2527/2004.823785x
Ščetar, M., Kurek, M., ReŽek Jambrak, A., Debeaufort, F., and Galić, K. (2019). Effect of high power ultrasound on physical–chemical properties of polypropylene films aimed for food packaging: Structure and surface features. Polym. Bull. 76, 1007–1021. doi: 10.1007/s00289-018-2416-9
Sentandreu, M. A., Coulis, G., and Ouali, A. (2002). Role of muscle endopeptidases and their inhibitors in meat tenderness. Trends Food Sci. Technol. 13, 400–421. doi: 10.1016/S0924-2244(02)00188-7
Shen, Q. W., Swartz, D. R., Wang, Z., Liu, Y., Gao, Y., Zhang, D., et al. (2016). Different actions of salt and pyrophosphate on protein extraction from myofibrils reveal the mechanism controlling myosin dissociation. J. Sci. Food Agr. 96, 2033–2039. doi: 10.1002/jsfa.7314
Shi, H., Khan, I. A., Zhang, R., Zou, Y., Xu, W., Wang, D., et al. (2022). Evaluation of ultrasound-assisted L-histidine marination on beef M. semitendinosus: Insight into meat quality and actomyosin properties. Ultrason. Sonochem. 85, 105987. doi: 10.1016/j.ultsonch.2022.105987
Shi, H., Zhang, X., Chen, X., Fang, R., Zou, Y., Wang, D., et al. (2020). How ultrasound combined with potassium alginate marination tenderizes old chicken breast meat: Possible mechanisms from tissue to protein. Food Chem. 328, 127144. doi: 10.1016/j.foodchem.2020.127144
Sikes, A., and Tobin, A. (2021). “Shock wave processing for meat tenderization,” in Innovative Food Processing Technologies Elsevier, Eds K. Knoerzer and K. Muthukumarappan, 426–38. doi: 10.1016/B978-0-08-100596-5.23011-4
Smith, N., Cannon, J., Novakofski, J., McKeith, F., and O'Brien, W. (1991). Tenderization of semitendinosus muscle using high intensity ultrasound. IEEE 1991 Ultrasonics Symposium, 1371–74. doi: 10.1109/ULTSYM.1991.234038
Smuder, A. J., Kavazis, A. N., Hudson, M. B., Nelson, W. B., and Powers, S. K. (2010). Oxidation enhances myofibrillar protein degradation via calpain and caspase-3. Free Radic. Biol. Med. 49, 1152–1160. doi: 10.1016/j.freeradbiomed.2010.06.025
Stadnik, J., Dolatowski, Z. J., and Baranowska, H. M. (2008). Effect of ultrasound treatment on water holding properties and microstructure of beef (m. semimembranosus) during ageing. LWT. 41, 2151–2158. doi: 10.1016/j.lwt.2007.12.003
Sun, Q., Zhao, X., Zhang, C., Xia, X., Sun, F., Kong, B., et al. (2019). Ultrasound-assisted immersion freezing accelerates the freezing process and improves the quality of common carp (Cyprinus carpio) at different power levels. LWT. 108, 106–112. doi: 10.1016/j.lwt.2019.03.042
Tan, S. M., De Kock, H. L., Dykes, G. A., Coorey, R., and Buys, E. M. (2018). Enhancement of poultry meat, nutritional profile, legislation and challenges. S. Afr. J. Anim. 48, 199–212. doi: 10.4314/sajas.v48i2.1
Tang, L., and Yongsawatdigul, J. (2021). High-intensity ultrasound improves threadfin bream surimi gelation at low NaCl contents. J. Food Sci. 86, 842–851. doi: 10.1111/1750-3841.15637
Tantamacharik, T., Carne, A., Agyei, D., Birch, J., and Bekhit, E. (2018). “Use of Plant Proteolytic Enzymes for Meat Processing,”. in: Biotechnological Applications of Plant Proteolytic Enzymes, Eds M. Guevara and G. Daleo (Springer Cham), 43–67. doi: 10.1007/978-3-319-97132-2_3
Taylor, R. G., Geesink, G., Thompson, V. F., Koohmaraie, M., and Goll, D. E. (1995). Is Z-disk degradation responsible for postmortem tenderization? J. Anim. Sci. 73, 1351–1367. doi: 10.2527/1995.7351351x
Tornberg, E. (1996). Biophysical aspects of meat tenderness. Meat Sci. 43, 175–191. doi: 10.1016/0309-1740(96)00064-2
Torres, R. A., Pétrier, C., Combet, E., Carrier, M., and Pulgarin, C. (2008). Ultrasonic cavitation applied to the treatment of bisphenol A. Effect of sonochemical parameters and analysis of BPA by-products. Ultrason. Sonochem. 15, 605–611. doi: 10.1016/j.ultsonch.2007.07.003
Umego, E. C., He, R., Ren, W., Xu, H., and Ma, H. (2021). Ultrasonic-assisted enzymolysis: Principle and applications. Peocess Biochem. 100, 59–68. doi: 10.1016/j.procbio.2020.09.033
Uytterhaegen, Claeys E., and Demeyer, D. (1994). Effects of exogenous protease effectors on beef tenderness development and myofibrillar degradation and solubility. J. Anim. Sci. 72, 1209–1223. doi: 10.2527/1994.7251209x
Vandenberghe-Descamps, M., Sulmont-Ross,é, C., Septier, C., Follot, C., Feron, G., Labour,é, H., et al. (2018). Impact of blade tenderization, marinade and cooking temperature on oral comfort when eating meat in an elderly population. Meat Sci. 145, 86–93. doi: 10.1016/j.meatsci.2018.06.004
Vanderhout, R. J., Leishman, E. M., Abdalla, E. A., Barbut, S., Wood, B. J., Baes, C. F., et al. (2022). Genetic parameters of white striping and meat quality traits indicative of pale, soft, exudative meat in Turkeys (Meleagris gallopavo). Front. Genet. 13, 842584. doi: 10.3389/fgene.2022.842584
Veiseth, E., Shackelford, S. D., Wheeler, T. L., and Koohmaraie, M. (2004). Factors regulating lamb longissimus tenderness are affected by age at slaughter. Meat Sci. 68, 635–640. doi: 10.1016/j.meatsci.2004.05.015
Vidal, V. A. S., Santana, J. B., Paglarini, C. S., da Silva, M. A. A. P., Freitas, M. Q., Esmerino, E. A., et al. (2020). Adding lysine and yeast extract improves sensory properties of low sodium salted meat. Meat Sci. 159, 107911. doi: 10.1016/j.meatsci.2019.107911
Vilar, E. G., Ouyang, H., O'Sullivan, M. G., Kerry, J. P., Hamill, R. M., O'Grady, M. N., et al. (2020). Effect of salt reduction and inclusion of 1% edible seaweeds on the chemical, sensory and volatile component profile of reformulated frankfurters. Meat Sci. 161, 108001. doi: 10.1016/j.meatsci.2019.108001
Visy, A., Jónás, G., Szakos, D., Horváth-Mezofi, Z., Hidas, K. I., Bark,ó, A., et al. (2021). Evaluation of ultrasound and microbubbles effect on pork meat during brining process. Ultrason. Sonochem. 75, 105589. doi: 10.1016/j.ultsonch.2021.105589
Wang, A., Kang, D., Zhang, W., Zhang, C., Zou, Y., Zhou, G., et al. (2018b). Changes in calpain activity, protein degradation and microstructure of beef M. semitendinosus by the application of ultrasound. Food Chem. 245, 724–730. doi: 10.1016/j.foodchem.2017.12.003
Wang, L. L., Han, L., Ma, X. L., Yu, Q. L., and Zhao, S.-. N. (2017). Effect of mitochondrial apoptotic activation through the mitochondrial membrane permeability transition pore on yak meat tenderness during postmortem aging. Food Chem. 234, 323–331. doi: 10.1016/j.foodchem.2017.04.185
Wang, W., Chen, W., Zou, M., Lv, R., Wang, D., Hou, F., et al. (2018a). Applications of power ultrasound in oriented modification and degradation of pectin: a review. J. Food Eng. 234, 98–107. doi: 10.1016/j.jfoodeng.2018.04.016
Wang, Y., Zhao, J., Zhang, W., Liu, C., Jauregi, P., Huang, M., et al. (2020). Modification of heat-induced whey protein gels by basic amino acids. Food Hydrocoll. 100, 105397. doi: 10.1016/j.foodhyd.2019.105397
Wen, C., Zhang, J., Yao, H., Zhou, J., Duan, Y., Zhang, H., et al. (2019). Advances in renewable plant-derived protein source: the structure, physicochemical properties affected by ultrasonication. Ultrason. Sonochem. 53, 83–98. doi: 10.1016/j.ultsonch.2018.12.036
Whipple, G., and Koohmaraie, M. (1992). Effects of lamb age, muscle type, and 24-hour activity of endogenous proteinases on postmortem proteolysis. J. Anim. Sci. 70, 798. doi: 10.2527/1992.703798x
Wójciak, K. M., Stasiak, D. M., Stadnik, J., Ferysiuk, K., and Kononiuk, A. (2019). Th The influence of sonication time on the biogenic amines formation as a critical point in uncured dry-fermented beef manufacturing. Int. J. Food Sci. Tech. 54, 75–83. doi: 10.1111/ijfs.13906
Xiong, G., Fu, X., Pan, D., Qi, J., Xu, X., Jiang, X., et al. (2020). Influence of ultrasound-assisted sodium bicarbonate marination on the curing efficiency of chicken breast meat. Ultrason. Sonochem. 60, 104808. doi: 10.1016/j.ultsonch.2019.104808
Xiong, G.-Y., Zhang, L.-L., Zhang, W., and Wu, J. (2012). Influence of ultrasound and proteolytic enzyme inhibitors on muscle degradation, tenderness, and cooking loss of hens during aging. Czech. J. Food Sci. 30, 195–205. doi: 10.17221/136/2011-CJFS
Xue, S., Xu, X., Shan, H., Wang, H., Yang, J., Zhou, G., et al. (2018). Effects of high-intensity ultrasound, high-pressure processing, and high-pressure homogenization on the physicochemical and functional properties of myofibrillar proteins. Innov. Food Sci. Emerg. Technol. 45, 354–360. doi: 10.1016/j.ifset.2017.12.007
Yao, J., Zhou, Y., Chen, X., Ma, F., Li, P., Chen, C., et al. (2018). Effect of sodium alginate with three molecular weight forms on the water holding capacity of chicken breast myosin gel. Food Chem. 239, 1134–1142. doi: 10.1016/j.foodchem.2017.07.027
Yao, Y., Zhang, W., and Liu, S. (2009). Feasibility study on power ultrasound for regeneration of silica gel—a potential desiccant used in air-conditioning system. Appl. Energy. 86, 2394–2400. doi: 10.1016/j.apenergy.2009.04.001
Yeung, C. K., and Huang, S. C. (2017). Effects of ultrasound pretreatment and ageing processing on quality and tenderness of pork loin. J. Food Nutr. Res-Slove. 5, 809–816. doi: 10.12691/jfnr-5-11-3
Yeung, C. K., Huang, S. C., and Research, N. (2017). Effects of ultrasound pretreatment and ageing processing on quality and tenderness of pork loin. J. Food Nutr. Res-slov. 5, 809–816.
Yu, Z. L., Zeng, W. C., and Lu, X. L. (2013). Influence of ultrasound to the activity of tyrosinase. Ultrason. Sonochem. 20, 805–809. doi: 10.1016/j.ultsonch.2012.11.006
Yu, Z. L., Zeng, W. C., Zhang, W. H., Liao, X. P., and Shi, B. (2014). Effect of ultrasound on the activity and conformation of α-amylase, papain and pepsin. Ultrason. Sonochem. 21, 930–936. doi: 10.1016/j.ultsonch.2013.11.002
Zeece, M. G., Katoh, K., Robson, R. M., and Parrish, J. R. (1986). Effect of Cathepsin D on Bovine Myofibrils under Different Conditions of pH and Temperature. J. Food Sci. 51, 769–773. doi: 10.1111/j.1365-2621.1986.tb13930.x
Zhang, J., Yu, Q., Han, L., Han, M., and Han, G. (2020). Effects of lysosomal iron involvement in the mechanism of mitochondrial apoptosis on postmortem muscle protein degradation. Food Chem. 328, 127174. doi: 10.1016/j.foodchem.2020.127174
Zhang, W., Xiao, S., and Ahn, D. U. (2013). Protein oxidation: basic principles and implications for meat quality. Crit. Rev. Food Sci. 53, 1191–1201. doi: 10.1080/10408398.2011.577540
Zhang, Y., and Abatzoglou, N. (2020). Design. Fundamentals, applications and potentials of ultrasound-assisted drying. Chem. Eng. Res. Des. 154, 21–46. doi: 10.1016/j.cherd.2019.11.025
Zhao, L., Zhou, Z., He, Q., Tang, C., Huang, M., Li, M., et al. (2018). Effects of ultrasonic-assisted bromelain hydrolysis on tenderization of duck meat. Food Sci. 39, 93–100. doi: 10.7506/spkx1002-6630-201812015
Zhou, Y., Chen, C., Chen, X., Li, P., Ma, F., Lu, Q., et al. (2014). Contribution of three ionic types of polysaccharides to the thermal gelling properties of chicken breast myosin. J. Agric. Food Chem. 62, 2655–2662. doi: 10.1021/jf405381z
Zhu, X., Ning, C., Li, S., Xu, P., Zheng, Y., Zhou, C., et al. (2018). Effects of l-lysine/l-arginine on the emulsion stability, textural, rheological and microstructural characteristics of chicken sausages. Int J. Food Sci. 53, 88–96. doi: 10.1111/ijfs.13561
Zhuang, X., Wang, L., Jiang, X., Chen, Y., and Zhou, G. (2020). The effects of three polysaccharides on the gelation properties of myofibrillar protein: Phase behaviour and moisture stability. Meat Sci. 170, 108228. doi: 10.1016/j.meatsci.2020.108228
Zou, Y., Jiang, D., Xu, P., Huang, Y., Fang, R., Wang, D., et al. (2020). Evaluation of the postmortem ageing process of beef M. semitendinosus based on ultrasound-assisted L-histidine treatment. Ultrason. Sonochem. 69, 105265. doi: 10.1016/j.ultsonch.2020.105265
Zou, Y., Shi, H., Chen, X., Xu, P., Jiang, D., Xu, W., et al. (2019). Modifying the structure, emulsifying and rheological properties of water-soluble protein from chicken liver by low-frequency ultrasound treatment. Int. J. Biol. Macromol. 139, 810–817. doi: 10.1016/j.ijbiomac.2019.08.062
Zou, Y., Xu, P., Wu, H., Zhang, M., Sun, Z., Sun, C., et al. (2018b). Effects of different ultrasound power on physicochemical property and functional performance of chicken actomyosin. Int. J. Biol. Macromol. 113, 640–647. doi: 10.1016/j.ijbiomac.2018.02.039
Keywords: meat, meat maturation, tenderization mechanism, ultrasound-assisted tenderization, quality
Citation: Dong Y, Zhang H, Mei J, Xie J and Shao C (2022) Advances in application of ultrasound in meat tenderization: A review. Front. Sustain. Food Syst. 6:969503. doi: 10.3389/fsufs.2022.969503
Received: 15 June 2022; Accepted: 10 August 2022;
Published: 25 August 2022.
Edited by:
José Antonio Teixeira, University of Minho, PortugalReviewed by:
Alma Alarcon-Rojo, Autonomous University of Chihuahua, MexicoCopyright © 2022 Dong, Zhang, Mei, Xie and Shao. This is an open-access article distributed under the terms of the Creative Commons Attribution License (CC BY). The use, distribution or reproduction in other forums is permitted, provided the original author(s) and the copyright owner(s) are credited and that the original publication in this journal is cited, in accordance with accepted academic practice. No use, distribution or reproduction is permitted which does not comply with these terms.
*Correspondence: Jun Mei, am1laUBzaG91LmVkdS5jbg==; Jing Xie, anhpZUBzaG91LmVkdS5jbg==
Disclaimer: All claims expressed in this article are solely those of the authors and do not necessarily represent those of their affiliated organizations, or those of the publisher, the editors and the reviewers. Any product that may be evaluated in this article or claim that may be made by its manufacturer is not guaranteed or endorsed by the publisher.
Research integrity at Frontiers
Learn more about the work of our research integrity team to safeguard the quality of each article we publish.