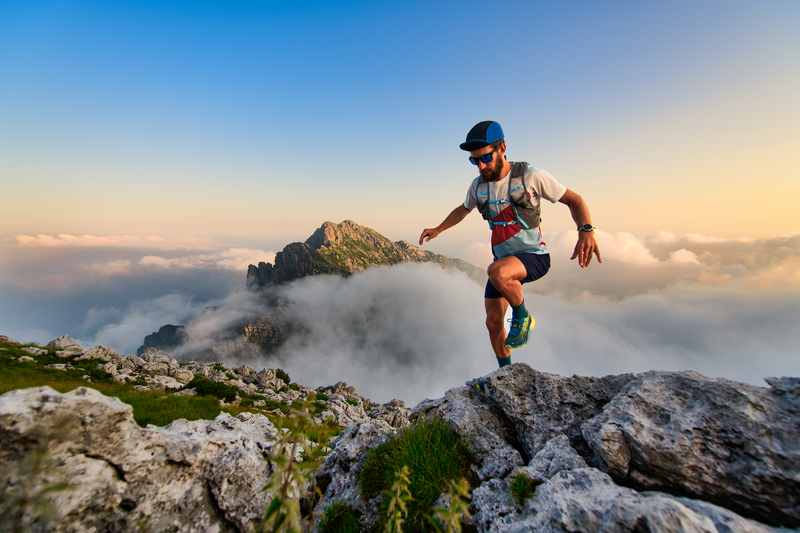
95% of researchers rate our articles as excellent or good
Learn more about the work of our research integrity team to safeguard the quality of each article we publish.
Find out more
ORIGINAL RESEARCH article
Front. Sustain. Food Syst. , 17 October 2022
Sec. Climate-Smart Food Systems
Volume 6 - 2022 | https://doi.org/10.3389/fsufs.2022.959591
Semi-arid regions faced with increasingly scarce freshwater resources must manage competing demands in the food-energy-water nexus. A possible solution modifies soil hydrologic properties using biosurfactants to reduce evaporation and improve water retention. In this study, two different soil textures representative of agricultural soils in Kansas were treated with a direct application of the biosurfactant, Surfactin, and an indirect application via inoculation of Bacillus subtilis. Evaporation rates of the wetted soils were measured when exposed to artificial sunlight (1000 W/m2) and compared to non-treated control soils. Experimental results indicate that both treatments alter soil moisture dynamics by increasing evaporation rates by when soil moisture is plentiful (i.e., constant rate period) and decreasing evaporation rates by when moisture is scarce (i.e., slower rate period). Furthermore, both treatments significantly reduced the soil moisture content at which the soil transitioned from constant rate to slower rate evaporation. Out of the two treatments, inoculation with B. subtilis generally produced greater changes in evaporation dynamics; for example, the treatment with B. subtilis in sandy loam soils increased constant rate periods of evaporation by 43% and decreased slower rate evaporation by 49%. In comparing the two soil textures, the sandy loam soil exhibited a larger treatment effect than the loam soil. To evaluate the potential significance of the treatment effects, a System Dynamics Model operationalized the evaporation rate results and simulated soil moisture dynamics under typical daily precipitation conditions. The results from this model indicate both treatment methods significantly altered soil moisture dynamics in the sandy loam soils and increased the probability of the soil exhibiting constant rate evaporation relative to the control soils. Overall, these findings suggest that the decrease in soil moisture threshold observed in the experimental setting could increase soil moisture availability by prolonging the constant rate stage of evaporation. As inoculation with B. subtilis in the sandy loam soil had the most pronounced effects in both the experimental and simulated contexts, future work should focus on testing this treatment in field trials with similar soil textures.
Semi-arid regions, such as southwest Kansas, are at the center of the Food-Energy-Water nexus. Food, energy, and water are also linked to societal concerns, such as poverty and economic growth (Finley and Seiber, 2014; Jägermeyr, 2020). Agriculture plays an important role in southwest Kansas, requiring energy and water for food production. The depleting Ogallala Aquifer, which this region relies on, is one of a select few aquifers absorbing the brunt of the Great Plains' freshwater demands (Siebert et al., 2010; Gleeson et al., 2012; Baumhardt et al., 2020; Ale et al., 2021). The Ogallala Aquifer depleted 267.5 acre-feet (i.e., 8.2%) of its total storage between 1950 and 2007 (Evett et al., 2014). In Kansas alone, the aquifer lost 63 acre-feet of water (i.e., 19.7% of the total Kansas storage) in the same timeframe (Gilson et al., 2001; Evett et al., 2014). As the water supply continues to deplete, the depths required to retrieve the water increase, requiring more energy to pump irrigation water (Vora et al., 2019; Zhou et al., 2020; Ale et al., 2021). This water depletion and increase in required pump energy create an even more convoluted and interdependent relationship between food, water, and energy.
Irrigation has the most significant impact on the Ogallala Aquifer's depletion compared to any other water use sector. It is an essential part of increasing crop yields and has a significant economic impact on producers' profit (Siebert et al., 2010; Gleeson et al., 2012; Finley and Seiber, 2014; Jägermeyr, 2020). In Kansas, irrigation accounts for 83% of reported water use, with the Ogallala aquifer providing 90% of that water (Kansas Department of Agriculture, 2019). In 2009, 70% of southwest Kansas' crop value came from irrigated land even though the irrigated area only accounted for 44% of the total area (Rogers and Lamm, 2012). Water stress induced by climate change may also affect land value, potentially reducing land rental rates by $3 billion annually over the next 30 years (Hendricks, 2018).
Methods of water application (e.g., irrigation methods and soil moisture monitoring) that reduce evaporation losses and increase the ratio of water applied to water consumed by crops are thoroughly researched and frequently adopted (Rogers and Lamm, 2012; Evett et al., 2014; Jägermeyr, 2020). These technologies utilized by producers can help in prioritizing higher soil moisture levels during critical growth stages, and conversely, allowing drought during others (Geerts and Raes, 2009; Fang et al., 2015). These methods focus on increasing the efficiency of water use by changing the method of water application, which can accidentally result in higher water consumption by producers (Jägermeyr, 2020; Pérez-Blanco et al., 2020).
Instead of focusing on water application, other methods of improving water use efficiency involve increasing soil water retention so that a greater portion of applied water effectively infiltrates into the soil and is used by crops. Mulching, for example, has been shown to improve the efficiency of water use in semi-arid growing regions by slowing the rate of evaporation (Chakraborty et al., 2008). Like other porous media, evaporation from soil can be classified into three periods: constant-rate, falling-rate, and slower-rate period (Hillel, 1998; Shokri et al., 2008, 2009b; Shokri and Or, 2011; Or et al., 2013). In the constant rate period, water is evaporated from the top surface of the soil through forced or natural convection while the top layer is saturated, and a hydraulic connection is maintained between saturated and unsaturated parts through capillary action. The capillary action driving evaporation during the constant rate period is also the transport mechanism used by crops for transpiration. The breakdown of this capillary flow defines the lower limits of plant available water (Braudeau et al., 2005). When this hydraulic connection breaks down, the porous media experiences a sharp decrease in evaporation rate defined as the falling rate period. For crops, the breakdown of capillary flow, and the associated reduction in hydraulic conductivity, in the falling rate period indicates the beginning of water stress (Feng et al., 2016). During the falling rate period, liquid islands are formed between particles of the porous system and later the evaporation enters into the diffusion dominated slower rate period with negligible evaporation when the liquid islands break down and most of the porous sample becomes unsaturated (Hillel, 1998; Lehmann et al., 2008; Shokri et al., 2009a, 2010). Zribi et al. (2015) demonstrated that mulching reduces the evaporation rate during the constant rate stage, when soil moisture is relatively high, and suggest mulching will be most effective in high-frequency irrigation systems as a result. Liao et al. (2021) also found that mulching reduces evaporation in the constant rate stage, but they also reported reduced evaporation in the falling rate stage when soil moisture is relatively scarce. Additionally, Liao et al. (2021) examined the soil moisture threshold separating the constant and falling rate stages of evaporation and found little difference between the bare soil and mulching treatments.
An alternative solution to improve water field capacity and reduce evaporation rates from the soil is the use of surfactants as soil amendments (Hallett, 2007; Matveeva et al., 2019). As wetting agents, surfactants have the potential to modify soil hydrological characteristics and improve soil moisture conditions without requiring the infrastructure of irrigation technologies or the application of mulching material (Abu-Zreig et al., 2003). Furthermore, they can potentially prolong wetter soil moisture conditions and increase peak soil moisture after wetting events (Raddadi et al., 2018; Lowe et al., 2019). Due to their amphiphilic nature, these surfactants can accumulate at a solid-liquid interface, preventing their degradation and reducing the surface tension between the water and the soil, thereby modifying soil wetting properties (Fernández-Gálvez and Mingorance, 2010; Lehrsch et al., 2011). Once the surfactant is introduced to soil, the surfactant's hydrophobic (non-polar) end associates with a non-polar or hydrophobic site on the soil particles. As water penetrates through the soil matrix, water molecules are attracted to the polar end of the surfactant. The polar end of the surfactant serves as an attachment site for water molecules, increasing water adsorption and reducing evaporation rates (Fernández-Gálvez and Mingorance, 2010; Sachdev and Cameotra, 2013; Kotoky and Pandey, 2019).
Several studies tested the use of surfactant amendments to improve soil water holding capacity and diminish soil water repellency (SWR), a characteristic of soils which are hydrophilic when saturated but then become increasingly hydrophobic as they dry out (Mao et al., 2019). For example, surfactants can be applied to control the capillary evaporation process by changing the wettability of liquid in soils, reducing water in the unconfined spaces, and thereby reducing the evaporation area (He et al., 2015). Oostindie et al. (2008) reported consistently higher soil water content in sandy soil by mechanically applying a commercially available triblock co-polymer surfactant in a two-year field experiment. The surfactant applications resulted in a 4–32% increase in soil water content for the untreated soil and a 28–36% increase in soil water content for the surfactant-treated soil. The study concluded that surfactant applications have additional advantages: remediation and prevention of SWR, more homogeneous and better soil wetting, and more water available to plant rhizosphere (i.e., the soil-root interface). Lehrsch et al. (2011) were able to obtain an increase in the volumetric water content by 3% (p ≤ 0.08) at matric potentials from 0 to−20 kPa using an alkyl polyglycoside surfactant. The surfactant increased the volume of water held in pores with diameters ≥ 15 μm. However, many synthetic surfactants residues are considered hazardous to the environment and to humans and also persist in the environment because they are often non-biodegradable (Fenibo et al., 2019). With these disadvantages, it has become necessary to identify alternative approaches that are environmentally sustainable.
Surfactants of biological origin (i.e., biosurfactants) are low molecular weight, surface-active amphiphilic compounds produced by bacteria, yeast, and fungi. Although biosurfactants are used regularly in the pharmaceutical, cosmetics, and food industries (Sachdev and Cameotra, 2013), they have received less attention in sustainable agriculture. However, biosurfactants can play an essential role in keeping the soil water content above the soil moisture threshold separating the constant rate and falling rate stages (Abu-Zreig et al., 2003; Mitra et al., 2006; Hallett, 2007, 2008; Fernández-Gálvez and Mingorance, 2010). Because they are biodegradable, biocompatible, have low toxicity, and remain stable during environmental changes, they are an eco-friendly and inexpensive alternative to synthetic wetting agents (Fenibo et al., 2019; Kotoky and Pandey, 2019; Phulpoto et al., 2020). In addition, they can provide a variety of other benefits to agricultural soils by enhancing nutrient availability to plant-associated microbes or by eliminating plant pathogens and pollutants (Sachdev and Cameotra, 2013). Many microbes in the rhizosphere are known to produce biosurfactants, indicating that these molecules can play a significant role in plant health and development (Kotoky and Pandey, 2019). In semi-arid regions specifically, surfactant-producing microbes are a crucial component of soil crusts that influence soil hydrological processes including infiltration and evaporation (Belnap and Lange, 2003).
Surfactin, a secondary metabolite produced by Bacillus subtilis (Kiesewalter et al., 2020) is one of the most effective biosurfactants because it can reduce the surface tension of water from 72 to 27 mN/m with effective emulsification activity (Phulpoto et al., 2020). Surfactin has been demonstrated to improve soil wettability and soil water capacity (Sachdev and Cameotra, 2013; Phulpoto et al., 2020), has been used as a bioremediation agent (i.e., an agent to break down pollutants in the soil), and has been applied for enhanced oil recovery. For example, Lowe et al. (2019) studied the addition of B. subtilis to sandy soil to measure water soil dynamics and found that the production of Surfactin increased the soil water retention in all treatments studied. Significantly higher water content was measured in treated samples compared to control samples, with a mean of 0.33 cm3 of water per cm3 of soil and p < 0.001 for the treated samples. In another observation, the control samples remained at low water content throughout the experiment, with a final soil water content of 0.064 cm3 of water per cm3 of soil. B. subtilis also produces biofilms in soils that are primarily composed of exopolymeric substances that could further modify the soil to a hydrophilic state. Biofilms are produced in low-nutrient environments and are enhanced when Surfactin reaches a critical threshold level (Dervaux et al., 2014). These findings suggest that inoculation of B. subtilis is a promising technique to increase soil water capacity and sustainably reduce evaporation rates.
Amending soils with B. subtilis could also be a more practical approach than direct application of the wetting molecule. B. subtilis production is highly scalable through culture-based methods, and agroindustry waste products can potentially be used as growth media for onsite biosurfactant production while providing the additional environmental benefit of recovering waste (Johnson et al., 2021). Additionally, B. subtilis can be sporulated to be resistant to environmental stressors, making it shelf-stable and suitable for packaging, transport, and storage. Finally, B. subtilis may provide long-term wetting as it colonizes and establishes its niche in the soil.
The purpose of this research is to evaluate two treatments that alter the hydrologic properties of two types of soil found in Kansas. To that end, the first objective is to measure the evaporation rates of soils treated with Surfactin and B. subtilis across soil types. The second objective is to evaluate the feasibility of the treatments for each soil type by testing whether the probability of the soil being above or below the soil moisture threshold is significantly affected by the treatments using a systems dynamics model.
Samples of two different soil textures were collected for this project from the Kansas State University experimental fields in Manhattan, Kansas; site coordinates are listed in Supplementary Table S1. The chosen samples represent the distribution of soil hydraulic properties in Kansas's agricultural lands (Jaafar et al., 1978). Loam and sandy loam soil textures were chosen due to their high potential for increased returns from improved soil water repellency (Raddadi et al., 2018; Lowe et al., 2019). The soil collection occurred in one-kilogram batch sizes from 5 to 15 cm in depth and was categorized into loam and sandy loam, as listed in Supplementary Table S1. The samples were stored at 4°C before the analysis (Cernohlávková et al., 2009). Supplementary Table S2 summarizes the soil physicochemical characteristics for each texture.
The laboratory experiment was designed to evaluate the effects of two treatments on the evaporation rates and was compared to a set of control samples. The treatments are:
I. Direct application of the biosurfactant Surfactin, with no microorganisms added to agricultural soils to amend hydrologic characteristics.
II. Inoculation of B. subtilis into agricultural soils to produce the biosurfactant, Surfactin, as shown by Raddadi et al. (2018) and Lowe et al. (2019), to amend hydrologic characteristics.
III. Control: Addition of equivalent amounts of water.
The experimental treatments included the addition of Surfactin at 1.29 × 10−5 moles of Surfactin per kg of soil. For the surfactant applications, 1.667 mL of Surfactin solution at 100 ppm were added to a 2-cm-diameter beaker with a 12.5 g soil sample, with an additional 1.51 g of ultrapure water. Surfactin (CAS # 24730-31-2) was obtained from Millipore-Sigma (Merck KGaA© 2020). B. subtilis subsp. subtilis was obtained from the American Type Culture Collection (ATCC 6051) and maintained a 25% glycol solution at −80°C. In the second treatment, the inoculation of the bacteria was performed as described by Lowe et al. (2019). B. subtilis was inoculated in soil by adding 1.67 mL of a concentration containing 107cells/mL in a 2-cm-diameter beaker with 12.5 ± 0.01 g of soil sample. During the microorganism preparation, production of a spore stock suspension occurred by growing B. subtilis in tryptic soy agar at 28°C, harvesting, and stored the cells in the dark at 4°C. Spores were heat-activated (70 °C for 30 min) followed by germination in a solution of 10 mM of Tris-HCl containing 10 mM of L-alanine for two hours at 37°C. Lowe et al. (2019) described the preparation and spore's activation method. The solution was diluted to 1 × 107 cells/mL by OD600 measurements (Epoch2 Microplate Reader, Biotek). For the final inoculation, 1.67 mL of cell solution were added to the soil samples with an additional 1.51 g of ultrapure water for a final cell density of 1.33 × 106 cells/g of soil. In the last treatment, a control sample was run with 3.18 mL of ultrapure water for each texture. N = 3 independent trials were run for each set of samples.
To determine the evaporation rates under constant light and uniform heat flux, the treated soil samples were placed under a solar light simulator (Abet Technologies LS-10500), with a heat flux of 1,000 W/m2 and a 22-mm beam diameter; a 90° beam tuner was used to project the beam vertically (Figure 1). The heat flux was measured with a 2.36-cm-diameter LI-COR LI-200R pyranometer with a sensitivity of 75-μA per 1,000 W/m2. The pyranometer's output was measured with an LI-2500A light meter (LI-COR®). The experimental trials were run during a four-month period between April and July. Each sample remained under the solar simulator for 48 hours, and the change in water mass was recorded every 5 min, providing the data for calculating the evaporation rate curves. The change in mass was recorded using a digital scale FX-1200i, maximum capacity 1,200 ± 0.01 g (A&D Weighing®). The relative humidity (RH) was monitored with OM-24 Multi-Use Temperature and Humidity Data Loggers (Omega Engineering®), with a specification of ±0.5°C of temperature and ±3% of RH sensitivity.
The change in mass over time for each of the trials in every soil-treatment combination was used to estimate the evaporation rates, as well as the soil moisture threshold. The phases of evaporation were delineated using a regression approach with the recorded mass of water as the dependent variable in a single threshold model (Gonzalo and Pitarakis, 2002). The estimating equation takes the following form:
where yi, d, t is the mass of water in grams, t is the time in minutes, posti, d, t is the threshold variable indicating whether the sample is currently experiencing constant or slower rate evaporation (a value of zero for constant rate period and a value of one during the slower period of evaporation), is an interaction of the t and posti, d, tvariables, and εi, d, t is the error term for each observation. The subscripts are i∈1, 2, 3 to denote the trial, d to denote the treatment and soil combination (e.g., Surfactin applied on loam), and t to denote the time. The coefficient β0 is a constant, β1 is the coefficient on time, β2 is the coefficient for posti, d, t, and β3 is the coefficient for the interaction of time and the indicator variable, posti, d, t.
To determine the evaporation rate during the constant rate and slower rate stages, as well as the soil moisture threshold separating them, Equation 1 was estimated iteratively using Ordinary Least Squares regression for each replicate in a soil and treatment combination across all possible delineations of constant and slower rate evaporation by varying the dummy variable, posti, d, t (Wooldridge, 2010). Each iteration of the regression tested the goodness-of-fit for a different point in time when the evaporation rate changed from constant to slower by varying the time when posti, d, t changed from having a value of zero to one. The optimal value of the variable separating the constant and falling rate stages of evaporation for replicate i and soil-treatment combination d, defined as , minimized the root mean squared error (RMSE) metric of model fit (Davidson and MacKinnon, 2004). A similar procedure to select threshold values is presented in Gonzalo and Pitarakis (2002). The fitted regression lines for the optimal value of the soil moisture threshold are provided in Figure 2 for each set of replicates by soil and treatment type.
Figure 2. Observed data showing loss of water mass over time for the three replicates in each combination of soil type and treatment. The inflection points for each regression line were chosen by minimizing the RMSE, the square root of the difference between observed and predicted values, across all values of the postidt variable in Equation 1. (A,D) are the control samples for loam and sandy loam texture; (B,E) are the Surfactin treated samples for loam and sandy loam texture; (C,F) are the B. subtilis treated samples for loam and sandy loam texture.
The point in time at which replicate i for soil-treatment combination d switched from the constant rate stage to the slower rate stage of evaporation is defined as: and posti, d, t = 1. The points at which the slope of the fitted regression lines in Figure 2 change correspond with the values of the optimal switching point, . After using the density of water to convert the mass to volume in cm3, the mass of water at the soil moisture threshold, , was used to generate the critical soil moisture threshold values in Table 1. The critical soil moisture threshold for each treatment and soil type combination, d, defined as , was calculated as follows:
Table 1. Average evaporation rates during constant rate and slower rate periods, as well as the critical soil moisture threshold dividing the two periods (found by method presented in Section Estimating evaporation rates and soil moisture threshold).
where 8.1 cm3 is the soil volume. To recover the rates of mass loss for constant rate evaporation and slower rate evaporation, the partial derivate of equation one is taken with respect to time after substituting for posti, d, t :
By replacing the coefficients in Equation 3 with their corresponding estimates, and , and setting equal to zero or one, the evaporation rates for each replicate in a soil and treatment combination during the constant rate and slower rate stages can be computed. The estimated rate of mass loss during the constant rate stage in grams of water lost per 5-minute interval, , was then converted into the corresponding evaporation rate, , in millimeters of water lost per day. Similarly, the estimated rate of mass loss during the slower rate stage, , was converted into the corresponding evaporation rate, . The superscripts const and slow are used to indicate whether variables concern the constant or slower rate stages of evaporation. Within each treatment and soil combination, d, the arithmetic means of the two evaporation rates for each set of , were then computed and are reported in Table 1. Defining and as the standard errors for the estimated values of and , then the standard error for the evaporation rates across all three replicates, and for the constant and slower rate stages respectively, were calculated using the following formula and reported in Table 1:
For each value of the evaporation rate stage r, const or slow, the standard error for each respective evaporation rate stage was generated by inputting the three trials' standard errors for soil-treatment combination d and evaporation rate stage r into the appropriate terms in Equation 4.
Following treatment with Surfactin, either directly or indirectly through inoculation of B. subtilis, both the loam and sandy loam soils experienced similar changes in evaporation dynamics and soil water retention. The mass of water over time for each set of experiments is presented in Figure 2 and Table 1.
For the loam soil, treatment with Surfactin caused the soil to transition from constant rate evaporation to slower rate evaporation earlier compared to the control samples. Treatment with B. subtilis, in contrast, shifted the transition between the constant and slower rate periods of evaporation to occur at lower soil moisture contents and later in time. The second and third replicates of the B. subtilis treatment on the loam soil, depicted in Figure 2C, displayed a negative mass of water values toward the end of the time series, potentially due to additional evaporation of residual moisture present in the soil before the drying experiments began. The earlier occurrence of a transition from the constant rate to slower rate evaporation periods for the loam soil replicates treated with Surfactin, reflected in the change in slope of the mass of water curve in Figure 2B, suggests the treatment increased the rate at which water was initially evaporated from the soil. This was likely due to Surfactin's hydrophilic properties that increase the ease of readily evaporating bulk water from the soil (He et al., 2015). However, as the water starts to become scarce in the slower evaporation stage, the evaporation rates decrease due to the hydrophilic properties of the Surfactin associated with the soil, increasing the soil wettability and capillarity effects (He et al., 2015).
For the sandy loam soils, treatment with Surfactin and B. subtilis displayed a change in the slope for the mass of water curve which was not present for any of the control replicates. Compared to the control samples for the sandy loam soil (Figure 2D), treatment with Surfactin (Figure 2E) and B. subtilis, (Figure 2F) indicate greater rates of evaporation during the constant rate period followed by decreased rates of evaporation during the slower rate period. Both treatments caused the transition from constant to slower evaporation rate periods to occur at lower soil moisture content. The difference between the control and treated samples for the sandy loam soils is most apparent in the control samples' mass of water curves displayed in Figure 2D. The control samples for the sandy loam soil lack an abrupt change in slope, meaning constant rate and slower rate evaporation are difficult to differentiate. This could be because the control replicates did not dry out as quickly in comparison to the treated samples for the sandy loam soil. As such, the control replicates may not have reached the point at which the slope in the curve changed.
While other wetting mechanisms by B. subtilis were possible, wetting was likely due to production of Surfactin or other amphiphilic metabolites. Production of biosurfactants by Bacillus in soil is wellknown, particularly for remediation applications (Sachdev and Cameotra, 2013), and soil provides a spatially confined environment that facilitates quorum-sensing processes, which includes Surfactin expression (Kearns and Losick, 2003). Further, surfactants are also expressed in soil to provide enhanced motility (Sachdev and Cameotra, 2013). Finally, the observations made here are in agreement to those made by Lowe et al. (2019), where B. subtilis addition to sandy soil was used to breakdown soil water repellency and increase the soil water retention (Lowe et al., 2019).
Figure 3 shows the evaporation rate of the three experimental trials of each soil type and treatment, as well as the averaged constant rate and slower rate of evaporation estimated using the mass loss data depicted in Figure 2. The difference between the treated and the control samples is most apparent in the sandy loam soil displayed in Figures 3D–F; the loam soil, shown in Figures 3A–C, exhibits the same behavior but to a lesser degree. For either treatment, increased evaporation rates in the constant rate period and decreased evaporation rates in the slower rate period were observed in both soils compared to the control.
Figure 3. Effect of Surfactin and B. subtilis treatment on constant and slower evaporation rates by soil type. Evaporation rates were calculated by converting the mass of water lost in five-minute intervals into millimeters of water evaporated per day. Observed evaporation rates display a 90-minute moving average to smooth extreme values. (A,D) are the control samples for loam and sandy loam texture; (B,E) are the Surfactin treated samples for loam and sandy loam texture; (C,F) are the B. subtilis treated samples for loam and sandy loam texture.
As shown in Table 1, adding Surfactin to the loam soils increased the average constant evaporation rate by 15.6% and decreased the average slower evaporation rate by 24.7% compared to the control; adding B. subtilis increased the average constant evaporation rate by 12.2% and decreased the average slower evaporation rate by 43.8% compared to the control. However, B. subtilis had a 2.95% lower average constant evaporation rate and a 25.4% lower average slower evaporation rate compared to the Surfactin treated samples. For sandy loam soils, adding Surfactin increased the average constant evaporation rate by 34.0% and decreased the average slower evaporation rate by 30.3% compared to the control; adding B. subtilis increased the average constant evaporation rate by 43.0% and decreased the average slower evaporation rate by 48.6% compared to the control. However, B. subtilis had a 6.73% higher average constant evaporation rate and a 26.3% lower average slower evaporation rate compared to Surfactin.
In both the loam and sandy loam soils, the increase in the constant evaporation rates was the anticipated impact of direct (i.e., Surfactin) or indirect addition (i.e., inoculation with B. subtilis) of the amphiphilic surfactant. The loam soil experienced a greater increase in the constant rate evaporation period after the Surfactin was directly applied to the soil, while the sandy soil experienced a greater increase with B. subtilis inoculation. However, the direct and indirect application of Surfactin had a much larger effect on the sandy loam than on the loam soils. These differences in the treatment effects across soil types are also observed in studies of the effects of soil crusts on evaporation as soil crusts are known to modify soil water repellency as well (Xiao et al., 2010). A potential explanation for this is the effects of the treatments on the soil moisture threshold; they reduce the threshold soil moisture value resulting in higher evaporation rates until a lower soil moisture value, compared to the control, is reached.
Loam and sandy loam soils exhibited a decrease in slower evaporation rates for the direct and indirect treatments with Surfactin. In both soil types, soil inoculated using B. subtilis resulted in a larger decrease in the slower evaporation rate than the directly applied Surfactin. One possibility for the decrease continues from the SWR threshold explanation discussed with the constant rate of evaporation. The slower rate period will begin with a lower soil moisture value compared to the control. Given the positive relationship between evaporation and soil moisture, the reduced soil moisture values of treated samples entering the slower period of evaporation could cause the reduction in evaporation rates.
Alternatively, the biosurfactant's impact on surface tension in the soil throughout time could have played a role. This mechanism would be similar to the results found in He et al. (2015), which showed the evaporation rate can decline as the concentration of surfactant increases. In the soil samples, the surfactant would have increased in concentration as soil moisture declined. As such, the concentration of surfactant would be greater during slower periods of evaporation when soil moisture is relatively low. However, given the differences in working liquid and experimental designs between these experiments and those of He et al. (2015), this second explanation for the decreased slower period of evaporation rate is less likely.
While the increased evaporation rate during the constant rate stage may appear counterproductive to the goal of water conservation at first, it can be advantageous because of the positive relationship between surface soil moisture and evaporation lower in the soil profile (Mahrt and Pan, 1984). The increased constant evaporation rate would hasten the formation of a dry surface layer, a shallow layer of dry soil wherein evaporation is driven by water vapor diffusion rather than capillary action (Swenson and Lawrence, 2014). The faster formation of this layer induced by the treatments could quickly shield lower portions of the soil profile from the more extractive capillary-action driven evaporation processes (Yamanaka and Yonetani, 1999; Yamanaka et al., 1999). Furthermore, the decreased slower evaporation rates indicate the treatments mitigate evaporation of the remaining moisture.
Finally, the increased constant evaporation rates suggest water would easily infiltrate into the root zone. As Surfactin is produced naturally within the soil microbiome, this expectation conforms with studies of infiltration rates in soil crusts which find biological, rather than physical, soil crusts exhibit greater infiltration rates (Chamizo et al., 2012). Unlike the mechanical nature of physical soil crust formation due to the dispersion of fine soil particles, biological soil crusts are defined by symbiotic assemblages of species commonly including bacteria and fungi. As such, the hydrologic impacts of a biological soil crust are determined by the activity of the organisms involved. However, given the mixed results reported concerning the impact of soil crusts on infiltration, further experimental research is required to determine the effect of the treatments employed here on infiltration rates (Belnap, 2006).
The value of additional soil moisture during the growing season is in constant flux due to growth-stage-dependent water demands for each crop, state-dependent inflow and outflow processes, and the random nature of rainfall events. For example, the value of water changes abruptly on either side of the soil moisture threshold dividing the constant and falling rate stages of evaporation depending on the current objective. If an irrigator intends to seal soil moisture lower in the root zone, the value of additional water in the surface layer is negative because this may push the soil above the soil moisture threshold and increase evaporation. Given these complexities, rigorously evaluating whether the addition of Surfactin or the inoculation of B. subtilis is desirable in an agricultural production environment requires an approach capable of accommodating these complexities. System dynamics (SD) was developed to address this sort of complex problem where stochastic processes, non-linear equations of motion, and feedback mechanisms are present. Therefore, to test the feasibility of the Surfactin and inoculation with B. subtilis, a system dynamics model of soil moisture in the upper soil profile was created and simulated using Vensim (Eberlein and Peterson, 1992).
The purpose of this treatment would be to modify soil hydrological characteristics in the upper layers of soil to retain soil moisture lower in the soil profile. To determine how effective the treatments may be at achieving this objective, the model is structured to simulate soil moisture dynamics in the upper ten centimeters of the soil for a one hundred-day growing season. Ten centimeters was chosen because of its similarity to the 10–15 cm depth of the soil sampling in the field and the existence of prior research studying soil hydrology with a 10 cm treated soil layer (Debano, 1975). As depicted in Figure 4, it is designed to parsimoniously mimic the fundamental soil moisture balance equation using one inflow (infiltration) and one outflow (evaporation) (Rodriguez-Iturb, 2000). Built-in feedback mechanisms between the present soil moisture level and the two flows address the non-linearities created by the soil moisture threshold separating the constant rate and slower rate evaporation periods.
Figure 4. System dynamic model of soil moisture during a season. Random precipitation events occur, then the feedback from the present soil moisture level causes infiltration to cease when the soil has reached field capacity. The feedback between the present soil moisture level and the evaporation rate determines whether constant or falling rate evaporation occurs according to the soil moisture threshold.
The behavior for each combination of soil and treatment type was simulated four times beginning with completely saturated soil and using model parameters drawn from the laboratory experimental results described in Section Evaporation rates and soil moisture threshold and Supplementary Table S3. Within the simulation, the occurrence of rainfall on each day was determined by a Bernoulli trial, p = 0.38, such that the arrival of rainfall events over the course of the 100-day model run followed a Poisson process. If the outcome of the Bernoulli trial for a given day in the simulation produced a rainfall event, the intensity of the rainfall event was determined by a random draw from an exponential distribution with λ = 105.45−1 (Todorovic and Yevjevich, 1969). The probability of a rainfall event occurring and the rate parameter for the exponential distribution, λ, were calibrated using daily precipitation data during the summer months, June to August, of 2015 through 2020 from the Global Historical Climatology Network (Menne et al., 2012). The precipitation data were filtered to include 19 stations in Finney County, Kansas because of the semi-arid nature of Southwest Kansas. Four precipitation patterns were produced using 100 realizations of the stochastic rainfall process described above and were then held consistent across each of the six combinations of soil and treatment. The exact parameterization of the precipitation regime and other variables is displayed in Supplementary Table S4.
The simulated soil moisture dynamics from the system dynamics model were compared across soil types and treatments to determine the feasibility of each potential amendment. To determine each treatment's efficacy, the daily soil moisture values across the 100-day simulated season were recorded for each model run, and then the values for each soil and treatment were combined across the four runs to produce a cumulative distribution. The Wilcoxon rank-sum test, or Mann-Whitney two-sample statistic, was used to detect significant differences between the treatment and control soil moisture distributions for each soil type (Wilcoxon, 1945; Mann and Whitney, 1947). Then, to determine the efficacy of the treatment, the probability of a random draw from the treated soil's distribution being above the soil moisture threshold while a random draw from the control soil's distribution is below the threshold was computed. Both the statistical tests and the probabilities comparing treatment and control distributions were calculated using Stata Statistical Software (StataCorp®).
The distributions produced from the simulated soil moisture dynamics displayed in Figure 5 demonstrate the implications of the treatment's effects for surface soil exposed to typical precipitation patterns in southwest Kansas. For the loam soils, displayed in Figures 5A–C, neither treatment caused a significant difference in the distribution of seasonal soil moisture as shown by the Wilcoxon rank-sum test results in Table 2. The null hypotheses that the soil moisture in the control soil, θcontr, is equivalent to that of the Surfactin treated soil, θsurf, or B. subtilis treated soil, θbact, were not rejected at a significance level of α < 0.10. As such, the probabilities of the treated samples being above their respective soil moisture thresholds while the control samples are below them, 0.48 and 0.53 for the Surfactin and B. subtilis treated soils respectively, were not considered significant.
Figure 5. Histogram of daily simulated seasonal soil moisture from the system dynamics model by soil and treatment. Each treatment's density function represents simulated soil moisture values for a 100-day growing season. The Kernel density approximation indicates the expected probabilities as the number of models runs approaches infinity. Constant rate and slower rate evaporation occur above and below the soil moisture threshold for each graph respectively. The percent of simulated days spent above the soil moisture threshold are displayed at the top right of each panel. (A,D) are the control samples for loam and sandy loam texture; (B,E) are the Surfactin treated samples for loam and sandy loam texture; (C,F) are the B. subtilis treated samples for loam and sandy loam texture.
Table 2. Test results comparing the simulated effects of Surfactin and B. subtilis treatments on soil moisture from the system dynamics model.
Both treatments resulted in significant differences between the control and treated sandy loam soils as the exact p-values, or Exact Prob in Table 2, were below the 0.10 significance level. The probability of the sandy loam soil treated with Surfactin being above the soil moisture threshold, experiencing constant rate evaporation, while the control soil was below the threshold, experiencing slower rate evaporation, was estimated to be 0.54. The corresponding probability for the B. subtilis treated soil was estimated to be 0.55. This increased probability of the treatments experiencing constant rate evaporation is also evidenced in Figures 5D–F. While the increased evaporation rates during the constant rate period cause a leftward shift in sandy loam distributions in Figures 5E,F (toward drier soil moisture levels), the treatments' effect on lowering the soil moisture threshold increases the portion of the simulated growing season spent above the threshold.
By including uncertainty in evaporation rates and randomly generated rainfall data, the system dynamics model simulates whether the treatments will induce a favorable shift in soil moisture dynamics and fulfill one of the principle roles of the microbiome for sustainable agricultural production, suppressing biotic and abiotic stress (Suman et al., 2022). For the sandy loam soils, the simulated data indicate the treatments would reduce drought stress given the significantly increased probability of the soil moisture being above the threshold separating the constant and slower rate stages. While, the significant difference in soil moisture distributions for the treated and control sandy loam soils is predominantly driven by treated soils' greater constant evaporation rates, the reduction in slower evaporation rates may play a more significant role in altering dynamics when rainfall is relatively scarce. For example, in a study of the impact of drought on wheat plants inoculated two bacterial strains, Kasim et al. (2013) found the benefits of inoculation with a different microbe in the Bacillus genus, Bacillus amyloliquefaciens, increased as the drought increased in duration.
Due to this research's focus on the effects of each treatment on evaporation dynamics in the absence of other mediating factors, these results do not account for the impacts of plant-microbe or microbe-microbe interactions on plants' ability to tolerate drought stress (Barnes and Tringe, 2022). However, in isolating the impact of Surfactin and B. subtilis, these results facilitate understanding of higher-order processes in the microbiome by highlighting a mechanism by which surfactant-producing microbes affect soil moisture conditions. Additionally, unlike the ability of another Bacillus species to mitigate salinity induced abiotic stress studied by Shahzad et al. (2017), the results in this study demonstrate B. subtilis can significantly alter soil moisture conditions even if it has not colonized a plant's root system. Lastly, while the effects of the treatments on infiltration rate and maximum water holding were not tested experimentally and remained consistent for each soil type in the system dynamics model as a result, any potential changes to these parameters are unlikely to fundamentally alter these results for the surface soil layer. The implications of changes to either of these parameters are greater for soil moisture dynamics lower in the soil profile and require further investigation.
Direct and indirect addition of Surfactin significantly modified soil moisture dynamics in both sandy loam and loam soils. The constant rate of evaporation was significantly higher relative to the control samples, while the slower evaporation rate was significantly lower. The effect was most pronounced in the sandy loam soils, which demonstrated little difference between constant and slower periods of evaporation without treatment. Results from the system dynamics model demonstrate the implications of these changes over a more extended time period with realistic precipitation patterns. The increase in constant rate period of evaporation caused the soil to dry out more quickly in the treated samples despite intermittent rainfall events, and the reduced slower evaporation rate caused soil moisture to persist at lower levels for longer in the absence of precipitation.
The research findings suggest that the addition of Surfactin, either directly or indirectly through inoculation of B. subtilis, could preserve precious soil moisture available to agricultural crops in semi-arid growing regions. This study shows the addition of Surfactin affects evaporation dynamics in loam and sandy loam soils at the lab-scale. Faster formation of a dry surface layer due to the increase in constant period of evaporation could preserve precious moisture lower in the root zone, and the reduction in slower evaporation rate would mitigate evaporation after the surface layer has dried. While both treatments may be effective in reducing evaporative demand, the effects may be most pronounced in sandier soils or those characterized by coarser textures. Given the more pronounced effects of the B. subtilis treatment in experimental settings and the similar efficacy in a simulated environment, indirect treatment with this microorganism could prove equally effective, while taking advantage of the benefits of its biological origin, including its rapid scale-up through culture and due to the fact that it is highly adaptable to agricultural soils, Polonca (2020) where it can establish a niche in the soil microbiome to continuously produce Surfactin. Developing an improved understanding the soil environments that facilitate B. subtilis colonization and production of Surfactin will be important for further advancement of this approach. For example, the presence of specific genera of bacteria in the soil, such as Lysinibacillus (Kiesewalter et al., 2020), may stimulate Surfactin production from B. subtilis to facilitate wetting, while use of mineral fertilizers can have a negative impact on B. subtilis colonization (Bueno et al., 2022) and therefore would also be expected to impact its wetting potential.
Future work should investigate B. subtilis in a field trial setting, given the absence of plant-microbe and microbe-microbe interactions in this study. The impacts of inoculation with other strains from the Bacillus genus should also be tested, given its higher prevalence of strains with resistance to drought and extreme temperature stress (Kumar et al., 2014). Similarly, due to the improved water retention following inoculation of maize plants with Enterobacter sakazakii observed by Javeed et al. (2019), the effects of inoculating soils with microbes from genera other than Bacillus may also be studied in the absence plants to determine the mechanisms by which each genus alters soil moisture dynamics. Finally, the optimal concentration and application rates of B. subtilis should be investigated as these will determine the financial feasibility of using inoculation in a production agriculture setting.
The raw data supporting the conclusions of this article will be made available by the authors, without undue reservation.
MG and PC conducted the laboratory experiments. MC-H created the system dynamics model. All authors planned and designed the experiments, analyzed the data, and contributed to the manuscript.
This project was supported and funded by the NSF Research Traineeship Rural Resiliency 1828571 (primary support) and NSF Grant No. 1651451 (PC and JM support).
The authors gratefully acknowledge the support and assistance of Dr. Dorivar Ruiz Diaz and Graduate Student Diego Charbonnier Bascou from the Department of Agronomy, Kansas State University. The authors also thank Dr. Nathan Hendricks from the Department of Agricultural Economics at Kansas State University for his help and time.
The authors declare that the research was conductedin the absence of any commercial or financial relationships that could be construed as a potential conflict of interest.
All claims expressed in this article are solely those of the authors and do not necessarily represent those of their affiliated organizations, or those of the publisher, the editors and the reviewers. Any product that may be evaluated in this article, or claim that may be made by its manufacturer, is not guaranteed or endorsed by the publisher.
The Supplementary Material for this article can be found online at: https://www.frontiersin.org/articles/10.3389/fsufs.2022.959591/full#supplementary-material
Abu-Zreig, M., Rudra, R. P., and Dickinson, W. T. (2003). Effect of application of surfactants on hydraulic properties of soils. Biosyst. Eng. 84, 363–72. doi: 10.1016/S1537-5110(02)00244-1
Ale, S., Himanshu, S. K., Mauget, S. A., Hudson, D., Goebel, T. S., Liu, B. R., et al. (2021). Simulated dryland cotton yield response to selected scenario factors associated with soil health. Front. Sustain. Food Syst. 4, 307. doi: 10.3389/fsufs.2020.617509
Barnes, E. M., and Tringe, S. G. (2022). Exploring the roles of microbes in facilitating plant adaptation to climate change. Biochem. J. 479, 327–335. doi: 10.1042/BCJ20210793
Baumhardt, R. L,, Dockal, J. R., Johnson, G. L., Brauer, D. K, and Schwartz, R. C. (2020). Controlling stormwater runoff that limits water availability and dryland crop productivity. Front. Sustain. Food Syst. 4, 181. doi: 10.3389/fsufs.2020.533687
Belnap, J. (2006). The potential roles of biological soil crusts in dryland hydrologic cycles. Hydrol. Process. 20, 3159–3178. doi: 10.1002/hyp.6325
Belnap, J., and Lange, O. (2003). Biological soil crusts: structure, function, and management. Ecol. Stud. Ser. 150, 167–174. doi: 10.1007/978-3-642-56475-8
Braudeau, E, Sene, M, and Mohtar, R. H. (2005), Hydrostructural characteristics of two African tropical soils. Eur. J. Soil Sci. 56, 375–388. doi: 10.1111/j.1365-2389.2004.00679.x
Bueno, C. B., Dos Santos, R. M., de Souza Buzo, F., de Andrade da Silva, M. S. R., and Rigobelo, E. C. (2022). Effects of chemical fertilization and microbial inoculum on Bacillus subtilis colonization in soybean and maize plants. Front. Microbiol. 13, 901157. doi: 10.3389/fmicb.2022.901157
Cernohlávková, J., Jarkovský, J., Nešporová, M., and Hofman, J. (2009). Variability of soil microbial properties: effects of sampling, handling and storage. Ecotoxicol. Environ. Saf. 72, 2102–2108. doi: 10.1016/j.ecoenv.2009.04.023
Chakraborty, D., Nagarajan, S., Aggarwal, P., Gupta, V. K, Tomar, R. K, Garg, R. N., et al. (2008). Effect of mulching on soil and plant water status, and the growth and yield of wheat (Triticum Aestivum L.) in a semi-arid environment. Agric. Water Manag. 95, 1323–34. doi: 10.1016/j.agwat.2008.06.001
Chamizo, S., Cantón, Y., Lázaro, R., Solé-Benet, A., and Domingo, F. (2012). Crust composition and disturbance drive infiltration through biological soil crusts in semiarid ecosystems. Ecosystems. 15, 148–161. doi: 10.1007/s10021-011-9499-6
Davidson, R., and MacKinnon, J. G. (2004). Econometric Theory and Methods. New York, NY: Oxford University Press.
Debano, L. (1975). “Infiltration, evaporation, and water movement as related to water repellency,” in Soil Conditioners, eds W. Gardner and W. Moldenhauer. doi: 10.2136/sssaspecpub7.c15
Dervaux, J., Magniez, J. C., and Libchaber, A. (2014). On growth and form of Bacillus Subtilis biofilms. Interface Focus. 4, 20130051. doi: 10.1098/rsfs.2013.0051
Eberlein, R. L., and Peterson, D. W. (1992). Understanding models with VensimTM. Eur. J. Oper. Res. 59, 216–19. doi: 10.1016/0377-2217(92)90018-5
Evett, S. R., Colaizzi, P. D., Oshaughnessy, S. A., Lamm, F. R., Trout, T. J., and Kranz, W. L. (2014). “The future of irrigation on the Us great plains,” in Proceedings of the 26th Annual Central Plains Irrigation Conference, 2–25. Available online at: https://www.ars.usda.gov/research/publications/publication/?seqNo115=302456 (accessed September 23, 2022).
Fang, Q. X., Ma, L., Nielsen, D. C., Trout, T. J., and Ahuja, L. R. (2015). Quantifying corn yield and water use efficiency under growth stage-based deficit irrigation conditions. Front. Plant Sci. 5, 1–24. doi: 10.2134/advagricsystmodel5.c1
Feng, W., Lindner, H., Robbins, N. E., and Dinneny, J. R. (2016). Growing out of stress: The role of cell- and organ-scale growth control in plant water-stress responses. Plant Cell. 28, 1769–1782. doi: 10.1105/tpc.16.00182
Fenibo, E. O., Ijoma, G. N, Selvarajan, R., and Chikere, C. B. (2019). Microbial surfactants: the next generation multifunctional biomolecules for applications in the petroleum industry and its associated environmental remediation. Microorganisms 7, 581. doi: 10.3390/microorganisms7110581
Fernández-Gálvez, J., and Mingorance, M. D. (2010). Vapour and liquid hydrophobic characteristics induced by presence of surfactants in an agricultural soil. Geoderma 154, 321–27. doi: 10.1016/j.geoderma.2009.11.002
Finley, J. W., and Seiber, J. N. (2014). The nexus of food, energy, and water. J. Agric. Food Chem. 62, 6255–6262. doi: 10.1021/jf501496r
Geerts, S., and Raes, D. (2009). Deficit irrigation as an on-farm strategy to maximize crop water productivity in dry areas. Agric. Water Manag. 96, 1275–1284. doi: 10.1016/j.agwat.2009.04.009
Gilson, P., Zollinger, B., Aistrup, J. A., and Heinrichs, J. (2001). The Value of Ogallala Aquifer Water in Southwest Kansas. Fort Hays State University, the Docking Institute of Public Affairs, Center. Available online at: http://www.gmd3.org/pdf/ogal1.pdf
Gleeson, T., Wada, Y., Bierkens, M. F. P., and van Beek, L. P. H. (2012). Water balance of global aquifers revealed by groundwater footprint. Nature. 488, 197–200. doi: 10.1038/nature11295
Gonzalo, J., and Pitarakis, J. Y. (2002). Estimation and model selection based inference in single and multiple threshold models. J. Econ. 110, 319–52. doi: 10.1016/S0304-4076(02)00098-2
Hallett, P. D. (2007). “An introduction to soil water repellency,” in Proceedings of 8th International Symposiym Adjuvants for Agrochemicals, ed R. E. Gaskin (Christchurch, New Zealand: Hand Multimedia).
Hallett, P. D. (2008). A brief overview of the causes, impacts and amelioration of soil water repellency—a review. Soil Water Res. 3, S21–29. doi: 10.17221/1198-SWR
He, F., Wang, Z., Wang, L., Li, J., and Wang, J. (2015). Effects of surfactant on capillary evaporation process with thick films. Int. J. Heat Mass Transf. 88, 406–10. doi: 10.1016/j.ijheatmasstransfer.2015.04.084
Hendricks, N. P. (2018). Potential benefits from innovations to reduce heat and water stress in agriculture. J. Assoc. Environ. Resourc. Econ. 5, 545–76. doi: 10.1086/697305
Jaafar, M. N., Kanemasu, E. T., and Powers, W. L. (1978). Estimating soil factors for nine Kansas soils used in an evapotranspiration model. Trans. Kans. Acad. Sci. 81, 57–63. doi: 10.2307/3627357
Jägermeyr, J. (2020). Agriculture's historic twin-challenge toward sustainable water use and food supply for all. Front. Sustain. Food Syst. 4, 35. doi: 10.3389/fsufs.2020.00035
Javeed, H. M. R., Qamar, R., Rehman, A. U., Ali, M., Rehman, A., Farooq, M., et al. (2019). Improvement in soil characteristics of sandy loam soil and grain quality of spring maize by using phosphorus solublizing bacteria. Sustainability 11, 7049. doi: 10.3390/su11247049
Johnson, P., Trybala, A., Starov, V., and Pinfield, V. J. (2021). Effect of synthetic surfactants on the environment and the potential for substitution by biosurfactants. Adv. Colloid Interface Sci. 288, 102340. doi: 10.1016/j.cis.2020.102340
Kansas Department of Agriculture (2019). Water Use Data Collection and Fact Sheet FACT SHEET Water Use Data Collection and Use. Available online at: https://agriculture.ks.gov (accessed September 23, 2022).
Kasim, W. A., Osman, M. E., Omar, M. N., et al. (2013). Control of drought stress in wheat using plant-growth-promoting bacteria. J. Plant Growth Regul. 32, 122–130. doi: 10.1007/s00344-012-9283-7
Kearns, D. B., and Losick, R. (2003). Swarming motility in undomesticated Bacillus subtilis. Mol Microbiol. 49, 581–590. doi: 10.1046/j.1365-2958.2003.03584.x
Kiesewalter, H. T., Lozano-Andrade, C. N., Strube, M. L., and Kovács, Á. T. (2020). Secondary metabolites of Bacillus Subtilis impact the assembly of soil-derived semisynthetic bacterial communities. Beilstein J. Org. Chem.. 16, 2983–98. doi: 10.3762/bjoc.16.248
Kotoky, R., and Pandey, P. (2019). Rhizosphere mediated biodegradation of Benzo(A)Pyrene by surfactin producing soil bacilli applied through melia azedarach rhizosphere. Int. J. Phytoremed. 22, 363–372. doi: 10.1080/15226514.2019.1663486
Kumar, G. P., Ahmed, S. K. M. H., Desai, S., Amalraj, E. L. D., and Rasul, A. (2014). In vitro screening for abiotic stress tolerance in potent biocontrol and plant growth promoting strains of pseudomonas and Bacillus spp. Int. J. Bacteriol. 2014, 195946. doi: 10.1155/2014/195946
Lehmann, P., Assouline, S., and Or, D. (2008). Characteristic lengths affecting evaporative drying of porous media. Phys. Rev. E. 77, 056309. doi: 10.1103/PhysRevE.77.056309
Lehrsch, G. A., Sojka, R. E., Reed, J. L., Henderson, R. A., and Kostka, S. J. (2011). Surfactant and irrigation effects on wettable soils: runoff, erosion, and water retention responses. Hydrol. Process. 25, 766–77. doi: 10.1002/hyp.7866
Liao, Y., Cao, H. X., Liu, X., Li, H. T., Hu, Q. Y., and Xue, W. K. (2021). By increasing infiltration and reducing evaporation, mulching can improve the soil water environment and apple yield of orchards in semiarid areas. Agric. Water Manag. 253, 106936. doi: 10.1016/j.agwat.2021.106936
Lowe, M. A., Mathes, F, Loke, M. H., McGrath, G., Murphy, D. v., and Leopold, M. (2019). Bacillus Subtilis and surfactant amendments for the breakdown of soil water repellency in a sandy soil. Geoderma 344, 108–18. doi: 10.1016/j.geoderma.2019.02.038
Mahrt, L., and Pan, H. (1984). A two-layer model of soil hydrology. Boundary-Layer Meteorol. 29, 1–20. doi: 10.1007/BF00119116
Mann, H. B., and Whitney, D. R. (1947). On a test of whether one of two random variables is stochastically larger than the other. Ann. Math. Stat. 18, 50–60. doi: 10.1214/aoms/1177730491
Mao, J., Nierop, K. G. J., Dekker, S. C., Dekker, L., and Chen, B. (2019). Understanding the mechanisms of soil water repellency from nanoscale to ecosystem scale: a review. J. Soils Sediments. 19, 171–185. doi: 10.1007/s11368-018-2195-9
Matveeva, N. v., Milanovsky, E. Y., and Rogova, O. B. (2019). The method of preparing soil samples for soil-water contact angle measurement using sessile-drop technique. Dokuchaev Soil Bull. Number 97, 91–112. doi: 10.19047/0136-1694-2019-97-91-112
Menne, M. J., Durre, I., Vose, R. S., Gleason, B. E., and Houston, T. G. (2012). An overview of the global historical climatology network-daily database. J. Atmos. Ocean. Technol. 29, 897–910. doi: 10.1175/JTECH-D-11-00103.1
Mitra, S., Vis, E., Kumar, R., Plumb, R., and Fam, M. (2006). Wetting agent and cultural practices increase infiltration and reduce runoff losses of irrigation water. Biologia 61, S353–57. doi: 10.2478/s11756-006-0188-4
Oostindie, K., Dekker, L. W., Wesseling, J. G., and Ritsema, C. J. (2008). Soil surfactant stops water repellency and preferential flow paths. Soil Use Manag. 24, 409–15. doi: 10.1111/j.1475-2743.2008.00185.x
Or, D., Lehmann, P., Shahraeeni, E., and Shokri, N. (2013). Advances in soil evaporation physics—a review. Vadose Zone J. 12, 1–16. doi: 10.2136/vzj2012.0163
Pérez-Blanco, C. D., Hrast-Essenfelder, A., and Perry, C. (2020). Irrigation technology and water conservation: a review of the theory and evidence. Rev. Environ. Econ. Policy. 14, 216–39. doi: 10.1093/reep/reaa004
Phulpoto, I. A., Yu, Z., Hu, B., Wang, Y., Ndayisenga, F., Li, J., et al. (2020). Production and characterization of surfactin-like biosurfactant produced by novel strain Bacillus Nealsonii S2MT and it's potential for oil contaminated soil remediation. Microbial. Cell Factories 19, 1–12. doi: 10.1186/s12934-020-01402-4
Polonca, S. (2020). Environment shapes the intra-species diversity of Bacillus subtilis Isolates. Microb. Ecol. 79, 853–864. doi: 10.1007/s00248-019-01455-y
Raddadi, N., Giacomucci, L., Marasco, R., Daffonchio, D., Cherif, A., and Fava, F. (2018). Bacterial polyextremotolerant bioemulsifiers from arid soils improve water retention capacity and humidity uptake in sandy soil. Microbial. Cell Factories 17, 1–12. doi: 10.1186/s12934-018-0934-7
Rodriguez-Iturb, I. (2000). Ecohydrology: a hydrologic perspective of climate-soil-vegetation dynamies. Water Resourc. Res. 36, 3–9. doi: 10.1029/1999WR900210
Rogers, D. H., and Lamm, F. R. (2012). “Kansas irrigation trends,” in Proceedings of the 24th Annual Central Plains Irrigation Conference (Colby, Kansas). Available online at: https://www.ksre.k-state.edu/irrigate/oow/p12/Rogers12Trends.pdf (accessed September 23, 2022).
Sachdev, D. P., and Cameotra, S. S. (2013). Biosurfactants in agriculture. Appl. Microbiol. Biotechnol. 97, 1005–1016. doi: 10.1007/s00253-012-4641-8
Shahzad, R., Khan, A. L., Bilal, S., Waqas, M., Kang, S.-M., and Lee, I.-J. (2017). Inoculation of abscisic acid-producing endophytic bacteria enhances salinity stress tolerance in Oryza sativa. Environ. Exper. Bot. 136, 68–77. doi: 10.1016/j.envexpbot.2017.01.010
Shokri, N., Lehmann, P., and Or, D. (2008). Effects of hydrophobic layers on evaporation from porous media. Geophys. Res. Lett. 35, 1–4. doi: 10.1029/2008GL035230
Shokri, N., Lehmann, P., and Or, D. (2009a). Critical evaluation of enhancement factors for vapor transport through unsaturated porous media. Water Resourc. Res. 45, W02415. doi: 10.1029/2009WR007769
Shokri, N., Lehmann, P., and Or, D. (2010). Evaporation from layered porous media. J. Geophys. Res. Solid Earth. 115, B06.204. doi: 10.1029/2009JB006743
Shokri, N., Lehmann, P., and Or, D. (2009b). Characteristics of evaporation from partially wettable porous media. Water Resourc. Res. 45, 1–12. doi: 10.1029/2008WR007185
Shokri, N., and Or, D. (2011). What determines drying rates at the onset of diffusion controlled stage-2 evaporation from porous media? Water Resourc. Res. 47, 1–8. doi: 10.1029/2010WR010284
Siebert, S., Burke, J., Faures, J. M., Frenken, K., Hoogeveen, J., Döll, P., et al. (2010). Groundwater use for irrigation—a global inventory. Hydrol. Earth Syst. Sci. 14, 1863–1880. doi: 10.5194/hess-14-1863-2010
Suman, J., Amitava, R., Devika, O. S., Sonam, S., Chinmay, G., and Chandrakala, J. (2022). Microbiome as a key player in sustainable agriculture and human health. Front. Soil Sci. 2. doi: 10.3389/fsoil.2022.821589
Swenson, S. C., and Lawrence, D. M. (2014). Assessing a dry surface layer-based soil resistance parameterization for the community land model using GRACE and FLUXNET-MTE Data. J. Geophys. Res. 119, 10,299–10,312. doi: 10.1002/2014JD022314
Todorovic, P., and Yevjevich, V. (1969). Stochastic process of precipitation. Hydrol. Pap. 35. Colorado State University, Fort Collins.
Vora, N., Fath, B. D., and Khanna, V. (2019). A systems approach to assess trade dependencies in U.S. Food-energy-water nexus. Environ. Sci. Technol. 53, 10941–10950. doi: 10.1021/acs.est.8b07288
Wilcoxon, F. (1945). Individual comparisons by ranking methods. Biometrics Bulletin. 1, 80–83. doi: 10.2307/3001968
Wooldridge, J. M. (2010). Econometric Analysis of Cross Section and Panel Data, 2nd edn. Vol. 1. Cambridge, MA: The MIT Press.
Xiao, B., Zhao, Y.-G., and Shao, M.-A. (2010). Characteristics and numeric simulation of soil evaporation in biological soil crusts. J. Arid Environ. 74, 121–130. doi: 10.1016/j.jaridenv.2009.06.013
Yamanaka, T., Takeda, A., and Shimada, J. (1999). Evaporation beneath the soil surface: Some observational evidence and numerical experiments. Hydrol. Process. 12, 2193–2203. doi: 10.1002/(SICI)1099-1085(19981030)12:13/14<2193::AID-HYP729>3.0.CO;2-P
Yamanaka, T., and Yonetani, T. (1999). Dynamics of the evaporation zone in dry sandy soils. J. Hydrol. 217, 135–148. doi: 10.1016/S0022-1694(99)00021-9
Zhou, Y., Gholizadeh, H., LaVanchy, G. T., and Hasan, E. (2020). Inspecting the food-water nexus in the ogallala aquifer region using satellite remote sensing time series. Remote Sens. 12, 2257. doi: 10.3390/rs12142257
Zribi, W., Aragüés, R., Medina, E., and Faci, J. M. (2015). Efficiency of inorganic and organic mulching materials for soil evaporation control. Soil Tillage Res. 148, 40–45. doi: 10.1016/j.still.2014.12.003
SWR, Soil Water Repellency.
y, Mass of Water in grams.
β0, β1, β2, and β3, Coefficients for regression constant, time, indicator variable for constant or slower rate evaporation, and interaction term between time and indicator variable.
t, Time in minutes. post, Binary dummy variable with value equal to 0 during constant rate evaporation and 1 during slower rate evaporation.
e, Error variance for each observation.
, Evaporation rate during constant or slower rate stages, respectively, in millimeters per day. , Standard error for evaporation rate during constant or slower rate stages, respectively.
, Critical soil moisture threshold at which evaporation changes from constant rate to slower rate stage for each treatment and soil type combination expressed as the water volume (cm3) per total volume of soil (cm3).
i, Trial number.
d, Treatment and soil combination.
t, Time.
, The accent, , indicates the estimated value of the corresponding variable.
Keywords: Bacillus subtilis, biosurfactant, water retention, soil moisture, system dynamic model, irrigation, water resources, sustainability
Citation: Gutierrez MM, Cameron-Harp MV, Chakraborty PP, Stallbaumer-Cyr EM, Morrow JA, Hansen RR and Derby MM (2022) Investigating a microbial approach to water conservation: Effects of Bacillus subtilis and Surfactin on evaporation dynamics in loam and sandy loam soils. Front. Sustain. Food Syst. 6:959591. doi: 10.3389/fsufs.2022.959591
Received: 01 June 2022; Accepted: 21 September 2022;
Published: 17 October 2022.
Edited by:
Bruno José Rodrigues Alves, Brazilian Agricultural Research Corporation (EMBRAPA), BrazilReviewed by:
Arvind Kumar Rai, Indian Council of Agricultural Research (ICAR), IndiaCopyright © 2022 Gutierrez, Cameron-Harp, Chakraborty, Stallbaumer-Cyr, Morrow, Hansen and Derby. This is an open-access article distributed under the terms of the Creative Commons Attribution License (CC BY). The use, distribution or reproduction in other forums is permitted, provided the original author(s) and the copyright owner(s) are credited and that the original publication in this journal is cited, in accordance with accepted academic practice. No use, distribution or reproduction is permitted which does not comply with these terms.
*Correspondence: Melanie M. Derby, ZGVyYnltQGtzdS5lZHU=
Disclaimer: All claims expressed in this article are solely those of the authors and do not necessarily represent those of their affiliated organizations, or those of the publisher, the editors and the reviewers. Any product that may be evaluated in this article or claim that may be made by its manufacturer is not guaranteed or endorsed by the publisher.
Research integrity at Frontiers
Learn more about the work of our research integrity team to safeguard the quality of each article we publish.