- 1Food Science and Nutrition Program, Department of Chemistry, Carleton University, Ottawa, ON, Canada
- 2Department of Physical and Environmental Sciences University of Toronto Scarborough, Toronto, ON, Canada
- 3Institute of Biochemistry, Carleton University, Ottawa, ON, Canada
The aim of this study was to assess the effect of inoculated endophytic bacteria on the concentrations of vitamins E (tocopherols), K (phylloquinone), B1 (thiamine), B2 (riboflavin), C (ascorbic acid) and the peroxyl radical scavenging capacity of hydroponically grown sweet basil. Endophytic strains were all isolated from hydrocarbon-stressed herbaceous plants or from basil showing superior growth. Plants inoculated with the endophytes displayed up to 40% increase (p < 0.05) in the concentration of the reduced form of vitamin C relative to control [0.56 mg/g fresh weight (FW)] which indicated less oxidative stress in the presence of endophytes. In the case of γ-tocopherol, the highest content [25.8 μg/g of fresh weight (FW)] in inoculated basils was significantly higher compared to control plants (18.5 ± 1.2 μg/g FW) (p < 0.05). Antioxidant activity (ROO∙ radicals scavenging) was as high as 94 ± 4 μM Trolox equivalents (TE)/g FW vs. 53 ± 5 μM TE/g FW for the control basil. Concentrations of vitamins C, B1, and B2 were not affected by most strains. The results showed that endophytic bacteria have the capacity to alter free radical quenching capacity and vitamin concentrations in basil plants and, that their effect is strain and nutrient dependent.
Introduction
Sweet basil (Ocimum basilicum L.) is an annual aromatic herb native to India and Asia that is today cultivated in many parts of the world (Makri and Kintzios, 2008; Falowo et al., 2019; Skrypnik et al., 2019). Although basil has ornamental and therapeutic uses (Kaurinovic et al., 2011), worldwide, it is mostly used as culinary herb (Lin et al., 2021). Basils add flavor to foods, and also provide minerals (calcium, iron, potassium, magnesium, sodium), and vitamins (e.g., A, C, E, and K) (Ghasemzadeh et al., 2016). The presence of secondary metabolites like carotenoids and polyphenols can reduce oxidative damage in plants, processed foods, or in the human body after consumption (Skrypnik et al., 2019). The amount of nutrients and phytochemicals in crops, in general vary based on factors such as environmental conditions, genotype, and maturity (Paradiković et al., 2011; Ivanović et al., 2019). Sweet basil is generally grown in open fields and in greenhouses where nutrients are provided by the soil environment (Skrypnik et al., 2019). However, soil-less cultivation in hydroponic and aquaponic systems is popular for sustainability purposes, reproducibility, efficient use of water, and year-round availability of fresh vegetables (Sgherri et al., 2010; Neocleous and Nikolaou, 2019). Soil-free techniques are usually set up in vertical integration systems often in indoor urban locations, addressing the limitation of available space and soil quality (Saha et al., 2016). There are two main types of soil-less cultivation, hydroponic where the plant nutrients are dissolved in a water solution, and aquaponic in which waste water from fish culture is used to grow herbaceous crops (Yang and Kim, 2020). These soil-less techniques lack endophytic bacteria also known as plant growth-promoting bacteria (PGPB) (Paradiso et al., 2017). The use of endophytic bacteria in the soil-less systems may alter the nutritional balance and phytochemical contents of the growing plants. For instance inoculation of soil-grown strawberry with strains of bacteria Bacillus and Paraburkholderia improved the growth, and content of antioxidant molecules (anthocyanins, carotenoids) in fruits (Rahman et al., 2018), while there was an increase synthesis of vitamin B6 in tomato plants inoculated with Bacillus subtilis (Chandrasekaran et al., 2019).
Endophytic microorganisms can impart several benefits to the host plants ranging from growth, biocontrol, and nutrient accumulation to bioremediation. Strains of the bacterial endophyte Azospirillum brasilense showed depending on the inoculating conditions several beneficial effects. These include enhanced yields, root and shoot growth, or greater formation of indole-3-acetic acid in seedlings of cucumber, tomato, and lettuce (Terry et al., 2000; Mangmang et al., 2015). An increase resistance to diseases was also reported in tomato plants (Fujita et al., 2017). Other bacterial endophytes belonging to Agrobacterium, Pseudomonas, Enterobacter, and Rhizobium species increased the growth and production of indole-3-acetic acid in potato plants (Naqqash et al., 2016). In lettuce, the fungus Beauveria bassiana had an endophytic role by promoting resistance to pathogens and by inducing an increase in micro-nutrient (zinc, iron, manganese) tissue contents (Macuphe et al., 2021) while Piriformospora indica enhanced by 7.6-fold the concentration of zinc (Padash et al., 2016). In a previous work, we reported the promotion of root and shoot growth of aquaponically grown basil, lettuce, and bok choy plants due to inoculation with bacteria endophytes (Mayer et al., 2019). The main objective of this study was then to determine if fat- and water-soluble vitamins in basil could also be affected by some of those endophytes. A secondary objective was to determine the peroxyl radical scavenging activity of extracts and correlate that to the concentrations of antioxidant vitamins.
Materials, methods, and instruments
Chemicals and reagents
The standards α-tocopherol, ∂-tocopherol, γ-tocopherol, phylloquinone (K1), thiamine hydrochloride, and riboflavin were obtained from Sigma Aldrich (Oakville, ON, Canada). L-ascorbic acid, butylated hydroxytoluene (BHT), hexane, zinc chloride, sodium acetate, Taka-Diastase from Aspergillus oryzae, trichloroacetic acid, potassium ferrocyanide (III), 1,4-Dithiothreitol (DTT), N-Ethylmaleimide (NEM), phosphoric acid, α-α1-bipyridil, iron (III) chloride, 2,2′-azobis(2-methylpropionamidine) dihydrochloride (AAPH), 6-hydroxy-2,5,7,8- tetramethylchroman-2-carboxylic acid (Trolox), and rutin trihydrate, were also from Sigma Aldrich (Oakville, ON, Canada). Fluorescein was obtained from Fisher Scientific Co. (Nepean, ON, Canada). Acetone, methanol, potassium phosphate monobasic, acetic acid, 2-propanol, chloroform, hydrochloric acid, and potassium phosphate dibasic were obtained from VWR Canada, (Montreal, QC). High-purity water was produced in the laboratory by an Alpha-Q system (Millipore, Marlborough, MA, USA). The high-performance liquid chromatographic (HPLC) system used for quantification of vitamins was a Waters Breeze™ 2 model that included a 1,525 Binary pump, 2,707 autosampler maintained at 8°C, 2,475 Fluorescence Detector, and Empower™ version 3 software from Waters Corporation (Mississauga, ON, Canada).
Microbial strains, plant inoculation, and growing conditions
Microbial strains
Most of the bacterial endophytes tested in this work; strains A (Plantibacter flavus), B (Plantibacter flavus), C (Curtobacterium flaccumfaciens), D (Methylobacterium oryzae), E (Sinorhizobium medicae), G (Rizhobium selenireductens), and H (Curtobacterium herbarum) were isolated from herbaceous plants growing in contaminated soils (Lumactud et al., 2016). Strains A, B, G and H were found to promote root and aerial biomass of basil and other hydroponically grown plants (Mayer et al., 2019). Stains I (Bacillus toyonensis), and J (Bacillus fluminis) were isolated from basil plants showing superior root and shoot growth in hydroponic systems. Strains C and D had beneficial effects on the growth of Arabidopsis and were included to see if they may also have an effect on basils (Mayer et al., 2019). Strains A and B were combined as a consortium treatment because they show different plant growth promoting effects and genomic structure as detailed in Mayer et al. (2019). In all cases, species identifications were derived from the sequence of 16S rRNA gene fragments amplified from DNA extracts as described in Lumactud et al. (2016).
Plant inoculation
Basil seeds were germinated in coconut-fiber plugs (Rapid Rooter sponge plugs, from International Horticultural Technologies, LLC, Hollister, CA, USA), one seed per plug. Plugs were exposed to UV light for 40 min and incubated at 60°C for 3 days before use. Plugs with germinated seeds were placed in sterile (autoclavable) trays, containing 12 plugs/ seedling per each treatment (Figure 1) and distilled water. On day five, the inoculation of seedlings with microbial strains was performed. For that, each strain was grown in tryptic soy broth culture for 24 h under 27°C, at 140 RPM. The number of cells for each microbial strain was standardized by cell counting and by spectrophotometer. The broth was centrifuged for 5 min at 5,000 RPM, cells were washed with phosphate buffer solution (PBS buffer) and centrifuged again. The pellet was resuspended in PBS buffer and an aliquot (200 μL) of liquid containing ~103 cells were added over each seedling. The control plants were not inoculated with bacteria, but they were provided with 200 μL of sterile PBS (the same volume of PBS that was used to suspend the bacterial pellet after centrifugation). The procedure was repeated for each treatment. After 10 days, the plugs were transferred to the aquaponic system. On days 15th, 35th, and 45th, macro and micronutrients were applied in the tanks according to the manufacturing protocol. Biocontrol of aphids was effectively performed with ladybugs. After 20 days, basil leaves and stems were collected and stored at −80°C for further analysis.
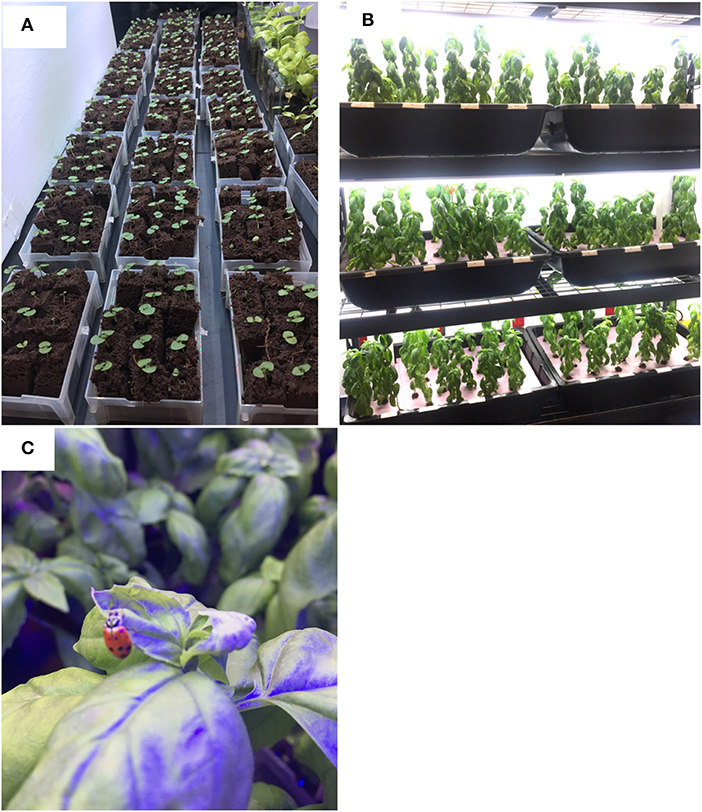
Figure 1. (A) Basil seedlings after 5 days of germination, in sterile growth sponges; (B) Adult basil plants, in the hydroponics system at the University of Toronto; (C) Lady bug (Coccinellidae) on basil leaves, applied on the plants for biological control of aphids.
Determination of tocopherols
The extraction of tocopherols was based on a previous literature report (Knecht et al., 2015). Frozen basil leaves were first ground in a mortar. Then 500 mg were transferred to a tube and homogenized with a homogenizer (Model OM 02403, Fisher Scientific Co.), mixed with 0.5 mL of aqueous ascorbic acid solution (11%w/v) and 1 mL of acetone 0.025% BHT to prevent oxidation. The mixture was placed for 2 min in an ultrasonic bath (Model FS30, Fisher Scientific Co.), vortex (1 min), and centrifuged 2,100g, 2 min, 6°C (Legend Micro 21R Centrifuge, Thermo Fisher Scientific). The acetone upper layer was collected and transferred to a separate test tube, the extraction was repeated two more times. Supernatants were combined and dried under nitrogen stream gas at 40°C. The dried extract was reconstituted using 500 μL of methanol/acetone/water mixture (54:40:6 v/v), filtered through 0.45 μm nylon membrane (Dikmatech, Canada) for HPLC quantification of α- and γ-tocopherols which were the two forms of vitamin E in the extracts.
The separation of tocopherols was performed using ACME™ Amide C18, 3 μm, 120Å, 100 × 4.6 mm column (Canadian Life Science, Peterborough, ON, Canada) for 30 min at a flow rate of 1 mL/min with an isocratic solvent mixture (93:7, A: B). Solvent A was 0.1% of acetic acid in water while solvent B was 100% methanol. The sample injection volume was 20 μL. Both α- and γ-tocopherols were detected using a fluorescence detector (excitation 295 nm, emission 330 nm) and using external standards constructed with five concentrations between 2.5 and 20 μg/mL for α-tocopherol and γ-tocopherol. Samples chromatograms are provided in Figures 2A,B.
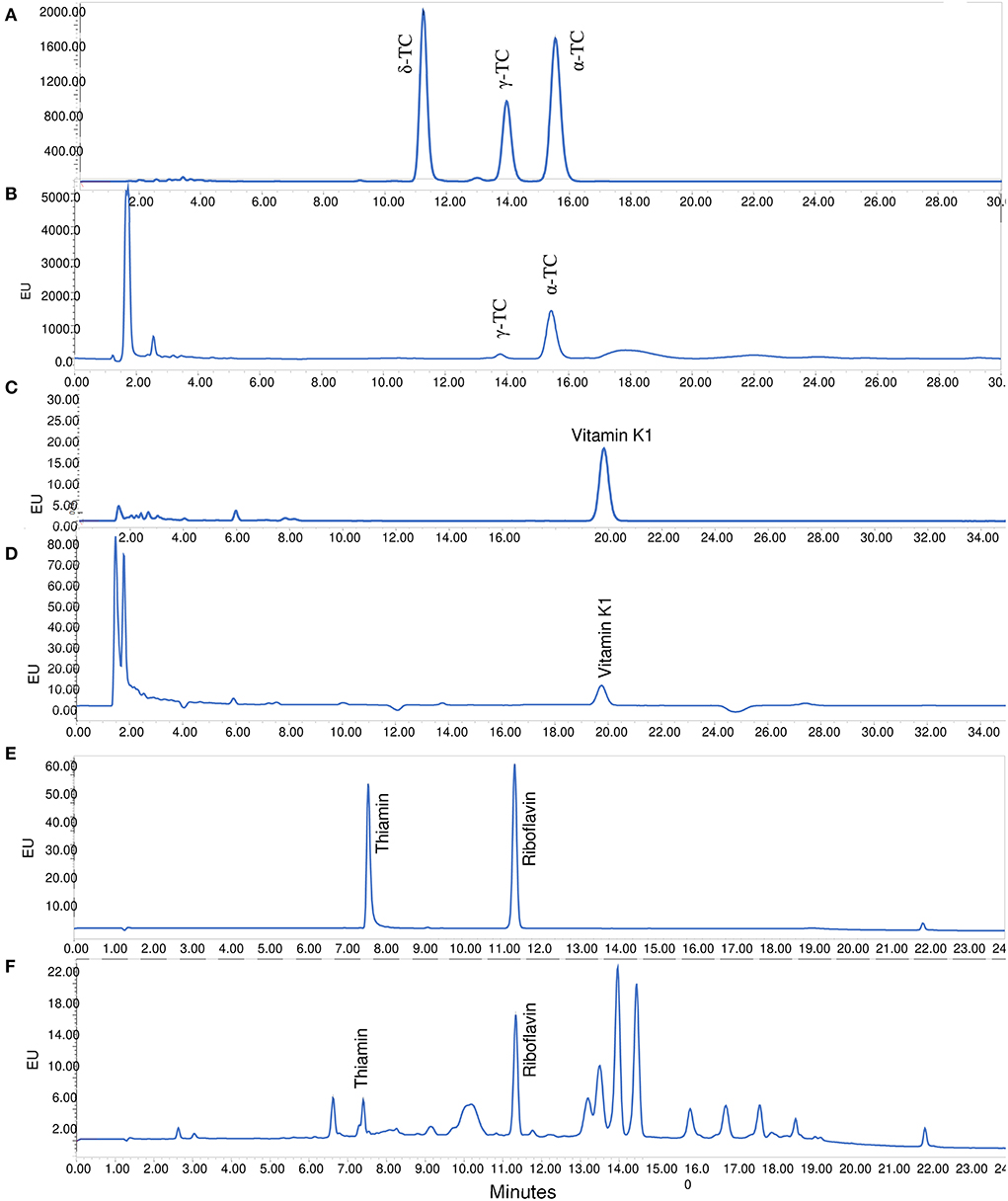
Figure 2. Samples HPLC chromatograms. (A) Standards mixture of vitamin E or tocopherols; (B) tocopherols in basil; (C) standard vitamin K1 or phylloquinone; (D) phylloquinone in a basil; (E) Standards mixture of thiamin (B1) and riboflavin (B2); (F) B1 and B2 in a basil plant.
Determination of phylloquinone (vitamin K1)
The extraction was adapted from previous work (Koivu et al., 1997). To mortar crushed basil leaves (500 mg), 4.5 mL of 2-propanol were added and homogenized with the tissue mixer followed by sonication for 5 min in the ultrasonic bath. Extraction was continued by the addition of 4 mL of hexane and 2 mL of water, then vortex and centrifuged 1,500 g (5 min). The hexane's upper layer was removed, dried under nitrogen stream, reconstituted with 500 μL of chloroform: methanol (1:4), and filtered for analysis on HPLC (Vardavas et al., 2006).
The reconstituted hexane extract was separated on ACME™ Amide C18, 3 μm, 120 Å, 100 × 4.6 mm column. The injection volume was 20 μL, while the eluent was composed of solvent A (1% of acetic acid in water) and solvent B (100% Methanol containing (10 mM zinc chloride, 5 mM sodium acetate, 5 mM acetic acid). The linear gradient was 95% to 5% solvent A in 30 min. Fluorescence detection of phylloquinone was achieved with excitation and emission wavelengths of 243 and 430 nm, respectively. A calibration curve constructed with five concentrations of the standard (10–50 μg/mL) was used to quantify the amount of phylloquinone in each extract. The use of zinc chloride is to convert phylloquinone (K1) to its fluorescent hydroquinone derivative (K1H2). Sample chromatograms are provided in Figures 2C,D.
Determination of thiamine (B1) and riboflavin (B2)
Thiamine and riboflavin quantification was based on previous work (Esteve et al., 2001). The analysis was accomplished by homogenizing 500 mg of crushed leaves in 5 mL of 0.1 M HCl followed by heating at 100°C for 5 min to hydrolyze proteins and free the vitamins. In the next step, solutions were cooled in water followed by the pH adjustment to 4.5, the addition of 1.25 mL of takadiastase (10% w/v), and incubation for 3 h at 50°C was performed to cleave both thiamine phosphate esters and flavoproteins. To end the process, the mixture was heated at 100°C for 5 min. To obtain the extracts, 0.5 mL of 50% (w/v) trichloroacetic acid was added and centrifuged to precipitate and remove the proteins. Supernatants were collected as extracts and analyzed the same day. Prior to the analysis, 1 mL of each extract was mixed with 75 μL of potassium ferrocyanate in 15% of NaOH, vortex, and let stand for 10 min to allow the oxidation of thiamin to thiochrome. Authentic standard mixtures of thiamine and riboflavin were oxidized the same way.
The oxidized extracts and standard mixtures were filtered through 0.45 μm nylon membrane and injected (20 μL) into HPLC. The separation was performed on Waters Atlantis dC18™ 100 Å, 5 μm, 4.6 × 150 mm column (Waters Corp., Mississauga, ON, Canada). The solvents 0.1% of acetic acid in water (solvent A) and 100% acetonitrile (solvent B). The flow rate was 1.4 mL/min while the linear gradient conditions were as followed: 0–4 min 100% A, 4–6 min 97% A, 6–15 min 85% A, 15–20 min 80% A, 20–30 min 20% A. An equilibration time of 10 min was allowed between injections. Fluorescence detection of both vitamins was achieved at excitation and emission wavelengths of 422 and 515 nm, respectively. The quantification was achieved using calibration curves made with mixtures of standard thiamine (0.2–3 μg/mL) and riboflavin (0.015–0.3 μg/mL). Standard curves were used to determine vitamins concentrations in the extracts. Samples chromatograms are provided in Figures 2E,F.
Determination of ascorbic acid
Vitamin C in the form of total ascorbate, oxidized and reduced ascorbates were determined spectrophotometrically based on literature reports (Hodges et al., 2001; Gillespie and Ainsworth, 2007). In brief, 500 mg of crushed basil leaves were homogenized with 2 mL of freshly prepared 6% TCA (trichloroacetic acid) and centrifuged at 13,000 g for 5 min at 4°C. Total ascorbate was determined by incubating (25°C, 10 min) a mixture of 200 μL of either supernatant (extract), or standard. L-ascorbic acid (0–1 mM) or 6% TCA (blank), 100 μL of 75 mM KH2PO4 buffer, and 100 μL of 10 mM DTT. The excess DTT was removed with the addition of 100 μL of 0.5% (w/v) N-ethylmaleimide (NEM). For the determination of reduced ascorbate, the process was similar except that DTT and NEM were substituted with water. The experiment was continued by adding to each of the tubes 500 μL of 10 % (w/v) TCA, 400 μL of 43% o-phosphoric acid, 400 μL of 4% α-α1-dipyridyl in 70% ethanol, and 200 μL of 3% FeCl3 followed by incubation at 37°C for 1 h to convert the analytes into UV-Vis absorbable molecules that were quantified at 525 nm. Oxidized ascorbate values were obtained from the difference between total ascorbate and reduced forms.
Oxygen radical absorbance capacity assay
The antioxidant capacity of basil extracts was determined through the oxygen radical absorbance capacity (ORAC) assay which is based on the ability of antioxidant compounds to quench AAPH (2,2'-Azobis-2-methyl-propanimidamide, dihydrochloride) radical and prevent the decay of a fluorescent probe. Mortar crushed leaves (250 mg) were homogenized with 2 mL of aqueous acetone (50%) and then centrifuged (4,500 g, 30 min, 4°C) to collect the extracts. The analysis was carried out as per the method described in 96-well black microplates (Jodayree et al., 2012). Wells contained 120 μL of fluorescein (0.08 mM) and then 20 μL of either phosphate buffer (blank), Trolox standard (6.25–100 μM), or basil extract were added. The microplate was incubated at 37°C for 20 min followed by the addition of 60 μl of AAPH (140 mM) to each well of the plate. Fluorescence readings were at 485/20 nm excitation and 528/20 nm emission for 50 min with 1 min reading intervals on FLx800™ model reader (Bio-Tek Instruments, Inc., Winooski, VT). The final ORAC values were calculated using a linear equation from the calibrated curve. The radical scavenging activities or ORAC values were expressed as μM Trolox equivalents (TE) per gram of fresh weight.
Statistical analysis
Four biological replicates per treatment were analyzed. Data were expressed as mean ± standard error of the mean. Multivariate analysis of variance (MANOVA) was performed on basil samples in relation to vitamin contents and antioxidant activities while one-way analysis of variance (ANOVA) was used to assess the effect of the inoculation on each vitamin concentration or on the antioxidant activity. Significant differences were evaluated using Duncan's multiple range test (p < 0.05). Principal component analysis (PCA) was performed to determine the interrelationships between the variables (loading plot) and to detect sample patterns, groupings, similarities, or differences (score plot) between the basil samples. The statistical package used was SAS (SAS OnDemand, 9.4, 2017, SAS Institute Inc., Cary, NC, USA). The PCA loading plot and the score plot were generated with R software (R version 4.1.0, The R Foundation for Statistical Computing Platform, Vienna, Austria).
Results
The data from statistical analyses showed that inoculation with the endophytes had significant effects on both vitamin contents and radical scavenging activities. According to those analyses, there are significant differences in α- and γ-tocopherols, reduced ascorbate, riboflavin, and antioxidant activity data as summarized in Table 1.
Tocopherols (vitamin E)
The concentrations are provided in Table 1. Basils inoculated with strains D, G, and F consortium of strains (A + B) maintained total tocopherol concentrations in relation to the non-inoculated control basils (P > 0.05). There were, however, significant decreases in total tocopherols from 52.2 μg/g f.w. in the control to 39.4–26.1 μg/g f.w. representing a 25–50% decrease (p < 0.05) with the lowest value being associated with strain A. Strains with lower total tocopherols also have lower α-tocopherol but a different trend was observed for γ-tocopherol. Strain H in basil, for example, had the highest content of γ-tocopherol (25.8 μg/g of fresh weight) and this was significantly higher (p < 0.05) compared to control basil (18.5 ± 1.2 μg/g).
Phylloquinone (vitamin K1)
Four of the inocula (A, B, C, and J) produced relatively high contents of phylloquinone (11.0–12.1 μg/g of f.w.) and although this represents an increase of up to 20% relative to the control (10.0 ± 0.2 μg/g of f.w.) they were not different (p > 0.05) due to large variation. These four inocula resulted in significantly higher phylloquinone concentrations (p < 0.05) than those basil plants inoculated with strain D (8.6 ± 0.6 μg/g of f.w.).
Thiamine (vitamin B1) and riboflavin (vitamin B2)
The concentrations of riboflavin in basil plants inoculated with strains A, B, D, E, F, and I did not differ from those in controls (0.126 ± 0.008 μg/g f.w.). Four of the endophytes (C, G, H, J) significantly decreased (p < 0.05) riboflavin contents from 38 to 54%. Strain G caused the largest decrease of thiamine levels (about 78%). The consortium F, (a mixture of strains A and B) had no effect on thiamine relative to controls but produced lower thiamine concentrations relative to inoculation with individual strains.
Ascorbic acid (vitamin C)
In addition, to thiamine and riboflavin, a third water-soluble vitamin was quantified. In foods and biological systems, vitamin C exists in two forms, ascorbic acid or ascorbate (the reduced and most active form) and dehydroascorbic acid or dehydroascorbate (oxidized form). The sum of the two forms constitutes the total vitamin C (or total ascorbate), they can provide an indication of a metabolic state or oxidative state in the organisms. Total ascorbate, reduced, and oxidized ascorbate data of inoculated and control aquaponic basils (Table 1) indicated that four of the inocula C, F, G, J had significantly higher (p < 0.05) concentrations (0.88–0.98 mg/g) of the reduced form of vitamin C relative to control (0.56 ± 0.05 mg/g) indicating lower oxidative stress in those basil plants. Despite the similarity in total ascorbate concentrations, the ratio of oxidized to reduced forms was up to 2-fold higher in the control basil plants in comparison to the inoculated plants. The control basil was then under higher oxidative stress.
Peroxyl radical scavenging activity
Peroxyl radicals (ROO∙) are the most common reactive species in foods and biological systems. The standard assay to determine the ability of individual compounds or mixtures like plant extracts to neutralize the oxidant effects of ROO∙ is the Oxygen Radical Absorption Capacity assay - ORAC (Huang et al., 2002). Peroxyl radical scavenging capacity is expressed as μM Trolox equivalents (TE)/g f.w. (Table 1). Basils inoculated with strain G exhibited higher ORAC than controls (94 ± 4 μM TE/g f.w. vs. 53 ± 5 μM TE/g f.w.) (p < 0.05) and any other inoculum.
Principal component analysis
Data from the eight vitamins and ORAC levels were used to visualize the measured parameters in multivariate space. The plant scores on the first two principal components (PC1 and PC2) are shown in Figure 3, they account for 33.9%, and 19.1% of the variance in the data respectively. The loading plot Figure 3A showed that PC1 was positively correlated with all forms of vitamin C although most strongly associated with reduced ascorbate. PC2 demonstrated a positive correlation with riboflavin, thiamine, and phylloquinone while displaying a negative correlation with γ-tocopherol. The PCA shows that the endophyte inocula produce a range of reproducible effects in the basil plants. Notably, the effects of H, A, B, and C are highly reproducible, offering proof that these bacteria are not impacting the basil metabolisms in a random fashion.
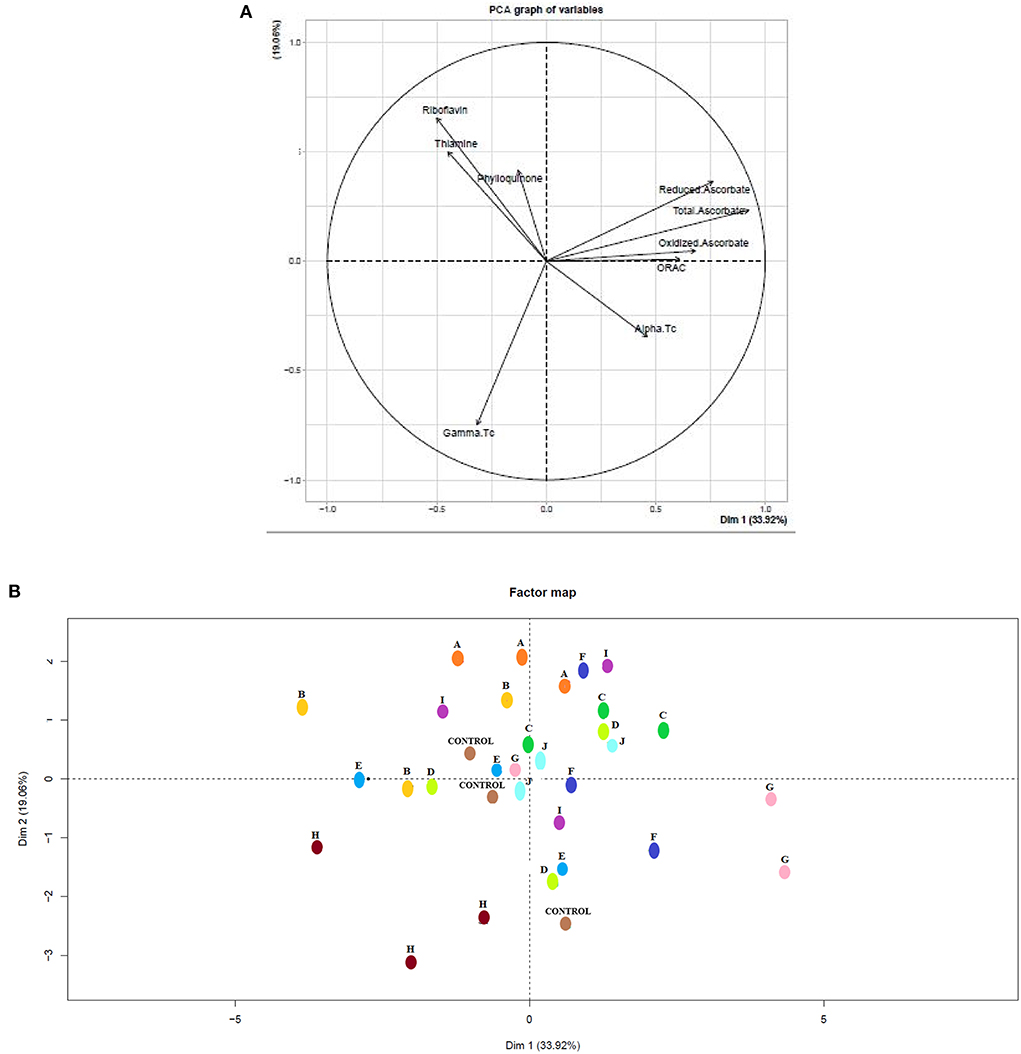
Figure 3. Principal component analysis (PCA) plots. (A) Loading plot for different variables on PC1&PC2. The loading plots represent the original variables in the space of the PCs; they reveal the magnitude and direction of correlation of the original variables with the 1st and 2nd PCs. Measured variables were alpha-tocopherol, gamma-tocopherol, phylloquinone, thiamine, riboflavin, total ascorbate, reduced ascorbate, oxidized ascorbate, ORAC values. (B) PCA scores plot for basil samples on PC1 and PC2 marked in different colors in accordance. Basil inoculated with strains of A (Plantibacter flavus), B (Plantibacter flavus), C (Curtobacterium flaccumfaciens), D (Methylobacterium oryzae), E (Sinorhizobium medicae), Consortium F (A + B), G (Rizhobium selenireductens), H (Curtobacterium herbarum), I (Bacillus toyonensis), and J (Bacillus fluminis).
Discussion
In this work, the concentrations of two fat-soluble and three water-soluble vitamins of inoculated basils were compared to those of non-inoculated (control) plants growing in a hydroponic system. Plants normally interact with soil systems that harbor tens of thousands of different species of bacteria per gram (Roesch et al., 2007) and associate with both bacteria and fungi as endophytic partners. Commercial aquaponic systems are drastically less microbiologically diverse than soils, so the plants have far less opportunity to form associations with potentially beneficial microbes. We hypothesized that inoculation with endophytic PGPB might alter their metabolic profiles. We saw antioxidant activity increased by strain G (Rhizobium selenireductuns), and reduced form of Vitamin C increased by four of our inocula (G—Rizhobium selenireductens, C—Curtobacterium flacciflamiens, F—Plantibacter flavus, and J—Bacillus fluminis). Inoculation effects on B1, B2, and E vitamins were largely negative, and there was no effect on phylloquinone.
Vitamin C is a redox molecule that can protect the plant cells from oxidative stress, and cofactor of enzymes involved in mitosis and growth of the plant (Baslam et al., 2013). The ratio of reduced ascorbate to oxidized ascorbate is the lowest in control (p < 0.05) relative to all inocula. Four of our endophyte inoculum treatments significantly increased ascorbic acid levels. This result was also seen in strawberries but with a Phyllobacterium strain (Flores-Félix et al., 2015). This is an indication that the control basil plants were under higher oxidative stress compared to those inoculated with endophytes. A possible explanation is that the endophytes may have reduced the sensitivity of basils to salt-induced stress and decreased the concentration of reactive hydrogen peroxide molecules as documented for strawberries (Keutgen and Pawelzik, 2007). It has also been shown in hydroponically grown basil, that boron caused an increase of oxidized ascorbate (Landi et al., 2013). In soil-grown basil plants under different organic fertilization treatments reduced ascorbic acid was quantified using the dichloroindophenol titration method, reporting a value of 0.24–0.36 mg/g f.w. (Naiji and Souri, 2018) which are lower than the current values obtained in this work but, the method of analysis and the growing conditions are different.
The peroxyl radical scavenging data were obtained to complement the vitamin data. In fact, increased oxidative stress in the plant can lower its antioxidant capacity (Keutgen and Pawelzik, 2007). Here we saw a significant increase in peroxyl radical quenching capacity in basil inoculated with strain G. Increased oxidants in the basil leaves such as hydrogen peroxide, singlet oxygen, and superoxide anion radicals can reduce the redox balance of ascorbic which is also equivalent to a reduced antioxidant power (Landi et al., 2013). In strawberries, for example, inoculation with Bacillus amyloliquefaciens BChi1 or Paraburkholderia BRRh-4 increased the DPPH radical scavenging activity of fruits (Rahman et al., 2018). It is believed that changes in secondary metabolites such as polyphenols are important for enhancing the antioxidant capacity of the plant such as found in regular grown basil where the variation of ROO∙ scavenging capacities were correlated to the contents of the two polyphenols isoquercitrin and rutin (Wakagi et al., 2016). ORAC data of this work (35.8–94.2 μM TE/g f.w.) are similar to the average of 48.05 μM TE/g for fresh basil reported by the USDA (United States Department of Agriculture) (Haytowitz and Bhagwat, 2010) and for fresh basils of different growing seasons (44.5–143.8 μM TE/g f.w.) (Wakagi et al., 2016). Lower values (7.6–9.3 μM TE/g) for eight varieties of soil-grown Asian basils have also been described (Mishra et al., 2020). Considering that there were an enhanced antioxidant activity and an increase in the ratio of reduced to oxidized forms of vitamins C, it can be stipulated that the inocula can help release plant stress or make them more resilient against stressful environmental factors meanwhile, further experiments are needed.
The two forms of vitamin E detected in the aquaponic grown basil samples were α- and γ-tocopherols which is consistent with a previous study, where no β-tocopherol was detected in typical hydroponic or soil-grown basils (Sgherri et al., 2010). Inoculum H significantly increased levels of γ-tocopherol but decreased levels of alpha-tocopherol, so there was no overall change in total Vitamin E levels. Interestingly, inoculation with either A or B strains resulted in significantly lower α- and total tocopherols but their combination (F) demonstrated an enhancing effect with no difference relative to control. The effect of the studied endophytes varies depending on the microorganism and the form of vitamin E which is consistent with the literature (Baslam et al., 2013). A previous work demonstrated that the age of hydroponically grown basil is a key factor in vitamin content—the authors saw 84 and 56% decreases of α- and γ-tocopherols, respectively, in 35 days hydroponically grown basils in comparison to those that were grown for 20 days (Sgherri et al., 2010).
Phylloquinone (vitamin K1) is the form of vitamin K present in plants and its major dietary sources are green leafy vegetables that can account for up to 60% of individual intakes (Booth, 2012). It was then of interest to determine its content in the basil inoculated with endophytes. Phylloquinone is mainly located in the photosynthetic tissues of plants, thus its content has been linked to its green color (Otles and Cagindi, 2007). Inocula A, B, C, and J were the ones with relatively higher concentrations of K1, and although not significant, this is still interesting as an adequate intake of vitamin K promotes blood heath (co-factor of the synthesis of the blood-clotting protein, prothrombin) and bone health through its effect on the carboxylation of osteocalcin in bone. The analysis of phylloquinone content in vegetables, spices, and seasonings purchased at Canadian food stores reported between 3.7 and 11.9 μg/g with basil having 6.12 μg/g. Another work reported a concentration of 29.2 μg/g in basil collected from an organic farm (Alibas et al., 2021). Values of phylloquinone obtained in this work (8.6–12.1 μg/g f.w.) are then lower than the value in organic basil but higher compared to store-bought basil, although the origin and growing conditions are not known.
Thiamine (B1) and riboflavin (B2) are two water-soluble vitamins that play essential roles in several body processes such as energy transformation, nervous transmission, and replication of genes (Koivu et al., 1997; San José Rodriguez et al., 2012). In plants, they act as antioxidants, and coenzymes, they also induce disease resistance against several pathogens (Boubakri et al., 2016). Like phylloquinone, green vegetables are an important source (Yoshii et al., 2019). It is interesting that all of our inocula decreased Thiamine levels in these basil plants—an observation that might be explained by bacterial competition for this vitamin. The average value in nutrient databases of thiamine in fresh basil is 0.34 ± 0.07 μg/g f.w. while that of riboflavin is 0.76 ± 0.03 μg/g f.w. (USDA, 2019). Although the origin and growth conditions are not specified in the nutrient databases, thiamine (0.15–0.69 μg/g f.w.) data from this study are similar to database values, while for riboflavin the reported concentrations (0.06–0.13 μg/g f.w.) are lower.
The variability of nutrients in endophyte inoculated crops is generally dependent on plant species, the endophyte strain, the endophyte concentration, the inoculation method, and growth conditions (Mangmang et al., 2015; Naqqash et al., 2016; Pii et al., 2018). At a concentration of 1 × 106 conidia/mL for example, Beauveria bassiana significantly decreased protein contents in lettuce leaves while 10- and 100-times higher concentrations did not affect protein contents (Macuphe et al., 2021). The data may not directly be extrapolated as Beauveria bassiana is a fungal strain and the vegetables are different.
Endophytes can promote plant growth by several mechanisms as reviewed by Santoyo et al. (2016). They can increase the level of phytohormones such as auxin, gibberellins, cytokinin, or abscisic acid (Sharma et al., 2021) which in turn stimulate the plant metabolic pathways to alter the concentration of vitamins, secondary metabolites, or enhance stress tolerance (Etesami and Maheshwari, 2018; Sharma et al., 2021). Auxin-producing bacteria for example enhanced the concentration of secondary metabolites, yields of essential oil, root branching, growth, and the number of glandular trichomes in different aromatic herbs, spices, and seed crops (Çakmakçi et al., 2020). The impact of bacterial endophytes depends on the species, the strain, the plant host, and the growth phase of the host plant (Naqqash et al., 2016; Etesami and Maheshwari, 2018; Singha et al., 2021). In this work we examined a limited number of endophytes on basil plants only at one time point. However, sampling of younger and older plants atdifferent time points might reveal different results. Most of the bacteria, with the exception of Bacillus strains I and J, were in partnerships with species other than basil. A broader study, focussing on more strains isolated from basil or close relatives might show up more effects.
As a first step, this work aimed to study the effect of the small number of known PGPB strains on the content of vitamins and antioxidant activity. Data showed that the inocula enhanced the resistance to oxidative (i.e., higher ratios of reduced to oxidised absorbates). Other mechanisms, however, remain to be investigated in a future work. Although, the tested microorganisms are known PGPB strains, proper quantification of bacteria colonies in host tissues preferably by strain specific quantitative PCR is very important because it can explain the difference in concentration of biosynthesised nutrients. This is a limitation of the current work that can be addressed in future work. Additionally, the quantification of secondary metabolites such as polyphenols, and carotenoids in the same extract is required to determine the effect of the inocula and the contribution of these secondary metabolites to the antioxidant activity.
Conclusion
The enhancement of nutritional quality in plants via endophytic organisms is a valuable tool to produce functional foods. This work shows that endophytic bacteria have the potential to alter vitamin profiles. In particular, the antioxidant status of basil could be affected. Further studies are required to elucidate the mechanism involved in these studies paying particular attention to the growth stage of the plant, the concentration, as well as the genetic makeup of the inoculated strains.
Data availability statement
The original contributions presented in the study are included in the article/supplementary material, further inquiries can be directed to the corresponding authors.
Author contributions
GC and PD: methodology, data curation, formal analysis, and writing. RF and AT: conceptualization, investigation, supervision, data curation, and writing. All authors contributed to the article and approved the submitted version.
Funding
This work was carried out with financial supports from Weston Family Foundation Seeding Food Innovation program (Grant 2018-2416 SFI18-0244); MITACS Accelerate program (Grant IT15020).
Conflict of interest
The authors declare that the research was conducted in the absence of any commercial or financial relationships that could be construed as a potential conflict of interest.
Publisher's note
All claims expressed in this article are solely those of the authors and do not necessarily represent those of their affiliated organizations, or those of the publisher, the editors and the reviewers. Any product that may be evaluated in this article, or claim that may be made by its manufacturer, is not guaranteed or endorsed by the publisher.
References
Alibas, I., Yilmaz, A., Asik, B. B., and Erdogan, H. (2021). Influence of drying methods on the nutrients, protein content and vitamin profile of basil leaves. J. Food Compos. Anal. 96, 103758. doi: 10.1016/j.jfca.2020.103758
Baslam, M., Garmendia, I., and Goicoechea, N. (2013). Enhanced accumulation of vitamins, nutraceuticals and minerals in lettuces associated with arbuscular mycorrhizal fungi (Amf): a question of interest for both vegetables and humans. Agriculture 3, 188–209. doi: 10.3390/agriculture3010188
Booth, S. L. (2012). Vitamin K: Food composition and dietary intakes. Food Nutr. Res. 56. doi: 10.3402/fnr.v56i0.5505
Boubakri, H., Gargouri, M., Mliki, A., Brini, F., Chong, J., and Jbara, M. (2016). Vitamins for enhancing plant resistance. Planta 244, 529–543. doi: 10.1007/s00425-016-2552-0
Çakmakçi, R., Mosber, G., Milton, A. H., Alatürk, F., and Ali, B. (2020). The effect of auxin and auxin-producing bacteria on the growth, essential oil yield, and composition in medicinal and aromatic plants. Curr. Microbiol. 77, 564–577. doi: 10.1007/s00284-020-01917-4
Chandrasekaran, M., Paramasivan, M., and Chun, S. C. (2019). Bacillus subtilis CBR05 induces Vitamin B6 biosynthesis in tomato through the de novo pathway in contributing disease resistance against Xanthomonas campestris pv. vesicatoria. Sci. Rep. 9, 1–10. doi: 10.1038/s41598-019-41888-6
Esteve, M. J., Fairé, R., Frígola, A., and García-Cantabella, J. M. (2001). Simultaneous determination of thiamin and riboflavin in mushrooms by liquid chromatography. J. Agric. Food Chem. 49, 1450–1454. doi: 10.1021/jf001040p
Etesami, H., and Maheshwari, D. K. (2018). Use of plant growth promoting rhizobacteria (PGPRs) with multiple plant growth promoting traits in stress agriculture: action mechanisms and future prospects. Ecotoxicol. Environ. Saf. 156, 225–246. doi: 10.1016/j.ecoenv.2018.03.013
Falowo, A. B., Mukumbo, F. E., Idamokoro, E. M., Afolayan, A. J., and Muchenje, V. (2019). Phytochemical constituents and antioxidant activity of sweet basil (Ocimum basilicum L.) essential oil on ground beef from boran and nguni cattle. Int. J. Food Sci 2019. doi: 10.1155/2019/2628747
Flores-Félix, J. D., Silva, L. R., Rivera, L. P., Marcos-García, M., García-Fraile, P., Martínez-Molina, E., et al. (2015). Plants probiotics as a tool to produce highly functional fruits: the case of phyllobacterium and vitamin C in strawberries. PLoS ONE. 10:e0122281. doi: 10.1371/journal.pone.0122281
Fujita, M., Kusajima, M., Okumura, Y., Nakajima, M., Minamisawa, K., and Nakashita, H. (2017). Effects of colonization of a bacterial endophyte, Azospirillum sp. B510, on disease resistance in tomato. Biosci. Biotechnol. Biochem. 81, 1657–1662. doi: 10.1080/09168451.2017.1329621
Ghasemzadeh, A., Ashkani, S., Baghdadi, A., Pazoki, A., Jaafar, H. Z. E., and Rahmat, A. (2016). Improvement in flavonoids and phenolic acids production and pharmaceutical quality of sweet basil (Ocimum basilicum L.) by ultraviolet-B irradiation. Molecules 21, 1203. doi: 10.3390/molecules21091203
Gillespie, K. M., and Ainsworth, E. A. (2007). Measurement of reduced, oxidized and total ascorbate content in plants. Nat. Protoc. 2, 871–874. doi: 10.1038/nprot.2007.101
Haytowitz, D. B., and Bhagwat, S. (2010). ORAC of Selected Foods, Release 2. Nutr. Data Lab. Beltsv. Hum. Nutr. Res. Cent. Agric. Res. Serv. U.S. Dep. Agric, 1–46. Available online at: http://www.ars.usda.gov/Services/docs.htm?docid=15866 (accessed December 8, 2021).
Hodges, D. M., Forney, C. F., and Wismer, W. V (2001). Antioxidant responses in harvested leaves of two cultivars of spinach differing in senescence rates. J. Am. Soc. Hortic. Sci. 126, 611–617. doi: 10.21273/JASHS.126.5.611
Huang, D., Ou, B., Hampsch-Woodill, M., Flanagan, J. A., and Prior, R. L. (2002). High-throughput assay of oxygen radical absorbance capacity (ORAC) using a multichannel liquid handling system coupled with a microplate fluorescence reader in 96-well format. J. Agric. Food Chem. 50, 4437–4444. doi: 10.1021/jf0201529
Ivanović, L., Milašević, I., Topalović, A., Ð*urović, D., Mugoša, B., KneŽević, M., et al. (2019). Nutritional and phytochemical content of Swiss chard from Montenegro, under different fertilization and irrigation treatments. Br. Food J. 121, 411–425. doi: 10.1108/BFJ-03-2018-0142
Jodayree, S., Smith, J. C., and Tsopmo, A. (2012). Use of carbohydrase to enhance protein extraction efficiency and antioxidative properties of oat bran protein hydrolysates. Food Res. Int. 46, 69–75. doi: 10.1016/j.foodres.2011.12.004
Kaurinovic, B., Popovic, M., Vlaisavljevic, S., and Trivic, S. (2011). Antioxidant capacity of ocimum basilicum L. and Origanum vulgare L. extracts. Molecules 16, 7401–7414. doi: 10.3390/molecules16097401
Keutgen, A. J., and Pawelzik, E. (2007). Modifications of strawberry fruit antioxidant pools and fruit quality under NaCl stress. J. Agric. Food Chem. 55, 4066–4072. doi: 10.1021/jf070010k
Knecht, K., Sandfuchs, K., Kulling, S. E., and Bunzel, D. (2015). Tocopherol and tocotrienol analysis in raw and cooked vegetables: a validated method with emphasis on sample preparation. Food Chem. 169, 20–27. doi: 10.1016/j.foodchem.2014.07.099
Koivu, T. J., Piironen, V. I., Henttonen, S. K., and Mattila, P. H. (1997). Determination of phylloquinone in vegetables, fruits, and berries by high-performance liquid chromatography with electrochemical detection. J. Agric. Food Chem. 45, 4644–4649. doi: 10.1021/jf970357v
Landi, M., Pardossi, A., Remorini, D., and Guidi, L. (2013). Antioxidant and photosynthetic response of a purple-leaved and a green-leaved cultivar of sweet basil (Ocimum basilicum) to boron excess. Environ. Exp. Bot. 85, 64–75. doi: 10.1016/j.envexpbot.2012.08.008
Lin, K. H., Huang, M. Y., and Hsu, M. H. (2021). Morphological and physiological response in green and purple basil plants (Ocimum basilicum) under different proportions of red, green, and blue LED lightings. Sci. Hortic. 275, 109677. doi: 10.1016/j.scienta.2020.109677
Lumactud, R., Shen, S. Y., Lau, M., and Fulthorpe, R. (2016). Bacterial endophytes isolated from plants in natural oil seep soils with chronic hydrocarbon contamination. Front. Microbiol. 7, 755. doi: 10.3389/fmicb.2016.00755
Macuphe, N., Oguntibeju, O. O., and Nchu, F. (2021). Evaluating the endophytic activities of beauveria bassiana on the physiology, growth, and antioxidant activities of extracts of lettuce (Lactuca sativa l.). Plants 10, 1178. doi: 10.3390/plants10061178
Makri, O., and Kintzios, S. (2008). Ocimum sp. (Basil): Botany, cultivation, pharmaceutical properties, and biotechnology. J. Herbs Spices Med. Plants 13, 123–150. doi: 10.1300/J044v13n03_10
Mangmang, J. S., Deaker, R., and Rogers, G. (2015). Early seedling growth response of lettuce, tomato and cucumber to Azospirillum brasilense inoculated by soaking and drenching. Hortic. Sci. 42, 37–46. doi: 10.17221/159/2014-HORTSCI
Mayer, E., de Quadros, P. D., and Fulthorpe, R. (2019). Plantibacter flavus, Curtobacterium herbarum, Paenibacillus taichungensis, and Rhizobium selenitireducens endophytes provide host-specific growth promotion of Arabidopsis thaliana, basil, lettuce, and bok choy plants. Appl. Environ. Microbiol. 85, e00383–19. doi: 10.1128/AEM.00383-19
Mishra, L. K., Sarkar, D., Mentreddy, R., and Shetty, K. (2020). Evaluation of phenolic bioactive-linked anti-hyperglycemic and Helicobacter pylori inhibitory activities of Asian Basil (Ocimum spp.) varieties. J. Herb. Med. 20, 100310. doi: 10.1016/j.hermed.2019.100310
Naiji, M., and Souri, M. K. (2018). Nutritional value and mineral concentrations of sweet basil under organic compared to chemical fertilization. Acta Sci. Pol. Hortorum Cultus 17, 167–175. doi: 10.24326/asphc.2018.2.14
Naqqash, T., Hameed, S., Imran, A., Hanif, M. K., Majeed, A., and van Elsas, J. D. (2016). Differential response of potato toward inoculation with taxonomically diverse plant growth promoting rhizobacteria. Front. Plant Sci. 7, 144. doi: 10.3389/fpls.2016.00144
Neocleous, D., and Nikolaou, G. (2019). Antioxidant seasonal changes in soilless greenhouse sweet peppers. Agronomy 9, 1–15. doi: 10.3390/agronomy9110730
Otles, S., and Cagindi, O. (2007). Determination of vitamin K1 content in olive oil, chard and human plasma by RP-HPLC method with UV-Vis detection. Food Chem. 100, 1220–1222. doi: 10.1016/j.foodchem.2005.12.003
Padash, A., Shahabivand, S., Behtash, F., and Aghaee, A. (2016). A practicable method for zinc enrichment in lettuce leaves by the endophyte fungus Piriformospora indica under increasing zinc supply. Sci. Hortic. 213, 367–372. doi: 10.1016/j.scienta.2016.10.040
Paradiković, N., Vinković, T., Vinković Vrček, I., Žuntar, I., Bojić, M., and Medić-Šarić, M. (2011). Effect of natural biostimulants on yield and nutritional quality: an example of sweet yellow pepper (Capsicum annuum L.) plants. J. Sci. Food Agric. 91, 2146–2152. doi: 10.1002/jsfa.4431
Paradiso, R., Arena, C., De Micco, V., Giordano, M., Aronne, G., and De Pascale, S. (2017). Changes in leaf anatomical traits enhanced photosynthetic activity of soybean grown in hydroponics with plant growth-promoting microorganisms. Front. Plant Sci. 8, 674. doi: 10.3389/fpls.2017.00674
Pii, Y., Graf, H., Valentinuzzi, F., Cesco, S., and Mimmo, T. (2018). The effects of plant growth-promoting rhizobacteria (PGPR) on the growth and quality of strawberries. Acta Hortic. 1217, 231–238. doi: 10.17660/ActaHortic.2018.1217.29
Rahman, M., Rahman, M., Sabir, A. A., Mukta, J. A., Khan, M. M. A., Mohi-Ud-Din, M., et al. (2018). Plant probiotic bacteria Bacillus and Paraburkholderia improve growth, yield and content of antioxidants in strawberry fruit. Sci. Rep. 8, 2504. doi: 10.1038/s41598-018-20235-1
Roesch, L. F. W., Fulthorpe, R. R., Riva, A., Casella, G., Hadwin, A. K. M., Kent, A. D., et al. (2007). Pyrosequencing enumerates and contrasts soil microbial diversity. ISME J. 1, 283–290. doi: 10.1038/ismej.2007.53
Saha, S., Monroe, A., and Day, M. R. (2016). Growth, yield, plant quality and nutrition of basil (Ocimum basilicum L.) under soilless agricultural systems. Ann. Agric. Sci. 61, 181–186. doi: 10.1016/j.aoas.2016.10.001
San José Rodriguez, R., Fernández-Ruiz, V., Cámara, M., and Sánchez-Mata, M. C. (2012). Simultaneous determination of vitamin B 1 and B 2 in complex cereal foods, by reverse phase isocratic HPLC-UV. J. Cereal Sci. 55, 293–299. doi: 10.1016/j.jcs.2011.12.011
Santoyo, G., Moreno-Hagelsieb, G., del Carmen Orozco-Mosqueda, M., and Glick, B. R. (2016). Plant growth-promoting bacterial endophytes. Microbiol. Res. 183, 92–99. doi: 10.1016/j.micres.2015.11.008
Sgherri, C., Cecconami, S., Pinzino, C., Navari-Izzo, F., and Izzo, R. (2010). Levels of antioxidants and nutraceuticals in basil grown in hydroponics and soil. Food Chem. 123, 416–422. doi: 10.1016/j.foodchem.2010.04.058
Sharma, V., Singh, A., Sharma, D., Sharma, A., Phogat, S., Chakraborty, N., et al. (2021). Stress mitigation strategies of plant growth-promoting rhizobacteria: plant growth-promoting rhizobacteria mechanisms. Plant Sci. Today 8, 25–32. doi: 10.14719/pst.1543
Singha, K. M., Singh, B., and Pandey, P. (2021). Host specific endophytic microbiome diversity and associated functions in three varieties of scented black rice are dependent on growth stage. Sci. Rep. 11, 12259. doi: 10.1038/s41598-021-91452-4
Skrypnik, L., Novikova, A., and Tokupova, E. (2019). Improvement of phenolic compounds, essential oil content and antioxidant properties of sweet basil (Ocimum basilicum L.) depending on type and concentration of selenium application. Plants 8, 1–13. doi: 10.3390/plants8110458
Terry, E., De Los, A., Pino, M., and Medina, N. (2000). Application times of an Azospirillum bioproduct in tomato growth, development and yield. Cultiv. Trop. 21, 5–8. https://www.redalyc.org/articulo.oa?id=193230160001
USDA (2019). Composition of Foods Raw, Processed, Prepared USDA National Nutrient Database for Standard Reference, Release 28. Available online at: https://data.nal.usda.gov/dataset/composition-foods-raw-processed-prepared-usda-national-nutrient-database-standard-reference-release-28-0 (accessed March 3, 2022).
Vardavas, C. I., Majchrzak, D., Wagner, K. H., Elmadfa, I., and Kafatos, A. (2006). The antioxidant and phylloquinone content of wildly grown greens in Crete. Food Chem. 99, 813–821. doi: 10.1016/j.foodchem.2005.08.057
Wakagi, M., Taguchi, Y., Watanabe, J., Ogita, T., Goto, M., Arai, R., et al. (2016). Determination of the antioxidative activities of herbs harvested in Japan by oxygen radical absorbance capacity methods. Food Sci. Technol. Res. 22, 301–305. doi: 10.3136/fstr.22.301
Yang, T., and Kim, H. J. (2020). Characterizing nutrient composition and concentration in tomato-, basil-, and lettuce-based aquaponic and hydroponic systems. Water 12, 1259. doi: 10.3390/w12051259
Keywords: sweet basil, vitamins, antioxidant, hydroponics, endophytes
Citation: Campos Espinosa GY, Dörr de Quadros P, Fulthorpe RR and Tsopmo A (2022) Vitamin contents and antioxidant capacity of hydroponic grown sweet basil inoculated with endophytic bacteria. Front. Sustain. Food Syst. 6:954956. doi: 10.3389/fsufs.2022.954956
Received: 27 May 2022; Accepted: 03 August 2022;
Published: 24 August 2022.
Edited by:
Everlon Cid Rigobelo, São Paulo State University, BrazilReviewed by:
Gholamreza Khaksar, Chulalongkorn University, ThailandPooja Suneja, Maharshi Dayanand University, India
Copyright © 2022 Campos Espinosa, Dörr de Quadros, Fulthorpe and Tsopmo. This is an open-access article distributed under the terms of the Creative Commons Attribution License (CC BY). The use, distribution or reproduction in other forums is permitted, provided the original author(s) and the copyright owner(s) are credited and that the original publication in this journal is cited, in accordance with accepted academic practice. No use, distribution or reproduction is permitted which does not comply with these terms.
*Correspondence: Roberta R. Fulthorpe, cm9iZXJ0YS5mdWx0aG9ycGVAdXRvcm9udG8uY2E=; Apollinaire Tsopmo, YXBvbGxpbmFpcmVfdHNvcG1vQGNhcmxldG9uLmNh